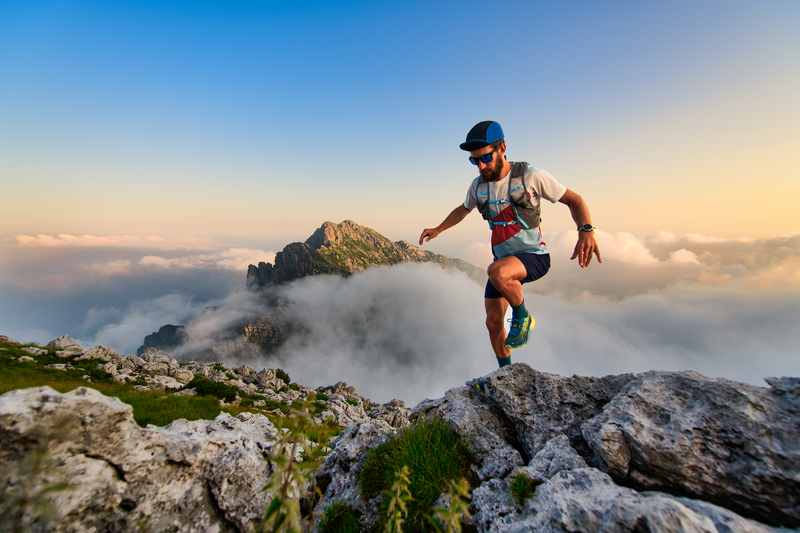
95% of researchers rate our articles as excellent or good
Learn more about the work of our research integrity team to safeguard the quality of each article we publish.
Find out more
ORIGINAL RESEARCH article
Front. Plant Sci. , 18 October 2022
Sec. Plant Physiology
Volume 13 - 2022 | https://doi.org/10.3389/fpls.2022.998267
This article is part of the Research Topic Ethylene: A key regulatory molecule in plants, Volume II View all 8 articles
Plants are often faced with an array of adverse environmental conditions and must respond appropriately to grow and develop. In angiosperms, the plant hormone ethylene is known to play a protective role in responses to abiotic stress. Here we investigated whether ethylene mediates resistance to abiotic stress in the liverwort Marchantia polymorpha, one of the most distant land plant relatives of angiosperms. Using existing M. polymorpha knockout mutants of Mpein3, and Mpctr1, two genes in the ethylene signaling pathway, we examined responses to heat, salinity, nutrient deficiency, and continuous far-red light. The Mpein3 and Mpctr1 mutants were previously shown to confer ethylene insensitivity and constitutive ethylene responses, respectively. Using mild or sub-lethal doses of each stress treatment, we found that Mpctr1 mutants displayed stress resilience similar to or greater than the wild type. In contrast, Mpein3 mutants showed less resilience than the wild type. Consistent with ethylene being a stress hormone, we demonstrated that ethylene production is enhanced by each stress treatment. These results suggest that ethylene plays a role in protecting against abiotic stress in M. polymorpha, and that ethylene has likely been conserved as a stress hormone since before the evolutionary divergence of bryophytes from the land plant lineage approximately 450 Ma.
Environmental stresses, such as high temperature, salinity, nutrient deficiency, drought, and flooding, can gravely impact plant growth and development. Plants have evolved an ability to deal with these stresses using hormone signaling networks that help maintain growth and development (Verma et al., 2016). In angiosperms, one of the plant hormones that mediates responses to biotic and abiotic stresses is ethylene gas, which affects many aspects of growth and development (Abeles et al., 1992; Dubois et al., 2018; Chen et al., 2022). For example, plants synthesize ethylene in response to abiotic stress [e.g., under flooding (Fukao and Bailey-Serres, 2008), iron deficiency (Romera et al., 1999), or high salinity (Achard et al., 2006)]. The protective role of ethylene signaling has been shown using ethylene mutants. For example, ethylene biosynthesis mutants in petunia are more sensitive to salt stress (Naing et al., 2022), as are tobacco ethylene-insensitive mutants (Cao et al., 2007). In Arabidopsis thaliana, ethylene-insensitive mutants are less resistant to heat stress (Larkindale and Knight, 2002), have reduced basal thermotolerance (Huang et al., 2021), and are defective in shade avoidance (i.e., a low ratio of Red : Far-Red light) (Pierik et al., 2009).
The thalloid liverwort Marchantia polymorpha is a model organism whose features include rapid growth, asexual reproduction (via propagules called gemmae), ease of performing genetic crosses, and established transformation methods (Ishizaki et al., 2016). M. polymorpha has a relatively small, sequenced genome (~280 Mb) that generally carries low genetic redundancy (Bowman et al., 2017). M. polymorpha belongs to possibly the earliest of land plant lineages (Wellman et al., 2003), and thus studies in M. polymorpha can provide evolutionary insights into plant hormones in land plant evolution.
Relatively little is known regarding the roles of ethylene in bryophytes. M. polymorpha is capable of producing ethylene, and its genome carries homologs of the entire ethylene signaling pathway as known in angiosperms (Li et al., 2020). Using CRISPR/Cas9 in M. polymorpha, Li et al. (2020) generated knockout alleles for two critical components in the ethylene signaling pathway, MpEIN3 and MpCTR1. As shown in Li et al. (2020), MpEIN3 is the single ortholog of the EIN3 master transcription factor (Chao et al., 1997), and MpCTR1 is the single ortholog of the CTR1 protein kinase (Kieber et al., 1993), which signals immediately downstream of the ethylene receptors (Binder, 2020). CTR1 regulates EIN2, which leads to stabilization/accumulation of EIN3 (Binder, 2020). In M. polymorpha, MpEIN3 and MpCTR1 were found to have conserved roles as positive and negative regulators, respectively, of ethylene responses; that is, Mpein3 mutants exhibit ethylene insensitivity, while Mpctr1 mutants display constitutive responses to ethylene, like their orthologs in A. thaliana (Li et al., 2020). Ethylene responses in M. polymorpha include enhanced thallus growth, stimulation of gemmae cup formation, and increased gemmae dormancy (Li et al., 2020). Consequently, compared to the wild type, Mpctr1 plants are larger, have more gemma cups per thallus area, and have a higher proportion of dormant gemmae, whereas Mpein3 plants are smaller, have a lower density of gemma cups, and have a higher proportion of non-dormant gemmae (Li et al., 2020).
