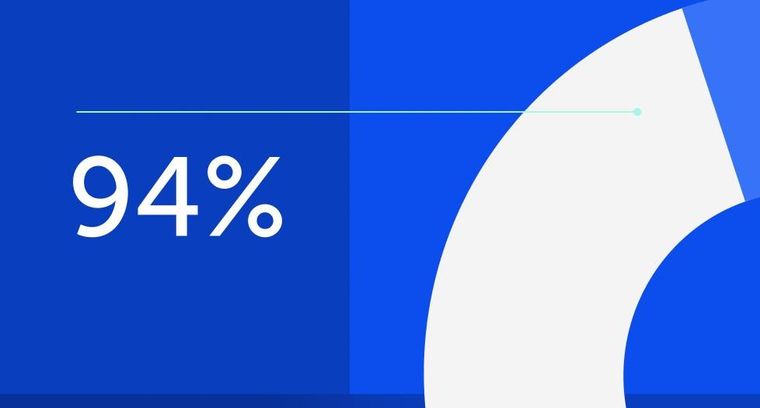
94% of researchers rate our articles as excellent or good
Learn more about the work of our research integrity team to safeguard the quality of each article we publish.
Find out more
REVIEW article
Front. Plant Sci., 14 September 2022
Sec. Plant Metabolism and Chemodiversity
Volume 13 - 2022 | https://doi.org/10.3389/fpls.2022.994058
This article is part of the Research TopicOmics Data-based Identification of Plant Specialized Metabolic GenesView all 13 articles
Vitamin E, also known as tocochromanol, is a lipid-soluble antioxidant that can only be produced by photosynthetic organisms in nature. Vitamin E is not only essential in human diets, but also required for plant environment adaptions. To synthesize vitamin E, specific prenyl groups needs to be incorporated with homogentisate as the first step of reaction. After decades of studies, an almost complete roadmap has been revealed for tocochromanol biosynthesis pathway. However, chlorophyll-derived prenyl precursors for synthesizing tocochromanols are still a mystery. In recent years, by employing forward genetic screening and genome-wide-association approaches, significant achievements were acquired in studying vitamin E. In this review, by summarizing the recent progresses in vitamin E, we provide to date the most updated whole view of vitamin E biosynthesis pathway. Also, we discussed about the role of vitamin E in plants stress response and its potential as signaling molecules.
Vitamin E (also called tocochromanols) is an essential micronutrient for humans, which is produced by phototrophs such as plants, and some algae (Falk and Munné-Bosch, 2010). As antioxidant, vitamin E can convert free radicals into less reactive compounds, playing a pivotal role in human health (Zingg, 2007). Insufficient consumption of vitamin E could cause many diseases, including cancers, Alzheimer’s disease and cardiovascular disease, but not limited (Sen et al., 2007; Falk and Munné-Bosch, 2010; Sozen et al., 2019).
Tocochromanol molecule contains a polar chromanol ring head and a prenyl side chain. According to the various types of side chains, tocochromanols can be defined as tocopherol, tocotrienol, plastochromanol-8 (PC-8) and tocomonoenol (Figure 1). Tocopherol contains fully saturated aliphatic side chain, while the side chain of tocotrienol contains three extra trans double bonds. PC-8 has similar unsaturated as tocotrienol but longer side chain, whereas tocomonoenol has only one double bond on its side chain (Tian et al., 2007; Sadre et al., 2010; Stacey et al., 2016). Based on the differences in numbers and positions of methyl groups on the chromanol ring head, tocochromanol isoforms can be classified as α, β, γ, and δ (Figure 1). While for PC-8, only δ-form was found in nature (Kamal-Eldin and Appelqvist, 1996).
Figure 1. Plant tocochromanol biosynthesis pathway and sources of metabolites. Metabolite names were colored for recognizing different tocochromanol synthesis pathways. Abbreviations of metabolites: HPP, 4-hydroxyphenylpyruvate; HGA, homogentisate; Phytyl-P, phytyl-phosphate; PDP, phytyl-diphosphate; THGGPP, tetrahydrogeranylgeranyl pyrophosphate; GGDP, geranylgeranyl pyrophosphate; SPP, solanesyl pyrophosphate; MPBQ, 2-methyl-6-phytyl-1,4-benzoquinol; DMPBQ, 2,3-dimethyl-6-phytyl-1,4-benzoquinol. Abbreviations of enzymes: TAT1, tyrosine aminotransferase 1; TAT2, tyrosine aminotransferase 2; HPPD, 4-hydroxyphenylpyruvate dioxygenase; CLD1, chlorophyll dephytylase 1; PPH, pheophytin pheophorbide hydrolase; VTE, vitamin E biosynthetic enzyme.
Tocopherols are ubiquitously synthesized in all plant species and especially abundant in photosynthetic tissues and seeds. Tocotrienols are mostly concentrated in monocot species like maize (Zea mays) embryo (Falk and Munné-Bosch, 2010), and the three extra double bonds in tocotrienols were believed to able to confer greater potential for scavenging peroxyl radicals (Suzuki et al., 1993). PC-8 was first identified in the leaves of the rubber tree and later found to be also enriched in Brassica napus, tomato fruit, and tuber of Dioscorea alata (Goffman and Mollers, 2000; Cheng et al., 2007; Zbierzak et al., 2010), and the concentrations of leaf PC-8 are differed by species and developmental stages (Whittle et al., 1965; Kruk et al., 2014). Studies of tocomonoenols suggested that they were mainly accumulated in the seed oil from palm, Slovenia pumpkin and sunflower (Matsumoto et al., 1995; Butinar et al., 2011; Hammann et al., 2015), but the exact function of tocomonoenols in planta are yet to be thoroughly verified.
In the past few decades, Arabidopsis thaliana has been employed as a plant model for dissecting tocopherol function and its biosynthesis pathway, and many key VTE (ViTamin E) enzymes were identified. Recently, by applying EMS-based forward genetic screening and genome-wide association study (GWAS), several key chlorophyll metabolic enzymes were identified to be required for tocopherol biosynthesis, including POR, CLD1 and VTE7 etc. (Lin et al., 2016; Diepenbrock et al., 2017; Wang et al., 2018; Hershberger et al., 2022; Wu et al., 2022). In this review, we summarize the recent progresses in tocochromanol biosynthesis, discussing the role of tocochromanol in plant stress response, and its potentials in signal transduction.
