- 1College of Agronomy, Qingdao Agricultural University, Qingdao, Shandong, China
- 2Dryland Farming Institute, Hebei Academy of Agriculture and Forestry Sciences, Hengshui, China
- 3Department of Biological Sciences, Royal Holloway University of London, Egham, United Kingdom
Spodoptera frugiperda (Lepidoptera: Noctuidae), a pest with an amazing appetite, damages many crops and causes great losses, especially maize. Understanding the differences in different maize cultivars’ responses to S. frugiperda infestation is very important for revealing the mechanisms involved in the resistance of maize plants to S. frugiperda. In this study, a comparative analysis of two maize cultivars, the common cultivar ‘ZD958’ and the sweet cultivar ‘JG218’, was used to investigate their physico-biochemical responses to S. frugiperda infestation by a pot experiment. The results showed that the enzymatic and non-enzymatic defense responses of maize seedlings were rapidly induced by S. frugiperda. Frist, the hydrogen peroxide (H2O2) and malondialdehyde (MDA) contents of infested maize leaves were significantly increased and then decreased to the level of the control. Furthermore, compared with the control leaves, the puncture force values and the total phenolics, total flavonoids, and 2,4-dihydroxy-7-methoxy-1,4-benzoxazin-3-one contents of infested leaves were significantly increased within a certain time. The superoxide dismutase and peroxidase activities of infested leaves were significantly increased in a certain period of time, while the catalase activities decreased significantly and then increased to the control level. The jasmonic acid (JA) levels of infested leaves were significantly improved, whereas the salicylic acid and abscisic acid levels changed less. Signaling genes associated with phytohormones and defensive substances including PAL4, CHS6, BX12, LOX1, and NCED9 were significantly induced at certain time points, especially LOX1. Most of these parameters changed greater in JG218 than in ZD958. Moreover, the larvae bioassay showed that S. frugiperda larvae weighed more on JG218 leaves than those on ZD958 leaves. These results suggested that JG218 was more susceptible to S. frugiperda than ZD958. Our findings will make it easier to develop strategies for controlling S. frugiperda for sustainable maize production and breeding of new maize cultivars with increased resistance to herbivores.
1 Introduction
An herbivore attack causes serious destruction to plant growth and considerable loss in crop yield. Over the course of coevolution and interactions between plants and herbivores, host plants have developed a variety of defenses against herbivores (War et al., 2012). Host plant resistance is considered to be one of the important ways for reducing the losses, which can be constitutive or inducible (Fürstenberg-Hägg et al., 2013). In the absence of herbivores, constitutive defenses for herbivores are the fundamental expression of physical and chemical defensive traits, whereas induced defenses are only activated after an attack by herbivores (Anstett et al., 2016). Plant-induced defenses are very effective in defensing against following herbivore attacks (Mertens et al., 2021), which can be direct or indirect. Direct induced resistance is mediated by the accumulation of defense-related proteins to defend plants against herbivores, or plants may produce noxious chemicals to prevent herbivores from feeding, ovipositing, or growing (War et al., 2012); whereas indirect induced resistance causes the release of a mixture of volatile compounds that specifically attract the plant’s natural enemies (predators or parasitoids) (Aljbory and Chen, 2018).
Secondary metabolites, such as compounds containing nitrogen and sulfur, terpenoids, and phenolics, plant defensive proteins, which are connected in the activation and enhancement of defense systems in plants, are inducible defenses against herbivores (War et al., 2012; Divekar et al., 2022). In order to shield plants from herbivores, they are synthesized to perform defense functions and control defense signaling pathways. Many of these signal transduction pathways are mediated by a network of phytohormones, including jasmonic acid (JA), salicylic acid (SA), and abscisic acid (ABA) (Guo et al., 2019). The regulation of plant-induced resistance to herbivores, especially, is significantly influenced by the accumulation of JA and SA (Pieterse et al., 2012; Yang et al., 2015; Guo et al., 2019). These phytohormones may act individually, synergistically, or antagonistically to activate signal transduction upon herbivore feeding. Many genes are involved in these processes and metabolic pathways, such as phenylalanine ammonia-lyase (PAL) genes (Cass et al., 2015), lipoxygenase (LOX) genes (Njaci et al., 2020), 9-cis-epoxycarotenoid dioxygenase (NCED) genes (Han et al., 2004), benzoxazinoid (BX) genes (Tzin et al., 2017), and chalcone synthase (CHS) genes (Dehghan et al., 2014). Knowing how the plant’s defensive traits are controlled and expressed by genes will be helpful in developing new breeds with enhanced defense properties against herbivores.
Spodoptera frugiperda (S. frugiperda), a species of agricultural pest known as the fall armyworm (Lepidoptera: Noctuidae), is widespread and disruptive. With their chewing mouthparts, S. frugiperda larvae consume crop seedlings, leaves, and kernels, thus reducing crop yields (Zhang et al., 2022). A wide range of crops are targeted by this pest, but several crops, including maize, rice, sorghum, turf grasses, cotton, and peanuts, are preferred (Carroll et al., 2006). One of the world’s most important agricultural crops, maize is frequently used as food, feed, and industrial raw material. Therefore, maize plays a crucial role in ensuring economic and food security. S. frugiperda has rapidly spread since it entered China in January 2019, infecting grain-producing regions in southern and southwestern China and posing a significant threat to the maize crop (Jing et al., 2020; Gao et al., 2021). It is urgent to make safe and effective control strategies for S. frugiperda.
One important method for controlling pests in agriculture is plant-induced resistance. In the past couple of decades, plant-induced resistance to various stresses has made significant progress and has emerged as an important topic in plant–herbivore interactions (War et al., 2011; War et al., 2012). Therefore, to study the maize defense response to S. frugiperda is very valuable for the utilization of host plant resistance to control S. frugiperda. To date, research on the response of host plants to S. frugiperda were focused on plant volatile compound identification, genetic resistance, host plant preference, etc. (Costa et al., 2020; De Lange et al., 2020; Garlet et al., 2021; Hafeez et al., 2021; Haq et al., 2021; Jacques et al., 2021; Lv et al., 2021; Murad et al., 2021; Yactayo-Chang et al., 2021; Peterson et al., 2022). However, the performance and the mechanism of maize plants in response to S. frugiperda still need to be studied further. Understanding the differences in different maize cultivars’ responses to S. frugiperda is vital to reveal the mechanisms involved in the resistance of maize plants to S. frugiperda. Therefore, the present study was performed using two different kinds of maize cultivars with different genetic backgrounds to assess the resistance differences to S. frugiperda feeding. The study includes larval weight gain, change of puncture force, accumulation of total phenolic compounds, total flavonoids, and 2,4-dihydroxy-7-methoxy-1,4-benzoxazin-3-one (DIMBOA), contents of hydrogen peroxide (H2O2) and malondialdehyde (MDA), activities of antioxidant enzymes superoxide dismutase (SOD), peroxidase (POD), and catalase (CAT), and levels of phytohormones jasmonic acid (JA), salicylic acid (SA), and abscisic acid (ABA) in the S. frugiperda-infested leaves and control (CK). The level of defense substances and phytohormone biosynthesis genes in response to S. frugiperda infestation was also assessed using gene expression analysis. It can provide a theoretical basis for developing crop cultivars and pest management for sustainable crop production.
2 Materials and methods
2.1 Plant materials
Two different kinds of maize cultivars with different genetic backgrounds were used. ‘Zhengdan958’ (ZD958) is one of the most common cultivars, which is the hybrid of Zheng58 × Chang7-2 and one of the most widely planted hybrid in China. ‘Jinguan218’ (JG218) is another sweet corn, which is the hybrid of Sweet62 × Sweet 601 and one of the most widely planted hybrid in China. The uncoated seeds of ZD958 and JG218, produced in the year 2021, were bought from Beijing Denong Seed Industry Co., Ltd. and Beijing Sihai Seed Industry Co., Ltd., respectively. The seeds were stored in a cool chamber at 10°C and relative humidity of 40%. All experiments were conducted using healthy 15-day-old ZD958 (~30 cm in height) and JG218 (~25 cm in height) cultivar seedlings. The maize seeds were sown in plastic pots (7 cm in height and 6 cm in diameter) with commercial nutrient soil (Pindstrup, Denmark) and placed in a climate-controlled room (temperature of 25 ± 1°C, relative humidity of 60 ± 5%) under 16 -h light/8-h dark photoperiods and supplied with the same amount of water for each pot every 2 or 3 days. Three plants of each maize cultivar per pot were retained after the plant emergence. When S. frugiperda was introduced, the maize plants were transferred into ventilated cages (70 × 70 × 50 cm). The cages were maintained in a climate-controlled condition as described above.
2.2 Experimental treatments
S. frugiperda larvae were reared from eggs provided by Henan Jiyuan Baiyun Industry Co., Ltd., Henan Province, China. The eggs were kept in an incubator (temperature of 27 ± 1°C, relative humidity of 30–40%) until the emergence of the neonate larvae. After that, they were moved to rear at room temperature (25 ± 1°C). Before feeding on maize plants, the third-instar larvae were starved for 1 h. Then, three larvae fed on the leaves of each plant at 2:00 PM. After 1 h, the larvae were removed to limit both the extent and the time frame of feeding damage (Carroll et al., 2006). The maize leaves were harvested at 0, 3, 6, and 12 h after the larvae were removed and then immediately used or stored at -80°C according to the object of the study. All biochemical parameters were determined on both the infected and uninfected (control) leaves of each cultivar (Figure 1). At each time point, three biological replicates of the experiment were set up, and plants of three pots were used for each replicate.
2.3 Larvae bioassay
After having been starved for 2 h, the third-instar larvae randomly selected were placed in 90-mm-diameter petri dishes with enough freshly detached leaves of ZD958 and JG218 for 24 h. A single third-instar larva was placed in each petri dish (Njaci et al., 2020). A total of 10 petri dishes were used as a biological replicate, with a total of 30 petri dishes for three biological replicates. All petri dishes were placed at room temperature (temperature of 25 ± 1°C, relative humidity of 60 ± 5%) with 16-h light/8-h dark photoperiod. The body weight of the larvae was determined using an automatic electro-balance every 3 h.
2.4 Measurement of puncture force (F) of maize leaves
A Handpi screen view electric test stand (Yueqing Handpi Instruments Co., Ltd., China) with a cylindrical probe of 0.5 mm in diameter and 5 mm in length was used to puncture the leaf samples. The measurement range of the instrument is set to 0–10 N. The leaf samples were set tightly on the stage with clips, and the probe moved down toward the stage at a speed of 30 mm/min. A creep meter that was outfitted with force analysis software for automatic computer analysis was used to measure the puncture force values (N). Higher values indicated that more force was required to puncture the tissues, while lower values indicated that crispness was higher. Three biological replicates were set up; for each replicate, measurements were taken separately from three pieces (~4 cm in length of the damaged leaf or the counterpart of the control leaf) of each of the middle region of the second and third leaf, respectively, and the average of 10 values was used to calculate the puncture force value of each segment. The sample’s puncture force value was calculated by taking the averages of the three pieces in each replicate. The above-mentioned procedure was carried out at room temperature (Gutiérrez-Rodríguez et al., 2013).
2.5 Measurement of H2O2 concentration of maize leaves
Based on a previous method (Quandahor et al., 2021), 0.15 g of leaf samples in an ice bath was homogenized with 5 ml of 0.1% (w/v) trichloroacetic acid (TCA). Then, after centrifugation at 10,000 × g for 10 min, the supernatant (0.5 ml) was mixed with 0.5 ml of 10 mM potassium phosphate (K3PO4) buffer (pH = 6.8) and 1 ml of 1 M potassium iodide. After 5 min, the supernatant’s absorption was read at a wavelength of 390 nm using a spectrophotometer (UV- Cary60, Agilent, USA). This step was repeated three times per biological replicate for a total of three biological replicates.
2.6 Determination of the MDA content of maize leaves
The MDA content was measured following a previously described method with slight modifications (Ahmad et al., 2021). Fresh leaf sample (0.5 g) was homogenized in 5.0 ml of 10% TCA solution and centrifuged at 4,000 × g for 10 min, and the supernatant was moved to new tubes. Then, 2 ml of the supernatant was mixed with 2 ml of 0.6% TCA solution. The mixture was thoroughly vortexed, heated for 15 min in boiling water, cooled immediately, and centrifuged for 5 min at 10,000 × g. Using a spectrophotometer (UV- Cary60, Agilent, USA), the absorbance values were measured at 532-, 450-, and 600-nm wavelengths. Three technical repeats were performed per biological replicate for a total of three biological replicates.
2.7 Extraction and determination of the total phenolic and flavonoid contents of maize leaves
The fresh leaf samples were ground to homogenize in liquid nitrogen. Then, 1.0 g each of the powder samples were extracted by ultrasound for 60 min at 40°C using 10 ml of 80% methanol, and the supernatant of the extracted samples were obtained after centrifugation at 7,000 × g for 20 min. Folin–Ciocalteu reagent was used to detect the total phenolic compound content. The samples (0.5 ml) were mixed with 2.5 ml of a 10-fold diluted Folin–Ciocalteu reagent and 2 ml of 7.5% sodium carbonate in tubes. Before being measured with a spectrophotometer (UV- Cary60, Agilent, USA) at 760 nm, the tubes were covered and left to stand for 30 min at room temperature (Złotek et al., 2014). Gallic acid (0 to 15 μg/ml) was used as standard solution to determine the total phenolic content. The total flavonoid contents of the leaf extracts were determined using aluminum chloride reagent. In brief, 1.5 ml of 80% methanol, 0.1 ml of 10% aluminum chloride hexahydrate, 0.1 ml of 1 M sodium acetate, and 2.8 ml of deionized water were combined with 0.5 ml of the extract solution. After 40 min, at a wavelength of 415 nm, the mixture absorption of the control was read (Złotek et al., 2014). Using the same method as described above, the standard curve for total flavonoids was established using the rutin standard solution ranging from 0 to 50 µg/ml. Three technical repeats were performed per biological replicate for a total of three biological replicates.
