- 1Department of Biology, Plant Cell Biology, University of Marburg, Marburg, Germany
- 2Institute for Biosciences, Physiology of Plant Metabolism, University of Rostock, Rostock, Germany
- 3Institute for Biosciences, Ecology, University of Rostock, Rostock, Germany
- 4BIOSS Centre for Biological Signalling Studies, University of Freiburg, Freiburg, Germany
For studying land plant evolution, the establishment and optimization of model organisms representing streptophytic algae, sister to land plants, is essential. Long-term cultivation experiments with Chara braunii S276 were performed over 8 years, since 4 years (Nov. 2018) under constant conditions. Additionally, short-term experiments for optimization of culture conditions were performed with three strains of C. braunii (S276, NIES-1604 and Lausiger Teiche, LaT-2708). Germination success after application of sterilization agents, addition of gibberellic acid and under different incubation conditions with respect to pre-treatment, irradiance regime and substrate was investigated in order to develop protocols for generative cultivation of at least unialgal cultures. The resulting cultivation protocols for C. braunii S276, allowing maintenance of vegetative as well as generative cultures are presented in detail, including protocols for germination induction and growth of sterilized and unsterilized oospores.
1 Introduction
The transition from water to land by plants that occurred at least 500 Ma ago and the associated molecular, cellular and physiological adaptations led to an enormous extant plant diversity (Gerrienne et al., 2016; De Vries and Archibald, 2018; De Vries and De Vries, 2018). Numerous genomes and transcriptomes of plants have recently become available (One Thousand Plant Transcriptomes, 2019; Provart et al., 2021; Miryeganeh et al., 2022), allowing studies of evolutionary patterns and traits across plants. The Phragmoplastophyta comprise the Coleochaetophyceae, Charophyceae, Zygnematophyceae and Embryophyta (land plants). Since various studies suggest that Zygnematophyceae are the closest relatives of land plants (Wickett et al., 2014; Cheng et al., 2019), different genomes and transcriptomes became available, e.g. of the unicellular desmid Penium margaritaceum (Jiao et al., 2020), or two species of the subclass Spirogloeophycidae, Spirogloea muscicola and Mesotaenium endlicherianum (Cheng et al., 2019). The number is expected to increase in future. Within the morphologically complex Characeae, the genome of Chara braunii S276 (Nishiyama et al., 2018) and organellar genomes of Nitellopsis obtusa (Sleith and Karol, 2021) brought Charophyceae research into the genomics era of algae.
Whilst model organisms are available for various classes of bryophytes (Rensing et al., 2008; Szövényi et al., 2015; Shimamura, 2016; Rensing et al., 2020), ferns (De Vries and De Vries, 2018) or brown algae (Coelho et al., 2012), a model system per se does not exist within streptophytic algae (Hedges, 2002). But, different species have been utilized as model system for specific purposes, such as electrophysiology or developmental studies (Beilby, 2019; Zhou and von Schwartzenberg, 2020). Additionally, most algal culture collections are lacking Charophyceae and there is no axenic strain available at all. The recently sequenced genome of the C. braunii strain S276 does enable functional analyses of, e.g., hormone signaling pathways, such as for auxin (Vosolsobĕ et al., 2020; Schmidt, 2021), strigolactones (Delaux et al., 2012), gibberellins (Godlewski and Kwiatkowska, 1980; Kwiatkowska and Godlewski, 1980) or genes involved in stress response (Wang et al., 2021). However, the use of a Chara strain, that is adopted to constant environmental and sediment conditions, could allow the comparability of e.g., transcriptomic analyses across laboratory boundaries.
Chara braunii Gmelin, 1826 (sect. Charopsis), named in memory of A. Braun (Gmelin, 1826), is a cosmopolitan species, being red listed in some regions while dominating in others (Zhákova, 2003; Helcom, 2013; Schubert et al., 2015). This species is mainly distributed in shallow temporary wetlands and other aquatic systems such as lakes, fish ponds, pools or flooded field areas (Raabe, 2017). As species with a short annual life cycle compared to other Charophyceae species, C. braunii produces a high amount of oospores within few months. Reproduction could occur generatively by means of oospores, but can also sustain by fragmentation of thalli (Casanova, 2014). For the northern hemisphere, germination windows are in late spring and early autumn from August to September (Franke and Doege, 2016; Raabe, 2017). The high morphological variability within Chara braunii resulted in unambiguous opinions in regard to the taxonomic status and led to the description of various varieties and forms (Wood, 1965). Results of morphology and phylogenetic analyses of C. braunii reveal the existence of genetic differences in rbcL genes between the Hawaiian NIES-1604 and different Japanese C. braunii specimens that are separated in two cluster (Kato et al., 2008). In contrast to this, studies of Polish specimens, Japanese specimens and eight clones from worldwide herbaria material have shown relatively small size ranges for length or width (Proctor, 1970; Boszke et al., 2008; Kato et al., 2008).
Culture protocols for Charophyceae, especially for short-term cultivation, have been published and tested a variety of media compositions, characterized by different combinations of substrates and media ranging from aerated or synthetic seawater (Forsberg, 1965b; Wüstenberg et al., 2011), tap water (Ernst, 1917), media made from clay extracts (Imahori and Iwasa, 1965; Proctor, 1967), to completely synthetic media (Anderson, 1958; Wetzel and McGregor, 1968; Andrews et al., 1984). Sediments were taken from lakes/rivers (Smart and Barko, 1984), or as combinations with composts (Okazaki et al., 1984b), boiled peat with boiled sea sand (Kuczewski, 1906) over soil extract and rotten leaves (Proctor, 1967) to combinations of leaf mould with black soil/river sand and lime (Sakayama et al., 2004; Sato et al., 2014). Some of them even claiming axenic growth and germination (Forsberg, 1965a; Forsberg, 1965b; Forsberg, 1965c; Chowdary, 2014) they have been shown to be inapplicable for the establishment of long-term cultures due to extensive growth of epiphytes or decreasing fertility and growth rate over time. Moreover, most of the existing culture protocols for Chara braunii rely on organic-rich substrates as leaf mud, natural river sediments or layers of black sand or fertilizer (Sakayama et al., 2004; Zhao et al., 2012; Sato et al., 2014), even provoking overgrowth by epiphytes during long-term cultivation.
Light regimes and underwater spectral distribution, have been identified as one of the main factors for the regulation of growth and developmental processes such as seed/oospore germination (Sokol and Stross, 1992; Stross et al., 1995; Rensing et al., 2016; Küster et al., 2004; Pinnel et al., 2005). The adaptation to light regimes is regulated by photoreceptors, including the three classes, phytochroms, cryptochroms/phototropins and UV-B receptors. Phytochroms, classified in light- labile and light-stabile ones, are one of the major photoreceptors families and regulate on biochemical and physiological level processes such as germination, growth and photosynthesis (Inoue et al., 2016; Léger-Daigle et al., 2022). Structural, phytochroms exhibit both termini, one of cyanobacterial and one of proteobacterial origin (Buchberger and Lamparter, 2015). Evolutionary, the phytochrome signaling pathway is originated in chlorophytes with Chlamydomonas reinhardtii as earliest diverging organism having UVR8 orthologs (Han et al., 2019). It is assumed that this evolutionary hallmark evolved by the transition from deep seas into shallow water areas. Within Charophyceae, the existence of phytochrome- mediated systems was long-time hypothesized (Sokol and Stross, 1992). Sequence data of three chlorophytes, one moss, one lycophyte and six flowering plants have verified the presence of 11 orthologs of light signaling genes (Han et al., 2017) although their functional implication and interaction are currently unknown (Han et al., 2019).
This study aims to identify optimal culture conditions for C. braunii regarding light regime and substrate. Both, long-term cultivation and short-term experiments varying the factors substrates (compost, sediment, quartz or sea-sand), media and irradiance regimes were carried out and combined with nutritional substrate analyses. Oospores of used strains were analyzed for size and morphological differences. Additionally, germination experiments of C. braunii oospores under different light regimes were performed.
2 Materials and methods
2. 1 Strains
For cultivation and germination, three different C. braunii strains were used: C. braunii S276, NIES-1604, and C. braunii Lausiger Teiche, LaT-2708 (named after the date of sampling, 27.08.2019). In addition to this, oospores of C. braunii from Ranstadt (Hesse, Germany) were included in oospore analysis.
The non-axenic Japanese freshwater strain S276 (KU-2549; KU-MACC) (Sato et al., 2014; Kawai et al., 2020) originates from Lake Kasumigaura (Ibaraki, Japan). The algae used here, were obtained from KU-MACC and cultivated over a period of 8 years at the Universities of Freiburg and Marburg. The Hawaiian freshwater strain NIES-1604 is closely related to S276, isolated by M. Ishimoto in 1998, and kept at the Microbial Culture Collection at the National Institute for Environmental Studies (NIES). On-site cultivation conditions for NIES-1604 comprise mSWC-2 or SWCN-2 media at 20°C, and 16-20 µmol photons/(s*m2) in a light:dark cycle of 10L:14D (Okazaki et al., 1984a; Kato et al., 2008; Sato et al., 2014). Few specimens of C. braunii LaT-2708 from Lausiger Teiche (Bad Schmiedeberg, Saxony-Anhalt, Germany) were sampled in 2019 by H. Schubert. The occurrence was first recorded during monitoring by H. Korsch in 2010 (Korsch, 2010). Specimens of C. braunii from Ranstadt (Hesse, Germany) were collected by U. Raabe in August 2016. Oospores were cultivated for post-maturation on window sills in west direction.
2.2 Long-term cultivation of strains
Figure 1 schematically illustrates the cultivation of S276 and NIES-1604 at the University of Marburg. The strains S276 and NIES-1604 were cultivated under relative constant day:night conditions of 22°C:16°C in a light:dark cycle of 16L:8D. Fluorescent lamps (cLED white bi‐phosphor 4000K moisture‐protected LEDs, CLF PlantClimatics GmbH, Germany) provided constant light intensities at a range between 25 and 38µmol photons/(s*m2) above the vessels and between 20 and 30 µmol photons/(s*m2) below the lids. Manufacturer’s spectral composition of White Light (WL) is given in Figure S4.1. These conditions applied, unless explicitly modified for individual experiments. After substrate optimization, culture vessels of different sizes containing double autoclaved layers of compost and quartz sand (0.4-0.8mm in diameter) in a ratio of 10mL compost:100mL quartz per 1L. Compost and quartz sand volumes were calibrated using 15 or 50mL Falcon tubes. In some cases, 0.1g lime per 100mL of quartz were layered between the compost and quartz sand.
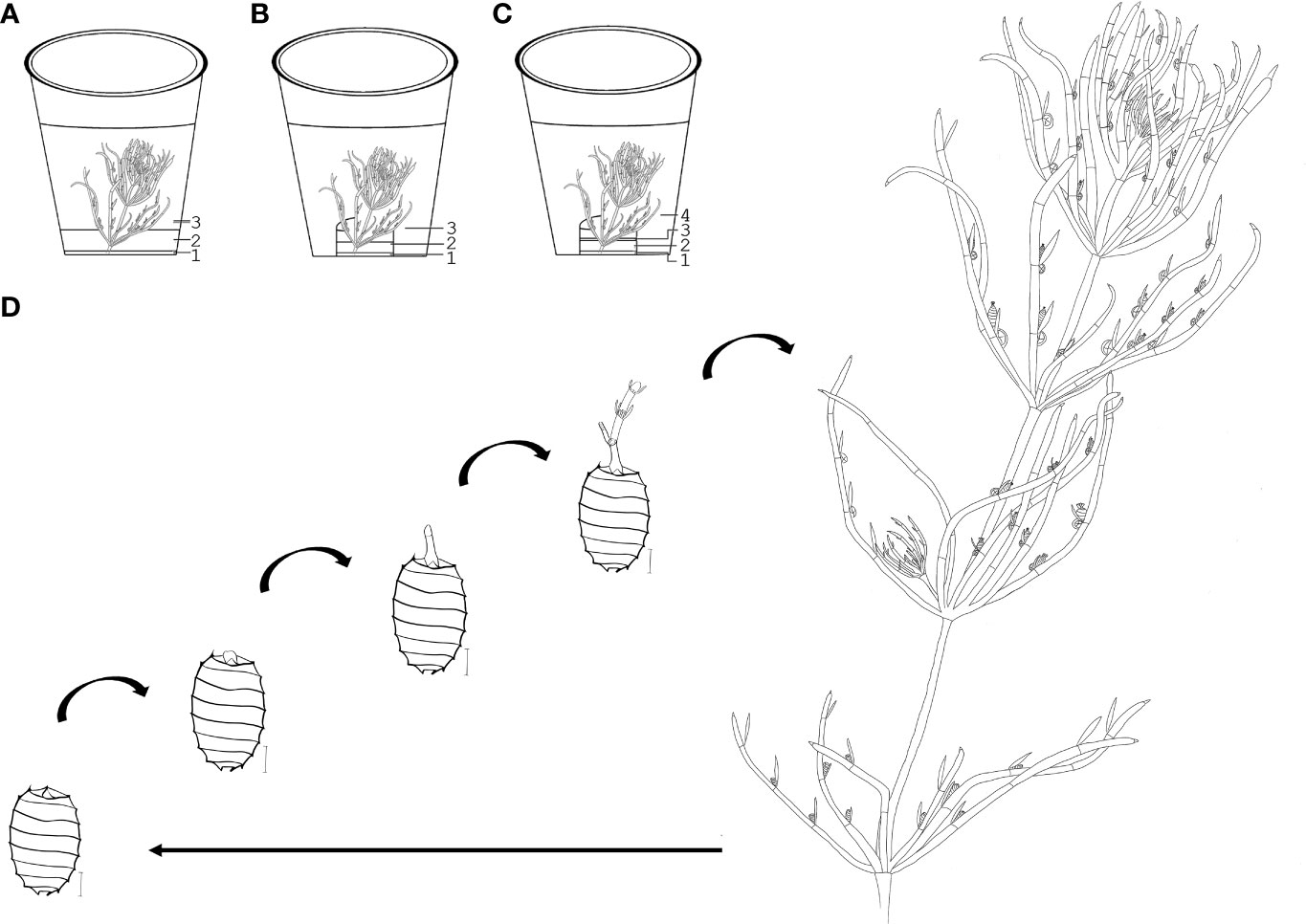
Figure 1 Cultivation methods and morphological units of C. braunii. (A) direct layering of substrate and quartz sand in cultivation vessels (B) layering of substrate and quartz sand in separate autoclaved glass bowl (C) layering of substrate, quartz sand and agar in a separate autoclaved glass bowl. (D) upper part of a (C) braunii thallus (W – whorl, int – internode). 1 – substrate, 2 – quartz sand, 3 – distilled H2O or Wüstenberg media, 4 – agar.
