- 1Department of Microbiology, Central University of Haryana, Mahendergarh, India
- 2Department of Environmental Microbiology and Biotechnology, Institute of Microbiology, Faculty of Biology, University of Warsaw, Warsaw, Poland
Land that has little to no utility for agriculture or industry is considered marginal land. This kind of terrain is frequently found on the edge of deserts or other arid regions. The amount of land that can be used for agriculture continues to be constrained by increasing desertification, which is being caused by climate change and the deterioration of agriculturally marginal areas. Plants and associated microorganisms are used to remediate and enhance the soil quality of marginal land. They represent a low-cost and usually long-term solution for restoring soil fertility. Among various phytoremediation processes (viz., phytodegradation, phytoextraction, phytostabilization, phytovolatilization, phytofiltration, phytostimulation, and phytodesalination), the employment of a specific mechanism is determined by the state of the soil, the presence and concentration of contaminants, and the plant species involved. This review focuses on the key economically important plants used for phytoremediation, as well as the challenges to plant growth and phytoremediation capability with emphasis on the advantages and limits of plant growth in marginal land soil. Plant growth-promoting bacteria (PGPB) boost plant development and promote soil bioremediation by secreting a variety of metabolites and hormones, through nitrogen fixation, and by increasing other nutrients’ bioavailability through mineral solubilization. This review also emphasizes the role of PGPB under different abiotic stresses, including heavy-metal-contaminated land, high salinity environments, and organic contaminants. In our opinion, the improved soil fertility of marginal lands using PGPB with economically significant plants (e.g., Miscanthus) in dual precession technology will result in the reclamation of general agriculture as well as the restoration of native vegetation.
1 Introduction
Marginal lands are increasing at an alarming rate due to various anthropogenic activities. Marginal lands are defined as having inferior soil with inadequate agricultural attributes and crop yield that is occasionally polluted. The state of the world’s land and water resources for food and agriculture is associated with the condition of our productive land and ecosystems and reflects problematic trends in resource consumption (FAO, 2022). A rapid decline in natural and seminatural ecosystems over the last centuries and severe climatic changes along with increasing human pressure have led to more frequent extreme weather events, higher rates of land degradation, and potential habitat losses. Furthermore, excessive use of fertilizer impacts the health of soil negatively. Thus, remedial actions are needed to prevent land degradation, on which 98% of the world’s food is produced.
The degradation of land, soil, and water resources as a result of human activity diminishes their production potential, biodiversity, and environmental services that support healthy and resilient livelihoods. Energy crops planted in these regions can aid in land reclamation and significantly reduce greenhouse gas emissions (Zhuang et al., 2011; Schröder et al., 2018). Overgrazing (35%), intensive agriculture (28%), deforestation (30%), manufacturing fuel wood (7%), and industrialization (4%) are the main drivers of soil deterioration (Schröder et al., 2018). Land might be marginal for a variety of reasons, including a lack of water supply, low chemical and/or microbiological soil quality, pollution from previous industrial activity, topographic obstacles such as an extreme slope, or when inaccessible or very remote from transportation networks. Most marginal lands are also characterized by heavy metal (HM) contamination, organic pollutants, strong acidification or alkalization, high salinity, limited water, etc. (Jiang et al., 2021; Shahane and Shivay, 2021). According to Fan et al. (2020), for energy crop production, about 43.75 million hectares (Mha) of marginal land is available in South China, 11.36 Mha in the US, 1.4 Mha in the UK, and almost 45 Mha in Europe. On the other hand, Jiang et al. (2021) assessed the total marginal land worldwide suitable for the cultivation of the energy crop Pistacia chinensis using a machine learning method and reported that a total of 1311.85 Mha of marginal land is mostly distributed in Southern Africa, the southern part of North America, the western part of South America, Southeast Asia, Southern Europe, and the east and southwest coasts of Oceania.
Plant growth is adversely affected by increased salinity or HM concentration, too high or low pH, low water availability, and the presence of other contaminants. Wilting and abscission of leaves, decreased leaf regions, and decreased water loss through transpiration are all physiological responses to stress in plants. Reduced turgor pressure under stress is one of the most delicate physiological mechanisms that allows cells to develop in a stressed environment. Drought stress disrupts water passage from the xylem to surrounding elongating cells in higher plants resulting in cell elongation suppression. In addition, drought leads to a reduction in leaf area, plant height, and plant development as cell elongation, mitosis, and spreading are impaired. Osmotic modification, which supports the active accumulation of solutes in the cytoplasm to maintain the cell’s water balance, can reduce the negative effects of stress (Yadav et al., 2020).
Plants employ various mechanisms, such as escape, avoidance, and tolerance, to counteract various stresses, and many plants have been described for their soil remediation ability. Plants can remove or immobilize various pollutants from the soil by employing different phytoremediation strategies, such as phytodegradation, phytoextraction, phytostabilization, etc. (Yan et al., 2020). PGPB also helps to improve the remediation ability of plants by stimulating plant growth through the secretion of various types of metabolites and hormones, solubilizing minerals, fixing nitrogen, and protecting plants against pathogens. PGPB also helps in alleviating various other types of biotic and abiotic stresses faced by plants (Backer et al., 2018). In this review, various phytoremediation strategies and the role of PGPB in enhancing the plant’s potential for phytoremediation have been discussed. In addition, a number of plant growth-promoting activities of PGPB have also been reported.
2 Phytoremediation
Phytoremediation is a decontamination technique wherein plants, such as grasses, shrubs, and trees, help to clean up the environment by degrading, accumulating, or stabilizing the contaminants. Phytoremediation techniques generate very little secondary waste while removing contaminants from the environment in an environmentally friendly way and at a very low cost (Shah and Daverey, 2020).
2.1 Types of phytoremediation and their mechanisms
Based on the soil conditions, pollutants, and plant species, phytoremediation of HM-contaminated soils includes many methods and processes such as phytodegradation (phytotransformation), phytofiltration, phytoextraction (phytoaccumulation), phytostabilization, rhizodegradation, phytodesalination, and phytovolatilization, as shown in Figure 1 (Saleem et al., 2020b; Nedjimi, 2021; Sabreena et al., 2022). The most extensively employed phytoremediation methods in the remediation of HM-contaminated soils are phytostabilization, phytoextraction, phytovolatilization, and phytofiltration, whereas strategies like phytodegradation and rhizodegradation are used for the degradation of organic pollutants (Yan et al., 2020). The effectiveness of phytoremediation depends primarily on the plant species used for contaminant removal. Plant species with high metal tolerance and high extraction or immobilization ability are very effective and are usually used for phytoremediation (Visconti et al., 2022). There are several newly emerged phytoremediation techniques such as phytoremediation buffers, phytoremediation vegetation, phytoremediation caps, phytoremediation plantings, and percolation and phytoremediation (Riaz et al., 2022).
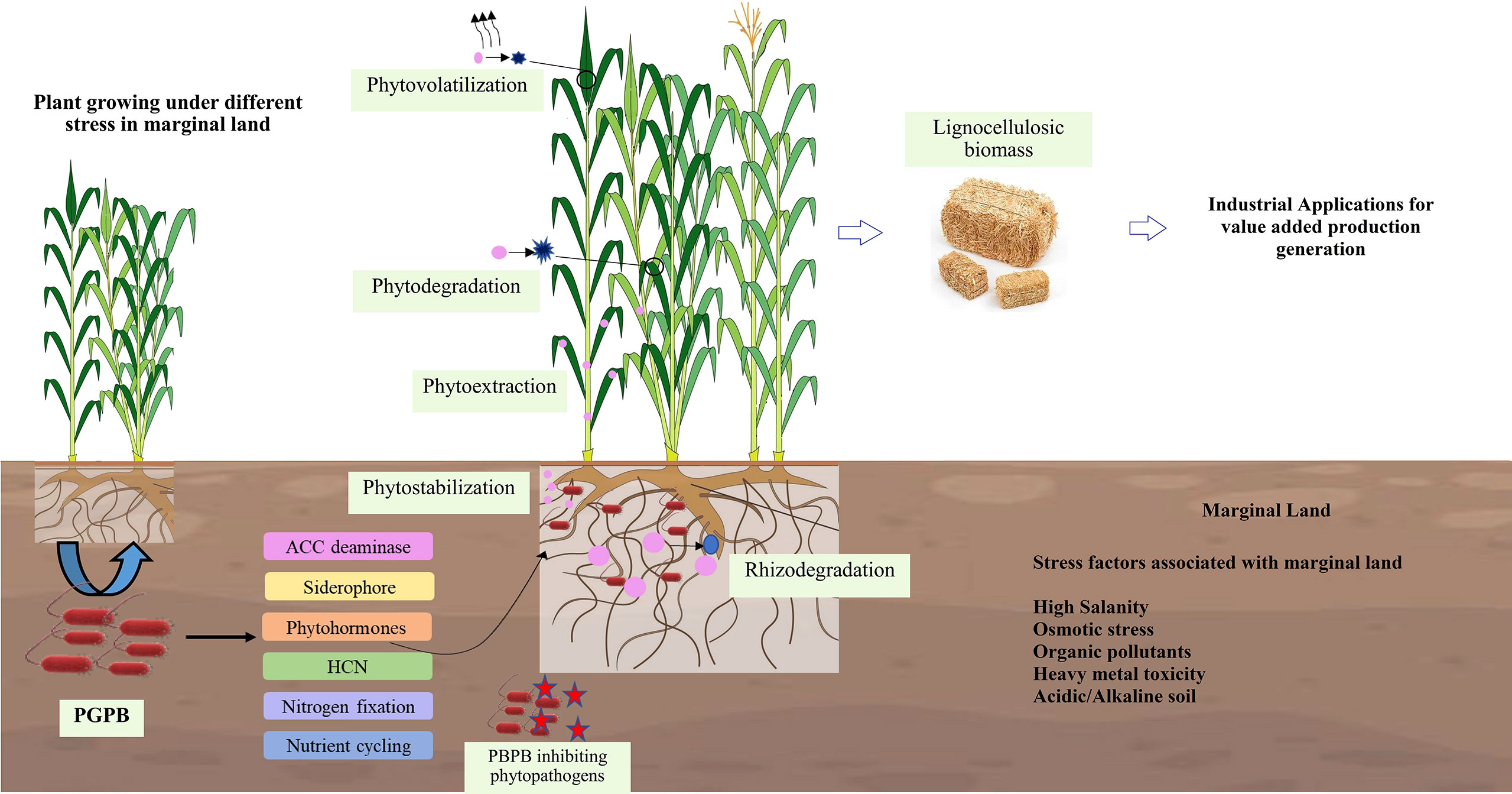
Figure 1 Phytoremediation methods and the role of PGPB in assisting plants for the remediation of marginal lands.
2.1.1 Phytostabilization or Phytoimmobilization
Phytostabilization is a cost-effective and less invasive phytotechnology that stabilizes hazardous contaminants such as HMs in the rhizosphere or roots by using tolerant plants that bind the contaminants in soil reducing their mobility within ecosystems and food chains (Shackira and Puthur, 2019). It involves a temporary accumulation of contaminants by plants in their belowground parts, which only immobilize and deactivate toxic metal ions instead of permanently removing them from the contaminated soil by forming metal complexes with root exudates, cell wall bonds, precipitates, or reducing HMs in the rhizosphere, which are sequestered in the vacuoles of root cells with the help of microorganisms (Zgorelec et al., 2020; Kafle et al., 2022). For effective phytostabilization, the selection of suitable plant species is very important. The plant should be HM tolerant and have a dense root system because roots play an important role in HM immobilization, stabilizing the soil structure, and preventing soil erosion (Shackira and Puthur, 2019; Zine et al., 2020). In addition, plant species and varieties characterized by low translocation factors are also highly desirable (Huang et al., 2021).
2.1.2 Phytodegradation
Phytodegradation is the degradation of contaminants taken up by plants through metabolic processes, or the degradation of pollutants outside the plant by enzymes (such as dehalogenases, nitro reductases, and peroxidases) released by roots (Jhilta et al., 2021; Nedjimi, 2021). Rhizosphere microorganisms perform most of the phytodegradation activities in a favorable rhizosphere environment. The ability of plants to modify the rhizospheric microbial community composition (Poria et al., 2021b) and microbial interaction within it has a significant impact on the degradation of pollutants. There are many reports which suggest that plants promote the degradation of contaminants by rhizospheric effects (Kotoky and Pandey, 2020a; Kotoky and Pandey, 2020b; Nebeská et al., 2021).
2.1.3 Phytoextraction or phytoaccumulation
Contaminants from soil or water are taken up by plants and permanently accumulated in above-ground biomass through translocation during phytoextraction or phytoaccumulation (Ali et al., 2020). Phytoextraction involves several steps, starting with HM mobilization in the rhizosphere, followed by uptake by plant roots and translocation to above-ground parts of the plant. The final step is the immobilization and compartmentalization of HM ions in the aerial tissues of the plant (Yan et al., 2020).
2.1.4 Phytovolatilization
In this phytoremediation strategy, plants release volatile forms of HMs by transpiration through the leaves after absorbing HMs from the soil and converting them to less toxic volatile forms (Bortoloti and Baron, 2022). Compared to other phytoremediation strategies, phytovolatilization is more beneficial as it removes contaminants without harvesting and disposing of the plants and converts them to gaseous compounds (Yan et al., 2020).
2.1.5 Phytofiltration
In this strategy, reclamation of soil and water with a low content of contaminants is carried out using seedlings (blastofiltration), shoots (caulofiltration), or roots (rhizofiltration). In rhizofiltration, HMs are either adsorbed on the root surface or absorbed by the roots. Root exudates can alter the pH of rhizosphere, resulting in HM precipitation at plant roots and a reduction in HM transport to subsurface water. Plants used for rhizofiltration are first cultivated in clean water (hydroponics) to establish an extensive root system, and then contaminated water is utilized to acclimate the plants. These plants are then transferred to the contaminated site for HM removal. The roots are removed and discarded once they become soaked with HMs (Ashraf et al., 2019).
