- 1Department of Plant and Microbial Biology, University of Zurich, Zurich, Switzerland
- 2Agroscope, Molecular Ecology and Swiss Institute of Bioinformatics, Zurich, Switzerland
- 3ETH Zürich, Institute of Molecular Systems Biology, Zurich, Switzerland
Rhizobia fix nitrogen within root nodules of host plants where nitrogenase expression is strictly controlled by its key regulator NifA. We recently discovered that in nodules infected by the beta-rhizobial strain Paraburkholderia phymatum STM815, NifA controls expression of two bacterial auxin synthesis genes. Both the iaaM and iaaH transcripts, as well as the metabolites indole-acetamide (IAM) and indole-3-acetic acid (IAA) showed increased abundance in nodules occupied by a nifA mutant compared to wild-type nodules. Here, we document the structural changes that a P. phymatum nifA mutant induces in common bean (Phaseolus vulgaris) nodules, eventually leading to hypernodulation. To investigate the role of the P. phymatum iaaMH genes during symbiosis, we monitored their expression in presence and absence of NifA over different stages of the symbiosis. The iaaMH genes were found to be under negative control of NifA in all symbiotic stages. While a P. phymatum iaaMH mutant produced the same number of nodules and nitrogenase activity as the wild-type strain, the nifA mutant produced more nodules than the wild-type that clustered into regularly-patterned root zones. Mutation of the iaaMH genes in a nifA mutant background reduced the presence of these nodule clusters on the root. We further show that the P. phymatum iaaMH genes are located in a region of the symbiotic plasmid with a significantly lower GC content and exhibit high similarity to two genes of the IAM pathway often used by bacterial phytopathogens to deploy IAA as a virulence factor. Overall, our data suggest that the increased abundance of rhizobial auxin in the non-fixing nifA mutant strain enables greater root infection rates and a role for bacterial auxin production in the control of early stage symbiotic interactions.
Introduction
Rhizobia are nitrogen (N2)-fixing symbiotic bacteria that trigger the formation of root organs in leguminous plants, called nodules, where biological nitrogen fixation (BNF) takes place (Poole and Allaway, 2000; Masson-Boivin et al., 2009; Oldroyd et al., 2011). The establishment of this symbiotic interaction relies on a sophisticated molecular dialog between bacterial and plant partners (Lee and Hirsch, 2006; Ferguson et al., 2010; Oldroyd et al., 2011; Via et al., 2016). Plants first exude flavonoids in the rhizosphere, which chemoattract rhizobia to the roots (Lee and Hirsch, 2006; Cooper, 2007; Ferguson et al., 2010; Via et al., 2016). In response to flavonoids, bacteria induce the expression of the nod (nodulation) genes responsible for the biosynthesis of lipochitooligosaccharides known as nodulation factors (NFs). The perception of NFs leads to root hair deformation and curling that entrap the bacteria in an infection pocket (Gage and Margolin, 2000; Gage, 2004; Ferguson et al., 2010; Oldroyd et al., 2011). In parallel, NFs activate a signaling cascade in the plant cells that leads via a calcium spiking response to massive cell division in the root cortex. Then, multiplying bacteria penetrate the infection threads (ITs) formed toward the nodule primordia, where rhizobia are released into plant cells and differentiated into N2-fixing bacteroids (Gage, 2004; Lee and Hirsch, 2006; Oldroyd et al., 2011; Poole et al., 2018). The expression of the rhizobial nif (nitrogen fixation) genes is nodule-specific and is activated by the alternative RNA polymerase sigma factor σ54 (or RpoN) together with its enhancer binding protein NifA (Hauser et al., 2007; Lardi et al., 2018). The impact of the loss of NifA function on nodulation, in addition to the absence of nitrogenase activity (Fix–), has been described in several legume symbioses. Despite the different nodule types, the Fix– nodules triggered by nifA mutant symbionts were often reported impaired in leghemoglobin production, to structurally decay, and/or eventually turn necrotic. For instance, the roots of Lotus japonicus colonized with the Mesorhizobium loti MAFF303099 nifA2 mutant display minute white nodules (Nukui et al., 2006) while Medicago truncatula plants inoculated with the Sinorhizobium meliloti 1021 nifA mutant develop Fix– mature nodules but, in contrast to wild-type symbionts, the bacteria prematurely die shortly after bacteroid elongation (Berrabah et al., 2015). A Bradyrhizobium diazoefficiens strain USDA110 nifA mutant showed an increased number of nodules all over the soybean root system and the induced nodules were necrotic (Fischer et al., 1986; Parniske et al., 1991). During symbiosis with Aeschynomene americana, the Fix– Bradyrhizobium sp. DOA9 nifAp nifAc double mutant induced smaller nodules without impacting nodulation frequency (Wongdee et al., 2018). These typical Fix– phenotypes led to suboptimal host plant development, hence indicating that NifA function is essential for symbiotic nitrogen fixation and nodule maintenance (Berrabah et al., 2015).
Phytohormones, especially auxin and cytokinin are also involved in coordinating the nodulation process (Ferguson et al., 2010; Oldroyd et al., 2011; Liu et al., 2018; Velandia et al., 2022). Auxins were first discovered as plant hormones playing crucial roles in plant growth, development, embryogenesis and tropism (Zhao, 2010). Several studies have shown that auxin transport and localization are important for nodule organogenesis during rhizobia-legume symbiosis since auxin influences nodule initiation, differentiation and vascular bundle formation (Mathesius, 2008; Kohlen et al., 2018; Shrestha et al., 2020; Torres et al., 2021). Interestingly, certain rhizobia are also capable of synthetizing auxins, mostly indole-3-acetic acid (IAA), which can directly interfere with plant auxin homeostasis (Mathesius, 2008; Spaepen and Vanderleyden, 2011) and functions as a signaling molecule (Spaepen et al., 2007; Spaepen and Vanderleyden, 2011). The bacterial pathways for IAA synthesis either depend on or are independent of tryptophan (TRP) as precursor (Duca et al., 2014; Zhang et al., 2019). Although the TRP-independent pathway is not well studied yet, five pathways have been categorized as TRP-dependent: the indole-3-acetamide (IAM), the indole-3-pyruvic acid (IPA), the tryptamine, the indole-3-acetonitrile and the tryptophan side-chain oxidase pathways (Spaepen et al., 2007; Zhang et al., 2019). In microorganisms, the two most common and better studied pathways are the IAM and IPA pathways (Supplementary Figure 1; Spaepen et al., 2007; Morffy and Strader, 2020). In the IAM pathway that is mainly present and used by phytopathogenic bacteria, a monooxygenase (encoded by iaaM) converts TRP into IAM, which is then hydrolyzed to IAA and ammonia by the IAM hydrolase (encoded by iaaH). The IPA pathway converts TRP into IAA in three steps. First an aminotransferase converts TRP to IPA that is then decarboxylated to indole-3-acetaldehyde (IAAld) by the indole-3-pyruvate decarboxylase IpdC. IPA is finally oxidized to IAA by the IAAld dehydrogenase (Spaepen and Vanderleyden, 2011; Zhang et al., 2019; Morffy and Strader, 2020). Although this pathway can be found in some plant pathogens, it has been mostly associated with beneficial bacteria (Spaepen et al., 2007; Spaepen and Vanderleyden, 2011; Patten et al., 2013). In rhizobia-legume symbiosis, bacteria contribute to the production of IAA in nodules (Spaepen et al., 2007; Spaepen and Vanderleyden, 2011). Studies on Rhizobium leguminosarum bv. viciae LPR1105 genetically engineered to express iaaM from Pseudomonas savastanoi and tms2 from Agrobacterium tumefaciens, suggested that IAA plays a role in the maintenance of a functional root nodule in Vicia hirsute, since nodules of this genetic variant exhibited a more efficient nitrogenase activity, a bigger size and a delayed senescence (Camerini et al., 2008).
Paraburkholderia phymatum STM815 is one of the main model organisms to study beta-rhizobia-legume symbiosis (Moulin et al., 2001) and stands out for its ability to establish N2-fixing symbiosis with a wide range of legumes, including the economically important Phaseolus vulgaris (common bean) (Elliott et al., 2006; Gyaneshwar et al., 2011; Mishra et al., 2012; Moulin et al., 2014; Lemaire et al., 2016; Lardi et al., 2017a). We previously showed that P. phymatum NifA is essential for nitrogenase activity during symbiosis and that a P. phymatum nifA mutant induced an increased number of P. vulgaris nodules compared to plants infected with the wild-type strain (Lardi et al., 2017b). Furthermore, a combined metabolomics and dual RNA-sequencing analysis of common bean nodules induced by a P. phymatum nifA mutant, revealed that NifA down-regulates the expression of P. phymatum’s auxin genes iaaM and iaaH thus leading to IAM and IAA accumulation. In contrast to the above-mentioned studies on legume symbioses with different rhizobial nifA mutants, the loss of NifA in P. phymatum did not trigger an immune response against the mutant bacteria (Bellés-Sancho et al., 2021a).
Here, we further investigate the effect in the absence of NifA on nodule architecture as well as the role of NifA in controlling the expression of iaaMH during different stages of symbiosis, from the plant-rhizobia recognition step until the development of a mature root nodule. Our data showed that nodules induced by the P. phymatum nifA mutant developed normally despite their clustered organization. We show that NifA represses the expression of the iaaMH genes in all stages of symbiosis. Furthermore, by constructing a mutant strain lacking the iaaMH operon, we demonstrated that P. phymatum produces IAA via the IAM pathway inside P. vulgaris root nodules. While this pathway does not seem to be essential for a functional symbiosis, the up-regulation of iaaMH in a strain lacking NifA was responsible for the increased number of nodule clusters. Finally, orthologs of the P. phymatum iaaMH genes were found in many strains of important phytopathogens such as Pseudomonas syringae and Agrobacterium tumefaciens, suggesting a related role in plant infection.
Materials and methods
Bacterial strains, media, and cultivation
Bacterial strains, primers and plasmids used in this work are listed in Supplementary Table 1. Escherichia coli and P. phymatum strains were routinely cultivated in Luria-Bertani (LB) (Miller, 1972) media and its modified version without salt (LB-NaCl), respectively (Liu et al., 2020). Escherichia coli strains were grown aerobically at 37°C and 220 rpm, while P. phymatum strains were incubated aerobically at 28°C and 180 rpm. When required, the growth medium was supplemented with the following antibiotics at the indicated concentrations: chloramphenicol (Cm, 20 μg/mL for E. coli and 80 μg/mL for P. phymatum), kanamycin (Km, 25 μg/mL for E. coli and 50 μg/mL for P. phymatum) or trimethoprim (Tm, 50 μg/mL for E. coli and 100 μg/mL for P. phymatum). For the gfp-expression analysis with germinated seeds, bacteria were grown on (A) BG medium [(A) B-minimal media supplemented with 0.3 mM NH4Cl and 10 mM glucose as carbon source] (Liu et al., 2020).
Construction of Paraburkholderia phymatum GFP reporter strains and mutant strains
To construct the promotor fusion, the promotor region of Bphy_7769 was amplified by PCR with Bphy_7769p_EcoRI_F and Bphy_7769p_SalI_R primers, using as a template P. phymatum STM815 genomic DNA (gDNA). The 207 bp-long product was digested with the restriction enzymes EcoRI and SalI and cloned into the multiple cloning sites in front of the gfp gene in the vector pPROBE-NT using the same restriction sites as the insert. After transformation in E. coli Top10, the cloned sequence was verified by sequencing at Microsynth (Balgach, St. Gallen, Switzerland). The constructed plasmid was transferred into P. phymatum wild-type and a nifA insertional mutant (Lardi et al., 2017b) by triparental mating. The deletion mutant of the iaaMH genes was constructed by cloning an antibiotic resistance gene in between two external flanking sequences of the genes to be deleted. The primers Bphy7769_up_F_EcoRI and Bphy7769_up_R_NdeI were used to amplify the Bphy_7769 upstream fragment of 529 bp and the primers Bphy7768_dn_F_XhoI and Bphy7768_dn_R_EcoRI for the 525 bp-length to get the Bphy_7768 downstream fragment. A trimethoprim cassette dhfr followed by a transcription terminator was digested with NdeI from the p34E-TpTer plasmid (Shastri et al., 2017) and cloned in between the upstream and downstream fragments. The resulting 1,945 bp long DNA fragment was then inserted into the suicide pSHAFT2 vector, resulting in the pSHAFT2:ΔiaaMH plasmid, which was verified by sequencing. The pSHAFT2:ΔiaaMH plasmid was transferred into P. phymatum wild-type by triparental mating. Clones sensitive to Cm and resistant to Tm were selected and purified. The genomic integration of the construct was verified by PCR using Bphy7770_veri_F and Bphy7767_veri_R primers. The auxin deletion mutant was called ΔiaaMH. To construct the nifA-ΔiaaMH double mutant, the pSHAFT-nifA plasmid (Lardi et al., 2017b) was conjugated in P. phymatum ΔiaaMH and clones resistant to Cm and Tm were selected. To generate the complemented strain of the ΔiaaMH mutant and nifA-ΔiaaMH double mutant, both iaaM and iaaH genes with their native promoter were amplified by PCR using the primers Bphy7769_comp_F_XbaI and Bphy7768_comp_R_XbaI. The 3,246 bp-length product was then digested with XbaI and cloned in forward and reverse direction into the multicloning site of the vector pBBR1MCS2 with respect to the direction of lacZα gene. These two iaaMH complemented constructs differ on their promoters: while the expression of iaaMH cloned in forward direction is driven by the promotor of lacZα (called pBBR1MCS2:iaaMH_placZ), the transcription of iaaMH genes cloned in reverse direction relies on their native promotor (pBBR1MCS2:iaaMH). The constructed ΔiaaMH or nifA-ΔiaaMH complemented strains were called ΔiaaMH + pBBR-iaaMH and nifA-ΔiaaMH + pBBR-iaaMH when iaaMH was expressed by their natural promoter or ΔiaaMH/nifA-ΔiaaMH + pBBR-iaaMH(placZ) when they were under the control of the strong promoter. The correct sequence was verified by sequencing using the LacZ_R and Bphy7768_seq_R primers.
Seedling preparation, plant infection, and growth condition
Phaseolus vulgaris, cv. Negro jamapa (common bean) seeds were surface-sterilized with absolute ethanol for 5 mins, 35% solution of H2O2 for another 5 mins and subsequently washed 10 times with sterile deionized water (Talbi et al., 2010). Seeds were placed on 0.8% agarose plates and incubated 48 h at 28°C in the dark for germination. Sprouted seeds were then transferred into yogurt-jars containing sterile vermiculite (VTT-Group, Muttenz, Switzerland) and 160 mL of nitrogen-free Jensen media (Hahn and Hennecke, 1984). Bacterial strains were grown in liquid LB-NaCl overnight as previously described. Then, cultivated cells were collected, washed twice with (A)B-minimal medium (Liu et al., 2020) and adjusted to a final OD600 of 0.025 (107 cells per mL) (Lardi et al., 2017b). One mL was inoculated directly on each germinated seedling. Plants were grown in the green-house with a day/night temperature of 25/22°C with 16 h of light in a constant humidity of 60%. Plants were watered twice a week with sterile deionized water and harvested after 21 days of incubation.