Cultivation of C. braunii LaT-2708 in modified Wüstenberg medium using sea sand of 0.06 to 0.3mm grain size diameter was conducted first on a windowsill in south direction from August 2019 to February 2020 (August to September, windows were concealed with a white curtain in accordance to Kuczewski, 1906). After the initial experimental period, the cultures were relocated for one month to exclude direct light penetration (laboratory bench), followed by cultivation in east direction from March to May 2020. Temperature and light irradiances were documented using datalogger (MX2202 HOBO Pendant® MX Temperature & Light Data Logger, see Figure S4.5).
2.3 Short-term experiments
For all short-term experiments, the method of thallus transfer was used. Meaning, the upper thallus part of minimum 2-3 cm was cut off using a sterile tweezers, and transferred into new culture vessels of different sizes (290ml, 340ml, 600ml, 850ml, 1l). For media experiments with S276, mSWC-2 medium (Sakayama et al., 2004) and modified Wüstenberg medium with Ca3(PO4)2 and CoCl2 were used. For LaT-2708, Wüstenberg medium with Ca3(PO4)2 was used (Wüstenberg et al., 2011).
Algae were analysed by morphological and developmental parameters such as lengths of thalli, internodes, stipulodes and branchlets plus the presence of contaminations, oogonia, antheridia and oospores. Parameters were tested for normal distribution by Shapiro-Wilk tests using GraphPad Prism 9.3.1 (Graph Pad Software, San Diego, USA). Differences between strains, irradiances, substrates, media and combinations over time were tested using Two-way ANOVA and Tukey’s multiple comparison tests. Significance p-levels were 0.0332 (*), 0.0021 (**), 0.0002 (***) and <0.0001 (****).
2.3.1 Substrate
Algae of the strain S276 were incubated under long day conditions (22°C, 16L:8D, WL) for four weeks using two different composts with five replicates each (n=5 vessels): (1) compost from the Botanical Garden of the University of Marburg (BGUM) and (2) a commercial, certified compost (Gardol®, GAR). The same parameters were analyzed as described above. Additionally, nutritional composition of two batches BGUM (2018, 2021) and the commercial compost (2020) as well as the fluid medium were analyzed by the ''Landesbetrieb Hessisches Landeslabor''. Macronutrient analyses were performed using calcium-acetate-lactate extraction, trace elements and heavy metals via calcium chloride extraction, cold vapour atomic absorption spectrometry (CV-AAS) and inductively-coupled-plasma mass spectrometry (ICP-MS) and optical emission spectrometry (ICP-EOS). Total nitrogen content, organic carbon and humus were determined via dry incineration.
2.3.2 Irradiance
Algae of the strains S276 and NIES-1604 were cultured for four weeks under two different illuminations on BGUM18 substrate in mSWC-2 medium. Three replicates (n=3) of S276 and NIES-1604 were cultivated under WL in a rhythm of 16L:8D and intensities of 70 µm photons/(s*m2) (HI), whereas six replicates were incubated under low light intensities of 25-30 µm photons/(s*m2) (LI). During this time, samples were frequently evaluated for the increase in biomass, the development of gametangia and contamination. Samples were photographed weekly and measured using Image J.
Short-term cultivation experiments with LaT-2708, started in December 2019. Thalli originating from the long-term cultivation and represent descendants of the south directed culture. Seven replicates (n=7/light condition) each of C. braunii LaT-2708 were incubated for six weeks under four different light conditions (measured below the lid): (1) 10-20 µmol photons/(s*m2), (2) 60-70 µmol photons/(s*m2) (3) 90-100 µmol photons/(s*m2), whilst group (4) was cultivated on the windowsill. Incubation temperature was constant at 20°C for (1) – (3). Temperature and light data for the windowsill culture are given in Figure S4.5.
2.4 Germination experiments
Oospores were sterilized after modified protocols based on Sakayama et al. (2004) and Sato et al. (2014). All used substances and manufacturer’s information are listed in Table S3. After sterilization, oospores were plated on LB-, KNOP- and Saboraud-agar plates and incubated at 23°C for testing the sterility success. LB- and KNOP-agar plates were prepared according to Meyberg and Schwartzenberg (2020), Saboraud agar plates consist of 40g/L dextrose, 10g/L peptone and 20g/L agar.
All experiments that were carried out with oospores from C. braunii S276, NIES-1604 and LaT-2708 are listed in Table 3. Additional information to individual experiments is listed below, manufacturer`s information are given in Table S3. Spectral distributions of far-red and white light are given in Figure S4.1 – S4.4.
2.4.1 Sterilization protocol
2.4.1.1 Pre-preparations
● double autoclaving of substrate (compost, lake sediment) with an intervening rest period of at least 24 hours and subsequent chilling period of media before use
● double autoclaving of deionized water and germination media modified after Wüstenberg et al., 2011 by using Ca3(PO4)2 and CoCl2 for S276 and Ca3(PO4)2 for LaT-2708.
● autoclaved agarose solution (1%, Roth®)
● preparing of fresh sodium hypochlorite solution (3%)
2.4.1.2 Oospore treatment and sterilisation
● separation of 15 oospores per 2mL reaction tube in 1mL deionized water
● discarding of supernatant, adding 1mL sodium hypochlorite solution and incubation while shaking for 5minutes (VWR® Tube Rotator and Rotisseries)
● washing 8 times with deionized water for at least 5 minutes (shaking)
● storing in 1 mL deionized water until further use
● control of sterilization by incubation on agar plates
2.4.1.3 Preparation of germination plates
● layering of substrate, oospores and cooled (just before solidification) agarose solution in sterile microtiter plates or glass vessels (we used 50 mL – 290 mL for germination experiments)
● adding germination medium (1 mL for 24 well plates, 3 mL for 6 well plates)
● closing with micropore hypoallergenic papertape (3M)
2.4.1.4 Incubation
● 22°C, 16h light:8h dark, WL < 30 µmol photons/(s*m2) until oospores germinate (refilling of evaporated media)
2.4.1.5 After germination
Germlings were transferred after seven to 25 weeks to either mSWC-2 or nutrient agar plates (1% Bold agar with or without compost extract (Nichols and Bold, 1965), for a gradual acclimation incubation from < 30 µmol photons/(s*m2) to 50 µmol photons/(s*m2) (22°C, 16L:8D, WL) and subsequent transfer of thalli mSWC-2 media with an additional agar layer of 10 mL to suppress nutrient release from compost into the fluid phase.
2.4.2 Additional germination experiment information
● gibberellic acid: 1µM (dissolved in distilled water and sterile filtered over 30µm)
● red light pulses: well plates were incubated over a period of 48h hours under red light with dark conditions in between. Red light pulses of 30 minutes were given at incubation start as well as after 4.5 hours and 9 hours; after 48 hours well-plates were constant incubated under white light
● sediment originated from Lausiger Teiche (Bad Schmiedeberg, Saxony-Anhalt, Germany)
● spectral distribution of fluorescent lamps (experiment 5) is given in Figure S4.4.
2.5 Oospore analysis
Oospores of S276, NIES-1604, LaT-2708 and Ranstadt were analyzed by quantitative and qualitative morphology parameters, defined after Soulié-Märsche and García, 2014. Therefore, oospores were placed on microscope slides with an adhesive surface and documented with a stereomicroscope in lateral, apical and basal view (Leica DM6000 CS). Oospore colour, shape and appendixes such as claws or cages were determined with LI to avoid misinterpretation by photodocumentation. NIES-1604 oospores were pre-treated with sonication for 2minutes at 20°C and 10% Power (Sonorex Super 10P). Parameters, such as number of striae, length, width, fossa width, basal impressions and angle of stria to the longitudinal axis were measured with ImageJ. Statistical data analyses for differences between strains were performed using GraphPad Prism 9.3.1, see paragraph 2.3. (Graph Pad Software, San Diego, USA).
3 Results
3.1 Long-term cultivation
To find an optimal cultivation protocol that facilitates the reduction of epiphytes, different methods have been tested over the past 4 years (2018-2022). Most of them resulted in contamination and subsequent death of used thalli. These tests are listed in Table 1, methods that led to an extremely contaminated state were not further focused on.
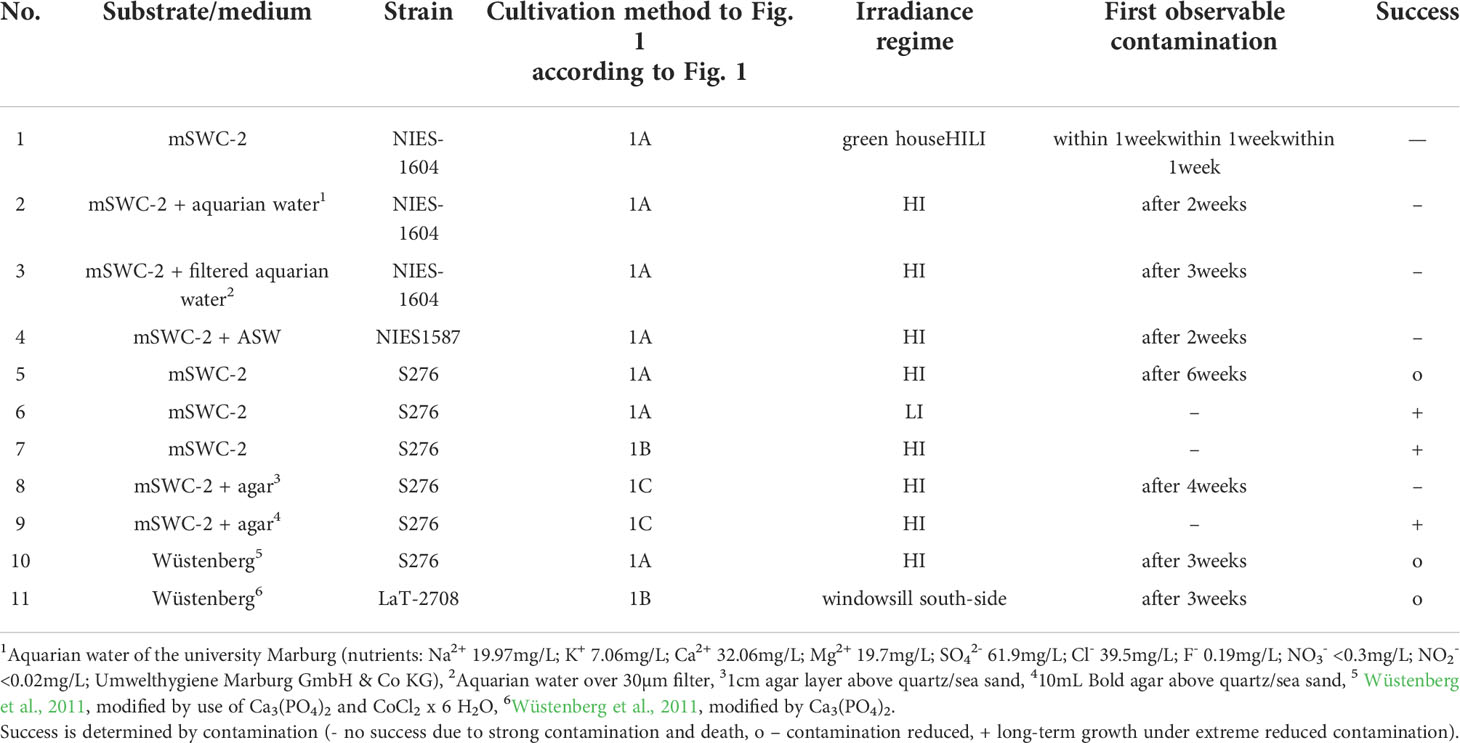
Table 1 Overview of tested substrate/media compositions on the strains NIES-1604, S276 (NIES-1591, NIES-1593) and Lausiger Teiche (LaT-2708).