2.8 Determination of the benzoxazine content of maize leaves
A previously described method was used to measure the content of benzoxazine in maize leaves, with some slight modifications (Bao et al., 2021). In short, 2 ml of 70% acetonitrile and 1% acetic acid in water were used to oscillate 0.5 g of fresh maize leaves for 2 h at 60°C. After being centrifuged at 4,000 × g for 10 min at room temperature, the mixture solutions were filtered through 0.45-μm filters. Then, at 40°C, 10 μl of the filtrate was analyzed by liquid chromatography (Agilent 1100, USA) equipped with UV spectra (DAD detector) and a Xtimate C18 column (250 mm × 4.6 mm i.d., 5 μm; Yuexu, China). Furthermore, 280 nm was selected as the wavelength, and 0.01% phosphoric acid in water (phase A) and acetonitrile (phase B) made up the mobile phase. The following was set up for the gradient elution program: 0–0.01 min at 5% B, 0.01–32 min at 5–30% B, 32–36 min at 30–80% B, 36–37 min at 80–5% B, and 37–46 min at 5% B. Additionally, the DIMBOA content (in μg/g fresh weight) was calibrated using standards of 20 μg/ml DIMBOA (Shanghai Yuanye Bio-Technology Co., Ltd., China). One technical repeat was performed per biological replicate for a total of three biological replicates.
2.9 Determination of the antioxidant enzyme activity of maize leaves
Under liquid nitrogen, 0.5 g of fresh leaf sample was homogenized with a mortar and pestle in each treatment using 5 ml of 100 mM precooled phosphate buffer (pH 7.4) containing 1 mM ethylenediamine tetraacetic acid and 5% polyvinylpyrrolidone. At 4°C, the crude homogenate was centrifuged for 10 min at 5,000 × g. The supernatant was used to detect the SOD, POD, and CAT activities. The absorbance was read using a spectrophotometer (UV- Cary60, Agilent, USA). The ability of SOD to prevent the photochemical reduction of nitroblue tetrazolium (NBT) at 560 nm was used to determine its activity. A reduction of NBT of approximately 50% was considered to be one unit of enzyme activity (He et al., 2014). The optical density change per minute at 470 nm was used to calculate the POD activity (Zhang et al., 2020). The H2O2 decomposition was used to measure the CAT activity as a decline in the absorbance at 240 nm (He et al., 2014). The absorption values were repeated three times per biological replicate for a total of three biological replicates.
2.10 Plant hormone quantification of maize leaves
The plant hormones were extracted from 0.1 g of leaves using 0.9 ml of phosphate-buffered saline (pH 7.4). The crude homogenate was subjected to centrifugation at 3,000 × g for 15 min at 4°C. The supernatant was used to measure the contents of JA, SA, and ABA using commercially available assay kits (from Hefei Laier Biotechnology Co., Ltd., Anhui Province, China) according to the user manual provided by the manufacturer. The absorbance was read using a multifunctional ultraviolet fluorescent enzyme-labeled instrument (TECAN Infinite 200, Switzerland). Three technical repeats were performed per biological replicate for a total of three biological replicates.
2.11 Plant hormones and secondary metabolite-related gene expression analyses of maize leaves
Real-time quantitative PCR (qRT-PCR) was used to examine gene expression. Huayueyang Quick RNA Isolation Kit (Huayueyang Biotechnology, Beijing, China) was used to extract the total RNA of each sample in accordance with the protocol provided by the manufacturer. Reverse transcription was carried out using HiScript® Q RT SuperMix for qPCR (+ gDNA wiper) (Vazyme, Nanjing, China). PCR was performed using ChamQ SYBR qPCR Master Mix (Vazyme) as described previously (Li et al., 2018). The gene-specific primers are listed in Supplementary File S1. ZmActin (Zm00001d010159) was used as an internal control. StepOnePlusTM Real-Time PCR system (Applied Biosystems, Ghent, Belgium) was used for qRT-PCRs, with three technical replicates for each biological replicate in a total of three biological replicates. The gene expression level in the infestation leaves at 0 h was set to 1, and the levels in other infested and control leaves were given relative to this for each cultivar. Using the 2−ΔΔCt method (Livak and Schmittgen, 2001), the relative expression levels of the genes were determined.
2.12 Data analyses
All experimental data were presented as mean ± standard deviations (SD) from repeat assays. The data was analyzed statistically with one-way ANOVA using SPSS 11.0. The differences of these parameters between the S. frugiperda-infested and the control leaves were compared using the Tukey test. P <0.05 was considered significant for differences.
3 Results
3.1 Weight gain of S. frugiperda larvae
To investigate the response of different maize cultivars to S. frugiperda infestation, a larvae bioassay on the detached leaves of ZD958 and JG218 was carried out. In order to make the larvae much hungrier and discharge more feces or urine, they were starved and used after 2 h. The results showed that the differences between the detached leaves of ZD958 and JG218 on larval weight gain were significant from 6 h onwards after S. frugiperda was fed (F = 27704.287, df =17, P = 0.000). A higher larval weight gain ratio was observed on the JG218 leaves (0–83.28%) compared with those from the ZD958 leaves (0–70.64%) (Figure 2). It suggests that JG218 was more susceptible to S. frugiperda than ZD958. The different genetic backgrounds of ZD958 and JG218 may be the reason.
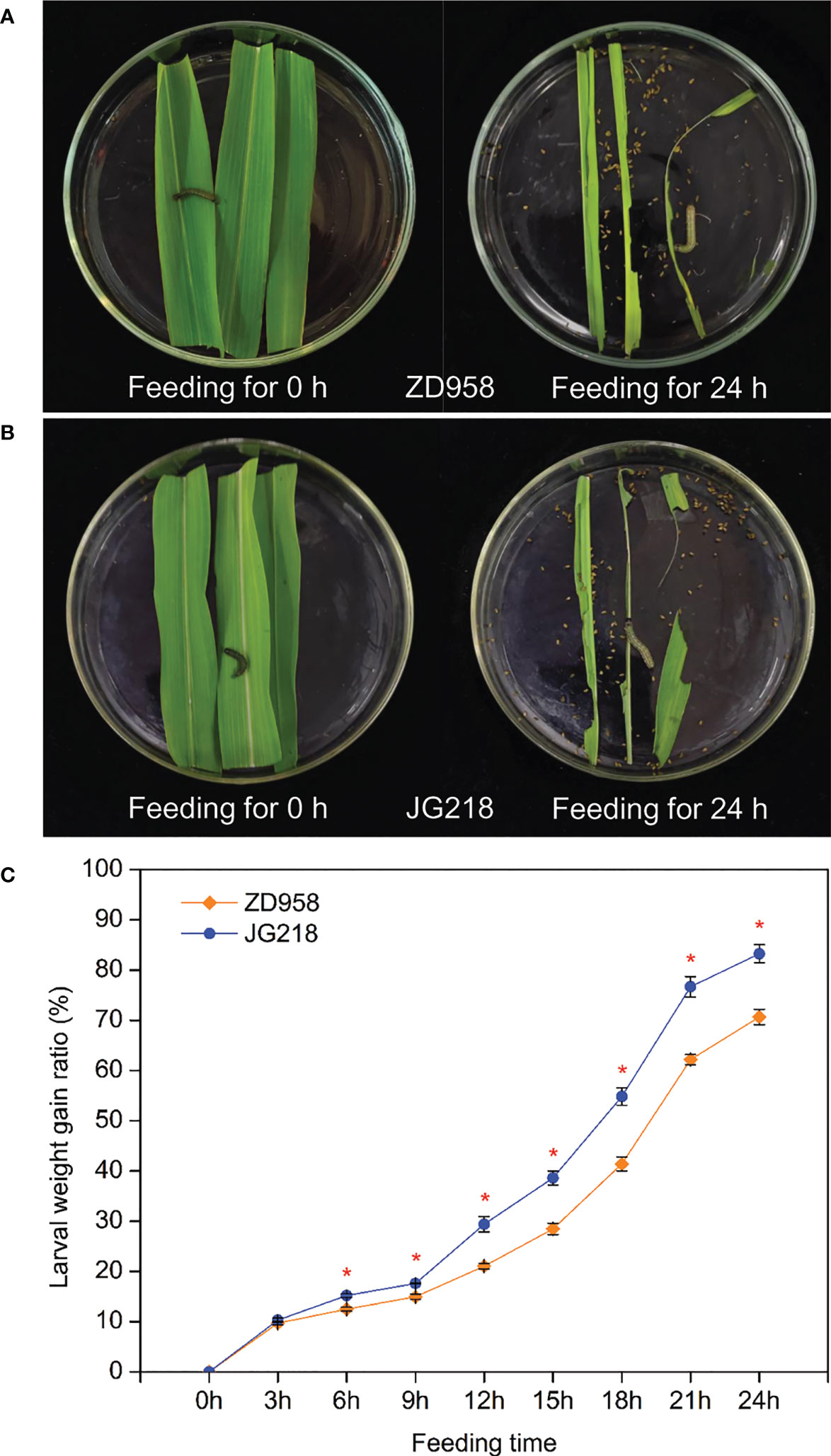
Figure 2 Larval weight gain for S. frugiperda fed on the detached leaves of ZD958 (A) and JG218 (B). (C) Larval weight gain ratio. Data are shown as means ± SD (n = 3, every n represents the mean of 10 values). The asterisk indicates significant differences (P < 0.05) between ZD958 and JG218.
3.2 Mechanical properties of maize leaves
The mechanical properties of maize leaves were analyzed using puncture force. The change trend of puncture force in both maize cultivars was quite similar. Notably, the level of puncture force increased in both maize cultivars from 3 h onwards after S. frugiperda infestation, relative to the control leaves, reached the maximum, and had a statistically significant difference at 12 h (Figure 3). The increase ranged from 5.88% to 27.92% in ZD958 (F = 2.74, df =7, P = 0.045) and from 10.47% to 32.88% in JG218 (F = 4.81, df =7, P = 0.004), respectively. In both the S. frugiperda-infested and the control leaves, the levels of puncture force in ZD958 were significantly higher than that in JG218 at the same point of time. It suggested that more force was required to puncture the tissues of ZD958 than that of JG218.
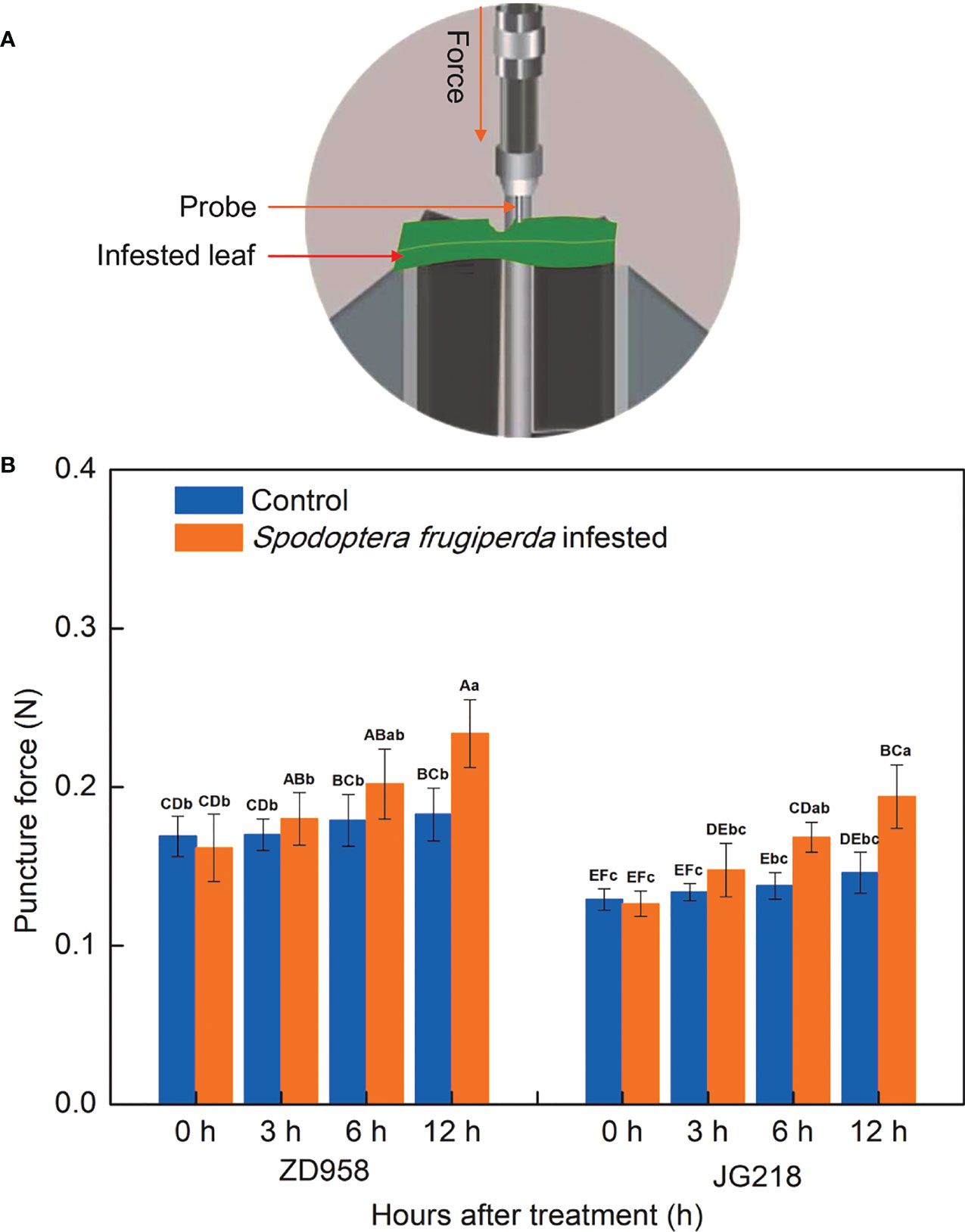
Figure 3 Effect of S. frugiperda infestation on puncture force levels in maize leaves. (A) Force needed to puncture the leaf. (B) Puncture force levels. Data are shown as means ± SD (n = 3, every n represents the mean of three pieces of each of the middle region of the second and third leaf, respectively). Different letters indicate significant differences at P < 0.05. Capital letters represent differences between the two maize cultivars, while lowercase letters indicate differences between treatments within the same cultivar.