Characterization of symbiotic properties in planta
To assess the symbiotic properties, nodules of 21 days old plants inoculated with P. phymatum strains were harvested. The number of nodules, dry weight per nodule and nitrogenase activity were determined as previously described (Lardi et al., 2017a,b). To obtain the nodule occupancy, at least one nodule per inoculum was surface sterilized as previously described (Lardi et al., 2017a). Nodules were crushed and bacteroids were isolated by plating on LB-NaCl and counting of the colony forming units (CFU). For the quantification of the nodule-clustering phenotype, each root system (n = 15 plants) was completely washed with deionized water and individual basal roots were severed from the primary root and vertically aligned onto one polyester clear sleeve per plant. The individual root systems were scanned (300 dpi, ImageScanner III, GE Healthcare) and the following parameters were recorded from the images with ImageJ. We measured the primary and basal root lengths and numbers and recorded for every nodule of each root its dimensions (ROI area and shape descriptors) and its position on the root axis relative to the proximal extremity of the root.
Histological preparations
To obtain precise cytological features, the cell walls of P. vulgaris nodules were stained with Calcofluor White M2R (Sigma-Aldrich, Buchs, St. Gallen, Switzerland). In brief, fresh nodules midpoint transversal sections were obtained under the binoculars with sterilized fine blades and immediately covered with 20 μl of a filter-sterilized 1 mg/mL Calcofluor White solution in deionized water. After 5 mins of incubation, excess of dye, cell debris and free bacteria were removed by washing thrice with sterile tap water. The sectioned nodules were directly mounted in 10% glycerol on glass slides for acquisitions. Live/Dead staining was performed using the BacLight Bacterial Viability Kit (L7012, Invitrogen, Bleiswijk, Netherlands) according to the manufacturer guidelines. The procedure was applied to bacteroids extracted from fresh nodules after disrupting the tissue in 400 μl sterile 0.9% NaCl with a disposable micropestle and 2 mins centrifugation at 1,500 rpm, or to bacteria recovered from bacteroids into selective medium. For direct assessments of bacteroids viability into host cells, live/dead straining procedure was performed simultaneously to Calcofluor White preparations. All images were obtained from a confocal laser scanning microscope (DM5500Q; Leica, Wetzlar, Germany) fitted with a TCS SPE confocal unit (Leica, Wetzlar, Germany), an ACSAPO 40 × oil-immersion objective (NA = 1.15, Leica, Wetzlar, Germany) or an ACSAPO 10 × dry objective (NA = 0.3, Leica, Wetzlar, Germany), and laser lines set at 405, 488, and 532 nm with fixed detection windows corresponding to the maximum emission peaks of the respective fluorophores, using the LAS software (Leica, Germany). In each experimental set, the acquisition parameters were identical between samples. The obtained images were analyzed with ImageJ.1
In planta gfp-expression analysis
To visualize the induction of iaaMH expression on the surface of germinated bean roots, overnight cultures of P. phymatum gfp-reporter strains with empty pPROBE, pnodB, and piaaMH in the wild-type and nifA mutant strain were washed twice with 10 mM MgSO4 and inoculated in melted 0.8% agarose (A)BG medium to a final OD600 of 0.05. Germinated seeds were placed in the middle of the plate with the tip of the primary root submerged into the medium while the agarose medium was still liquid. After solidification, plates were incubated for 3 days at 28°C. Three independent biological replicates were prepared for this assay. The fluorescence was then quantified using a Leica M205 FCA fluorescent stereo microscope equipped with a DFC 7000 T CCD camera and the relative fluorescent signal was acquired through an ET GFP filter set (470/40 nm excitation, 525/50 nm emission). The GFP expression in bean inoculated with the P. phymatum gfp-reporter strains was monitored 10, 14, and 21 days after inoculation. Next, the sections were placed on a glass slide and observed under the microscope. Deeper inspection of the tissue sections was performed using the confocal microscope with additional Calcofluor White staining when required. The obtained images were analyzed using ImageJ.
Metabolite extraction and data analysis
The metabolite abundance in P. vulgaris root nodules inoculated with different P. phymatum auxin-mutant strains was compared. As previously described, approximately 30 mg of nodules or uninfected roots were immediately frozen with liquid nitrogen and metabolites were extracted using cold methanol (Lardi et al., 2016, 2018; Bellés-Sancho et al., 2021a,b). Three independent biological inoculants were processed with one or two plants per inoculum. Next, the extracts were injected twice and analyzed by non-targeted flow injection-time-of-flight mass spectrometry on an Agilent 6550 QTOF instrument (Agilent Technologies, Santa Clara, CA, USA), using the settings previously described (Fuhrer et al., 2011). A total of 285 ions were detected with distinct m/z within a tolerance of 0.001 Da and were matched to expected deprotonated molecules. The abundance of the metabolites involved in IAA production [according to the KEGG database (Kanehisa and Sato, 2020)] were estimated and compared according to the ion count values for each sample. The complete metabolomics data set, including a list of detected ions, annotations and intensities, is listed in the Supplementary Table 2.
Auxin production quantification
The estimation of the amount of auxinic compounds secreted by P. phymatum wild-type, nifA mutant, iaaMH mutant and the corresponding iaaMH complemented strains, was performed as previously described (Bellés-Sancho et al., 2021a). Briefly, overnight cultures were routinely grown in LB-NaCl and cells were collected by centrifugation (5 mins at 5,000 rpm) and washed twice with LB-NaCl. An initial cell density of OD600 = 0.05 was inoculated into 20 mL of LB-NaCl per strain and cultivated for 16 h at 28°C with 180 rpm shaking. Supernatants were collected by centrifugation and mixed with Salkowski’s reagent in a volume ratio of 1:2 (Gordon and Weber, 1951). After 20 mins of incubation in the dark, the mixtures’ absorbances were measured at 535 nm and compared to a standard curve of pure IAA (1003530010, Sigma-Aldrich, Buchs, St. Gallen, Switzerland). Three biological replicates were performed per strain.
Bioinformatic analysis
To identify bacteria that encode orthologs of the iaaMH genes and orthologs of nifA, nifH, and nodA (in P. phymatum STM815, these genes are all located on the 595,108 bp symbiotic plasmid pBPHY02), the protein sequences of IaaM (WP_012406795.1), IaaH (WP_012406794.1), NifA (WP_012406756.1), NifH (WP_012406781.1), and NodA (WP_012406745.1) were searched together against the NCBI’s Identical Protein Groups (IPG) resource using cblaster (Gilchrist and Chooi, 2021). The maximum intergenic distance allowed between conserved hits was set to 600 kb and the presence of at least IaaM and IaaH was required, using cblaster’s default BLAST parameters (e-value threshold of 0.01, minimum protein identity of 30% and query coverage of 50%). To visualize genes between and in the vicinity of these five query genes, the intermediate genes option was enabled and synteny plots of the 150 best-scoring clusters were generated using cblasters plot_cluster mode. By default, cblaster only reports orthologs that are assigned to a cluster, meaning they cannot exceed the specified maximum intergenic distance and must occur on the same contig, a limitation for scaffold-level assemblies. Therefore, the cblaster session file was parsed using a custom Python script to extract both BLAST hits that could be assigned to a cluster as well as those that could not. Specifically, for each strain identified by cblaster, each query protein on top of IaaM and IaaH was checked for having a hit located in a cluster. If at least one hit was part of a cluster, the most similar hit to the query in the cluster was chosen. Otherwise, the hit with overall highest similarity was chosen, regardless of its genomic position. Similarity was defined as the product of sequence coverage and sequence identity, both normalized to 1 (range 0.15–1.0). Strains were grouped by species and the minimal and maximal cluster score assigned by cblaster (based on the number and similarity of genes in the cluster), as well as the minimal and maximal similarity for each gene was calculated. The resulting summary table (Supplementary Table 3) was grouped by genus and species and ordered by their respective maximum cluster scores. The table was then filtered to only contain assigned species (all “sp.” entries were removed) and some additional entries were removed (having a contamination, not being a genome assembly or failing NCBI’s taxonomy check). A phylogenetic tree based on the IaaH and IaaM orthologs in the species listed in Supplementary Table 3 was generated. A single genome was chosen to represent each species: The NCBIs representative genome was chosen if it was identified by cblaster, otherwise the best genome was selected based on NCBIs taxonomy check, completeness of the genome, and the number of contigs reported. The orthologs of both proteins were separately aligned using Clustal Ω 1.2.4 (Sievers et al., 2011) and the automatic model selection mode of RAxML 8.2.12 (Stamatakis, 2014) (PROTGAMMAAUTO) was used to determine the best protein selection model for each protein. The alignments of both genes were concatenated using BioPython 1.79 (Cock et al., 2009) and RAxML was used to create 100 bootstrap trees with partitions defined such that the best model is used for each protein (LG for IaaH and JTT for IaaM, still in PROTGAMMA mode). The resulting bootstrap trees were concatenated and bootstrap values were added to the best tree using RAxML with -f b flag. The tree was visualized using FigTree. To look at conservation on the DNA level, the nucleotide sequence from Bphy_7758 to Bphy_7769 (NC_010627.1:528492-542282) was blasted against NCBIs nt database (downloaded 05.05.2022) using blastn 2.12.0 + with the default settings from NCBIs website. The results were converted to GFF format using the NCBIXML module from BioPython and inspected in IGV (Thorvaldsdóttir et al., 2013). The GC content in the region of the predicted P. phymatum iaa operon (Bphy_7767-9; Burkholderia genome database2; NC_010627:532782-547282) was analyzed using a custom Python script as a sliding window analysis with a window size of 2 kb and a step size of 0.4 kb. A second sliding window analysis with a window size equal to the operon length of 4,500 bases was used to determine if the GC content of the predicted iaa operon significantly deviated from the average GC content of windows of similar size from the plasmid pBPHY02 (NC_010627). The GC content of the predicted operon was calculated separately and its location among all sorted window GC contents was determined. The results were visualized with Matplotlib 3.5.1 (Hunter, 2007; Caswell et al., 2021).
Statistical analysis
The statistical analysis for the characterization of the gfp expression data, symbiotic properties, Salkowski’s indolics quantification and the metabolite’s ion count analysis of the nodules was performed with GraphPad Prism 6.0 using an ordinary one-way ANOVA with Tukey’s multiple comparison (p-value ≤ 0.05). 1D clustering of the pooled nodules positions was performed according to the Jenks natural breaks classification method with 10 classes and 25,000 iterations. A population of 500 randomized values ranging from 0 to 250 mm was used as negative control.
Results
Paraburkholderia phymatum lacking NifA (Fix–) does not develop abnormal nodules in common bean
The Fix– P. phymatum STM815 nifA mutant triggers hypernodulation in P. vulgaris (Lardi et al., 2017b), a phenotype we associated with the repression of the autoregulation of nodulation (AON) system (Bellés-Sancho et al., 2021a). To evaluate the structural effects on common bean nodules induced by the P. phymatum nifA mutant, 11-days old bean plants inoculated with the nifA mutant were compared to inoculations with wild-type and nifA complemented symbionts. The examined bean plants infected with the nifA mutant harbored an increased number of nodules that often clustered in mature parts of the roots (Figure 1A) and unusual structures. This phenotype was reverted by genetic complementation of the nifA mutant. Closer inspection of the mature atypical structures under the microscope revealed that they were in fact composed of manifold nodules that seem fused with each other (Figure 1B). Transversal sections of single young, round nodules and larger, flatter mature nodules displayed no notable histological differences between plants infected with wild-type and nifA, and their overall organization remained consistent with established determinate nodule architectures (Figures 1C,D). However, the sections of clustered nifA nodules showed various levels of fusion of cortical, parenchymal and endodermal tissues, shared vasculature but distinct infection zones, i.e., originating from discrete ITs (Figures 1E,F). We did not find any nifA nodule with necrotic or disorganized internal architecture. Mature wild-type, nifA and nifA complemented nodules indistinctively exhibited the coloration characteristic of leghemoglobin. The organogenesis of nodules occupied with the nifA mutant strain was thus not impaired and the observed cluster-like structures seem to be derived from multiple primordia initiated at very close sites.
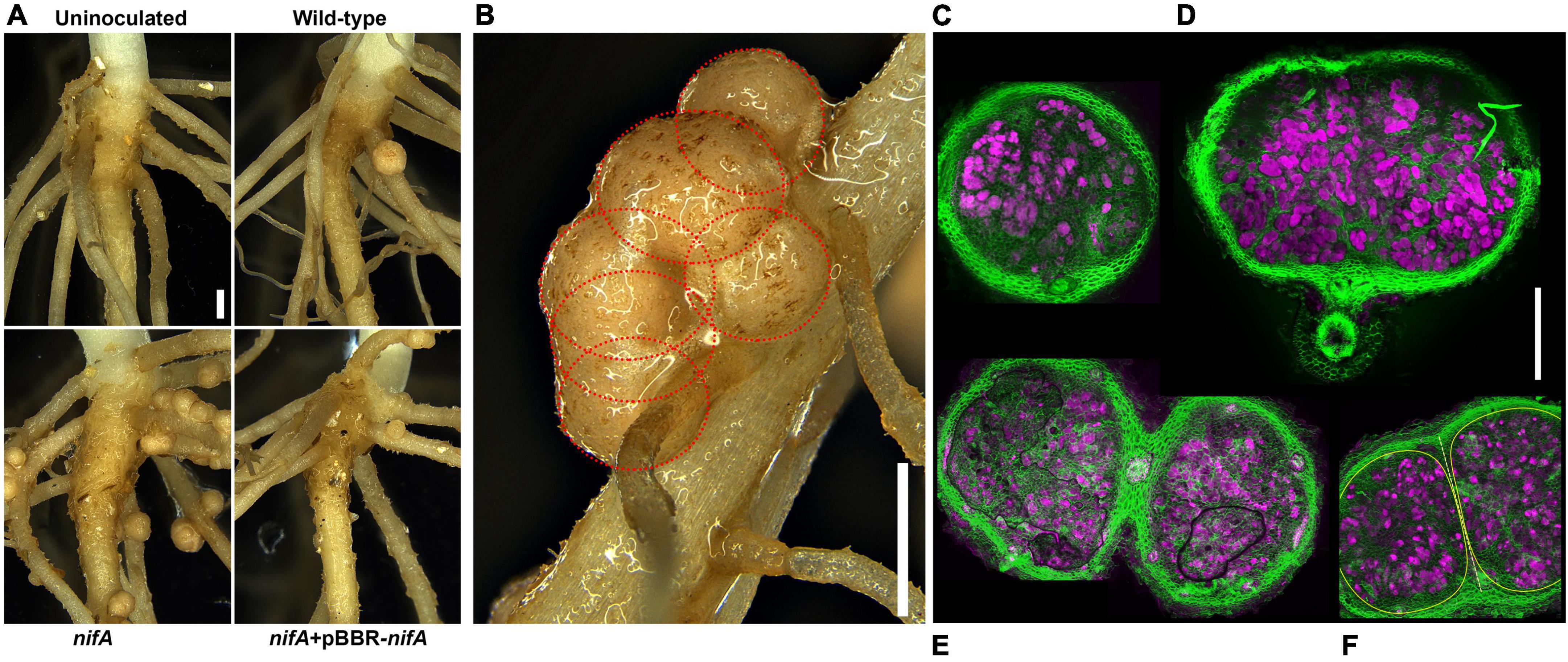
Figure 1. nifA-triggered nodules that fuse during development. (A) Representative root-shoot junctions of 11 days post inoculation (dpi) Phaseolus vulgaris inoculated with or without the indicated strains. Bar = 1 mm. (B) Close-up view of an unusual structure formed by Paraburkholderia phymatum nifA-occupied nodules. The red dotted circles delineate the boundaries of six independent but intertwining nodules. Bar = 1 mm. (C–F) Confocal laser scanning micrographs of nodular transversal sections. Maximum z-projections of 30 μm × 1 μm optical sections. Calcofluor white staining of plant cell walls (green) and Syto9 DNA staining of bacteroids (magenta). Single wild-type or nifA-triggered young (C, here colonized by wild-type P. phymatum) or mature (D, here colonized by P. phymatum nifA mutant) nodules are indistinguishable at macroscopic scale, respectively. (E) Two closely developing nifA nodules sharing cortical and vascular tissues (black lines delineate air bubbles produced in the mounting of the sample). (F) Fused nifA nodules (yellow outlines) separated by a cortical wall (dashed line). Bar = 500 μm.