In a few vessels, the presence of cyanobacteria was observed after transferring of plants. This could be identified via microscopic analyses as e.g. Nostoc sp. or Chlorococcales and confirmed via genetic analyses. In most cases, C. braunii develop male and unfertilized female gametangia but fertilization seem to be inhibited.
3.2 Short-term cultivation
3.2.1 Substrate
Incubation of S276 using two different composts showed significant differences in oogonia formation after one week of incubation (p<0.0001) due to the absence at the beginning and formation within one week using GAR. However, the range of variability for gametangia-specific traits and the formation of new whorls and lateral branches was larger for Gardol® (GAR) than for compost from the Botanical Garden University Marburg (BGUM18, Table S1). Using GAR, an increased rate of thallus decay, a reduced and decelerated production of antheridia and oogonia on newly formed lateral branches was observed. In contrast, thalli that were incubated with BGUM18 as substrate, formed and released matured oospores from the main thalli and lateral branches. Seven to eight lateral branches were developed per thalli using BGUM18 and five to eight per thalli on GAR (Table S1).
The analysis of the compost types, BGUM from 2018 (BGUM18) and 2021 (BGUM21) and GAR for their nutritional composition (Table 2) showed differences in macro- and micronutrient composition. For example, the BGUM18 contained half the phosphorus (P2O5) and fourfold lower amount of potassium oxide (K2O) compared to GAR. Additionally, cations of trace elements such as copper, boron, zinc and cadmium are twofold to fourfold lower in BGUM18 compared to GAR, whereas the total contents, plant unavailable nutrients, were lower in GAR than BGUM18 and BGUM21. Contents of manganese and molybdenum were slightly increased for GAR as compared to BGUM18/21. Between BGUM18 and BGUM21 few differences exist, but seem not to be decisive for cultivation and growth than compared to GAR.
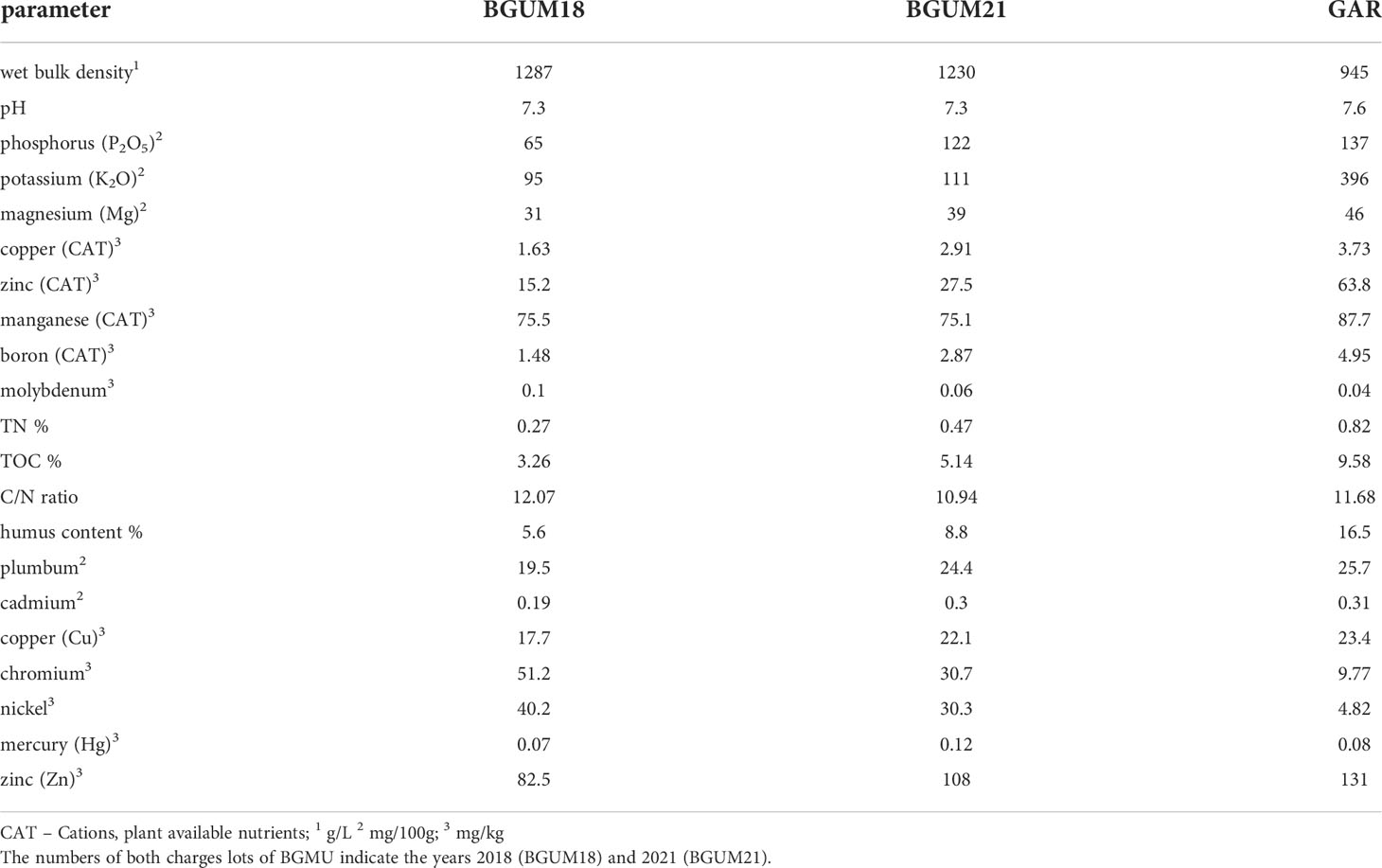
Table 2 Results of nutrient analysis of both substrates, the compost from the Botanical Garden of Marburg (BGUM) and Gardol® (GAR).
3.2.2 Modified Wüstenberg medium
Cultivation of C. braunii S276 using a modified Wüstenberg approach by Ca3(PO4)2 and CoCl2 instead of mSWC-2 resulted in a lack of lateral branches (p=0.0138), internode length (p=0.0146), contamination (p<0.0001) and oospore formation and release from the thallus during the experimental phase. The development of lateral branches could not be observed using Wüstenberg medium, whereas branching was regularly observed by cultivation in mSWC-2 medium (Figure 2). However, maturation of fertilized oogonia to black oospores occurred faster in mSWC-2 medium compared to Wüstenberg medium (Table S1). Moreover, in two out of three vessels with Wüstenberg medium white (not fertilized) oogonia on lower whorls showed signs of contamination and delayed ripening of oospores (Figure 2).
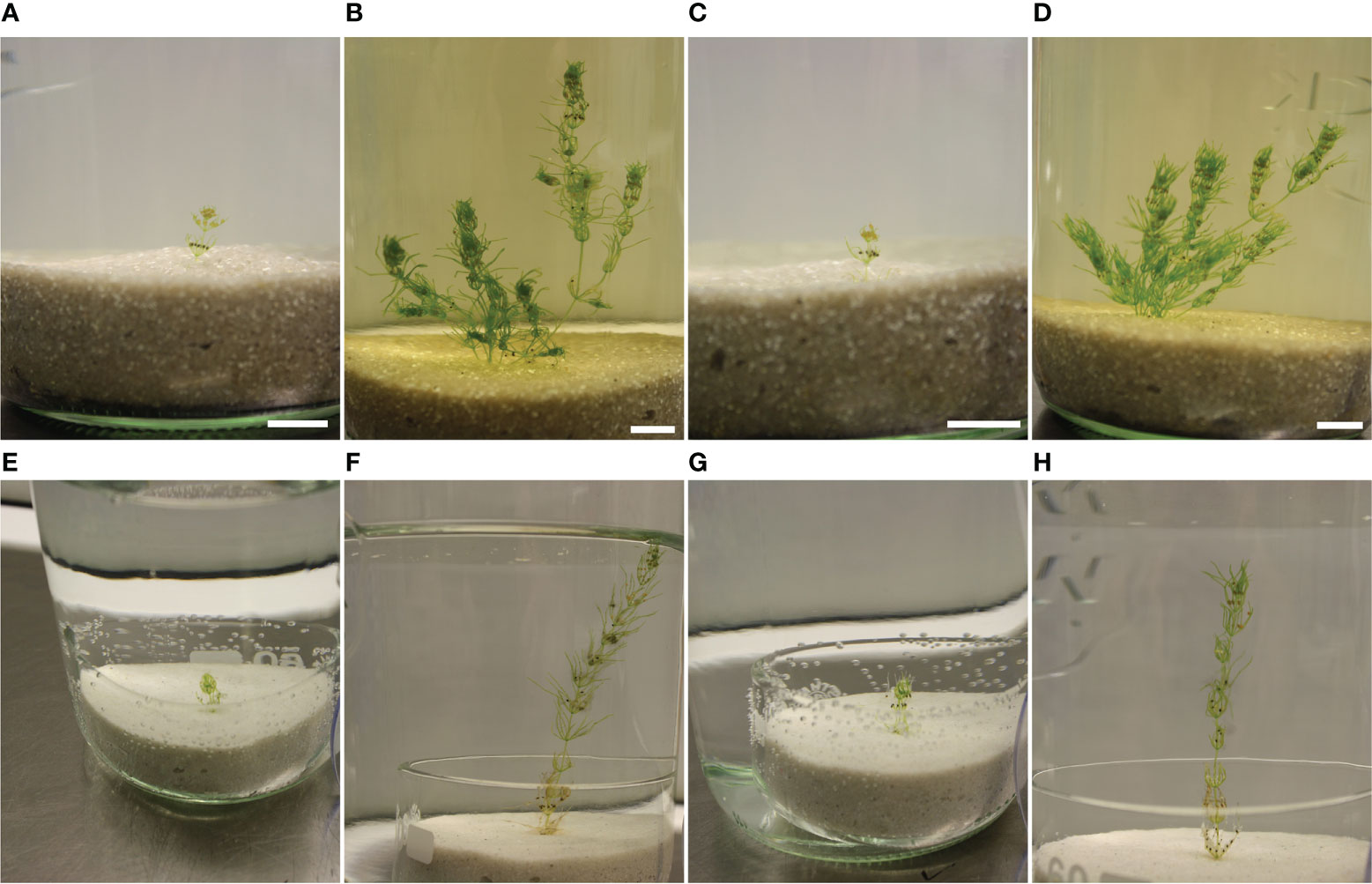
Figure 2 Overview of C. braunii S276 cultivation using modified Wüstenberg media or mSWC-2 media. (A–D). Cultivation of C. braunii using mSWC-2 media, A+C start of cultivation, B+D after a period of 7 weeks. (E–H) Cultivation of C. braunii in modified Wüstenberg medium over 7 weeks (F, H).
3.2.3 Irradiance regime
C. braunii NIES-1604 and S276 were incubated under low irradiances (30 µmol photons/(s*m2), termed LI) and higher irradiances (70µm photons/(s*m2), HI) for four weeks. Generally, differences between strains were higher than between irradiance regimes (Table S1). Within the strains, morphologically significant differences and contaminations could only be detected for cultures of NIES-1604 under both, LI and HI in terms of the presence of gametangia. Cultures of S276, however, did not show differences between LI and HI in terms of morphological traits.
Comparing the strains under LI or HI conditions, contamination and the number of oospores on all whorls differ significantly (p<.0001). Compared to S276, a lesser amount of oogonia was produced by NIES-1604 after two (p<.0001) and three weeks (p<.0001) of incubation. The number of lateral branches under HI conditions was higher for S276 (4-8 new lateral branches) than for NIES-1604 (1-3), whereas under LI conditions, both NIES-1604 (1-2) and S276 (1-5) developed fewer lateral branches. No obvious contamination could be detected for S276 during the whole experimental period, whereas all thalli of NIES-1604 were visibly contaminated after one week of incubation with increasing contamination during the further course of the experiment (p<.0001).
Under both conditions, HI and LI, S276 released mature oospores from thalli, whereas only few oospores were released by NIES-1604 under HI conditions. Differences of S276 between HI and LI after 2 weeks resulted from differences of starting material and the non-formation of new oospores within 2 weeks of incubation. Numbers ranged for NIES-1604 under HI from 60 to 103, whereas S276 released under LI 48 to 176 and under HI 120-865 oospores during the whole period of observation (18 months) 18 months. Most oospores of NIES-1604 remained on the thalli or on fragmented whorls.
Lateral thalli of C. braunii LaT-2708 were cultured after strain establishment (16 weeks) for 8 weeks under four different irradiances to test the irradiance effect on cultivation, oospore formation and maturation (Figure 3). During this period, only thalli under lowest irradiances produced continuous oospores whereas the number of oospores under intermediate irradiances and on windowsill decreases over time. Only thalli under highest irradiances have completely stopped oospore formation.