2.1.6 Rhizodegradation or phytostimulation
Rhizodegradation is the biodegradation of organic pollutants in the soil, which involves the secretion of certain enzymes by rhizospheric microorganisms that degrade or convert heavily polluted organic pollutants into less harmful substances. The rhizodegradation process is accelerated by microbes that take up nutrients (amino acids, carbohydrates, etc.) from the root secretions of the plant, increasing its efficiency and accelerating the extraction and removal of contaminants (Ashraf et al., 2019; Sabreena et al., 2022). Dissolution of the pollutant at the source is a key element of rhizodegradation, which focuses on the complete mineralization of the organic pollutant by transferring it to the plant or atmosphere. Soil type and plant species has an effect on rhizodegradation. Bacillus, Burkholderia, Pseudomonas, Agrobacterium, Alcaligenes, Arthrobactor, and Micrococcus are bacteria frequently reported to be involved in rhizodegradation, as are mycorrhizal fungi such as Glomus (P.P and Puthur, 2021).
2.1.7 Phytodesalination
Phytodesalination is the most widely used method to decontaminate saline soils and uses halophytic plants. In comparison to other phytoremediation approaches, knowledge about this strategy is still poor. Halophytes are thought to be naturally well-adapted to live in HM- contaminated surroundings in contrast to most glycophyte plants. The plant’s ability to desalinate soil is determined by the species, as well as soil properties like salinity, sodicity, and porosity, as well as other climatic elements like rainfall (Sabreena et al., 2022).
Several new sustainable phytoremediation techniques are also being used for soil remediation. Such a technique can be used to remediate areas like brownfields, mine wastes, and landfill trash that have low to moderate levels of contamination. Known as phytoremediation buffers, the technique uses buffer strips in conjunction with riparian corridors where appropriate plant species are planted in the stripes along stream channels to remove toxins from surface water and groundwater flowing into rivers. This method is incredibly useful in preventing the further spread and mixing of water pollutants into groundwater supplies. Phytoremediation can result in contaminants entering the food chain, and some phytoremediation techniques do not completely remove contaminants from the environment. To reduce this risk of environmental and food contamination, another combined technique, percolation, along with phytoremediation, can be used to remove and percolate contaminants from the polluted soils. Using infiltration and percolation through vegetative covers, this technique is designed to remove soil pollutants. Phytoremediation caps also serve the purpose of reducing contaminant input to natural resources by reducing infiltration and precipitation and improving cap integrity. To reduce the spread of contaminants from their active hotspots, phytoremediation vegetation cover systems are used to establish barriers and prevent the further spread of contaminants. This is an economically feasible system because it is self-regenerating and can control soil erosion (Riaz et al., 2022)
2.2 Major economically important plants used for phytoremediation
Phytoremediation is a cost-effective and ecologically sound method of removing contaminants from soil and water. Adsorption, transport and translocation, hyperaccumulation or transformation, and mineralization are all processes that plants use to remove HMs from the environment (Pandey et al., 2015). Phytoremediation has already been documented for Festuca arundinacea, Hordeum vulgare, Thlaspi caerulescens, Linum usitatissimum, Pteris vittata, and Brassica juncea, among other economically important plants (Saleem et al., 2020a). Marginal lands are suitable for the growth of numerous energy crops, such as Arundo donax, Brassica juncea, Jatropha curcas, Miscanthus species, Ricinus communis, and Salix spp., which have been used to remediate polluted areas (P.P and Puthur, 2021).
Plants with a high degree of ecological adaptability and economic value are selected for their phytoremediation capacity so that, in addition to removing contaminants, they offer other social, environmental, and economic prospects, such as carbon sequestration, increasing soil’s organic biomass, promoting microbial biodiversity, biomass for value-added products such as bioenergy production, platform chemicals, fragrances and flavorings, construction and soundproofing materials for houses, and raw material for the paper pulp industry, etc. These plants, which include Saccharum munja, Saccharum spontaneum, Vetiveria zizanioides, Cymbopogon flexuosus, Ocimum basilicum, Ricinus communis, Jatropha curcas, Miscanthus giganteus (Pandey et al., 2015), Cyperus alternifolius, Amaranthus retroflexus, Celosia cristata, and Bambusa vulgaris, have been reported for their bioremediation and phytodesalination activity (Ameri Siahouei et al., 2020). A list of some economically important plants for phytoremediation is presented in Table 1.
2.3 Factors limiting plant growth and phytoremediation potential
Phytoremediation is a potential method for removing contaminants from soil, but it has several drawbacks. Decontamination using plants is a time-consuming process due to its complete dependence upon the type and amount of particular contaminants in soil and the plant variety. The majority of plants with phytoremediation potential are slow growing and have lower biomass, limiting their efficacy. Some pollutants are found in soil in a very tightly bound state, limiting their bioavailability and making mobilization difficult. Because plants are not tolerant to extremely high levels of pollutants, phytoremediation is only successful in low-to- moderately contaminated soil/water. Moreover, other soil properties like pH, clay content, and organic matter also limit phytoremediation efficiency (Jaskulak et al., 2020). Effective phytoremediation also necessitates favorable weather and climatic conditions for plants. Storage, handling, and correct disposal of metal-derived phyto-biomass are also difficult due to the constraints mentioned above. Compaction and composting of phyto-biomass reduce bulk and transportation costs while increasing dissolved metal-organic compound leaching. As a result, a key concern with phytoremediation is the long-term disposal of phyto-biomass (Shah and Daverey, 2020). Because plant roots can only uptake contaminants in their immediate proximity and cannot reach deep layers of soil, phytoremediation has a major drawback: It only removes a fraction of the contaminants from the soil. Furthermore, soil may contain many contaminants at the same time, and the plants used to remediate the soil may not be tolerant or hyperaccumulators for all of the chemicals, rendering the process less effective (Pathak et al., 2020).
These challenges can be overcome by increasing the phytoremediation potential of plants by using various breeding techniques to improve their stress tolerance. Plants contain many genes responsible for stress tolerance to metals and are involved in the uptake and transport of these metals. Breeding techniques transfer these genes to increase the potential of plants to thrive under metal stress conditions. Using these techniques, many resistant varieties have been bred such as Parang 401 (Fe chlorosis resistant rice), BRRI-29, BR-26, and BR-14 (As tolerant commercial rice), Pirsabak 2004 (Pb tolerant wheat variety), Micro-Tom (Cd tolerant tomato), and Strongfield (Cd tolerant wheat variety). It has also been reported that complementary interaction of different groups of non-allelic genes provides stress tolerance. In rice, the complementary interaction of two groups of non-allelic genes (Ic1 and Ic2; Ic3 and Ic4) is responsible for Fe uptake. Ic1 is complementary with Ic3 and Ic2 with Ic4 and shows tolerance to Fe chlorosis (Elango et al., 2022). Overexpression of these genes may also contribute to increasing the phytoremediation efficiency of various plants. Heavy metal ATPases such as HMA3 and HMA4 are also responsible for the accumulation and tolerance of Zn and Cd. Overexpression of heavy metal ATPase 3 (TcHMA3) in Noccaea caerulea improved tolerance to Zn and Cd (Sorour et al., 2022).
Another alternative to overcome these challenges is the use of different additives that can help to increase the phytoremediation efficiency of different plants. In one study, different organic fertilizers, including leonardite, bone meal, vermicompost, chicken manure, and bat manure, were employed individually and in combination to test their inoculation effect on phytoremediation efficiency of Acacia mangium, Jatropha curcas, and Manihot esculenta. Acacia mangium and Manihot esculenta reportedly grew better when treated with bone meal and bat dung and with leonardite and bat dung amendments, respectively, while Jatropha curcas reportedly grew better when treated with bone meal, which improved the phytoremediation properties of Cd (Taeprayoon et al., 2022). Biochar can immobilize heavy metals and, therefore, can be used to remediate contaminated soil. Under non-flooded conditions, bamboo biochar boosted Cd and Zn transport from roots to aboveground parts by 68.85% and 102.27%, respectively, compared to no BBC amendment (Li et al., 2022). The use of multi-stress tolerant PGPB with high bioremediation ability against various contaminants in combination with different plants can also be explored.
3 PGPB and their role in mitigating abiotic stresses
Agricultural productivity is affected by various environmental stress factors, which are divided into abiotic and biotic stresses. Abiotic stress contributes to a 50% loss in productivity and biotic stress to 30% (Kumar and Verma, 2018). Salinity, drought, flooding, HM contamination, extreme temperatures, and pH are major abiotic stress factors. PGPBs are well-known for their ability to mitigate the negative effects of stress on plants by influencing processes related to their stress response. In the natural environment, plants coexist with various microorganisms (microbiota), belonging to different domains and kingdoms: Archaea, bacteria, fungi, other eukaryotes, and viruses. The rhizosphere provides nutrients and ecological niches for the growth of microbiota, while microorganisms (PGPB) provide beneficial compounds, including phytohormones, which defend plants from diseases and pathogens (Schirawski and Perlin, 2018; Singh et al., 2019; Poria et al., 2021a). The application of PGPB formulations is beneficial for plant development and a way to transform damaged and uncultivable land into healthy soil (Gouda et al., 2018).
3.1 PGPB as a salinity tolerant agent
It is estimated that up to 33% of total agricultural land worldwide is salinized (Otlewska et al., 2020). The main mechanism which contributes to salinity tolerance in plants is the translocation of sodium to vacuoles, thus reducing the amount of sodium in the cytoplasm. Research shows that PGPB treatment is associated with increased expression of genes encoding the Salt Overly Sensitive 1 (SOS1) exchanger located in the plasma membrane and other genes connected with the SOS pathway. The main consequence of salinity stress is the increase in the production of phytohormones such as abscisic acid, salicylic acid, and ethylene, which are responsible for activating the signaling cascade of various genes that are involved in enhancing salt tolerance (Ramakrishna et al., 2020). Studies suggest that soil inoculation with specific PGPB strains (Bacillus aryabhattai H19-1 and B. mesonae H20-5) is linked to increased antioxidant enzyme activity, metabolism of abscisic acid, and proline accumulation, under salinity stress (Sung-Je et al., 2019).
Production of ACC deaminase by PGPB is the best-studied mechanism that contributes to salinity tolerance in plants. Ali et al. (2014) observed that in the presence of PGPB Pseudomonas spp., tomato plants under 165 mM of salt stress have shown a 2.5- to 3.5-fold increase in dry weight compared to uninoculated controls and treatments inoculated with Pseudomonas spp. mutants that were deficient in ACC deaminase. Another important mechanism to combat salinity stress is the production of exopolysaccharides (EPS) and biofilm formation. Inoculation of chickpea with Halomonas variabilis HT1 and Planococcus rifietoensis RT4 strains improved fresh weight by 153% and 177%, respectively, under a NaCl concentration of 100 mM (Qurashi and Sabri, 2012).
3.2 PGPB as a HM-tolerant agent
Approximately 20 Mha of land around the globe is affected by HM contamination (Liu et al., 2018). HMs occur naturally in the Earth’s crust, and their overuse in industrial production has resulted in the contamination of large areas of land (Liu et al., 2018). In plants and other biological organisms, contact with HMs can affect components of cellular organelles and important enzymes. PGPB can mitigate the effects of exposure to HMs in plants and increase biomass production (Tchounwou et al., 2012; Ma et al., 2016b). The HM-polluted environment can be restored for crop production by either eliminating it or converting it to a non-bioavailable form (Tak et al., 2013).
Microbial populations exposed to HMs develop the resistance for these HMs to survive. These PGPB may become tolerant to HMs due to their biological properties or use direct detoxification and, therefore, become resistant (Ledin, 2000). Such mechanisms developed by PGPB include metal-protein complex formation, biotransformation, methylation, and demethylation (Tak et al., 2013). For instance, Bacillus thuringiensis strain GDB - 1 enhanced the metal removal activities of Alnus firma by acting on Pb, Zn, As, Cd, Cu, and Ni or reducing their toxicity by accumulating those metals in the seedlings of Alnus firma (Babu et al., 2013). Another study showed that maize inoculated with Proteus mirabilis T2Cr and CrP450 reduced Cr toxicity and increased Cr tolerance. It was found that the fresh weight in maize increased by 114% compared to the uninoculated control (Islam et al., 2016). In one study, the metal tolerant PGPR Pseudomonas and Bacillus increased the hyperaccumulation capacity of Helianthus annuus L. and caused a 1.7–2.5-fold accumulation of Zn and Cd in Helianthus annuus L. shoots (Sorour et al., 2022). The uptake, localization, intracellular transport, and efflux of Zn are regulated by Zn-related transporters, which include the yellow stripe-like (YSL) transporter, the copper transporter (COPT/Ctr), the P1B-type heavy metal ATPase (HMA), the cation efflux (CE) transporter, the Zn- and Fe-regulated transporter-like protein (ZIP), and the Zn-induced facilitator1 (ZIF1) transporter family. ZNTs are zinc-resistant transporters and iron-resistant transporter-like protein (ZIP) family micronutrient transporters. These transporters can be targeted for improving the resistance of plants against heavy metals. Similarly, Arbuscular mycorrhiza fungi (AMF) increased the resistance of E. grandis to high-Zn stress by improving nutrient uptake and regulating Zn uptake at the gene transcription level. With AMF symbiosis under high-Zn conditions, 10 genes (ZNT:4, COPT/Ctr:2, YSL:3, CE:1) were upregulated, while 19 genes (ZNT:9, COPT/Ctr:2, YSL:3, ZIFL:4, CE:1) were downregulated (Wang et al., 2022).
3.3 PGPB as a drought-tolerant agent
Drought affects more than 160 Mha of rain-fed land used to grow cereals and legumes. Moreover, due to climate change, water deficiency will affect even more lands, as global warming is projected to increase by around 0.2 to 0.5 each decade in Asian regions and by up to 1.6 in South Africa (Berger et al., 2016).
Drought triggers a signaling pathway that accumulates reactive oxygen species (ROS) in plants, which can damage cell membranes, proteins, and DNA in the absence of detoxification mechanisms (Saikia et al., 2018; Yang and Guo, 2018). These mechanisms may be enzymatic involving enzymes such as catalase (CAT), peroxidase (POD), and superoxide dismutase (SOD), or non-enzymatic, relying on antioxidant compounds such as polyphenols and ascorbic acid (Tiepo et al., 2020). Inoculation with PGPB also enhances the antioxidant response of plants. For instance, inoculation of Urochloa ruziziensis leaves with Azosprillum brasilense increased the content of CAT and POD in plant tissues, which may have contributed to increased drought tolerance (Bulegon et al., 2016). PGPB can also help the plant endure drought stress by secreting exopolysaccharides (Sun et al., 2020) and by ACC deaminase synthesis. In one study, a consortium of three PGPB strains was shown to improve chlorophyll content in drought-stressed black gram plants by more than 100% and garden pea plants by up to 280% (Saikia et al., 2018).