In situ live/dead staining of mature nodule transversal sections confirmed that wild-type, nifA and nifA complemented bacteroids occupying infected plant cells showed comparable survival rates, with a similar distribution of live/dead cells (Figure 2A and Supplementary Figure 2). However, we observed that nodules colonized by the nifA mutant showed a decreased number of infected cells and an overall lower occupancy of the nodular tissues. Further, plant cells infected by the nifA mutant appeared bigger, longer and rounder than wild-type infected cells (Figure 2B). Interestingly, these alterations were not totally mitigated in nodules occupied by the nifA complemented strain. As cell death of nifA bacteroids occurs in elongating cells (Berrabah et al., 2015), we sought to compare the morphologies of the wild-type and mutant bacteroids obtained from surface-sterilized, crushed nodules. P. phymatum STM815 bacteroids appeared as a mixture of rod-shaped and spherical cells, irrespective of the strain identity, with a significant number of bacteroids undergoing cell division (Figure 2C). We also recovered the bacteroids by growing them on fresh medium with appropriate antibiotic selection and subjected them to the same analysis. All strains grew as rod-shaped bacteria with similar dimensions to the corresponding bacteroids, with a slight increase in dividing cells number for the nifA mutant. It is noteworthy to mention that live/dead staining of these cells did not show significant differences between strains (data not shown). Our data suggest that P. vulgaris nodules occupied by nifA do not drastically differ from wild-type colonized nodules. The only noticeable difference we could associate with NifA loss were disturbances in infected plant cell size and frequency.
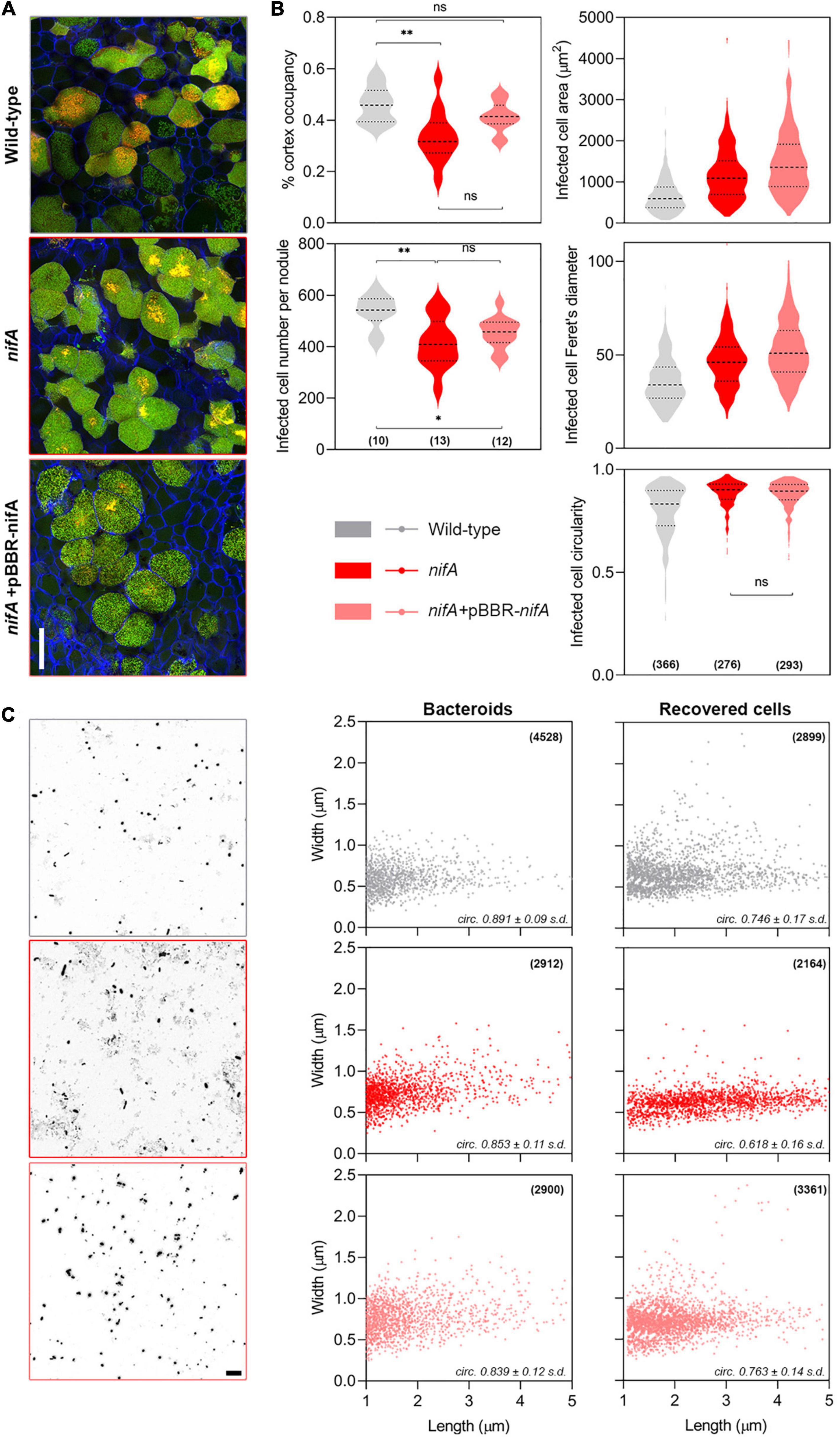
Figure 2. Phenotypic characterization of Phaseolus vulgaris nodules occupied by Paraburkholderia phymatum wild-type, nifA and nifA-complemented strains. (A) Live/Dead staining of infected cells. Confocal laser scanning micrographs of nodular transversal sections. Maximum z-projections of 10 μm × 0.5 μm optical sections. Propidium iodide (red), Syto9 (green), and Calcofluor White (blue). Note the similar distribution of red/green signals between the different samples. All samples are shown at the same magnification, bar = 50 μm. (B) Nodules and plant cells parameters. Numbers in brackets indicate the number of independent samples. Data represent two independent assays. Ordinary one-way ANOVA with Tukey’s post hoc test. The absence of annotation between samples implies p < 0.001. Otherwise **p < 0.01, *p < 0.05, ns, not significant. (C) Bacterial cells parameters. Left, high-contrast bright field micrographs of bacteroids preparations obtained from crushed fresh nodules. Bar = 10 μm. Right, cytometric properties of bacteroids obtained from crushed nodules and bacteroids cultured in selective medium. Cell dimensions were extracted from CLSM micrographs with MicrobeJ. Numbers in brackets indicate sampled cells number. The calculated cell circularity is indicated in italics. s.d., standard deviation of mean.
Paraburkholderia phymatum iaaMH are expressed in presence of germinated seeds and repressed by NifA
To examine the expression of the iaaMH genes in the early stages of symbiosis, 2 day-old germinated P. vulgaris seeds were placed on soft-agar nitrogen-limited medium plates inoculated with P. phymatum STM815 wild-type and a nifA mutant containing the iaaMH-gfp reporter plasmid (hereafter WT-piaaMH and nifA-piaaMH, respectively). P. phymatum wild-type containing the empty pPROBE vector (WT-pPROBE) was used as negative control. As a positive control, the P. phymatum reporter strain pnodB, containing the promotor of the nodB gene (Bphy_7722) fused to gfp, was employed (WT-pnodB) (Hug et al., 2021). The gene nodB is involved in the synthesis of the backbone of NFs, and its expression is known to be induced by flavonoids secreted by the host plant (Guerreiro et al., 1997). After 3 days of incubation at 28°C, the gfp expression of the reporter strains co-inoculated with the germinated seeds was examined under the fluorescence microscope. While the negative control only displayed root autofluorescence, the iaaMH-gfp reporter in the wild-type strain was expressed at a similar level as the nodB-gfp fusion in the vicinity of the root apex (Figure 3A). GFP signals consistently decreased with increasing distance to the root to reach background levels at approx. 3 cm from the apex (Figure 3B). This indicates that nodB and iaaMH promotor activities depended on diffusible root exudates in our assays. In the nifA mutant, iaaMH-gfp signals were two-fold up-regulated compared to wild-type levels close to and also 3 cm away from the root apex (Figure 3B). These results suggest that NifA represses the expression of iaaMH and that root exudates induce iaaMH expression.
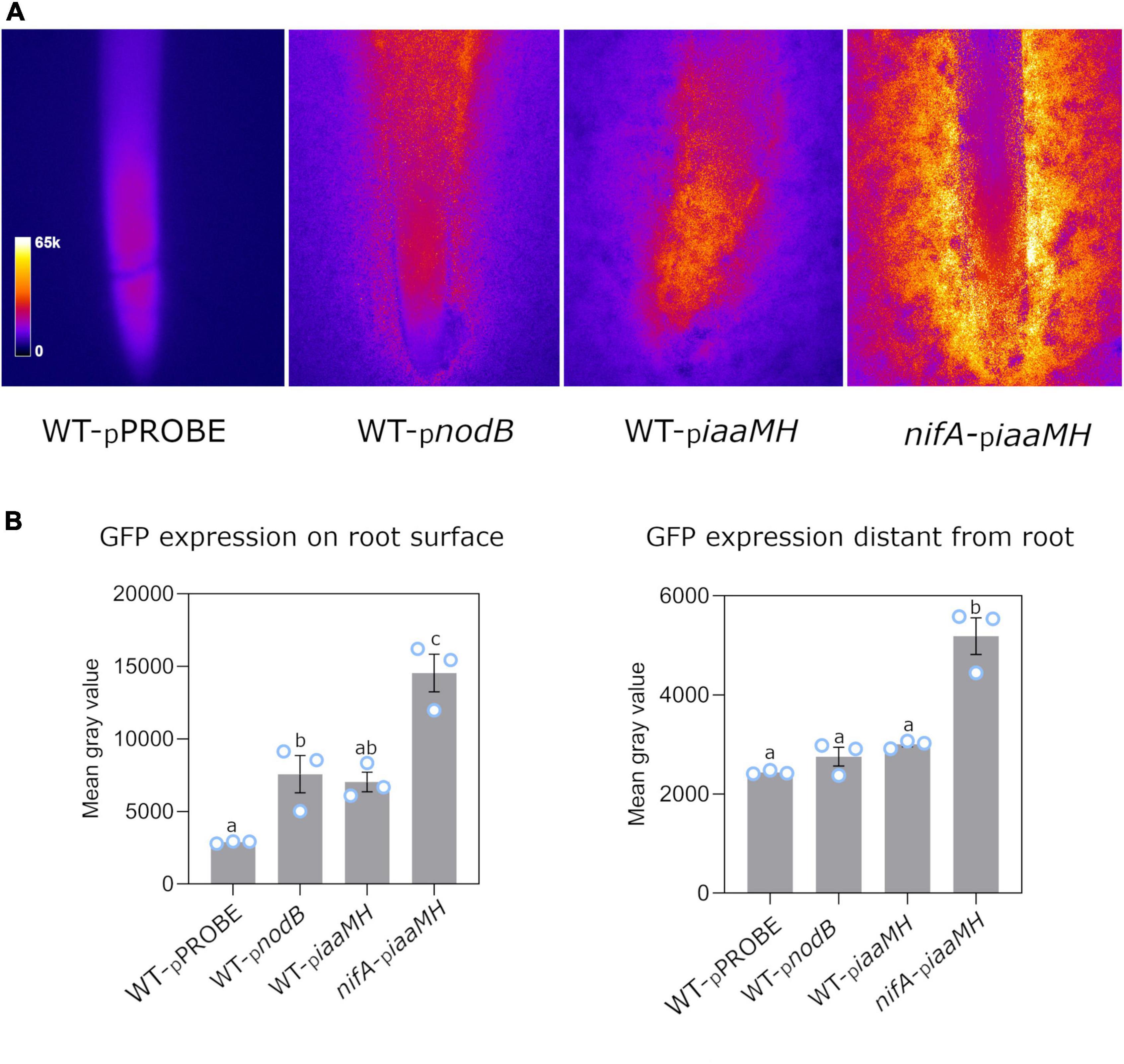
Figure 3. NifA-dependent iaaMH-gfp expression on germinated Phaseolus vulgaris roots in Paraburkholderia phymatum STM815 wild-type (WT-piaamH) and in a nifA mutant (nifA-piaaMH). A gfp reporter construct was used. P. phymatum wild-type containing the empty pPROBE (WT-pPROBE) and the nodB promoter sequence (WT-pnodB) were used as a negative and a positive control, respectively. (A) Representative stereomicrographs of one of the three biological replicates inoculated per strain. Fire lookup table (LUT) indicates fluorescent signal intensity (see insert, left panel). (B) Quantitative analysis of gfp expression on P. vulgaris root surface and at 3 cm distance from the root. Blue circles represent individual data points. Error bars indicate the standard error of the mean (SEM). Significant differences between samples were analyzed with one-way ANOVA with Tukey’s test (p-value ≤ 0.05). Same letters indicate that samples are not statistically significantly different (a–c).
