- 1State Key Laboratory of Subtropical Silviculture, School of Forestry and Biotechnology, Zhejiang A&F University, Hangzhou, Zhejiang, China
- 2Department of Biotechnology, COMSATS University Islamabad, Abbottabad, Pakistan
Plants adjust their stomatal aperture for regulating CO2 uptake and transpiration. S-type anion channel SLAC1 (slow anion channel-associated 1) is required for stomatal closure in response to various stimuli such as abscisic acid, CO2, and light/dark transitions etc. Arabidopsis slac1 mutants exhibited defects in stimulus-induced stomatal closure, reduced sensitivity to darkness, and faster water loss from detached leaves. The global transcriptomic response of a plant with defective stimuli-induced stomatal closure (particularly because of defects in SLAC1) remains to be explored. In the current research we attempted to address the same biological question by comparing the global transcriptomic changes in Arabidopsis slac1-3 mutant and wild-type (WT) under dark, and dehydration stress, using RNA-sequencing. Abscisic acid (ABA)- and dark-induced stomatal closure was defective in Arabidopsis slac1-3 mutants, consequently the mutants had cooler leaf temperature than WT. Next, we determined the transcriptomic response of the slac1-3 mutant and WT under dark and dehydration stress. Under dehydration stress, the molecular response of slac1-3 mutant was clearly distinct from WT; the number of differentially expressed genes (DEGs) was significantly higher in mutant than WT. Dehydration induced DEGs in mutant were related to hormone signaling pathways, and biotic and abiotic stress response. Although, overall number of DEGs in both genotypes was not different under dark, however, the expression pattern was very much distinct; whereas majority of DEGs in WT were found to be downregulated, in slac1-3 majority were upregulated under dark. Further, a set 262 DEGs was identified with opposite expression pattern between WT and mutant under light–darkness transition. Amongst these, DEGs belonging to stress hormone pathways, and biotic and abiotic stress response were over-represented. To sum up, we have reported gene expression reprogramming underlying slac1-3 mutation and resultantly defective stomatal closure in Arabidopsis. Moreover, the induction of biotic and abiotic response in mutant under dehydration and darkness could be suggestive of the role of stomata as a switch in triggering these responses. To summarize, the data presented here provides useful insights into the gene expression reprogramming underlying slac1-3 mutation and resultant defects in stomatal closure.
Introduction
Stomatal pores are surrounded by a pair of guard cells. The emergence of latter is considered as a major landmark in the land plants’ evolution and adaptation to harsh environmental conditions (Umezawa et al., 2010). Stomata open and close in response to diverse stimuli and are involved in efficiently taking up CO2 for photosynthesis and regulating water loss through transpiration (Jezek and Blatt, 2017).
The opening and closing of the stomatal aperture are brought about by changes in turgor pressure through ion and water channel proteins present in the guard cell plasma membrane (Schroeder et al., 2001; Kim et al., 2010; Engineer et al., 2016). Stomatal movements are tightly regulated under the influence of environmental stimuli and endogenous factors, such as light, water status, carbon dioxide (CO2), ABA, calcium (Ca2+), and reactive oxygen species (ROS; Schroeder et al., 2001; Young et al., 2006).
It is now well established that light-induced stomatal opening is regulated by plasma membrane inward-rectifying K+ channels while stomatal closure involves outward K+ channels and anion channels. When plants are challenged with stressful conditions, the activation of guard cell anion channels becomes a key step in inducing stomatal closure. Different signaling components including second messengers, phospholipases, protein kinases, and phosphatases are involved in regulation of stomatal movements. Moreover, stomatal opening and closure are also governed by changes in gene expression.
