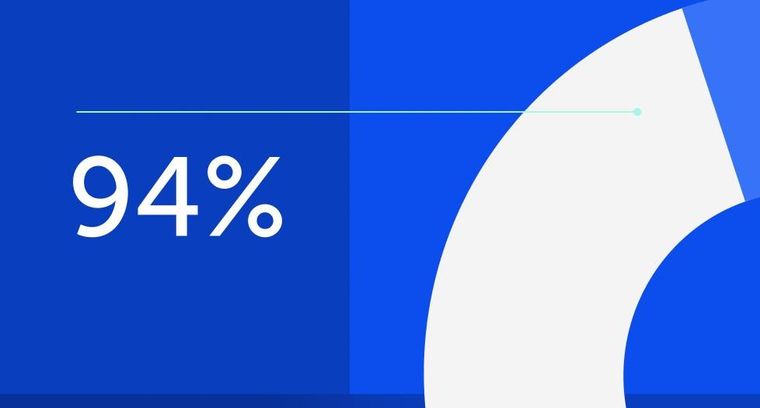
94% of researchers rate our articles as excellent or good
Learn more about the work of our research integrity team to safeguard the quality of each article we publish.
Find out more
ORIGINAL RESEARCH article
Front. Plant Sci., 07 October 2022
Sec. Functional Plant Ecology
Volume 13 - 2022 | https://doi.org/10.3389/fpls.2022.993051
This article is part of the Research TopicInsights in Functional Plant Ecology 2022View all 10 articles
Weed management involving tillage and/or herbicides has generally led to a decline of plant diversity in agroecosystems, with negative impacts on ecosystem services provision. The use of plant covers has become the predominant alternative in vineyard management, with numerous studies focusing on analyzing the advantages and disadvantages of plant covers compared to the aforementioned management. Although the impacts of weed management on taxonomic diversity have been widely studied, many gaps remain on their effects on plant functional diversity. As plant functional diversity is linked to the delivery of key ecosystem services in agroecosystems, understanding these effects could enable the development of more sustainable practices. From 2008 to 2018, a long-term trial was carried out in a Mediterranean vineyard to assess different agricultural practices. In this article, we examined how weed management, as well as irrigation use, could affect plant functional diversity. Based on 10 functional traits, such as plant height, specific leaf area or seed mass, we measured different indices of functional diversity and used null models to detect processes of trait convergence and divergence. Our results revealed that weed management and irrigation use had a significant effect on plant functional diversity. Mown plots showed the highest functional richness but were functionally convergent, since mowing was a strong functional filter on most of the traits. Tillage also behaved as a functional filter on some vegetative traits, but favored the divergence of certain reproductive traits. Herbicide-treated and irrigated plots showed the highest values of functional divergence by promoting more competitive species with more divergent trait values. The effect of weed management on these community assembly processes was shaped by the use of irrigation in vineyard rows, leading to functional divergence in those vegetative traits related to resource acquisition and seed mass. These results suggest that greater functional diversity may be associated with the bias caused by higher occurrence of competitive species (e.g. Convolvulus arvensis, Sonchus asper) with contrasting values for certain traits. Therefore, since these species are considered harmful to crops, higher plant functional diversity might not be a desirable indicator in agroecosystems.
Agricultural intensification is the main process driving the widespread biodiversity loss observed in European farmland in recent decades (Emmerson et al., 2016). This intensification process has caused a marked decline in European arable weeds (Storkey et al., 2012), decreasing both taxonomic and functional diversity (José-María et al., 2010; Carmona et al., 2020), which could have negative impacts on the provision of key ecosystem services in agroecosystems such as crop pollination (Bretagnolle and Gaba, 2015) or pest control (Martínez-Uña et al., 2013; Crowder and Jabbour, 2014). In this context of agricultural intensification, since crop losses due to weeds can be substantial (Oerke, 2006), weed management has targeted weed eradication using recurrent tillage and/or herbicides (MacLaren et al., 2020). These weed management practices have led to environmental impacts such as soil erosion and soil fertility decline, which pose a serious threat to Mediterranean agroecosystems (Novara et al., 2011; Prosdocimi et al., 2016). To deal with these problems, greener weed management practices have been promoted over the past few years. In vineyards, plant cover (usually mown) has become widespread as a viable option to conventional management based on soil tillage, with positive effects such as the improvement of soil properties or the enhancement of biodiversity (Winter et al., 2018; Novara et al., 2019; Guerra et al., 2022), although exhibiting in some cases a reduction on vineyard vigor and yield (Monteiro and Lopes, 2007; Celette and Gary, 2013). The impact of weed management on weed communities has been extensively documented, revealing that management practices such as tillage, herbicide or mowing can exert selective pressure on these communities, altering their composition and/or taxonomic diversity (Doradoand López-Fando, 2006; José‐María et al., 2010; Grundy et al., 2011; Larson et al., 2021; Guerra et al., 2022). However, gaps remain on how these management practices might affect plant functional diversity.
Plant functional diversity can be defined as the value, range, distribution and relative abundance of plant functional traits present in a given ecosystem (Díaz et al., 2007), being closely related to ecosystem properties and provision of key ecosystem services such as carbon sequestration or maintenance of soil fertility (Dıíaz and Cabido, 2001; Díaz et al., 2007; Conti and Díaz, 2013). Thus, plant functional diversity can explain variations in ecosystem functions even when species richness does not (Cadotte et al., 2011). These links to ecosystem processes has made plant functional diversity a central issue for ecology in the last two decades, leading to the proposal of numerous indices for its estimation in different ecosystems (Petchey and Gaston, 2002; Botta-Dukát, 2005; Mason et al., 2005; Villéger et al., 2008).
In agroecosystems, previous works have shown how agricultural intensification reduces functional diversity of weed communities in arable systems (Carmona et al., 2020) and olive groves (Tarifa et al., 2021), although only Carmona et al. (2020) explored in depth the effect on several indicators of functional diversity. However, these studies did not analyze potential differences due to different weed managements. In vineyards, recent short-term studies have partially analyzed the effects of weed management on some aspects of plant functional diversity. Thus, Kazakou et al. (2016) estimated functional richness, one of the three indices proposed by Villéger et al. (2008) to measure functional diversity, finding that spontaneous cover showed higher functional richness than tillage. Hall et al. (2020) estimated functional diversity using Rao’s quadratic entropy (Rao, 1982), observing higher functional diversity in vineyards managed with permanent vegetation cover compared to those observed in bare soil management (herbicide or tillage). Mainardis et al. (2020) found that functional diversity, estimated by functional dispersion and functional divergence, was higher under mowing than under tillage.
Plant functional diversity indices combined with null models have also been used to identify community assembly processes driven by functional traits (Götzenberger et al., 2012; Botta-Dukát and Czúcz, 2016), testing the existence of the two main mechanisms underlying the assemblage of plant communities: habitat filtering and limiting similarity. Trait convergence is usually related to habitat filtering mechanisms (Keddy, 1992), in which environmental or management factors act as abiotic filters restricting the range of species trait values (e.g. Lhotsky et al., 2016). In recent years, it has been described that weed management can affect the functional structure of weed communities favoring one set of functional traits over others (Fried et al., 2012; Guerra et al., 2021) which could be indicative, although not proven, of trait convergence caused by habitat filtering. In contrast, trait divergence could occur according to the principle of limiting similarity (Macarthur and Levins, 1967) which suggests that species can coexist more easily if they diverge in their traits, thereby decreasing competition between them (e.g. Thompson et al., 2010). Nevertheless, convergent and divergent patterns can occur simultaneously within the same community (Bernard-Verdier et al., 2012; Spasojevic and Suding, 2012) and may differ between traits, with convergence being more common for vegetative traits while divergence is more likely for reproductive traits (Grime, 2006). In agroecosystems, the study of these processes that condition plant functional diversity has been limited to grasslands (e.g. Mason et al., 2011; Bernard‐Verdier et al., 2012), lacking studies that test the existence of such processes according to weed management.
The aim of this study was to examine how weed management, as well as irrigation use, might affect functional diversity of weed communities. In particular, we have focused on analyzing the relationship between weed management and trait convergence or divergence patterns. Based on previous studies (Guerra et al., 2021; Guerra et al., 2022), we further hypothesize that functional diversity could also vary depending on the management adjacent to each plot studied. For example, Guerra et al. (2022) reported that species richness was higher in herbicide-treated plots close to mowing than in plots close to tillage. In addition, we have recently observed that at herbicide-treated and irrigated rows, with intermediate values of bare soil, the most competitive species were favored (Guerra et al., 2021; 2022). Given that competition is a factor that can shape plant functional diversity (Navas and Violle, 2009), this work also studied the relationship between functional diversity and competitiveness (CSR strategy; Grime, 1974). Therefore, the purpose of this article is to contribute to increase the knowledge on the influence of agronomic management on plant functional diversity, so that implications for agroecological weed management can be analyzed.