Paraburkholderia phymatum iaaMH expression is constitutive and controlled by NifA during the establishment of Paraburkholderia phymatum–Phaseolus vulgaris symbiosis
To investigate in which step(s) of P. vulgaris nodule organogenesis the P. phymatum iaaMH genes are expressed and controlled by NifA, we used the reporter strains previously described to track the changes in iaaMH expression and bacterial colonization patterns during the different development stages of the symbiosis. Germinated seedlings of P. vulgaris were inoculated with the P. phymatum iaaMH reporter strain in a wild-type (WT-piaaMH) and nifA mutant background (nifA-piaaMH). The plants were incubated for 10, 14 (during the formation of young nodules), and 21 days (optimal nitrogen fixation activity) and the GFP fluorescence signals in developing and mature nodules were microscopically monitored, either non-invasively or from transversal sections. As expected, negative control plants containing the empty pPROBE vector did not show specific GFP signals, although auto fluorescence occurred in mature nodules (Figure 4A). However, the iaaMH promoter was active at all tested time points during the infection process. This is congruent with the data presented in the previous section, pointing to an early activation of bacterial auxin production. Additionally, in absence of NifA, the iaaMH genes were expressed at greater levels compared to the wild type in all stages of nodule organogenesis, suggesting that NifA is tightly controlling auxin biosynthesis throughout the symbiosis. Although we cannot exclude that P. phymatum expressing low levels of iaaMH-gfp were present in our samples and initiated infection sites, we observed a plethora of specific GFP signals strongly associated with the plant tissues. This gfp expression was not due to surface-attached bacteria, as the thorough washing steps we applied to the roots easily removed such microcolonies. We could readily visualize iaaMH-gfp expression from nascent primordia to mature nodules in plants colonized with both strains (Figure 4A). Roots infected with WT-piaaMH occasionally showed fusing infected primordia and numerous scattered GFP punctuae. We found this pattern strikingly enhanced on P. vulgaris roots inoculated with nifA-piaaMH (Figure 4A and Supplementary Figure 3), where iaaMH-gfp promotor activity was regularly observed in multiple adjacent developing nodule primordia. By closely inspecting the distribution of the GFP signals outside of the nodular outgrowths, we located them in direct proximity to root hair cells (Figure 4B). Confocal microscopy established that the majority of these fluorescent signals represent P. phymatum ITs. We could partition these infection sites into three groups: (1) elongated ITs that failed reaching the cortical layer, (2) branching cortical ITs with emerging nodular primordia and (3) large and diffuse ITs occupying infected epidermal cells (Figure 4B). We could not determine if the colonized epidermal cells were preferentially root hairs in the latter group. At day 21 post infection, both plants colonized with WT-piaaMH or nifA-piaaMH showed nodules with leghemoglobin coloration and equivalent GFP signals in their respective infected cells (Figure 4C and Supplementary Figure 4).
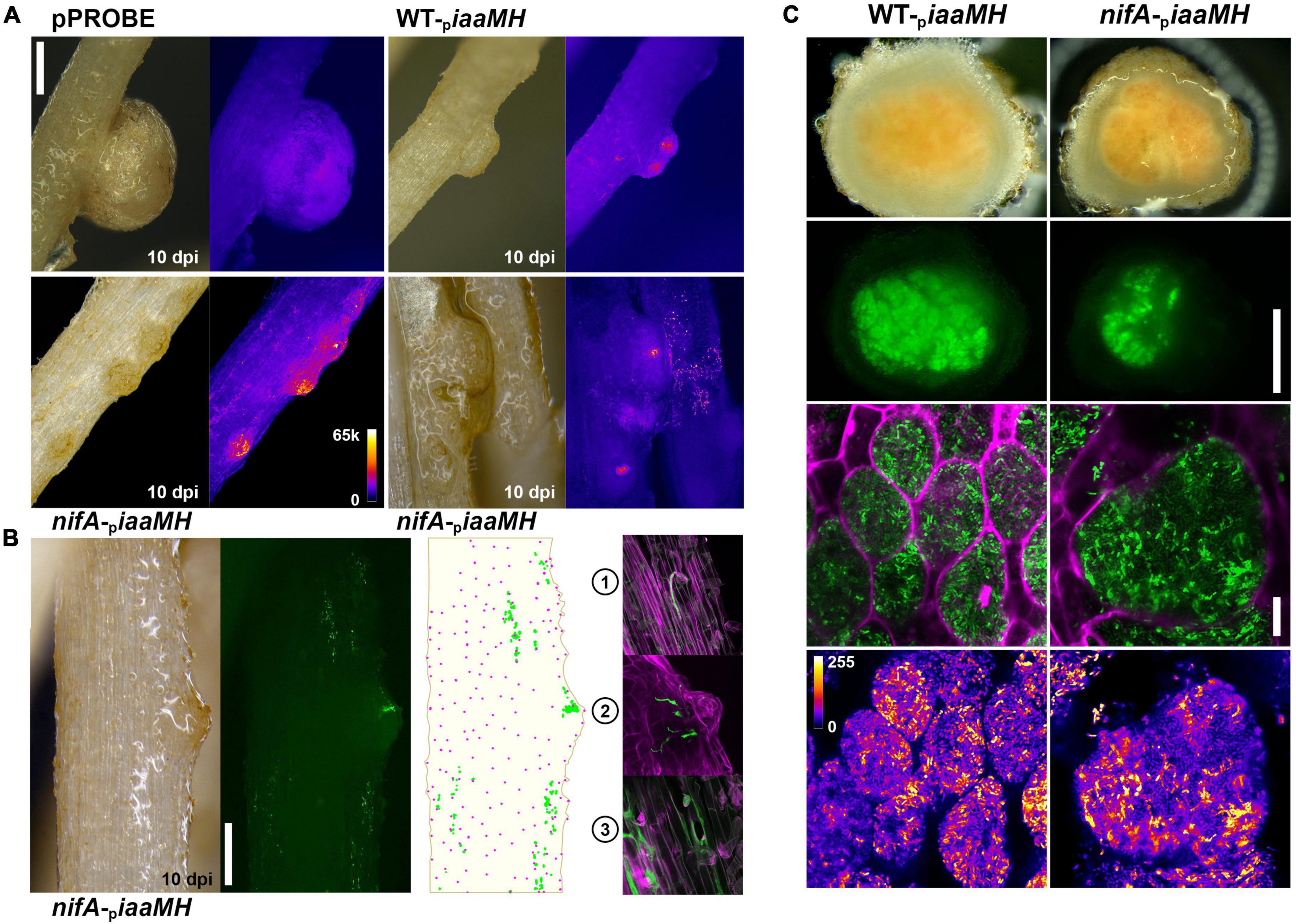
Figure 4. In situ monitoring of iaaMH promotor activity. (A) piaaMH-driven gfp expression in Phaseolus vulgaris roots in the given conditions. Upper-left, note the absence of specific fluorescence in mature nodules occupied by pPROBE-only inoculants. Upper-right, wild-type Paraburkholderia phymatum occasionally triggers fusing nodules. Note the fluorescent signals scattered on the root epidermis. Lower left, note the higher GFP fluorescence in single and fused developing nodules or on the epidermis infected by the nifA mutant. Lower right, two nifA-occupied roots displaying either developing nodules or heavily colonized epidermal area. All micrographs were acquired with the same acquisition settings, Fire LUT, 16-bit depth. Bar = 500 μm. (B) GFP signals distribution on a nifA-colonized root. The cartoon depicts the overlapping of GFP signals (green) and identified root hair positions (magenta) from the micrograph. Bar = 500 μm. (1) An arrested IT. (2) A branching IT and nodular outgrowth. (3) ITs and densely colonized epidermal cells. (C) Upper panel, transversal sections of piaaMH:gfp expressing 21 dpi mature nodules colonized by wild-type and nifA strains. Note the coloration indicating leghemoglobin production in both nodule types. nifA-piaaMH infected cells lose more frequently the fluorescence provided by the pPROBE construct. Bar = 500 μm. Lower panel, piaaMH-driven bacteroids gfp expression in infected cells (green). Calcofluor staining (magenta). Maximum z-projections of 10x × 0.5 μm LSCM optical sections. Lower images display the green signal quantitatively. Fire LUT, 8-bit depth. Bar = 10 μm.
Paraburkholderia phymatum iaaMH genes are essential for the production of indole-acetamide and indole-3-acetic acid in Phaseolus vulgaris nodules
The involvement of the P. phymatum iaaMH genes in the production of indole-acetamide (IAM) and indole-3-acetic acid (IAA) was confirmed using a metabolomics analysis on nodules infected by P. phymatum wild-type, the nifA insertional mutant, an iaaMH deletion mutant (ΔiaaMH) and a nifA-ΔiaaMH double mutant strain. Metabolites of P. vulgaris nodules induced by these different P. phymatum strains were extracted 21 days after inoculation and were compared to those extracted from uninfected roots. Three independent biological replicates inoculated per strain were analyzed using a non-targeted metabolomics approach by flow injection time-of-flight mass spectrometry (Lardi et al., 2016, 2018; Bellés-Sancho et al., 2021a,b). A total of 285 ions were matched to deprotonated metabolites and were annotated based on the accurate mass. Out of all detected metabolites, we focused on the ion counts belonging to IAM and IAA. As we previously reported, plants inoculated with P. phymatum wild-type produced significantly higher amounts of IAM and IAA than non-inoculated plants (Bellés-Sancho et al., 2021a), while the levels of these two auxins in the nifA mutant were found elevated compared to the wild-type nodules (Figure 5; Bellés-Sancho et al., 2021a). The amount of IAM and IAA in nodules induced by the single ΔiaaMH and double nifA-ΔiaaMH mutant decreased to the same levels as observed in non-inoculated roots, suggesting that bacterial auxin production accounted for the main portion of IAA and IAM found in wild-type and nifA nodules (Figure 5). We therefore conclude that the presence of the iaaMH genes lead to IAA and IAM production and confirm that the key regulator of nitrogen fixation, NifA, negatively regulates IAA and IAM amounts in P. phymatum-P. vulgaris nodules. Only few metabolites were found to be differentially abundant in nodules infected with a ΔiaaMH mutant. In addition to IAA and IAM, significantly lower amounts of the metabolites xanthine, linamarin, 4-phospho-L-aspartate and pantothenic acid were found in the nodules infected with the ΔiaaMH mutant compared to the wild-type (Supplementary Table 4). Moreover, in nodules formed by the ΔiaaMH mutant, the intermediate of the lysine degradation pathway saccharopine, the precursor of jasmonic acid alpha-linoleic acid, the serotonin degradation product 5-hydroxylacetic acid and 1-nitronaphthalene-5,6-oxide were detected at higher levels.
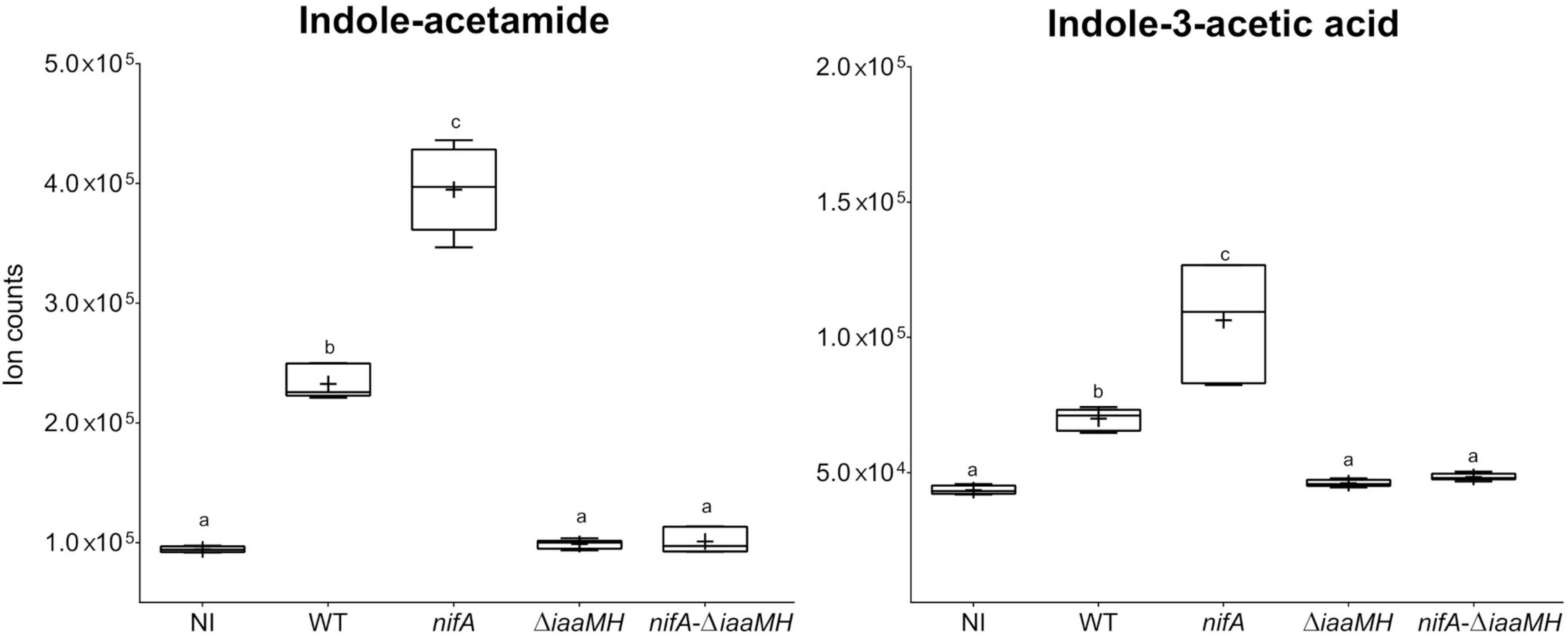
Figure 5. Ion counts observed for indole-acetamide (IAM) and indole-3-acetic acid (IAA). The respective levels are indicated for Phaseolus vulgaris non-inoculated roots (NI) and nodules occupied by Paraburkholderia phymatum wild-type (WT), nifA mutant (nifA), as well as ΔiaaMH and nifA-ΔiaaMH double mutant strains. Plus-symbols (+) indicate the arithmetic mean while whiskers represent minimum and maximum values. Differences between samples were analyzed with one-way ANOVA with Tukey’s test (p-value ≤ 0.05). Statistically significant differences are indicated with different letters (a–c).
NifA-dependent clustering of Phaseolus vulgaris nodules is induced by the presence of Paraburkholderia phymatum iaaMH genes
To evaluate the possible contribution of P. phymatum’s auxin production in the NifA-dependent hypernodulation and nodule clustering phenotype in P. vulgaris (Lardi et al., 2017b; Bellés-Sancho et al., 2021a), the symbiotic properties of P. phymatum wild-type, nifA mutant, ΔiaaMH and the nifA-ΔiaaMH double mutant were characterized. Compared to the plants inoculated with P. phymatum wild-type, plants in symbiosis with ΔiaaMH did not show any significant difference in any of the properties tested (number of nodules, dry weight per nodule, normalized nitrogenase activity), suggesting that the absence of bacterial auxin alone did not significantly affect symbiosis with the common bean (Figure 6 and Supplementary Table 5). Moreover, the ΔiaaMH strain complemented with iaaMH expressed from its natural promoter also showed no difference in the symbiotic properties compared to the mutant strain. The hypernodulation phenotype of the nifA mutant was reverted to wild-type levels when the nifA gene was complemented on a plasmid. Similar to the phenotype observed in plants inoculated with the nifA mutant, the nifA-ΔiaaMH double mutant showed a significantly higher number of nodules compared to the plants inoculated with the wild-type. However, the nodule number in the double mutant was slightly reduced compared to the nifA mutant, although this difference was not significant (Figure 6). This suggests that bacterial auxin contributes to increase hypernodulation when NifA is absent. Like the nifA mutant, the nifA-ΔiaaMH mutant showed no nitrogenase activity, indicating that NifA controls expression of the nitrogenase independently of the presence of the auxin biosynthesis genes. We next determined the number of bacteroids inside nodule occupied by the wild-type and the different nifA and iaaMH mutant strains and found that the nodule occupancy was similar for each strain (Supplementary Figure 5). Remarkably, plants inoculated with ΔiaaMH and the nifA-ΔiaaMH double mutant complemented with iaaMH under control of the strong lacZ promotor induced abnormal, thicker roots without nodules, presumably due to an excess of exogenous auxin production (Supplementary Figure 6). These results confirm that P. phymatum iaaMH is not essential for a functional symbiosis with P. vulgaris. However, auxin seems to influence the nodulation frequency observed with the nifA mutant.