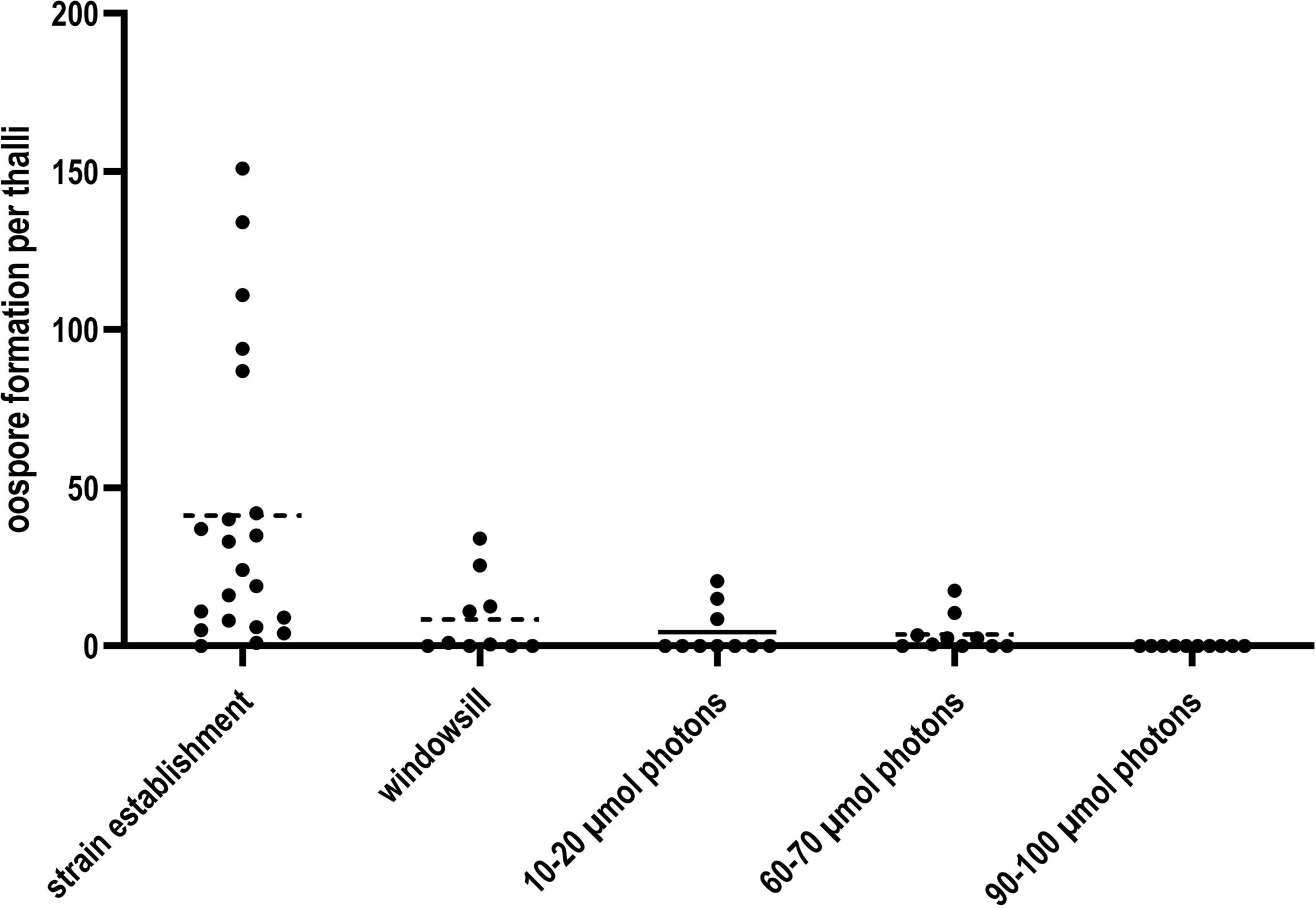
Figure 3 Oospore formation of C. braunii LaT-2708 per thalli over 16 weeks strain establishment and the four tested different light irradiances.
In terms of morphology, slight differences among the three irradiances could be achieved by use of fluorescent tubes. The length and width of the last produced internode differed significantly among the irradiance regimes. Shortest and smallest first internodes were obtained under 50 to 60 µmol photons/(s*m2), whereas longest and widest first internodes were determined for highest irradiances of 90 to 100 µmol photons/(s*m2) (Table S2).
3.3 Germination experiments
The results of germination assays comparing storage temperatures (4°C or room temperature of approximately 24°C), substrates, the influence of gibberellic acid (GA) and irradiance regimes (white light (WL), red light pulses (RP) or white light with far-red light (WL+FR)) as well as combinations of them are listed in Table 3, spectral distributions in Figures S4.1-S4.4.
With respect to pre-treatment, germination rates ranged between 0 and 66.7% for non-sterilized oospores and between 0 and 23.3% for sodium hydrochloride (NaOCl)- treated oospores. Oospores that were treated with hydrogen peroxide do not germinate.
The results show that oospore germination is dependent on the combination of (1) temperature, (2) sterility and (3) the presence of GA and RP. Significant differences can be detected for all assays with (a) low temperatures (4°C) and non-sterilized oospores (ANOVA, p<.0001), (b) room temperatures and a low solution of sodium hypochlorite (3%) (ANOVA,.0003 ≤ p ≤.0079) or (c) room temperatures, non-sterilized oospores and a combination of GA and RP (ANOVA, p<.0001). Using the latter combination, the highest germination rate for non-sterilized oospores (66.7%) was obtained. Lower rates were achieved from oospores stored at room temperatures without further treatment (3.3%). Short-term stratification, long-term desiccation, GA or RP, if applied individually, resulted in no germination at all of Chara braunii S276 and NIES-1604 oospores. For NaOCl-treated oospores, the highest germination rate of 23.3% was achieved by oospores stored at room temperature without additional treatments. Germination was also induced on oospores stored at room temperature that were treated with red light pulses (10%) or on oospores stored under cold conditions (4°C, 3.3%).
In germination assays with habitat sediment form Lausiger Teiche (Experiment 6, Table 3) as substrate, only oospores from LaT-2708 germinated with a rate of 56.67%. Due to material availability, comparable assays with substrates from Hawaii or Japan could not be carried out.
After germination in 24-well plates under 25-30 µmol photons/(s*m2), germlings were transferred either directly into 290 mL vessels using the mSWC-2 method (50-60 µmol photons/(s*m2)) or after an intermediate period in Bold-agar with/without compost extract (20-30 µmol photons/(s*m2) subsequently transferred to 290 mL vessels under higher irradiances of 50-60 µmol photons/(s*m2). Directly transferred germlings either died or showed contaminations after several weeks of incubation and did not develop oospores irrespective of the presence of gametangia. In contrast, germlings that rested for five months in Bold agar with/without compost extract in Wüstenberg medium before being transferred into 290 mL and 600 mL vessels exhibited vegetative growth and developed oospores, except for one individual, after transfer into compost-quartz and distilled water.
Using this modified protocol, the germination of oospores could successfully be initiated, including the subsequent production of thalli that were able to complete the entire life cycle (Figure 4).
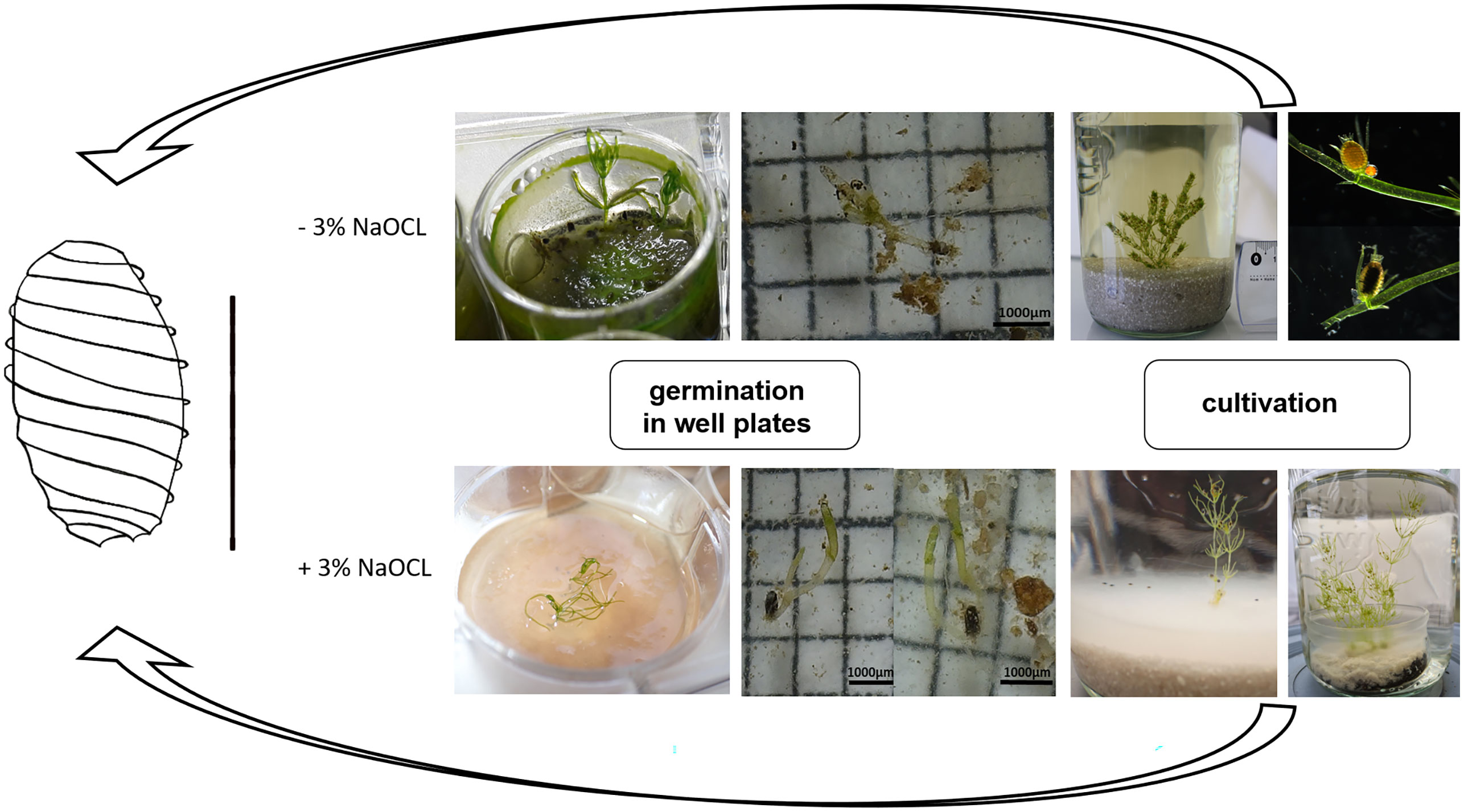
Figure 4 Life cycle cultivation protocol of C. braunii. Germlings of C. braunii established by germination of non-sterilized (-3% NaOCl; upper panels) or sterilized (+3% NaOCl, lower panels) oospores in well plates. Subsequent cultivation using compost-quartz sand or compost-quartz sand-agar combinations with distilled water led to growth of thalli and reproductive units.
Morphological differences were observed between C. braunii S276 grown on Bold agar with or without compost extract in gametangia formation and banding pattern (Spear et al., 1969). Germlings in agar with compost extract showed a brownish banding, restricted to branchlets only (Figure 5A) and developed no gametangia, whereas those in Bold agar without compost extract developed alkaline bands on internodes and, less pronounced, on branchlets as well (Figures 5B-D). Both, antheridia and oogonia were developed on thalli grown in Bold agar. This difference was independent from preceding NaOCl-treatment of the oospores, pointing to a compost effect manifested at the vegetative part.