To produce tocochromanols, homogentisate (HGA) will be condensed with different prenyl chains by homogentisate phytyltransferase [HPT, also named VTE2 (ViTamin E 2 loci)], in one-to-one ratio (Figure 1). HPT genes can be found in all green plants, and some algae including cyanobacterium Synechocystis (Collakova and DellaPenna, 2001; Savidge et al., 2002). For tocopherols, HGA and phytyl diphosphate (PDP) are catalyzed by VTE2 to generate 2-methyl-6-phytyl-1,4-benzoquinol (MPBQ). VTE2 can also use the tetrahydrogeranylgeranyl pyrophosphate (THGGPP) to generate 2-methyl-6-tetrahydrogeranylgeranyl-1,4-benzoquinol (MTHGGBQ) for producing tocomonoenol in Arabidopsis seeds (Pellaud et al., 2018). In monocots, homogentisate geranylgeranyl transferases (HGGTs) are seed-specific and plastid-targeted, which can condense geranylgeranyl pyrophosphate (GGDP) instead of PDP with HGA to generate 2-methyl-6-geranylgeranyl-1,4-benzoquinol (MGGBQ) for tocotrienol biosynthesis (Cahoon et al., 2003; Yang et al., 2011). Although HGGT and VTE2 are close in structure, their enzyme activities toward different substrates vary quite much. For example, the activity of barley HPPT toward GGDP is 6 times higher than that of PDP; the VTE2 enzyme confers 9 times higher activity toward PDP than that of GGDP. Interestingly, barley HPPT can restore the levels of both tocopherols and tocotrienols in Arabidopsis vte2 mutant (Yang et al., 2011). Moreover, homogentisate solanesyltransferase (HST) can condense HGA and solanesyl pyrophosphate (SPP) to produce the 2-methyl-6-solanesyl-1,4-benzoquinol (MSBQ), the precursor of PC-8 (Sadre et al., 2006; Tian et al., 2007).
The downstream of vitamin E biosynthesis pathway is divided into two branches. In one branch, the MPBQ, MGGBQ, MSBQ, and MTHGGBQ are methylated by a methyltransferase (MT, VTE3) to produce DMPBQ, DMGGBQ, DMTHGGBQ, and DMSBQ (PQ-9), respectively, the precursors of α- and γ-tocochromanols (Cheng et al., 2003; Van Eenennaam et al., 2003). Then the methylated compounds are cyclized by tocopherol cyclase (TC, VTE1) to produce γ-tocochromanols (Cheng et al., 2003). In the other branch of this pathway, the demethylated compounds (MPBQ, MGGBQ, MSBQ, and MTHGGBQ) are cyclized directly by VTE1 to produce δ-tocochromanols (Cheng et al., 2003). In the final step, the γ- and δ-tocochromanols, respectively, are methylated to produce α- and β-tocochromanols by γ-tocopherol methyltransferase (γ-TMT, VTE4) (Shintani and DellaPenna, 1998; Bergmüller et al., 2003).
Biosynthesis of tocochromanols is mainly carried out in the plastid, but one of its main precursors HGA, which provides the chromanol ring head for tocochromanols, is produced during L-tyrosine (Tyr) degradation in the cytoplasm (Figure 1). Through the shikimate pathway, tyrosine aminotransferases (TATs) catalyze reversible reaction between Tyr and 4-hydroxyphenylpyruvate (HPP), and at least two homologous genes TAT1 and TAT2 were identified in the genome of Arabidopsis thaliana (Siehl et al., 2014; Stacey et al., 2016; Wang et al., 2016). Arabidopsis TAT1 gene loss of function mutant showed reduced tocopherols under normal condition (Riewe et al., 2012), but the tat2 single mutants have no effect on Tyr and tocopherol levels. In the tat1 tat2 double mutants, compared with wild-type and tat single mutants, more Tyr were accumulated and fewer tocopherols were maintained, and this effect was amplified under high-light stress (Wang et al., 2019). TAT1 and TAT2 thus work redundantly in Tyr degradation and tocopherol biosynthesis, with TAT1 playing a major role. Then, HPP is converted to HGA by the 4-hydroxyphenylpyruvate dioxygenase (HPPD), which is encoded by a single-copy gene in Arabidopsis (Tsegaye et al., 2002). However, HPPDs possess different subcellular localizations according to studies in various plant species (Pellaud and Mène-Saffrané, 2017). For example, HPPD proteins of Spinacia oleracea, Lemna gibba and maize are targeted to plastids, while in carrot and Arabidopsis they are located in the cytoplasm (Löffelhardt and Kindl, 1979; Fiedler et al., 1982; Garcia et al., 1997; Siehl et al., 2014; Wang et al., 2016).
Another main precursor of tocopherols comes from phytyl diphosphate (PDP), which is generated from two steps of phosphorylation relay using phytol (Gutbrod et al., 2019). The first step is to generate phytyl-phosphate via VTE5 (phytol kinase), which accounts for 80% and 50% total tocopherol biosynthesis in Arabidopsis seeds and leaves, respectively (Valentin et al., 2006). The second step is executed by VTE6 (phytyl phosphate kinase), and due to over accumulation of phytyl-phosphate, vte6 mutant plant confers severe growth defects. By introducing vte5 into the vte6 background, the vte5 vte6 double mutant resembles wild-type in plant growth, but showing tocopherol deficient and high levels of accumulated chlorophylls (Vom Dorp et al., 2015). GGDP, SPP and THGGPP are the polyprenyl chain precursors of tocotrienols, PC-8 and tocomonoenols, respectively (Mène-Saffrané, 2018). Of note, all of the four tocochromanol isoprenoid side chains can be produced by GGDP synthases (Pellaud and Mène-Saffrané, 2017).
However, accumulated studies have suggested that the phytol group used for tocopherol biosynthesis mostly comes from the chlorophyll degradation (Valentin et al., 2006; Gutbrod et al., 2019). Thus, finding the relevant hydrolases that can bring down phytol group from chlorophyll and/or chlorophyll derivatives is key for dissecting tocopherol biosynthesis pathway. The first chlorophyllase (CLH) was isolated from Citrus sinensis, which shows activity toward chlorophylls, and two CLH genes (AtCLH1 and AtCLH2) were found in Arabidopsis (Jacob-Wilk et al., 1999; Tsuchiya et al., 1999). The highest transcripts of AtCLH1 and AtCLH2 were found in young leaves and their levels decline gradually during leaf maturation (Chen et al., 2014; Tian et al., 2021). However, previous studies had shown that the two Arabidopsis CLHs were not required for chlorophyll breakdown during leaf senescence. In addition, CLH proteins are targeted to vacuole instead of chloroplasts. Based on functional genomic approach with the features of senescence-related regulation and chloroplast targeting, Schelbert et al. identified a hydrolase called pheophytin pheophorbide hydrolase (PPH) in Arabidopsis. The pph mutant showed stay-green phenotype during senescence, compared with its wild-type control. In vitro, PPH confers specific activity toward pheophytin but not chlorophylls (Schelbert et al., 2009). Recently, through EMS screening for Arabidopsis heat-sensitive progenies, an Arabidopsis CHLOROPHYLL DEPHYTYLASE1 (CLD1) gene was identified. A G-to-A transition at position 957 of cld1 gene results in the replacement of Gly-193 by Asp (G193D), which confers much higher activity of cld1 toward both chlorophylls and pheophytin than that of its native CLD1 (Lin et al., 2016). Following studies found about 15% tocopherol increase in CLD1 and cld1 overexpression plants, but no significant difference in its miRNA lines (Lin and Charng, 2017). Although CLHs, PPH and CLD1 can cleave phytol directly from chlorophyll and/or pheophytin, none of the single or high order mutants (clh1/clh2, pph, and pph/clh1/clh2) significantly affect tocopherol contents. Moreover, overexpression of the four genes only moderately increased the levels of tocopherol, suggesting the relevant alpha/beta hydrolase is yet to be identified (Zhang et al., 2014; Lin and Charng, 2017). Recently, GWAS of seed tocopherols was applied using 814 Arabidopsis natural variation lines (part of the 1001 Arabidopsis Genome Panel, Alonso-Blanco et al., 2016), a novel seed-specific alpha/beta hydrolase gene AtVTE7 was identified. AtVTE7 is targeted to the chloroplast envelope and accounts for 55% of total seed tocopherols. Consistent with the results in Arabidopsis, the maize orthologous gene ZmVTE7 controls 38% and 49% total tocopherols in kernel and leaf, respectively (Albert et al., 2022). Of note, Arabidopsis AtVTE7 is only detected in seed, does not affect tocopherol trait in leaf. Although VTE7 can provide phytol from chlorophyll degradation for tocopherol biosynthesis, this enzyme mainly affects the levels of chlorophyll biosynthetic intermediates, instead of bulk chlorophyll levels (Albert et al., 2022).