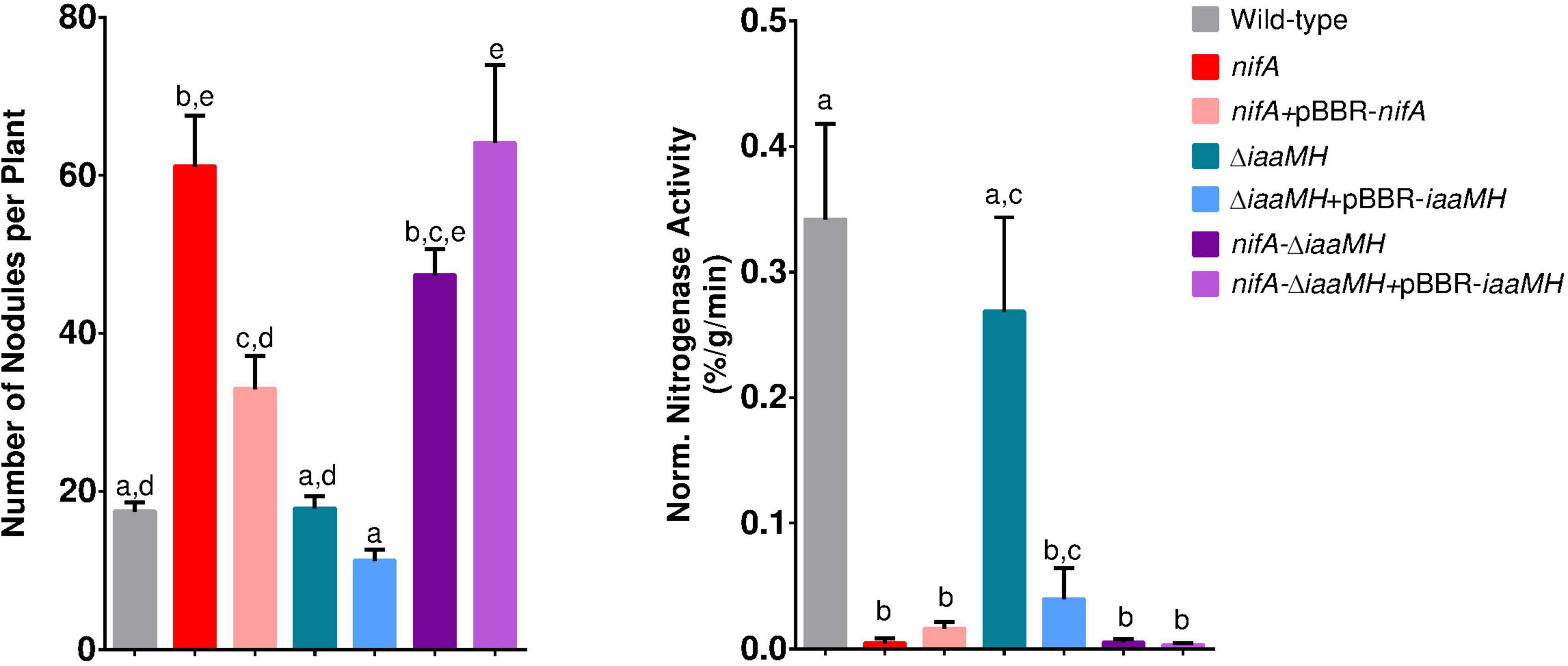
Figure 6. Symbiotic properties of Paraburkholderia phymatum wild-type, nifA mutant, nifA complemented strain, ΔiaaMH, ΔiaaMH complemented strains, nifA-ΔiaaMH double mutant and nifA-ΔiaaMH double mutant complemented with iaaMH. At least four biological replicates were used, consisting of a minimum of four plants per replicate. Error bars indicate the standard error of the mean (SEM). For each histogram, values with the same letter are not significantly different (a–e) (ANOVA, Tukey’s test with p-value ≤ 0.05).
To determine the impact of NifA and IaaMH functions and their interplay at a finer resolution, we conducted a precise phenotypical characterization of common bean plants 21 days post inoculation (dpi) by monitoring their root parameters in presence of different strains. We first recorded the position of each individual nodule along the axis of each tap and basal root from 15 plants. At this stage, all plants presented comparable statures and apparent health. Figure 7A shows the first centimeters of representative basal root colonization. We used this positional information to build a map of nodule frequency distribution for the entire root system of the individual samples (Figure 7B). This dataset allowed us to conclude that, irrespective to the tested bacterial strain, the bulk of P. vulgaris nodulation occurred within the first 75 mm of the root system, with a large portion of nodules developing in the few first centimeters of the roots. This indicated us that the changes in auxin production measured in nifA and iaaMH mutants did not dramatically influence nodule positioning along the root developmental axis. As an imbalance in auxin homeostasis has often been reported to alter root development and growth, we sought to measure the status of the root system in our experiment. The number of basal roots developed per plant were not significantly different between the tested conditions (Figure 7C). We also observed no significant differences in the lengths of tap roots or basal roots between samples, respectively (Figure 7C). Although we did not measure these parameters, the lateral root and root hair densities also appeared homogeneous in all tested plants. Thus, we found no compelling evidence of a direct bacterial auxin effect on the systemic root system architecture, indicating that its contribution to the symbiosis might be locally restricted. In contrast, the loss of NifA function nearly tripled the number of developed nodules on tap and basal roots (Figure 7D). This increase was restored to wild-type levels in plants colonized with the complemented nifA strain, although the number of nodules per basal root remained significantly greater than wild-type numbers. Interestingly, the ΔiaaMH-colonized plants displayed a nodule number per root close to wild type, even slightly lower, a tendency that seemed more pronounced in inoculations with the functionally complemented strain ΔiaaMH + pBBR-iaaMH. Finally, the inactivation of iaaMH in the nifA mutant background resulted in a clear mitigation of the nifA nodulation phenotype, the latter being restored in plants inoculated with a nifA strain harboring the complemented iaaMH mutant. Taken together, our data strongly suggest that NifA governs root nodular density via negative control of iaaMH expression in P. phymatum. However, by visualizing the 1D distribution of the positions of the whole population of nodules on the roots of each condition tested here, it appeared that the obtained dot plots displayed comparable shapes and local densities. We then hypothesized that the hypernodulation triggered in nifA-colonized plants might solely be explained by an enrichment of developed nodules in pre-defined root areas (Figure 7E). We first verified that the sizes of the nodules colonized by the various strains did not significantly differ (Figure 7E). To our surprise, the nodules occupied by the iaaMH mutant appeared smaller. Nevertheless, we retained a mean diameter of 1 mm for all nodules. By calculating the distance between consecutive nodules along the axis of individual roots, the clustering of nifA nodules was manifest (Figure 7E). This phenotype was alleviated by either the complementation of the NifA function or by disrupting IaaMH-mediated auxin synthesis in the nifA background. Restoring iaaMH functionality in the nifA strain led to the nifA phenotype. Single manipulations of the iaaMH genes did not influence nodule clustering when compared to roots colonized with the P. phymatum wild-type. We next categorized the frequency of consecutive nodules spacing into 1 mm bins (Supplementary Figure 7A) and uncovered that 46.3% of all nifA-occupied nodules aggregated into root sections under 3 mm in length. In comparison, nodules occupied with the nifA complemented strain (31.0%), ΔiaaMH (23.3%), ΔiaaMH + pBBR-iaaMH (36.4%), or nifA-ΔiaaMH (37.7%) showed similar clustering to wild-type-colonized nodules (21.5%) in such segments. Within this window, the nodules hosting the nifA-ΔiaaMH + pBBR-iaaMH strain displayed a clustering frequency of 53.8%, congruent with nifA values. To establish if the observed nodular clusters were indiscriminately scattered onto the root axis, we therefore subjected our samples’ 1D root nodular coordinates to Jenks natural breaks optimization (Supplementary Figure 7B). Our computations broke the different datasets into 10 classes with goodness of variance fits over 98%. We deduced from the classes limits the root windows in which nodulation occurred for all tested conditions. Regardless of the inoculants and the number of nodules, P. vulgaris nodulation occurred on roots in discrete windows consistently increasing in size while progressing toward the root apex, a pattern remarkably reminiscent of the lateral root emergence developmental process. Within the first 60–75 mm of roots, all inoculated plants displayed a uniform windowing ranging from 10 to 20 mm, approximatively (blue boxes in Figure 7A). In younger parts of the roots, nearer the apices, the nodules of roots colonized by the nifA, nifA complemented, nifA-ΔiaaMH and nifA-ΔiaaMH + pBBR-iaaMH strains displayed larger nodulation windows. Consistent with the rest of our data, plants hosting wild-type, ΔiaaMH or ΔiaaMH + pBBR-iaaMH shared very similar distributions. In comparison, a population of randomized values were evenly distributed in 10 windows of about 20 mm along a theoretical root axis of 250 mm.
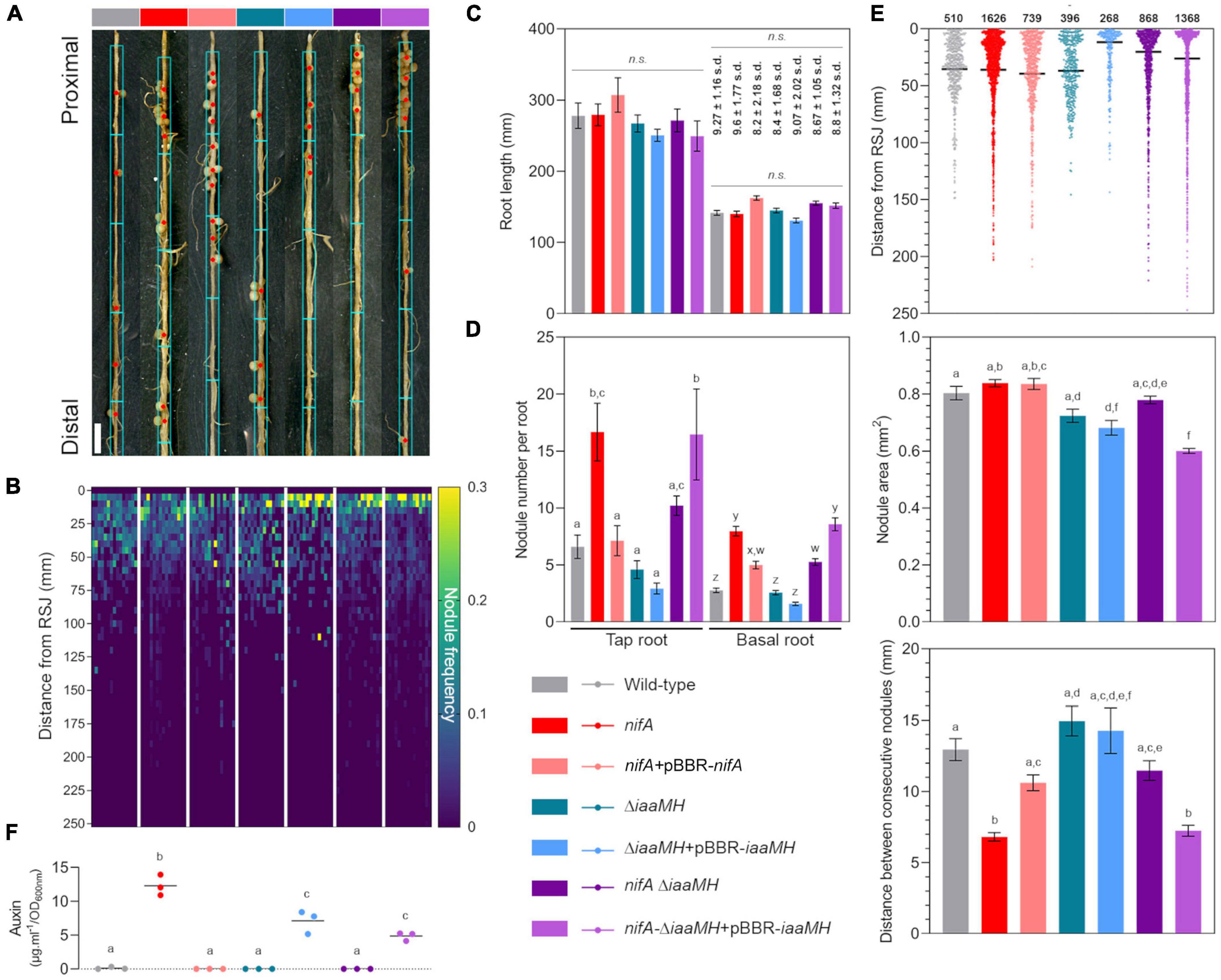
Figure 7. Detailed characterization of Paraburkholderia phymatum-Phaseolus vulgaris symbiosis nodulation patterns in the presence of discrete strains. (A) Representative 21 dpi P. vulgaris basal roots infected with the indicated strain. Red dots indicate the centroid of each single or clustered nodules. Cyan boxes illustrate the suggested nodulation windows along the root axis. Bar = 5 mm. (B) Frequency of nodulation in the entire root system of 15 individual plants in response to the given inoculants. The positions of individual nodules relative to the root-shoot junction were pooled into 5 mm bins. (C) Mean tap and basal root lengths of the sampled plants. Whiskers represent standard error. Bold numbers indicate the mean number of basal roots and standard deviation of the mean. Significant differences between samples means were analyzed with one-way ANOVA with Tukey’s post hoc test (p-value ≤ 0.05). Same letters indicate that means are not statistically significantly different when required. n.s., not significant. (D) Mean nodule number per root type. Whiskers represent standard error. (E) Total nodules parameters for the sampled plants. Individual dots represent single nodule position on the root axis. Black bars represent means. Whiskers represent standard error. Bold numbers indicate the total number of sampled nodules. Significant differences between samples means were analyzed with one-way ANOVA with Tukey’s post hoc test (p-value ≤ 0.05). Same letters indicate that means are not statistically significantly different. (F) Quantification of indolics production in liquid cultures of the given strains in μg⋅ml–1 in relation to the cultures’ optical density OD600. Individual dots represent each different biological replicate. Different letters account for significantly different values.
Taken together, our data suggest that the clustered hypernodulation observed in nifA-colonized plants results from a strong increase in developed nodules originating from discrete, facilitating root sites. In analogy to lateral root development, such sites might be genetically or hormonally primed to respond to internal auxin signaling. To confirm that the nodular levels of auxinics we previously measured (Bellés-Sancho et al., 2021a) originated from bacterial production under NifA-IaaMH regulation, we estimated the amount of indolics in our strains after planktonic growth (Figure 7F). In comparison to wild-type P. phymatum that produced traces of indolics, the nifA mutant synthetized considerable amounts (37.9 μg/ml). This trait was returned to wild-type levels in the nifA-complemented and nifA-ΔiaaMH strains. As expected, the ΔiaaMH was unable to produce auxinics. However, we found that the presence of pBBR-iaaMH in either the ΔiaaMH or nifA-ΔiaaMH backgrounds displayed similar elevated levels of auxinics, thus implying that the production of IAA in these strains is not entirely controlled by NifA. This might, at least partially, explain the lower nodule number observed in plants hosting ΔiaaMH + pBBR-iaaMH and the smaller nodule sizes triggered by strains expressing the pBBR-iaaMH plasmid. We conclude that the P. phymatum IaaMH-dependent auxin synthesis is directly involved into the initiation and development of P. vulgaris nodules in predetermined susceptible zones along the root axis.