3.3 H2O2 and MDA contents in maize leaves
After S. frugiperda infestation, the H2O2 concentration in both maize cultivars tended to first increase and then decrease from 0 h onwards, with a little difference between the two cultivars (Figure 4A). Compared with the control leaves, the H2O2 concentration in the infested leaves of ZD958 first significantly increased the maximum at 3 h by the largest proportion to 21.00% and then significantly decreased the lowest at 12 h with the proportion to 33.88% (F = 14.191, df =7, P = 0.000). The H2O2 concentration in the infested leaves of JG218 was first significantly increased, reached a maximum at 6 h by the proportion to 35.77% (F = 2.932, df =7, P = 0.035), and then decreased to the level of the control at 12 h. Meanwhile, after S. frugiperda infestation, the MDA contents in both maize cultivars also tended to first increase and then decrease to the level of the control from 3 h onwards but remained lower compared with the control at 12 h (Figure 4B). Compared with the control leaves, the MDA content in the S. frugiperda-infested leaves was significantly higher at 3 h for ZD958 (F = 7.264, df =7, P = 0.001) while significantly higher at 3 and 6 h for JG218 (F = 63.849, df =7, P = 0.000). At 3 h after S. frugiperda infestation, the MDA contents in ZD958 and JG218 increased by the largest proportion to 27.32% and 51.09%, respectively.
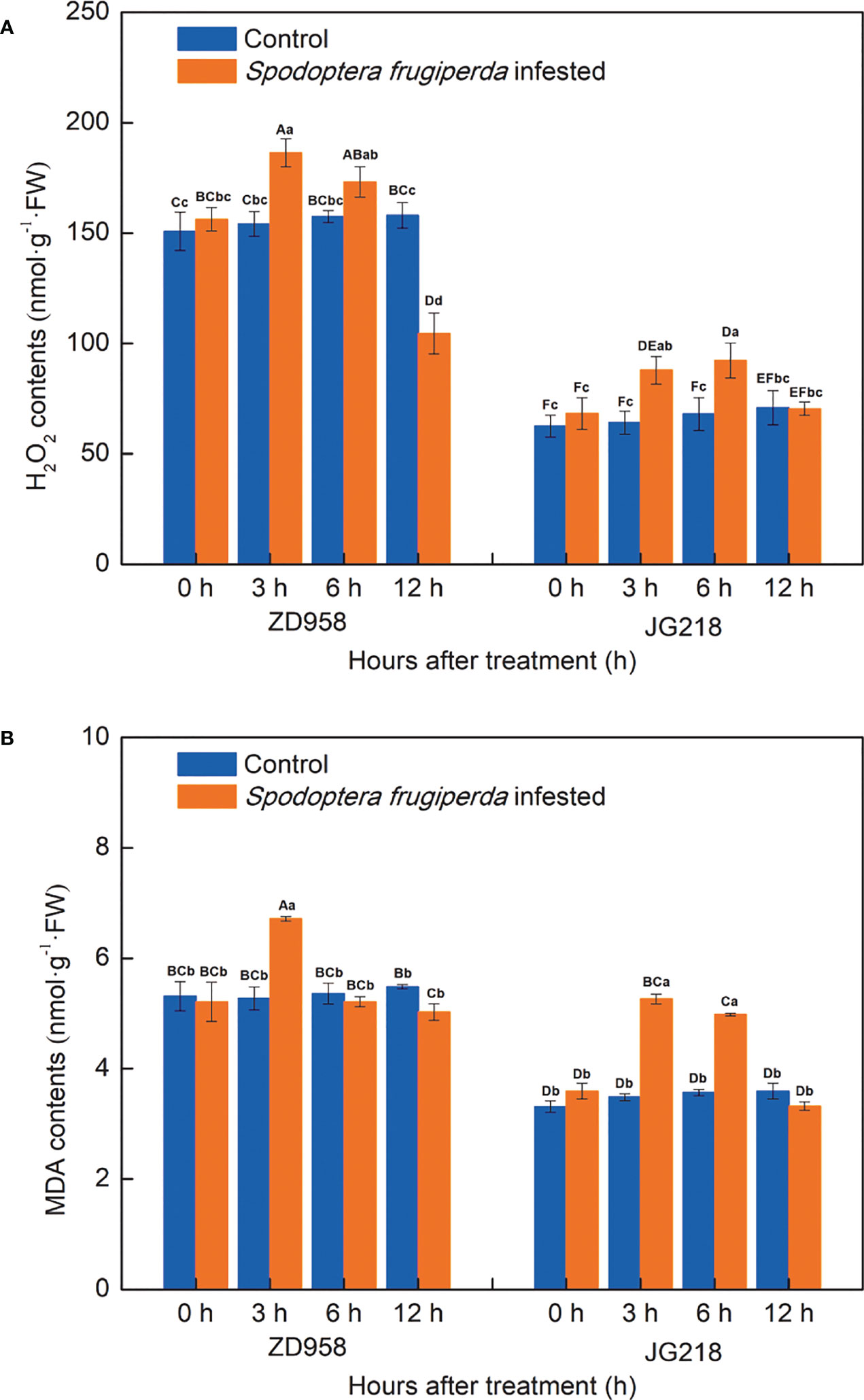
Figure 4 Effect of S. frugiperda infestation on H2O2 (A) and malondialdehyde (B) concentration in maize leaves. Data are shown as means ± SD (n = 3, every n represents the mean of three seedlings). Different letters indicate significant differences at P < 0.05. Capital letters represent differences between the two maize cultivars, while lowercase letters indicate differences between treatments within the same cultivar.
3.4 Total phenolic and flavonoid contents in maize leaves
A greater difference existed in the total phenolic contents of the S. frugiperda-infested leaves and the control leaves. In both maize cultivars, the total phenolic contents of the S. frugiperda-infested leaves tended to first increase and then decrease with prolonged time, reached a maximum at 6 h, but remained higher compared with the control at 12 h, and the differences were significant from 3 to 12 h (Figure 5A). The total phenolic contents in the S. frugiperda-infested leaves of ZD958 and JG218 increased from 1.12% to 16.78% (F = 48.731, df =7, P = 0.000) and from 7.25% to 27.24% (F = 31.741, df =7, P = 0.000), respectively. Similarly, the change trend of the total flavonoid contents was the same between the two maize cultivars. The total flavonoid contents of the S. frugiperda-infested leaves also tended to first increase and then decrease to the level of the control, reached a maximum at 6 h, and remained lower compared with the control at 12 h, but the differences were significant at 3 and 6 h for ZD958 and only at 6 h for JG218 (Figure 5B). The increase ranged from 15.40% to 30.34% in ZD958 (F = 5.185, df =7, P = 0.003) and from 8.28% to 37.15% in JG218 (F = 8.461, df =7, P = 0.000), respectively.
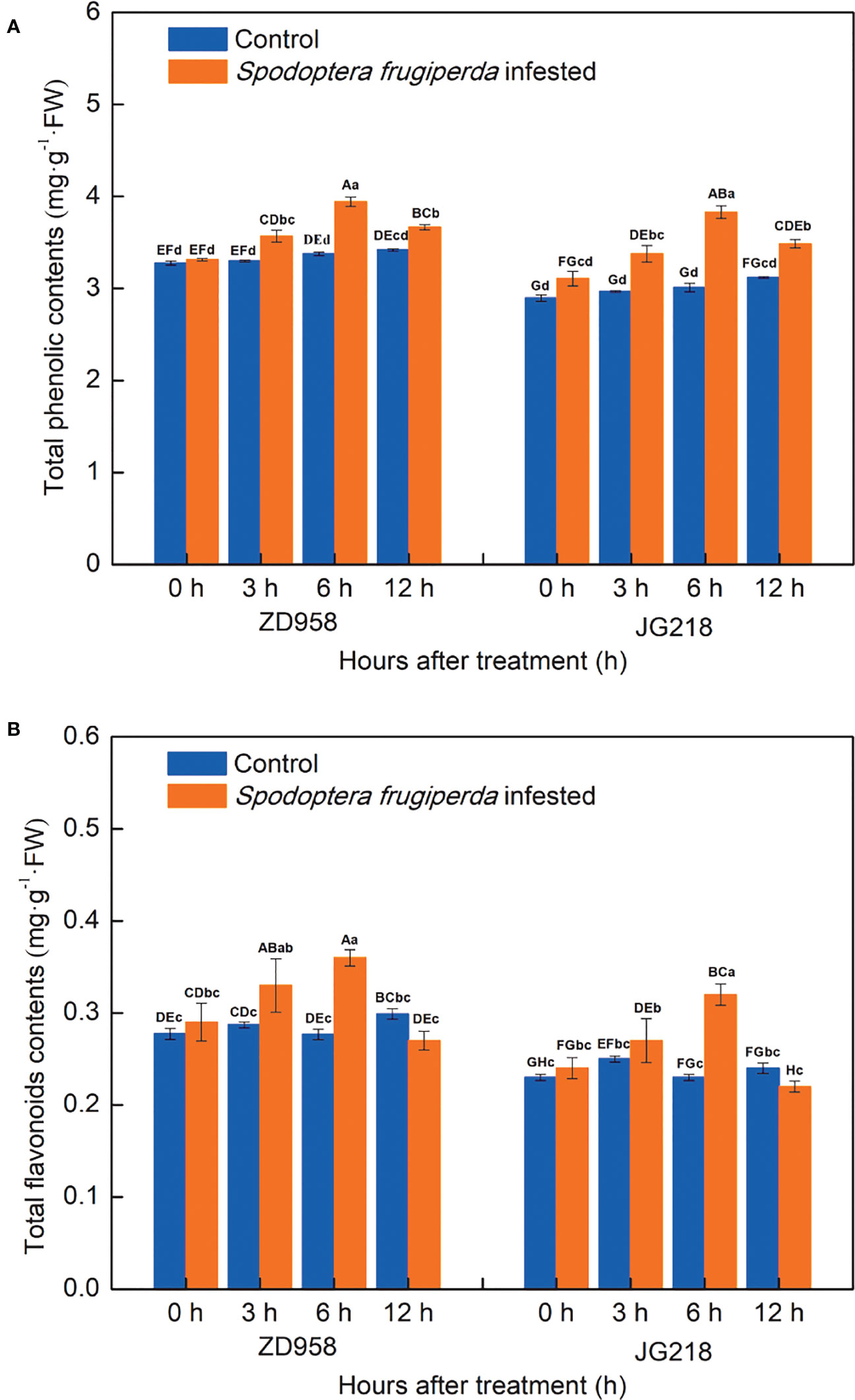
Figure 5 Effect of S. frugiperda infestation on total phenolic compound (A) and flavonoid (B) contents in maize leaves. Data are shown as means ± SD (n = 3, every n represents the mean of three seedlings). Different letters indicate significant differences at P < 0.05. Capital letters represent differences between the two maize cultivars, while lowercase letters indicate differences between treatments within the same cultivar.
3.5 DIMBOA contents in maize leaves
The change trend of DIMBOA contents in both maize cultivars was different (Figure 6). Compared with the control, DIMBOA contents in the infested leaves of ZD958 first significantly increased and then significantly decreased, reached a maximum at 3 h by the largest proportion to 46.85%, and decreased the lowest value at 12 h by the proportion to 50.10% (F = 278.711, df =7, P = 0.000); while DIMBOA contents in the infested leaves of JG218 were first significantly decreased, then significantly increased, and significantly decreased at the late time, with a maximum at 6 h and the largest proportion to 57.27%, a minimum at 3 h with the proportion to 35.59% (F = 222.149, df =7, P = 0.000). Both the S. frugiperda-infested and the control leaves, from 0 h onwards, the DIMBOA contents in ZD958 were significantly higher than that in JG218 at the same point of time, except for at 6 h in the S. frugiperda-infested leaves.