4 PGPB’s role in phytoremediation
Phytoremediation is one of the few universal methods that can be used to treat almost all types of marginal land due to the diversity and high remedial ability of different plants (Thijs et al., 2017). However, in some marginal lands, especially with highly toxic contaminants, or other stressed environments, the efficiency of phytoremediation may be lower. One way to improve the effectiveness of phytoremediation is by the employment of microorganisms which can support the growth of plants under such stressed environments and contribute to the mobility of contaminants in soil (Yan et al., 2020). Soil bioaugmentation with an exogenous/endogenous pool of microbes or stimulations of the activities of autochthonic microbiota, which can establish beneficial relationships with plants, can improve the phytoremediation abilities of these plants (Baneshi et al., 2014; Ferrarini et al., 2021; Rabani et al., 2022). The role of different PGPBs in the phytoremediation of several contaminants is illustrated in Table 2.
4.1 PGPB enhanced plant growth on marginal land under HM contamination conditions
The microbial enhancement of the phytoremediation of marginal soils contaminated with HMs may be the result of (i) promoting the growth of plants (Saleem et al., 2019; Debiec-Andrzejewska et al., 2020; Zainab et al., 2021), (ii) increasing plant tolerance against the metal’s toxicity (Khan and Bano, 2018), (iii) increasing the solubility and mobility of HMs (Ferrarini et al., 2021), or (iv) biotransformation of metals to less toxic chemical compounds (Karthik et al., 2017; Debiec-Andrzejewska et al., 2020). Most PGPBs are characterized by more than one plant growth-promoting property; thus, enhancement in the phytoremediation efficiency of HM-contaminated areas is usually complex and is a result of the synergistic effect of various plant-beneficial properties of bacteria (Ahemad, 2015; Sansinenea, 2019; Debiec-Andrzejewska et al., 2020).
Various phytoremediation strategies are used depending on many factors, such as (i) type of HM contamination (one or many metals), (ii) its concentrations, as well as the (iii) availability of plants and microbes species in the contaminated areas (Ullah et al., 2015). Phytoextraction is a commonly used method for HM-contaminated environments. The efficiency of phytoextraction in highly contaminated areas depends largely on the presence of plants and bacteria with high resistance to HMs (Girolkar et al., 2021). PGPBs seem to be highly beneficial for the phytoextraction process since their presence contributes to the additional increase of plant tolerance to HMs. For example, three bacterial strains resistant to Cu, Burkholderia cepacia J62, Pseudomonas thivervalensis Y1-3-9, and Microbacterium oxydans JYC17, contributed significantly to an increase in the biomass of Brassica napus L. and the Cu enrichment in the above-ground part by up to 63.4%, 55.3%, and 63.4%, respectively, compared to uninoculated soil (Ren et al., 2019). In other studies, endophytic and Cd resistance Pseudomonas fluorescens Sasm05 promoted the growth of Sedum alfredi and significantly increased their ability to accumulate Cd by increasing production of IAA and by the upregulation of the gene expression responsible for the uptake and transport of Cd by plants (Chen et al., 2017a; Wu et al., 2020a). The IAA production by metal-resistant Pseudomonas libanensis TR1 and Pseudomonas reactans Ph3R3 enhanced plant growth and Cu and Zn accumulation by Brassica oxyrrhina under HMs and drought stress (Ma et al., 2016b). Lead-tolerant Bacillus altitudinis KP14 enhanced the biomass of Miscanthus x giganteus (MxG) by up to 77%, due to its ability to solubilize phosphorus and produce IAA, ACC deaminases, ammonia, siderophores, and hydrogen cyanide, as well as its high antifungal activity (Pranaw et al., 2020).
The role of microorganisms in phytoextraction was also confirmed in the case of Ni (Ma et al., 2011; Durand et al., 2016; Chen et al., 2017b), Pb (He et al., 2020; Konkolewska et al., 2020), Cd (He et al., 2020), Mn (Huang et al., 2020; Li et al., 2020), Cr (Ahemad, 2015), and As (Debiec-Andrzejewska et al., 2020). Soil bioaugmentation with nickel-resistant bacterium Psychrobacter sp. SRS8 resulted in significant growth promotion of R. communis and H. annuus and doubled nickel phytoextraction efficiency (Ma et al., 2010). PGPB activity increased Ni bioaccumulation in plant tissues by improving their Ni resistance (Chen et al., 2017b). The improved Solanum nigrum growth along with greater tolerance to Pb and Cd stress was observed after inoculation with Bacillus sp. QX8 and QX13 (He et al., 2020). Similarly, the inoculation of Bacillus cereus HM5 and B. thuringiensis HM7 increased the absorption of Mn by B. papyrifera by promoting plant root function maintenance and by reducing oxidative stress (Huang et al., 2020). Phytoextraction may also be facilitated by HM stress mitigation for plants by the activity of PGPB (Ahemad, 2015). This mechanism was confirmed in the case of Cr soil contamination (by the microbial Cr(IV)-reducing potential) (Ahemad, 2015) and As (microbial As(III)-oxidizing potential) (Debiec-Andrzejewska et al., 2020).
The choice of HM phytoremediation strategy also depends on the ability of plants to translocate the metal from roots to aboveground parts of plants. This is the so-called translocation factor (TF). Plants species with a TF above 1 are considered a good candidate for phytoextraction, but plants characterized with a TF below 1 are considered appropriate phytostabilizors (Ma et al., 2015). Phytostabilization is usually applied when the HM concentration in soil is relatively low and their potential release to other environments does not present too much risk. This phytoremediation method was used for the stabilization of Zn by Brassica juncea and Ricinus communis inoculated with Psychrobacter sp. SRS8 and Pseudomonas sp. A3R3 (Ma et al., 2015). Surprisingly, Fe and Ni in these plants-microbial partnerships were phytoextracted too. Another study revealed that Cd and Zn rhizoaccumulation by Sedum plumbizincicola enhanced by E6S strain homologous to Achromobacter piechaudii (Ma et al., 2016c). The main mechanism of the microbial enhancement of phytostabilization is the reduced solubility and/or mobility of HMs both in the soil and inside plants. Among these processes are adsorption, biosorption, bioaccumulation, biotransformation, (bio)precipitation and complexation of HMs, and alkalization of soil (Manoj et al., 2020). The key role in adsorption is played by anionic-charged EPS and extracellular membrane functional groups, which can adsorb cationic-charged metals (Wang et al., 2010; Karthik et al., 2017). Biosorption and bioaccumulation processes are directly correlated with passive or active transport to microbial cells, and after that, metals are intracellularly immobilized by precipitation, accumulation, sequestration, and/or transformation (Ma et al., 2016a). Microbes are also able to biotransform HMs, thus decreasing their mobility and bioavailability.
The last commonly used method for the phytoremediation of HM-contaminated lands is phytovolatilization. This method is used in the case of HMs that can be biotransformed to less toxic volatile compounds. Phytovolatilization is, therefore, mainly used to treat lands contaminated with As, Se, and Hg, and the efficiency of this method may be also strengthened by the activity of PGPB. Plant-beneficial strains Stenotrophomonas maltophilia and Agrobacterium sp. were useful in effective phytovolatilization of As during the investigation of phytoremediation technology with the use of Arundo donax L (Yan et al., 2020). The mechanism for As phytovolatilization depends on the As-speciation in soil, but generally, it relies on the intracellular reduction of As(V) to As(III) by plants, the addition of methyl groups to As(III), and then further reduction of the methylated form of As (Chen et al., 2017c). Assisting microorganisms in this process relies on an increase in the As uptake efficiency from the soil, which causes more As to phytovolatilize (Guarino et al., 2020). Microbial enhancement of phytovolatilization was also confirmed in the case of Se contamination. Phytovolatilization relies on the transformation of toxic Se (as selenate) to the less toxic dimethyl selenide gas (Pivetz, 2001). An example of the enhanced Se phytovolatilization was the activity of environmental isolates BJ2 and BJ15, which supported the uptake and evaporation of selenium by Brassica juncea L. It was shown that bacteria contributed to the high efficiency of volatilization and accumulation of Se in plant tissues, which were enhanced by 35% and 70%, respectively (De Souza et al., 1999). The microbial enhancement of Hg phytovolatilization efficiency is based on decreasing the toxicity of this element by reduction of organomercurials to Hg(II) (Matsui et al., 2016) or Hg(II) to less toxic and volatile Hg(0) (Silver and Hobman, 2007). Zea mays inoculation by endophytic bacteria Serratia marcescens BacI56 and Pseudomonas sp. BacI38 increased Hg volatilization by 47.16% and 62.42%, respectively (Mello et al., 2020). Despite the confirmed cases of microbial-assisted Hg phytovolatilization, knowledge about molecular and physiological aspects of this mechanism is still poor (Tiodar et al., 2021).
4.2 PGPB-enhanced plant growth on marginal land with high salinity
Marginal land can have high salt concentration due to a variety of reasons, and sources of this high salinity can be natural resulting from (i) salt accumulation over a long time, (ii) weathering of rocks and minerals that release soluble salts including chloride, sulfates, and carbonates of sodium, calcium, and magnesium, or (iii) deposition of oceanic salt carried by wind and rain (Mukhopadhyay and Maiti, 2010). Anthropogenic sources are the result of the (i) replacement of perennial vegetation with annual crops, (ii) inappropriate fertilization or soil irrigation with salt-rich water, or (iii) insufficient drainage systems (Mukhopadhyay and Maiti, 2010). High salinity in soil contributes to ion imbalance and hyperosmotic pressure, leading to oxidative stress in plants (Heydarian et al., 2016). High salt concentrations may also decrease the availability of essential nutrients in the soil which leads to a reduced uptake of some nutrients by the plants (Pankaj et al., 2019). High salinity environments have been shown to elevate ethylene concentration, which is a typical reaction of plants under stress conditions (Heydarian et al., 2016). PGPB helps plants to alleviate salinity stress in marginal lands by reducing ethylene precursors through the production of ACC-deaminase (Ali et al., 2014; Sofy et al., 2021) and the production of biofilms covering the surface of the roots, limiting the physical contact of roots with contaminants (Kasim et al., 2016). PGPB also increases water use efficiency by transpiration regulation, stomatal conductance, and reduction of reactive oxygen species concentrations in inoculated plants (Amaresan et al., 2020).
Application of ACC deaminase-producing Pseudomonas spp. on barley and oat for phytoremediation of marginal lands with high salinity resulted in promotion of the growth of barley and oats roots in saline soil by 200% and 50%, respectively, and contributed to an increase in the shoot’s biomass by 100%–150%. This significantly increased plant biomass was able to accumulate higher amounts of salt (Chang et al., 2014). Similarly, Pseudomonas putida KT2440 and Novosphingobium sp. HR1a promoted the growth of Citrus macrophylla. The strain HR1a contributed to increased accumulation of IAA in leaves, while the strain KT2440 inhibited root chloride and proline accumulation under salt stress (Vives-Peris et al., 2020). Spinach (Spinacia oleracea L) inoculated with halotolerant (Pseudomonas sp., Thalassobacillus sp., and Terribacillus sp.) and chitinolytic (Pseudomonas spp., Sanguibacter spp., Bacillus spp.) bacterial strains with high antifungal activity resulted in better plant growth and reduced salinity (Anees et al., 2020).
4.3 PGPB enhanced plant growth on marginal lands contaminated with organic compounds
Due to the strong hydrophobic characteristics of many organic contaminants, phytoextraction efficiency is usually lower than that of phytodegradation or phytostimulation (Schwitzguébel, 2017). Bacteria use the metabolites secreted by the plant as carbon sources to stimulate their biodegradation ability, which, in turn, reduces the stress effect of the contaminant and promotes the growth and development of plants (Girolkar et al., 2021).
Among PGPB useful in phytostimulation are highly resistant microbes to hydrocarbons, which can degrade organic contaminants aerobically. Particularly desirable are strains capable of degrading crude oil hydrocarbon, the most pervasive class of environmental organic contaminants worldwide (Ławniczak et al., 2020). The source of PGPB in phytostimulation methods is usually autochthonic microbiota, whose activity is stimulated by plants. Soil bioaugmentation with an exogenous pool of microorganisms as well as combined methods is also applied (Schwitzguébel, 2017; Girolkar et al., 2021).
Acinetobacter, Alcanivorax, Bacillus, Corynebacterium, Gordonia, Hahella, Immundisolibacter, Luteimonas, Marinobacter, Mycobacterium, Ochrobactrum, Pseudomonas, Rhodococcus, and Sphingomonas are some of the reported genera capable of degrading organic pollutants effectively (Kukla et al., 2014; Oliveira et al., 2014; Fatima et al., 2015; Pawlik et al., 2017). Despite the high diversity of these strains, the rhizosphere microorganisms are regarded as the most effective in hydrocarbon degradation (Ruley et al., 2020). Biosurfactant production by these bacterial strains helps in increasing the bioavailability of petroleum compounds, which plays an important role in biodegradation (Ławniczak et al., 2020).
The positive effect of PGPB was observed in the phytoremediation of Ni-pyrene-contaminated soil with the use of Scripus triqueter (Chen et al., 2017b). Although PGPB presence decreased the plant’s biomass, their activity facilitated the increase in the plant’s resistance to pyrene and promotion of pyrene degradation (Chen et al., 2017b). High efficiency of the phytostimulation was achieved in crude petroleum oil-contaminated soil with the use of Bassia scoparia supported by associated rhizosphere microorganisms (Moubasher et al., 2015). A similar effect of PGPB was observed in the case of petroleum hydrocarbon degradation by Italian ryegrass (Hussain et al., 2018), petroleum oily sludge phytoremediation with the use of legumes plant Cajanus cajan, and rhizospheric microorganisms (Allamin et al., 2020), and diesel contaminant degradation by Zea mays L. supported by alkane‐degrading bacterial strains (Ummara et al., 2021).
Phytostimulation may also be used as an additional strategy for the remediation of organic compounds on contaminated lands. For example, in the polychlorinated biphenyl (PCB) removal by switchgrass (Panicum virgatum), phytostimulation with the use of plant-beneficial bacteria Burkholderia xenovorans LB400 was an additional strategy to the main treatment mechanism – phytoextraction. In this case, a quite effective translocation of PCB was confirmed, but enhanced microbial activity in the rhizosphere was also observed (Liang et al., 2014).