The iaaH and iaaM genes are present in the genus Paraburkholderia and are related to auxin production genes from phytopathogenic species
To identify orthologs of P. phymatum STM815’s iaaMH genes in other bacterial species, an in silico analysis using the respective P. phymatum protein sequences as query for a cblaster search was carried out (see section “Bioinformatic analysis” in “Materials and methods”). Strains retrieved from this analysis were required to have both orthologs located adjacent to each other and to have a full species name (sp. hits were not allowed as they might bias the results). Among the 1,001 strains satisfying these criteria, we found 174 Pseudomonas syringae, 134 Agrobacterium tumefaciens, 121 Burkholderia vietnamensis, 93 Pseudomonas chloroaphis, 72 Dickeya dicanthola, 65 Agrobacterium rhizogenes, and 62 Agrobacterium vitis strains. These top seven species accounted for more than 70% of all hits. Overall, the strains belong to 79 different species many of which were represented by a single strain, including several Paraburkholderia species (Supplementary Table 3). To create a phylogenetic tree, we selected one representative strain per species, preferentially including NCBIs representative genome, reference genomes or complete genomes over fragmented Illumina assemblies (see section “Bioinformatic analysis” in “Materials and methods”). The phylogenetic analysis revealed several distinct groups of strains containing IaaM and IaaH (Figure 8A). In order to investigate potential functional links between proteins related to auxin production with other rhizobial traits (such as nitrogen fixation and nodulation) at a genomic level, we analyzed the co-occurrence of nifA, nifH and nodA genes in bacterial strains that encode the iaaMH genes (Supplementary Table 3). Orthologs of all five genes were only present in 30 strains belonging to several species from the genus Paraburkholderia, namely P. phenoliruptix, P. ribeironis, P. atlantica, P. youngii, P. dipogonis, P. nodosa, P. guartelaensis, P. mimosarum, P. caribensis, P. diazotrophica, P. franconis, P. piptadeniae and P. phenazinium, and three strains of Trinickia symbiotica. Notably, P. phenoliruptix was the strain with the highest homology score for all five gene products present on the same contig (minimum and maximum score 3.21 and 8.48, respectively). The homologous region in this strain showed 100% sequence coverage and 99.4% nucleotide identity with the one of P. phymatum (Figure 8B). Three strains belonging to Dickeya genus (D. chrysanthemi, D. dianthicola, and D. dadantii) presented, in addition to iaaMH, the nifA and nifH genes. Among the strains that only carried orthologs of the P. phymatum iaaMH genes (without nif or nod genes), high similarity was found in species belonging to the genera Agrobacterium, Pantoea, Pseudomonas, Rhizobium, Xanthomonas, Pectobacterium, Acinetobacter, Photorhabdus, Xenorhabdus, Liberibacter, Noviherbaspirillum, Streptomyces, and Rhodococcus. Outside of the Paraburkholderia group, the IaaMH orthologs of the phytopathogenic strains A. vitis, A. rhizogenes, and A. tumefaciens showed the highest similarity with the P. phymatum genes. Orthologs of P. phymatum IaaMH (maximum and minimum score 3.08) were also found in Burkholderia strains belonging to the Burkholderia cepacia complex (Bcc) that are opportunistic human pathogens, with the addition of B. vietnamiensis and B. ubonensis which also carry nif genes (Eberl and Vandamme, 2016). The region containing the P. phymatum iaa operon is located on the symbiotic plasmid and showed a significantly lower GC content (a difference of 0.074) compared to the rest of the plasmid (0.592) (Figures 8C,D). Furthermore, our bioinformatic analysis predicted that Bphy_7770 (the gene upstream of iaaM), codes for a putative IS5 family transposase. This result together with the phylogenetic reconstruction analyses suggests that the iaaMH genes in P. phymatum and other Paraburkholderia strains may have been acquired horizontally from a phytopathogenic ancestor.
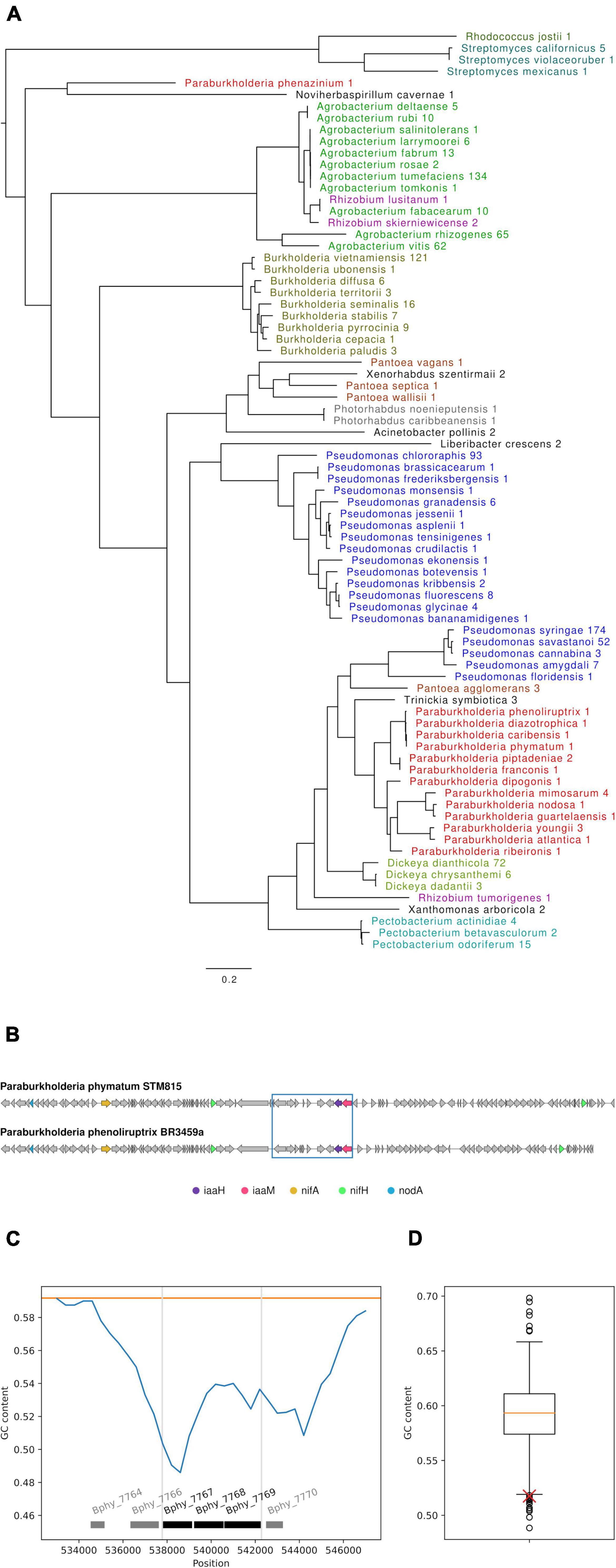
Figure 8. In silico analysis of Paraburkholderia phymatum iaaMH genes. (A) Maximum likelihood phylogenetic tree of co-occuring orthologs of IaaH and IaaM in other species identified by cblaster. The numbers indicate how many strains per species were found. When more than one strain was identified for a species, only the genome best representing the species was chosen (B) Synteny of iaaM (pink), iaaH (purple), nifA (yellow), nifH (green), and nodA (blue) between P. phymatum STM815 (top) and P. phenolirupterix BR3459a (bottom). Note that in both strains, two copies of nifH are present. A BLAST of the DNA sequence from Bphy_7758 to Bphy_7769 (blue box) identified P. phenoliruptrix BR3459a as the only other strain containing the whole sequence (100% sequence coverage of 13,791 nucleotides, 99.4% nucleotide identity). (C) GC content (blue line) of the P. phymatum iaaMH genes and the surrounding genomic region on the symbiotic plasmid, analyzed with a sliding window analysis (see “Bioinformatic analysis” section in “Materials and methods”). The mean GC content of the plasmid is shown as orange line, the extent of the predicted auxin operon is shown by gray vertical lines and the genes in black. A potential IS5 family transposase gene (Bphy_7770) is located to the right side of iaaM. (D) Boxplot showing the GC content distribution of all windows of similar size on the symbiotic plasmid (NC_010627.1). The GC content of the window containing the three predicted auxin operon genes (red cross) shows a significantly lower GC content.
Discussion
We present here the first study of the nodule architecture and organization induced by a beta-rhizobial nifA mutant and associate it to beta-rhizobial auxin synthesis during symbiosis with P. vulgaris.
As reported earlier (Bellés-Sancho et al., 2021a), the P. phymatum nifA mutant triggers nodule clustering (Figures 1A,B). Other than this phenotype, no abnormalities were found in nifA nodules (Figures 1C–F) or root traits. We reasoned that this phenotype might originate from the deregulation of early nodule proliferation, presumably from primary cortical initiation sites. Plant cells infected with the nifA mutant were found larger than the ones infected with the wild type (Figure 2B), a subtle change that we can attribute to the effects of auxin, as the hormone drives cell elongation (Majda and Robert, 2018). No difference was observed between wild-type and nifA bacteroids (Figure 2A), which is in contrast to reports showing that the lack of NifA provokes bacteroid death in S. meliloti 1021-M. truncatula symbiosis (Berrabah et al., 2015) seemingly sanctioning inefficient Fix– symbionts via a plant immunity response.
Interestingly, in alpha-rhizobia, NifA was reported to positively control the production of rhizobial hormones (Nukui et al., 2006; Murset et al., 2012; Nett et al., 2022). For instance, NifA in B. diazoefficiens USDA 110 has been shown to control the synthesis of gibberellin, a plant hormone also produced by bacteria, which induced bigger nodules in soybean in later stages of the symbiosis (Nett et al., 2022). We showed here that during the early phase of the P. phymatum-P. vulgaris symbiosis, the bacterial iaaMH genes are induced by root exudates (Figure 3). In line with this observation, a previous transcriptomics analysis had shown that P. phymatum iaaMH expression was 1.8-fold upregulated when exposed to Mimosa pudica root exudates (Klonowska et al., 2018). Root exudates are composed of a blend of primary and secondary metabolites, including the amino acid TRP, a precursor of IAA (Spaepen et al., 2007; Vives-Peris et al., 2020). In other strains, TRP induces the expression of IAA biosynthetic genes from either the IPA pathway in Enterobacter cloacae UW5 (Ryu and Patten, 2008) or the IAM pathway in P. syringae pv. syringae and D. dadantii 3937 (Duca and Glick, 2020). Flavonoids are also compounds excreted by the roots, which can induce the expression of genes related to IAA synthesis, as shown in Rhizobium sp. NGR234, where the IPA pathway is induced by the transcriptional regulator NodD (Theunis et al., 2004). In addition, flavonoids not only influence bacterial auxin production, but can also inhibit plant auxin transport in roots during nodule formation, leading to auxin accumulation in the infection zone (Mathesius et al., 1998; Brown et al., 2001; Wasson et al., 2006; Peer and Murphy, 2007; Breakspear et al., 2014). A previous transcriptome analysis performed by our group had shown that the expression of the iaaHM operon, at the time annotated as an amine oxidase, was upregulated in P. vulgaris nodules compared to free-living conditions (Lardi et al., 2017b), which would suggest a possible role during symbiosis. However, in this study no obvious symbiotic role of IAA and IAM were observed (Figure 6). We cannot exclude that the absence of a symbiotic phenotype is related to the plant host and the type of nodules (determinate or indeterminate) the plant forms, since legumes use auxin to achieve the initial buildup in different layers of the cortex (Kohlen et al., 2018). For instance, in studies where the bacterial auxin balance was disrupted by overproducing IAA in R. leguminosarum RD20, no difference was observed in determinate P. vulgaris nodules compared to wild type, while in Vicia sativa and M. truncatula (both forming indeterminate nodules) less nodules were formed by this strain (Pii et al., 2007; Camerini et al., 2008). A future characterization of the P. phymatum iaaMH mutant using other plant hosts that form indeterminate nodules will help to further decipher the role of rhizobial auxin in distinct nodule types. In addition, it is important to mention that auxin induces different effects depending on concentration and plant sensitivity toward auxin, i.e., concentrations outside the optimal range lead to an inhibition of plant growth (Persello-Cartieaux et al., 2003; Remans et al., 2008). Noteworthy, abnormal root development and no nodule formation were observed when plants were inoculated with a strain artificially over-expressing iaaMH (Supplementary Figure 6).
Although the P. phymatum iaaMH mutant was not impaired in its symbiotic abilities, the overexpression of the iaaMH genes in a nifA mutant was responsible for the formation of clusters of nodules in adjoining nodule initiation sites on the plant root (Figures 1, 7). This phenotype might arise from the increased number of ITs in roots infected by a nifA mutant compared to the wild type (Figure 4B and Supplementary Figure 3). In line with the current hypothesis that auxin positively regulates epidermal infections (Velandia et al., 2022), auxins overexpression in the P. phymatum nifA mutant seems to act locally and promote the occurrence of infection events. This is reminiscent of the L. japonicum-M. loti symbiosis, where auxin was proposed to be a promotor of the initiation or elongation of the IT formation. In fact, the inhibition of the plant TAR-YUC auxin biosynthesis pathway impaired the IT elongation (Nadzieja et al., 2018). In M. truncatula infected with S. meliloti, it has been shown that a local auxin accumulation correlates with nodule numbers (Roy et al., 2017; Kohlen et al., 2018). In fact, the M. truncatula supernodulation mutant sunn (supernumerary nodules, an AON mutant) (Schnabel et al., 2005), showed an increased level of local auxin transport and expression of the auxin response gene GH3 at the zone of nodule initiation (van Noorden et al., 2006, 2007). Nevertheless, we suggest that P. phymatum NifA activity mainly interplays with the P. vulgaris AON system, finally leading to a nodule number increase in predetermined root zones. In addition, the overproduction of bacterial auxin when NifA is absent is rather altering colonization frequency than being essential for nodulation. The induced infectivity driven by auxin could be associated with an increased competitive fitness of the strain, since P. phymatum is known to outcompete other Paraburkholderia species in nodulating common bean (Lardi et al., 2017a).