Research was carried out in an experimental vineyard at the IMIDRA farm El Socorro (Colmenar de Oreja, Madrid), located at 755 m in the central plateau of the Iberian Peninsula, developed on a clay-loam soil of type Calcic Haploxeralf. Climate is Mediterranean, with mean annual temperature of 13.7°C and annual precipitation of 421.4 mm (data from the farm weather station for the period 2001–2021). Grapevines (c.v. Tempranillo) were grown on trellises, with deficit drip irrigation applied from June to September in vineyard rows.
In 2008, a randomized block trial with four replications was set up to assess different vineyard management systems. Based on this trial, a study was designed in 2015 to examine how different weed management (herbicide, tillage, mowing), as a function of irrigation within the vineyard (non-irrigated inter-rows, irrigated rows) could affect the functional diversity of plant communities (Figure 1). Irrigation was considered since the deficit irrigation applied during the dry period generates clearly contrasting conditions between inter-rows (non-irrigated) and rows (irrigated) that could have a significant effect on plant functional diversity. Accordingly, five types of plots were identified: a) herbicide-treated rows (irrigated); b) mown inter-rows (non-irrigated); c) mown rows (irrigated); d) tilled inter-rows (non-irrigated); e) tilled rows (irrigated). Herbicide-treated inter-rows (non-irrigated) were not included in the original design as this is a very unusual praxis in Mediterranean vineyards, where herbicide application is restricted to rows. Herbicide treatment consisted of two applications of glyphosate (1440 g ai ha-1) throughout the grapevine growth cycle (Figure 1). Mowing of the spontaneous plant cover (two passes) was performed with a flail mower in vineyard inter-rows and with an inter-vine mower in rows. Tillage (two-three passes) was carried out with a cultivator in vineyard inter-rows and with an inter-vine tiller in vineyard rows.
Figure 1 Diagram of the experimental design and location of the sampling points (upper part), 3D image depicting sites 2-1-2 (lower left) and schematic chronogram of the weeding operations carried out in the study field (lower right). Irrigation treatment: I: irrigated row; NI, non-irrigated inter-row. Site: 1, tilled inter-rows adjacent to herbicide-treated plots; 2, herbicide-treated rows adjacent to tilled plots; 3, tilled inter-rows adjacent to tilled plots; 4, tilled rows; 5, mown inter-rows adjacent to herbicide-treated plots; 6, herbicide-treated rows adjacent to mown plots; 7, mown inter-rows adjacent to mown plots; 8, mown rows.
According to our initial hypothesis that the functional diversity of a plot could vary as a function of adjacent management, we distinguished eight different environmental sites in the experimental design (Figure 1). For example, it was considered that the functional diversity in herbicide-treated rows next to mowing (site 6) might be different from that found in herbicide-treated rows next to tillage (site 2). For further details on study site and experimental design, see Guerra et al. (2022).
In each of the 32 plots (4 blocks × 8 sites), six sampling points separated by a distance of 5.5 m were fixed (Figure 1). Sampling points were placed in pairs (inter-row and row, respectively) spaced 1 m apart. In each of them, a 33 × 66 cm frame was placed, identifying all plant species and assessing the coverage percent of each species (Andújar et al., 2010), as well as the percentage of bare soil. For each species, mean percentage cover was calculated by pooling the data obtained in the six sampling points of each plot. Castroviejo (1986-2012) was used for species identification, updating the species name according to the International Plant Names Index (IPNI, 2022).
In order to measure functional diversity, 10 functional traits previously associated with plant response to weed management (Guerra et al., 2021), were selected: five vegetative traits related to resource-acquiring capacity (Raunkiaer life form [RLF], plant height vegetative [PHV], specific leaf area [SLA], leaf dry matter content [LDMC], leaf area [LA]), and five reproductive traits related to regenerative ability (seed mass [SM], seed bank longevity index [SLI], onset of flowering [OFL], duration of flowering period [DFP], dispersal syndrome [DS]). An overview of the traits used can be found in Table 1.
This study included only species with a significant presence, excluding in the analyses those with less than 0.25% relative cover based on the overall value of all plots. Then, plant trait data were compiled from plant trait databases such as TRY Database (Kattge et al., 2020) and stored in a new database (Guerra et al., 2021). Further details on the traits and database used can be found in Guerra et al. (2021).
Functional diversity based on three components, i.e. functional richness, functional evenness and functional divergence (Mason et al., 2005), were analyzed in this work. Functional richness and functional evenness were computed according to two indices proposed by Villéger et al. (2008): FRic, which quantifies the functional space volume filled by the community based on the calculation of the convex hull volume (see Cornwell et al., 2006); and FEve, which measures the uniformity of abundance distribution in this volume to quantify the regularity with which the different species, weighted by their abundance, fill this functional space.
Functional divergence was measured from Rao’s quadratic entropy (RaoQ), which is often used as an index of functional divergence (Schleuter et al., 2010) although encompasses both richness and functional divergence (Mouchet et al., 2010). Hence, RaoQ is the mean distance between species weighted by species abundance (Botta-Dukát, 2005). This index is strongly related to functional dispersion index (FDis) proposed by Laliberté and Legendre (2010) and to the community weighted variance (CWV) defined by Sonnier et al. (2010). It should be noted that FDis and CWV were initially calculated, yielding very similar results to RaoQ (Supplementary Figure 1). ConsequentlRaoQ was used because it is a more common index than the previous ones in plant functional diversity studies.
For computing functional diversity indices, a functional distance matrix based on trait values for each species was initially generated using the Gower distance (Gower, 1971). On this functional matrix, a principal coordinate analysis (PCoA) was performed and the resulting PCoA axes were used as new “traits” to compute in each plot the indices mentioned above, using the function dbFD from the R package FD (Laliberté and Legendre, 2010).
Thereafter, a null model approach was applied for functional diversity indices (except for FEve, which was excluded according to Mason et al., 2013), testing whether observed FRic and RaoQ values, the latter calculated for the set of traits and also for each trait individually, differed from random expected values. This approach was implemented for two purposes: Firstly, to remove any trivial effect of species richness on functional diversity indices (in line with Mason et al., 2013; Pakeman et al., 2017; Carmona et al., 2020). This is particularly relevant for FRic, given its strong positive correlation with species richness (Villéger et al., 2008; Mouchet et al., 2010) such as was revealed in a preliminary analysis (Supplementary Figure 2). After removing this effect, RaoQ becomes a “pure measure” of functional divergence (Mason et al., 2013). Secondly, null models were developed to test changes in community assembly processes (similarly to Bernard‐Verdier et al., 2012; Mason et al., 2012; Mason et al., 2013). Although both RaoQ and FRic have proven to be reliable measures for this purpose, RaoQ seems to be the most suitable for detecting trait convergence and divergence (Botta-Dukát and Czúcz, 2016).
Figure 2 Effect size of FRic (ES FRic) depending on site (A) and effect size of RaoQ (ES RaoQ) depending on the interaction of weed management and irrigation (WM:I) (B). Marginal R2 (R2m) provides the variance explained by the fixed effects. Same letters on the boxes indicate no significant differences. H, herbicide; M, mowing; T, tillage; NI, non-irrigation; I, irrigation. Sites: 1, tilled inter-rows adjacent to herbicide-treated plots; 2, herbicide-treated rows adjacent to tilled plots; 3, tilled inter-rows adjacent to tilled plots; 4, tilled rows; 5, mown inter-rows adjacent to herbicide-treated plots; 6, herbicide-treated rows adjacent to mown plots; 7, mown inter-rows adjacent to mown plots; 8, mown rows. Outliers are indicated with an asterisk (*).
Implemented null models were selected based on previous work (Mason et al., 2013; Botta-Dukát and Czúcz, 2016; Götzenberger et al., 2016). For FRic, randomized matrix was obtained with the independent swap algorithm (function “independentswap” in picante) while maintaining the occurrence frequency of the species and the species richness of each plot. For RaoQ, species abundance within each plot was shuffling maintaining sample species richness (function “richness” in picante). Null models were generated from randomized matrices using the R package picante (Kembel et al., 2010), performing 1000 randomizations for each model to ensure an accurate estimate (Gotelli, 2000).