Numerous biotechnological strategies that promote plant tolerance and metal accumulation, such as the modification of HM transporter genes and their uptake systems, along with the improvement of HM ligand production, can also be used to improve phytoremediation (Bhat et al., 2022). Overexpression of HM transporter gene in Arabidopsis thaliana (YCF1 gene) improved tolerance to and accumulation of Pb and Cd. Similarly, transgenic plants that overexpressed the Nicotiana tabacum NtCBP4 protein displayed enhanced Pb hypersensitivity and accumulation. HM-binding ligands, phytochelatins, glutathione, and cystine-rich peptides, such as metallothioneins, are utilized for HM detoxification. In peas, due to the overexpression of the metallothioneins gene PsMTA, significant Cu buildup in roots was detected. Similarly, transgenic Brassica juncea with overexpression of E. coli GSH synthetase gene showed improved Cd tolerance (Bhat et al., 2022).
5 Economic perspectives of bioremediation and marginal land utilization for biomass production
The global bioremediation market was valued at USD 105.68 billion in 2019 and is expected to grow at a compound annual growth rate (CAGR) of 15.5% to reach USD 334.70 billion by 2027. In light of contemporary green technologies to improve waste management, awareness of bioremediation is growing. Due to their affordability and ease of use, microbiological counterparts to bioremediation are being utilized more frequently than other environmentally friendly techniques. Growing urbanization causes land to become marginalized, or less suited for agricultural, in many regions of industrialized and developing countries. The phytoremediation segment held the majority of the market share in 2019. Plants are extensively used to kickstart the process and are driving the growth of the segment. In terms of market share, North America accounted for approximately 41.8% of the global bioremediation market in 2019. The global microbial bioremediation market is quite fragmented, with a few large and medium-sized market players accounting for most of the revenue. The major players are implementing a variety of strategies, including mergers and acquisitions, strategic agreements and contracts, and the development, testing, and deployment of more effective microbial bioremediation systems. As governments across the globe are trying to attempt to focus on environmental protection, the market for bioremediation technology and services is expected to grow significantly during the forecast period. The bioremediation market is expected to reach new heights by 2030. This is due to the above-mentioned factors as well as the tremendous growth in research and development activities throughout the world (Emergen Research, 2022).
The global microbial bioremediation market is segmented into bacteria, fungi, and archaea. Bacteria are the most used organisms in the bioremediation process. Table 3 shows the United States Environmental Protection Agency’s national contingency plan for planned bioremediation products (EPA, 2022).
Another economic aspect of bioremediation is the use of contaminated soil for the production of industrially important raw materials such as biomass while carrying out remediation. Global economic growth and industrialization require a continuous supply of energy, which dramatically decreases natural energy resources. Therefore, an intense search for alternative and renewable energy sources to meet the demands of an ever-evolving world is underway (Mehmood et al., 2017). The latest estimates show that about 19% of global energy demand is met by renewable sources, of which biomass accounts for 9.3% (Edrisi and Abhilash, 2016). Among the renewable energy sources currently available, plant biomass is regarded as one of the most promising (Mehmood et al., 2017). In comparison to fossil fuels, the primary benefit of biomass as an energy source is its beneficial effect on the global CO2 balance in the atmosphere, since more CO2 is absorbed by plants during photosynthesis than is emitted to the atmosphere during biofuel combustion (Baumgarten et al., 2017). Bioenergy derived from a plant’s biomass also has a considerable influence on the mitigation of various environmental problems; e.g., high-yielding plants may be used for the phytoremediation of contaminated soils. Thus, bioenergy plant cultures may constitute an additional possibility for marginal land restoration (Edrisi and Abhilash, 2016).
The availability of land for biofuel production is limited (arable lands cannot be considered due to the high demand for food production). More and more frequently, the utilization of marginal and degraded lands is a viable solution to meet the energy needs of the people (Pancaldi and Trindade, 2020). The total world surface area of marginal lands is estimated at 13.1 Gha, of which 152 Mha (14% of the total marginal land area in the world) are available only in China. It is also estimate, that out of this 152 Mha, 60% may be utilized for bioenergy crop plantation. The current bioenergy potential of plants growing on marginal lands may range from 30 to 1000 Exa-Joule (EJ; 1 EJ=1018 J) of primary energy per year (Haberl et al., 2010). Considering the imperatives of sustainable economic development, it is estimated that the bio-energy potential by 2050 will be in the range of 130 - 270 EJ per year (Hoogwijk et al., 2009).
Several bioenergy crops are characterized by having high resistance to stressful environmental conditions and achieving high biomass in poor soils without special fertilization or other agricultural inputs (Hood, 2016). The most promising energy crop is Miscanthus species, which is regarded as a second-generation biofuel plant capable of producing high amounts of biomass due to effective photosynthesis and high water and nutrient usage efficiencies (Lewandowski et al., 2019; Pidlisnyuk et al., 2020). Plants such as Agave spp., Cynara cardunculus (cardoon), Panicum virgatum L. (switchgrass), Phalaris arundinacea L. (reed canarygrass), Sida hermaphrodita (sida), Ulmus pumila (Siberian elm), Sorghum bicolor L. (sweet sorghum), and Salix spp. (willow) are also considered good candidates for bioenergy crops (Saballos, 2008; Soldatos, 2015; Mehmood et al., 2017). Some of them are more adaptive to changing environmental conditions, but all of them have shown high potential for effective biomass production (Soldatos, 2015; Mehmood et al., 2017). Table 4 shows some important bioenergy crops and their yields.
Despite the high potential and diversity of bioenergy crops, the cost of cultivation and biofuel production is one of the most important factors in deciding their successful commercialization (Mehmood et al., 2017). Current studies indicate that bioenergy produced from the plant’s biomass is still more costly than fossil fuel production; e.g., the cost of cellulosic ethanol is three times higher than the current price of gasoline on an energy equivalent basis (Carriquiry et al., 2011).
Economic analysis showed that the profitability of the cultivation of Miscanthus in Europe and the USA depended largely on uncertain assumptions such as environmental factors. Depending on environmental conditions like the amount of rainfall or temperature, Miscanthus yield may vary from 10–48 t dry matter per ha. The significant differences in yield have a direct influence on biomass prices, which may vary in the range of 48–134 €/t dry matter, and thus on the price of bioenergy, which increases in direct proportion. Depending on the species and environmental conditions, the lifespan of plants plays an important role in the profitability of Miscanthus cultures and is estimated at 10–20 years (Witzel and Finger, 2016).
In terms of cost, biomass production can depend on many factors, including the species of cultivated plants. For example, in the case of switchgrass, the biomass production cost ranged from $40–61 Mg to $53–74 Mg in Tennessee and Oklahoma in the USA, respectively. Furthermore, the target cost for the commercial production of alcohols from biomass was estimated at US$ 38 Mg. Modeling studies on the commercialization of switchgrass found that the US$ 11–27 Mg expenditure on switchgrass biomass production is justifiable if losses due to sedimentation and soil erosion caused by plant roots are reduced (Mehmood et al., 2017). Another example showed that the cost of the production of biomass in the case of giant reed was up to US$85 per ton, and this species was regarded as the most profitable among two other bioenergy plants, Miscanthus and switchgrass (Soldatos, 2015).
There are many barriers to growing energy crops on marginal lands. These include (i) the lack of solid and established markets for trade, (ii) the high cost of crop establishment and uncertainty about crop quality, (iii) unstable prices over the long term (Saballos, 2008; Soldatos, 2015; Mehmood et al., 2017; Pancaldi and Trindade, 2020). Farmers can receive direct or indirect support in the form of subsidies to make the cultivation of energy crops on marginal lands more lucrative in the long run. Unfortunately, the current level of support varies widely across countries and is often insufficient (Mehmood et al., 2017). The cultivation of energy crops on marginal lands to produce alternative fuels is a highly promising option keeping in view the increasing demand for fuel and the decline in arable land. To fully leverage the potential of bioenergy crops, we need to better understand the environmental impact of large-scale production of energy crops using marginal lands worldwide.
Two aspects influence the economics of phytoremediation: application potential and cost compared to traditional treatments (Wan et al., 2016). Using conventional methods involving excavation and filling or ex-situ cleaning of the soil would cost approximately €280,000 and € 680,000 per hectare, respectively, compared with the economic value of phytoremediation of €14,850 and €14,600 per hectare, respectively, as determined by hedonic price analysis and substitution costs. The conventional method generally reduces soil fertility because soil is either landfilled or washed away during the cleaning process (Lewandowski et al., 2006). The plants used for phytoremediation can be used for metal recovery, bioenergy production, packaging materials, and house and furniture construction. However, some phytoremediation techniques are not able to remove the contaminants from soil, such as phytostabilization, and in most of these techniques, the concentration of contaminants in the plant exceeds critical limits so that they can enter the food chain (Parveen et al., 2022). Therefore, contaminants such as HMs can be extracted from plants after remediation using various industrial techniques (phytomining), and high profits can be made due to the costly nature of certain HMs (Riaz et al., 2022).
6 Future research directions
Plants used in bioremediation can provide several benefits, including efficient utilization of polluted biomass to produce various value-added products like pigments, platform chemicals, etc., and energy production through cogeneration. Moreover, metals recovered from plant combustion can be utilized as raw materials in industrial processes. Several future areas of research are waiting to be explored. First is estimating the influence of various contaminants on each other’s dynamics and their toxicity to soil microbial populations in the presence of selective plant species such as Miscanthus sp. The use of genetically modified plants may provide additional benefits, but their use should be carefully considered on a case-by-case basis and not generalized. Special care should be taken to explore genetically modified bacteria supporting phytoremediation and improving soil health, organic carbon dynamics, and soil biodiversity. This allows the use of plants that previously could not be used in contaminated soils due to their high sensitivity to a particular metal. This is especially important with regard to plants that have other outstanding properties, such as very rapid growth. So far, the impact of climatic change on the dynamics of various contaminants in plants and associated microbial metabolites has been overlooked.
7 Conclusion
Plants and bacteria both provide phytoremediation of hazardous pollutants, but bacteria also protect plants from a variety of stresses (HM toxicity, osmotic stress, salinity stress, etc.) and promote plant growth through numerous PGP processes (hormones and siderophores secretion, mineral solubilization, nitrogen fixation, etc.). Despite decades of research, phytoremediation is still considered a relatively new option because there are few long-term field trials. Microorganisms play an important role in the phytoremediation of soils by directly degrading or biotransforming contaminants and indirectly improving the growth of phytoremediated plants. In particular, the study of bacteria that have evolved to be resistant to high metal/pollutant concentrations and their host linkages can add a new dimension to existing phytoremediation techniques. Soil inoculation with PGPB and metal-solubilizing microbes show a lot of potential for boosting hyperaccumulators’ health, biomass yield, and metal accumulation capabilities. Further research is needed on the effectiveness of microbe-assisted phytoremediation under abiotic stresses such as climate change-induced drought and salinity. Furthermore, more research is needed to better understand the interactions between microbes and their host plants in metal-contaminated ecosystems.
Author contributions
Conceptualization, KP. Writing—original draft preparation, VP, KD-A, AF, ML, and NA. Writing—review and editing, KP and SS. Supervision, KP. All authors have read and agreed to the published version of the manuscript.
Funding
This research was funded by the grant from the project “The Fly Ash as the Precursors of Functionalized Materials for Applications in Environmental Engineering, Civil Engineering and Agriculture” (no. POIR.04.04.00-00-14E6/18-00), carried out within the TEAM-NET programme of the Foundation for Polish Science co-financed by the European Union under the European regional development fund.
Acknowledgments
VP acknowledges the DBT-JRF fellowship (award letter no. DBTHRDPMU/JRF/BET-22/I/2022-23/45) provided by the Department of Biotechnology, Government of India.
Conflict of interest
The authors declare that the research was conducted in the absence of any commercial or financial relationships that could be construed as a potential conflict of interest.
Publisher’s note
All claims expressed in this article are solely those of the authors and do not necessarily represent those of their affiliated organizations, or those of the publisher, the editors and the reviewers. Any product that may be evaluated in this article, or claim that may be made by its manufacturer, is not guaranteed or endorsed by the publisher.