Here we carried out a genetic study to investigate whether ethylene signaling plays a protective role against abiotic stresses in M. polymorpha. We used the Mpctr1 and Mpein3 mutants to examine their ability to tolerate heat, salinity, nutrient deficiency, and continuous far-red light. We also examined whether abiotic stresses can induce ethylene biosynthesis in M. polymorpha. Our results demonstrate that ethylene is a stress hormone in M. polymorpha, providing protection against diverse abiotic stresses. Given that liverworts belong to one of the earliest lineages of land plants, these findings suggest that ethylene’s role as a stress hormone is at least as old as land colonization by plants.
The M. polymorpha Australian (MEL accession) lines used in this study were previously described in Li et al. (2020): wild-type lines WT1 and WT2 (formerly named “WT3”), and three CRISPR/Cas9 knockout alleles of Mpein3 (L1, L5, L6) and three CRISPR/Cas9 knockout alleles of Mpctr1 (L1, L3, L4). Plants were maintained asexually by gemmae propagation. Individual gemmae were plated on solid growth medium in 60 mm x 15 mm Petri dishes with one gemma per dish unless otherwise indicated. All stresses were applied to gemmae starting from day 0 (the day of plating the gemmae), except for the treatments described in the Ethylene Production Assay section.
For non-stress conditions, plants were grown axenically from gemmae at 21 °C under continuous white light at 85-95 μmol m-2s-1 on a solid growth medium consisting of half-strength Gamborg’s B5 salts (Gamborg et al., 1968), pH 5.5, supplemented with 0.2% sucrose and 1% plant agar (Sigma). For heat stress, the plants were incubated in chambers at the stated temperatures. For salt stress, the medium was supplemented with the stated concentrations of NaCl. For nutrient deficiency, the Gamborg’s B5 salts were diluted to the stated concentrations (while keeping other medium contents the same). For far-red light treatment, continuous far-red light (730 nm) at 60 μmol m-2s-1 was added to the normal illumination (<0.15 R:FR ratio).
For ethylene biosynthesis measurements, plants were incubated in liquid growth medium in vials as described in the Ethylene Production Assay section.
Plant size was assessed by measuring the two-dimensional area using ImageJ (https://imagej.nih.gov/ij/). Plants were photographed using a digital camera (Nikon D3200). Using ImageJ, the color threshold was adjusted to cover the plants as much as possible with ‘RGB’ as the color space on the dark background setting. The wand (tracing) tool was used to measure the area after setting the pixels to a known length (in cm).
Relative ground cover area was determined by normalizing the average ground cover area of the control plants to 100%, and then calculating the average ground cover area of the treated plants as a percentage of the control.
The density of gemma cups was the number of gemma cups on a plant (counted by eye) divided by the ground cover area of that plant. For the relative density of gemma cups, the average density of gemma cups for the control plants was normalized to 100%, and then the average density of gemma cups for the treated plants was calculated as a percentage of the control.
These experiments were performed three times with similar results. For the 28 °C and 29 °C treatments we combined the data from the experimental replicates, but in all other cases, we show the data from one experiment rather than combining the data from replicate experiments.
Extraction and measurement of chlorophyll content were performed as described in Welburn (1994) using dimethyl sulfoxide as the organic solvent. Thalli pieces were excised from 41-day old plants, weighed, and air dried before submerging them in 10 mL DMSO. Samples were then incubated at 60 °C for 1 hour with periodic shaking. Absorbance was determined at 649 nm and 665 nm using a GENESYS 10UV-Vis spectrophotometer. The chlorophyll experiment was performed once with n = 4-6 plants.
Wild-type gemmalings (10-day old) were transferred from solid growth medium in Petri dishes to liquid medium in sterile 4-mL glass vials with septa-fitted airtight screw caps (Fisher Scientific). One plant was placed in each vial with only the rhizoids immersed in the liquid medium (with the dorsal side of the thallus facing upwards). The vials were placed in a growth chamber for 48 hours, after which 250 µL of gas from the headspace was removed through the septum using a syringe needle and injected into a gas chromatograph (Shimadzu GC-2010 PLUS) in order to measure ethylene. Following the ethylene measurements, the plants were weighed after briefly laying them on filter paper to absorb excess liquid.
For the control without treatment, the medium in the vial consisted of 300 µL of normal growth medium without agar. For heat stress, the vials were incubated at 29 °C. For salt stress, the liquid medium additionally contained 10 mM NaCl. For nutrient stress, the Gamborg’s B5 salts in the liquid medium were diluted 1/20. These experiments were performed three times with similar results. The data from one of these experiments is presented.
For far-red light treatment, the vials were laid on their sides for greater exposure to the far-red light. This experiment was performed once.
All probability analyses were performed using Prism (v.9.4.0, GraphPad). For comparisons between two groups, we used the two tailed t-test when the data were normally distributed and both groups had the same SD. For comparisons among multiple groups, we used one-way ANOVA with Tukey’s HSD post hoc test for populations that have normality of residuals and the same SD. When SD values were unequal (Figure S2B), we used the Brown–Forsythe and Welch ANOVA tests with Tamhane’s T2 post hoc test.
For the normalized graphs, we estimated the standard error of the mean (SEM) for the ratio between stress-treated mean value and the control mean value using the Taylor approximation of the variance for a ratio between two variables.
To determine the role of ethylene in heat stress tolerance, we assessed growth inhibition of two wild-type lines and the Mpein3 and Mpctr1 mutants (three alleles each) at elevated temperatures (27 °C to 30 °C) in comparison to growth at the normal temperature (21 °C). Gemmae were incubated at constant temperature starting at day 0 (the day gemmae were plated). After 14 days, we measured the “ground cover area” (i.e., the two-dimensional sizes) of the resulting plants. The 30 °C incubation was essentially lethal, as it severely inhibited gemmaling growth of all three genotypes and negatively impacted development, i.e., the plants were small and misshapen and did not produce gemmae (Figures S1A, B). At 30 °C, all three genotypes were inhibited to similar extents relative to their respective average sizes at the normal growth temperature (Figure S1C).