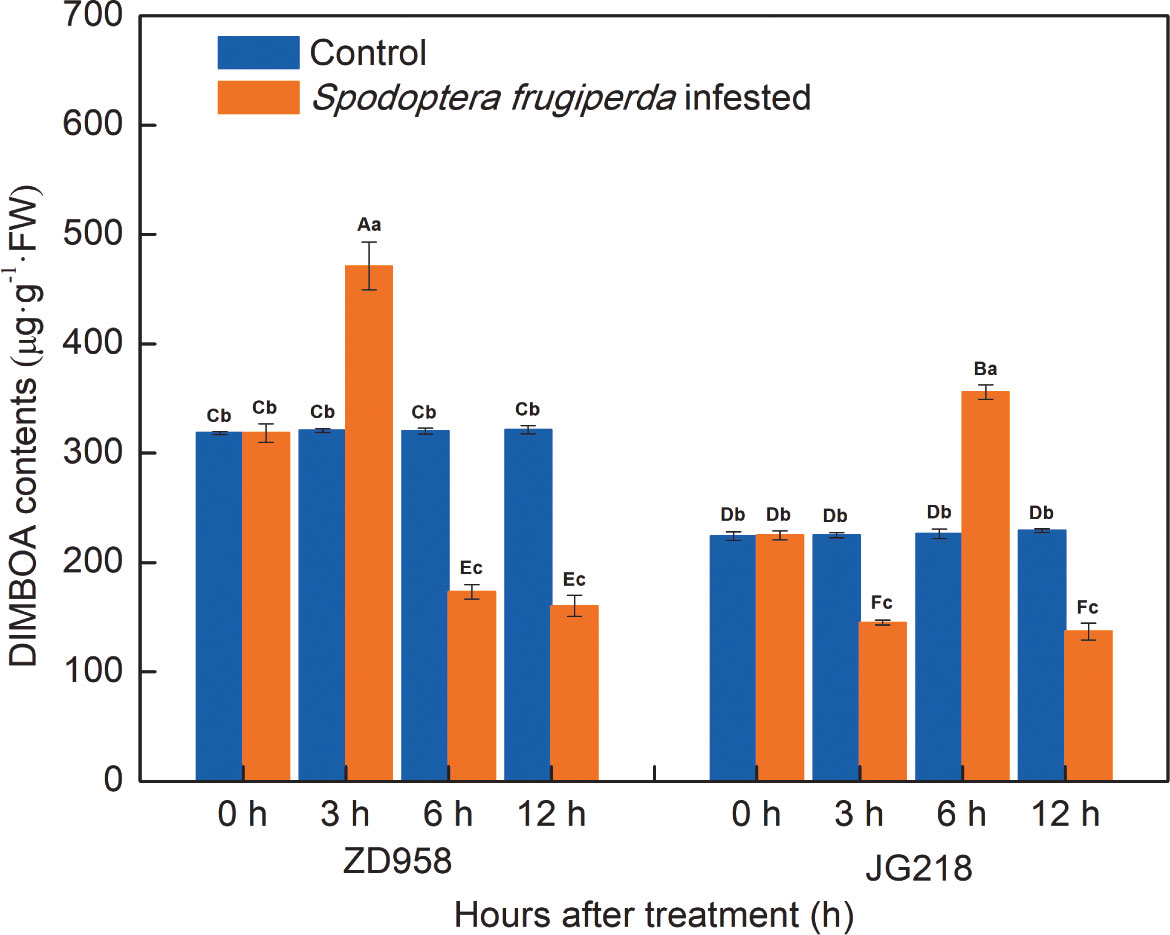
Figure 6 Effect of S. frugiperda infestation on 2,4-dihydroxy-7-methoxy-1,4-benzoxazin-3-one contents in maize leaves. Data are shown as means ± SD (n = 3, every n represents the mean of three seedlings). Different letters indicate significant differences at P < 0.05. Capital letters represent differences between the two maize cultivars, while lowercase letters indicate differences between treatments within the same cultivar.
3.6 Antioxidant enzyme activity in maize leaves
After S. frugiperda infestation, the change trend of SOD activities in the leaves of both maize cultivars is similar, tended to first increase and then decrease to the level of the control, with the maximum at 3 h (Figure 7A). The SOD activities in the infested-leaves of both maize cultivars were significantly higher at 3 and 6 h compared with the control, but no statistically significant difference at 12 h. The increase ranged from 3.86% to 17.54% in ZD958 (F = 36.667, df =7, P = 0.001) and from 8.48% to 39.68% in JG218 (F = 53.234, df =7, P = 0.000), respectively. Similarly, POD activities in the infested-leaves of both maize cultivars also tended to first increase and then decrease, reached the maximum at 6 h, but a little differences between two cultivars (Figure 7B). Compared with the control, POD activities in the infested-leaves were significantly higher for ZD958 at 3 and 6 h (F = 10.111, df =7, P = 0.001), and higher but no significant difference at 12 h; while significantly higher for JG218 at 6 and 12 h (F = 49.806, df =7, P = 0.001). The increase ranged from 5.02% to 25.50% in ZD958 and from 6.68% to 32.74% in JG218, respectively. By contrast, the change trend of CAT activities in the leaves of both maize cultivars was different with the SOD and POD activities, tended to first significantly decrease (F = 27.066, df =7, P = 0.000 for ZD958; F = 56.514, df =7, P = 0.001 for JG218) and then increase to slightly higher than the control but no significant difference and showed a minimum at 3 h (Figure 7C). Both the S. frugiperda-infested and the control leaves, from 0 h onwards, the SOD, POD and CAT activities in ZD958 were significantly higher than that in JG218 at the same point of time, except for the CAT activity at 6 h in the S. frugiperda-infested leaves.
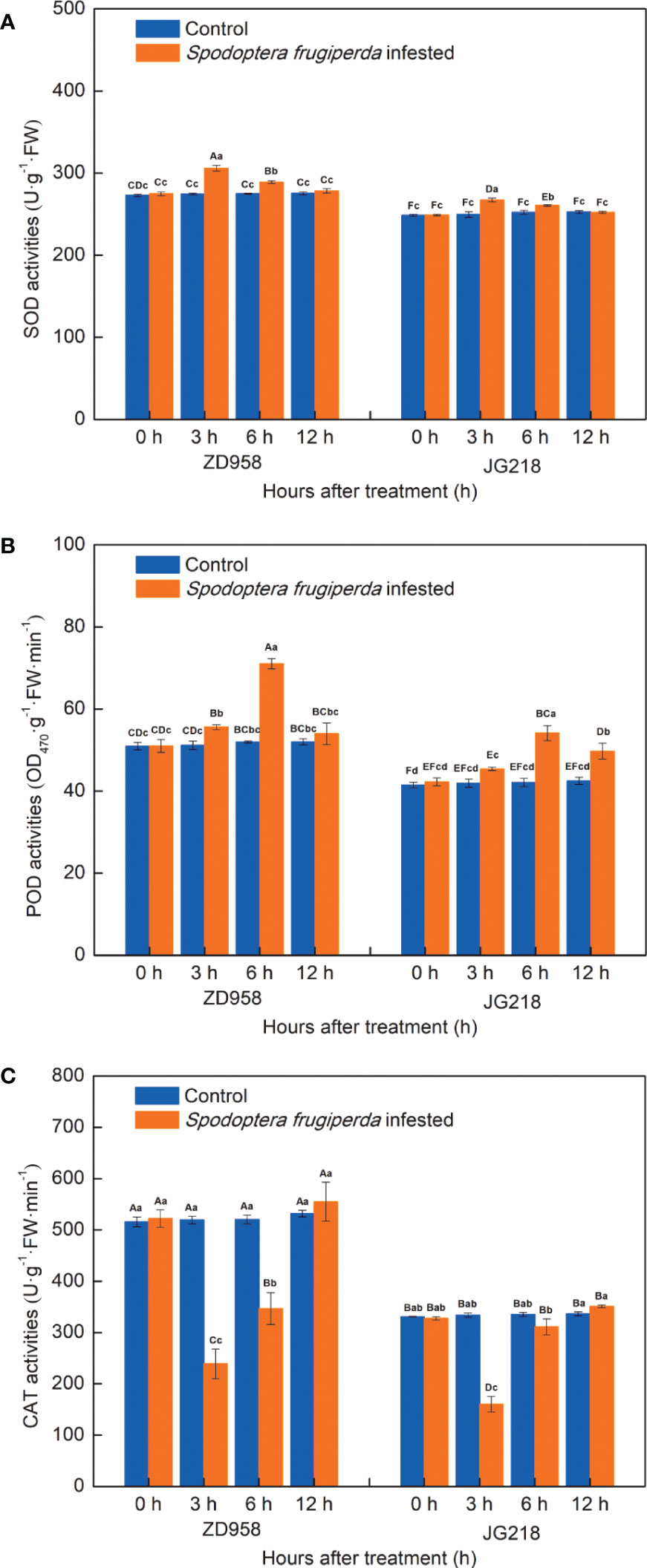
Figure 7 Effect of S. frugiperda infestation on antioxidant enzyme activity in maize leaves. (A) superoxide dismutase activity. (B) Peroxidase activity. (C) Catalase activity. Data are shown as means ± SD (n = 3, every n represents the mean of three seedlings). Different letters indicate significant differences at P < 0.05. Capital letters represent differences between the two maize cultivars, while lowercase letters indicate differences between treatments within the same cultivar.
3.7 JA, SA, and ABA contents in maize leaves
In order to investigate the endogenous plant hormones in response to S. frugiperda infestation, we analyzed the contents of JA, SA and ABA. Notably, the level of endogenous JA significantly increased in JG218 (F = 113.967, df =7, P = 0.000) from 3 h onwards while in ZD958 (F = 20.055, df =7, P = 0.000) from 6 h onwards after S. frugiperda infestation, relative to the control leaves (Figure 8A). At 12 h after S. frugiperda infestation, the levels of JA in the S. frugiperda-infested leaves of ZD958 and JG218 were 1.53 and 2.52 times as high as the control, respectively. By contrast, the levels of SA in both maize cultivars do not appear to be different between the S. frugiperda-infested and the control leaves, except for a significant decrease at 3 h (F = 1.956, df =7, P = 0.126 for ZD958; F = 0.737, df =7, P = 0.645 for JG218) (Figure 8B). Similarly, compared with the control, the levels of ABA in the infested leaves of both maize cultivars were first decreased and then increased, and remained lower at the late time in ZD958 (F = 2.382, df =7, P = 0.071), but significantly lower at 3 h and significantly higher at 12 h in JG218 (F = 6.836, df =7, P = 0.001) (Figure 8C). It indicated that JA might play a central role in response to S. frugiperda infestation and ABA might be involved.
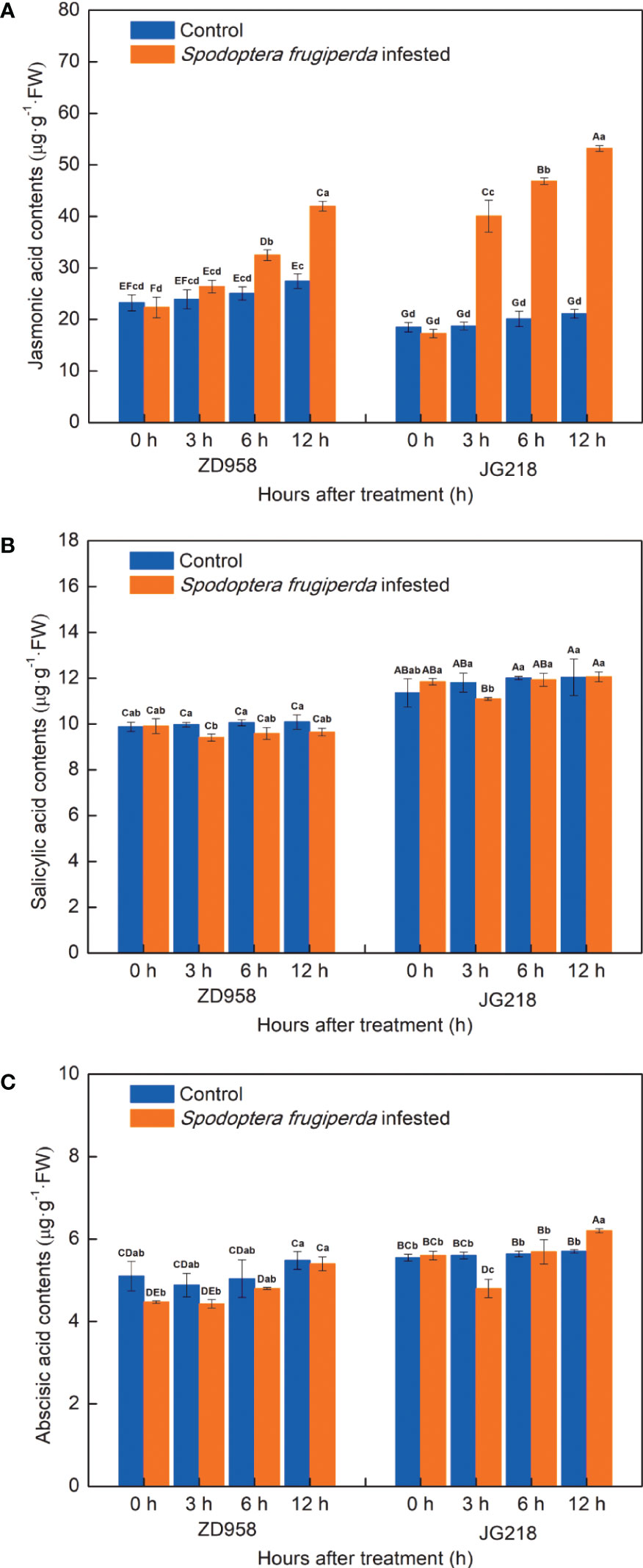
Figure 8 Effect of S. frugiperda infestation on salicylic acid (A), jasmonic acid (B), and abscisic acid (C) contents in maize leaves. Data are shown as means ± SD (n = 3, every n represents the mean of three seedlings). Different letters indicate significant differences at P < 0.05. Capital letters represent differences between the two maize cultivars, while lowercase letters indicate differences between treatments within the same cultivar.