References
Abuzaid, A. S., Jahin, H. S., Asaad, A. A., Fadl, M. E., Abdelrahman, M., Scopa, A. (2021). Accumulation of potentially toxic metals in Egyptian alluvial soils, berseem clover (Trifolium alexandrinum l.), and groundwater after long-term wastewater irrigation. Agriculture 11. doi: 10.3390/agriculture11080713
Ahemad, M. (2015). Enhancing phytoremediation of chromium-stressed soils through plant-growth-promoting bacteria. J. Genet. Eng. Biotechnol. 13, 51–58. doi: 10.1016/j.jgeb.2015.02.001
Ahmed, R., Taha, Y.-E., Ali, H. M. A. (2021). Phytoremediation of crude oil contaminated soil by Acacia farnesiana l. willd. and spraying glutathione. Univ. Thi-Qar J. Sci. 8, 59–66. doi: 10.32792/utq/utjsci/v8/1/10
Ali, S., Abbas, Z., Rizwan, M., Zaheer, I. E., Yavaş, İ., Ünay, A., et al. (2020). Application of floating aquatic plants in phytoremediation of heavy metals polluted water: A review. Sustainability 12. doi: 10.3390/su12051927
Ali, S., Charles, T. C., Glick, B. R. (2014). Amelioration of high salinity stress damage by plant growth-promoting bacterial endophytes that contain ACC deaminase. Plant Physiol. Biochem. 80, 160–167. doi: 10.1016/j.plaphy.2014.04.003
Ali, A., Guo, D., Li, Y., Shaheen, S. M., Wahid, F., Antoniadis, V., et al. (2021). Streptomyces pactum addition to contaminated mining soils improved soil quality and enhanced metals phytoextraction by wheat in a green remediation trial. Chemosphere 273, 129692. doi: 10.1016/j.chemosphere.2021.129692
Allamin, I. A., Halmi, M. I. E., Yasid, N. A., Ahmad, S. A., Abdullah, S. R. S., Shukor, Y. (2020). Rhizodegradation of petroleum oily sludge-contaminated soil using Cajanus cajan increases the diversity of soil microbial community. Sci. Rep. 104094. doi: 10.1038/s41598-020-60668-1
Al Sbani, N. H., Abdullah, S. R. S., Idris, M., Hasan, H. A., Halmi, M. I. E., Jehawi, O. H., et al. (2021). PAH-degrading rhizobacteria of Lepironia articulata for phytoremediation enhancement. J. Water Process Eng. 39, 101688. doi: 10.1016/j.jwpe.2020.101688
Amaresan, N., Murugesan, S., Kumar, K., Sankaranarayanan, A. (2020). Microbial mitigation of stress response of food legumes. CRC Press Boca Raton, 300. doi: 10.1201/9781003028413
Ameri Siahouei, R., Zaeimdar, M., Moogouei, R., Jozi, S. A. (2020). Potential of Cyperus alternifolius, Amaranthus retroflexus, Closia cristata and Bambusa vulgaris to phytoremediate emerging contaminants and phytodesalination; insight to floating beds technology. Caspian J. Environ. Sci. 18, 309–317. doi: 10.22124/cjes.2020.4277
Anees, M., Qayyum, A., Jamil, M., Rehman, F. U., Abid, M., Malik, M. S., et al. (2020). Role of halotolerant and chitinolytic bacteria in phytoremediation of saline soil using spinach plant. Int. J. Phytoremediation 22, 653–661. doi: 10.1080/15226514.2019.1707160
Ashraf, S., Ali, Q., Zahir, Z. A., Ashraf, S., Asghar, H. N. (2019). Phytoremediation: Environmentally sustainable way for reclamation of heavy metal polluted soils. Ecotoxicol. Environ. Saf. 174, 714–727. doi: 10.1016/j.ecoenv.2019.02.068
Babu, A. G., Kim, J.-D., Oh, B.-T. (2013). Enhancement of heavy metal phytoremediation by Alnus firma with endophytic Bacillus thuringiensis GDB-1. J. Hazard. Mater. 250-251, 477–483. doi: 10.1016/j.jhazmat.2013.02.014
Backer, R., Rokem, J. S., Ilangumaran, G., Lamont, J., Praslickova, D., Ricci, E., et al. (2018). Plant growth-promoting rhizobacteria: Context, mechanisms of action, and roadmap to commercialization of biostimulants for sustainable agriculture. Front. Plant Sci. 9. doi: 10.3389/fpls.2018.01473
Baneshi, M. M., Rezaei Kalantary, R., Jonidi Jafari, A., Nasseri, S., Jaafarzadeh, N., Esrafili, A. (2014). Effect of bioaugmentation to enhance phytoremediation for removal of phenanthrene and pyrene from soil with Sorghum and Onobrychis sativa. J. Environ. Health Sci. Eng. 12, 24. doi: 10.1186/2052-336X-12-24
Baumgarten, W., Ivanina, V., Hanzhenko, O. (2017). “"Biomass production on marginal lands-catalogue of bioenergy crops",” in EGU general assembly conference abstracts Germany: European Geosciences Union, 7904.
Berger, J., Palta, J., Vadez, V. (2016). Review: An integrated framework for crop adaptation to dry environments: Responses to transient and terminal drought. Plant Sci. 253, 58–67. doi: 10.1016/j.plantsci.2016.09.007
Bernal, M. P., Grippi, D., Clemente, R. (2021). Potential of the biomass of plants grown in trace element-contaminated soils under Mediterranean climatic conditions for bioenergy production. Agronomy 11. doi: 10.3390/agronomy11091750
Bhat, S. A., Bashir, O., Ul Haq, S. A., Amin, T., Rafiq, A., Ali, M., et al. (2022). Phytoremediation of heavy metals in soil and water: An eco-friendly, sustainable and multidisciplinary approach. Chemosphere 303, 134788. doi: 10.1016/j.chemosphere.2022.134788
Bortoloti, G. A., Baron, D. (2022). Phytoremediation of toxic heavy metals by Brassica plants: A biochemical and physiological approach. Environ. Adv. 8, 100204. doi: 10.1016/j.envadv.2022.100204
Bulegon, L., Guimarães, V., Laureth, J. (2016). Azospirillum brasilense affects the antioxidant activity and leaf pigment content of Urochloa ruziziensis under water stress. Pesquisa Agropecuaria Trop. 46, 343–349. doi: 10.1590/1983-40632016v4641489
Carriquiry, M. A., Du, X., Timilsina, G. R. (2011). Second generation biofuels: Economics and policies. Energy Policy 39, 4222–4234. doi: 10.1016/j.enpol.2011.04.036
Chang, P., Gerhardt, K. E., Huang, X.-D., Yu, X.-M., Glick, B. R., Gerwing, P. D., et al. (2014). Plant growth-promoting bacteria facilitate the growth of barley and oats in salt-impacted soil: Implications for phytoremediation of saline soils. Int. J. Phytoremediation 16, 1133–1147. doi: 10.1080/15226514.2013.821447
Cheng, S.-F., Huang, C.-Y., Chen, K.-L., Lin, S.-C., Lin, Y.-C. (2016). Phytoattenuation of lead-contaminated agricultural land using Miscanthus floridulus–an in situ case study. Desalination Water Treat 57, 7773–7779. doi: 10.1080/19443994.2015.1033477
Chen, Y., Han, Y.-H., Cao, Y., Zhu, Y.-G., Rathinasabapathi, B., Ma, L. Q. (2017c). Arsenic transport in rice and biological solutions to reduce arsenic risk from rice. Front. Plant Sci. 8. doi: 10.3389/fpls.2017.00268
Chen, X., Liu, X., Zhang, X., Cao, L., Hu, X. (2017b). Phytoremediation effect of Scirpus triqueter inoculated plant-growth-promoting bacteria (PGPB) on different fractions of pyrene and Ni in co-contaminated soils. J. Hazard. Mater. 325, 319–326. doi: 10.1016/j.jhazmat.2016.12.009
Chen, B., Luo, S., Wu, Y., Ye, J., Wang, Q., Xu, X., et al. (2017a). The effects of the endophytic bacterium Pseudomonas fluorescens Sasm05 and IAA on the plant growth and cadmium uptake of Sedum alfredii hance. Front. Microbiol. 8. doi: 10.3389/fmicb.2017.02538
Debiec-Andrzejewska, K., Krucon, T., Piatkowska, K., Drewniak, L. (2020). Enhancing the plants growth and arsenic uptake from soil using arsenite-oxidizing bacteria. Environ. Pollut. 264, 114692. doi: 10.1016/j.envpol.2020.114692
De Souza, M. P., Chu, D., Zhao, M., Zayed, A. M., Ruzin, S. E., Schichnes, D., et al. (1999). Rhizosphere bacteria enhance selenium accumulation and volatilization by Indian Mustard1. Plant Physiol. 119, 565–574. doi: 10.1104/pp.119.2.565
Dhaliwal, S. S., Taneja, P. K., Singh, J., Bhatti, S. S., Singh, R. (2020). Cadmium accumulation potential of Brassica species grown in metal spiked loamy sand soil. Soil Sediment Contam.: Int. J. 29, 638–649. doi: 10.1080/15320383.2020.1758031
Dominguez, J. J. A., Inoue, C., Chien, M.-F. (2020). Hydroponic approach to assess rhizodegradation by sudangrass (Sorghum x drummondii) reveals pH- and plant age-dependent variability in bacterial degradation of polycyclic aromatic hydrocarbons (PAHs). J. Hazard. Mater. 387, 121695. doi: 10.1016/j.jhazmat.2019.121695
Dos Santos, N. M. C., Da Costa, V., De Araújo, F. V., Alencar, B. T. B., Ribeiro, V. H. V., Okumura, F., et al. (2018). Phytoremediation of Brazilian tree species in soils contaminated by herbicides. Environ. Sci. Pollut. Res. 25, 27561–27568. doi: 10.1007/s11356-018-2798-0
Durand, A., Piutti, S., Rue, M., Morel, J. L., Echevarria, G., Benizri, E. (2016). Improving nickel phytoextraction by co-cropping hyperaccumulator plants inoculated by plant growth promoting rhizobacteria. Plant Soil 399, 179–192. doi: 10.1007/s11104-015-2691-2
Edrisi, S. A., Abhilash, P. C. (2016). Exploring marginal and degraded lands for biomass and bioenergy production: An Indian scenario. Renewable Sustain. Energy Rev. 54, 1537–1551. doi: 10.1016/j.rser.2015.10.050
Eissa, M. A. (2019). Effect of compost and biochar on heavy metals phytostabilization by the halophytic plant old man saltbush [Atriplex nummularia lindl]. Soil Sediment Contam.: Int. J. 28, 135–147. doi: 10.1080/15320383.2018.1551325
Elango, D., Devi, K. D., Jeyabalakrishnan, H. K., Rajendran, K., Thoomatti Haridass, V. K., Dharmaraj, D., et al. (2022). Agronomic, breeding, and biotechnological interventions to mitigate heavy metal toxicity problems in agriculture. J. Agric. Food Res. 10, 100374. doi: 10.1016/j.jafr.2022.100374
Emergen Research (2022) Microbial bioremediation market, by pollutants (Organic pollutants and inorganic pollutants), by organisms, by type, by technology, by application, by end-use, and by region forecast to 2030. Available at: https://www.emergenresearch.com/industry-report/microbial-bioremediation-market (Accessed 15th September 2022).
EPA (2022), 260–2342. U.S. Environmental Protection Agency National Contingency Plan Product Schedule. NCP Product Schedule Manager, U.S. Environmental Protection Agency, Office of Land and Emergency Management (OLEM), Office of Emergency Management (OEM) and Regulations Implementation Division (RID). NCP Subpart J Information Line, at (202).
Fan, P., Hao, M., Ding, F., Jiang, D., Dong, D. (2020). Quantifying global potential marginal land resources for switchgrass. Energies 13. doi: 10.3390/en13236197
FAO (2022). The state of the world’s land and water resources for food and agriculture – systems at breaking point (Rome, Italy: FAO). doi: 10.4060/cb9910en
Fatima, K., Afzal, M., Imran, A., Khan, Q. M. (2015). Bacterial rhizosphere and endosphere populations associated with grasses and trees to be used for phytoremediation of crude oil contaminated soil. Bull. Environ. Contam. Toxicol. 94, 314–320. doi: 10.1007/s00128-015-1489-5
Ferrarini, A., Fracasso, A., Spini, G., Fornasier, F., Taskin, E., Fontanella, M. C., et al. (2021). Bioaugmented phytoremediation of metal-contaminated soils and sediments by hemp and giant reed. Front. Microbiol. 12. doi: 10.3389/fmicb.2021.645893
Ghazaryan, K. A., Movsesyan, H. S., Khachatryan, H. E., Ghazaryan, N. P., Minkina, T. M., Sushkova, S. N., et al. (2018). Copper phytoextraction and phytostabilization potential of wild plant species growing in the mine polluted areas of Armenia. Geochemist.: Exploration Environ. Anal. 19, 155–163. doi: 10.1144/geochem2018-035
Girolkar, S., Thawale, P., Juwarkar, A. (2021). “"Chapter 12 - bacteria-assisted phytoremediation of heavy metals and organic pollutants: challenges and future prospects,",” in Bioremediation for environmental sustainability. Eds. Kumar, V., Saxena, G., Shah, M. P. (USA: Elsevier), 247–267. doi: 10.1016/B978-0-12-820318-7.00012-5
Gouda, S., Kerry, R. G., Das, G., Paramithiotis, S., Shin, H.-S., Patra, J. K. (2018). Revitalization of plant growth promoting rhizobacteria for sustainable development in agriculture. Microbiol. Res. 206, 131–140. doi: 10.1016/j.micres.2017.08.016
Guarino, F., Miranda, A., Castiglione, S., Cicatelli, A. (2020). Arsenic phytovolatilization and epigenetic modifications in Arundo donax l. assisted by a PGPR consortium. Chemosphere 251, 126310. doi: 10.1016/j.chemosphere.2020.126310
Haberl, H., Beringer, T., Bhattacharya, S. C., Erb, K.-H., Hoogwijk, M. (2010). The global technical potential of bio-energy in 2050 considering sustainability constraints. Curr. Opin. Environ. Sustain. 2, 394–403. doi: 10.1016/j.cosust.2010.10.007
Hamidpour, M., Nemati, H., Abbaszadeh Dahaji, P., Roosta, H. R. (2020). Effects of plant growth-promoting bacteria on EDTA-assisted phytostabilization of heavy metals in a contaminated calcareous soil. Environ. Geochemist. Health 42, 2535–2545. doi: 10.1007/s10653-019-00422-3
He, X., Xu, M., Wei, Q., Tang, M., Guan, L., Lou, L., et al. (2020). Promotion of growth and phytoextraction of cadmium and lead in Solanum nigrum l. mediated by plant-growth-promoting rhizobacteria. Ecotoxicol. Environ. Saf. 205, 111333. doi: 10.1016/j.ecoenv.2020.111333
Heydarian, Z., Yu, M., Gruber, M., Glick, B. R., Zhou, R., Hegedus, D. D. (2016). Inoculation of soil with plant growth promoting bacteria producing 1-Aminocyclopropane-1-Carboxylate deaminase or expression of the corresponding acdS gene in transgenic plants increases salinity tolerance in Camelina sativa. Front. Microbiol. 7. doi: 10.3389/fmicb.2016.01966
Hood, E. E. (2016). Plant-based biofuels. F1000Research 5, Rev–1185. doi: 10.12688/f1000research.7418.1
Hoogwijk, M., Faaij, A., De Vries, B., Turkenburg, W. (2009). Exploration of regional and global cost–supply curves of biomass energy from short-rotation crops at abandoned cropland and rest land under four IPCC SRES land-use scenarios. Biomass Bioenergy 33, 26–43. doi: 10.1016/j.biombioe.2008.04.005
Hoseini, S., Moogouei, R., Borghei, M., Abedi, Z., Ramezani, M. (2022). Potential of wheat (Triticum aestivum) and quinoa (Chenopodium quinoa) for phytodesalination of calcium, magnesium, sodium, potassium and chlorine under different water salinity stresses. J. Iranian Plant Ecophysiol. Res. 17, 38–53. doi: 10.30495/iper.2022.690262
Huang, W. X., Chen, X. W., Wu, L., Yu, Z. S., Gao, M. Y., Zhao, H. M., et al. (2021). Root cell wall chemistry remodelling enhanced arsenic fixation of a cabbage cultivar. J. Hazard. Mater. 420, 126165. doi: 10.1016/j.jhazmat.2021.126165
Huang, H., Zhao, Y., Fan, L., Jin, Q., Yang, G., Xu, Z. (2020). Improvement of manganese phytoremediation by Broussonetia papyrifera with two plant growth promoting (PGP) Bacillus species. Chemosphere 260, 127614. doi: 10.1016/j.chemosphere.2020.127614
Hussain, F., Hussain, I., Khan, A. H. A., Muhammad, Y. S., Iqbal, M., Soja, G., et al. (2018). Combined application of biochar, compost, and bacterial consortia with Italian ryegrass enhanced phytoremediation of petroleum hydrocarbon contaminated soil. Environ. Exp. Bot. 153, 80–88. doi: 10.1016/j.envexpbot.2018.05.012
Hu, Z., Wang, Y., Fang, Z., Shi, G., Lou, L., Ren, K., et al. (2020). Italian Ryegrass–rice rotation system for biomass production and cadmium removal from contaminated paddy fields. J. Soils Sediments 20, 874–882. doi: 10.1007/s11368-019-02470-9
Hussain, A., Kamran, M.A., Javed, M.T., Hayat, K., Farooq, M.A., Ali, N., et al. (2019). Individual and combinatorial application of Kocuria rhizophila and citric acid on phytoextraction of multi-metal contaminated soils by Glycine max L. Environmental and Experimental Botany 159, 23–33. doi: 10.1016/j.envexpbot.2018.12.006
Iram, S., Basri, R., Ahmad, K. S., Jaffri, S. B. (2019). Mycological assisted phytoremediation enhancement of bioenergy crops Zea mays and Helianthus annuus in heavy metal contaminated lithospheric zone. Soil Sediment Contam.: Int. J. 28, 411–430. doi: 10.1080/15320383.2019.1597011
Islam, M. S., Hosen, M. M. L., Uddin, M. N. (2019). Phytodesalination of saline water using ipomoea aquatica, Alternanthera philoxeroides and Ludwigia adscendens. Int. J. Environ. Sci. Technol. 16, 965–972. doi: 10.1007/s13762-018-1705-z
Islam, F., Yasmeen, T., Arif, M. S., Riaz, M., Shahzad, S. M., Imran, Q., et al. (2016). Combined ability of chromium (Cr) tolerant plant growth promoting bacteria (PGPB) and salicylic acid (SA) in attenuation of chromium stress in maize plants. Plant Physiol. Biochem. 108, 456–467. doi: 10.1016/j.plaphy.2016.08.014
Jaskulak, M., Grobelak, A., Vandenbulcke, F. (2020). Modelling assisted phytoremediation of soils contaminated with heavy metals – main opportunities, limitations, decision making and future prospects. Chemosphere 249, 126196. doi: 10.1016/j.chemosphere.2020.126196
Jhilta, P., Dipta, B., Rana, A. (2021). “"Phytoremediation of heavy metals and radionuclides: Sustainable approach to environmental management,",” in Phytoremediation for environmental sustainability. Ed. Prasad, R. (Singapore: Springer Nature Singapore). doi: 10.1007/978-981-16-5621-7_5
Jiang, C., Guan, K., Khanna, M., Chen, L., Peng, J. (2021). Assessing marginal land availability based on land use change information in the contiguous united states. Environ. Sci. Technol. 55, 10794–10804. doi: 10.1021/acs.est.1c02236
Jude, K., Tanee, F. B. G., Mensah, S. I. (2019). Enhancing phytoremediation potential of Andropogon tectorum (Schumach & thonn) in petroleum hydrocarbon contaminated soil using cassava peel. Scientia Africana 18, 23–36. Available at: https://www.ajol.info/index.php/sa/article/view/199472.