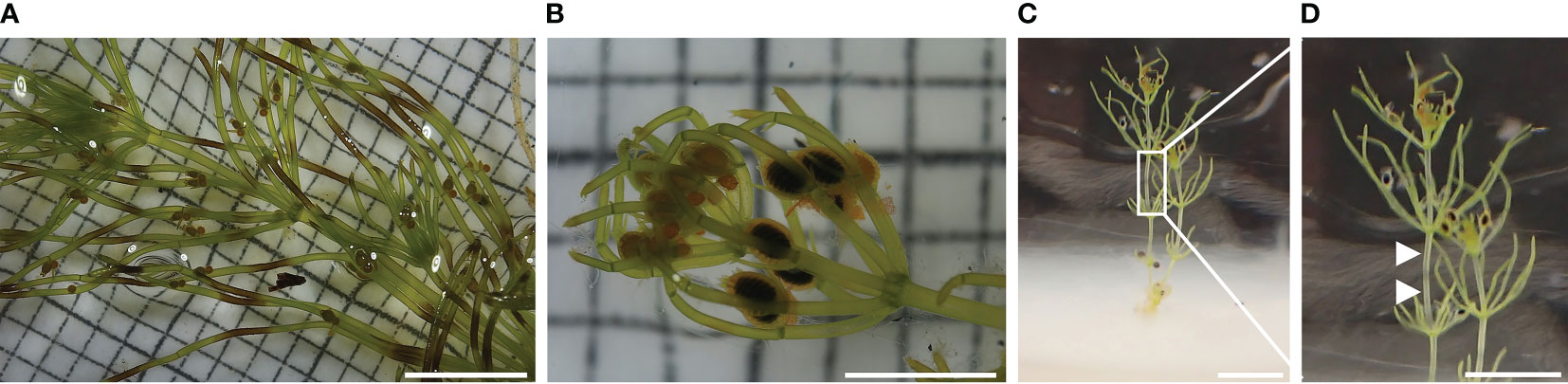
Figure 5 Thalli grown from germinated C. braunii S276 oospores after acclimation using Bold agar with or without compost extract. (A) thalli grown by germinated, not sterilized oospore with brownish banding pattern on branchlets after acclimation in Bold agar with compost extract, (B) thalli grown by germinated, sterilized oospore without brownish banding pattern. (C) thalli grown by germinated, sterilized oospore after acclimation in Bold agar without compost extract showing alkaline bands on internodes. (D) Detail view of Chara braunii internode with alkaline bands (arrows).
3.4 Interpopulation oospore variation
The principal component analysis of C. braunii oospore features of four different populations illustrate a regional separation of S276 oospores from Japan, Hawaii and Germany (Figure 6). S276 oospores are longer and broader than oospores from Hawaii (ANOVA, p < 0.0001) or Germany (ANOVA, p < 0.0001) (Table 4). Additionally, S276 oospores differ significant from LaT-2708 or Ranstadt by their fossa widths (ANOVA, p<0.0009) or basal impressions (ANOVA, p<0.0001). Oospore features of German sites, LaT-2708 and Ranstadt, overlapped completely.
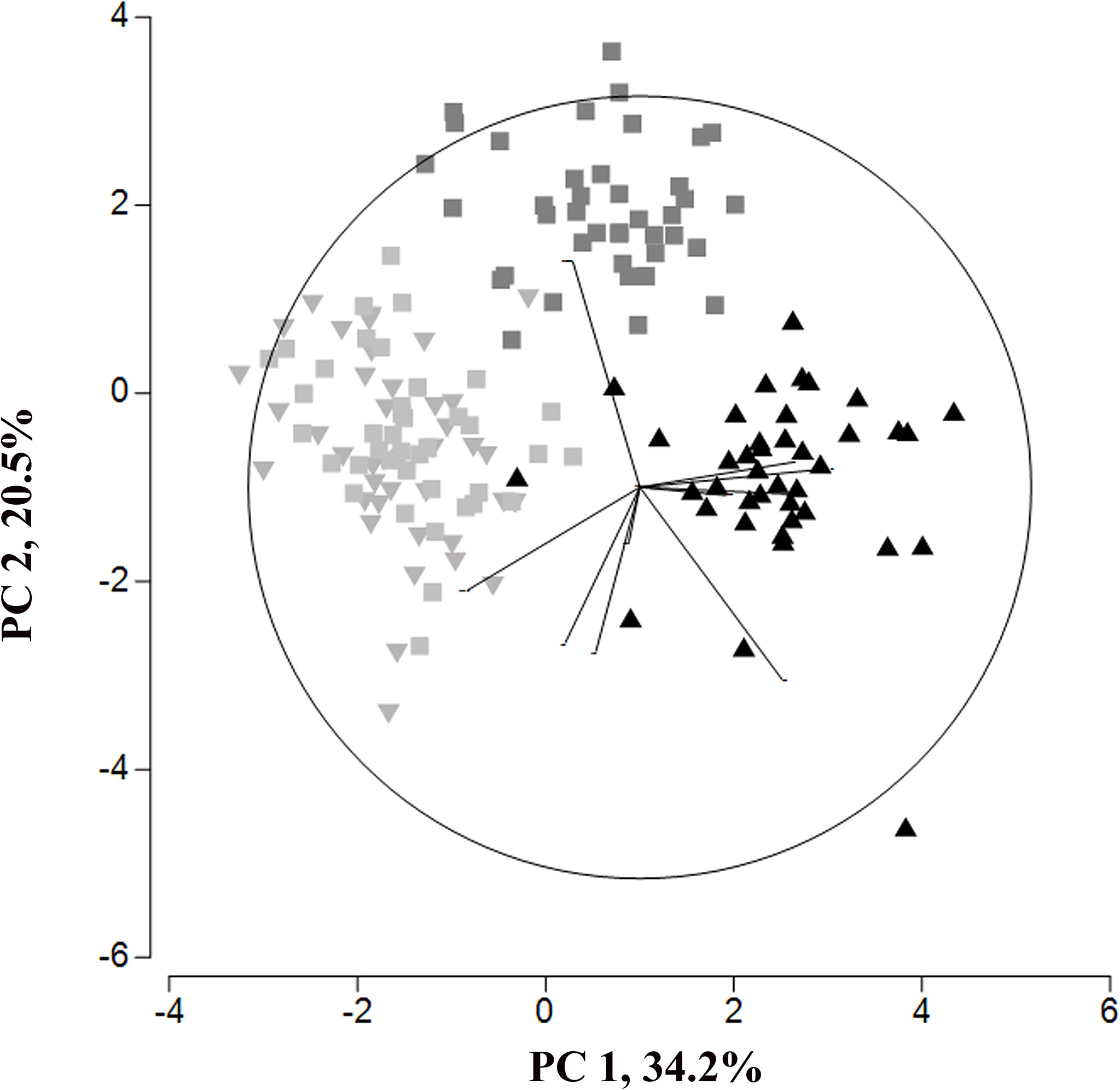
Figure 6 Principal Component analysis of oospore feature from four different C. braunii populations (black triangle - S276, Lake Kasumigaura; grey inverted triangle – LaT-2708, Lausiger Teiche; light grey squares – Ranstadt; dark grey squares – NIES-1604, Hawaii).

Table 4 Measurements of Chara braunii oospore characteristics from Japan (S276), Hawaii (NIES-1604) and Germany (LaT-2708 and Ranstadt).
4 Discussion
4.1 Cultivation
Long-term in vitro cultivation is essential for the establishment of model systems applicable in basic as well as applied research. However, maintaining cultures over long periods of time is a challenging task and requires the identification and optimization of parameters that determine plant development, growth, and reproduction, adjusted to the species. In Arabidopsis thaliana, long-term cultivation has been linked to age-dependent epigenetic changes such as decreasing proliferation rates, increasing senescence in gene activity, and DNA-methylation (Kwiatkowska et al., 2014). In the moss model Physcomitrium patens, cultured since the 1960ties, it could be demonstrated that prolonged vegetative propagation leads to accumulation of deleterious somatic mutations (Haas et al., 2020). Such effects can be avoided by regular sexual reproduction, making the ability to complete the life cycle in cultivation an important asset. However, studies reporting long-term effects appearing under constant cultivation conditions are rare compared to the vast number of short-term acclimation studies.
Extracting the set of factor combinations necessary for the successful in vitro cultivation of Charophyceae from the overwhelming complexity of environmental variables is a task requiring a stepwise design followed by multidimensional assays. Results of lab-cultivation approaches, most of them short-term acclimation ones, have been published targeting mainly on the effects of light regime, temperature, substrate, media composition or even the influence of biotic environmental components as, e.g., crustaceans or water slugs (Richter, 1894; Kuczewski, 1906; Ernst, 1917; Karling, 1924; Ding et al., 1991). However, for long term cultivation of model systems factors as, e.g., naturally adhering microorganisms, should be considered as well.
The results presented here showed that long-term maintenance of C. braunii S276 by vegetative propagation over 8 years is possible by means of existing protocols, but were accompanied by increasing biofilm-formation and decreased fertility. The addition of organic substrates (BGUM18, BGUM21, Table 2) and quarz in combination with deionized water, allowed for constant completion of life cycle without apparent senescence effects. In this context, periodic transfer of thalli to newly prepared culture vessels were shown as option to restrict biofilm formation, although the release of endophytic grown cyanobacteria and algae, e.g. Coleochaete sp. (Cimino and Delwiche, 2002) can be triggered.
For contaminant-reduced cultures, fully synthetic media (with respect to substrate as well as medium) would serve best in theory, because they can serve directly for axenic cultivation of sterilized organisms as well. In Wüstenberg medium, the development of lateral branches was supressed, indicating that the axial bud cell (“Achselursprungszelle”) (Kuczewski, 1906) is formed but did not develop. Addition of compost and use of deionized water as liquid component of the culture overcame this problem; under such conditions lateral formation was regularly observed. The question to what extent the two components – addition of compost and withdrawing nutrients from the liquid phase - contributed to the initialization of lateral development must be investigated in forthcoming long term experiments. Simultaneously, an increased occurrence of microorganisms (fungi), especially on female gametangia, was observable (Figure 7). Different associated fungi’s have been identified as endo- and epiphytes (Kataržytė et al., 2017). Detailed interactions and effects on fructification as well as underling gene metabolisms have been proven in further studies.
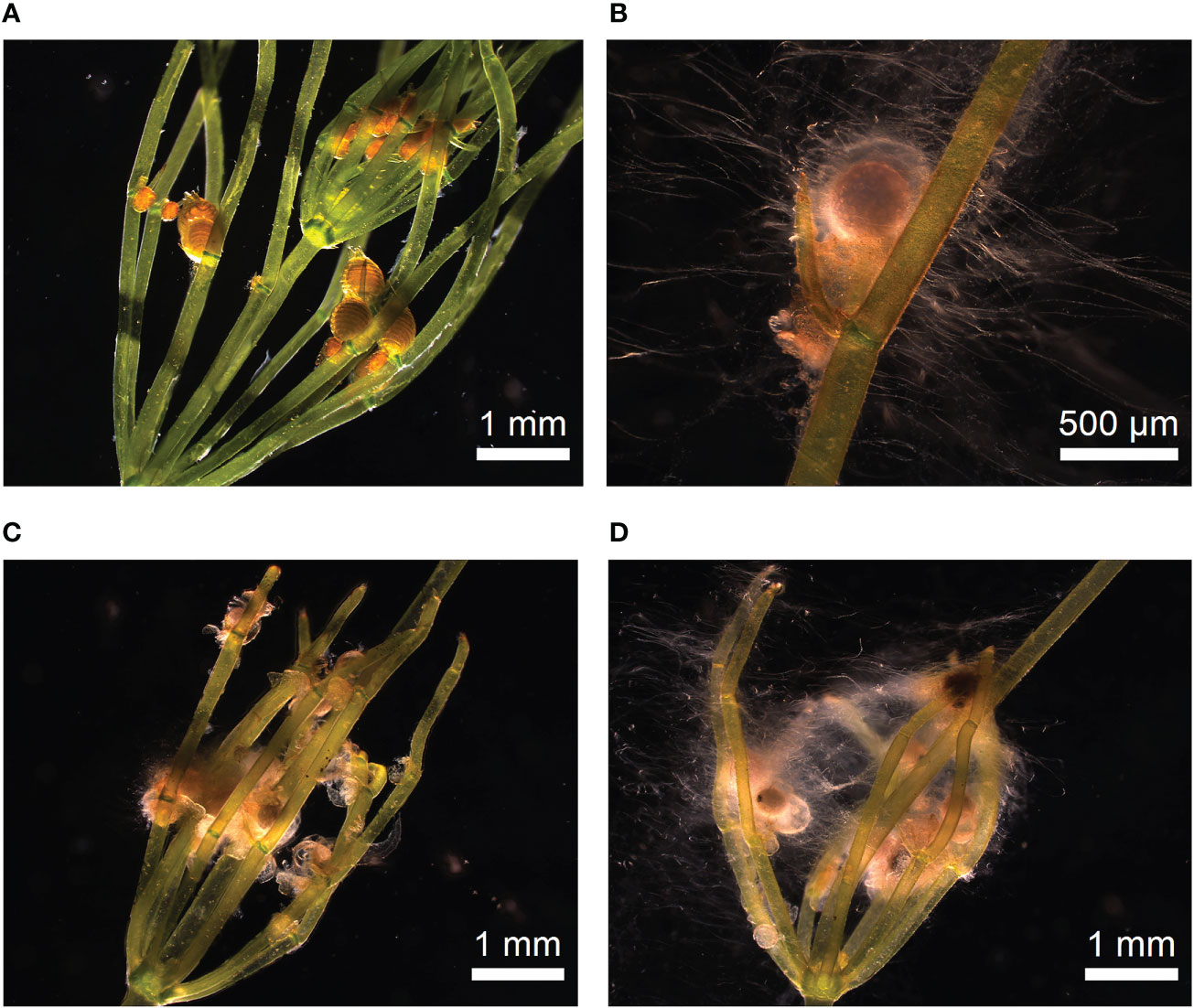
Figure 7 Occurrence of microbes during oogenesis. (A) Thalli with fresh antheridia and oogonia after two weeks of incubation. Gametangia showed no visible microbial occurrence. (B–D) Microbe occurrence during ripening of gametangia and fertilisation.