Incubation at 29 °C exerted sub-lethal stress. After 14 days at 29 °C, all lines showed a more compact, circular thallus shape (e.g., Figure 1A). Mpctr1 plants were still larger than the wild type, but both the wild type and Mpctr1 were inhibited by roughly 47% relative to their respective average sizes (two-dimensional ground cover area) at 21 °C. In contrast, the Mpein3 ethylene-insensitive plants were inhibited by 77% relative to their average size at 21 °C (Figures 1B, C). We also assessed the number of gemma cups per thallus area at 29 °C versus 21 °C. Mpein3 produced no gemma cups at 29 °C (Table S1) and were more sensitive in terms of relative gemma cup density compared to Mpctr1 and the wild type (Figure 1D). These results indicated that ethylene hormone signaling provides some tolerance to heat stress at 29 °C.
Figure 1 Mpein3 mutants are more sensitive to 29°C heat stress than Mpctr1 mutants or the wild type (WT). (A) Representative images of 14-day old WT, Mpein3, and Mpctr1 plants grown at 21°C or 29°C. (B) Average size (ground cover area) of WT, Mpein3, and Mpctr1 plants grown at 21°C and 29°C for 14 days. Bars show the overall mean ± SD. per genotype. Each data point represents the mean per WT line or mutant allele ± SD. For 21°C, n=24 WT (12 each of WT1 (white), WT2 (grey)); n=36 Mpein3 (11 L1 (light pink), 12 L5 (pink), 13 L6 (magenta) and n=36 Mpctr1 (12 L1 (light blue), 11 L3 (turquoise), 13 L4 (dark blue)). For 29°C, n=24 WT (12 each of WT1, WT2); n=32 Mpein3 (12 L1, 11 L5, 9 L6) and n=36 Mpctr1 (12 each of L1, L3, L4). *** = P<0.0001. P values were determined using a two-tailed t-test. (C) Relative ground cover area (based on the data in B) shown as a percentage of the corresponding control for each genotype. Bars show the mean ± SEM. Different letters indicate significant difference at P< 0.05, determined using one way ANOVA followed by Tukey’s HSD post hoc test (P<0.0001). (D) Relative density of gemma cups (for the plants treated with 29°C in B) shown as a percentage of the corresponding 21°C control for each genotype. (Absolute values are shown in Table S1.) Bars show the mean ± SEM. Different letters indicate significant difference at P< 0.05, determined using one way ANOVA followed by Tukey’s HSD post hoc test (P<0.0001).
Interestingly, incubation at 27 °C and 28 °C gave enhanced growth of all genotypes compared to their respective average sizes at 21 °C (Figure S2). Thus, 27 °C and 28 °C stimulate, rather than inhibit, gemmaling growth in M. polymorpha. At 28 °C, the relative growth of Mpein3 was greater than that of Mpctr1 (Figure S2E). Because this enhanced growth was observed even in the Mpein3 ethylene-insensitive mutant, the enhancement appeared to be independent of ethylene signaling.
To examine the role of ethylene in tolerance to salinity, which exerts both ionic and osmotic stress, we first incubated gemmae of the wild type and Mpein3 mutant alleles for 14 days on growth medium supplemented with 0 mM, 25 mM, 75mM, and 150 mM NaCl. Images of representative 14-day old plants indicate that growth was inhibited by 25 mM and 75 mM NaCl, while 150 mM NaCl was essentially lethal to both genotypes (Figure S3). These results were consistent with the findings of Tanaka et al. (2018), who showed that growth of wild-type M. polymorpha is dramatically inhibited on medium containing 50 mM NaCl and completely inhibited on 250 mM NaCl.
On medium containing 10 mM NaCl, we found that growth of the wild type was only mildly inhibited (Figure 2). We then tested Mpein3 and Mpctr1 mutants on 10 mM NaCl. Interestingly, Mpctr1 plants fared better than the wild type, displaying no significant effects. In contrast, growth of Mpein3 plants was inhibited to a greater degree than the wild type (Figures 2A–C). At this dose, gemma cups still formed normally on wild-type and Mpctr1 plants, yet Mpein3 plants failed to produce gemma cups similar to what we observed at 29 °C (Figure 2D and Table S1). These results indicated that ethylene hormone signaling plays a role in tolerance to mild salt stress.
Figure 2 Mpein3 mutants are more sensitive to NaCl than Mpctr1 mutants or WT. (A) Representative images of 14-day old WT, Mpein3, and Mpctr1 plants grown with or without 10 mM NaCl added to the growth medium. (B) Average size (ground cover area) of WT, Mpein3, and Mpctr1 plants grown on medium containing 0 mM NaCl and 10 mM NaCl for 14 days. Bars show the overall mean ± SD per genotype. Each data point represents the mean per WT line or mutant allele ± SD. For 0 mM NaCl, n=24 WT (12 each of WT1 (white), WT2 (grey)); n=32 Mpein3 (12 L1 (light pink), 8 L5 (pink), 12 L6 (magenta)); n=35 Mpctr1 (12 L1 (light blue), 12 L3 (turquoise), 11 L4 (dark blue)). For 10 mM NaCl, n=24 WT (12 each of WT1, WT2); n=29 Mpein3 (9 L1, 11 L5, 9 L6); n=35 Mpctr1 (12 L1, 11 L3, 12 L4) *** = P<0.0001. P values were determined using a two-tailed t-test. (C) Relative ground cover area (based on the data in B) shown as a percentage of the corresponding control for each genotype. Bars show the mean ± SEM. Different letters indicate significant difference at P< 0.05, determined using one way ANOVA followed by Tukey’s HSD post hoc test (P<0.0001). (D) Relative density of gemma cups (for the plants treated with 10 mM NaCl in B) shown as a percentage of the corresponding 0 mM NaCl control for each genotype. (Absolute values are shown in Table S1.) Bars show the mean ± SEM. Different letters indicate significant difference at P< 0.05, determined using one way ANOVA followed by Tukey’s HSD post hoc test (P<0.0001).