Ju, W., Jin, X., Liu, L., Shen, G., Zhao, W., Duan, C., et al. (2020). Rhizobacteria inoculation benefits nutrient availability for phytostabilization in copper contaminated soil: Drivers from bacterial community structures in rhizosphere. Appl. Soil Ecol. 150, 103450. doi: 10.1016/j.apsoil.2019.103450
Kafle, A., Timilsina, A., Gautam, A., Adhikari, K., Bhattarai, A., Aryal, N. (2022). Phytoremediation: Mechanisms, plant selection and enhancement by natural and synthetic agents. Environ. Adv. 8, 100203. doi: 10.1016/j.envadv.2022.100203
Karaghool, H. (2022). “"The employment of endophytic bacteria for phytodegradation of pyridine",” in IOP conference series: Earth and environmental science (Baghdad, Iraq: IOP Publishing).
Karthik, C., Barathi, S., Pugazhendhi, A., Ramkumar, V. S., Thi, N. B. D., Arulselvi, P. I. (2017). Evaluation of Cr(VI) reduction mechanism and removal by Cellulosimicrobium funkei strain AR8, a novel haloalkaliphilic bacterium. J. Hazard. Mater. 333, 42–53. doi: 10.1016/j.jhazmat.2017.03.037
Kasim, W. A., Gaafar, R. M., Abou-Ali, R. M., Omar, M. N., Hewait, H. M. (2016). Effect of biofilm forming plant growth promoting rhizobacteria on salinity tolerance in barley. Ann. Agric. Sci. 61, 217–227. doi: 10.1016/j.aoas.2016.07.003
Ke, T., Guo, G., Liu, J., Zhang, C., Tao, Y., Wang, P., et al. (2021a). Improvement of the Cu and cd phytostabilization efficiency of perennial ryegrass through the inoculation of three metal-resistant PGPR strains. Environ. Pollut. 271, 116314. doi: 10.1016/j.envpol.2020.116314
Ke, T., Zhang, J., Tao, Y., Zhang, C., Zhang, Y., Xu, Y., et al. (2021b). Individual and combined application of Cu-tolerant Bacillus spp. enhance the Cu phytoextraction efficiency of perennial ryegrass. Chemosphere 263, 127952. doi: 10.1016/j.chemosphere.2020.127952
Khan, N., Bano, A. (2018). “"Role of PGPR in the phytoremediation of heavy metals and crop growth under municipal wastewater irrigation,",” in Phytoremediation: Management of environmental contaminants, vol. 6 . Eds. Ansari, A. A., Gill, S. S., Gill, R., Lanza, G. R., Newman, L. (Cham: Springer International Publishing), 135–149. doi: 10.1007/978-3-319-99651-6_5
Konkolewska, A., Piechalak, A., Ciszewska, L., Antos-Krzemińska, N., Skrzypczak, T., Hanć, A., et al. (2020). Combined use of companion planting and PGPR for the assisted phytoextraction of trace metals (Zn, Pb, cd). Environ. Sci. pollut. Res. 27, 13809–13825. doi: 10.1007/s11356-020-07885-3
Kotoky, R., Pandey, P. (2020a). Rhizosphere assisted biodegradation of benzo(a)pyrene by cadmium resistant plant-probiotic Serratia marcescens S2I7, and its genomic traits. Sci. Rep. 105279. doi: 10.1038/s41598-020-62285-4
Kotoky, R., Pandey, P. (2020b). Rhizosphere mediated biodegradation of benzo(A)pyrene by surfactin producing soil bacilli applied through Melia azedarach rhizosphere. Int. J. Phytoremediation 22, 363–372. doi: 10.1080/15226514.2019.1663486
Kowitwiwat, A., Sampanpanish, P. (2020). Phytostabilization of arsenic and manganese in mine tailings using Pennisetum purpureum cv. Mott supplemented with cow manure and acacia wood-derived biochar. Heliyon 6, e04552. doi: 10.1016/j.heliyon.2020.e04552
Kukla, M., Płociniczak, T., Piotrowska-Seget, Z. (2014). Diversity of endophytic bacteria in Lolium perenne and their potential to degrade petroleum hydrocarbons and promote plant growth. Chemosphere 117, 40–46. doi: 10.1016/j.chemosphere.2014.05.055
Kumar, A., Verma, J. P. (2018). Does plant–microbe interaction confer stress tolerance in plants: A review? Microbiol. Res. 207, 41–52. doi: 10.1016/j.micres.2017.11.004
Lachapelle, A., Yavari, S., Pitre, F. E., Courchesne, F., Brisson, J. (2021). Co-Planting of Salix interior and Trifolium pratense for phytoremediation of trace elements from wood preservative contaminated soil. Int. J. Phytoremediation 23, 632–640. doi: 10.1080/15226514.2020.1847034
Ławniczak, Ł., Woźniak-Karczewska, M., Loibner, A. P., Heipieper, H. J., Chrzanowski, Ł. (2020). Microbial degradation of hydrocarbons–basic principles for bioremediation: A review. Molecules 25. doi: 10.3390/molecules25040856
Ledin, M. (2000). Accumulation of metals by microorganisms — processes and importance for soil systems. Earth-Science Rev. 51, 1–31. doi: 10.1016/S0012-8252(00)00008-8
Lewandowski, I., Bahrs, E., Dahmen, N., Hirth, T., Rausch, T., Weidtmann, A. (2019). Biobased value chains for a growing bioeconomy. GCB Bioenergy 11, 4–8. doi: 10.1111/gcbb.12578
Lewandowski, I., Schmidt, U., Londo, M., Faaij, A. (2006). The economic value of the phytoremediation function – assessed by the example of cadmium remediation by willow (Salix ssp). Agric. Syst. 89, 68–89. doi: 10.1016/j.agsy.2005.08.004
Liang, Y., Meggo, R., Hu, D., Schnoor, J. L., Mattes, T. E. (2014). Enhanced polychlorinated biphenyl removal in a switchgrass rhizosphere by bioaugmentation with Burkholderia xenovorans LB400. Ecol. Eng. 71, 215–222. doi: 10.1016/j.ecoleng.2014.07.046
Li, X., Cao, Y., Xiao, J., Salam, M. M. A., Chen, G. (2022). Bamboo biochar greater enhanced Cd/Zn accumulation in salix psammophila under non-flooded soil compared with flooded. Biochar 4, 7. doi: 10.1007/s42773-022-00139-0
Li, Y., Lin, J., Huang, Y., Yao, Y., Wang, X., Liu, C., et al. (2020). ). bioaugmentation-assisted phytoremediation of manganese and cadmium co-contaminated soil by polygonaceae plants (Polygonum hydropiper l. and Polygonum lapathifolium l.) and Enterobacter sp. FM-1. Plant Soil 448, 439–453. doi: 10.1007/s11104-020-04447-x
Liu, S., Ali, S., Yang, R., Tao, J., Ren, B. (2019). A newly discovered cd-hyperaccumulator Lantana camara l. J. Hazard. Mater. 371, 233–242. doi: 10.1016/j.jhazmat.2019.03.016
Liu, L., Li, W., Song, W., Guo, M. (2018). Remediation techniques for heavy metal-contaminated soils: Principles and applicability. Sci. Total Environ. 633, 206–219. doi: 10.1016/j.scitotenv.2018.03.161
Li, Q., Xing, Y., Fu, X., Ji, L., Li, T., Wang, J., et al. (2021). Biochemical mechanisms of rhizospheric Bacillus subtilis-facilitated phytoextraction by alfalfa under cadmium stress – microbial diversity and metabolomics analyses. Ecotoxicol. Environ. Saf. 212, 112016. doi: 10.1016/j.ecoenv.2021.112016
Manoj, S. R., Karthik, C., Kadirvelu, K., Arulselvi, P. I., Shanmugasundaram, T., Bruno, B., et al. (2020). Understanding the molecular mechanisms for the enhanced phytoremediation of heavy metals through plant growth promoting rhizobacteria: A review. J. Environ. Manage. 254, 109779. doi: 10.1016/j.jenvman.2019.109779
Ma, Y., Oliveira, R. S., Freitas, H., Zhang, C. (2016a). Biochemical and molecular mechanisms of plant-Microbe-Metal interactions: Relevance for phytoremediation. Front. Plant Sci. 7. doi: 10.3389/fpls.2016.00918
Ma, Y., Prasad, M. N. V., Rajkumar, M., Freitas, H. (2011). Plant growth promoting rhizobacteria and endophytes accelerate phytoremediation of metalliferous soils. Biotechnol. Adv. 29, 248–258. doi: 10.1016/j.biotechadv.2010.12.001
Ma, Y., Rajkumar, M., Rocha, I., Oliveira, R. S., Freitas, H. (2015). Serpentine bacteria influence metal translocation and bioconcentration of Brassica juncea and Ricinus communis grown in multi-metal polluted soils. Front. Plant Sci. 5. doi: 10.3389/fpls.2014.00757
Ma, Y., Rajkumar, M., Vicente, J., Freitas, H. (2010). Inoculation of Ni-resistant plant growth promoting bacterium psychrobacter sp. strain SRS8 for the improvement of nickel phytoextraction by energy crops. Int. J. Phytoremediation 13, 126–139. doi: 10.1080/15226511003671403
Ma, Y., Rajkumar, M., Zhang, C., Freitas, H. (2016b). Inoculation of Brassica oxyrrhina with plant growth promoting bacteria for the improvement of heavy metal phytoremediation under drought conditions. J. Hazard. Mater. 320, 36–44. doi: 10.1016/j.jhazmat.2016.08.009
Masoudian, Z., Salehi-Lisar, S. Y., Norastehnia, A. (2020). Phytoremediation potential of Azolla filiculoides for sodium dodecyl benzene sulfonate (SDBS) surfactant considering some physiological responses, effects of operational parameters and biodegradation of surfactant. Environ. Sci. pollut. Res. 27, 20358–20369. doi: 10.1007/s11356-020-08286-2
Matsui, K., Yoshinami, S., Narita, M., Chien, M.-F., Phung, L. T., Silver, S., et al. (2016). Mercury resistance transposons in bacilli strains from different geographical regions. FEMS Microbiol. Lett. 363, fnw013. doi: 10.1093/femsle/fnw013
Ma, Y., Zhang, C., Oliveira, R. S., Freitas, H., Luo, Y. (2016c). Bioaugmentation with endophytic bacterium E6S homologous to Achromobacter piechaudii enhances metal rhizoaccumulation in host Sedum plumbizincicola. Front. Plant Sci. 7. doi: 10.3389/fpls.2016.00075
Mehmood, M. A., Ibrahim, M., Rashid, U., Nawaz, M., Ali, S., Hussain, A., et al. (2017). Biomass production for bioenergy using marginal lands. Sustain. Prod. Consump. 9, 3–21. doi: 10.1016/j.spc.2016.08.003
Mello, I. S., Targanski, S., Pietro-Souza, W., Frutuoso Stachack, F. F., Terezo, A. J., Soares, M. A. (2020). Endophytic bacteria stimulate mercury phytoremediation by modulating its bioaccumulation and volatilization. Ecotoxicol. Environ. Saf. 202, 110818. doi: 10.1016/j.ecoenv.2020.110818
Moubasher, H. A., Hegazy, A. K., Mohamed, N. H., Moustafa, Y. M., Kabiel, H. F., Hamad, A. A. (2015). Phytoremediation of soils polluted with crude petroleum oil using Bassia scoparia and its associated rhizosphere microorganisms. Int. Biodeterior. Biodegradation 98, 113–120. doi: 10.1016/j.ibiod.2014.11.019
Mukhopadhyay, S., Maiti, S.K.J.T.B. (2010). Natural mycorrhizal colonization in tree species growing on the reclaimed coalmine overburden dumps: Case study from jharia coalfields, India. Annu. Rev. Plant Biol. 3, 761–770. doi: 10.1146/annurev.arplant.59.032607.092911
Narayanan, M., Kandasamy, G., He, Z., Kandasamy, S., Alfarhan, A. H., Pugazhendhi, A. (2021). Phytoextraction competence of J. curcas l. @ on ore waste dump of the bauxite mine under the influence of multi potential Bacillus cereus. Environ. Technol. Innovation 21, 101221. doi: 10.1016/j.eti.2020.101221
Nebeská, D., Trögl, J., Ševců, A., Špánek, R., Marková, K., Davis, L., et al. (2021). Miscanthus x giganteus role in phytodegradation and changes in bacterial community of soil contaminated by petroleum industry. Ecotoxicol. Environ. Saf. 224, 112630. doi: 10.1016/j.ecoenv.2021.112630
Nedjimi, B. (2021). Phytoremediation: a sustainable environmental technology for heavy metals decontamination. SN Appl. Sci. 3, 286. doi: 10.1007/s42452-021-04301-4
Oliveira, V., Gomes, N. C. M., Almeida, A., Silva, A. M. S., Simões, M. M. Q., Smalla, K., et al. (2014). Hydrocarbon contamination and plant species determine the phylogenetic and functional diversity of endophytic degrading bacteria. Mol. Ecol. 23, 1392–1404. doi: 10.1111/mec.12559
Otlewska, A., Migliore, M., Dybka-Stępień, K., Manfredini, A., Struszczyk-Świta, K., Napoli, R., et al. (2020). When salt meddles between plant, soil, and microorganisms. Front. Plant Sci. 11. doi: 10.3389/fpls.2020.553087