3.8 Phytohormones and secondary metabolite-related gene expression
PAL and CHS, the key enzymes in phenylpropanoid pathway, are involved in the biosynthesis of phenolics, flavonoids and SA (Figure 9A). After S. frugiperda infestation, the expression level of PAL4 (Zm00001d051166) gene had a significant difference between the S. frugiperda-infested and the control leaves from 3 h onwards for ZD958 (F = 413.498, df =7, P = 0.000), while a significant difference between the S. frugiperda-infested and the control leaves from 0 to 6 h for JG218 (F = 1041.224, df =7, P = 0.000) (Figure 9E). At 3 h after S. frugiperda infestation, the expression of PAL4 reached a peak, and increased by 7.70-fold for ZD958 and 8.14-fold for JG218, relative to the control leaves. CHS6 (Zm00001d016014) also showed a similar tendency as PAL4 (Figure 9E). After S. frugiperda infestation, CHS6 had a significant difference between the S. frugiperda-infested and the control leaves from 3 h onwards for ZD958 (F = 744.339, df =7, P = 0.002), while a significant difference between the S. frugiperda-infested and the control leaves at 3 and 6 h for JG218 (F = 487.082, df =7, P = 0.001), and with a peak level of 3.87-fold for ZD958 and 4.41-fold for JG218 at 6 h, respectively. LOXs play an important role in the biosynthesis of JA (Figure 9B). After S. frugiperda infestation, the expression level of LOX1 (Zm00001d042541) also had a significant difference between the S. frugiperda-infested and the control leaves from 3 h onwards for both maize cultivars and increased by 64.13-fold for ZD958 (F = 2292.333, df =7, P = 0.003) and 83.79-fold for JG218 at 3 h (F = 2601.748, df =7, P = 0.000), relative to the control leaves (Figure 9E). In the ABA biosynthetic pathway, NCED is the key enzyme (Figure 9C). After S. frugiperda infestation, the expression level of NCED9 (Zm00001d013689) first decreased at 3 h, then increased from 6 to 12 h for both maize cultivars and had a significant difference between the S. frugiperda-infested and the control leaves at 12 h for ZD958 (F = 35.94, df =7, P = 0.001), while a significant difference between the S. frugiperda-infested and the control leaves at 3 and 12 h for JG218 (F = 35.705, df =7, P = 0.001) (Figure 9E). BXs are associated with DIMBOA biosynthesis (Figure 9D). The expression level of BX12 (Zm00001d029353) continued to increase from 0 to 12 h for both maize cultivars after S. frugiperda infestation and had a significant difference between the S. frugiperda-infested and the control from 3 h onwards (Figure 9E). At 12 h, the levels of BX12 in the S. frugiperda-infested leaves of ZD958 (F = 624.264, df =7, P = 0.002) and JG218 (F = 303.731, df =7, P = 0.000) were 8.09 and 8.95 times as high as the control, respectively.
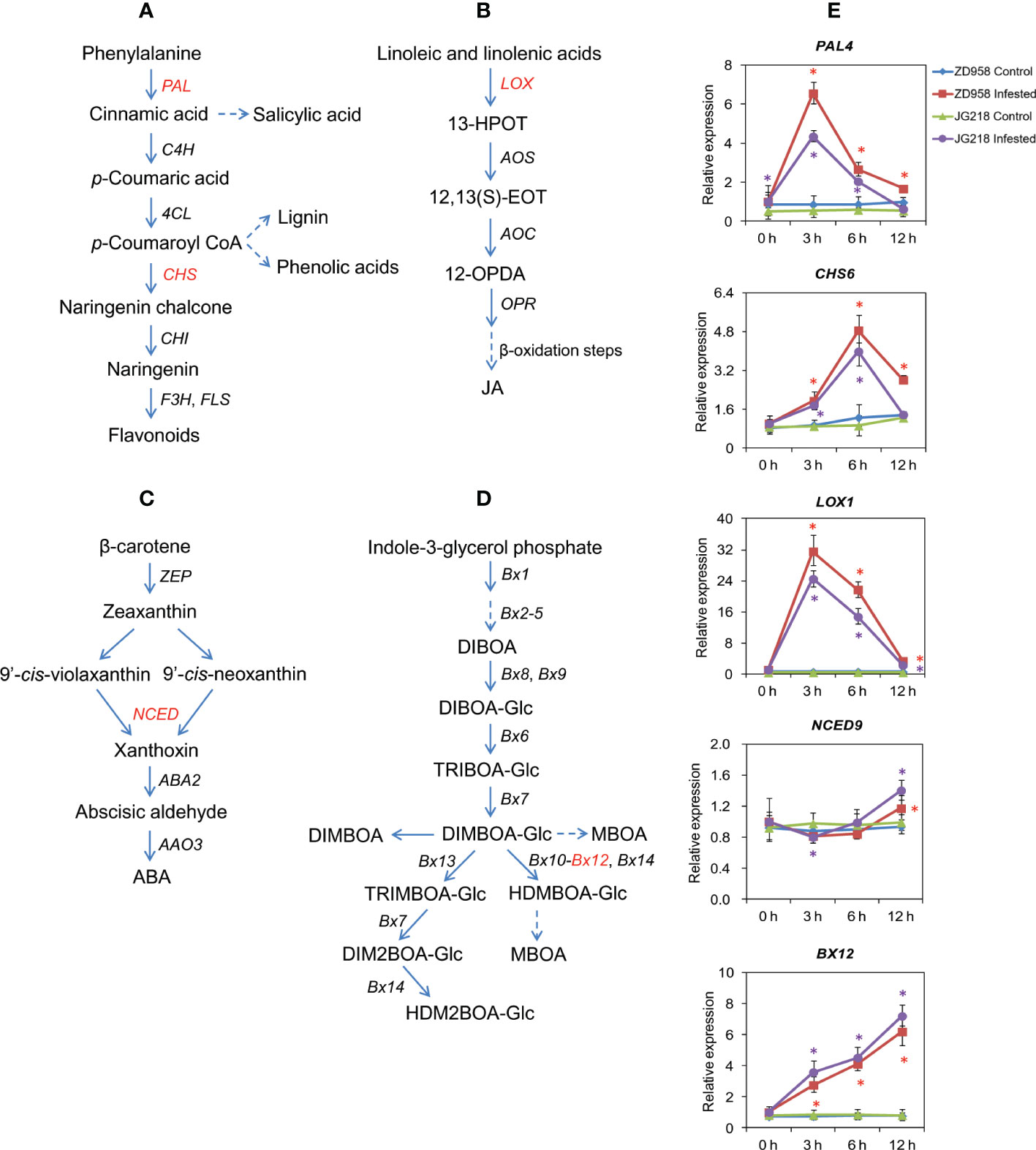
Figure 9 Effect of S. frugiperda infestation on phytohormones and secondary metabolite-related gene expression in maize leaves. (A) Biosynthetic pathway of flavonoids and salicylates. (B) Biosynthetic pathway of jasmonic acid. (C) Biosynthetic pathway of abscisic acid. (D) Biosynthetic pathway of benzoxazinoid. (E) qRT-PCR analysis of phytohormones and secondary metabolite-related genes. Data are shown as means ± SD (n = 3, every n represents the mean of three seedlings). Significant differences at P < 0.05 between the control and S. frugiperda-infested groups are indicated with an asterisk. The red asterisk represents significant differences for ZD958 and the purple asterisk for JG218.
4 Discussion
4.1 Rapid generation of excessive MDA and H2O2 in maize leaves’ response to S. frugiperda infestation
Plants show various responses to stress, including morphological, biochemical, and molecular traits. Under stressful conditions, it is often found that excessive hydrogen peroxide production and oxidative pressure related with high H2O2 concentrations and antioxidant mechanisms that help scavenge redundant reactive oxygen species (ROS) in plants (Choudhury et al., 2013). The primary symptom of plants to biotic stress is the activation of their defensive systems, such as ROS generation, especially H2O2. In plant cells, when H2O2 enters the cell membrane, destructive processes are started and biological molecules are oxidized, consequently resulting in cell apoptosis (Quan et al., 2008). Herbivore attack often increases ROS generation in plants, including H2O2 (Tyagi et al., 2022). Greater accumulation of MDA indicates enhanced production of ROS. Our results showed that, after S. frugiperda infestation, both maize cultivars accumulated more H2O2 compared with their corresponding control plants from 0 to 6 h; compared with the control, the MDA content was significantly higher at 3 h for ZD958, while significantly higher at 3 and 6 h for JG218. As already mentioned, the higher concentration of H2O2 probably result in peroxidative cell membrane damage. It is consistent with the increased MDA content. Furthermore, after S. frugiperda infestation, the maximum increase of H2O2 and MDA contents was greater in JG218 (33.88% for H2O2 and 51.09% for MDA) than that in ZD958 (21.00% for H2O2 and 27.32% for MDA). It suggested that the oxidative damage of cell membrane in JG218 was more serious than that in ZD958, and JG218 was more susceptible to S. frugiperda than ZD958. It is evidenced that the larval weight gain ratio of JG218 leaves (0–83.28%) was higher than that of ZD958 leaves (0–70.64%) within 24 h.
4.2 SOD, POD, and CAT participate in maize plant’s defensive response to S. frugiperda infestation
It is well-known that antioxidant mechanisms are the effective ROS scavenging systems. SOD, POD, and CAT are three antioxidant enzymes that play a crucial role in maintaining a healthy balance between the production and elimination of ROS (Nadarajah, 2020). SOD catalyzes the dismutation reaction of superoxide into H2O2, and POD and CAT act on the peroxide to reduce it. In contrast, CAT increases resistance to the cell wall and triggers the expression of defensive genes (Zhao et al., 2016). Induction of antioxidative enzymes in plants by herbivory has attracted more and more attentions (War et al., 2012). In cowpea plants, the higher SOD and POD levels were observed in S. frugiperda injured leaves of the landrace Juti which showed higher resistance levels (Costa et al., 2020). In cucurbits, after melon fly infestation, in healthy and infected tissues of chayote and bottle gourd (resistant fruits) compared with bitter gourd, snake gourd, and cucumber (susceptible cucurbits fruits), higher activities of SOD, POD, polyphenol oxidase (PPO) and CAT were observed (Somegowda et al., 2021). After gall wasp Dryocosmus kuriphilus (GWDK) infestation, at all three stages of development, the activities of the POD and SOD enzymes were higher in the resistant chestnut variety leaves than they were in the susceptible chestnut variety leaves. On the other hand, the activities of the CAT and PPO enzymes were mostly higher in the susceptible chestnut variety leaves (Zhu et al., 2022). In present study, both the S. frugiperda-infested and the control leaves, from 0 h onwards, the activities of SOD, POD, and CAT in ZD958 were significantly higher than that in JG218 at the same point of time, except for CAT at 6 h in the S. frugiperda-infested leaves, which is compatible with the above mentioned that the activities of antioxidant enzymes are higher in resistant ones. Furthermore, SOD activities in both maize cultivars tended to significantly increase and then decrease to the level of the control, POD activities in ZD958 also tended to significantly increase and then decrease to the level of the control while in JG218 tended to increase and then decrease but remained significantly higher compared with the control, and CAT activities were significantly decreased and then increased to the level of the control. It suggested that maize plants activated their antioxidant enzyme system after S. frugiperda infestation and the damage to maize plants was stabilized at the late time. The results were further evidenced by the increase and subsequent decrease of lipid peroxidation product MDA accompanied by H2O2 contents in the S. frugiperda-infested leaves with the time prolong.