To investigate the role of ethylene in resilience under nutrient deficiency, we incubated gemmae of the Mpein3 and Mpctr1 mutant alleles and the two wild-type lines on growth medium containing diluted Gamborg’s B5 salts. The normal growth medium consists of half-strength (2-fold dilution) of Gamborg’s B5 salts. On medium containing 1/30, 1/40, or 1/50 dilutions of Gamborg’s B5 salts, the growth of all three genotypes was severely inhibited (Figure S4). However, on a medium containing a 1/20 dilution of Gamborg’s B5 salts, growth of wild-type and Mpctr1 plants was only partially affected, whereas growth of Mpein3 plants was inhibited to a much greater extent, as shown by the relative growth inhibition (Figures 3A–C). These findings suggested that ethylene signaling provides a degree of protection against mild nutrient deficiency.
Figure 3 Mpein3 mutants are more sensitive to nutrient deficiency than Mpctr1 mutants or WT. (A) Representative images of 14-day old WT, Mpein3, and Mpctr1 plants grown on regular medium (Control, containing a 2-fold dilution of Gamborg’s B5 salts) or containing a 1/20 dilution of Gamborg’s B5 salts. (B) Average size (ground cover area) of WT, Mpein3, and Mpctr1 plants grown on regular medium and medium containing a 1/20 dilution of Gamborg’s B5 salts for 14 days. Bars show the overall mean ± SD per genotype. Each data point represents the mean per WT line or mutant allele ± SD. n=20 WT (10 each of WT1 (white), WT2 (grey)); n=30 Mpein3 (10 each of L1 (light pink), L3 (pink), L5 (magenta)); n=30 Mpctr1 (10 each of L1 (light blue), L3 (turquoise), L4 (dark blue)). *** = P<0.0001. P values were determined using a two-tailed t-test. (C) Relative ground cover area (based on the data in B) shown as a percentage of the corresponding control for each genotype. Bars show the mean ± SEM. Different letters indicate significant difference at P< 0.05, determined using one way ANOVA followed by Tukey’s HSD post hoc test (P<0.0001). (D) Relative density of gemma cups (for the plants grown on 1/20 Gamborg’s B5 salts in B) shown as a percentage of the corresponding control for each genotype. (Absolute values are shown in Table S1). Bars show the mean ± SEM. Different letters indicate significant difference at P< 0.05, determined using one way ANOVA followed by Tukey’s HSD post hoc test (P<0.0001).
In Mpctr1 and wild-type plants, the 1/20 dilution of Gamborg’s B5 salts interestingly promoted the earlier formation of gemma cups compared to the control conditions and resulted in a higher number of gemma cups per ground cover area compared to the control (Figure 3D and Table S1). Gemma cups in control plants began to be visible on day 12 in Mpctr1 plants and day 14 in the wild type. With 1/20 dilution of Gamborg’s B5 salts, the Mpctr1 and wild-type plants showed gemma cup formation on days 10 and 12, respectively. In contrast, Mpein3 plants produced no gemmae cups under this stress, as seen for the 29 °C and 10 mM NaCl treatments.
At higher dilutions (1/30 and above), the center of the plants turned red, consistent with the induction of flavonoid production by nutrient deficiency in M. polymorpha (Albert et al., 2018).
In angiosperms, a high level of continuous far-red light relative to red light exerts various effects on plant morphology and, in some cases, results in longer, narrower leaves (Tan et al., 2022). Here we incubated gemmae of Mpein3 and Mpctr1 mutants and the wild type under normal white light supplemented with or without continuous far-red light (60 μmol m-2s-1) (<0.15 R:FR ratio). Between 21 days and 50 days of treatment, the wild type and Mpctr1 plants appeared to have longer, narrower thalli that were more highly branched (Figure 4A). In contrast, Mpein3 mutants displayed a highly compact morphology with short, rounded thalli (Figure 4A). By 50 days under the far-red light treatment, the Mpein3 thalli had largely turned brown, whereas the wild type and Mpctr1 plants remained mostly green (Figure 4A).
Figure 4 Mpein3 mutants are more sensitive to continuous far-red light than Mpctr1 mutants or WT. (A) Representative images of WT, Mpein3, and Mpctr1 plants at 21 and 50 days of growth under continuous far-red light (in combination with normal white light). Each Petri dish (100 mm diameter) contains four plants. (B) Average chlorophyll content of 41-day old WT, Mpein3, and Mpctr1 plants grown under continuous white light (control) with and without continuous far-red light. Bars show the overall mean ± SD per genotype. Each data point represents the mean per WT line or mutant allele ± SD. n=4 WT (2 each of WT1 (white), WT2 (grey)); n=6 Mpein3 (2 each of L1 (light pink), L3 (pink), L5 (magenta)); n=6 Mpctr1 (2 each of L1 (light blue), L3 (turquoise), L4 (dark blue)). P values were determined using a two-tailed t-test. N.S., not significant. (C) Relative chlorophyll content (based on the data in B) shown as a percentage of the corresponding control for each genotype. Bars show the mean ± SEM. Different letters indicate significant difference at P< 0.05, determined using one way ANOVA followed by Tukey’s HSD post hoc test (P<0.0001) (P value for WT versus Mpctr1 = 0.051).
Measurement of chlorophyll content in 41-day old plants with and without far-red light treatment showed that wild-type and Mpctr1 plants had a higher level of chlorophyll that was not significantly reduced by far-red light treatment, whereas Mpein3 plants had a lower level of chlorophyll that was reduced by 44%. (Figures 4B, C). These results suggested that ethylene signaling plays a role in far-red light tolerance in M. polymorpha.
Given that ethylene signaling plays a role in protection against the above stresses, we hypothesized that abiotic stress would induce ethylene biosynthesis. To test this, we treated 10-day old wild-type plants separately with and without high temperature (29 °C), salt (10 mM NaCl), nutrient deficiency (20-fold dilution of Gamborg’s B5 medium), or continuous far-red light and then measured ethylene production. Plants were treated individually in an airtight vial on liquid medium, and after 48 hours of treatment, we measured the concentration of ethylene in the headspace by gas chromatography. All three stress conditions (29 °C, 10 mM NaCl, and nutrient deficiency) showed an increase in ethylene levels compared to the controls without stress treatment (Figure 5A). While not necessarily considered an abiotic stress, the treatment with continuous far-red light also led to ethylene production (Figure 5B). These results show that environmental stresses can induce ethylene biosynthesis in M. polymorpha, consistent with our data indicating that ethylene signaling promotes resilience under abiotic stress.