Subsequently, functional diversity indices were calculated from randomized matrices, measuring the degree to which the observed values of a variable were different from its expected values and later calculating the effect size. The standard method for measuring effect size in a null model is to compute standardized effect size (SES; Gotelli and McCabe, 2002), but Botta-Dukát (2018) has recently pointed out that this method is not suitable when, as in our case, the symmetry assumption is not satisfied. For this reason, it was decided to measure the effect size (ES) according to Chase et al. (2011) as has been performed previously for other cases with not-symmetric distribution of the null model (e.g. Bernard‐Verdier et al., 2012; Lhotsky et al., 2016):
where
being number (NULL< obs) the number of occasions in which the value obtained from the null model (NULL, expected value) was less than the observed value (obs) and number (NULL = obs) the number of occasions in which the value obtained from the null model was equal to the observed value. This procedure was performed separately for both FRic and RaoQ. Thus, two new indices were obtained from the effect sizes of FRic (ES FRic) and RaoQ (ES RaoQ) with values ranging from +1 to -1, where positive values indicate a higher observed value than expected, and vice versa. Trait convergence or divergence were assumed if the observed RaoQ values were, respectively, lower or higher than the estimated RaoQ values by the null model (similarly to Bernard‐Verdier et al., 2012; Boonman et al., 2021). Since sometimes community assemblage patterns cannot be described by a single multivariate trait index (Spasojevic and Suding, 2012; Carmona et al., 2015), ES RaoQ has been calculated for the set of all traits (multi-trait) and for each single trait. Besides, although RaoQ (and by extension, ES RaoQ) is less affected by trait outliers than FRic (Cornwell and Ackerly, 2009), functional divergence will be higher the closer the abundant species are located to the boundary of the functional trait space (Botta-Dukát and Czúcz, 2016). Taking this into account, it was assumed that the occurrence of abundant species with extreme values for certain traits could be associated with trait divergence. Abundant species were considered to be those dominant and subordinate species (Grime, 1998) with a relative cover of more than 3% (arbitrary value), which could fundamentally govern the community assemblage processes under the mass ratio hypothesis (Grime, 1998; Dıíaz and Cabido, 2001). Therefore, the relationship between the most abundant species and ES RaoQ was also analyzed.
The CSR theory (Grime, 1974), which has been extensively applied to the study of plant communities (e.g. Cerabolini et al., 2010; Guerra et al., 2021), categorizes plant species within a triangular scheme consisting of three dimensions: a competitiveness dimension (C-dimension), a stress-tolerance dimension (S-dimension) and a ruderality dimension (R-dimension). The competitive dimension is related to the ability of plant species to compete with their neighbors, with competitor plants typically being large herbaceous species with rapid resource acquisition (Grime, 1974; Hodgson et al., 1999). Building on this theoretical framework, Hodgson et al. (1999) developed a method for CSR classification based on functional traits of herbaceous plants.
Recently, a correlation between the functional structure of weed communities and the CSR strategy has been observed (Guerra et al., 2021). In order to analyze the relationship between competitiveness and functional diversity, we applied a procedure similar to that used by Ricotta et al. (2016) to calculate here a competitiveness index (Cindex). For this purpose, the competitiveness dimension (C-dimension) of each of the selected species was firstly estimated according to Hodgson et al. (1999). Then, community-weighted mean (CWM; Garnier et al., 2004) of C-dimension values were computed with the R package FD for each of the plots (i.e. plot-level C-dimension values weighted by species abundance). Thus, Cindex was calculated as:
where pi is the relative abundance of species i, and C-dimensioni is the C-dimension value of species i.
The effect of weed management (WM) and irrigation (I) on functional diversity indices was analyzed by means of linear mixed models with the R package lme4 (Bates et al., 2015), except for RaoQ models which were fitted via beta regressions models, with “loglog” as link function, using the R package betareg (Cribari-Neto and Zeileis, 2010). Due to the inherent constraints of the experimental design, WM and I were always considered as nested fixed factors (WM:I), although the individual effects of WM and I were also explored. In addition, the influence that adjacent management might have on each plot was examined by fitting alternative models with “site” as a fixed effect. In all fitted models, year and block were considered as random effects.
To screen the best models for each variable, the corrected Akaike’s information criterion (AICc) was used, choosing the model with the lowest AICc value (Burnham and Anderson, 2002). Model fit was estimated by mean of pseudo R2 (only for beta regression models), marginal R2 (variance explained by fixed effects) and conditional R2 (variance explained by the whole model) using the R package performance (Lüdecke et al., 2021). To test whether fixed effects of the selected models were statistically significant, type-III ANOVA tests were performed using the R package car (Fox and Weisberg, 2019). When a significant effect was observed, a post-hoc analysis was performed using the pairwise t-test adjusted for Bonferroni correction for pairwise comparison.
In order to detect trait convergence or divergence patterns, values of ES RaoQ were tested as to whether they were significantly different from zero using a one-sample t-test (for normally distributed data) and a Wilcoxon signed-ranks test (for non-normally distributed data). In addition, to analyse the impact of the most abundant species on trait divergence, the relationships between ES RaoQ (both multi-trait and single-trait estimations) and relative cover of these species were assessed using Pearson’s correlation test (when data were fitted to a normal distribution) and Spearman’s correlation test (for non-normally distributed data).
Finally, the relationship between Cindex and ES RaoQ was explored through linear regression models using ES RaoQ as the response variable. All analyses were carried out in R 4.1.0 (R Core Team, 2022).
A total of 59 plant species were detected, of which 29 showed a significant occurrence (Supplementary Table 1). Complete trait information for these 29 species, reported elsewhere (Guerra et al., 2021), was employed to compute functional diversity indices. Nonetheless, trait data for the most abundant species are provided (see Supplementary Tables 2, 3). All models except the one constructed for ES FRic showed lower AICc values when WM:I was used as predictor variable (Table 2).
Table 2 Summary of models built to analyze the effect of weed management (WM) and irrigation (I) on functional diversity indices.
A moderate influence of WM:I on functional richness ( = 0.16) was observed (Table 2). Thus, functional richness was significantly higher in mown irrigated plots compared to tilled irrigated plots, while intermediate values were reached in herbicide-treated irrigated plots (Table 3). However, when the influence of species richness was removed (i.e. ES FRic), opposite values were obtained. Model for ES FRic was best fitted when “site” was used as predictor variable (= 0.44). Thus, herbicide-treated sites (site 2 and 6) showed significantly different ES FRic values between them (Figure 2A). Indeed, herbicide-treated rows next to tillage (i.e. site 2), showed similar values to tilled plots but significantly greater than herbicide-treated rows next to mowing (i.e. site 6). A slightly lower effect of WM:I on functional evenness was found ( = 0.10), with no significant differences observed among managements. In contrast, a marked influence of WM:I on functional divergence (RaoQ) was observed (pseudo R2 = 0.43), reaching significantly greater values in herbicide-treated irrigated plots than in mown irrigated plots (Table 3). This effect is even more marked for ES RaoQ ( = 0.55). Besides, irrigation also had a significant effect on functional divergence (Table 2). Thus, higher RaoQ and ES RaoQ values were observed in irrigated plots, although for RaoQ, these differences were only significant when comparing among mown plots (Table 3).
Table 3 Mean values (± SE) by plots of functional diversity indices based on the interaction of weed management and irrigation (WM:I).
Results related to ES RaoQ for the whole set of traits showed a process of trait convergence in tilled non-irrigated plots and in mown plots, being especially marked in mown non-irrigated plots, while trait divergence was observed in herbicide-treated irrigated plots (Figure 2B). Therefore, mowing significantly promoted trait convergence while trait divergence was enhanced in irrigated plots, supporting what was observed in the exploratory analysis (Supplementary Tables 4, 5). The results of ES RaoQ values for each single trait provided more detailed information (Figure 3; Table 4). Trait convergence observed in tilled non-irrigated plots was mainly due to the negative ES RaoQ values detected for RLF, PHV and LA. In mown plots, convergence was found for all traits except for RLF and, in the case of mown irrigated plots, also for LA and SM. In contrast, a trend towards trait divergence was observed in most traits in herbicide-treated irrigated plots, but this divergence was only significant for LA and SM. It should be noted that a strong functional convergence was observed for PHV in all plots except in herbicide-treated irrigated plots, where the median value was above zero. In tilled irrigated plots, high functional divergence was also observed for SM and, to a lesser extent, for SLI and OFL. However, the ES RaoQ value for the whole set of traits was not significantly higher than zero, since trait convergence was detected for leaf traits and PHV. In addition, when the effect of irrigation on the divergence of single traits was explored, it was revealed that irrigation only had a significant effect on divergence for RLF, PHV, LA and SM (Supplementary Table 5). Thus, for these traits, irrigated plots were more functionally divergent than non-irrigated plots.