4.3 Phenolic and flavonoid compounds involved in maize plant’s defensive response to S. frugiperda infestation
Apart from antioxidant enzymes, plants also have a mechanism for synthesizing defensive substances in response to invading herbivores, such as plant secondary metabolites. Plant secondary metabolites are essential for plant–environment interactions. They are produced synthetically and can also be induced by biotic and abiotic stresses. Of the secondary metabolites, polyphenols are the most widely distributed and significant class of plant stress-resistant compounds (Singh et al., 2021). Additionally, polyphenols are crucial in the cyclic reduction of ROS, which, in turn, trigger a series of reactions that initiate the activation of defense-related enzymes (Blokhina et al., 2003; Nadarajah, 2020). According to chemical structure, phenolic acids, flavonoids, stilbenes, and lignans are the major groups of polyphenols which have been actively engaged in protecting plants against herbivores (Divekar et al., 2022). Many research have already reported that the accumulation of phenolics and flavonoids in plants makes them resistant to a variety of abiotic and biotic stresses. Specifically, cucurbits with melon fly infestation tissues, compared with healthy and apparently healthy tissues, had significantly higher total phenolic and flavonoid levels (Somegowda et al., 2021). Phenolic acids and flavonoids, particularly quercetin, have also increased in white cabbage upon infestation by cabbage butterflies and flea beetles (Kovalikova et al., 2019). These reports are line with our results that the total phenolic and total flavonoid contents were higher in the S. frugiperda-infested leaves compared with the control leaves, except for the total flavonoid contents at 6 h. It indicated that maize plants initiated a defensive response after S. frugiperda infestation. Furthermore, after S. frugiperda infestation, the changes of total phenolic and total flavonoid contents were greater in JG218 than those in ZD958, suggesting that ZD958 had higher resistance than JG218. In addition, POD is responsible for the catalytic synthesis of lignins and oxidative phenols, which contribute to the strengthening of the cell structure and make it challenging for insects to insert their mouth stylets (de Lima Toledo et al., 2021). In this study, after S. frugiperda infestation, the increase of POD activities and total phenolic contents is in agreement with that of previous reports. Moreover, after S. frugiperda infestation, the level of puncture force increased in both maize cultivars also indicated that the plant cell structure was strengthened.
4.4 DIMBOA is an important defensive compound in maize plant’s defensive response to S. frugiperda infestation
The members of the most extensively studied class of defensive compounds, benzoxazinoids, are crucial to plant resistance to numerous pathogens and pests (Wouters et al., 2016). They are found in the plant family Poaceae (formerly Gramineae) and some dicots, mostly during the early stages of plant development. The main benzoxazinoid found in young maize plants is 2,4-dihydroxy-7-methoxy-1,4-benzoxazin-3-one (DIMBOA), which is considered to be the most important chemical factor in resistance to leaf-feeding insects (Santiago et al., 2017; Kudjordjie et al., 2019). 2-(2,4-Dihydroxy-7-methoxy-1,4-benzoxazin-3-one)-β-d-glucopyranose (DIMBOA-Glc) as well as 2-hydroxy-4,7-dimethoxy-1,4-benzoxazin-3-one glucoside (HDMBOA-Glc) and 6-methoxy-benzoxazolin-2-one (MBOA) were the products of benzoxazinoid biosynthesis (Shavit et al., 2018). DIMBOA is released when plant tissues are damaged either by humans or by nature. Therefore, pathogen infections, insect feeding, and artificial plant damage may cause DIMBOA production (Zhou et al., 2018). In durum wheat, after 96 h of infestation, both Sitobion avenae and Rhopalosiphum padi aphid feeding significantly induced the predominant metabolites DIMBOA and its glycosylated form DIMBOA-Glc (Shavit et al., 2018). In maize leaves, at 24 h, HDMBOA-Glc and MBOA had the highest induction levels and were significantly upregulated. DIMBOA and DIMBOA-Glc were significantly reduced with continued O. furnacalis feeding (Guo et al., 2017; Guo et al., 2019). In this study, significant increases were observed for DIMBOA contents in the S. frugiperda-infested leaves and then followed by a significant decline at a later time, and the DIMBOA contents in ZD958 were significantly higher than those in JG218 at the same point of time, except at 6 h in the S. frugiperda-infested leaves. It suggested that ZD958 had higher resistance than JG218. The DIMBOA contents first significantly increased and then significantly decreased, which could be explained in such a way that S. frugiperda induced the accumulation of DIMBOA first, and then DIMBOA turned into DIMBOA derivatives.
4.5 JA might play a central role in S. frugiperda-induced signaling
Not only do phytohormones play an important role in plant development but also they serve as defense against various herbivores (Erb et al., 2012; War et al., 2012; Ku et al., 2018). The phytohormones JA and SA play a significant role in plant defense through multiple signal transductions (Ding and Ding, 2020; Ghorbel et al., 2021; Wang et al., 2021). JA is regarded as a key player in the induction of plant responses against herbivore attacks (Koo and Howe, 2009; Bosch et al., 2014; Escobar-Bravo et al., 2019) through the induction of a variety of defensive substances like polyamines, quinones, terpenoids, alkaloids, phenylpropanoids, glucosinolates, and antioxidants (Zhou and Memelink, 2016; Eisenring et al., 2018). Similarly, by a variety of metabolic processes, SA also regulates plant growth and defense (War et al., 2012)—for instance, to reduce the damage caused by the cotton bollworm Helicoverpa armigera, the SA signaling pathway in tomato produces various antioxidant enzymes (Peng et al., 2004). It is evident that SA induces more resistance against insects that penetrate and suck, whereas JA is primarily involved in defense against herbivores that eat leaves by chewing (Schweiger et al., 2014; Aljbory and Chen, 2018). The cross-talk between the ABA signaling pathway and other phytohormones like cytokinin, JA, and SA can alter how plants interact with herbivorous insects (Guo et al., 2016)—for instance, both wheat and rice plants were attacked by hessian fly, which resulted in an increase of SA, 12-oxo-phytodienoic acid, and JA but a decrease of ABA (Zhu et al., 2011). The content of JA, SA, and ABA in potato was significantly increased under aphid stress, suggesting that the cross-talks of phytohormones defend against aphid attack (Quandahor et al., 2020). In pepper plants, after an aphid attack, JA and its metabolite JA-Ile increased significantly, the SA levels remained unaltered until 7 days post-infestation, and the ABA content tended to rise later in the aphid infestation (Florencio-Ortiz et al., 2020). In soybean plants, despite the fact that aphid infestation resulted in higher SA levels in all soybean genotypes, the constitutive elevated levels of JA and ABA were an important factor in soybean tolerance to soybean aphids (Chapman et al., 2018). These results suggested that the phytohormones typically interact antagonistically or synergistically, acting in concert. In this study, S. frugiperda is a polyphagous chewing pest. The JA content of the infested leaves was significantly increased in JG218 from 3 h onwards while in ZD958 from 6 h onwards after S. frugiperda infestation, which was up to 2.52 and 1.53 times as high as the control. The SA content of both maize cultivars first significantly decreased at 3 h and then increased to the level of the control at a later time. The ABA content did not appear to be different between the S. frugiperda-infested and control leaves of ZD958 while significantly lower at 3 h and significantly higher at 12 h in the infested leaves of JG218, indicating that JA had a central role in S. frugiperda-induced signaling with overlapping and synergistic effects on SA and ABA pathways. Our results agree that the JA pathway is typically initiated by chewing insects, and JA signaling is the fundamental pathway that controls the plants’ defensive responses to herbivores (Schweiger et al., 2014; Howe et al., 2018). Previous studies have shown that the biosynthesis of alkaloids, terpenoids, and phenylpropanoids, among other secondary metabolites, is thought to be related to JA in plants (Bali et al., 2019). Furthermore, it has been reported that JA is able to induce the activities of antioxidant enzymes such as SOD, POD, CAT, and ascorbate peroxidase in plants under stresses (Sirhindi et al., 2016; Zhu et al., 2022). The increase in total phenolic and total flavonoid contents and SOD and POD activities in this study suggest that JA might play a vital role in inducing plant responses against S. frugiperda attack through the induction of various defensive compounds and antioxidant enzymes.
4.6 Genes associated with PAL4, CHS6, BX12, LOX1, and NCED9 play an important role in maize plant’s defensive response to S. frugiperda
Understanding the resistance mechanisms and identifying potent genes involved in plant defense mechanisms rely heavily on gene expression and post-transcriptional regulation (Zhang et al., 2019). Genomic reprogramming takes place as a result of the infestation, which ultimately controls the synthesis of proteins or the production of secondary compounds. It has been demonstrated that benzoxazine plays a significant role in maize’s pest resistance. DIMBOA is the main benzoxazinoid found in maize. BX genes are associated with DIBOA biosynthesis pathway (Tzin et al., 2017). In this study, BX12 catalyzing the reaction of DIMBOA-Glc to HDMBOA-Glc was highly upregulated in the S. frugiperda-infested leaves, which is consistent with previous results, such that four genes associated with benzoxazinone synthesis were highly elicited when armyworms attacked maize (Yang et al., 2020). During Ostrinia furnacalis feeding in maize, all BX genes, with the exception of BX1, BX5, BX7, and BX8, were significantly upregulated (Guo et al., 2019). These results indicate that the most prevalent responsive defense compound in maize plants is benzoxazine. In the plant defensive pathway, the phenylpropanoid pathway occupies a central position (Yadav et al., 2020). PAL is the initial enzyme in the phenylpropanoid pathway (Dehghan et al., 2014) and also responds to the phytohormones methyl jasmonate, ethylene, JA, and SA. Another significant enzyme in the phenylpropanoid cascade is CHS, and it catalyzes flavonoid biosynthesis (Dehghan et al., 2014). Phenylpropanoids have previously been shown to be induced to accumulate in the tissues of cucurbits in response to melon fly infestation, white cabbage exposed to cabbage butterflies, and flea beetles and cotton fed with Helicoverpa armigera and Spodoptera litura (Kovalikova et al., 2019; Dixit et al., 2020; Somegowda et al., 2021). In this study, on certain time points after S. frugiperda infestation, PAL4 and CHS6 were significantly upregulated, and the accumulation of total phenolics and total flavonoids was significantly increased. It has a similarity with a previous finding that suggests that the transcript levels of the genes in the phenylpropanoid pathway and the phenolic acid, proanthocyanidin, and tannin accumulation in cotton rise after an infestation of chewing insects (Dixit et al., 2020). Moreover, PAL also plays a role in the biosynthesis of SA (Lefevere et al., 2020). A study in tomato found that PAL gene associated with SA pathway was significantly upregulated at each time interval after exposure to aphids (Hanan et al., 2020). However, compared with the control, the content of SA in both maize cultivars only significantly decreased at 3 h after S. frugiperda infestation; no significant difference was observed at the other time points in this study. The predominant function of JA in mediating plant defense against attack by chewing insects is well known, and LOX genes are involved in the upstream reaction of the JA pathway (War et al., 2012). In this study, LOX1 gene involved in the biosynthesis of JA was significantly induced in both maize cultivars by S. frugiperda infestation. This is in line with previous studies which found that most genes, including LOX1, involved in the JA pathway in maize were significantly upregulated following O. furnacalis herbivory (Guo et al., 2019). Two JA-related genes LOX and OPR3 were significantly induced in bean plants fed by pea leafminer larvae for 4 days (Yang et al., 2021). The hormone ABA is important in regulating the relations of plant and abiotic stress. 9-cis-epoxycarotenoid dioxygenase (NCED) plays a key role in the ABA biosynthesis pathway (Han et al., 2004). The role of ABA in plant–herbivore interactions has gained attention—for example, upon infestation by striped stem borer, the β-carotene hydroxylase and NCED genes were significantly upregulated in rice seedlings at 3 or 6 h (Li et al., 2020). Additionally, NCED gene expression significantly increased following O. furnacalis feeding (Guo et al., 2019). In our study, NCED9 was significantly upregulated in both maize cultivars only at 12 h after S. frugiperda infestation. This suggests that ABA has been a part of maize’s defensive response since the beginning of the S. frugiperda infestation. Combined with the total phenolic, flavonoid, and DIMBOA contents, the JA, SA, and ABA levels, and the PAL4, CHS6, BX12, LOX1, and NCED9 expression levels, we found that whether or not the accumulation of these substances synchronized with the expression level of these genes depended on the upstream, midstream, or downstream genes involved in the biosynthetic pathway that they regulate. The relationship of JA, SA, and ABA is an interesting topic to be further explored.
5 Conclusion
In conclusion, our results demonstrated that S. frugiperda rapidly triggered off the antioxidative response of maize plants, and JG218 was more sensitive to S. frugiperda than ZD958. It is mainly reflected by the following aspects: first, the H2O2 and MDA contents of infested leaves were rapidly and significantly increased and then decreased to the level of the control. Compared with the control leaves, the puncture force values and the total phenolic, total flavonoid, and DIMBOA contents of infested leaves were significantly increased as well within a certain time. The SOD and POD activities of infested leaves were significantly increased in a certain period of time, while the CAT activities decreased significantly and then increased to the control level. The JA levels of infested leaves were significantly improved, whereas the SA and ABA levels changed less. Genes associated with phytohormones and defensive substances including PAL4, CHS6, BX12, LOX1, and NCED9 were significantly induced at certain time points, especially LOX1 whose function needs to be further studied. Most of these parameters changed greater in JG218 than in ZD958. Moreover, the larvae bioassay showed that S. frugiperda larvae weighed more on JG218 leaves than those on ZD958 leaves. It can make us understand better the resistance mechanisms of maize plants to S. frugiperda, providing a theoretical basis for developing crop cultivars’ defense against herbivores and for pest management for sustainable crop production.
Data availability statement
The original contributions presented in the study are included in the article/Supplementary Material. Further inquiries can be directed to the corresponding authors.
Author contributions
XJ and HL contributed to the conception of the study and project administration. JY, CM and RJ conducted the experiments and data analyses. HL and JY wrote the manuscript. XJ revised the manuscript. HY, YZ and HZ gave advice. All authors carefully read, revised, and approved the submitted version.