In addition to the alpha/beta hydrolases that are directly involved in phytol production, many chlorophyll-metabolism-related genes also contribute to tocopherol homeostasis. For instance, plant NYE [Non-Yellowing, also named SGR (Stay-Green)] are chloroplast-localized Mg-dechelatase proteins, and during chlorophyll degradation NYEs closely cooperate with other chlorophyll catabolic enzymes including PPH (Armstead et al., 2007; Sato et al., 2007; Zhang et al., 2014). Indeed, the seed tocopherol levels of the Arabidopsis double mutant nye1 nye2 modestly reduced, compared with that in the wild-type (Zhang et al., 2014). Chlorophyll synthesis needs the esterizing of chlorophyllide with either GGDP or PDP. The RNAi lines of CHLOROPHYLL SYNTHASE (CHLSYN) exhibit significantly reduced chlorophylls but up to 2 times increased tocopherols (Zhang et al., 2015). Moreover, two QTLs that encode homologs of protochlorophyllide reductase (POR1 and 2) were revealed in maize, and the two por loci had the highest phenotypic variance explained for all four forms of tocopherols calculated (Diepenbrock et al., 2017; Wang et al., 2018). Thus, disturbing the genes involved chlorophyll metabolism can affect tocopherol biosynthesis, suggesting a precise mechanism for balancing chlorophyll metabolism and tocopherol biosynthesis in plants.
As important antioxidants, tocochromanols can be boosted to high levels during various biotic and abiotic stresses (Figures 2, 3) (Bao et al., 2020). Meanwhile, plants with low levels of tocochromanols are more susceptible to different stressful treatments, suggesting a crucial role of vitamin E in plant environment adaptions.
Figure 2. Representative HPLC traces of Arabidopsis leaf tocopherols subjected to different growth conditions (high light, heat, and dark). Tocol is used as internal standard [based on Bao et al. (2020), with modification].
Figure 3. A proposed working model of the vitamin E in signal transduction Chilling stress can be sensed by COLD1 to regulate calcium signal and tocochromanol homeostasis for transcriptional reprogramming in the nucleus. Other stresses including high-light and heat can also transduce retrograde signaling between chloroplast and nucleus for miRNA biogenesis, via manipulating PAP (3′-phosphoadenosine-5′-phosphate) and tocochromanols.
Temperature and light intensity are the two key environmental factors that can affect crop yield (Pretty et al., 2010). During high light and heat stress, tocopherols (especially α-tocopherol) are induced to accumulate at high levels, and elevated α-tocopherols were believed to be required for protecting photosystem from savaging singlet oxygen and maintaining the stability of chloroplast (Kruk et al., 2005). Indeed, tocopherol deficient Arabidopsis mutants are more vulnerable to high light (Kobayashi and DellaPenna, 2008). When grown under low temperature, vitamin E deficient mutants are retarded in plant growth (Maeda et al., 2006), which mainly attributes to defects in phloem loading, coincide with the findings in maize and potato (Solanum tuberosum) (Russin et al., 1996; Hofius et al., 2004). The combination of high light and low temperature causes strong lipid peroxidation and photooxidative stresses to Arabidopsis, and this effect was exemplified in vte1 mutant (Havaux et al., 2005). In maize, compared with high temperature, more tocopherols and tocotrienols were produced under low temperature (Xiang et al., 2019). In addition, tomato SlVTE5 silenced plants display a strong chlorotic phenotype with low levels of α-tocopherol under the stress combined with high-light and high-temperature (Spicher et al., 2017). Moreover, high-light stress also triggers the expression of HPPDs in Medicago sativa and Lactuca sativa for counteracting and survival strategies (Ren et al., 2011; Jiang et al., 2017).
Drought is one of the most common stresses in limiting farming, which leads to significant yield losses (Godwin and Farrona, 2020). The capacity of HPPDs to resist drought has also been demonstrated in various plant species, including Lactuca sativa, Medicago and sweet potato (Ren et al., 2011; Jiang et al., 2017; Kim et al., 2021). In rice, OsVTE1 is induced to significantly high levels under drought stress (Ouyang et al., 2011), and ectopic overexpression of AtVTE1 in tobacco can enhance tolerance to drought stress via reducing lipid peroxidation, electrolyte leakage and H2O2 content (Liu et al., 2008). Moreover, overexpression of MsVTE4 increases the levels of both α-tocopherols and total tocopherols in alfalfa, alleviating oxidative damages, leading to higher tolerance to drought stress (Ma et al., 2020).
Soil with unfavorable high level of soluble salts causes salinity stress in plants, limiting crop yield and the area for farming. In tobacco (Nicotiana tabacum) VTE2 silenced plants, total tocopherols decreased 98%, and ion homeostasis was disturbed with sorbitol and methyl viologen treatment (Abbasi et al., 2007). Meanwhile, in tobacco VTE4 silenced plants, α- and γ-tocopherols were found to play diversified roles in plant stress tolerance (Abbasi et al., 2007). On the other hand, overexpressing AtVTE4 can reduce superoxide contents, lipid peroxidation and ion leakage under salt stress (Jin and Daniell, 2014). By employing Arabidopsis tocopherol deficient mutants vte1 (deficient in α- and γ-tocopherols) and vte4 (over accumulation of γ-tocopherols), Surówka et al. (2020) revealed that α-tocopherols had stronger regulatory effect than γ-tocopherols through modulating chloroplast biosynthesis pathways and ROS/osmotic-associated compounds under salt stress. Based on the studies above, AtVTE2 and AtVTE4 have been engineered in potato breeding for counteracting heavy metal stress (Upadhyaya et al., 2021).