Figure 5 Abiotic stress enhances ethylene production in M. polymorpha. Ethylene production was measured in 10-day old wild-type plants after 48 hr incubation under (A) elevated temperature (29°C), salinity (10 mM NaCl), or nutrient deficiency (1/20 dilution of Gamborg’s B5 salts) (with vials incubated upright) or (B) under white light plus far-red light (with vials incubated on their sides). Bars show the overall mean ± SD per genotype. Each data point represents the mean per wild-type line. n=10 (5 each of WT1 (white), WT2 (grey)). *** = P<0.0001. P values were determined using a two-tailed t-test.
Our results indicate that ethylene serves as a stress hormone in the liverwort M. polymorpha. Specifically, we found that ethylene biosynthesis increases when M. polymorpha is under abiotic stress, and that ethylene signaling provides a degree of protection against abiotic stress. We base these conclusions on mild or sub-lethal doses of abiotic stresses that inhibit M. polymorpha growth: heat (29 °C), salt (10 mM NaCl), nutrient deficiency (20-fold dilution of Gamborg’s B5 salts), and continuous far-red light. Under these conditions, the growth of Mpctr1 constitutive ethylene response mutants and wild-type plants was only partially inhibited, whereas growth of Mpein3 ethylene-insensitive mutants was substantially inhibited, indicating that ethylene signaling through MpCTR1 and MpEIN3 plays a role in stress resilience. In A. thaliana, AtEIN3 activates several downstream transcription factors to initiate basal thermotolerance (Huang et al., 2021), and the stabilization of AtEIN3 under salt stress prevents ROS accumulation leading to salt tolerance (Peng et al., 2014). Similarly, AtEIN3 plays a role in ethylene-mediated responses to nutrient deficiency (García et al., 2021). Our findings suggest that ethylene signaling may have conserved functions in abiotic stress signaling in M. polymorpha.
Under heat and nutrient deficiency, the wild type displayed essentially the same extent of stress tolerance (i.e., growth) as the Mpctr1 mutants. This observation is expected because these abiotic stresses enhance ethylene production in the wild type (Figure 5), and the concomitant increase in ethylene signaling likely results in a response that equals the constitutive ethylene response conferred by the Mpctr1 mutant alleles. Under mild salt stress, the Mpctr1 mutants actually displayed greater resilience than the wild type. While the underlying mechanisms for this result are unknown, this illustrates the opposing roles of MpCTR1 and MpEIN3 as negative and positive regulators of abiotic stress responses, respectively.
Interestingly, the growth of M. polymorpha was enhanced at 27 °C and 28 °C, even in the Mpein3 mutants. This faster growth might result from biological processes being sped up at higher temperatures. We speculate that prolonged exposure to 27 °C or 28 °C (beyond our experimental timeframe) might eventually be detrimental to growth. Given that constant exposure to 30 °C severely limited growth of all genotypes, while constant exposure to 27 °C or 28 °C enhanced growth of all genotypes, ethylene appears to play a role within a narrow window of heat stress in M. polymorpha under the growth conditions we used. We also observed that nutrient deficiency enhanced production of gemma cups in both the wild type and the Mpctr1 mutants.
We also examined the role of ethylene signaling in M. polymorpha in response to continuous far-red light (<0.15 R:FR ratio). Far-red light evokes several physiological responses but is not necessarily considered an abiotic stress. In fact, the removal or reduction of phytochrome activity has been shown to enhance resilience under stress in both A. thaliana (Yang et al., 2016) and tomato (Cao et al., 2018). In the laboratory, far-red light is used to induce sexual reproduction in M. polymorpha (Ishizaki et al., 2016). Far-red light, which is enriched beneath a canopy, can lead to extension growth as a shade avoidance response (Pierik et al., 2009; Tan et al., 2022). When treated with far-red light, we observed morphological changes (elongation, narrowing, and more branching) of thalli in the wild type and Mpctr1. These changes conceivably comprise a shade avoidance response. Given that the Mpein3 mutants lacked this response to far-red light, the response appears to involve ethylene signaling and is perhaps related to the role of ethylene in promoting plant growth in M. polymorpha (Li et al., 2020). Such growth could occur through increased cell expansion and cell division, as shown for epidermal cells of ethylene-treated gemmae (Li et al., 2020). Alternatively, there could be a direct interaction between the phytochrome signaling pathway and EIN3 as in A. thaliana (Shi et al., 2016).
All four of the stresses we tested enhanced ethylene biosynthesis. While M. polymorpha clearly synthesizes ethylene, we note that the ethylene biosynthesis pathway has yet to be identified in M. polymorpha and in non-seed plants in general (Li et al., 2022). In angiosperms, the ethylene biosynthesis pathway is well known and involves the conversion of 1-aminocyclopropane-1-carboxylic acid (ACC) to ethylene by the enzyme ACC oxidase. Non-seed plants, however, lack the genes for ACC oxidase (Li et al., 2018). At best, ACC is a weak ethylene precursor in M. polymorpha (Li et al., 2020).
In angiosperms, stress-induced ethylene production and signaling lead to the activation of multiple stress-responsive pathways, often via Ethylene Response Factor (ERF) transcription factors (Debbarma et al., 2019). Abiotic stressors such as salinity, drought, heat, flooding, and nutrient stress all lead to a characteristic burst in ROS levels (Choudbury et al., 2017) and a drop in photosynthesis (Sharma et al., 2020). Drought, salt and heat stress also activate osmotic responses which entail the production of heat-shock proteins, osmolytic proteins and osmolytes (Hasanuzzaman et al., 2013; van Zelm et al., 2020), while nutrient and salinity stress trigger the synthesis of ion transporters (van Zelm et al., 2020). Whether these stress responses are activated in M. polymorpha in an ethylene-dependent manner remains to be studied, but initial genomic and transcriptomic analyses of salt- and heat-stressed M. polymorpha plants revealed that genes involved in oxidative stress (ROS metabolism) were conserved and enriched (Tanaka et al., 2018; Marchetti et al., 2021). Additionally, transcriptional changes in ethylene signaling genes have been observed under conditions of phosphate starvation in M. polymorpha (Rico-Resendiz et al., 2021), although the phenotypic consequences remain unknown.