Abscisic acid- and drought stress induce anion efflux through anion channels, causing membrane depolarization in guard cells. One of the main guard cell anion channels SLAC1 (slow anion channel-associated 1) was identified as an S-type anion channel during the screenings for ozone-sensitive and CO2 insensitive mutants (Negi et al., 2008; Vahisalu et al., 2008). SLAC1 is required for stomatal closure in response to various stimuli such as abscisic acid, CO2, light/dark transitions, ozone, Ca2+ ions, humidity change, nitric oxide, and hydrogen peroxide. Localized in plasma membrane of guard cells, SLAC1 constitutes a trimer in which each subunit forms a channel pore independently (Chen et al., 2010). SLAC1 is a highly conserved S-type anion channel in diverse plant species. SLAC1 homologues have been identified in various species ranging from green algae to higher plants (Hedrich and Geiger, 2017; Ren et al., 2021). Rice slac1 mutants showed higher stomatal conductance and photosynthetic rate under well-watered conditions (Kusumi et al., 2012). In maize, ZmSLAC1 is involved in stomatal closure mainly by mediating the nitrate efflux (Qi et al., 2018).GhSLAC1 is an essential element for stomatal closure in response to drought in cotton (Ren et al., 2021).In Arabidopsis, five members of SLAC/SLAH family have been reported. Among those, AtSLAC1 and AtSLAH3 are responsible for chloride and nitrate efflux across guard cell plasma membrane (Negi et al., 2008; Vahisalu et al., 2008; Meyer et al., 2010; Qi et al., 2018).
Drought stress triggers increase in ABA which is an important stimulus for SLAC1 activation. After being bound by ABA, PYR/PYL/RCAR receptors interact with PP2C phosphatases and, thereby, previously inhibited Open stomata 1 (OST1) kinase is released which, through phosphorylation, activates SLAC1 channel (Murata et al., 2001; Mustilli et al., 2002; Yoshida et al., 2006; Geiger et al., 2009; Lee et al., 2009; Nishimura et al., 2010; Brandt et al., 2012). This leads to anion efflux from guard cells and subsequent stomatal closure. Besides OST1, other kinases like Leucine-rich repeat kinase (LRR-RLK), Calcium-dependent protein kinases (CPK/CDPK), Mitogen-activated protein kinase (MPK), and Calcineurin-B like protein (CBL)-CBL-interacting protein kinase (CIPK) are also involved in SLAC1 regulation (Mori et al., 2006; Cheong et al., 2007; Jammes et al., 2009; Geiger et al., 2010; Brandt et al., 2012; Scherzer et al., 2012; Maierhofer et al., 2014; Khokon et al., 2015; Prodhan et al., 2018). Moreover, few other stimuli like salicylic acid (SA), methy1 jasmonate (MeJA), and bacterial invasion also trigger stomatal closure via SLAC1 (Melotto et al., 2008; Khokon et al., 2011; Yan et al., 2015). In the light of above-cited literature, SLAC1 seems to be a converging point for different stomata closure pathways.
In Arabidopsis, loss-of-function mutations in SLAC1 result in strong impairment of S-type anion channel activity and inhibition of stimulus-induced stomatal closure (Negi et al., 2008; Vahisalu et al., 2008). The slac1 mutant exhibited reduced sensitivity to darkness. Moreover, higher CO2-induced increase in leaf temperature was also inhibited in slac1-2 mutant. The detached leaves of slac1 mutants lost water significantly faster than WT, pointing to defects in transpiration regulation under drought stress (Negi et al., 2008; Vahisalu et al., 2008). Mutations in slac1 also unexpectedly affected the stomatal opening induced by light, low CO2, and higher air humidity. This phenotype was found to be associated with higher concentration of resting cytosolic Ca2+ and drastically low activity of inward K+ channels in slac1 guard cells (Laanemets et al., 2013).
Although handful literature is available on SLAC1 regulation mechanism, however, the effects of slac1 mutation, and resultant defects in stomatal closure, on global gene expression need to be explored yet. In current study, we have attempted to answer this question by determining global transcriptomic changes in Arabidopsis slac1-3 mutant under dark and dehydration stress, by using RNA-sequencing.
Materials and methods
Plant materials and growth conditions
The wild-type (WT) Arabidopsis Columbia ecotype was used for the experiments unless otherwise indicated. In all the experiments Arabidopsis slac1-3 mutant was used. Seeds were surface sterilized with Plant Preservative Mixture (PPM) by keeping at 4°C for 2 days. The seeds were then placed on 1/2 strength Murashige and Skoog (MS) medium (pH 5.7–5.8), containing 1% sucrose and 0.8% agar, and the plates were kept in incubator at 22°C with 12:12 h, light:dark cycle settings for 2 weeks. The seedlings were then transplanted into the soil. Plants were grown at 12:12 h, light:dark, 23:18°C, and 60:70% humidity cycle.