Tocochromanols not only protect plant from abiotic stress, but also contribute to the resistance of biotic stress. Pseudomonas syringae can infect a wide range of plant species and leads to serious economic loss (Bohlmann and Keeling, 2008), which is served as a model for dissecting the mechanism of plant-pathogen interactions (Xin et al., 2018). Arabidopsis vte2 mutants exhibit stronger lipid peroxidation and produce less SA (salicylic acid, a key phytohormone in transducing pathogen signal), resulting in susceptibility to P. syringae (Stahl et al., 2019). As a necrotrophic fungus, Botrytis cinereal infects many important plant species, including Arabidopsis (Staats et al., 2005). Through detailed analysis and comparison of lipid and hormone changes in B. cinereal-infected Arabidopsis vte1 and vte4 mutants, Cela et al. found that altered tocopherol compositions could reduce plant tolerance and delay the activation of defense pathway (Cela et al., 2018).
Vitamin E has long been assessed and studied as an antioxidant, but emerging evidences strongly suggested that vitamin E may also serve as signaling molecules in plants. Reactive oxygen species (ROS) such hydroperoxide and single oxygen that produced in the photosystem have been shown as important signals in the communications between chloroplast and gene expression in the nucleus (Figure 3) (Foyer and Noctor, 2003, 2005). When single oxygen accumulated in the chloroplast, tocopherols will be oxidized to produce tocopheryl-radical and hydroperoxide. Reversibly, these two products can be reduced to tocopherols by introducing ascorbate (also known as vitamin C) (Neely et al., 1988). Thus, through eliminating ROS, vitamin E maintains chloroplast redox state and modulates retrograde signaling from the chloroplast to nucleus (Figure 3) (Krieger-Liszkay and Trebst, 2006).
Cross-talks between vitamin E and phytohormones were also revealed. For instance, tocopherol deficient vte1 mutants accumulate more jasmonic acid (JA) and anthocyanin than that of wide-type, causing growth retardation in both high-light and low temperature conditions (Munné-Bosch et al., 2007). During low phosphate (Pi) treatment, both vte1 and vte4 mutants accumulate more JA and SA than that in the wide-type. In addition, the expression levels of over 500 transcription factors are significantly affected in vte1 and vte4 plants, indicating that tocopherols are involved in phytohormone signaling and transcriptional reprogramming (Allu et al., 2017). In another case, ethylene-responsive cis elements were found in the promoter region of Mango (Mangifera indica) MiHPPD gene. During fruit ripening and leaf senescence, elevated endogenous ethylene can induce MiHPPD expression, giving rise to tocopherols contents (Singh et al., 2011). Overall, interactions and communications between vitamin E and phytohormones contribute to plant environment adaptations via fine-tuning downstream gene expressions.
The quantitative trait locus COLD1 was identified recently in japonica rice that can confer tolerance to chilling stress. For sensing low temperature, COLD1 can interact with RGA1 (G-protein α subunit) to activate the Ca2+ channel and accelerate GTPase activity of G-protein. Influx of calcium, an important intracellular second messenger, activates gene transcription of vitamin E and vitamin K1 biosynthesis pathways, promoting plant cold tolerance (Luo et al., 2021). Another tocopherol-mediated chloroplast-to-nucleus signaling event was discovered during the study of heat stress-associated microRNA (miRNA) biogenesis. The metabolite 3′-phosphoadenosine-5′-phosphate (PAP) has been broadly racialized as crucial secondary messenger in plant stress responding. Heat stress promotes accumulation of tocopherols, and in turn produces more PAP, which inhibits the nuclear exoribonucleases (XRN), stimulating the biogenesis of microRNAs including miR398 to enhance plant heat tolerance (Fang et al., 2019).
Studies in human and animals suggested that tocopherol-binding protein (TBP) is important for the distribution and transport of α-tocopherol among different tissues. In the latest research, Bermúdez et al. identified the SlTBP (Solanum lycoperisicum tocopherol-binding protein) as a homolog of the human α-tocopherol transfer protein (HsTTP). In vitro biochemical assay suggested that SlTBP possesses α-tocopherol binding ability. SlTBP is chloroplast-targeted, and knocking down SlTBP expression in tomato confers disorders in tocopherol, carotenoid and lipid compositions. Finding of TBP in plants sheds light on understanding vitamin E transport, implying its potential as signaling molecules (Bermúdez et al., 2018).
Studies in Arabidopsis indicated that 90% and more of the total tocopherols (∼5 ng/mg fresh weight) in leaves are α-tocopherol, while in Arabidopsis seeds, tocopherols (∼370 ng/mg dry seed) are dominated by the γ isoform. Crucial physiological functions of vitamin E for plants were exemplified by the observation that tocopherol defective Arabidopsis mutants were severely affected in seed longevity, germination and seeding growth (Sattler et al., 2004). Differential regulation of the same cassette of genes for tocochromanol biosynthesis in different tissues warrants for future explorations. Chlorophyll and its relevant derivatives are the most abundant pigments in green plants, and accumulated evidences suggested that chlorophyll-derived phytol groups are the main source for vitamin E biosynthesis (Gutbrod et al., 2019). VTE7 is a novel alpha/beta hydrolase that fits in the missing gap between chlorophyll metabolism and vitamin E production, accounting for more than 50% of total tocopherol biosynthesis in seeds. However, its exact targets still need to be verified. In recent years, tocopherols were found to be involved in both cold response and PAP-mediated retrograde signal transduction. In addition, tocopherol binding protein (TBP) was identified in tomato. Thus, role of tocopherols in acting as signaling transducers are promising and deserved to be investigated in depth. More importantly, as an essential nutrient, engineering balanced vitamin E in crops like soybean, rapeseed will advance plant breeding and benefit human health.
YB concepted the topic of this manuscript and revised the manuscript. YN and QZ drafted the manuscript with YB. All authors contributed to the article and approved the submitted version.
This work was supported by China Agriculture Research System of MOF and MARA.
The authors declare that the research was conducted in the absence of any commercial or financial relationships that could be construed as a potential conflict of interest.
All claims expressed in this article are solely those of the authors and do not necessarily represent those of their affiliated organizations, or those of the publisher, the editors and the reviewers. Any product that may be evaluated in this article, or claim that may be made by its manufacturer, is not guaranteed or endorsed by the publisher.