Compost, natural material resulting from aerobic transformation of organic material by microorganism, is characterized by different composition depending on the raw material and used for increase of soil fertility or plant nutrition. In Germany, quality assurance and certification are implemented by the RAL-quality label (RAL-GZ 251) through the Federal Compost Quality Association. The reproducibility of cultivation results in Charophyceae studies are often restricted by use of composts with not fully defined components (Imahori and Iwasa, 1965; Smart and Barko, 1984; Sakayama et al., 2004; Sato et al., 2014). On the other hand, withdrawing nutrients from the liquid phase consequently requires full-scale nutrition via rhizoids including trace elements. In order to develop a completely synthetic medium optimized concentrations of essential elements must be identified in respect to bioavailable forms and chemical reactions. In our approaches and in agreement to observation in natural habitats (Schubert et al., 2018), higher concentrations of available phosphorous, potassium, copper, zinc and boron (labile forms, CAT) cause an increase in phytoplankton and microbes (Cotner and Biddanda, 2002; Gross et al., 2007; Talling, 2010). Simultaneously, decreased potassium concentrations are directly linked with photosynthesis (Tränkner et al., 2018). A chlorophyll decrease is described for C. zeylanica (Anderson, 1958). Furthermore, interplays of e.g. sulphate, chloride, potassium and boron’s by increased solubility of potassium-boron’s through higher chloride or sulphate concentrations could effect chemical nutrient cycles but details about interplays are not known so far (Li et al., 2020). For the green alga Chlorella vulgaris inhibitory effects of increased boron concentration have been shown including activation of defense mechanisms (Chen et al., 2019), which are linked to increased DNA-methylation and microRNA (miRNA) expression (Wei et al., 2009; Zemach and Zilberman, 2010; Mahbub et al., 2020).
Besides substrate and medium, irradiance is a main factor for autotrophs and several acclimation studies on Charophyceae have demonstrated irradiance effects on oospore lengths, presence of cortication, gametangia development and length growth (Richter, 1894; Karling, 1924; Maszewski, 1980; Sato et al., 2014). However, the variability of this abiotic factor regarding, e.g. spectral distribution, intensity, rhythm, or even angle of incidence, could also hinder the comparability of transcriptomic analyses of e.g., stress or hormonal responses by changes in expression of light complex harvesting genes (Han et al., 2019). Our long-term and short-term studies obtained from C. braunii S276 and NIES-1604 under vertical (from above the vessels) WL illumination in LI and HI conditions revealed that differences in morphological and cultural traits are higher between strains than between intensities. Under both conditions, C. braunii S276 showed a release of oospores but no visible contamination. Additionally, under HI conditions, a higher biomass production by lateral branches was observed. In contrast to the experiments with Wüstenberg media, lateral branches were formed by both strains under LI and HI conditions, with a reduced number under LI conditions. The impact of changing light conditions on branching remains inconclusive for these experiments but appears less essential than the nutrient-driven lack of formation of lateral branches in Wüstenberg medium. Consequently, the protocol developed can be considered to serve sufficiently uniform material for further studies. Cultivation in deionized water in combination with nutrition at irradiances of 25-40 µmol photons/(s*m2) and 22°C resulted not only at high growth rates (approximately four to nine new whorls) and regular branching, but also the formation and release of oospores. Temperature has been shown an essential factor for fertilization (Croy, 1979). In our studies at cultivation temperatures of 16°C, C. braunii produced both, male and female gametangia, but no fertilization occurred. A suppression of oogonial primordium development at irradiances above 10 µmol photons/(s*m2) as reported in a previous study of Sato et al. (2014) was not observed. The reason for this remains unclear, but given the large differences between the emission characteristics of fluorescence tubes, an effect of spectral composition is a likely candidate, highlighting the importance of following the protocol also in details as, e.g. brand and type of illumination sources.
In contrast, the angle of incidence on windowsill cultures is subject to seasonal change. Variability in cardinal-directed orientation of Chara cultures in accordance with natural environmental conditions in aquatic systems is intended to simulate the most natural solar gradient possible for in vitro cultures. These results are partly comparable to what could be shown for Chara fragilis by Karling (1924). However, the number of oospores was smaller than in experiments with C. braunii S276 under constant WL conditions. Cultures that were collected at the beginning of September and cultivated on east-south windowsills died, probably due to the insufficient light intensity necessary for thalli regeneration. Our results have proven that mimicking natural variability in irradiance is not a prerequisite for successful long-term cultivation. Moreover, in contrast to windowsill-cultivation the use of artificial light sources at constant irradiance regimes as done here can serve for season-independent growth and reproduction, making standardized material available throughout the year.
4.2 Germination
In order to establish axenic cultures, sterilized plant material must be achieved. Except for the presence of endobacteria, this can be done best by germination of sterilized oospores, which are very resistant to harsh environmental conditions. In addition, controlled induction of germination is a prerequisite for establishing sexually reproducing cultures to prevent depression effects of long-term cultivations as outlined above. Germination of seeds and spores is known to be influenced by abiotic factors such as temperature, light availability, salinity and nutrient conditions, which needs to be investigated the same way as outlined above for vegetative growth. Although theoretical models for breaking the dormancy and inducing germination, such as the hypothetical model for physiological dormancy of Hilhorst (Hilhorst, 1998), exist, the actual application to Charophyceae oospores under in vitro conditions is time-consuming and non-trivial. Studies on influential factors such as thermal stratification (Forsberg, 1965c; Forsberg, 1966; Sokol and Stross, 1986; Casanova and Brock, 1996; Stross, 1989), redox potential changes (Proctor, 1967; Bonis et al., 1995; Casanova and Brock, 1996), organic material (Zenker, 2003; Holzhausen et al., 2017), growth inhibitors (Sabbatini et al., 1987), or light regimes (Forsberg, 1965c; Takatori and Imahori, 1971; Sokol and Stross, 1992; Holzhausen et al., 2017) have been conducted for several Chara, Tolypella and Nitella species, demonstrating a large degree of species specificity.
Our results, obtained from non-treated and treated oospores of C. braunii S276, support the theory of genetically fixed germination programmes. Stratification of C. braunii S276 oospores inhibited germination, whereas storage at room temperature of approximately 22°C ( ± 2°C) promoted germination. Red light stimuli combined with gibberellic acid were first described by Sederias and Colman (2007) to induce germination in C. vulgaris and also affected germination of C. braunii S276 in our experiments. In contrast to C. vulgaris, the effect of red light on germination of C. braunii could only be detected for oospores stored at room temperature and not for stratified oospores. Germination induction of seeds via red light treatment is known for many plant species, e.g. for European forest seeds (Kolodziejek et al., 2017). These results could be linked with the natural origin of the wildtype material of C. braunii S276 from the region of Zonobiom V with temperature-dependent seasons and short cool winters (Pott, 2005). Germination assays under LI conditions combined with far-red light failed in this study. Our results show, that C. braunii for sure is not a negatively photoblastic species, which would only germinate in darkness. Moreover, the observed stimulating effect of red light pulses suggest the potential involvement of a phytochrome-like receptor system in germination induction.
Germination rates of oospores under WL cLED’s were lower than those obtained by combination of red light, gibberellic acid and BGUM18 substrate, but approximately the same as induced by the red light stimulus as individual factor. These results fully coincide with studies of Takatori and Imahori on C. delicatula (1971). The spectra of the cLEDs used here correspond, except for the short-wavelength edge, well with underwater spectra in shallow coastal lagoons (Sagert and Schubert, 1999).
Since germination of oospores could not be induced via treatment with gibberellic acid alone, but seemed to increase the promoting effect of red light, combination effects of other factors are not unlikely and offer a fascinating field for further investigations by, e.g. transcriptomic analyses of unravelling the germination process in detail.
The link of light and nitrogen availability affecting germination of seeds is not fully understood but could be demonstrated to affect germination of several plant species (Hilhorst and Carssen, 2000; Kolodziejek et al., 2017) as well as Charophyceae (Rodrigo et al., 2007). In our study, no dedicated experiment was performed but slight variations in C:N ratios between the different types of compost used (12:1 for BGUM18, 10.9:1 for BGUM21 and 11.7:1 for GAR) could be at least part of the explanation, why successful germination occurred only with BGUM18, having the highest C:N ratio.
4.3 Interpopulation oospore variation
The results of analyzing the oospore variation among four C. braunii populations have shown the significant separation of S276 oospores from NIES-1604 as well as the German strains LaT-2708 and Ranstadt on the basis of length, width, fossa width and basal impression. Additonally, oospores of NIES-1604 differ in length and width. Compared to existing data, oospores of S276 and NIES-1604 correspond to the given ranges (Proctor, 1970; Haas, 1994; Krause, 1997; Boszke et al., 2008). Oospores of the German sites, Lausiger Teiche and Ranstadt are shorter and smaller compared to literature data and the remaining strains. Between both German strains, no differences could be detected which underpin the results of regional studies in India, Poland or Sweden with relative uniform C. braunii oospores. In regard to recent phylogenetic studies, the results of oospore studies could confirm the results of genetic entities by Kato et al., 2008 by morphological differences between S276 and NIES-1604. Additionally, German strains differ from both, the Hawaiian and Japanese strain and indicate the existence of an additional entity by regional separation based on morphological data. Nonetheless, within German sites and shorter regional boundaries, no differences could be detected and imply the same entity.
5 Conclusion
In summary, the results showed a successfully optimized cultivation and germination protocol for C. braunii, allowing for stable long-term maintenance of cultures with generative reproduction and able to provide sterile material. In any case the protocol presented here offers a unique opportunity for transcriptomic studies with uniform material and to study the potential microbial effects on the ontogenesis of Charophyceae, whose existence have been hypothesized by Holzhausen, 2016 already. Nonetheless, these studies also suggest that more research is needed in the fascinating field of Charophyceae cultivation and germination, e.g. by optimizing of nutritional media or conditions.
Data availability statement
The original contributions presented in the study are included in the article/Supplementary Material, further inquiries can be directed to the corresponding author.
Author contributions
Conceptualization – SAR, HS, AH; methodology – AH, CK, SR, NS; validation - AH, CK, SR, NS; formal analysis - AH, CK, SR, NS; investigation - AH, CK, SR, NS; resources – SAR, AH, HS; original draft preparation - AH; review and editing – SAR, HS; visualization - AH; supervision - AH, SAR; funding acquisition – SAR, HS. All authors contributed to the article and approved the submitted version.
Funding
This research was funded by DFG (CharMod; RE 1697/16-1; Schu983/23-1). The project was associated with MAdLand (http://madland.science, DFG priority program 2237, RE 1697/19-1). Open Access funding provided by the Open Access Publication Fund of Philipps-Universität Marburg with support of the Deutsche Forschungsgemeinschaft (DFG, German Research Foundation).
Acknowledgments
The authors are thankful for the constructive suggestions of two reviewers, Janine Fürst-Janssen and Eduardo Flores-Sandoval. Thanks are due to the “Hessische Landeslabor”, Jennifer Löber and Fabian Jacobi for preparation of compost and media analyses. Marco Göttig, Evelyn Vollmeister, Fabian Haas (University Marburg) and Susanne Thuemecke provided support for students, data analysis and imaging. Uwe Raabe provided plant material (Ranstadt) and was involved in species discussions; Arne Schoor, Christian Porsche and Birgit Munzert (University Rostock) provided technical support in cultivation setup and spectrophotometric analyses, which is gratefully acknowledged by the authors.
Conflict of interest
The authors declare that the research was conducted in the absence of any commercial or financial relationships that could be construed as a potential conflict of interest.
Publisher’s note
All claims expressed in this article are solely those of the authors and do not necessarily represent those of their affiliated organizations, or those of the publisher, the editors and the reviewers. Any product that may be evaluated in this article, or claim that may be made by its manufacturer, is not guaranteed or endorsed by the publisher.
Supplementary material
The Supplementary Material for this article can be found online at: https://www.frontiersin.org/articles/10.3389/fpls.2022.987741/full#supplementary-material
Supplementary Figure 4 | Spectral distribution of light sources. (1). cLED white bi‐phosphor 4000K moisture‐protected LEDs, PlantClimatics GmbH, (2) cLED far-red, PlantCLimatics GmbH, (3) fluorescent lamps (Signify GmbH (Philips)), (4) fluorescent lamps Phillips TLD 36W/950 (Signify GmbH (Philips)), (5) windowsill culture, data were obtained by datalogger (MX2202 HOBO Pendant® MX Temperature & Light Data Logger).
References
Anderson, R. G. (1958). The growth and reproduction of chara in a definable nutrient medium. Ph.D (University Of Nebraska, Lincoln (Nebraska)).
Andrews, M., McInroy, S., Raven, J. A. (1984). Culture of Chara hispida. Br. Phycological J. 19(3), 277–280.