Figure 3 Effect size of RaoQ (ES RaoQ) for each single trait depending on the interaction of weed management and irrigation (WM:I). H, herbicide; M, mowing; T, tillage; NI, non-irrigation; I, irrigation. For pairwise comparison, a Student’s t-test was conducted, with P-values adjusted using the Bonferroni correction. Same letters on the boxes indicate no significant differences. Trait code indicated in the subscripts: RLF, Raunkiær Life Form; PHV, plant height; LDMC, leaf dry matter content; LA, leaf area; SLA, specific leaf area; SM, seed mass; SLI, seed bank longevity index; OFL, onset of flowering; DFP, duration of flowering period; DS, dispersal syndrome. Outliers are indicated with an asterisk (*).
Table 4 Results of tests on ES RaoQ values to analyze trait convergence or divergence, using a one-sample t-test (for normally distributed data) and a Wilcoxon signed-rank test (for non-normally distributed data) to test whether ES RaoQ values are significantly different from 0.
The highest ES RaoQ values found for PHV, LA and SM were strongly associated with two species having more extreme values for these traits: Sonchus asper (for PHV and LA) and Convolvulus arvensis (for SM) (Supplementary Figure 4). An increased occurrence of S. asper caused a substantial increase in ES RaoQ for PHV and especially for LA, with ES RaoQ values above 0.5 for plots with a relative cover above 20% for this species (Figure 4A); while a small increase in the percentage of C. arvensis caused an exponential increase in ES RaoQ for SM (Figure 4B), with ES RaoQ higher than 0.5 from a percentage of C. arvensis of 4%. For further details, see Supplementary Figures 5, 6.
Figure 4 Relationship between the relative cover of Sonchus asper and effect size of RaoQ for leaf area (ES RaoQ LA) (A) and between the relative cover of Convolvulus arvensis and effect size of RaoQ for seed mass (ES RaoQ SM) (B). Dots, corresponding to plots, have been filled in by the interaction of weed management and irrigation (WM:I): HI, herbicide – irrigation; MNI, mowing – non-irrigation; MI, mowing – irrigation; TNI, tillage – non-irrigation; TI, tillage – irrigation. For each correlation, Spearman correlation coefficient (r) and its level of significance (***P< 0.000) are shown. Confidence intervals (95%) are shaded in light gray.
A strong correlation was found between Cindex and ES RaoQ (R2 = 0.80, P< 0.001), which was best fitted using a cubic regression model (Figure 5). Hence, functional divergence was greater the higher the competitiveness, but with a slight drop for Cindex above 0.45. An analysis of the ES RaoQ values for each single trait showed how there was a significant positive correlation between ES RaoQ and competitiveness for all traits except for LDMC and SLA (Supplementary Table 6). This correlation was particularly strong for PHV, LA and SM.
Figure 5 Relationship between competitiveness index (Cindex) and the effect size of RaoQ (ES RaoQ). Dots, corresponding to plots, have been filled in by the interaction of weed management and irrigation (WM:I): HI, herbicide – irrigation; MNI, mowing – non-irrigation; MI, mowing – irrigation; TNI, tillage – non-irrigation; TI, tillage – irrigation. R-squared (R2) provides the proportion of variance explained by Cindex. Confidence intervals (95%) are shaded in light gray.
To our knowledge, this is the first long-term study addressing how plant functional diversity is shaped by weed management and the first work to evidence trait convergence and divergence processes linked to weed management. Our results revealed that weed management affected different dimensions of functional diversity, with significant major impact on functional divergence. Indeed, trait divergence was observed in herbicide-treated irrigated plots, while trait convergence patterns were detected in tilled plots and especially in mown plots. Irrigation use, which implies different ecological conditions within the vineyard, also affected functional diversity with higher functional divergence in irrigated plots with respect to non-irrigated plots.
The findings obtained for functional richness were within expectations since, as the literature states, a significant positive correlation exists between functional and species richness (Villéger et al., 2008). In our study, mown plots with higher species richness (Guerra et al., 2022) were also those that filled a larger functional space, which is in consonance with the findings of Kazakou et al. (2016). However, when the effect of species richness on functional richness was removed (i.e. ES FRic), the results were reversed. For instance, in mown inter-rows the observed functional richness values were much lower than expected from the null model (ES FRic = -0.71), evidencing high functional redundancy, thus revealing the occurrence in these plots of a large number of species with similar traits. Herbicide effect on ES FRic differed according to adjacent management (Figure 2A), similar to that observed for species richness in Guerra et al. (2022). In this previous work it was noted that herbicide-treated plots next to mowing (site 6) showed higher number of species than herbicide-treated plots next to tillage (site 2). Taking this into account, the low ES FRic values observed at site 6 highlight that higher species richness did not lead in this case to a significant increase in functional richness (Supplementary Figure 3), suggesting that added species are functionally redundant.
Likewise, functional evenness was also lower in mown plots. These findings are in line with Carmona et al. (2020), who observed the lowest values of functional evenness and SES FRic (very similar to ES FRic) in less intensively managed plots. In addition, the lower values observed for ES FRic and FEve in mown plots suggest the existence of habitat filtering mechanisms (Mouchet et al., 2010; Pakeman, 2011), which is in line with the lower values of ES RaoQ found in these plots. Hence, mowing seems to be acting as an abiotic filter that promotes a process of functional convergence in the vast majority of traits, in line with previous work in meadows (Mudrák et al., 2016; Halassy et al., 2019). These results are in agreement with those discussed by Guerra et al. (2021), who observed a distinct suite of plant traits in response to mowing (species with higher stress tolerance, lower vegetative height, lower SLA and higher LDMC). Nonetheless, our findings are not consistent with previous studies reporting the highest values of RaoQ (or similar index FDis) in spontaneous plant covers (Hall et al., 2020) or mown rows (Mainardis et al., 2020). This could be explained by the fact that mowing effects on plant communities depend on the time scale considered, as we will discuss below. Hence, plant communities tend to become more homogeneous and functionally convergent after years of adopting conservation agriculture practices (Derrouch et al., 2021). In particular, mowing can lead over time to a homogeneous species composition (Lepš, 2014) and to functional convergence in some traits such as plant height (Halassy et al., 2019).
In tilled inter-rows, although in a more attenuated form, it was also possible to detect a process of functional convergence in some vegetative traits (RLF, PHV, LA), due to the abundant occurrence of short ruderal therophytes with small leaves such as Lamium amplexicaule, which are favored by tillage (Hall et al., 2020; Guerra et al., 2021). However, contrary to expectations, no trait convergence was observed in tilled non-irrigated plots for leaf economics traits (LDMC, SLA), which had been previously associated with tillage and agricultural intensification (Carmona et al., 2020; Hall et al., 2020; Guerra et al., 2021). Although tillage may be acting as a functional filter enhancing traits related to fast-growing ruderal species (Guerra et al., 2021), this habitat filtering process may have been hidden in tilled non-irrigated plots by two independent processes. Firstly, the high abundance of Stellaria media in these plots, with an extreme value for SLA, led to an increase in functional divergence (Supplementary Figures 5D and 6D). High SLA values are associated with high growth rates (Hunt and Cornelissen, 1997), enabling species to complete their life cycle in a short time (Grime and Hunt, 1975). This would give S. media an adaptive advantage to proliferate in environments subject to recurrent disturbances, such as tilled fields (Jernigan et al., 2017). Therefore, tillage could be exerting habitat filtering at the lower margin of the functional range by limiting the occurrence of species with low SLA, while not necessarily limiting the occurrence of species with more extreme values at the upper margin of the functional range, since high SLA values provide an adaptive advantage over tillage. Secondly, the habitat filtering could be hidden here by the abundance of species that are not typical of tillage (e.g. Medicago minima, Bromus madritensis) but which colonize these tilled spaces due to their massive occurrence in nearby mown plots, compatible with a spatial mass effect (Shmida and Wilson, 1985). Thus, the abundance in tilled non-irrigated plots of M. minima and B. madritensis, with LDMC values substantially higher than those of typical tillage species (e.g. L. amplexicaule), caused a marked increase in functional divergence (Supplementary Figure 6D).