Abbasi, A. R., Hajirezaei, M., Hofius, D., Sonnewald, U., and Voll, L. M. (2007). Specific roles of alpha- and gamma-tocopherol in abiotic stress responses of transgenic tobacco. Plant Physiol. 143, 1720–1738. doi: 10.1104/pp.106.094771
Albert, E., Kim, S., Magallanes-Lundback, M., Bao, Y., Deason, N., Danilo, B., et al. (2022). Genome-wide association identifies a missing hydrolase for tocopherol synthesis in plants. Proc. Natl. Acad. Sci. U.S.A. 119:e2113488119. doi: 10.1073/pnas.2113488119
Allu, A. D., Simancas, B., Balazadeh, S., and Munné-Bosch, S. (2017). Defense-related transcriptional reprogramming in Vitamin E-Deficient Arabidopsis mutants exposed to contrasting phosphate availability. Front. Plant Sci. 8:20. doi: 10.3389/fpls.2017.01396
Alonso-Blanco, C., Andrade, J., Becker, C., Bemm, F., Bergelson, J., Borgwardt, K. M., et al. (2016). 1,135 genomes reveal the global pattern of polymorphism in Arabidopsis thaliana. Cell 166, 481–491. doi: 10.1016/j.cell.2016.05.063
Armstead, I., Donnison, I., Aubry, S., Harper, J., Hortensteiner, S., James, C., et al. (2007). Cross-species identification of Mendel’s/locus. Science 315, 73–73. doi: 10.1126/science.1132912
Bao, Y., Magallenes-Lundback, M., Deason, N., and DellaPenna, D. (2020). High throughput profiling of tocochromanols in leaves and seeds of Arabidopsis and Maize. Plant Methods 16:14. doi: 10.1186/s13007-020-00671-9
Bergmüller, E., Porfirova, S., and Dormann, P. (2003). Characterization of an Arabidopsis mutant deficient in gamma-tocopherol methyltransferase. Plant Mol. Biol. 52, 1181–1190. doi: 10.1023/b:Plan.0000004307.62398.91
Bermúdez, L., del Pozo, T., Lira, B. S., de Godoy, F., Boos, I., Romano, C., et al. (2018). A tomato tocopherol-binding protein sheds light on intracellular alpha-tocopherol metabolism in plants. Plant Cell Physiol. 59, 2188–2203. doi: 10.1093/pcp/pcy191
Bohlmann, J., and Keeling, C. I. (2008). Terpenoid biomaterials. Plant J. 54, 656–669. doi: 10.1111/j.1365-313X.2008.03449.x
Butinar, B., Bucar-Miklavcic, M., Mariani, C., and Raspor, P. (2011). New vitamin E isomers (gamma-tocomonoenol and alpha-tocomonoenol) in seeds, roasted seeds and roasted seed oil from the Slovenian pumpkin variety ‘Slovenska golica’. Food Chem. 128, 505–512. doi: 10.1016/j.foodchem.2011.03.072
Cahoon, E. B., Hall, S. E., Ripp, K. G., Ganzke, T. S., Hitz, W. D., and Coughlan, S. J. (2003). Metabolic redesign of vitamin E biosynthesis in plants for tocotrienol production and increased antioxidant content. Nat. Biotechnol. 21, 1082–1087. doi: 10.1038/nbt853
Cela, J., Tweed, J. K. S., Sivakumaran, A., Lee, M. R. F., Mur, L. A. J., and Munné-Bosch, S. (2018). An altered tocopherol composition in chloroplasts reduces plant resistance to Botrytis cinerea. Plant Physiol. Biochem. 127, 200–210. doi: 10.1016/j.plaphy.2018.03.033
Chen, M. C. M., Yang, J. H., Liu, C. H., Lin, K. H., and Yang, C. M. (2014). Molecular, structural, and phylogenetic characterization of two chlorophyllase isoforms in Pachira macrocarpa. Plant Syst. Evol. 300, 633–643. doi: 10.1007/s00606-013-0908-5
Cheng, W. Y., Kuo, Y. H., and Huang, C. J. (2007). Isolation and identification of novel estrogenic compounds in yam tuber (Dioscorea alata cv. Tainung No. 2). J. Agric. Food Chem. 55, 7350–7358. doi: 10.1021/jf0711690
Cheng, Z. G., Sattler, S., Maeda, H., Sakuragi, Y., Bryant, D. A., and DellaPenna, D. (2003). Highly divergent methyltransferases catalyze a conserved reaction in tocopherol and plastoquinone synthesis in cyanobacteria and photosynthetic eukaryotes. Plant Cell 15, 2343–2356. doi: 10.1105/tpc.013656
Collakova, E., and DellaPenna, D. (2001). Isolation and functional analysis of homogentisate phytyltransferase from Synechocystis sp PCC 6803 and arabidopsis. Plant Physiol. 127, 1113–1124. doi: 10.1104/pp.010421
Diepenbrock, C. H., Kandianis, C. B., Lipka, A. E., Magallanes-Lundback, M., Vaillancourt, B., Gongora-Castillo, E., et al. (2017). Novel loci underlie natural variation in vitamin E levels in maize grain. Plant Cell 29, 2374–2392. doi: 10.1105/tpc.17.00475
Falk, J., and Munné-Bosch, S. (2010). Tocochromanol functions in plants: Antioxidation and beyond. J. Exp. Bot. 61, 1549–1566. doi: 10.1093/jxb/erq030
Fang, X. F., Zhao, G. Z., Zhang, S., Li, Y. X., Gu, H. Q., Li, Y., et al. (2019). Chloroplast-to-nucleus signaling regulates microRNA biogenesis in Arabidopsis. Dev. Cell 48, 371.e–382.e. doi: 10.1016/j.devcel.2018.11.046
Fiedler, E., Soll, J., and Schultz, G. (1982). The formation of homogentisate in the biosynthesis of tocopherol and plastoquinone in spinach chloroplasts. Planta 155, 511–515. doi: 10.1007/bf01607575
Foyer, C. H., and Noctor, G. (2003). Redox sensing and signalling associated with reactive oxygen in chloroplasts, peroxisomes and mitochondria. Physiol. Plant. 119, 355–364. doi: 10.1034/j.1399-3054.2003.00223.x
Foyer, C. H., and Noctor, G. (2005). Redox homeostasis and antioxidant signaling: A metabolic interface between stress perception and physiological responses. Plant Cell 17, 1866–1875. doi: 10.1105/tpc.105.033589
Garcia, I., Rodgers, M., Lenne, C., Rolland, A., Sailland, A., and Matringe, M. (1997). Subcellular localization and purification of a p-hydroxyphenylpyruvate dioxygenase from cultured carrot cells and characterization of the corresponding cDNA. Biochem. J. 325, 761–769. doi: 10.1042/bj3250761