Our findings in M. polymorpha indicate that this stress-related role of ethylene production and signaling likely predated the divergence of the bryophytes at least 450 million years ago from the land plant lineage. The role of ethylene as a stress hormone in bryophytes was previously reported for an ethylene-mediated submergence (flooding) response in the moss Physcomitrella patens (Yasumura et al., 2012). During flooding, wild-type P. patens plants alter their developmental programming towards colonizing behavior, and this escape strategy is dependent on ethylene signaling, given that an ethylene-insensitive receptor mutant was found to be defective in this response (Yasumura et al., 2012). Key components of the ethylene signaling pathway have been shown to be functionally conserved in the Charophycean alga Spirogyra pratensis (Ju et al., 2015), and therefore it is possible that ethylene signaling could have facilitated stress resilience prior to the evolutionary transition to land. Connections between abiotic stress and ethylene signaling have been observed in S. pratensis without detectable increases in ethylene biosynthesis under various stress conditions (Van de Poel et al., 2016). Ethylene might have played a role in facilitating the transition from an aquatic habitat to a terrestrial habitat to help plants deal with the environmental stresses of life on land, such as drought, heat, and UV radiation. Our findings demonstrate that ethylene biosynthesis and signaling play a role in abiotic stress resilience in M. polymorpha, indicating that ethylene has likely been conserved as a stress hormone for at least 450 million years of plant evolution.
While we have shown that ethylene plays a role in responses to abiotic stress in M. polymorpha, an interplay with other signaling pathways is likely to be involved (Verma et al., 2016; Dubois et al., 2018). Charophycean algae also contain stress-related pathways that enable them to adapt to changing environmental conditions (Holzinger et al., 2014; Hori et al., 2014; de Vries et al., 2018; de Vries et al., 2020). In M. polymorpha, tolerance to heat stress is linked to abscisic acid, jasmonic acid, and auxin signaling (Marchetti et al., 2021), and coordination of growth and oxidative stress response in M. polymorpha involves the conserved DELLA transcription factor (Hernández-García et al., 2021). Unraveling these stress response networks and their interaction with ethylene will be valuable for inferring which pathways may have been present in the ancestor of early land plants and for developing ways to mitigate the detrimental effects of abiotic stresses that are increasingly prevalent due to climate change.
The original contributions presented in the study are included in the article/Supplementary Material. Further inquiries can be directed to the corresponding author.
CC, PB, and LS conceived and designed the experiments. LS and DE carried out heat stress experiments. LS and DL carried out salt stress experiments. PB carried out nutrient deficiency experiments, chlorophyll measurements, and ethylene production assays and prepared the figures. DL carried out far-red light and salt stress experiments in BV’s lab. CC and PB wrote the manuscript with assistance from all of the authors. All authors contributed to the article and approved the submitted version.
This work was supported by an NSF grant (MCB-1714993) to CC and a VLAIO grant (HBC.2020.3207) and KU Leuven grant (C14/18/056) to BV. CC is supported in part by the Maryland Agricultural Experiment Station.
We thank Tom Dierschke (Monash University, Australia) in Dr. John L. Bowman’s laboratory for sharing his observation that the Mpein3 mutants are hypersensitive to far-red light. We thank Dr. Jianhong Chang for helpful discussions on statistical analyses. We also thank Husan Turdiev (Chang lab) for assistance with photographing M. polymorpha and Stijn Roden (Van de Poel lab) for shipping M. polymorpha lines. We thank members of the Chang lab for comments on the manuscript.
The authors declare that the research was conducted in the absence of any commercial or financial relationships that could be construed as a potential conflict of interest.
All claims expressed in this article are solely those of the authors and do not necessarily represent those of their affiliated organizations, or those of the publisher, the editors and the reviewers. Any product that may be evaluated in this article, or claim that may be made by its manufacturer, is not guaranteed or endorsed by the publisher.
The Supplementary Material for this article can be found online at: https://www.frontiersin.org/articles/10.3389/fpls.2022.998267/full#supplementary-material
Abeles, F. B. M., Page, W., Saltveit, M. E. (1992). Ethylene in plant biology (San Diego, CA: Academic Press).
Achard, P., Cheng, H., De Grauwe, L., Decat, J., Schoutteten, H., Moritz, T., et al. (2006). Integration of plant responses to environmentally activated phytohormonal signals. Science 311, 91–94. doi: 10.1126/science.1118642
Albert, N. W., Thrimawithana, A. H., McGhie, T. K., Clayton, W. A., Deroles, S. C., Schwinn, K. E., et al. (2018). Genetic analysis of the liverwort Marchantia polymorphar eveals that R2R3MYB activation of flavonoid production in response to abiotic stress is an ancient character in land plants. New Phytol. 218, 554–566. doi: 10.1111/nph.15002
Binder, B. M. (2020). Ethylene signaling in plants. J. Biol. Chem. 295, P7710–P7725. doi: 10.1074/jbc.REV120.0