Funding
This work was financially supported by the National Key Research and Development Program of China (2019YFE0120400), the Natural Science Foundation of Shandong Province, China (ZR2021MC107) and the China Scholarship Council (202108370191).
Conflict of interest
The authors declare that the research was conducted in the absence of any commercial or financial relationships that could be construed as a potential conflict of interest.
Publisher’s note
All claims expressed in this article are solely those of the authors and do not necessarily represent those of their affiliated organizations, or those of the publisher, the editors and the reviewers. Any product that may be evaluated in this article, or claim that may be made by its manufacturer, is not guaranteed or endorsed by the publisher.
Supplementary material
The Supplementary Material for this article can be found online at: https://www.frontiersin.org/articles/10.3389/fpls.2023.1065891/full#supplementary-material
References
Ahmad, A., Khan, W. U., Ali Shah, A., Yasin, N. A., Naz, S., Ali, A., et al. (2021). Synergistic effects of nitric oxide and silicon on promoting plant growth, oxidative stress tolerance and reduction of arsenic uptake in Brassica juncea. Chemosphere 262, 128384. doi: 10.1016/j.chemosphere.2020.128384
Aljbory, Z., Chen, M. S. (2018). Indirect plant defense against insect herbivores: A review. Insect Sci. 25, 2–23. doi: 10.1111/1744-7917.12436
Anstett, D. N., Chen, W., Johnson, M. T. (2016). Latitudinal gradients in induced and constitutive resistance against herbivores. J. Chem. Ecol. 42, 72–781. doi: 10.1007/s10886-016-0735-6
Bali, S., Jamwal, V. L., Kohli, S. K., Kaur, P., Tejpal, R., Bhalla, V., et al. (2019). Jasmonic acid application triggers detoxification of lead (Pb) toxicity in tomato through the modifications of secondary metabolites and gene expression. Chemosphere 235, 734–748. doi: 10.1016/j.chemosphere.2019.06.188
Bao, M., Li, J., Chen, H., Chen, Z. W., Xu, D. M., Wen, Y. Z. (2021). Enantioselective effects of imazethapyr on the secondary metabolites and nutritional value of wheat seedlings. Sci. Total Environ. 757, 143759. doi: 10.1016/j.scitotenv.2020.143759
Blokhina, O., Virolainen, E., Fagerstedt, K. V. (2003). Antioxidants, oxidative damage and oxygen deprivation stress: A review. Ann. Bot. 2, 179–194. doi: 10.1093/aob/mcf118
Bosch, M., Wright, L. P., Gershenzon, J., Wasternack, C., Hause, B., Schaller, A., et al. (2014). Jasmonic acid and its precursor 12-oxophytodienoic acid control different aspects of constitutive and induced herbivore defenses in tomato. Plant Physiol. 166, 396–410. doi: 10.1104/pp.114.237388
Carroll, M. J., Schmelz, E. A., Meagher, R. L., Teal, P. E. (2006). Attraction of Spodoptera frugiperda larvae to volatiles from herbivore-damaged maize seedlings. J. Chem. Ecol. 32, 1911–1924. doi: 10.1007/s10886-006-9117-9
Cass, C. L., Peraldi, A., Dowd, P. F., Mottiar, Y., Santoro, N., Karlen, S. D., et al. (2015). Effects of PHENYLALANINE AMMONIA LYASE (PAL) knockdown on cell wall composition, biomass digestibility, and biotic and abiotic stress responses in Brachypodium. J. Exp. Bot. 66, 4317–4335. doi: 10.1093/jxb/erv269
Chapman, K. M., Marchi-Werle, L., Hunt, T. E., Heng-Moss, T. M., Louis, J. (2018). Abscisic and jasmonic acids contribute to soybean tolerance to the soybean aphid (Aphis glycines matsumura). Sci. Rep. 8, 15148. doi: 10.1038/s41598-018-33477-w
Choudhury, S., Panda, P., Sahoo, L., Panda, S. K. (2013). Reactive oxygen species signaling in plants under abiotic stress. Plant Signal Behav. 8 (4), e23681. doi: 10.4161/psb.23681
Costa, E. N., Martins, L. O., Reis, L. C., Fernandes, M. G., de Paula Quintão Scalon, S. (2020). Resistance of cowpea genotypes to Spodoptera frugiperda (Lepidoptera: Noctuidae) and its relationship to resistance-related enzymes. J. Econ. Entomol. 113, 2521–2529. doi: 10.1093/jee/toaa179
Dehghan, S., Sadeghi, M., Pöppel, A., Fischer, R., Lakes-Harlan, R., Kavousi, H. R., et al. (2014). Differential inductions of phenylalanine ammonia-lyase and chalcone synthase during wounding, salicylic acid treatment, and salinity stress in safflower, Carthamus tinctorius. Biosci. Rep. 34, e00114. doi: 10.1042/BSR20140026
De Lange, E. S., Laplanche, D., Guo, H., Xu, W., Vlimant, M., Erb, M., et al. (2020). Spodoptera frugiperda caterpillars suppress herbivore-induced volatile emissions in maize. J. Chem. Ecol. 46, 344–360. doi: 10.1007/s10886-020-01153-x
de Lima Toledo, C. A., da Silva Ponce, F., Oliveira, M. D., Aires, E. S., Seabra Júnior, S., Lima, G. P. P., et al. (2021). Change in the physiological and biochemical aspects of tomato caused by infestation by cryptic species of Bemisia tabaci MED and MEAM1. Insects 12, 1105. doi: 10.3390/insects12121105
Ding, P., Ding, Y. (2020). Stories of salicylic acid: A plant defense hormone. Trends Plant Sci. 25, 549–565. doi: 10.1016/j.tplants.2020.01.004
Divekar, P. A., Narayana, S., Divekar, B. A., Kumar, R., Gadratagi, B. G., Ray, A., et al. (2022). Plant secondary metabolites as defense tools against herbivores for sustainable crop protection. Int. J. Mol. Sci. 23, 2690. doi: 10.3390/ijms23052690
Dixit, G., Srivastava, A., Rai, K. M., Dubey, R. S., Srivastava, R., Verma, P. C. (2020). Distinct defensive activity of phenolics and phenylpropanoid pathway genes in different cotton varieties toward chewing pests. Plant Signal Behav. 15, 1747689. doi: 10.1080/15592324.2020.1747689
Eisenring, M., Glauser, G., Meissle, M., Romeis, J. (2018). Differential impact of herbivores from three feeding guilds on systemic secondary metabolite induction, phytohormone levels and plant-mediated herbivore interactions. J. Chem. Ecol. 44, 1178–1189. doi: 10.1007/s10886-018-1015-4
Erb, M., Meldau, S., Howe, G. A. (2012). Role of phytohormones in insect-specific plant reactions. Trends Plant Sci. 17, 250–259. doi: 10.1016/j.tplants.2012.01.003
Escobar-Bravo, R., Chen, G., Kim, H. K., Grosser, K., van Dam, N. M., Leiss, K. A., et al. (2019). Ultraviolet radiation exposure time and intensity modulate tomato resistance to herbivory through activation of jasmonic acid signaling. J. Exp. Bot. 70, 315–327. doi: 10.1093/jxb/ery347
Florencio-Ortiz, V., Novák, O., Casas, J. L. (2020). Phytohormone responses in pepper (Capsicum annuum l.) leaves under a high density of aphid infestation. Physiol. Plant 170, 519–527. doi: 10.1111/ppl.13188
Fürstenberg-Hägg, J., Zagrobelny, M., Bak, S. (2013). Plant defense against insect herbivores. Int. J. Mol. Sci. 14, 10242–10297. doi: 10.3390/ijms140510242
Gao, Z., Chen, Y., He, K., Guo, J., Wang, Z. (2021). Sublethal effects of the microbial-derived insecticide spinetoram on the growth and fecundity of the fall armyworm (Lepidoptera: Noctuidae). J. Econ. Entomol. 114, 1582–1587. doi: 10.1093/jee/toab123
Garlet, C. G., Moreira, R. P., Gubiani, P. D. S., Palharini, R. B., Farias, J. R., Bernardi, O. (2021). Fitness cost of chlorpyrifos resistance in Spodoptera frugiperda (Lepidoptera: Noctuidae) on different host plants. Environ. Entomol. 50, 898–908. doi: 10.1093/ee/nvab046
Ghorbel, M., Brini, F., Sharma, A., Landi, M. (2021). Role of jasmonic acid in plants: the molecular point of view. Plant Cell Rep. 40, 1471–1494. doi: 10.1007/s00299-021-02687-4
Guo, J., Guo, J., He, K., Bai, S., Zhang, T., Zhao, J., et al. (2017). Physiological responses induced by Ostrinia furnacalis (Lepidoptera: Crambidae) feeding in maize and their effects on O. furnacalis performance. J. Econ. Entomol. 110, 739–747. doi: 10.1093/jee/tox060
Guo, J., Qi, J., He, K., Wu, J., Bai, S., Zhang, T., et al. (2019). The asian corn borer Ostrinia furnacalis feeding increases the direct and indirect defence of mid-whorl stage commercial maize in the field. Plant Biotechnol. J. 17, 88–102. doi: 10.1111/pbi.12949
Guo, H., Sun, Y., Peng, X., Wang, Q., Harris, M., Ge, F. (2016). Up-regulation of abscisic acid signaling pathway facilitates aphid xylem absorption and osmoregulation under drought stress. J. Exp. Bot. 67, 681–693. doi: 10.1093/jxb/erv481
Gutiérrez-Rodríguez, E., Lieth, H. J., Jernstedt, J. A., Labavitch, J. M., Suslow, T. V., Cantwell, M. I. (2013). Texture, composition and anatomy of spinach leaves in relation to nitrogen fertilization. J. Sci. Food Agric. 93 (2), 227–237. doi: 10.1002/jsfa.5780
Hafeez, M., Li, X., Ullah, F., Zhang, Z., Zhang, J., Huang, J., et al. (2021). Behavioral and physiological plasticity provides insights into molecular based adaptation mechanism to strain shift in spodoptera frugiperda. Int. J. Mol. Sci. 22, 10284. doi: 10.3390/ijms221910284
Han, S. Y., Kitahata, N., Sekimata, K., Saito, T., Kobayashi, M., Nakashima, K., et al. (2004). A novel inhibitor of 9-cis-epoxycarotenoid dioxygenase in abscisic acid biosynthesis in higher plants. Plant Physiol. 135, 1574–1582. doi: 10.1104/pp.104.039511
Hanan, A., Basit, A., Nazir, T., Majeed, M. Z., Qiu, D. (2020). Anti-insect activity of a partially purified protein derived from the entomopathogenic fungus Lecanicillium lecanii (Zimmermann) and its putative role in a tomato defense mechanism against green peach aphid. J. Invertebr. Pathol. 170, 107282. doi: 10.1016/j.jip.2019.107282
Haq, I. U., Khurshid, A., Inayat, R., Kexin, Z., Changzhong, L., Ali, S., et al. (2021). Silicon-based induced resistance in maize against fall armyworm [Spodoptera frugiperda (Lepidoptera: Noctuidae)]. PloS One 16, e0259749. doi: 10.1371/journal.pone.0259749
He, J., Ren, Y. F., Chen, X. L., Chen, H. (2014). Protective roles of nitric oxide on seed germination and seedling growth of rice (Oryza sativa l.) under cadmium stress. Ecotoxicol. Environ. Saf. 108, 114–119. doi: 10.1016/j.ecoenv.2014.05.021
Howe, G. A., Major, I. T., Koo, A. J. (2018). Modularity in jasmonate signaling for multistress resilience. Annu. Rev. Plant Biol. 69, 387–415. doi: 10.1146/annurev-arplant-042817-040047
Jacques, F. L., Degrande, P. E., Gauer, E., Malaquias, J. B., Scoton, A. M. N. (2021). Intercropped bt and non-bt corn with ruzigrass (Urochloa ruziziensis) as a tool to resistance management of Spodoptera frugiperda (JE smith 1797) (Lepidoptera: Noctuidae). Pest Manag. Sci. 77, 3372–3381. doi: 10.1002/ps.6381
Jing, D. P., Guo, J. F., Jiang, Y. Y., Zhao, J. Z., Sethi, A., He, K. L., et al. (2020). Initial detections and spread of invasive Spodoptera frugiperda in China and comparisons with other noctuid larvae in cornfields using molecular techniques. Insect Sci. 27, 780–790. doi: 10.1111/1744-7917.12700
Koo, A. J., Howe, G. A. (2009). The wound hormone jasmonate. Phytochemistry 70, 571–1580. doi: 10.1016/j.phytochem.2009.07.018
Kovalikova, Z., Kubes, J., Skalicky, M., Kuchtickova, N., Maskova, L., Tuma, J., et al. (2019). Changes in content of polyphenols and ascorbic acid in leaves of white cabbage after pest infestation. Molecules 24, 2622. doi: 10.3390/molecules24142622
Ku, Y. S., Sintaha, M., Cheung, M. Y., Lam, H. M. (2018). Plant hormone signaling crosstalks between biotic and abiotic stress responses. Int. J. Mol. Sci. 19, 3206. doi: 10.3390/ijms19103206