Godwin, J., and Farrona, S. (2020). Plant epigenetic stress memory induced by drought: A physiological and molecular perspective. Methods Mol. Biol. 2093, 243–259. doi: 10.1007/978-1-0716-0179-2_17
Goffman, F. D., and Mollers, C. (2000). Changes in tocopherol and plastochromanol-8 contents in seeds and oil of oilseed rape (Brassica napus L.) during storage as influenced by temperature and air oxygen. J. Agric. Food Chem. 48, 1605–1609. doi: 10.1021/jf9912755
Gutbrod, K., Romer, J., and Dörmann, P. (2019). Phytol metabolism in plants. Prog. Lipid Res. 74, 1–17. doi: 10.1016/j.plipres.2019.01.002
Hammann, S., Englert, M., Muller, M., and Vetter, W. (2015). Accelerated separation of GC-amenable lipid classes in plant oils by countercurrent chromatography in the co-current mode. Anal. Bioanal. Chem. 407, 9019–9028. doi: 10.1007/s00216-015-9068-5
Havaux, M., Eymery, F., Porfirova, S., Rey, P., and Dormann, P. (2005). Vitamin E protects against photoinhibition and photooxidative stress in Arabidopsis thaliana. Plant Cell 17, 3451–3469. doi: 10.1105/tpc.105.037036
Hershberger, J., Tanaka, R., Wood, J. C., Kaczmar, N., Wu, D., Hamilton, J. P., et al. (2022). Transcriptome-wide association and prediction for carotenoids and tocochromanols in fresh sweet corn kernels. Plant Genome 15:16. doi: 10.1002/tpg2.20197
Hofius, D., Hajirezaei, M. R., Geiger, M., Tschiersch, H., Melzer, M., and Sonnewald, U. (2004). RNAi-mediated tocopherol deficiency impairs photoassimilate export in transgenic potato plants. Plant Physiol. 135, 1256–1268. doi: 10.1104/pp.104.043927
Jacob-Wilk, D., Holland, D., Goldschmidt, E. E., Riov, J., and Eyal, Y. (1999). Chlorophyll breakdown by chlorophyllase: Isolation and functional expression of the Chlase1 gene from ethylene-treated Citrus fruit and its regulation during development. Plant J. 20, 653–661. doi: 10.1046/j.1365-313X.1999.00637.x
Jiang, J. S., Chen, Z. H., Ban, L. P., Wu, Y. D., Huang, J. P., Chu, J. F., et al. (2017). P-HYDROXYPHENYLPYRUVATE DIOXYGENASE from Medicago sativa is involved in vitamin E biosynthesis and abscisic acidmediated seed germination. Sci. Rep. 7:15. doi: 10.1038/srep40625
Jin, S. X., and Daniell, H. (2014). Expression of gamma-tocopherol methyltransferase in chloroplasts results in massive proliferation of the inner envelope membrane and decreases susceptibility to salt and metal-induced oxidative stresses by reducing reactive oxygen species. Plant Biotechnol. J. 12, 1274–1285. doi: 10.1111/pbi.12224
Kamal-Eldin, A., and Appelqvist, L.-A. (1996). The chemistry and antioxidant properties of tocopherols and tocotrienols. Lipids 31, 671–701. doi: 10.1007/bf02522884
Kim, S. E., Bian, X. F., Lee, C. J., Park, S. U., Lim, Y. H., Kim, B. H., et al. (2021). Overexpression of 4-hydroxyphenylpyruvate dioxygenase (IbHPPD) increases abiotic stress tolerance in transgenic sweetpotato plants. Plant Physiol. Biochem. 167, 420–429. doi: 10.1016/j.plaphy.2021.08.025
Kobayashi, N., and DellaPenna, D. (2008). Tocopherol metabolism, oxidation and recycling under high light stress in Arabidopsis. Plant J. 55, 607–618. doi: 10.1111/j.1365-313X.2008.03539.x
Krieger-Liszkay, A., and Trebst, A. (2006). Tocopherol is the scavenger of singlet oxygen produced by the triplet states of chlorophyll in the PSII reaction centre. J. Exp. Bot. 57, 1677–1684. doi: 10.1093/jxb/erl002
Kruk, J., Hollander-Czytko, H., Oettmeier, W., and Trebst, A. (2005). Tocopherol as singlet oxygen scavenger in photosystem II. J. Plant Physiol. 162, 749–757. doi: 10.1016/j.jplph.2005.04.020
Kruk, J., Szymanska, R., Cela, J., and Munné-Bosch, S. (2014). Plastochromanol-8: Fifty years of research. Phytochemistry 108, 9–16. doi: 10.1016/j.phytochem.2014.09.011
Lin, Y. P., and Charng, Y. Y. (2017). Supraoptimal activity of CHLOROPHYLL DEPHYTYLASE1 results in an increase in tocopherol level in mature arabidopsis seeds. Plant Signal. Behav. 12:3. doi: 10.1080/15592324.2017.1382797
Lin, Y. P., Wu, M. C., and Charng, Y. Y. (2016). Identification of a chlorophyll dephytylase involved in chlorophyll turnover in Arabidopsis. Plant Cell 28, 2974–2990. doi: 10.1105/tpc.16.00478
Liu, X. L., Hua, X. J., Guo, J., Qi, D. M., Wang, L. J., Liu, Z. P., et al. (2008). Enhanced tolerance to drought stress in transgenic tobacco plants overexpressing VTE1 for increased tocopherol production from Arabidopsis thaliana. Biotechnol. Lett. 30, 1275–1280. doi: 10.1007/s10529-008-9672-y
Löffelhardt, W., and Kindl, H. (1979). Conversion of 4-hydroxyphenylpyruvic acid into homogentisic acid at the thylakoid membrane of Lemna gibba. FEBS Lett. 104, 332–334. doi: 10.1016/0014-5793(79)80845-5
Luo, W., Huan, Q., Xu, Y. Y., Qian, W. F., Chong, K., and Zhang, J. Y. (2021). Integrated global analysis reveals a vitamin E-vitamin K1 sub-network, downstream of COLD1, underlying rice chilling tolerance divergence. Cell Rep. 36:17. doi: 10.1016/j.celrep.2021.109397
Ma, J. T., Qiu, D. Y., Gao, H. W., Wen, H. Y., Wu, Y. D., Pang, Y. Z., et al. (2020). Over-expression of a gamma-tocopherol methyltransferase gene in vitamin E pathway confers PEG-simulated drought tolerance in alfalfa. BMC Plant Biol. 20:16. doi: 10.1186/s12870-020-02424-1
Maeda, H., Song, W., Sage, T. L., and DellaPenna, D. (2006). Tocopherols play a crucial role in low-temperature adaptation and phloem loading in Arabidopsis. Plant Cell 18, 2710–2732. doi: 10.1105/tpc.105.039404
Matsumoto, A., Takahashi, S., Nakano, K., and Kijima, S. (1995). Identification of new vitamin E in plant oil. J. Am. Oil. Chem. Soc. 44, 593–597.