Plant treatments for RNA sequencing experiment
The aboveground part of WT and mutant plants was cut out at 2 pm (Beijing time) for control. For dark treatment, the material was collected after 2 h of darkness in the greenhouse. For dehydration stress, the aboveground part of the plant was cut off and harvested after 25 min of water loss under natural conditions, as previously reported (Zotova et al., 2018). Samples were immediately frozen in liquid nitrogen and stored at −80°C and then subjected to Illumina sequencing. The experiment was performed in three biological replicates with each replicate consisting of three seedlings.
Identification of differentially expressed genes and functional enrichment analysis
Bioinformatic analysis was performed using the OmicStudio tools at https://www.omicstudio.cn/tool. The DEGs were identified based on |log2 fold-change| ≥ 1 and value of p < 0.05. The genes with expression level greater than 5 were retained. Venn and GO enrichment analysis were also performed using OmicShare Tools.1 The heatmaps were drawn by TBtools (Chen et al., 2020).
Stomatal movement experiment
Fresh plant leaves from 3 weeks old plants were collected at about 2 pm (Beijing time) and placed in stomatal opening buffer (50 μM CaCl2, 5 mM KCl, 10 mM MES-Tris, and pH 6.15). The leaves were soaked in buffer with the back side up and exposed to light (200–250 μmol m−2 s−1) for 2 h to fully open their stomata. Then, the lower epidermis of the leaves was peeled off to make temporary pieces. These were then observed under Zeiss microscope and pictures were taken (as control). For dark treatment, the samples were collected after shading the plants for 2 h. For ABA treatment, 200 μM ABA was sprayed on the plant leaves and the samples of lower epidermis were collected after 25 min. Three replicates were included for each treatment, and at least 30 stomata were measured in each group. The width of stomatal aperture was measured from the images with the help of ImageJ.
Thermal imaging experiment
The plants (Col-0 and slac1-3 mutants) were grown for 6 weeks under normal conditions. For dark treatment, the plants were grown without light for 30 min. Plants grown under normal light conditions were used as controls. The aboveground part of the plants was cut and placed on white paper for taking pictures immediately. Three seedlings were observed under each condition. The images were captured with HIKVISION H10 thermal Imager.
Results
The dark- and ABA-induced stomatal closure was defective in Arabidopsis slac1-3 mutants
We determined the response of stomata to ABA and dark in epidermal peels of the WT and slac1-3 mutants of Arabidopsis thaliana. Under normal conditions, the stomatal aperture of slac1-3 mutant was slightly larger than that of WT (Figures 1A,B). When treated with ABA or dark, the stomatal aperture in WT significantly decreased; being about 50% of that of untreated plants. In contrast to this, under ABA treatment, stomatal aperture in slac1-3 mutant was not significantly different than its untreated control, showing a nearly complete insensitivity to ABA. When shifted from light to dark, although the stomatal aperture in slac1-3 mutants decreased compared with its non-treated counterpart, however, the extent of stomatal closure was still significantly less than dark-treated WT. These data demonstrate that ABA- and dark-induced stomatal closure is defective in slac1-3 mutant.
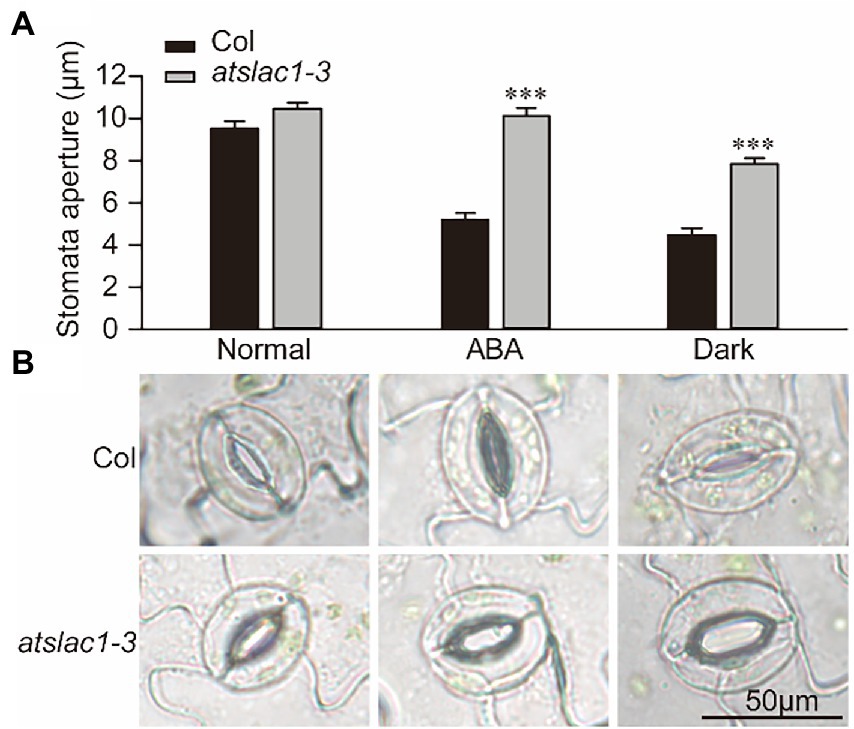
Figure 1. Arabidopsis slac1-3 mutants are insensitive to abscisic acid (ABA) and darkness. (A) Graphical presentation of stomatal aperture under ABA, dark treatment, or light. (B) Representative images of stomatal aperture in WT and slac1-3 mutants under ABA, dark, or light. Three replicates were included for each treatment, and at least 30 stomata were measured in each group. Bars represent mean ± S.E. *** Denotes statistically significant difference (t-test p < 0.01).