Beilby, M. J. (2019). Chara braunii genome: a new resource for plant electrophysiology. Biophys. Rev. 11, 235–239. doi: 10.1007/s12551-019-00512-7
Bonis, A., Lepart, J., Grillas, P. (1995). Seed bank dynamics and coexistence of annual macrophytes in a temporary and variable habitat. Oikos 74, 81–92. doi: 10.2307/3545677
Boszke, P., Pełechaty, M., Pukacz, A. (2008). Oospore dimensions and wall ornamentation in Chara braunii. Biologia 63, 457–460. doi: 10.2478/s11756-008-0079-y
Buchberger, T., Lamparter, T. (2015). Streptophyte phytochromes exhibit an n-terminus of cyanobacterial origin and a c-terminus of proteobacterial origin. BMC Res. Notes 8, 1–13. doi: 10.1186/s13104-015-1082-3
Casanova, M. T. (2014). A revision of Chara sect. Charopsis (Characeae: Charophyceae) in Australia, including specimens collected for bush blitz. Aust. Systematic Bot. 27, 403–414. doi: 10.1071/SB14032
Casanova, M. T., Brock, M. A. (1996). Can oospore germination pattems explain charophyte distribution in permanent and temporary wetlands? Aquat. Bot. 54, 297–312. doi: 10.1016/0304-3770(96)01032-7
Cheng, S., Xian, W., Fu, Y., Marin, B., Keller, J., Wu, T., et al. (2019). Genomes of subaerial zygnematophyceae provide insights into land plant evolution. Cell 179 1057-1067, e14. doi: 10.1016/j.cell.2019.10.019
Chen, X.-Q., Su, L., Yin, X., Pei, Y. (2019). Responses of Chlorella vulgaris exposed to boron: Mechanisms of toxicity assessed by multiple endpoints. Environ. Toxicol. Pharmacol. 70, 1057–1067. doi: 10.1016/j.etap.2019.103208
Chowdary, D. N. K. (2014). Exploring Chara as a model system to study the function of homeobox genes in macroalgae. University of Indian Institute of Science Education and Research, Pune. 1–55.
Cimino, M. T., Delwiche, C. F. (2002). Molecular and morphological data identify a cryptic species complex in endophytic members of the genus Coleochaete Bréb.(Charophyta: Coleochaetaceae). J. Phycology 38, 1213–1221. doi: 10.1046/j.1529-8817.2002.02067.x
Coelho, S., Scornet, D., Rousvoal, S., Peters, N., Dartevelle, L., Peters, A., et al. (2012). “Ectocarpus: A model organism for the brown algae,” in Cold spring harbor protocols, cold spring harbor (NY: Cold Spring Harbor Laboratory Press).
Cotner, J. B., Biddanda, B. A. (2002). Small players, Large role microbial influence on biogeochemical processes in pelagic aquatic ecosystems. Ecosystems 5, 105–121. doi: 10.1007/s10021-001-0059-3
Croy, C. D. (1979). Phylogenetic and biogeographic inferences as drawn from reproductive isolation within the Chara aspera Deth. ex. Willd. complex (Charophyta). Tech University, Texas 1–53
Delaux, P.-M., Xie, X., Timme, R. E., Puech-Pages, V., Dunand, C., Lecompte, E., et al. (2012). Origin of strigolactones in the green lineage. New Phytologist. 195(4), 857–871 doi: 10.1111/j.1469-8137.2012.04209.x
De, Vries, Archibald, J. M. (2018). Plant evolution: landmarks on the path to terrestrial life. New Phytol. 217, 1428–1434. doi: 10.1111/nph.14975
De Vries, S., De Vries, J. (2018). “Azolla: A model system for symbiotic nitrogen fixation and evolutionary developmental biology,” in Current advances in fern research. (Cham: Springer International Publishing)
Ding, D. Q., Amino, S., Mimura, T., T., N., Tazawa, M. (1991). Intercellular transport and subcellular distribution of photoassimilates in Chara corallina. J. Exp. Bot. 42, 1393–1398. doi: 10.1093/jxb/42.11.1393
Ernst, A. (1917). Experimentelle erzeugung erblicher parthenogenesis. Z. für Induktive Abstammungs-und Vererbungslehre 17, 203–250.
Forsberg, C. (1965a). Axenic culture of Chara globularis thuill. and chara zeylanica willd. Life Sci. 4, 225–226. doi: 10.1016/0024-3205(65)90123-2
Forsberg, C. (1965b). Nutritional studies of Chara in axenic cultures. Physiologia Plantarum 18, 275–290. doi: 10.1111/j.1399-3054.1965.tb06890.x
Forsberg, C. (1965c). Sterile germination of oospores of Chara and seeds of Najas marina. Physiologlia Plantarum 18, 128–137. doi: 10.1111/j.1399-3054.1965.tb06875.x
Forsberg, C. (1966). Sterile germination requirements of seeds of some water plants. Physiologlia Plantarum 19, 1105–1109. doi: 10.1111/j.1399-3054.1966.tb07103.x
Franke, T., Doege, A. (2016). “Chara braunii” In: Arbeitsgruppe Characeen Deutschlands. Armleuchteralgen. Die Characeen Deutschlands. (Berlin: Springer Spektrum), 253–261
Gmelin, C. C. (1826). Flora badensis: alsatica et confinium regionum cis et transrhenana plantas a lacu Bodamico usque ad confluentem Mosellae et Rheni sponte nascentes exhibens secundum systema sexuale cum iconibus ad naturam dileneatis (officina Aul. Mülleriana). 4.
Gerrienne, P., Servais, T., Vecoli, M. (2016). Plant evolution and terrestrialization during Palaeozoic times the phylogenetic context. Rev. Palaeobotany Palynology 227, 4–18. doi: 10.1016/j.revpalbo.2016.01.004
Godlewski, M., Kwiatkowska, M. (1980). Effect of gibberellic acid on the formation and development of antheridia and oogonia in Chara vulgaris. Acta Societatis Botanicorum Poloniae, 49(4), 459–469. doi: 10.5586/asbp.1980.041
Gross, E. M., Hilt, S. N. K., Lombardo, P., Mulderij, G. (2007). Searching for allelopathic effects of submerged macrophytes on phytoplankton–state of the art and open questions. Hydrobiologia 584, 77–88. doi: 10.1007/978-1-4020-6399-2_8
Haas, J. N. (1994). First identification key for charophyte oospores from central Europe. Eur. J. Phycology 29, 227–235. doi: 10.1080/09670269400650681
Haas, F. B., Fernandez-Pozo, N., Meyberg, R., Perroud, P. F., Gottig, M., Stingl, N., et al. (2020). Single nucleotide polymorphism charting of p. patens reveals accumulation of somatic mutations during in vitro culture on the scale of natural variation by selfing. Front. Plant Sci. 11, 813. doi: 10.3389/fpls.2020.00813
Han, X., Chang, X., Zhang, Z., Chen, H., He, H., Zhong, B., et al. (2019). Origin and evolution of core components responsible for monitoring light environment changes during plant terrestrialization. Mol. Plant 12, 847–862. doi: 10.1016/j.molp.2019.04.006
Han, J. D., Li, X., Jiang, C. K., Wong, G. K., Rothfels, C. J., Rao, G. Y. (2017). Evolutionary analysis of the LAFL genes involved in the land plant seed maturation program. Front. Plant Sci. 8, 439. doi: 10.3389/fpls.2017.00439
Hedges, S. B. (2002). The origin and evolution of model organisms. Nat. Rev. Genet. 3, 838–849. doi: 10.1038/nrg929
Helcom Red List Macrophyte Expert Group (2013). Species information sheet Chara braunii. Available at: https://www.helcom.fi/wp-content/uploads/2019/08/HELCOM-Red-List-Chara-braunii.pdf.
Hilhorst, H. W. M. (1998). “The regulation of secondary dormancy. the membrane hypothesis revisited,” in Seed science research, vol. 8. , 77–90.
Hilhorst, H. W. M., Carssen, C. M. (2000). “Effect of chemical environment on seed germination,” in Seeds: the ecology of regeneration in plant communities, 2 ed. Ed. Fenner, M. (CABI Publishing, Wallingford, UK). 293–309
Holzhausen, A. (2016). Resilienz aquatischer Ökosysteme - Vitalitätsabschätzungen von Diasporenbanken, dissertation. (Rostock: University Rostock) doi: 10.18453/rosdok_id00002020.
Holzhausen, A., Porsche, C., Schubert, H. (2017). Viability assessment and estimation of the germination potential of charophyte oospores: testing for site and species specificity. Bot. Lett. 165, 147–158. doi: 10.1080/23818107.2017.1393460
Imahori, K., Iwasa, K. (1965). Pure culture and chemical regulation of the growth of charophytes. Phycologia 4, 127–134. doi: 10.2216/i0031-8884-4-3-127.1
Inoue, K., Nishihama, R., Kataoka, H., Hosaka, M., Manabe, R., Nomoto, M., et al. (2016). Phytochrome signaling is mediated by PHYTOCHROME INTERACTING FACTOR in the liverwort Marchantia polymorpha. Plant Cell 28, 1406–1421. doi: 10.1105/tpc.15.01063
Jiao, C., Sorensen, I., Sun, X., Sun, H., Behar, H., Alseekh, S., et al. (2020). The Penium margaritaceum Genome: Hallmarks of the origins of land plants. Cell 181 1097-1111, e12. doi: 10.1016/j.cell.2020.04.019
Karling, J. S. (1924). A preliminary account of the influence of light and temperature on growth and reproduction in Chara fragilis. Bull. Torrey Botanical Club 51, 469–488. doi: 10.2307/2480252
Kataržytė, M., Vaičiūtė, D., Bučas, M., Gyraitė, G., Petkuvienė, J. (2017). Microorganisms associated with charophytes under different salinity conditions. Oceanologia 59, 177–186. doi: 10.1016/j.oceano.2016.10.002
Kato, S., Sakayama, H., Sano, S., Kasai, F., Watanabe, M. M., Tanaka, J., et al. (2008). Morphological variation and intraspecific phylogeny of the ubiquitous species Chara braunii (Charales, Charophyceae) in Japan. Phycologia 47, 191–202. doi: 10.2216/07-27.1
Kawai, H., Hanyuda, T., Akita, S., Uwai, S. (2020). The macroalgal culture collection in Kobe university (KU-MACC) and a comprehensive molecular phylogeny of macroalgae based on the culture strains. Appl. Phycology, 1–8. doi: 10.1080/26388081.2020.1745685
Kolodziejek, J., Patykowski, J., Wala, M. (2017). Effect of light, gibberellic acid and nitrogen source on germination of eight taxa from dissapearing European temperate forest, Potentillo albae-Quercetum. Sci. Rep. 7, 13924. doi: 10.1038/s41598-017-13101-z
Korsch, H. (2010). Ergebnisse der Kartierung der Armleuchteralgen im Süden von Sachsen-Anhalt. 2. Beitrag. Mitt. florist. Kart. Sachsen-Anhalt 15, 135–139.
Krause, W. (1997). “Charales (Charophyceae),” in Süßwasserflora von Mitteleuropa. Eds. Ettl, H., Gärtner, G., Heynig, H., Mollenhauer, D. (Gustav Fischer Verlag, Stuttgart).
Kuczewski, O. (1906). “Morphologische und biologische untersuchungen and chara delicatula f. bulbilifera,” in Botanisches centralblatt, BH, vol. 20. , 25–76.
Küster, A., Schaible, R., Schubert, H. (2004). Light acclimation of photosynthesis in three charophyte species. Aquat. Bot. 79, 111–124. doi: 10.1016/j.aquabot.2004.01.010
Kwiatkowska, M., Godlewski, M. (1980). Effects of gibberellic acid and AMO-1618 on the development of vegetative systems in generatively matured thalli of chara vulgaris. Botanicorum Poloniae Acta Societatis 49, 445–458. doi: 10.5586/asbp.1980.040
Kwiatkowska, A., Zebrowski, J., Oklejewicz, B., Czarnik, J., Halibart-Puzio, J., Wnuk, M. (2014). The age-dependent epigenetic and physiological changes in an arabidopsis T87 cell suspension culture during long-term cultivation. Biochem. Biophys. Res. Commun. 447, 285–291. doi: 10.1016/j.bbrc.2014.03.141
Léger-Daigle, R., Noisette, F., Bélanger, S., Cusson, M., Nozais, C. (2022). Photoacclimation and light thresholds for cold temperate seagrasses. Front. Plant Sci. 13. doi: 10.3389/fpls.2022.805065
Li, D., Zhou, G., Gu, S., Zhang, T., Meng, L., Guo, Y., et al. (2020). Thermodynamic and dynamic modeling of the boron species in aqueous potassium borate solution. ACS Omega 5, 15835–15842. doi: 10.1021/acsomega.0c00773
Mahbub, M., Hemm, L., Yang, Y., Kaur, R., Carmen, H., Engl, C., et al. (2020). mRNA localization, reaction centre biogenesis and thylakoid membrane targeting in cyanobacteria. Nat. Plants 6, 1179–1191. doi: 10.1038/s41477-020-00764-2
Maszewski, J. (1980). The effect of light factor on the development of thallus and generative organs in Chara vulgaris l. Acta Societatis Botanicorum Poloniae 49, 397–407. doi: 10.5586/asbp.1980.035
Meyberg, R., Schwartzenberg, K. (2020). “General instructions on plant in vitro culture. V1.0,” in MadLand culture collection. Available at: https://data.uni-marburg.de/handle/dataumr/82.