Mène-Saffrané, L. (2018). Vitamin E biosynthesis and its regulation in plants. Antioxidants 7:17. doi: 10.3390/antiox7010002
Munné-Bosch, S., Weiler, E. W., Alegre, L., Muller, M., Duchting, P., and Falk, J. (2007). alpha-Tocopherol may influence cellular signaling by modulating jasmonic acid levels in plants. Planta 225, 681–691. doi: 10.1007/s00425-006-0375-0
Neely, W. C., Martin, J. M., and Barker, S. A. (1988). Products and relative reaction rates of the oxidation of tocopherols with singlet molecular oxygen. Photochem. Photobiol. 48, 423–428. doi: 10.1111/j.1751-1097.1988.tb02840.x
Ouyang, S. Q., He, S. J., Liu, P., Zhang, W. K., Zhang, J. S., and Chen, S. Y. (2011). The role of tocopherol cyclase in salt stress tolerance of rice (Oryza sativa). Sci. China Life Sci. 54, 181–188. doi: 10.1007/s11427-011-4138-1
Pellaud, S., and Mène-Saffrané, L. (2017). Metabolic origins and transport of vitamin E biosynthetic precursors. Front. Plant Sci. 8:1959. doi: 10.3389/fpls.2017.01959
Pellaud, S., Bory, A., Chabert, V., Romanens, J., Chaisse-Leal, L., Doan, A. V., et al. (2018). WRINKLED1 and ACYL-COA:DIACYLGLYCEROL ACYLTRANSFERASE1 regulate tocochromanol metabolism in Arabidopsis. New Phytol. 217, 245–260. doi: 10.1111/nph.14856
Pretty, J., Sutherland, W. J., Ashby, J., Auburn, J., Baulcombe, D., Bell, M., et al. (2010). The top 100 questions of importance to the future of global agriculture. Int. J. Agric. Sustain. 8, 219–236. doi: 10.3763/ijas.2010.0534
Ren, W. W., Zhao, L. X., Zhang, L. D., Wang, Y. L., Cui, L. J., Tang, Y. L., et al. (2011). Molecular analysis of a homogentisate phytyltransferase gene from Lactuca sativa L. Mol. Biol. Rep. 38, 1813–1819. doi: 10.1007/s11033-010-0297-6
Riewe, D., Koohi, M., Lisec, J., Pfeiffer, M., Lippmann, R., Schmeichel, J., et al. (2012). A tyrosine aminotransferase involved in tocopherol synthesis in Arabidopsis. Plant J. 71, 850–859. doi: 10.1111/j.1365-313X.2012.05035.x
Russin, W. A., Evert, R. F., Vanderveer, P. J., Sharkey, T. D., and Briggs, S. P. (1996). Modification of a specific class of plasmodesmata and loss of sucrose export ability in the sucrose export defective1 maize mutant. Plant Cell 8, 645–658. doi: 10.1105/tpc.8.4.645
Sadre, R., Frentzen, M., Saeed, M., and Hawkes, T. (2010). Catalytic reactions of the homogentisate prenyl transferase involved in plastoquinone-9 biosynthesis. J. Biol. Chem. 285, 18191–18198. doi: 10.1074/jbc.M110.117929
Sadre, R., Gruber, J., and Frentzen, M. (2006). Characterization of homogentisate prenyltransferases involved in plastoquinone-9 and tocochromanol biosynthesis. FEBS Lett. 580, 5357–5362. doi: 10.1016/j.febslet.2006.09.002
Sato, Y., Morita, R., Nishimura, M., Yamaguchi, H., and Kusaba, M. (2007). Mendel’s green cotyledon gene encodes a positive regulator of the chlorophyll-degrading pathway. Proc. Natl. Acad. Sci. U.S.A. 104, 14169–14174. doi: 10.1073/pnas.0705521104
Sattler, S. E., Gilliland, L. U., Magallanes-Lundback, M., Pollard, M., and DellaPenna, D. (2004). Vitamin E is essential for seed longevity, and for preventing lipid peroxidation during germination. Plant Cell 16, 1419–1432. doi: 10.1105/tpc.021360
Savidge, B., Weiss, J. D., Wong, Y. H. H., Lassner, M. W., Mitsky, T. A., Shewmaker, C. K., et al. (2002). Isolation and characterization of homogentisate phytyltransferase genes from Synechocystis sp PCC 6803 and Arabidopsis. Plant Physiol. 129, 321–332. doi: 10.1104/pp.010747
Schelbert, S., Aubry, S., Burla, B., Agne, B., Kessler, F., Krupinska, K., et al. (2009). Pheophytin pheophorbide hydrolase (Pheophytinase) is involved in chlorophyll breakdown during leaf senescence in Arabidopsis. Plant Cell 21, 767–785. doi: 10.1105/tpc.108.064089
Sen, C. K., Khanna, S., and Roy, S. (2007). Tocotrienols in health and disease: The other half of the natural vitamin E family. Mol. Aspects Med. 28, 692–728. doi: 10.1016/j.mam.2007.03.001
Shintani, D., and DellaPenna, D. (1998). Elevating the vitamin E content of plants through metabolic engineering. Science 282, 2098–2100. doi: 10.1126/science.282.5396.2098
Siehl, D. L., Tao, Y. M., Albert, H., Dong, Y. X., Heckert, M., Madrigal, A., et al. (2014). Broad 4-hydroxyphenylpyruvate dioxygenase inhibitor herbicide tolerance in soybean with an optimized enzyme and expression cassette. Plant Physiol. 166, 1162–1176. doi: 10.1104/pp.114.247205
Singh, R. K., Ali, S. A., Nath, P., and Sane, V. A. (2011). Activation of ethylene-responsive p-hydroxyphenylpyruvate dioxygenase leads to increased tocopherol levels during ripening in mango. J. Exp. Bot. 62, 3375–3385. doi: 10.1093/jxb/err006
Sozen, E., Demirel, T., and Ozer, N. K. (2019). Vitamin E: Regulatory role in the cardiovascular system. IUBMB Life 71, 507–515. doi: 10.1002/iub.2020
Spicher, L., Almeida, J., Gutbrod, K., Pipitone, R., Dormann, P., Glauser, G., et al. (2017). Essential role for phytol kinase and tocopherol in tolerance to combined light and temperature stress in tomato. J. Exp. Bot. 68, 5845–5856. doi: 10.1093/jxb/erx356
Staats, M., van Baarlen, P., and van Kan, J. A. L. (2005). Molecular phylogeny of the plant pathogenic genus Botrytis and the evolution of host specificity. Mol. Biol. Evol. 22, 333–346. doi: 10.1093/molbev/msi020
Stacey, M. G., Cahoon, R. E., Nguyen, H. T., Cui, Y. Y., Sato, S., Nguyen, C. T., et al. (2016). Identification of homogentisate dioxygenase as a target for vitamin E biofortification in oilseeds. Plant Physiol. 172, 1506–1518. doi: 10.1104/pp.16.00941
Stahl, E., Hartmann, M., Scholten, N., and Zeier, J. (2019). A role for tocopherol biosynthesis in Arabidopsis basal immunity to bacterial infection. Plant Physiol. 181, 1008–1028. doi: 10.1104/pp.19.00618
Surówka, E., Potocka, I., Dziurka, M., Wrobel-Marek, J., Kurczynska, E., Zur, I., et al. (2020). Tocopherols mutual balance is a key player for maintaining Arabidopsis thaliana growth under salt stress. Plant Physiol. Biochem. 156, 369–383. doi: 10.1016/j.plaphy.2020.09.008
Suzuki, Y. J., Tsuchiya, M., Wassall, S. R., Choo, Y. M., Govil, G., Kagan, V. E., et al. (1993). Structural and dynamic membrane properties of alpha-tocopherol and alpha-tocotrienol: Implication to the molecular mechanism of their antioxidant potency. Biochemistry 32, 10692–10699. doi: 10.1021/bi00091a020