Interestingly, the P. phymatum iaa operon shares a high degree of homology with the iaa operon of phytopathogens (Figure 8 and Supplementary Table 3) such as Agrobacterium tumefaciens Q15/94 and P. syringae pv. savastanoi. While A. tumefaciens uses the IAM pathway as a virulence factor to induce crown gall tumors in plants (Zupan et al., 2000), in P. syringae IAA induces virulence (Djami-Tchatchou et al., 2020, 2022) and in P. savastanoi the iaaMH genes are involved in the colonization process of Mytus communis (Schiff et al., 2019). Orthologs of the P. phymatum STM815 iaaMH genes were also present in Burkholderia species belonging to the Bcc group, which includes human opportunistic pathogens (Supplementary Table 3). In P. phymatum and other nitrogen-fixing and nodulating Paraburkholderia strains, in addition to the close related Trinickia symbiotica but not in alpha-rhizobia, the iaaM and iaaH genes co-occur with nitrogen fixation (nif) and nodulation (nod) genes (Figure 8A and Supplementary Table 3), suggesting that the IAA-production could be a specific trait of plant-colonizing Paraburkholderia strains. The only exception was the endophytic P. phenazinium, which only presented the iaaMH (Sexton et al., 2020). Based on our phylogenetic analysis (Figure 8A) and the low GC content of the predicted P. phymatum iaa operon compared to the GC content average of the symbiotic plasmid (Figures 8C,D), the iaaMH from Paraburkholderia may have been horizontally acquired from a common phytopathogen ancestor. A common evolutionary origin of these genes in A. tumefaciens, A. rhizogenes, P. savastanoi, and P. agglomerans pv. gypsophilae has already been suggested, as well as their horizontal transfer between P. savastanoi pv. savastanoi NCPPB 3335, P. syringae pv. aceris M302273PT and P. syringae pv. syringae B728a (Morris, 1995; Patten et al., 2013; Aragón et al., 2014). Moreover, in the plant-associated bacterium P. agglomerans pv. gypsophilae, which causes opportunistic human infections, the iaaMH genes are located on a pathogenicity island with other virulence genes (Barash and Manulis-Sasson, 2009). Further analysis on whether the iaaMH operon is also associated with pathogenicity islands in other pathogenic species will provide valuable information on the evolution of these genes as virulence factors.
In summary, our data suggest that under the optimal control of NifA, IaaMH-driven auxin production might contribute to the successful establishment and progression of nodular primordia in response to cortical IT invasion. In absence of nifA, the unrestricted, exogenous bacterial auxin levels might intensify root hair infection and bypass the developmental check-points normally imposed by the host. This concept is supported by the extended symbiotic defects observed in plants inoculated with our iaaMH complementation strains. Circumventing the NifA fine tuning of bacterial auxin input in these strains presumably led to an improper homeostasis of the phytohormone and triggered root swellings, underdevelopment and poor nodulation frequency. Exploring the molecular mechanisms behind the mode of action of NifA as a negative regulator and the possible role of bacterial auxin as a symbiotic signal could grant deeper insights into the role of auxin biosynthesis in nitrogen-fixing and nodulating Paraburkholderia strains and will provide valuable information to improve competitiveness of rhizobia in the soil. We envision the potential pathogenic origin of the iaaMH genes as an evolutionary advantage trait used by root-colonizers to promote infection events in early symbiosis.
Data availability statement
The datasets presented in this study can be found in online repositories. The names of the repository/repositories and accession number(s) can be found in the article/Supplementary material.
Author contributions
PB-S, BH, CHA, AB, and GP contributed to conception and design the experiments. PB-S, YL, and AB performed the experiments. PB-S, BH, ES, CHA, LE, NZ, AB, and GP analyzed the data. PB-S, CHA, AB, and GP wrote the manuscript. All authors contributed to manuscript revision, read, and approved the submitted version.
Funding
This work was supported by the Swiss National Science Foundation (SNSF), project 31003A_179322 to GP.
Acknowledgments
We are grateful to Steven Winter, who helped to analyze the plant phenotype. We thank Karl Huwiler and Vicente Fco. Bellés-Sospedra for help in the green-house and for providing bean seeds, respectively. We acknowledge the two reviewers for their insightful comments.
Conflict of interest
The authors declare that the research was conducted in the absence of any commercial or financial relationships that could be construed as a potential conflict of interest.
Publisher’s note
All claims expressed in this article are solely those of the authors and do not necessarily represent those of their affiliated organizations, or those of the publisher, the editors and the reviewers. Any product that may be evaluated in this article, or claim that may be made by its manufacturer, is not guaranteed or endorsed by the publisher.
Supplementary material
The Supplementary Material for this article can be found online at: https://www.frontiersin.org/articles/10.3389/fpls.2022.991548/full#supplementary-material
Footnotes
References
Aragón, I. M., Pérez-Martínez, I., Moreno-Pérez, A., Cerezo, M., and Ramos, C. (2014). New insights into the role of indole-3-acetic acid in the virulence of Pseudomonas savastanoi pv. savastanoi. FEMS Microbiol. Lett. 356, 184–192. doi: 10.1111/1574-6968.12413
Barash, I., and Manulis-Sasson, S. (2009). Recent evolution of bacterial pathogens: The gall-forming Pantoea agglomerans case. Annu. Rev. Phytopathol. 47, 133–152. doi: 10.1146/annurev-phyto-080508-081803
Bellés-Sancho, P., Lardi, M., Liu, Y., Eberl, L., Zamboni, N., Bailly, A., et al. (2021a). Metabolomics and dual RNA-sequencing on root nodules revealed new cellular functions controlled by Paraburkholderia phymatum NifA. Metabolites 11:455. doi: 10.3390/metabo11070455
Bellés-Sancho, P., Lardi, M., Liu, Y., Hug, S., Pinto-Carbó, M. A., Zamboni, N., et al. (2021b). Paraburkholderia phymatum homocitrate synthase NifV plays a key role for nitrogenase activity during symbiosis with papilionoids and in free-living growth conditions. Cells 10:952. doi: 10.3390/cells10040952
Berrabah, F., Ratet, P., and Gourion, B. (2015). Multiple steps control immunity during the intracellular accommodation of rhizobia. J. Exp. Bot. 66, 1977–1985. doi: 10.1093/jxb/eru545
Breakspear, A., Liu, C., Roy, S., Stacey, N., Rogers, C., Trick, M., et al. (2014). The root hair “infectome” of Medicago truncatula uncovers changes in cell cycle genes and reveals a requirement for auxin signaling in rhizobial infection. Plant Cell 26, 4680–4701. doi: 10.1105/tpc.114.133496
Brown, D. E., Rashotte, A. M., Murphy, A. S., Normanly, J., Tague, B. W., Peer, W. A., et al. (2001). Flavonoids act as negative regulators of auxin transport in vivo in Arabidopsis. Plant Physiol. 126, 524–535. doi: 10.1104/pp.126.2.524
Camerini, S., Senatore, B., Lonardo, E., Imperlini, E., Bianco, C., Moschetti, G., et al. (2008). Introduction of a novel pathway for IAA biosynthesis to rhizobia alters vetch root nodule development. Arch. Microbiol. 190, 67–77. doi: 10.1007/s00203-008-0365-7
Caswell, T. A., Droettboom, M., Lee, A., Andrade, E. S., de, Hoffmann, T., Hunter, J., et al. (2021). Matplotlib/Matplotlib: REL: v3.5.1. Geneva: ZENODO. doi: 10.5281/ZENODO.5773480
Cock, P. J. A., Antao, T., Chang, J. T., Chapman, B. A., Cox, C. J., Dalke, A., et al. (2009). Biopython: Freely available Python tools for computational molecular biology and bioinformatics. Bioinformatics 25, 1422–1423. doi: 10.1093/bioinformatics/btp163
Cooper, J. E. (2007). Early interactions between legumes and rhizobia: Disclosing complexity in a molecular dialogue. J. Appl. Microbiol. 103, 1355–1365. doi: 10.1111/j.1365-2672.2007.03366.x
Djami-Tchatchou, A. T., Harrison, G. A., Harper, C. P., Wang, R., Prigge, M. J., Estelle, M., et al. (2020). Dual role of auxin in regulating plant defense and bacterial virulence gene expression during Pseudomonas syringae PtoDC3000 pathogenesis. Mol. Plant Microb. Interact. 33, 1059–1071. doi: 10.1094/MPMI-02-20-0047-R
Djami-Tchatchou, A. T., Li, Z. A., Stodghill, P., Filiatrault, M. J., and Kunkel, B. N. (2022). Identification of indole-3-acetic acid-regulated genes in Pseudomonas syringae pv. tomato strain DC3000. J. Bacteriol. 204:e0038021. doi: 10.1128/JB.00380-21
Duca, D. R., and Glick, B. R. (2020). Indole-3-acetic acid biosynthesis and its regulation in plant-associated bacteria. Appl. Microbiol. Biotechnol. 104, 8607–8619. doi: 10.1007/s00253-020-10869-5/Published
Duca, D., Lorv, J., Patten, C. L., Rose, D., and Glick, B. R. (2014). Indole-3-acetic acid in plant-microbe interactions. Antonie van Leeuwenhoek Int. J. Gen. Mol. Microbiol. 106, 85–125. doi: 10.1007/s10482-013-0095-y
Eberl, L., and Vandamme, P. (2016). Members of the genus Burkholderia: Good and bad guys. F1000Res 5:1007. doi: 10.12688/f1000research.8221.1
Elliott, G. N., Chen, W. M., Chou, J. H., Wang, H. C., Sheu, S. Y., Perin, L., et al. (2006). Burkholderia phymatum is a highly effective nitrogen-fixing symbiont of Mimosa spp. and fixes nitrogen ex planta. New Phytol. 173, 168–180. doi: 10.1111/j.1469-8137.2006.01894.x
Ferguson, B. J., Indrasumunar, A., Hayashi, S., Lin, M. H., Lin, Y. H., Reid, D. E., et al. (2010). Molecular analysis of legume nodule development and autoregulation. J. Integr. Plant Biol. 52, 61–76. doi: 10.1111/j.1744-7909.2010.00899.x
Fischer, H. M., Alvarez-Morales, A., and Hennecke, H. (1986). The pleiotropic nature of symbiotic regulatory mutants: Bradyrhizobium japonicum nifA gene is involved in control of nif gene expression and formation of determinate symbiosis. EMBO J. 5, 1165–1173. doi: 10.1002/j.1460-2075.1986.tb04342.x
Fuhrer, T., Heer, D., Begemann, B., and Zamboni, N. (2011). High-throughput, accurate mass metabolome profiling of cellular extracts by flow injection-time-of-flight mass spectrometry. Anal. Chem. 83, 7074–7080. doi: 10.1021/ac201267k
Gage, D. J. (2004). Infection and invasion of roots by symbiotic, nitrogen-fixing rhizobia during nodulation of temperate legumes. Microbiol. Mol. Biol. Rev. 68, 280–300. doi: 10.1128/mmbr.68.2.280-300.2004
Gage, D. J., and Margolin, W. (2000). Hanging by a thread: Invasion of legume pants by rhizobia. Curr Opin Microbiol. 3, 613–617. doi: 10.1016/S1369-5274(00)00149-1
Gilchrist, C. L. M., and Chooi, Y. H. (2021). Clinker & clustermap.js: Automatic generation of gene cluster comparison figures. Bioinformatics 37, 2473–2475. doi: 10.1093/bioinformatics/btab007
Gordon, S. A., and Weber, R. P. (1951). Colorimetric estimation of indole-3-acetic acid. Plant Physiol. 26, 192–195. doi: 10.1104/pp.26.1.192
Guerreiro, N., Redmond, J. W., Rolfe, B. G., and Djordjevic, M. A. (1997). New Rhizobium leguminosarum flavonoid-induced proteins revealed by proteome analysis of differentially displayed proteins. Mol. Plant Microb. Interact. 506, 506–516. doi: 10.1094/MPMI.1997.10.4.506
Gyaneshwar, P., Hirsch, A. M., Moulin, L., Chen, W. M., Elliott, G. N., Bontemps, C., et al. (2011). Legume-nodulating betaproteobacteria: Diversity, host range, and future prospects. Mol. Plant Microb. Interact. 24, 1276–1288. doi: 10.1094/MPMI-06-11-0172
Hahn, M., and Hennecke, H. (1984). Localized mutagenesis in Rhizobium japonicum. Mol. Gen. Genet. 193, 46–52.
Hauser, F., Pessi, G., Friberg, M., Weber, C., Rusca, N., Lindemann, A., et al. (2007). Dissection of the Bradyrhizobium japonicum NifA+σ54 regulon, and identification of a ferredoxin gene (fdxN) for symbiotic nitrogen fixation. Mol. Gen. Genom. 278, 255–271. doi: 10.1007/s00438-007-0246-9
Hug, S., Liu, Y., Heiniger, B., Bailly, A., Ahrens, C. H., Eberl, L., et al. (2021). Differential expression of Paraburkholderia phymatum Type VI Secretion Systems (T6SS) suggests a role of T6SS-b in early symbiotic interaction. Front. Plant Sci. 12:699590. doi: 10.3389/fpls.2021.699590
Kanehisa, M., and Sato, Y. (2020). KEGG Mapper for inferring cellular functions from protein sequences. Prot. Sci. 29, 28–35. doi: 10.1002/pro.3711
Klonowska, A., Melkonian, R., Miché, L., Tisseyre, P., and Moulin, L. (2018). Transcriptomic profiling of Burkholderia phymatum STM815, Cupriavidus taiwanensis LMG19424 and Rhizobium mesoamericanum STM3625 in response to Mimosa pudica root exudates illuminates the molecular basis of their nodulation comp. BMC Genom. 19:105. doi: 10.1186/s12864-018-4487-2
Kohlen, W., Ng, J. L. P., Deinum, E. E., and Mathesius, U. (2018). Auxin transport, metabolism, and signalling during nodule initiation: Indeterminate and determinate nodules. J. Exp. Bot. 69, 229–244. doi: 10.1093/jxb/erx308
Lardi, M., de Campos, S. B., Purtschert, G., Eberl, L., and Pessi, G. (2017a). Competition experiments for legume infection identify Burkholderia phymatum as a highly competitive β-rhizobium. Front. Microbiol. 8:1527. doi: 10.3389/fmicb.2017.01527
Lardi, M., Liu, Y., Giudice, G., Ahrens, C. H., Zamboni, N., and Pessi, G. (2018). Metabolomics and transcriptomics identify multiple downstream targets of Paraburkholderia phymatum σ54 during symbiosis with Phaseolus vulgaris. Int. J. Mol. Sci. 19:1049. doi: 10.3390/ijms19041049
Lardi, M., Liu, Y., Purtschert, G., de Campos, S. B., and Pessi, G. (2017b). Transcriptome analysis of Paraburkholderia phymatum under nitrogen starvation and during symbiosis with Phaseolus vulgaris. Genes (Basel) 8:389. doi: 10.3390/genes8120389
Lardi, M., Murset, V., Fischer, H. M., Mesa, S., Ahrens, C. H., Zamboni, N., et al. (2016). Metabolomic profiling of Bradyrhizobium diazoefficiens -induced root nodules reveals both host plant-specific and developmental signatures. Int. J. Mol. Sci. 17, 1–19. doi: 10.3390/ijms17060815
Lee, A., and Hirsch, A. M. (2006). Signals and responses: Choreographing the complex interaction between legumes and α- and β-rhizobia. Plant Signal Behav. 1, 161–168. doi: 10.4161/psb.1.4.3143
Lemaire, B., Chimphango, S. B. M., Stirton, C., Rafudeen, S., Honnay, O., Smets, E., et al. (2016). Biogeographical patterns of legume-nodulating Burkholderia spp.: From African fynbos to continental scales. Appl. Environ. Microbiol. 82, 5099–5115. doi: 10.1128/AEM.00591-16.Editor
Liu, H., Zhang, C., Yang, J., Yu, N., and Wang, E. (2018). Hormon meodulation of legume-rhizobial symbiosis. J. Integr. Plant Biol. 60, 632–648. doi: 10.1111/jipb.12653
Liu, Y., Bellich, B., Hug, S., Eberl, L., Cescutti, P., and Pessi, G. (2020). The exopolysaccharide cepacian plays a role in the establishment of the Paraburkholderia phymatum – Phaseolus vulgaris symbiosis. Front. Microbiol. 11:1600. doi: 10.3389/fmicb.2020.01600
Majda, M., and Robert, S. (2018). The role of auxin in cell wall expansion. Int. J. Mol. Sci. 19:951. doi: 10.3390/ijms19040951
Masson-Boivin, C., Giraud, E., Perret, X., and Batut, J. (2009). Establishing nitrogen-fixing symbiosis with legumes: How many rhizobium recipes? Trends Microbiol. 17, 458–466. doi: 10.1016/j.tim.2009.07.004
Mathesius, U. (2008). Auxin: At the root of nodule development? Funct. Plant Biol. 35, 651–668. doi: 10.1071/FP08177
Mathesius, U., Schlaman, H. R. M., Spaink, H. P., Sautter, C., Rolfe, B. G., and Djordjevic, M. A. (1998). Auxin transport inhibition precedes root nodule formation in white clover roots and is regulated by flavonoids and derivatives of chitin oligosaccharides. Plant J. 14, 23–34. doi: 10.1046/j.1365-313X.1998.00090.x
Miller, J. H. (1972). Experiments in molecular genetics. Cold Spring Harbor, NY: Cold Spring Harbor Lab Press, 352–355.