The observed convergence in vegetative traits (PHV, LDMC, SLA) in tilled irrigated plots, would suggest a process of habitat filtering due to tillage. However, trait convergence was counterbalanced here by divergence patterns observed for certain reproductive traits (SM, SLI, OFL), which were markedly high for SM. Trait divergence for SLI and OFL was a consequence of the coexistence in these plots of tillage specialist species with a persistent seed bank and early flowering (e.g. L. amplexicaule, Veronica hederifolia) together with species with a transient seed bank (e.g. Diplotaxis erucoides, M. minima) and later flowering (e.g. C. arvensis, B. madritensis). This suggests the existence of a partitioning of phenological and regeneration niches (Grubb, 1977), with different reproductive strategies, similar to what has been documented in previous studies (Cornwell and Ackerly, 2009; Bernard‐Verdier et al., 2012). Likewise, the greatest functional divergence observed for SM was mainly due to the occurrence of a single species, C. arvensis, with a vastly higher SM than the others (Figure 4B). This higher SM may provide C. arvensis a greater ability to compete for establishment sites (Turnbull et al., 2004), increase success in colonization and survival after tillage or herbicide application (Kazakou et al., 2021) and establish a persistent soil seed bank with physically dormant seeds (Xiong et al., 2018), enabling its permanence on arable land.
Similarly, the higher abundance of C. arvensis in irrigated plots also led to a significantly higher functional divergence for SM compared to non-irrigated plots. This was also the cause of the higher divergence observed in irrigated plots for RLF, as the geophyte life form of C. arvensis contrasts with the vast majority of therophytes in these communities.
Overall, our results revealed that functional divergence was greater in irrigated than in non-irrigated plots, since beyond RLF and SM, trait divergence was detected in irrigated plots for PHV and LA. This is consistent with the literature, which states that functional divergence may increase with increasing water availability, particularly in vegetative traits related to resource acquisition (Spasojevic and Suding, 2012; Carmona et al., 2015; Nogueira et al., 2018). Divergence for PHV and LA was mainly driven by the increased abundance in irrigated plots of more competitive species such as S. asper or D. erucoides (Guerra et al., 2021), which had a significantly greater vegetative height and leaf size than the rest. The PHV and LA are closely associated with competitive ability (Grime, 1974; Keddy et al., 2002). On this basis, a tall and large-leaved species such as S. asper could compete more effectively for resources, especially for light capture and water. These trait divergence patterns observed for these vegetative traits would indicate a partitioning of light interception strategies, in line with previous work in herbaceous communities (Spasojevic and Suding, 2012).
From the above, irrigation favored more competitive species that showed markedly divergent values for certain traits. This led to an overdispersion of these traits and thus to an increase in functional divergence indices, as clearly reflected by the strong positive correlation found between ES RaoQ and Cindex (Figure 5), albeit with a slight downward trend in functional divergence was observed at higher Cindex values. This is consistent with former studies indicating that functional divergence may be maximal at intermediate levels of competition, whereas when competitive processes are maximized, as in undisturbed productive habitats, trait convergence may occur as weaker competitors are excluded and traits that confer plant species greater competitive ability are enhanced (Grime, 2006; Navas and Violle, 2009; Mayfield and Levine, 2010).
In our study, competitiveness was significantly greater in herbicide-treated irrigated plots, where a significant trait divergence was detected for the whole set of traits. The higher abundance of S. asper in these plots, where it is the dominant species together with B. madritensis, would explain the higher functional divergence observed. But to understand why, it is first necessary to describe the particular dynamics that occur in these herbicide-treated irrigated plots. Except for cases of herbicide resistance (Heap and Duke, 2018), which are not reported in our study field, the application of non-residual herbicide glyphosate removes almost the totality of weeds, thus resulting in a completely bare soil. But in our case, after the last summer application there was a time window between applications of 8-9 months (see Figure 1) during which different species were able to colonize, germinate and grow in gaps caused by the herbicide treatment. Under these particular conditions, the two dominant species were S. asper and B. madritensis, in our opinion due to two reasons: (a) the most abundant species in nearby plots might have colonized the herbicide-generated gaps more profusely. In fact, the two most abundant species in mown plots, M. minima and B. madritensis, were respectively the third and the first most abundant species in herbicide-treated irrigated plots (Supplementary Figure 5); (b) both tillage and mowing seem to be more successful in limiting the occurrence of S. asper (Guerra et al., 2022). During the months between the last herbicide application and the following year sampling, these dominant species initiate an annual process of resource competition which, as mentioned above, would be enhanced in irrigated plots. In this competition for resources, in line with the “limiting similarity” theory (Macarthur and Levins, 1967), two species are more likely to coexist if they have differentiated niches, as in the case of S. asper and B. madritensis, with markedly different functional traits (see Supplementary Table 3). Thus, with respect to vegetative traits, S. asper is a noticeably taller species, with much larger leaves, lower LDMC and lower SLA than B. madritensis. These species also differ in all reproductive traits, with S. asper having much lighter seeds, a markedly higher SLI, different phenological traits and a different dispersal syndrome than B. madritensis. Considering the evidence of two clearly divergent species within the multi-trait functional space, which was also reflected in significant trait divergence detected through null models, would confirm the existence of niche differentiation processes in herbicide-treated irrigated plots.
Our study has evidenced how plant functional diversity can be affected by weed management through processes of trait convergence and divergence, also revealing that these processes can occur simultaneously, affecting both vegetative and reproductive plant traits. Trait divergence was significant in herbicide-treated irrigated plots, where marked differences between the two dominant species provided clear evidence of a process of niche differentiation. The effect of weed management on these processes was shaped by the application of irrigation in vineyard rows, leading primarily to functional divergence for vegetative traits linked to the ability to acquire resources.
On the whole, these community assembly processes were reflected in the estimated functional diversity indices. Hence, mown plots exhibited the highest functional richness. However, when species abundance was considered (i.e. RaoQ), herbicide-treated irrigated plots showed the highest functional diversity. Since ecosystem functions may be further conditioned by dominant plant species (mass-ratio hypothesis), RaoQ may be a more appropriate index to measure plant functional diversity. Indeed, higher functional diversity might not be a desirable indicator in agroecosystems, since higher RaoQ values were associated in this study with the occurrence of dominant species such as S. asper and C. arvensis, which are considered as noxious grapevine weeds (Guerra et al., 2022) and cause yield losses in a wide variety of crops (Boldt et al., 1998). Based on these assumptions, it would be advisable to propose agroecological weed management aimed not at maximizing functional diversity, but at favoring a certain range of plant trait values, reducing disservices (e.g. crop competition, pest hosting) and enhancing ecosystem services (e.g. pollination, carbon sequestration). For this purpose, CWM of plant traits could be a more useful tool than functional diversity indices (Lavorel, 2013; Weil et al., 2021). Therefore, further studies are required to clarify the relationship between weed management, plant functional traits and the provision of ecosystem services. This relationship should be the central core of agroecological weed management.
The original contributions presented in the study are included in the article/Supplementary Material. Further inquiries can be directed to the corresponding author.
JG, FC, CF-Q and JD conceived and designed the research; JG and JD conducted the plant surveys; JG processed the data, obtained abundance matrix and collected data on plant traits; JG analysed the data with support from JP and JD; JG led the writing of the manuscript under the supervision of JD. All authors contributed critically to the drafts and gave final approval for publication.
This work was supported by FEDER/MICINN-AEI, grant number AGL2017-83325-C4-1-R and by MICINN-AEI, grant number PID2020-113229RB-C41/AEI/10.13039/501100011033. The lead author has been granted a predoctoral research fellowship FPI-INIA2016-00035.
We would like to thank the great support provided by J.M. Martín and D. Campos, CSIC technical staff who participated in the field sampling. We also acknowledge support of the publication fee by the CSIC Open Access Publication Support Initiative through its Unit of Information Resources for Research (URICI).
The authors declare that the research was conducted in the absence of any commercial or financial relationships that could be construed as a potential conflict of interest.
All claims expressed in this article are solely those of the authors and do not necessarily represent those of their affiliated organizations, or those of the publisher, the editors and the reviewers. Any product that may be evaluated in this article, or claim that may be made by its manufacturer, is not guaranteed or endorsed by the publisher.