Bowman, J. L., Kohchi, T., Yamato, K. T., Jenkins, J., Shu, S., Ishizaki, K., et al. (2017). Insights into land plant evolution garnered from the Marchantia polymorpha genome. Cell 171, 287–304. doi: 10.1016/j.cell.2017.09.030
Cao, W.-H., Liu, J., He, X.-J., Mu, R.-L., Zhou, H.-L., Chen, S.-Y., et al. (2007). Modulation of ethylene responses affects plant salt-stress responses. Plant Physiol. 143 (2), 707–719. doi: 10.1104/pp.106.094292
Cao, K., Yu, J., Xu, D., Ai, K., Bao, E., Zou, Z. (2018). Exposure to lower red to far-red light ratios improve tomato tolerance to salt stress. BMC Plant Biol. 18, 92. doi: 10.1186/s12870-018-1310-9
Chao, Q., Rothenberg, M., Solano, R., Roman, G., Terzaghi, W., Ecker, J. R. (1997). Activation of the ethylene gas response pathway in Arabidopsis by the nuclear protein ETHYLENE-INSENSITIVE3 and related proteins. Cell 89, 1133–1144. doi: 10.1016/S0092-8674(00)80300-1
Chen, H., Bullock, D. A., Jr., Alonso, J. M., Stepanova, A. N. (2022). To fight or to grow: The balancing role of ethylene in plant abiotic stress responses. Plants 11, 33. doi: 10.3390/plants11010033
Choudbury, F. K., Rivero, R. M., Blumwald, E., Mittler, R. (2017). Reactive oxygen species, abiotic stress and stress combinations. Plant J. 90 (5), 856–868. doi: 10.1111/tpj.13299
Debbarma, J., Sarki, Y. N., Saikia, B., Boruah, H., Singha, D. L., Chikkaputtaiah, C. (2019). Ethylene response factor (ERF) family proteins in abiotic stresses and CRISPR–Cas9 genome editing of ERFs for multiple abiotic stress tolerance in crop plants: A review. Mol. Biotechnol. 61, 153–172. doi: 10.1007/s12033-018-0144-x
de Vries, J., Curtis, B. A., Gould, S. B., Archibald, J. M. (2018). Embryophyte stress signaling evolved in the algal progenitors of land plants. Proc. Natl. Acad. Sci. U.S.A. 115, E3471–E3480. doi: 10.1073/pnas.1719230115
de Vries, J., de Vries, S., Curtis, B. A., Zhou, H., Penny, S., Feussner, K., et al. (2020). Heat stress response in the closest algal relatives of land plants reveals conserved stress signaling circuits. Plant J. 103, 1025–1048. doi: 10.1111/tpj.14782
Dubois, M., Van den Broeck, L., Inzé, D. (2018). The pivotal role of ethylene in plant growth. Trends Plant Sci. 23, 311–323. doi: 10.1016/j.tplants.2018.01.003
Fukao, T., Bailey-Serres, J. (2008). Ethylene–a key regulator of submergence responses in rice. Plant Sci. 175, 43–51. doi: 10.1016/j.plantsci.2007.12.002
Gamborg, O. L., Miller, R. A., Ojima, K. (1968). Nutrient requirement of suspensions cultures of soybean root cells. Exp. Cell Res. 50, 151. doi: 10.1016/0014-4827(68)90403-5
García, M. J., Angulo, M., García, C., Lucena, C., Alcántara, E., Pérez-Vicente, R., et al. (2021). Influence of ethylene signaling in the crosstalk between fe, s, and p deficiency responses in Arabidopsis thaliana. Front. Plant Sci. 12. doi: 10.3389/fpls.2021.643585
Hasanuzzaman, M., Nahar, K., Alam Md, M., Roychowdhury, R., Fujita, M. (2013). Physiological, biochemical, and molecular mechanisms of heat stress tolerance in plants. Int. J. Mol. Sci. 14 (5), 9643–9684. doi: 10.3390/ijms14059643
Hernández-García, J., Sun, R., Serrano-Mislata, A., Inoue, K., Vargas-Chávez, C., Esteve-Bruna, D., et al. (2021). Coordination between growth and stress responses by DELLA in the liverwort Marchantia polymorpha. Curr. Biol. 31, P3678–3686.E11. doi: 10.1016/j.cub.2021.06.010
Holzinger, A., Kaplan, F., Blaas, K., Zechmann, B., Komsic-Buchmann, K., Becker, B. (2014). Transcriptomics of desiccation tolerance in the streptophyte green alga Klebsormidium reveal a land plant-like defense reaction. PloS One 9, e110630. doi: 10.1371/journal.pone.0110630
Hori, K., Maruyama, F., Fujisawa, T., Togashi, T., Yamamoto, N., Seo, M., et al. (2014). Klebsormidium flaccidum genome reveals primary factors for plant terrestrial adaptation. Nat. Commun. 5, 3978. doi: 10.1038/ncomms4978
Huang, J., Zhao, X., Bürger, M., Wang, Y., Chory, J. (2021). Two interacting ethylene response factors regulate heat stress response. Plant Cell 33, 338–357. doi: 10.1093/plcell/koaa026
Ishizaki, K., Nishihama, R., Yamato, K T., Kohchi, T. (2016). Molecular genetic tools and techniques for Marchantia polymorpha research. Plant Cell Physiol. 57 (2), 262–270. doi: 10.1093/pcp/pcv097
Ju, C., Van de Poel, B., Cooper, E. D., Thierer, J. H., Gibbons, T. R., Delwiche, C. F., et al. (2015). Conservation of ethylene as a plant hormone over 450 million years of evolution. Nat. Plants 1, 14004. doi: 10.1038/nplants.2014.4
Kieber, J. J., Rothenberg, M., Roman, G., Feldmann, K. A., Ecker, J. R. (1993). CTR1, a negative regulator of the ethylene response pathway in Arabidopsis, encodes a member of the raf family of protein kinases. Cell 72, 427–441. doi: 10.1016/0092-8674(93)90119-B
Larkindale, J., Knight, M. R. (2002). Protection against heat stress-induced oxidative damage in Arabidopsis involves calcium, abscisic acid, ethylene, and salicylic acid. Plant Physiol. 128, 682–695. doi: 10.1104/pp.010320
Li, F.-W., Brouwer, P., Carretero-Paulet, L., Cheng, S., de Vries, J., Delaux, P. M., et al. (2018). Fern genomes elucidate land plant evolution and cyanobacterial symbioses. Nat. Plants 4, 460–472. doi: 10.1038/s41477-018-0188-8
Li, D., Flores-Sandoval, E., Ahtesham, U., Coleman, A., Clay, J. M., Bowman, J. L., et al. (2020). Ethylene-independent functions of the ethylene precursor ACC in Marchantia polymorpha. Nature Plants 6, 1335–1344. doi: 10.1038/s41477-020-00784-y
Li, D., Mou, W., Van de Poel, B., Chang, C. (2022). Something old, something new: conservation of the ethylene precursor ACC as a signaling molecule. Curr. Opin. Plant Biol. 65, 102116. doi: 10.1016/j.pbi.2021.102116