Pancaldi, F., Trindade, L. M. (2020). Marginal lands to grow novel bio-based crops: A plant breeding perspective. Front. Plant Sci. 11. doi: 10.3389/fpls.2020.00227
Pandey, V. C., Pandey, D. N., Singh, N. (2015). Sustainable phytoremediation based on naturally colonizing and economically valuable plants. J. Cleaner Prod. 86, 37–39. doi: 10.1016/j.jclepro.2014.08.030
Pankaj, U., Singh, G., Verma, R. K. (2019). “"Chapter 20 - microbial approaches in management and restoration of marginal lands,",” in New and future developments in microbial biotechnology and bioengineering. Ed. Singh, J. S. (USA: Elsevier), 295–305. doi: 10.1016/B978-0-12-818258-1.00020-0
Parveen, S., Bhat, I. U. H., Khanam, Z., Rak, A. E., Yusoff, H. M., Akhter, M. S. (2022). Phytoremediation: In situ alternative for pollutant removal from contaminated natural media: A brief review. Biointerface Res. Appl. Chem. 12, 4945–4960. doi: 10.33263/BRIAC124.49454960
Pathak, S., Agarwal, A. V., Pandey, V. C. (2020). “"1 - phytoremediation–a holistic approach for remediation of heavy metals and metalloids,",” in Bioremediation of pollutants. Eds. Pandey, V. C., Singh, V. (USA: Elsevier), 3–16. doi: 10.1016/B978-0-12-819025-8.00001-6
Pawlik, M., Cania, B., Thijs, S., Vangronsveld, J., Piotrowska-Seget, Z. (2017). Hydrocarbon degradation potential and plant growth-promoting activity of culturable endophytic bacteria of lotus corniculatus and Oenothera biennis from a long-term polluted site. Environ. Sci. pollut. Res. 24, 19640–19652. doi: 10.1007/s11356-017-9496-1
Pawlik, M., Płociniczak, T., Thijs, S., Pintelon, I., Vangronsveld, J., Piotrowska-Seget, Z. (2020). Comparison of two inoculation methods of endophytic bacteria to enhance phytodegradation efficacy of an aged petroleum hydrocarbons polluted soil. Agronomy 10. doi: 10.3390/agronomy10081196
Pidlisnyuk, V., Mamirova, A., Pranaw, K., Shapoval, P. Y., Trögl, J., Nurzhanova, A. (2020). Potential role of plant growth-promoting bacteria in Miscanthus x giganteus phytotechnology applied to the trace elements contaminated soils. Int. Biodeterior. Biodegradation 155, 105103. doi: 10.1016/j.ibiod.2020.105103
Pivetz, B. E. (2001). "Ground water issue: phytoremediation of contaminated soil and ground water at hazardous waste sites" (USA: National Risk Mannagement Research Lab ADA Ok).
Poria, V., Rana, A., Kumari, A., Grewal, J., Pranaw, K., Singh, S. (2021a). Current perspectives on chitinolytic enzymes and their agro-industrial applications. Biology 10. doi: 10.3390/biology10121319
Poria, V., Singh, S., Nain, L., Singh, B., Saini, J. K. (2021b). “"Rhizospheric microbial communities: Occurrence, distribution, and functions,",” in Microbial metatranscriptomics belowground. Eds. Nath, M., Bhatt, D., Bhargava, P., Choudhary, D. K. (Singapore: Springer Singapore), 239–271. doi: 10.1007/978-981-15-9758-9_12
P.P, S., Puthur, J. T. (2021). Heavy metal phytoremediation by bioenergy plants and associated tolerance mechanisms. Soil Sediment Contam.: Int. J. 30, 253–274. doi: 10.1080/15320383.2020.1849017
Pranaw, K., Pidlisnyuk, V., Trögl, J., Malinská, H. (2020). Bioprospecting of a novel plant growth-promoting bacterium Bacillus altitudinis KP-14 for enhancing Miscanthus × giganteus growth in metals contaminated soil. Biology 9. doi: 10.3390/biology9090305
Qurashi, A. W., Sabri, A. N. (2012). Bacterial exopolysaccharide and biofilm formation stimulate chickpea growth and soil aggregation under salt stress. Braz. J. Microbiol. [publication Braz. Soc. Microbiol. 43, 1183–1191. doi: 10.1590/S1517-838220120003000046
Rabani, M. S., Hameed, I., Mir, T. A., A. Wani, B., Gupta, M. K., Habib, A., et al. (2022). “"Chapter 5 - microbial-assisted phytoremediation," in phytoremediation,”. Phytoremediation: Biotechnological Strategies for Promoting Invigorating Environs Eds. Bhat, R. A., Tonelli, F. M. P., Dar, G. H., Hakeem, K. (UK: Academic Press), 91–114. doi: 10.1016/B978-0-323-89874-4.00006-6
Rahman, F., Sugawara, K., Huang, Y., Chien, M.-F., Inoue, C. (2018). Arsenic, lead and cadmium removal potential of Pteris multifida from contaminated water and soil. Int. J. Phytoremediation 20, 1187–1193. doi: 10.1080/15226514.2017.1375896
Rajkumari, J., Choudhury, Y., Bhattacharjee, K., Pandey, P. (2021). Rhizodegradation of pyrene by a non-pathogenic Klebsiella pneumoniae isolate applied with Tagetes erecta l. and changes in the rhizobacterial community. Front. Microbiol. 12. doi: 10.3389/fmicb.2021.593023
Ramakrishna, W., Rathore, P., Kumari, R., Yadav, R. (2020). Brown gold of marginal soil: Plant growth promoting bacteria to overcome plant abiotic stress for agriculture, biofuels and carbon sequestration. Sci. Total Environ. 711, 135062. doi: 10.1016/j.scitotenv.2019.135062
Ramana, S., Tripathi, A. K., Kumar, A., Singh, A. B., Bharati, K., Sahu, A., et al. (2021). Potential of cotton for remediation of cd-contaminated soils. Environ. Monit. Assess. 193, 186. doi: 10.1007/s10661-021-08976-5
Ren, X.-M., Guo, S.-J., Tian, W., Chen, Y., Han, H., Chen, E., et al. (2019). Effects of plant growth-promoting bacteria (PGPB) inoculation on the growth, antioxidant activity, Cu uptake, and bacterial community structure of rape (Brassica napus l.) grown in Cu-contaminated agricultural soil. Front. Microbiol. 10. doi: 10.3389/fmicb.2019.01455
Riaz, U., Athar, T., Mustafa, U., Iqbal, R. (2022). “"Chapter 23 - economic feasibility of phytoremediation,",” in Phytoremediation. Eds. Bhat, R. A., Tonelli, F. M. P., Dar, G. H., Hakeem, K. (USA: Academic Press). doi: 10.1016/B978-0-323-89874-4.00025-X
Ruley, J. A., Tumuhairwe, J. B., Amoding, A., Westengen, O. T., Vinje, H. (2020). Rhizobacteria communities of phytoremediation plant species in petroleum hydrocarbon contaminated soil of the sudd ecosystem, south Sudan. Int. J. Microbiol. 2020, 6639118. doi: 10.1155/2020/6639118
Saballos, A. (2008). “"Development and utilization of sorghum as a bioenergy crop,",” in Genetic improvement of bioenergy crops. Ed. Vermerris, W. (New York, NY: Springer New York). doi: 10.1007/978-0-387-70805-8_8
Sabreena, H.S., Bhat, S. A., Kumar, V., Ganai, B. A., Ameen, F. (2022). Phytoremediation of heavy metals: An indispensable contrivance in green remediation technology. Plants 11. doi: 10.3390/plants11091255
Saikia, J., Sarma, R. K., Dhandia, R., Yadav, A., Bharali, R., Gupta, V. K., et al. (2018). Alleviation of drought stress in pulse crops with ACC deaminase producing rhizobacteria isolated from acidic soil of northeast India. Sci. Rep. 83560. doi: 10.1038/s41598-018-21921-w
Saleem, M. H., Ali, S., Hussain, S., Kamran, M., Chattha, M. S., Ahmad, S., et al. (2020a. Flax (Linum usitatissimum l.): A potential candidate for phytoremediation? biological and economical points of view. Plants 9. doi: 10.3390/plants9040496
Saleem, M. H., Ali, S., Rehman, M., Hasanuzzaman, M., Rizwan, M., Irshad, S., et al. (2020b). Jute: A potential candidate for phytoremediation of metals–a review. Plants 9. doi: 10.3390/plants9020258
Saleem, M. H., Kamran, M., Zhou, Y., Parveen, A., Rehman, M., Ahmar, S., et al. (2020c). Appraising growth, oxidative stress and copper phytoextraction potential of flax (Linum usitatissimum l.) grown in soil differentially spiked with copper. J. Environ. Manage. 257, 109994. doi: 10.1016/j.jenvman.2019.109994
Saleem, M. U., Naeem Asghar, H., Ahmad Zahir, Z., Shahid, M. (2019). EVALUATION OF LEAD TOLERANT PLANT GROWTH PROMOTING RHIZOBACTERIA FOR PLANT GROWTH AND PHYTOREMEDIATION IN LEAD CONTAMINATION. Rev. Internacional Contaminación Ambiental 35, 999–1009. doi: 10.20937/rica.2019.35.04.18
Sansinenea, E. (2019). “"Bacillus spp.: As plant growth-promoting bacteria,",” in Secondary metabolites of plant growth promoting rhizomicroorganisms: Discovery and applications. Eds. Singh, H. B., Keswani, C., Reddy, M. S., Sansinenea, E., García-Estrada, C. (Singapore: Singapore: Springer), 225–237. doi: 10.1007/978-981-13-5862-3_11
Saran, A., Fernandez, L., Cora, F., Savio, M., Thijs, S., Vangronsveld, J., et al. (2020a). Phytostabilization of Pb and cd polluted soils using Helianthus petiolaris as pioneer aromatic plant species. Int. J. Phytoremediation 22, 459–467. doi: 10.1080/15226514.2019.1675140
Saran, A., Imperato, V., Fernandez, L., Gkorezis, P., D’haen, J., Merini, L. J., et al. (2020b). Phytostabilization of polluted military soil supported by bioaugmentation with PGP-trace element tolerant bacteria isolated from Helianthus petiolaris. Agronomy 10, 459–467. doi: 10.3390/agronomy10020204
Schirawski, J., Perlin, M. H. (2018). Plant–microbe interaction 2017–the good, the bad and the diverse. Int. J. Mol. Sci. 19. doi: 10.3390/ijms19051374
Schröder, P., Beckers, B., Daniels, S., Gnädinger, F., Maestri, E., Marmiroli, N., et al. (2018). Intensify production, transform biomass to energy and novel goods and protect soils in Europe–a vision how to mobilize marginal lands. Sci. Total Environ. 616-617, 1101–1123. doi: 10.1016/j.scitotenv.2017.10.209
Schwitzguébel, J.-P. (2017). Phytoremediation of soils contaminated by organic compounds: hype, hope and facts. J. Soils Sediments 17, 1492–1502. doi: 10.1007/s11368-015-1253-9
Shackira, A. M., Puthur, J. T. (2019). “"Phytostabilization of heavy metals: Understanding of principles and practices,",” in Plant-metal interactions. Eds. Srivastava, S., Srivastava, A. K., Suprasanna, P. (Cham: Springer International Publishing), 263–282. doi: 10.1007/978-3-030-20732-8_13
Shahane, A. A., Shivay, Y. S. (2021). Soil health and its improvement through novel agronomic and innovative approaches. Front. Agron. 3. doi: 10.3389/fagro.2021.680456
Shah, V., Daverey, A. (2020). Phytoremediation: A multidisciplinary approach to clean up heavy metal contaminated soil. Environ. Technol. Innovation 18, 100774. doi: 10.1016/j.eti.2020.100774
Shen, S., Chen, J., Chang, J., Xia, B. (2020). Using bioenergy crop cassava (Manihot esculenta) for reclamation of heavily metal-contaminated land. Int. J. Phytoremediation 22, 1313–1320. doi: 10.1080/15226514.2020.1768512
Sikdar, A., Wang, J., Hasanuzzaman, M., Liu, X., Feng, S., Roy, R., et al. (2020). Phytostabilization of Pb-zn mine tailings with Amorpha fruticosa aided by organic amendments and triple superphosphate. Molecules 25. doi: 10.3390/molecules25071617
Silver, S., Hobman, J. L. (2007). “"Mercury microbiology: Resistance systems, environmental aspects, methylation,and human health,",” in Molecular microbiology of heavy metals. Eds. Nies, D. H., Silver, S. (Berlin, Heidelberg: Springer Berlin Heidelberg), 357–370. doi: 10.1007/7171_2006_085
Singh, D., Ghosh, P., Kumar, J., Kumar, A. (2019). “"Plant growth-promoting rhizobacteria (PGPRs): Functions and benefits,",” in Microbial interventions in agriculture and environment: Volume 2: Rhizosphere, microbiome and agro-ecology. Eds. Singh, D. P., Gupta, V. K., Prabha, R. (Singapore: Springer Singapore), 205–227. doi: 10.1007/978-981-13-8383-0_7