The Supplementary Material for this article can be found online at: https://www.frontiersin.org/articles/10.3389/fpls.2022.993051/full#supplementary-material
Andújar, D., Ribeiro, A., Carmona, R., Fernández-Quintanilla, C., Dorado, J. (2010). An assessment of the accuracy and consistency of human perception of weed cover. Weed Res. 50, 638–647. doi: 10.1111/j.1365-3180.2010.00809.x
Bates, D., Mächler, M., Bolker, B., Walker, S. (2015). Fitting linear mixed-effects models using lme4. J. Stat. Software 67, 1–48. doi: 10.18637/jss.v067.i01
Bernard-Verdier, M., Navas, M.-L., Vellend, M., Violle, C., Fayolle, A., Garnier, E. (2012). Community assembly along a soil depth gradient: contrasting patterns of plant trait convergence and divergence in a Mediterranean rangeland. J. Ecol. 100, 1422–1433. doi: 10.1111/1365-2745.12003
Boldt, P. E., Rosenthal, S. S., Srinivasan, R. (1998). Distribution of field bindweed and hedge bindweed in the USA. J. Prod. Agric. 11, 377–381. doi: 10.2134/jpa1998.0377
Boonman, C. C. F., Santini, L., Robroek, B. J. M., Hoeks, S., Kelderman, S., Dengler, J., et al. (2021). Plant functional and taxonomic diversity in European grasslands along climatic gradients. J. Veg. Sci. 32, e13027. doi: 10.1111/jvs.13027
Botta-Dukát, Z. (2005). Rao’s quadratic entropy as a measure of functional diversity based on multiple traits. J. Veg. Sci. 16, 533–540. doi: 10.1111/j.1654-1103.2005.tb02393.x
Botta-Dukát, Z. (2018). Cautionary note on calculating standardized effect size (SES) in randomization test. Community Ecol. 19, 77–83. doi: 10.1556/168.2018.19.1.8
Botta-Dukát, Z., Czúcz, B. (2016). Testing the ability of functional diversity indices to detect trait convergence and divergence using individual-based simulation. Methods Ecol. Evol. 7, 114–126. doi: 10.1111/2041-210X.12450
Bretagnolle, V., Gaba, S. (2015). Weeds for bees? a review. Agron. Sustain. Dev. 35, 891–909. doi: 10.1007/s13593-015-0302-5
Burnham, K. P., Anderson, D. R. (2002). Model selection and multimodel inference (New York: Springer).
Cadotte, M. W., Carscadden, K., Mirotchnick, N. (2011). Beyond species: functional diversity and the maintenance of ecological processes and services. J. Appl. Ecol. 48, 1079–1087. doi: 10.1111/j.1365-2664.2011.02048.x
Carmona, C. P., Guerrero, I., Peco, B., Morales, M. B., Oñate, J. J., Pärt, T., et al. (2020). Agriculture intensification reduces plant taxonomic and functional diversity across European arable systems. Funct. Ecol. 34, 1448–1460. doi: 10.1111/1365-2435.13608
Carmona, C. P., Mason, N. W. H., Azcárate, F. M., Peco, B. (2015). Inter-annual fluctuations in rainfall shift the functional structure of Mediterranean grasslands across gradients of productivity and disturbance. J. Veg. Sci. 26, 538–551. doi: 10.1111/jvs.12260
Celette, F., Gary, C. (2013). Dynamics of water and nitrogen stress along the grapevine cycle as affected by cover cropping. Eur. J. Agron. 45, 142–152. doi: 10.1016/j.eja.2012.10.001
Cerabolini, B. E. L., Brusa, G., Ceriani, R. M., De Andreis, R., Luzzaro, A., Pierce, S. (2010). Can CSR classification be generally applied outside Britain? Plant Ecol. 210, 253–261. doi: 10.1007/s11258-010-9753-6
Chase, J. M., Kraft, N. J. B., Smith, K. G., Vellend, M., Inouye, B. D. (2011). Using null models to disentangle variation in community dissimilarity from variation in α-diversity. Ecosphere 2, art24. doi: 10.1890/ES10-00117.1
Conti, G., Díaz, S. (2013). Plant functional diversity and carbon storage – an empirical test in semi-arid forest ecosystems. J. Ecol. 101, 18–28. doi: 10.1111/1365-2745.12012
Cornelissen, J. H. C., Lavorel, S., Garnier, E., Díaz, S., Buchmann, N., Gurvich, D. E., et al. (2003). A handbook of protocols for standardised and easy measurement of plant functional traits worldwide. Aust. J. Bot. 51, 335–380. doi: 10.1071/bt02124
Cornwell, W. K., Ackerly, D. D. (2009). Community assembly and shifts in plant trait distributions across an environmental gradient in coastal California. Ecol. Monogr. 79, 109–126. doi: 10.1890/07-1134.1
Cornwell, W. K., Schwilk, D. W., Ackerly, D. D. (2006). A trait-based test for habitat filtering: Convex Hull volume. Ecology 87, 1465–1471. doi: 10.1890/0012-9658(2006)87[1465:ATTFHF]2.0.CO;2
Cribari-Neto, F., Zeileis, A. (2010). Beta regression in R. J. Stat. Softw 34, 1–24. doi: 10.18637/jss.v034.i02
Crowder, D. W., Jabbour, R. (2014). Relationships between biodiversity and biological control in agroecosystems: Current status and future challenges. Biol. Control 75, 8–17. doi: 10.1016/j.biocontrol.2013.10.010
Daane, K. M., Hogg, B. N., Wilson, H., Yokota, G. Y. (2018). Native grass ground covers provide multiple ecosystem services in Californian vineyards. J. Appl. Ecol. 55, 2473–2483. doi: 10.1111/1365-2664.13145
Derrouch, D., Dessaint, F., Fried, G., Chauvel, B. (2021). Weed community diversity in conservation agriculture: Post-adoption changes. Agric. Ecosyst. Environ. 312, 107351. doi: 10.1016/j.agee.2021.107351
Díaz, S., Lavorel, S., Bello, F., Quétier, F., Grigulis, K., Robson, T. M. (2007). Incorporating plant functional diversity effects in ecosystem service assessments. Proc. Natl. Acad. Sci. U. S. A. 104, 20684–20689. doi: 10.1073/pnas.0704716104
Dıíaz, S., Cabido, M. (2001). Vive la différence: plant functional diversity matters to ecosystem processes. Trends Ecol. Evol. 16, 646–655. doi: 10.1016/S0169-5347(01)02283-2
Dorado, J., López-Fando, C. (2006). The effect of tillage system and use of a paraplow on weed flora in a semiarid soil from central Spain. Weed Res. 46, 424–431. doi: 10.1111/j.1365-3180.2006.00526.x
Emmerson, M., Morales, M. B., Oñate, J. J., Batáry, P., Berendse, F., Liira, J., et al. (2016). “Chapter two - how agricultural intensification affects biodiversity and ecosystem services,”, in Advances in ecological research Large-scale ecology: Model systems to global perspectives. Eds. Dumbrell, A. J., Kordas, R. L., Woodward, G. (Cambridge, MA:Academic Press), 43–97. doi: 10.1016/bs.aecr.2016.08.005
Fox, J., Weisberg, S. (2019). An r companion to applied regression, third edition (Thousand Oaks CA: Sage). Available at: https://socialsciences.mcmaster.ca/jfox/Books/Companion/.