Miryeganeh, M., Marletaz, F., Gavriouchkina, D., Saze, H. (2022). De novo genome assembly and in natura epigenomics reveal salinity-induced DNA methylation in the mangrove tree bruguiera gymnorhiza. New Phytol. 233, 2094–2110. doi: 10.1111/nph.17738
Nichols, H. W., Bold, H. C. (1965). Trichosarcina polymorpha gen et sp Nov. J. Phycol. 1, 34–38. doi: 10.1111/j.1529-8817.1965.tb04552.x
Nishiyama, T., Sakayama, H., De Vries, J., Buschmann, H., Saint-Marcoux, D., Ullrich, K. K., et al. (2018). The Chara genome: Secondary complexity and implications for plant terrestrialization. Cell 174, 448–464. doi: 10.1016/j.cell.2018.06.033
Okazaki, Y., Shimmen, T., Tazawa, M. (1984a). Turgor regulation in a brackish charophyte, Lamprothamnium succinctum i. artificial modification of intracellular osmotic pressure. Plant Cell Physiol. 25, 565–571. doi: 10.1093/oxfordjournals.pcp.a076746
Okazaki, Y., Shimmen, T., Tazawa, M. (1984b). Turgor regulation in a brackish charophyte, lamprothamnium succinctum II. changes in K+, Na+ and Cl- concentrations, membrane potential and membrane resistance during turgor regulation. Plant Cell Physiol. 25, 573–581. doi: 10.1093/oxfordjournals.pcp.a076747
One Thousand Plant Transcriptomes I (2019). One thousand plant transcriptomes and the phylogenomics of green plants. Nature 574, 679–685. doi: 10.1038/s41586-019-1693-2
Pinnel, N., Heege, T., Zimmermann, S. (2005). Spectral discrimination of submerged macrophytes in lakes using hyperspectral remote sensing data. SPIE Proceedings on Ocean Optics XVII. 1
Pott, R. (2005). “Allgemeine Geobotanik,” in Biogeosysteme und biodiversität (Berlin Heidelberg: Springer-Verlag Berlin Heidelberg). vol. 1.
Proctor, V. W. (1967). Storage and germination of Chara oospores. J. Phycology 3, 90–92. doi: 10.1111/j.1529-8817.1967.tb04638.x
Proctor, V. W. (1970). Taxonomy of Chara braunii - an experimental approach. J. Phycology 6, 317–321.
Provart, N. J., Brady, S. M., Parry, G., Schmitz, R. J., Queitsch, C., Bonetta, D., et al. (2021). Anno genominis XX: 20 years of Arabidopsis genomics. Plant Cell 33, 832–845. doi: 10.1093/plcell/koaa038
Raabe, U. (2017). Aktuelle nachweise von Chara braunii in der oberrheinischen Tiefebene bei Stockstadt (Hessen) und Iffezheim (Baden-Württemberg). Rostocker Meeresbiologische Beiträge 27, 37–42.
Rensing, S. A., Goffinet, B., Meyberg, R., Wu, S. Z., Bezanilla, M. (2020). The moss Physcomitrium patens (Physcomitrella) : A model organism for non-seed plants. Plant Cell 32, 1361–1376. doi: 10.1105/tpc.19.00828
Rensing, S. A., Lang, D., Zimmer, A. D., Terry, A., Salamov, A., Shapiro, H., et al. (2008). The Physcomitrella genome reveals evolutionary insights into the conquest of land by plants. Science 319, 64–67. doi: 10.1126/science.1150646
Rensing, S. A., Sheerin, D. J., Hiltbrunner, A. (2016). Phytochromes: More than meets the eye. Trends Plant Sci. 21, 543–546. doi: 10.1016/j.tplants.2016.05.009
Richter, J. (1894). Ueber reactionen der characeen auf äussere einflüsse. Eds. Druck, P., Höfling, V. V.
Rodrigo, M. A., Rojo, C., Álvarez-Cobelas, M., Cirujano, S. (2007). Chara hispida beds as a sink of nitrogen: Evidence from growth, nitrogen uptake and decomposition. Aquat. Bot. 87, 7–14. doi: 10.1016/j.aquabot.2007.01.007
Sabbatini, M. R., Argüello, J. A., Fernández, O. A., Bottini, R. A. (1987). Dormancy and growth-inhibitor levels in oospores of Chara contraria A. Braun ex Kütz. (Charophyta). Aquat. Bot. 28, 189–194. doi: 10.1016/0304-3770(87)90040-4
Sagert, S., Schubert, H. (1999). Unterwasserlichtklima der Darss-Zingster-Boddenkette. Rostocker Meeresbiologische Beiträge 7, 135–155.
Sakayama, H., Hara, Y., Nozaki, H. (2004). Taxonomic re-examination of six species of Nitella (Charales, charophyceae) from Asia, and phylogenetic relationships within the genus based on rbcL and atpB gene sequences. Phycologia 43, 91–104. doi: 10.2216/i0031-8884-43-1-91.1
Sato, M., Sakayama, H., Sato, M., Ito, M., Sekimoto, H. (2014). Characterization of sexual reproductive processes in Chara braunii (Charales, charophyceae). Phycological Res. 62, 214–221. doi: 10.1111/pre.12056
Schmidt, V. (2021). The role of auxin in streptophyte algae. master (Charles University Prague, Prague).
Schubert, H., Marquardt, R., Schories, D., Blindow, I. (2015). Biogeography of Chilean charophytes. Aquat. Bot. 120, 129–141. doi: 10.1016/j.aquabot.2014.06.005
Schubert, H., Blindow, I., Schories, D., Mages, M., von Tuempling, W., Woelfl, S. (2018). Biogeography of Chilean Charophytes – determined by climate or by water chemistry? Botany Letters 165, 129–145. doi: 10.1080/23818107.2017.1370612
Sederias, J., Colman, B. (2007). The interaction of light and low temperature on breaking the dormancy of Chara vulgaris oospores. Aquat. Bot. 87, 229–234. doi: 10.1016/j.aquabot.2007.06.008
Shimamura, M. (2016). Marchantia polymorpha: Taxonomy, phylogeny and morphology of a model system. Plant Cell Physiol. 57, 230–256. doi: 10.1093/pcp/pcv192
Sleith, R. S., Karol, K. G. (2021). Global high-throughput genotyping of organellar genomes reveals insights into the origin and spread of invasive starry stonewort (Nitellopsis obtusa). Biol. Invasions 23, 3471–3482. doi: 10.1007/s10530-021-02591-8
Smart, R. M., Barko, J. W. (1984). “Culture methodology for experimental investigations involving rooted submersed aquatic plants,” Aquatic plant control research program, Waterways Experiment Station, Vicksburg (Mississippi).
Sokol, R. C., Stross, R. G. (1986). Annual germination window in oospores of Nitella furcata (Charophyceae). J. Phycol. 22, 403–406. doi: 10.1111/j.1529-8817.1986.tb00044.x
Sokol, R. C., Stross, R. G. (1992). Phytochrome-mediated germination of very sensitive oospores. Plant Physiol. 100, 1132–1136. doi: 10.1104/pp.100.3.1132
Soulié-Märsche, I., García, A. (2015). Gyrogonites and oospores, complementary viewpoints to improve the study of the charophytes (Charales). Aquat. Bot. 120, 7–17. doi: 10.1016/j.aquabot.2014.06.003
Spear, D. G., Barr, J. K., Barr, C. E. (1969). Localization of hydrogen ion and chloride ion fluxes in Nitella. J. Gen. Physiol. 54, 397–414. doi: 10.1085/jgp.54.3.397
Stross, R. G. (1989). The temporal window of germination in oospores of Chara (Charophyceae) following primary dormancy in the laboratory. New Phytol. 113, 491–495. doi: 10.1111/j.1469-8137.1989.tb00360.x
Stross, R. G., Sokol, R. C., Schwarz, A.-M., Howard-Williams, C. (1995). Lake optics and depth limits for photogenesis and photosynthesis in charophyte meadows. Hydrobiologia 302, 11–19. doi: 10.1007/BF00006395
Szövényi, P., Frangedakis, E., Ricca, M., Quandt, D., Wicke, S., Langdale, J. A. (2015). Establishment of anthoceros agrestis as a model species for studying the biology of hornworts. BMC Plant Biol. 15, 98. doi: 10.1186/s12870-015-0481-x
Takatori, S., Imahori, K. (1971). Light reactions in the control of oospore germination of Chara delicatula. PHYCOLOGIA 10, 221–228. doi: 10.2216/i0031-8884-10-2-221.1
Talling, J. F. (2010). Potassium - a non-limiting nutrient in fresh waters? Fresh. Rev. 3 (2), 97–104. doi: 10.1608/FRJ-3.2.1
Tränkner, M., Tavakol, E., Jákli, B. (2018). Functioning of potassium and magnesium in photosynthesis, photosynthate translocation and photoprotection. Physiol. Plant 163, 414–431. doi: 10.1111/ppl.12747
Vosolsobĕ, S., Skokan, R., Petrasek, J. (2020). The evolutionary origins of auxin transport: what we know and what we need to know. J. Exp. Bot. 71, 3287–3295. doi: 10.1093/jxb/eraa169
Wang, S., Liang, H., Xu, Y., Li, L., Wang, H., Sahu, D. N., et al. (2021). Genome-wide analyses across viridiplantae reveal the origin and diversification of small RNA pathway-related genes. Commun. Biol. 4, 412. doi: 10.1038/s42003-021-01933-5
Wei, L., Zhang, D., Xiang, F., Zhang, Z. (2009). Differentially expressed miRNAs potentially involved in the regulation of defense mechanism to drought stress in maize seedlings. Int. J. Plant Sci. 170, 979–989. doi: 10.1086/605122
Wetzel, R. G., McGregor, D. L. (1968). Axenic culture and nutritional studies of aquatic macrophytes. Am. Midland Nat., 80, 52–64. doi: 10.2307/2423602
Wickett, N. J., Mirarab, S., Nguyen, N., Warnow, T., Carpenter, E., Matasci, N., et al. (2014). Phylotranscriptomic analysis of the origin and early diversification of land plants. Pnas, 111(45), e4859-e4868. doi: 10.1073/pnas.1323926111
WOOD, R. D. (1965). “Monograph of the characeae,” in A revision of the characeae. Ed. Wood, R.D., I. K. (Weinheim: J Cramer).
Wüstenberg, A., Pörs, Y., Ehwald, R. (2011). Culturing of stoneworts and submersed angiosperms with phosphate uptake exclusively from an artificial sediment. Freshw. Biol. 56, 1531–1539. doi: 10.1111/j.1365-2427.2011.02591.x
Zemach, A., Zilberman, D. (2010). Evolution of eukaryotic DNA methylation and the pursuit of safer sex. Curr. Biol. 20, R780–R785. doi: 10.1016/j.cub.2010.07.007
Zenker, A. (2003). Ökophysiologie von characeae. diploma (Ernst-Moritz-Arndt-University Greifswald).
Zhákova, L. V. (2003). “Chara braunii C.C. Gmel. 1826,” in Charophytes of the Baltic Sea. Eds. Schubert, H., Blindow, I. (Ruggel: A.R.G. Gantner).
Zhao, J. G., He, F. H., Chen, Z. H., Li, H. J., Hu, J. M., Lin, F. P. (2012). Effect of culture and extract solutions of macrophytes on the growth of three common algae. J. Freshw. Ecol. 27, 367–379. doi: 10.1080/02705060.2012.661936
Keywords: land plant evolution, Charophyceae, streptophyte algae, long-term cultivation, gibberellic acid, phytochrome effect, oospores
Citation: Holzhausen A, Stingl N, Rieth S, Kühn C, Schubert H and Rensing SA (2022) Establishment and optimization of a new model organism to study early land plant evolution: Germination, cultivation and oospore variation of Chara braunii Gmelin, 1826. Front. Plant Sci. 13:987741. doi: 10.3389/fpls.2022.987741
Received: 13 July 2022; Accepted: 17 October 2022;
Published: 10 November 2022.
Edited by:
Marek Mutwil, Max Planck Institute of Molecular Plant Physiology, GermanyReviewed by:
Eduardo Flores-Sandoval, Monash University, AustraliaJanine Fürst-Jansen, University of Göttingen, Germany
Copyright © 2022 Holzhausen, Stingl, Rieth, Kühn, Schubert and Rensing. This is an open-access article distributed under the terms of the Creative Commons Attribution License (CC BY). The use, distribution or reproduction in other forums is permitted, provided the original author(s) and the copyright owner(s) are credited and that the original publication in this journal is cited, in accordance with accepted academic practice. No use, distribution or reproduction is permitted which does not comply with these terms.
*Correspondence: Anja Holzhausen, YW5qYS5ob2x6aGF1c2VuQGJpb2xvZ2llLnVuaS1tYXJidXJnLmRl