Sofy, M. R., Aboseidah, A. A., Heneidak, S. A., Ahmed, H. R. (2021). ACC deaminase containing endophytic bacteria ameliorate salt stress in Pisum sativum through reduced oxidative damage and induction of antioxidative defense systems. Environ. Sci. Pollut. Res. 28, 40971–40991. doi: 10.1007/s11356-021-13585-3
Soldatos, P. (2015). Economic aspects of bioenergy production from perennial grasses in marginal lands of South Europe. Bioenergy Res. 8, 1562–1573. doi: 10.1007/s12155-015-9678-y
Sorour, A. A., Khairy, H., Zaghloul, E. H., Zaghloul, H. (2022). Microbe-plant interaction as a sustainable tool for mopping up heavy metal contaminated sites. BMC Microbiol. 22, 174. doi: 10.1186/s12866-022-02587-x
Sung-Je, Y., Weon, H.-Y., Song, J., Sang, M. K. (2019). Induced tolerance to salinity stress by halotolerant bacteria Bacillus aryabhattai H19-1 and B. mesonae H20-5 in tomato plants. J. Microbiol. Biotechnol. 29, 1124–1136. doi: 10.4014/jmb.1904.04026
Sun, L., Yang, Y., Wang, R., Li, S., Qiu, Y., Lei, P., et al. (2020). Effects of exopolysaccharide derived from Pantoea alhagi NX-11 on drought resistance of rice and its efficient fermentation preparation. Int. J. Biol. Macromolecules 162, 946–955. doi: 10.1016/j.ijbiomac.2020.06.199
Taeprayoon, P., Homyog, K., Meeinkuirt, W. (2022). Organic amendment additions to cadmium-contaminated soils for phytostabilization of three bioenergy crops. Sci. Rep. 12, 13070. doi: 10.1038/s41598-022-17385-8
Tak, H. I., Ahmad, F., Babalola, O. O. (2013). “"Advances in the application of plant growth-promoting rhizobacteria in phytoremediation of heavy metals,",” in Reviews of environmental contamination and toxicology. Ed. Whitacre, D. M. (New York, NY: Springer New York), 33–52. doi: 10.1007/978-1-4614-5577-6_2
Tchounwou, P. B., Yedjou, C. G., Patlolla, A. K., Sutton, D. J. (2012). “"Heavy metal toxicity and the environment," in molecular, clinical and environmental toxicology,” in Environmental toxicology, vol. 3 . Ed. Luch, A. (Basel: Springer Basel), 133–164. doi: 10.1007/978-3-7643-8340-4_6
Thijs, S., Sillen, W., Weyens, N., Vangronsveld, J. (2017). Phytoremediation: State-of-the-art and a key role for the plant microbiome in future trends and research prospects. Int. J. Phytoremediation 19, 23–38. doi: 10.1080/15226514.2016.1216076
Tiepo, A. N., Constantino, L. V., Madeira, T. B., Gonçalves, L. S. A., Pimenta, J. A., Bianchini, E., et al. (2020). Plant growth-promoting bacteria improve leaf antioxidant metabolism of drought-stressed Neotropical trees. Planta 251, 83. doi: 10.1007/s00425-020-03373-7
Tiodar, E. D., Văcar, C. L., Podar, D. (2021). Phytoremediation and microorganisms-assisted phytoremediation of mercury-contaminated soils: Challenges and perspectives. Int. J. Environ. Res. Public Health 18. doi: 10.3390/ijerph18052435
Ullah, A., Heng, S., Munis, M. F. H., Fahad, S., Yang, X. (2015). Phytoremediation of heavy metals assisted by plant growth promoting (PGP) bacteria: A review. Environ. Exp. Bot. 117, 28–40. doi: 10.1016/j.envexpbot.2015.05.001
Ummara, U., Noreen, S., Afzal, M., Ahmad, P. (2021). Bacterial bioaugmentation enhances hydrocarbon degradation, plant colonization and gene expression in diesel-contaminated soil. Physiologia Plantarum 173, 58–66. doi: 10.1111/ppl.13171
Visconti, D., Ventorino, V., Fagnano, M., Woo, S. L., Pepe, O., Adamo, P., et al. (2022). Compost and microbial biostimulant applications improve plant growth and soil biological fertility of a grass-based phytostabilization system. Environ. Geochemist. Health. doi: 10.1007/s10653-022-01235-7
Vishnupradeep, R., Bruno, L. B., Taj, Z., Karthik, C., Challabathula, D., et al. (2022). Tripti, Kumar, a Plant growth promoting bacteria improve growth and phytostabilization potential of Zea mays under chromium and drought stress by altering photosynthetic and antioxidant responses. Environ. Technol. Innovation 25, 102154. doi: 10.1016/j.eti.2021.102154
Vives-Peris, V., De Ollas, C., Gómez-Cadenas, A., Pérez-Clemente, R. M. (2020). Root exudates: from plant to rhizosphere and beyond. Plant Cell Rep. 39, 3–17. doi: 10.1007/s00299-019-02447-5
Vural, A., Safari, S. (2022). Phytoremediation ability of Helichrysum arenarium plant for au and Ag: Case study at demirören village (Gümüşhane, Turkey). Gold Bull 55, 129–136. doi: 10.1007/s13404-022-00313-z
Wang, X., Liang, J., Liu, Z., Kuang, Y., Han, L., Chen, H., et al. (2022). Transcriptional regulation of metal metabolism- and nutrient absorption-related genes in eucalyptus grandis by arbuscular mycorrhizal fungi at different zinc concentrations. BMC Plant Biol. 22, 76. doi: 10.1186/s12870-022-03456-5
Wang, S., Teng, S., Fan, M. (2010) “Interaction between heavy metals and aerobic granules” in Environmental management Ed. Sarkar, S.. UK: IntechOpen. doi: 10.5772/10106
Wan, X., Lei, M., Chen, T. (2016). Cost–benefit calculation of phytoremediation technology for heavy-metal-contaminated soil. Sci. total Environ. 563, 796–802. doi: 10.1016/j.scitotenv.2015.12.080
Witzel, C.-P., Finger, R. (2016). Economic evaluation of Miscanthus production – a review. Renewable Sustain. Energy Rev. 53, 681–696. doi: 10.1016/j.rser.2015.08.063
Wu, Y., Ma, L., Liu, Q., Vestergård, M., Topalovic, O., Wang, Q., et al. (2020a). The plant-growth promoting bacteria promote cadmium uptake by inducing a hormonal crosstalk and lateral root formation in a hyperaccumulator plant Sedum alfredii. J. hazard. mater. 395, 122661. doi: 10.1016/j.jhazmat.2020.122661
Wu, Y., Wang, M., Yu, L., Tang, S.-W., Xia, T., Kang, H., et al. (2020b). A mechanism for efficient cadmium phytoremediation and high bioethanol production by combined mild chemical pretreatments with desirable rapeseed stalks. Sci. Total Environ. 708, 135096. doi: 10.1016/j.scitotenv.2019.135096
Xiang, L., Song, Y., Bian, Y., Liu, G., Herzberger, A., Gu, C., et al. (2018). Manure amendment reduced plant uptake and enhanced rhizodegradation of 2,2′,4, 4′-tetrabrominated diphenyl ether in soil. Biol. Fertility Soils 54, 807–817. doi: 10.1007/s00374-018-1304-7
Xiao, M.-Z., Sun, R., Du, Z.-Y., Yang, W.-B., Sun, Z., Yuan, T.-Q. (2021). A sustainable agricultural strategy integrating cd-contaminated soils remediation and bioethanol production using sorghum cultivars. Ind. Crops Products 162, 113299. doi: 10.1016/j.indcrop.2021.113299
Xu, Q., Renault, S., Yuan, Q. (2019). Phytodesalination of landfill leachate using Puccinellia nuttalliana and Typha latifolia. Int. J. Phytoremediation 21, 831–839. doi: 10.1080/15226514.2019.1568383
Yadav, S., Modi, P., Dave, A., Vijapura, A., Patel, D., Patel, M. (2020). “"Effect of abiotic stress on crops,",” in Sustainable crop production. Eds. Hasanuzzaman, M.C.M.T.F.M., Fujita, M., Assis, T., Nogueira, R. (London: IntechOpen). doi: 10.5772/intechopen.88434
Yang, Y., Guo, Y. (2018). Unraveling salt stress signaling in plants. J. Integr. Plant Biol. 60, 796–804. doi: 10.1111/jipb.12689
Yan, A., Wang, Y., Tan, S. N., Mohd Yusof, M. L., Ghosh, S., Chen, Z. (2020). Phytoremediation: A promising approach for revegetation of heavy metal-polluted land. Front. Plant Sci. 11. doi: 10.3389/fpls.2020.00359
Yu, G., Jiang, P., Fu, X., Liu, J., Sunahara, G. I., Chen, Z., et al. (2020). Phytoextraction of cadmium-contaminated soil by Celosia argentea linn.: A long-term field study. Environ. Pollut. 266, 115408. doi: 10.1016/j.envpol.2020.115408
Zainab, N., Amna, Khan, A. A., Azeem, M. A., Ali, B., Wang, T., et al. (2021). “PGPR-mediated plant growth attributes and metal extraction ability,” in Industrially contaminated soils, vol. 11 . Ed. Sesbania sesban, L. (Basel, Switzerland: Agronomy). doi: 10.3390/agronomy11091820
Zamani, J., Hajabbasi, M. A., Mosaddeghi, M. R., Soleimani, M., Shirvani, M., Schulin, R. (2018). Experimentation on degradation of petroleum in contaminated soils in the root zone of maize (Zea mays l.) inoculated with Piriformospora indica. Soil Sediment Contam.: Int. J. 27, 13–30. doi: 10.1080/15320383.2018.1422693
Zeng, P., Guo, Z., Xiao, X., Peng, C., Feng, W., Xin, L., et al. (2019). Phytoextraction potential of Pteris vittata l. co-planted with woody species for as, cd, Pb and zn in contaminated soil. Sci. Total Environ. 650, 594–603. doi: 10.1016/j.scitotenv.2018.09.055
Zgorelec, Z., Bilandzija, N., Knez, K., Galic, M., Zuzul, S. (2020). Cadmium and mercury phytostabilization from soil using Miscanthus × giganteus. Sci. Rep. 106685. doi: 10.1038/s41598-020-63488-5
Zhang, Q., Kong, W., Wei, L., Wang, Y., Luo, Y., Wang, P., et al. (2020). Uptake, phytovolatilization, and interconversion of 2,4-dibromophenol and 2,4-dibromoanisole in rice plants. Environ. Int. 142, 105888. doi: 10.1016/j.envint.2020.105888
Zhang, H., Tian, Y., Wang, L., Zhang, L., Dai, L. (2013). Ecophysiological characteristics and biogas production of cadmium-contaminated crops. Bioresource Technol. 146, 628–636. doi: 10.1016/j.biortech.2013.07.148
Zhang, X., Yu, J., Huang, Z., Li, H., Liu, X., Huang, J., et al. (2021). Enhanced cd phytostabilization and rhizosphere bacterial diversity of Robinia pseudoacacia l. by endophyte Enterobacter sp. YG-14 combined with sludge biochar. Sci. Total Environ. 787, 147660. doi: 10.1016/j.scitotenv.2021.147660
Zhou, J.-W., Li, Z., Liu, M.-S., Yu, H.-M., Wu, L.-H., Huang, F., et al. (2020). Cadmium isotopic fractionation in the soil–plant system during repeated phytoextraction with a cadmium hyperaccumulating plant species. Environ. Sci. Technol. 54, 13598–13609. doi: 10.1021/acs.est.0c03142
Zhuang, D., Jiang, D., Liu, L., Huang, Y. (2011). Assessment of bioenergy potential on marginal land in China. Renewable Sustain. Energy Rev. 15, 1050–1056. doi: 10.1016/j.rser.2010.11.041
Keywords: phytoremediation, plant growth-promoting bacteria (PGPB), marginal land, biodegradation, heavy metals (HMs), organic pollutants
Citation: Poria V, Dębiec-Andrzejewska K, Fiodor A, Lyzohub M, Ajijah N, Singh S and Pranaw K (2022) Plant Growth-Promoting Bacteria (PGPB) integrated phytotechnology: A sustainable approach for remediation of marginal lands. Front. Plant Sci. 13:999866. doi: 10.3389/fpls.2022.999866
Received: 21 July 2022; Accepted: 04 October 2022;
Published: 21 October 2022.
Edited by:
Mengting Maggie Yuan, University of California, Berkeley, United StatesReviewed by:
Muhammad Yahya Khan, University of Agriculture, Faisalabad, PakistanGiovana Slanzon, University of Hawaii, United States
Copyright © 2022 Poria, Dębiec-Andrzejewska, Fiodor, Lyzohub, Ajijah, Singh and Pranaw. This is an open-access article distributed under the terms of the Creative Commons Attribution License (CC BY). The use, distribution or reproduction in other forums is permitted, provided the original author(s) and the copyright owner(s) are credited and that the original publication in this journal is cited, in accordance with accepted academic practice. No use, distribution or reproduction is permitted which does not comply with these terms.
*Correspondence: Kumar Pranaw, a3ByYW5hd0BnbWFpbC5jb20=; ay5wcmFuYXdAdXcuZWR1LnBs