Fried, G., Kazakou, E., Gaba, S. (2012). Trajectories of weed communities explained by traits associated with species’ response to management practices. Agric. Ecosyst. Environ. 158, 147–155. doi: 10.1016/j.agee.2012.06.005
Garnier, E., Cortez, J., Billès, G., Navas, M.-L., Roumet, C., Debussche, M., et al. (2004). Plant functional markers capture ecosystem properties during secondary succession. Ecology 85, 2630–2637. doi: 10.1890/03-0799
Gotelli, N. J. (2000). Null model analysis of species Co-occurrence patterns. Ecology 81, 2606–2621. doi: 10.1890/0012-9658(2000)081[2606:NMAOSC]2.0.CO;2
Gotelli, N. J., McCabe, D. J. (2002). Species Co-occurrence: A meta-analysis of j. m. diamond’s assembly rules model. Ecology 83, 2091–2096. doi: 10.1890/0012-9658(2002)083[2091:SCOAMA]2.0.CO;2
Götzenberger, L., Botta-Dukát, Z., Lepš, J., Pärtel, M., Zobel, M., de Bello, F. (2016). Which randomizations detect convergence and divergence in trait-based community assembly? A test of commonly used null models. J. Veg. Sci. 27, 1275–1287. doi: 10.1111/jvs.12452
Götzenberger, L., de Bello, F., Bråthen, K. A., Davison, J., Dubuis, A., Guisan, A., et al. (2012). Ecological assembly rules in plant communities–approaches, patterns and prospects. Biol. Rev. 87, 111–127. doi: 10.1111/j.1469-185X.2011.00187.x
Gower, J. C. (1971). A general coefficient of similarity and some of its properties. Biometrics 27, 857–871. doi: 10.2307/2528823
Grime, J. P. (1974). Vegetation classification by reference to strategies. Nature 250, 26–31. doi: 10.1038/250026a0
Grime, J. P. (1998). Benefits of plant diversity to ecosystems: immediate, filter and founder effects. J. Ecol. 86, 902–910. doi: 10.1046/j.1365-2745.1998.00306.x
Grime, J. P. (2006). Trait convergence and trait divergence in herbaceous plant communities: Mechanisms and consequences. J. Veg. Sci. 17, 255–260. doi: 10.1111/j.1654-1103.2006.tb02444.x
Grime, J. P., Hunt, R. (1975). Relative growth-rate: Its range and adaptive significance in a local flora. J. Ecol. 63, 393. doi: 10.2307/2258728
Grubb, P. J. (1977). The maintenance of species-richness in plant communities: The importance of the regeneration niche. Biol. Rev. 52, 107–145. doi: 10.1111/j.1469-185X.1977.tb01347.x
Grundy, A. C., Mead, A., Bond, W., Clark, G., Burston, S. (2011). The impact of herbicide management on long-term changes in the diversity and species composition of weed populations. Weed Res. 51, 187–200. doi: 10.1111/j.1365-3180.2010.00831.x
Guerra, J. G., Cabello, F., Fernández-Quintanilla, C., Dorado, J. (2021). A trait-based approach in a Mediterranean vineyard: Effects of agricultural management on the functional structure of plant communities. Agric. Ecosyst. Environ. 316, 107465. doi: 10.1016/j.agee.2021.107465
Guerra, J. G., Cabello, F., Fernández-Quintanilla, C., Peña, J. M., Dorado, J. (2022). How weed management influence plant community composition, taxonomic diversity and crop yield: A long-term study in a Mediterranean vineyard. Agric. Ecosyst. Environ. 326, 107816. doi: 10.1016/j.agee.2021.107816
Halassy, M., Botta-Dukát, Z., Csecserits, A., Szitár, K., Török, K. (2019). Trait-based approach confirms the importance of propagule limitation and assembly rules in old-field restoration. Restor. Ecol. 27, 840–849. doi: 10.1111/rec.12929
Hall, R. M., Penke, N., Kriechbaum, M., Kratschmer, S., Jung, V., Chollet, S., et al. (2020). Vegetation management intensity and landscape diversity alter plant species richness, functional traits and community composition across European vineyards. Agric. Syst. 177, 102706. doi: 10.1016/j.agsy.2019.102706
Heap, I., Duke, S. O. (2018). Overview of glyphosate-resistant weeds worldwide. Pest Manage. Sci. 74, 1040–1049. doi: 10.1002/ps.4760
Hodgson, J. G., Wilson, P. J., Hunt, R., Grime, J. P., Thompson, K. (1999). Allocating c-S-R plant functional types: A soft approach to a hard problem. Oikos 85, 282–294. doi: 10.2307/3546494
Hunt, R., Cornelissen, J. H. C. (1997). Components of relative growth rate and their interrelations in 59 temperate plant species. New Phytol. 135, 395–417. doi: 10.1046/j.1469-8137.1997.00671.x
IPNI (2022) International plant names index (Kew, Harvard University Herbaria and Libraries and Australian National Botanic Gardens: The Royal Botanic Gardens). Available at: http://www.ipni.org (Accessed April 2022).
Jernigan, A. B., Caldwell, B. A., Cordeau, S., DiTommaso, A., Drinkwater, L. E., Mohler, C. L., et al. (2017). Weed abundance and community composition following a long-term organic vegetable cropping systems experiment. Weed Sci. 65, 639–649. doi: 10.1017/wsc.2017.33
José-María, L., Armengot, L., Blanco-Moreno, J. M., Bassa, M., Sans, F. X. (2010). Effects of agricultural intensification on plant diversity in Mediterranean dryland cereal fields. J. Appl. Ecol. 47, 832–840. doi: 10.1111/j.1365-2664.2010.01822.x
Kattge, J., Bönisch, G., Díaz, S., Lavorel, S., Prentice, I. C., Leadley, P., et al. (2020). TRY plant trait database – enhanced coverage and open access. Glob. Change Biol. 26, 119–188. doi: 10.1111/gcb.14904
Kazakou, E., Fried, G., Cheptou, P.-O., Gimenez, O. (2021). Does seed mass drive interspecies variation in the effect of management practices on weed demography? Ecol. Evol. 11, 13166–13174. doi: 10.1002/ece3.8038
Kazakou, E., Fried, G., Richarte, J., Gimenez, O., Violle, C., Metay, A. (2016). A plant trait-based response-and-effect framework to assess vineyard inter-row soil management. Bot. Lett. 163, 373–388. doi: 10.1080/23818107.2016.1232205
Keddy, P. A. (1992). Assembly and response rules: two goals for predictive community ecology. J. Veg. Sci. 3, 157–164. doi: 10.2307/3235676
Keddy, P., Nielsen, K., Weiher, E., Lawson, R. (2002). Relative competitive performance of 63 species of terrestrial herbaceous plants. J. Veg. Sci. 13, 5–16. doi: 10.1111/j.1654-1103.2002.tb02018.x
Kembel, S. W., Cowan, P. D., Helmus, M. R., Cornwell, W. K., Morlon, H., Ackerly, D. D., et al. (2010). Picante: R tools for integrating phylogenies and ecology. Bioinformatics 26, 1463–1464. doi: 10.1093/bioinformatics/btq166
Laliberté, E., Legendre, P. (2010). A distance-based framework for measuring functional diversity from multiple traits. Ecology 91, 299–305. doi: 10.1890/08-2244.1
Larson, C. D., Menalled, F. D., Lehnhoff, E. A., Seipel, T (2021). Plant community responses to integrating livestock into a reduced-till organic cropping system. Ecosphere 12, e03412. doi: 10.1002/ecs2.3412
Lavorel, S. (2013). Plant functional effects on ecosystem services. J. Ecol. 101, 4–8. doi: 10.1111/1365-2745.12031
Lepš, J. (2014). Scale- and time-dependent effects of fertilization, mowing and dominant removal on a grassland community during a 15-year experiment. J. Appl. Ecol. 51, 978–987. doi: 10.1111/1365-2664.12255
Lhotsky, B., Kovács, B., Ónodi, G., Csecserits, A., Rédei, T., Lengyel, A., et al. (2016). Changes in assembly rules along a stress gradient from open dry grasslands to wetlands. J. Ecol. 104, 507–517. doi: 10.1111/1365-2745.12532
Lüdecke, D., Ben-Shachar, M. S., Patil, I., Waggoner, P., Makowski, D. (2021). Performance: An r package for assessment, comparison and testing of statistical models. J. Open Source Software 6, 3139. doi: 10.21105/joss.03139
Macarthur, R., Levins, R. (1967). The limiting similarity, convergence, and divergence of coexisting species. Am. Nat. 101, 377–385. doi: 10.1086/282505
MacLaren, C., Storkey, J., Menegat, A., Metcalfe, H., Dehnen-Schmutz, K. (2020). An ecological future for weed science to sustain crop production and the environment. a review. Agron. Sustain. Dev. 40, 24. doi: 10.1007/s13593-020-00631-6
Mainardis, M., Boscutti, F., Rubio Cebolla, M. D. M., Pergher, G. (2020). Comparison between flaming, mowing and tillage weed control in the vineyard: Effects on plant community, diversity and abundance. PloS One 15, e0238396. doi: 10.1371/journal.pone.0238396
Martínez-Uña, A., Martín, J. M., Fernández-Quintanilla, C., Dorado, J. (2013). Provisioning floral resources to attract aphidophagous hoverflies (Diptera: Syrphidae) useful for pest management in central Spain. J. Econ. Entomol. 106, 2327–2335. doi: 10.1603/EC13180
Mason, N. W. H., Bello, F., Mouillot, D., Pavoine, S., Dray, S. (2013). A guide for using functional diversity indices to reveal changes in assembly processes along ecological gradients. J. Veg. Sci. 24, 794–806. doi: 10.1111/jvs.12013