Marchetti, F., Cainzos, M., Cascallares, M., Distéfano, A. M., Setzes, N., López, G. A., et al. (2021). Heat stress in marchantia polymorpha: Sensing and mechanisms underlying a dynamic response. Plant Cell Environ. 44, 2134–2149. doi: 10.1111/pce.13914
Naing, A. H., Campol, J. R., Kang, H., Xu, J., Chung, M. Y., Kim, C. K. (2022). Role of ethylene biosynthesis genes in the regulation of salt stress and drought stress tolerance in petunia. Front. Plant Sci. 13. doi: 10.3389/fpls.2022.844449
Peng, J., Li, Z., Wen, X., Li, W., Shi, H., Yang, L., et al. (2014). Salt-induced stabilization of EIN3/EIL1 confers salinity tolerance by deterring ROS accumulation in Arabidopsis. PloS Genet. 10, e1004664. doi: 10.1371/journal.pgen.1004664
Pierik, R., Djakovic-Petrovic, T., Keuskamp, D. H., de Wit, M., Voesenek, L. A. C. J. (2009). Auxin and ethylene regulate elongation responses to neighbor proximity signals independent of gibberellin and DELLA proteins in Arabidopsis Plant Physiol. 149, 1701–1712. doi: 10.1104/pp.108.133496
Rico-Resendiz, F., Diaz-Santana, Z. H. U., Dipp-Alvarez, M., Cruz-Hernandez, A., Bowman, J. L., Herrera-Estrella, L., et al. (2021). Phosphate starvation triggers transcriptional changes in the biosynthesis and signaling pathways of phytohormones in Marchantia polymorpha. Biol. Life Sci. Forum 4, 89. doi: 10.3390/IECPS2020-08729
Romera, F. J., Alcantara, E., de la Guardia, M. D. (1999). Ethylene production by fe-deficient roots and its involvement in the regulation of fe-deficiency stress responses by strategy I plants. Ann. Bot. 83, 51–55. doi: 10.1006/anbo.1998.0793
Sharma, A., Kumar, V., Shahzad, B., Ramakrishnan, M., Sidhu, G.P. S., Bali, S.A., et al. (2020). Photosynthetic response of plants under different abiotic stresses: A review. J. Plant Growth Regul. 39, 509–531. doi: 10.1007/s00344-019-10018-x
Shi, H., Shen, X., Liu, R., Xue, C., Wei, N., Deng, X. W., et al. (2016). The red light receptor phytochrome b directly enhances substrate-E3 ligase interactions to attenuate ethylene responses. Dev. Cell 39, 597–610. doi: 10.1016/j.devcel.2016.10.020
Tanaka, H., Suzuki, R., Okabe, N., Suzuki, T., Kodama, Y. (2018). Salinity stress-responsive transcription factors in the liverwort Marchantia polymorpha. Plant Biotechnol. 35, 281–284. doi: 10.5511/plantbiotechnology.18.0501a
Tan, T., Li, S., Fan, Y., Wang, Z., Raza, M. A., Shafiq, I., et al. (2022). Far-red light: A regulator of plant morphology and photosynthetic capacity. Crop J. 10, 300–309. doi: 10.1016/j.cj.2021.06.007
Van de Poel, B., Cooper, E. D., van der Straeten, D., Chang, C., Delwiche, C. F. (2016). Transcriptome profiling of the green alga Spirogyra pratensis (Charophyta) suggests an ancestral role for ethylene in cell wall metabolism, photosynthesis and abiotic stress responses. Plant Physiol. 172, 533–545. doi: 10.1104/pp.16.00299
van Zelm, E., Zhang, Y. X., Testerink, C. (2020). Salt tolerance mechanisms of plants. Annu. Rev. Plant Biol. 71, 403–433. doi: 10.1146/annurev-arplant-050718-100005
Verma, V., Ravindran, P., Kumar, P. P. (2016). Plant hormone-mediated regulation of stress responses. BMC Plant Biol. 16, 86. doi: 10.1186/s12870-016-0771-y
Welburn, A. R. (1994). The spectral determination of chlorophylls a and b, as well as carotenoids, using various solvents with spectrophotometers of different resolution. J. Plant Physiol. 144, 307–313. doi: 10.1016/S0176-1617(11)81192-2
Wellman, C. H., Osterloff, P. L., Mohiuddin, U. (2003). Fragments of the earliest land plants. Nature 425, 282–285. doi: 10.1038/nature01884
Yang, D., Seaton, D. D., Krahmer, J., Halliday, K. J. (2016). Photoreceptor effects on plant biomass, resource allocation, and metabolic state. Proc. Natl. Acad. Sci. U.S.A. 113, 7667–7672. doi: 10.1073/pnas.1601309113
Keywords: abiotic stress, ethylene, liverwort, heat stress, salinity, nutrient deficiency, far-red (FR) light
Citation: Bharadwaj PS, Sanchez L, Li D, Enyi D, Van de Poel B and Chang C (2022) The plant hormone ethylene promotes abiotic stress tolerance in the liverwort Marchantia polymorpha. Front. Plant Sci. 13:998267. doi: 10.3389/fpls.2022.998267
Received: 19 July 2022; Accepted: 29 September 2022;
Published: 18 October 2022.
Edited by:
M. Iqbal R. Khan, Jamia Hamdard University, IndiaCopyright © 2022 Bharadwaj, Sanchez, Li, Enyi, Van de Poel and Chang. This is an open-access article distributed under the terms of the Creative Commons Attribution License (CC BY). The use, distribution or reproduction in other forums is permitted, provided the original author(s) and the copyright owner(s) are credited and that the original publication in this journal is cited, in accordance with accepted academic practice. No use, distribution or reproduction is permitted which does not comply with these terms.
*Correspondence: Caren Chang, Y2FyZW5jQHVtZC5lZHU=
†Present address: Dongdong Li, College of Agriculture and Biotechnology, Zhejiang University, Hangzhou, China
Disclaimer: All claims expressed in this article are solely those of the authors and do not necessarily represent those of their affiliated organizations, or those of the publisher, the editors and the reviewers. Any product that may be evaluated in this article or claim that may be made by its manufacturer is not guaranteed or endorsed by the publisher.
Research integrity at Frontiers
Learn more about the work of our research integrity team to safeguard the quality of each article we publish.