Mishra, R. P. N., Tisseyre, P., Melkonian, R., Chaintreuil, C., Miché, L., Klonowska, A., et al. (2012). Genetic diversity of Mimosa pudica rhizobial symbionts in soils of French Guiana: Investigating the origin and diversity of Burkholderia phymatum and other beta-rhizobia. FEMS Microbiol. Ecol. 79, 487–503. doi: 10.1111/j.1574-6941.2011.01235.x
Morffy, N., and Strader, L. C. (2020). Old Town Roads: Routes of auxin biosynthesis across kingdoms. Curr. Opin. Plant Biol. 55, 21–27. doi: 10.1016/j.pbi.2020.02.002
Morris, R. O. (1995). “Genes specifying auxin and cytokinin biosynthesis in prokaryotes,” in Plant Hormones: Physiology, biochemistry and molecular biology, ed. P. J. Davies (Dordrecht: Springer Netherlands), 318–339. doi: 10.1007/978-94-011-0473-9_15
Moulin, L., Klonowska, A., Caroline, B., Booth, K., Vriezen, J. A. C., Melkonian, R., et al. (2014). Complete Genome sequence of Burkholderia phymatum STM815T, a broad host range and efficient nitrogen-fixing symbiont of Mimosa species. Stand Genom. Sci. 9, 763–774. doi: 10.4056/sigs.4861021
Moulin, L., Munive, A., Dreyfus, B., and Boivin-Masson, C. (2001). Nodulation of legumes by members of the beta-subclass of Proteobacteria. Nature 411, 948–950. doi: 10.1038/35082070
Murset, V., Hennecke, H., and Pessi, G. (2012). Disparate role of rhizobial ACC deaminase in root-nodule symbioses. Symbiosis 57, 43–50. doi: 10.1007/s13199-012-0177-z
Nadzieja, M., Kelly, S., Stougaard, J., and Reid, D. (2018). Epidermal auxin biosynthesis facilitates rhizobial infection in Lotus japonicus. Plant J. 95, 101–111. doi: 10.1111/tpj.13934
Nett, R. S., Bender, K. S., and Peters, R. J. (2022). Production of the plant hormone gibberellin by rhizobia increases host legume nodule size. ISME J. 16, 1809–1817. doi: 10.1038/s41396-022-01236-5
Nukui, N., Minamisawa, K., Ayabe, S. I., and Aoki, T. (2006). Expression of the 1-aminocyclopropane-1-carboxylic acid deaminase gene requires symbiotic nitrogen-fixing regulator gene nifA2 in Mesorhizobium loti MAFF303099. Appl. Environ. Microbiol. 72, 4964–4969. doi: 10.1128/AEM.02745-05
Oldroyd, G. E. D., Murray, J. D., Poole, P. S., and Downie, J. A. (2011). The rules of engagement in the legume-rhizobial symbiosis. Annu. Rev. Genet. 45, 119–144. doi: 10.1146/annurev-genet-110410-132549
Parniske, M., Fischer, H.-M., Hennecke, H., and Werner, D. (1991). Accumulation of the phytoalexin glyceollin I in soybean nodules infected by a Bradyrhizobium japonicum nifA mutant. Zeitschrift für Naturforschung 46, 318–320. doi: 10.1515/znc-1991-3-424
Patten, C. L., Blakney, A. J. C., and Coulson, T. J. D. (2013). Activity, distribution and function of indole-3-acetic acid biosynthetic pathways in bacteria. Crit. Rev. Microbiol. 39, 395–415. doi: 10.3109/1040841X.2012.716819
Peer, W. A., and Murphy, A. S. (2007). Flavonoids and auxin transport: Modulators or regulators? Trends Plant Sci. 12, 556–563. doi: 10.1016/j.tplants.2007.10.003
Persello-Cartieaux, F., Nussaume, L., and Robaglia, C. (2003). Tales from the underground: Molecular plant-rhizobacteria interactions. Plant Cell Environ. 26, 189–199.
Pii, Y., Crimi, M., Cremonese, G., Spena, A., and Pandolfini, T. (2007). Auxin and nitric oxide control indeterminate nodule formation. BMC Plant Biol. 7:21. doi: 10.1186/1471-2229-7-21
Poole, P., and Allaway, D. (2000). Carbon and nitrogen metabolism in Rhizobium. Adv. Microb. Physiol. 43, 117–163. doi: 10.1016/s0065-2911(00)43004-3
Poole, P., Ramachandran, V., and Terpolilli, J. (2018). Rhizobia: From saprophytes to endosymbionts. Nat Rev. Microbiol. 16, 291–303. doi: 10.1038/nrmicro.2017.171
Remans, R., Beebe, S., Blair, M., Manrique, G., Tovar, E., Rao, I., et al. (2008). Physiological and genetic analysis of root responsiveness to auxin-producing plant growth-promoting bacteria in common bean (Phaseolus vulgaris L.). Plant Soil 302, 149–161. doi: 10.1007/s11104-007-9462-7
Roy, S., Robson, F., Lilley, J., Liu, C. W., Cheng, X., Wen, J., et al. (2017). MtLAX2, a functional homologue of the Arabidopsis auxin influx transporter AUX1, is required for nodule organogenesis. Plant Physiol. 174, 326–338. doi: 10.1104/pp.16.01473
Ryu, R. J., and Patten, C. L. (2008). Aromatic amino acid-dependent expression of indole-3-pyruvate decarboxylase is regulated by TyrR in Enterobacter cloacae UW5. J. Bacteriol. 190, 7200–7208. doi: 10.1128/JB.00804-08
Schiff, S., Tani, C., Cimmino, A., Mandalà, G., Cinelli, T., Evidente, A., et al. (2019). The colonization processes of Myrtus communis by strains of Pseudomonas savastanoi with a differential ability to produce phytohormones. Plant Pathol. 68, 1109–1119. doi: 10.1111/ppa.13021
Schnabel, E., Journet, E. P., de Carvalho-Niebel, F., Duc, G., and Frugoli, J. (2005). The Medicago truncatula SUNN gene encodes a CLV1-like leucine-rich repeat receptor kinase that regulates nodule number and root length. Plant Mol. Biol. 58, 809–822. doi: 10.1007/s11103-005-8102-y
Sexton, W. K., Fidero, M., Spain, J. C., Jiang, L., Bucalo, K., Cruse-Sanders, J. M., et al. (2020). Characterization of endophytic bacterial communities within greenhouse and field-grown rhizomes of three rare pitcher plant species (Sarracenia oreophila. S. leucophylla, and S. purpurea spp. venosa) with an emphasis on nitrogen-fixing bacteria. Plant Soil 447, 257–279. doi: 10.1007/s11104-019-04372-8
Shastri, S., Spiewak, H. L., Sofoluwe, A., Eidsvaag, V. A., Asghar, A. H., Pereira, T., et al. (2017). An efficient system for the generation of marked genetic mutants in members of the genus Burkholderia. Plasmid 89, 49–56. doi: 10.1016/j.plasmid.2016.11.002
Shrestha, A., Zhong, S., Therrien, J., Huebert, T., Sato, S., Mun, T., et al. (2020). Lotus japonicus Nuclear Factor YA1, a nodule emergence stage-specific regulator of auxin signalling. New Phytolog. 229, 1535–1552. doi: 10.1111/nph.16950
Sievers, F., Wilm, A., Dineen, D., Gibson, T. J., Karplus, K., Li, W., et al. (2011). Fast, scalable generation of high-quality protein multiple sequence alignments using Clustal Omega. Mol. Syst. Biol. 7:539. doi: 10.1038/msb.2011.75
Spaepen, S., and Vanderleyden, J. (2011). Auxin and plant-microbe interactions. Cold Spring Harb. Perspect. Biol. 3, 1–13. doi: 10.1101/cshperspect.a001438
Spaepen, S., Vanderleyden, J., and Remans, R. (2007). Indole-3-acetic acid in microbial and microorganism-plant signaling. FEMS Microbiol. Rev. 31, 425–448. doi: 10.1111/j.1574-6976.2007.00072.x
Stamatakis, A. (2014). RAxML Version 8: A tool for phylogenetic analysis and post-analysis of large phylogenies. Bioinformatics 30, 1312–1313. doi: 10.1093/bioinformatics/btu033
Talbi, C., Delgado, M. J., Girard, L., Ramírez-Trujillo, A., Caballero-Mellado, J., and Bedmar, E. J. (2010). Burkholderia phymatum strains capable of nodulating Phaseolus vulgaris are present in moroccan soils. Appl. Environ. Microbiol. 76, 4587–4591. doi: 10.1128/AEM.02886-09
Theunis, M., Kobayashi, H., Broughton, W. J., and Prinsen, E. (2004). Flavonoids, NodD1, NodD2, and Nod-Box NB15 modulate expression of the y4wEFG locus that is required for indole-3-acetic acid synthesis in Rhizobium sp. strain NGR234. Minamisawa Fukai 17, 1153–1161. doi: 10.1094/MPMI.2004.17.10.1153
Thorvaldsdóttir, H., Robinson, J. T., and Mesirov, J. P. (2013). Integrative genomics viewer (IGV): High-performance genomics data visualization and exploration. Brief. Bioinform. 14, 178–192. doi: 10.1093/bib/bbs017
Torres, D., Mongiardini, E., Donadío, F., Donoso, R., Recabarren-Gajardo, G., Gualpa, J., et al. (2021). Molecular and physiological analysis of indole-3-acetic acid degradation in Bradyrhizobium japonicum E109. Res. Microbiol. 172:103814. doi: 10.1016/j.resmic.2021.103814
van Noorden, G. E., Kerim, T., Goffard, N., Wiblin, R., Pellerone, F. I., Rolfe, B. G., et al. (2007). Overlap of proteome changes in Medicago truncatula in response to auxin and Sinorhizobium meliloti. Plant Physiol. 144, 1115–1131. doi: 10.1104/pp.107.099978
van Noorden, G. E., Ross, J. J., Reid, J. B., Rolfe, B. G., and Mathesius, U. (2006). Defective long-distance auxin transport regulation in the Medicago truncatula super numeric nodules mutant. Plant Physiol. 140, 1494–1506. doi: 10.1104/pp.105.075879
Velandia, K., Reid, J. B., and Foo, E. (2022). Right time, right place: The dynamic role of hormones in rhizobial infection and nodulation of legumes. Plant Commun. 18:100327. doi: 10.1016/j.xplc.2022.100327
Via, V. D., Zanetti, M. E., and Blanco, F. (2016). How legumes recognize rhizobia. Plant Signal Behav. 11, 1–6. doi: 10.1080/15592324.2015.1120396
Vives-Peris, V., de Ollas, C., Gómez-Cadenas, A., and Pérez-Clemente, R. M. (2020). Root exudates: From plant to rhizosphere and beyond. Plant Cell Rep. 39, 3–17. doi: 10.1007/s00299-019-02447-5
Wasson, A. P., Pellerone, F. I., and Mathesius, U. (2006). Silencing the flavonoid pathway in Medicago truncatula inhibits root nodule formation and prevents auxin transport regulation by rhizobia. Plant Cell 18, 1617–1629. doi: 10.1105/tpc.105.038232
Wongdee, J., Boonkerd, N., Teaumroong, N., Tittabutr, P., and Giraud, E. (2018). Regulation of nitrogen fixation in Bradyrhizobium sp. strain DOA9 involves two distinct NifA regulatory proteins that are functionally redundant during symbiosis but not during free-living growth. Front. Microbiol. 9:1644. doi: 10.3389/fmicb.2018.01644
Zhang, P., Jin, T., Sahu, S. K., Xu, J., Shi, Q., Liu, H., et al. (2019). The distribution of tryptophan-dependent indole-3-acetic acid synthesis pathways in bacteria unraveled by large-scale genomic analysis. Molecules 24:1411. doi: 10.3390/molecules24071411
Zhao, Y. (2010). Auxin biosynthesis and its role in plant development. Annu. Rev. Plant Biol. 61, 49–64. doi: 10.1146/annurev-arplant-042809-112308
Keywords: root-nodule, legume, indole-acetamide, nitrogenase, infection
Citation: Bellés-Sancho P, Liu Y, Heiniger B, von Salis E, Eberl L, Ahrens CH, Zamboni N, Bailly A and Pessi G (2022) A novel function of the key nitrogen-fixation activator NifA in beta-rhizobia: Repression of bacterial auxin synthesis during symbiosis. Front. Plant Sci. 13:991548. doi: 10.3389/fpls.2022.991548
Received: 11 July 2022; Accepted: 31 August 2022;
Published: 28 September 2022.
Edited by:
Davide Neri, Marche Polytechnic University, ItalyReviewed by:
Catalina Iulia Pislariu, Texas Woman’s University, United StatesOswaldo Valdes-Lopez, National Autonomous University of Mexico, Mexico
Copyright © 2022 Bellés-Sancho, Liu, Heiniger, von Salis, Eberl, Ahrens, Zamboni, Bailly and Pessi. This is an open-access article distributed under the terms of the Creative Commons Attribution License (CC BY). The use, distribution or reproduction in other forums is permitted, provided the original author(s) and the copyright owner(s) are credited and that the original publication in this journal is cited, in accordance with accepted academic practice. No use, distribution or reproduction is permitted which does not comply with these terms.
*Correspondence: Aurélien Bailly, YXVyZWxpZW4uYmFpbGx5QGJvdGluc3QudXpoLmNo; Gabriella Pessi, Z2FicmllbGxhLnBlc3NpQGJvdGluc3QudXpoLmNo