Mason, N. W. H., de Bello, F., Doležal, J., Lepš, J. (2011). Niche overlap reveals the effects of competition, disturbance and contrasting assembly processes in experimental grassland communities. J. Ecol. 99, 788–796. doi: 10.1111/j.1365-2745.2011.01801.x
Mason, N. W. H., Mouillot, D., Lee, W. G., Wilson, J. B. (2005). Functional richness, functional evenness and functional divergence: the primary components of functional diversity. Oikos 111, 112–118. doi: 10.1111/j.0030-1299.2005.13886.x
Mason, N. W. H., Richardson, S. J., Peltzer, D. A., Bello, F., Wardle, D. A., Allen, R. B. (2012). Changes in coexistence mechanisms along a long-term soil chronosequence revealed by functional trait diversity. J. Ecol. 100, 678–689. doi: 10.1111/j.1365-2745.2012.01965.x
Mayfield, M. M., Levine, J. M. (2010). Opposing effects of competitive exclusion on the phylogenetic structure of communities. Ecol. Lett. 13, 1085–1093. doi: 10.1111/j.1461-0248.2010.01509.x
Monteiro, A., Lopes, C. M. (2007). Influence of cover crop on water use and performance of vineyard in Mediterranean Portugal. Agric. Ecosyst. Environ. 121, 336–342. doi: 10.1016/j.agee.2006.11.016
Mouchet, M. A., Villéger, S., Mason, N. W. H., Mouillot, D. (2010). Functional diversity measures: an overview of their redundancy and their ability to discriminate community assembly rules. Funct. Ecol. 24, 867–876. doi: 10.1111/j.1365-2435.2010.01695.x
Mudrák, O., Janeček, Š., Götzenberger, L., Mason, N. W. H., Horník, J., de Castro, I., et al. (2016). Fine-scale coexistence patterns along a productivity gradient in wet meadows: shifts from trait convergence to divergence. Ecography 39, 338–348. doi: 10.1111/ecog.01723
Navas, M., Violle, C. (2009). Plant traits related to competition: how do they shape the functional diversity of communities? Community Ecol. 10, 131–137. doi: 10.1556/comec.10.2009.1.15
Nogueira, C., Nunes, A., Bugalho, M. N., Branquinho, C., McCulley, R. L., Caldeira, M. C. (2018). Nutrient addition and drought interact to change the structure and decrease the functional diversity of a Mediterranean grassland. Front. Ecol. Evol. 6. doi: 10.3389/fevo.2018.00155
Novara, A., Gristina, L., Saladino, S. S., Santoro, A., Cerdà, A. (2011). Soil erosion assessment on tillage and alternative soil managements in a Sicilian vineyard. Soil Tillage Res. 117, 140–147. doi: 10.1016/j.still.2011.09.007
Novara, A., Minacapilli, M., Santoro, A., Rodrigo-Comino, J., Carrubba, A., Sarno, M., et al. (2019). Real cover crops contribution to soil organic carbon sequestration in sloping vineyard. Sci. Total Environ. 652, 300–306. doi: 10.1016/j.scitotenv.2018.10.247
Oerke, E.-C. (2006). Crop losses to pests. J. Agric. Sci. 144, 31–43. doi: 10.1017/S0021859605005708
Pakeman, R. J. (2011). Functional diversity indices reveal the impacts of land use intensification on plant community assembly. J. Ecol. 99, 1143–1151. doi: 10.1111/j.1365-2745.2011.01853.x
Pakeman, R. J., Hewison, R. L., Riach, D., Fisher, J. M., Hurskainen, S., Fielding, D. A., et al. (2017). Long-term functional structure and functional diversity changes in Scottish grasslands. Agric. Ecosyst. Environ. 247, 352–362. doi: 10.1016/j.agee.2017.06.033
Petchey, O. L., Gaston, K. J. (2002). Functional diversity (FD), species richness and community composition. Ecol. Lett. 5, 402–411. doi: 10.1046/j.1461-0248.2002.00339.x
Prosdocimi, M., Cerdà, A., Tarolli, P. (2016). Soil water erosion on Mediterranean vineyards: A review. CATENA 141, 1–21. doi: 10.1016/j.catena.2016.02.010
Rao, C. R. (1982). Diversity and dissimilarity coefficients: A unified approach. Theor. Popul. Biol. 21, 24–43. doi: 10.1016/0040-5809(82)90004-1
Raunkiaer, C. (1934). The life forms of plants and statistical plant geography (Oxford: Oxford University Press).
R Core Team (2022). R: A language and environment for statistical computing (Vienna. Austria: R Foundation for Statistical Computing). Available at: https://www.R-project.org/.
Ricotta, C., de Bello, F., Moretti, M., Caccianiga, M., Cerabolini, B. E. L., Pavoine, S. (2016). Measuring the functional redundancy of biological communities: a quantitative guide. Methods Ecol. Evol. 7, 1386–1395. doi: 10.1111/2041-210X.12604
Schleuter, D., Daufresne, M., Massol, F., Argillier, C. (2010). A user’s guide to functional diversity indices. Ecol. Monogr. 80, 469–484. doi: 10.1890/08-2225.1
Shmida, A., Wilson, M. V. (1985). Biological determinants of species diversity. J. Biogeogr. 12, 1–20. doi: 10.2307/2845026
Sonnier, G., Shipley, B., Navas, M.-L. (2010). Plant traits, species pools and the prediction of relative abundance in plant communities: a maximum entropy approach. J. Veg. Sci. 21, 318–331. doi: 10.1111/j.1654-1103.2009.01145.x
Spasojevic, M. J., Suding, K. N. (2012). Inferring community assembly mechanisms from functional diversity patterns: the importance of multiple assembly processes. J. Ecol. 100, 652–661. doi: 10.1111/j.1365-2745.2011.01945.x
Storkey, J., Meyer, S., Still, K. S., Leuschner, C. (2012). The impact of agricultural intensification and land-use change on the European arable flora. Proc. R. Soc B Biol. Sci. 279, 1421–1429. doi: 10.1098/rspb.2011.1686
Tarifa, R., Martínez-Núñez, C., Valera, F., González-Varo, J. P., Salido, T., Rey, P. J. (2021). Agricultural intensification erodes taxonomic and functional diversity in Mediterranean olive groves by filtering out rare species. J. Appl. Ecol. 58, 2266–2276. doi: 10.1111/1365-2664.13970
Thompson, K., Petchey, O. L., Askew, A. P., Dunnett, N. P., Beckerman, A. P., Willis, A. J. (2010). Little evidence for limiting similarity in a long-term study of a roadside plant community. J. Ecol. 98, 480–487. doi: 10.1111/j.1365-2745.2009.01610.x
Thompson, K., Bakker, J. P., Bekker, R. M., Hodgson, J. G (1998). Ecological correlates of seed persistence in soil in the north-west European flora. J. Ecol. 86, 163–169. doi: 10.1046/j.1365-2745.1998.00240.x
Turnbull, L. A., Coomes, D., Hector, A., Rees, M. (2004). Seed mass and the competition/colonization trade-off: competitive interactions and spatial patterns in a guild of annual plants. J. Ecol. 92, 97–109. doi: 10.1111/j.1365-2745.2004.00856.x
Villéger, S., Mason, N. W. H., Mouillot, D. (2008). New multidimensional functional diversity indices for a multifaceted framework in functional ecology. Ecology 89, 2290–2301. doi: 10.1890/07-1206.1
Weil, S.-S., Martinez-Almoyna, C., Piton, G., Renaud, J., Boulangeat, L., Foulquier, A., et al. (2021). Strong links between plant traits and microbial activities but different abiotic drivers in mountain grasslands. J. Biogeogr. 48, 2755–2770. doi: 10.1111/jbi.14235
Winter, S., Bauer, T., Strauss, P., Kratschmer, S., Paredes, D., Popescu, D., et al. (2018). Effects of vegetation management intensity on biodiversity and ecosystem services in vineyards: A meta-analysis. J. Appl. Ecol. 55, 2484–2495. doi: 10.1111/1365-2664.13124
Keywords: biodiversity, agricultural management, spontaneous plant cover, mowing, tillage, herbicide, Mediterranean vineyards
Citation: Guerra JG, Cabello F, Fernández-Quintanilla C, Peña JM and Dorado J (2022) Plant functional diversity is affected by weed management through processes of trait convergence and divergence. Front. Plant Sci. 13:993051. doi: 10.3389/fpls.2022.993051
Received: 13 July 2022; Accepted: 15 September 2022;
Published: 07 October 2022.
Edited by:
Boris Rewald, University of Natural Resources and Life Sciences Vienna, AustriaReviewed by:
Daniel Nadal-Sala, Karlsruhe Institute of Technology (KIT), GermanyCopyright © 2022 Guerra, Cabello, Fernández-Quintanilla, Peña and Dorado. This is an open-access article distributed under the terms of the Creative Commons Attribution License (CC BY). The use, distribution or reproduction in other forums is permitted, provided the original author(s) and the copyright owner(s) are credited and that the original publication in this journal is cited, in accordance with accepted academic practice. No use, distribution or reproduction is permitted which does not comply with these terms.
*Correspondence: José Dorado, am9zZS5kb3JhZG9AY3NpYy5lcw==
Disclaimer: All claims expressed in this article are solely those of the authors and do not necessarily represent those of their affiliated organizations, or those of the publisher, the editors and the reviewers. Any product that may be evaluated in this article or claim that may be made by its manufacturer is not guaranteed or endorsed by the publisher.
Research integrity at Frontiers
Learn more about the work of our research integrity team to safeguard the quality of each article we publish.