Kudjordjie, E. N., Sapkota, R., Steffensen, S. K., Fomsgaard, I. S., Nicolaisen, M. (2019). Maize synthesized benzoxazinoids affect the host associated microbiome. Microbiome 7, 59. doi: 10.1186/s40168-019-0677-7
Lefevere, H., Bauters, L., Gheysen, G. (2020). Salicylic acid biosynthesis in plants. Front. Plant Sci. 11. doi: 10.3389/fpls.2020.00338
Li, H., Li, C., Deng, Y., Jiang, X., Lu, S. (2018). The pentatricopeptide repeat gene family in salvia miltiorrhiza: Genome-wide characterization and expression analysis. Molecules 23, 1364. doi: 10.3390/molecules23061364
Li, H., Zhou, Z., Hua, H., Ma, W. (2020). Comparative transcriptome analysis of defense response of rice to Nilaparvata lugens and Chilo suppressalis infestation. Int. J. Biol. Macromol. 163, 2270–2285. doi: 10.1016/j.ijbiomac.2020.09.105
Livak, K. J., Schmittgen, T. D. (2001). Analysis of relative gene expression data using real-time quantitative PCR and the 2(-delta delta C(T)) method. Methods 25, 402–408. doi: 10.1006/meth.2001.1262
Lv, D., Liu, X., Dong, Y., Yan, Z., Zhang, X., Wang, P., et al. (2021). Comparison of gut bacterial communities of fall armyworm (Spodoptera frugiperda) reared on different host plants. Int. J. Mol. Sci. 22, 11266. doi: 10.3390/ijms222011266
Mertens, D., Fernández de Bobadilla, M., Rusman, Q., Bloem, J., Douma, J. C., Poelman, E. H. (2021). Plant defence to sequential attack is adapted to prevalent herbivores. Nat. Plants 7, 1347–1353. doi: 10.1038/s41477-021-00999-7
Murad, N. F., Silva-Brandão, K. L., Brandão, M. M. (2021). Mechanisms behind polyphagia in a pest insect: Responses of Spodoptera frugiperda (J.E. smith) strains to preferential and alternative larval host plants assessed with gene regulatory networks. Biochim. Biophys. Acta Gene Regul. Mech. 1864, 194687. doi: 10.1016/j.bbagrm.2021.194687
Nadarajah, K. K. (2020). ROS homeostasis in abiotic stress tolerance in plants. Int. J. Mol. Sci. 21, 5208. doi: 10.3390/ijms21155208
Njaci, I., Ngugi-Dawit, A., Oduor, R. O., Kago, L., Williams, B., Hoang, L. T. M., et al. (2020). Comparative analysis delineates the transcriptional resistance mechanisms for pod borer resistance in the pigeonpea wild relative Cajanus scarabaeoides (L.) thouars. Int. J. Mol. Sci. 22, 309. doi: 10.3390/ijms22010309
Peng, J., Deng, X., Huang, J., Jia, S., Miao, X., Huang, Y. (2004). Role of salicylic acid in tomato defense against cotton bollworm, Helicoverpa armigera hubner. Z Naturforsch. C J. Biosci. 59, 856–862. doi: 10.1515/znc-2004-11-1215
Peterson, K., Cheremond, E., Brandvain, Y., Van Tassel, D., Murrell, E. (2022). Weight gain of Spodoptera frugiperda larvae (Lepidoptera: Noctuidae) on leaf and floral tissues of Silphium integrifolium (Asterales: Asteraceae) differs by plant genotype. Environ. Entomol. 51, 397–404. doi: 10.1093/ee/nvab146
Pieterse, C. M., van der Does, D., Zamioudis, C., Leon-Reyes, A., Van Wees, S. C. (2012). Hormonal modulation of plant immunity. Annu. Rev. Cell Dev. Biol. 28, 489–521. doi: 10.1146/annurev-cellbio-092910-154055
Quan, L. J., Zhang, B., Shi, W. W., Li, H. Y. (2008). Hydrogen peroxide in plants: A versatile molecule of the reactive oxygen species network. J. Integr. Plant Biol. 50 (1), 2–18. doi: 10.1111/j.1744-7909.2007.00599.x
Quandahor, P., Gou, Y., Lin, C., Coulter, J. A., Liu, C. (2021). Comparison of root tolerance to drought and aphid (Myzus persicae sulzer) resistance among different potato (Solanum tuberosum l.) cultivars. Sci. Rep. 11 (1), 628. doi: 10.1038/s41598-020-79766-1
Quandahor, P., Gou, Y., Lin, C., Mujitaba Dawuda, M., A Coulter, J., Liu, C. (2020). Phytohormone cross-talk synthesizes glycoalkaloids in potato (Solanum tuberosum l.) in response to aphid (Myzus persicae sulzer) infestation under drought stress. Insects 11, 724. doi: 10.3390/insects11110724
Santiago, R., Cao, A., Butrón, A., López-Malvar, A., Rodríguez, V. M., Sandoya, G. V., et al. (2017). Defensive changes in maize leaves induced by feeding of mediterranean corn borer larvae. BMC Plant Biol. 17, 44. doi: 10.1186/s12870-017-0991-9
Schweiger, R., Heise, A. M., Persicke, M., Müller, C. (2014). Interactions between the jasmonic and salicylic acid pathway modulate the plant metabolome and affect herbivores of different feeding types. Plant Cell Environ. 37, 1574–1585. doi: 10.1111/pce.12257
Shavit, R., Batyrshina, Z. S., Dotan, N., Tzin, V. (2018). Cereal aphids differently affect benzoxazinoid levels in durum wheat. PloS One 13, e0208103. doi: 10.1371/journal.pone.0208103
Singh, S., Kaur, I., Kariyat, R. (2021). The multifunctional roles of polyphenols in plant-herbivore interactions. Int. J. Mol. Sci. 22, 1442. doi: 10.3390/ijms22031442
Sirhindi, G., Mir, M. A., Abd-Allah, E. F., Ahmad, P., Gucel, S. (2016). Jasmonic acid modulates the physio-biochemical attributes, antioxidant enzyme activity, and gene expression in glycine max under nickel toxicity. Front. Plant Sci. 7. doi: 10.3389/fpls.2016.00591
Somegowda, M., Raghavendra, S., Sridhara, S., Rajeshwara, A. N., N Pramod, S., Shivashankar, S., et al. (2021). Defensive mechanisms in cucurbits against melon fly (Bactrocera cucurbitae) infestation through excessive production of defensive enzymes and antioxidants. Molecules 26, 6345. doi: 10.3390/molecules26216345
Tyagi, S., Shah, A., Karthik, K., Rathinam, M., Rai, V., Chaudhary, N., et al. (2022). Reactive oxygen species in plants: an invincible fulcrum for biotic stress mitigation. Appl. Microbiol. Biotechnol. 106 (18), 5945–5955. doi: 10.1007/s00253-022-12138-z
Tzin, V., Hojo, Y., Strickler, S. R., Bartsch, L. J., Archer, C. M., Ahern, K. R., et al. (2017). Rapid defense responses in maize leaves induced by Spodoptera exigua caterpillar feeding. J. Exp. Bot. 68, 4709–4723. doi: 10.1093/jxb/erx274
Wang, Y., Mostafa, S., Zeng, W., Jin, B. (2021). Function and mechanism of jasmonic acid in plant responses to abiotic and biotic stresses. Int. J. Mol. Sci. 22, 8568. doi: 10.3390/ijms22168568
War, A. R., Paulraj, M. G., Ahmad, T., Buhroo, A. A., Hussain, B., Ignacimuthu, S., et al. (2012). Mechanisms of plant defense against insect herbivores. Plant Signal Behav. 7, 1306–1320. doi: 10.4161/psb.21663
War, A. R., Sharma, H. C., Paulraj, M. G., War, M. Y., Ignacimuthu, S. (2011). Herbivore induced plant volatiles: Their role in plant defense for pest management. Plant Signal Behav. 6, 1973–1978. doi: 10.4161/psb.6.12.18053
Wouters, F. C., Blanchette, B., Gershenzon, J., Vassão, D. G. (2016). Plant defense and herbivore counter-defense: Benzoxazinoids and insect herbivores. Phytochem. Rev. 15, 1127–1151. doi: 10.1007/s11101-016-9481-1
Yactayo-Chang, J. P., Mendoza, J., Willms, S. D., Rering, C. C., Beck, J. J., Block, A. K. (2021). Zea mays volatiles that influence oviposition and feeding behaviors of Spodoptera frugiperda. J. Chem. Ecol. 47, 799–809. doi: 10.1007/s10886-021-01302-w
Yadav, V., Wang, Z., Wei, C., Amo, A., Ahmed, B., Yang, X., et al. (2020). Phenylpropanoid pathway engineering: An emerging approach towards plant defense. Pathogens 9, 312. doi: 10.3390/pathogens9040312
Yang, Y., Ahammed, G. J., Wu, C., Fan, S., Zhou, Y. (2015). Crosstalk among jasmonate, salicylate and ethylene signaling pathways in plant disease and immune responses. Curr. Protein Pept. Sci. 16, 450–461. doi: 10.2174/1389203716666150330141638
Yang, L., Gao, J., Zhang, Y., Tian, J., Sun, Y., Wang, C. (2020). RNA-Seq identification of candidate defense genes by analyzing Mythimna separata feeding-damage induced systemic resistance in balsas teosinte. Pest Manage. Sci. 76, 333–342. doi: 10.1002/ps.5519
Yang, J. N., Wei, J. N., Kang, L. (2021). Feeding of pea leafminer larvae simultaneously activates jasmonic and salicylic acid pathways in plants to release a terpenoid for indirect defense. Insect Sci. 28, 811–824. doi: 10.1111/1744-7917.12820
Zhang, P., Bai, J., Liu, Y., Meng, Y., Yang, Z., Liu, T. (2020). Drought resistance of ten ground cover seedling species during roof greening. PloS One 15, e0220598. doi: 10.1371/journal.pone.0220598
Zhang, J. J., Sun, L. L., Wang, Y. N., Xie, G. Y., An, S. H., Chen, W. B., et al. (2022). Serotonergic neurons in the brain and gnathal ganglion of larval Spodoptera frugiperda. Front. Neuroanat. 16. doi: 10.3389/fnana.2022.844171
Zhang, R., Zheng, F., Wei, S., Zhang, S., Li, G., Cao, P., et al. (2019). Evolution of disease defense genes and their regulators in plants. Int. J. Mol. Sci. 20, 335. doi: 10.3390/ijms20020335
Zhao, H., Sun, X., Xue, M., Zhang, X., Li, Q. (2016). Antioxidant enzyme responses induced by whiteflies in tobacco plants in defense against aphids: Catalase may play a dominant role. PloS One 11, e0165454. doi: 10.1371/journal.pone.0165454
Zhou, M., Memelink, J. (2016). Jasmonate-responsive transcription factors regulating plant secondary metabolism. Biotechnol. Adv. 34, 441–449. doi: 10.1016/j.biotechadv.2016.02.004
Zhou, S., Richter, A., Jander, G. (2018). Beyond defense: Multiple functions of benzoxazinoids in maize metabolism. Plant Cell Physiol. 59, 1528–1537. doi: 10.1093/pcp/pcy064
Zhu, L., Chen, M. S., Liu, X. (2011). Changes in phytohormones and fatty acids in wheat and rice seedlings in response to hessian fly (Diptera: Cecidomyiidae) infestation. J. Econ. Entomol. 104, 1384–1392. doi: 10.1603/EC10455
Zhu, M., Liu, Y., Cai, P., Duan, X., Sang, S., Qiu, Z. (2022). Jasmonic acid pretreatment improves salt tolerance of wheat by regulating hormones biosynthesis and antioxidant capacity. Front. Plant Sci. 13. doi: 10.3389/fpls.2022.968477
Zhu, C., Wang, W., Chen, Y., Zhao, Y., Zhang, S., Shi, F., et al. (2022). Transcriptomics and antioxidant analysis of two chinese chestnut (Castanea mollissima BL.) varieties provides new insights into the mechanisms of resistance to gall wasp Dryocosmus kuriphilus infestation. Front. Plant Sci. 13. doi: 10.3389/fpls.2022.874434
Keywords: Spodoptera frugiperda, plant defense, antioxidative enzyme, secondary metabolism, phytohormones
Citation: Yang J, Ma C, Jia R, Zhang H, Zhao Y, Yue H, Li H and Jiang X (2023) Different responses of two maize cultivars to Spodoptera frugiperda (Lepidoptera: Noctuidae) larvae infestation provide insights into their differences in resistance. Front. Plant Sci. 14:1065891. doi: 10.3389/fpls.2023.1065891
Received: 10 October 2022; Accepted: 20 January 2023;
Published: 10 February 2023.
Edited by:
Xiao Ming Zhang, Yunnan Agricultural University, ChinaReviewed by:
Rishikesh Ghogare, Texas A&M University, United StatesXiumin Fu, South China Botanical Garden (CAS), China
Copyright © 2023 Yang, Ma, Jia, Zhang, Zhao, Yue, Li and Jiang. This is an open-access article distributed under the terms of the Creative Commons Attribution License (CC BY). The use, distribution or reproduction in other forums is permitted, provided the original author(s) and the copyright owner(s) are credited and that the original publication in this journal is cited, in accordance with accepted academic practice. No use, distribution or reproduction is permitted which does not comply with these terms.
*Correspondence: Xuwen Jiang, bWp4dzg4OEAxNjMuY29t; Heqin Li, aHFsaWFhdUAxNjMuY29t