Tian, L., DellaPenna, D., and Dixon, R. A. (2007). The pds2 mutation is a lesion in the Arabidopsis homogentisate solanesyltransferase gene involved in plastoquinone biosynthesis. Planta 226, 1067–1073. doi: 10.1007/s00425-007-0564-5
Tian, Y. N., Zhong, R. H., Wei, J. B., Luo, H. H., Eyal, Y., Jin, H. L., et al. (2021). Arabidopsis CHLOROPHYLLASE 1 protects young leaves from long-term photodamage by facilitating FtsH-mediated D1 degradation in photosystem II repair. Mol. Plant 14, 1149–1167. doi: 10.1016/j.molp.2021.04.006
Tsegaye, Y., Shintani, D. K., and DellaPenna, D. (2002). Overexpression of the enzyme p-hydroxyphenolpyruvate dioxygenase in Arabidopsis and its relation to tocopherol biosynthesis. Plant Physiol. Biochem. 40, 913–920. doi: 10.1016/s0981-9428(02)01461-4
Tsuchiya, T., Ohta, H., Okawa, K., Iwamatsu, A., Shimada, H., Masuda, T., et al. (1999). Cloning of chlorophyllase, the key enzyme in chlorophyll degradation: Finding of a lipase motif and the induction by methyl jasmonate. Proc. Natl. Acad. Sci. U.S.A. 96, 15362–15367. doi: 10.1073/pnas.96.26.15362
Upadhyaya, D. C., Bagri, D. S., Upadhyaya, C. P., Kumar, A., Thiruvengadam, M., and Jain, S. K. (2021). Genetic engineering of potato (Solanum tuberosum L.) for enhanced alpha-tocopherols and abiotic stress tolerance. Physiol. Plant 173, 116–128. doi: 10.1111/ppl.13252
Valentin, H. E., Lincoln, K., Moshiri, F., Jensen, P. K., Qi, Q. G., Venkatesh, T. V., et al. (2006). The Arabidopsis vitamin E pathway gene5-1 mutant reveals a critical role for phytol kinase in seed tocopherol biosynthesis. Plant Cell 18, 212–224. doi: 10.1105/tpc.105.037077
Van Eenennaam, A. L., Lincoln, K., Durrett, T. P., Valentin, H. E., Shewmaker, C. K., Thorne, G. M., et al. (2003). Engineering vitamin E content: From Arabidopsis mutant to soy oil. Plant Cell 15, 3007–3019. doi: 10.1105/tpc.015875
Vom Dorp, K., Holzl, G., Plohmann, C., Eisenhut, M., Abraham, M., Weber, A. P. M., et al. (2015). Remobilization of phytol from chlorophyll degradation is essential for tocopherol synthesis and growth of Arabidopsis. Plant Cell 27, 2846–2859. doi: 10.1105/tpc.15.00395
Wang, H., Xu, S. T., Fan, Y. M., Liu, N. N., Zhan, W., Liu, H. J., et al. (2018). Beyond pathways: Genetic dissection of tocopherol content in maize kernels by combining linkage and association analyses. Plant Biotechno. J. 16, 1464–1475. doi: 10.1111/pbi.12889
Wang, M. M., Toda, K., and Maeda, H. A. (2016). Biochemical properties and subcellular localization of tyrosine aminotransferases in Arabidopsis thaliana. Phytochemistry 132, 16–25. doi: 10.1016/j.phytochem.2016.09.007
Wang, M. M., Toda, K., Block, A., and Maeda, H. A. (2019). TAT1 and TAT2 tyrosine aminotransferases have both distinct and shared functions in tyrosine metabolism and degradation in Arabidopsis thaliana. J. Biol. Chem. 294, 3563–3576. doi: 10.1074/jbc.RA118.006539
Whittle, K. J., Dunphy, P. J., and Pennock, J. F. (1965). Plastochromanol in the leaves of Hevea brasiliensis. Biochem. J. 96, 17C–19C. doi: 10.1042/bj0960017C
Wu, D., Li, X. W., Tanaka, R., Wood, J. C., Tibbs-Cortes, L. E., Magallanes-Lundback, M., et al. (2022). Combining GWAS and TWAS to identify candidate causal genes for tocochromanol levels in maize grain. Genetics 14:iyac091. doi: 10.1093/genetics/iyac091
Xiang, N., Li, C. Y., Li, G. K., Yu, Y. T., Hu, J. G., and Guo, X. B. (2019). Comparative evaluation on vitamin E and carotenoid accumulation in sweet corn (Zea mays L.) Seedlings under temperature stress. J. Agric. Food Chem. 67, 9772–9781. doi: 10.1021/acs.jafc.9b04452
Xin, X. F., Kvitko, B., and He, S. Y. (2018). Pseudomonas syringae: What it takes to be a pathogen. Nat. Rev. Microbiol. 16, 316–328. doi: 10.1038/nrmicro.2018.17
Yang, W. Y., Cahoon, R. E., Hunter, S. C., Zhang, C. Y., Han, J. X., Borgschulte, T., et al. (2011). Vitamin E biosynthesis: Functional characterization of the monocot homogentisate geranylgeranyl transferase. Plant J. 65, 206–217. doi: 10.1111/j.1365-313X.2010.04417.x
Zbierzak, A. M., Kanwischer, M., Wille, C., Vidi, P. A., Giavalisco, P., Lohmann, A., et al. (2010). Intersection of the tocopherol and plastoquinol metabolic pathways at the plastoglobule. Biochem. J. 425, 389–399. doi: 10.1042/bj20090704
Zhang, C. Y., Zhang, W., Ren, G. D., Li, D. L., Cahoon, R. E., Chen, M., et al. (2015). Chlorophyll synthase under epigenetic surveillance is critical for vitamin E synthesis, and altered expression affects tocopherol levels in Arabidopsis. Plant Physiol. 168, 1503–U1729. doi: 10.1104/pp.15.00594
Zhang, W., Liu, T. Q., Ren, G. D., Hortensteiner, S., Zhou, Y. M., Cahoon, E. B., et al. (2014). Chlorophyll degradation: The tocopherol biosynthesis-related phytol hydrolase in Arabidopsis seeds is still missing. Plant Physiol. 166, 70–79. doi: 10.1104/pp.114.243709
Keywords: vitamin E, VTE, tocopherol, tocochromanol, biosynthesis, pathway, stress, signal
Citation: Niu Y, Zhang Q, Wang J, Li Y, Wang X and Bao Y (2022) Vitamin E synthesis and response in plants. Front. Plant Sci. 13:994058. doi: 10.3389/fpls.2022.994058
Received: 14 July 2022; Accepted: 15 August 2022;
Published: 14 September 2022.
Edited by:
Zhi-Yan Du, University of Hawai’i at Mānoa, United StatesReviewed by:
Zhansheng Li, Institute of Vegetables and Flowers (CAAS), ChinaCopyright © 2022 Niu, Zhang, Wang, Li, Wang and Bao. This is an open-access article distributed under the terms of the Creative Commons Attribution License (CC BY). The use, distribution or reproduction in other forums is permitted, provided the original author(s) and the copyright owner(s) are credited and that the original publication in this journal is cited, in accordance with accepted academic practice. No use, distribution or reproduction is permitted which does not comply with these terms.
*Correspondence: Yan Bao, eWFuYmFvQHNqdHUuZWR1LmNu
†These authors have contributed equally to this work
Disclaimer: All claims expressed in this article are solely those of the authors and do not necessarily represent those of their affiliated organizations, or those of the publisher, the editors and the reviewers. Any product that may be evaluated in this article or claim that may be made by its manufacturer is not guaranteed or endorsed by the publisher.
Research integrity at Frontiers
Learn more about the work of our research integrity team to safeguard the quality of each article we publish.