Arabidopsis slac1-3 mutation resulted in lower leaf temperature
After establishing the defects in stimuli-induced stomatal closure, next, we aimed to confirm if this phenotype had any effect on leaf temperature, by using thermal imaging technique. Under light, slac1-3 mutants showed lower leaf temperature compared with WT (Figure 2). After the dark treatment for 30 min, whereas the temperature of WT leaves clearly increased, the same was only slightly increased in mutants compared with its untreated counterpart (Figure 2). These data confirmed the defective dark-induced stomatal closure in slac1-3 mutants.
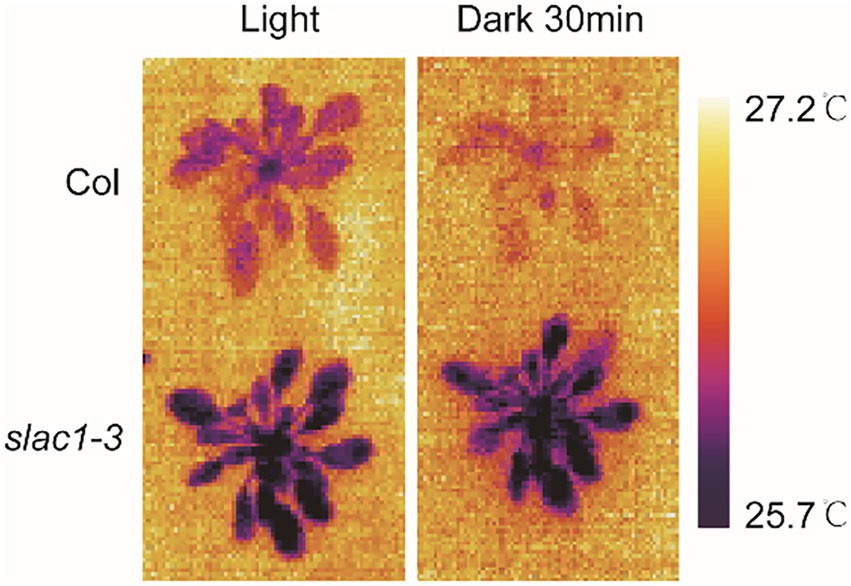
Figure 2. Determination of plant leaf temperature in Arabidopsis WT and slac1-3 mutants in light and dark conditions. Multiple images of both genotypes under light/dark conditions were captured with thermal Imager. The representative images are shown here.
Transcriptome profiling identified 891 genes upregulated only in Arabidopsis slac1-3 mutant under dehydration stress
Next, we determined the molecular response of slac1-3 mutant and WT in dehydration stress condition by carrying out global gene expression using RNA-sequencing. When exposed to dehydration for 25 min, slac1-3 mutant showed highly variable response, compared to WT, with respect to changes in gene expression. Overall, the number of differentially expressed genes (DEGs; |log2 fold-change| ≥ 1 and value of p < 0.05) was much higher in slac1-3 mutants than WT (1,956 vs. 1,156; Figure 3A). Interestingly, though the number of downregulated genes was not much different between mutant and WT (389 and 353), however, that of upregulated genes was much greater in slac1-3 mutants than in WT (1,567 vs. 803). Of these, 891 genes were only upregulated in slac1-3 mutants but not in WT in dehydration stress (Figure 3A).
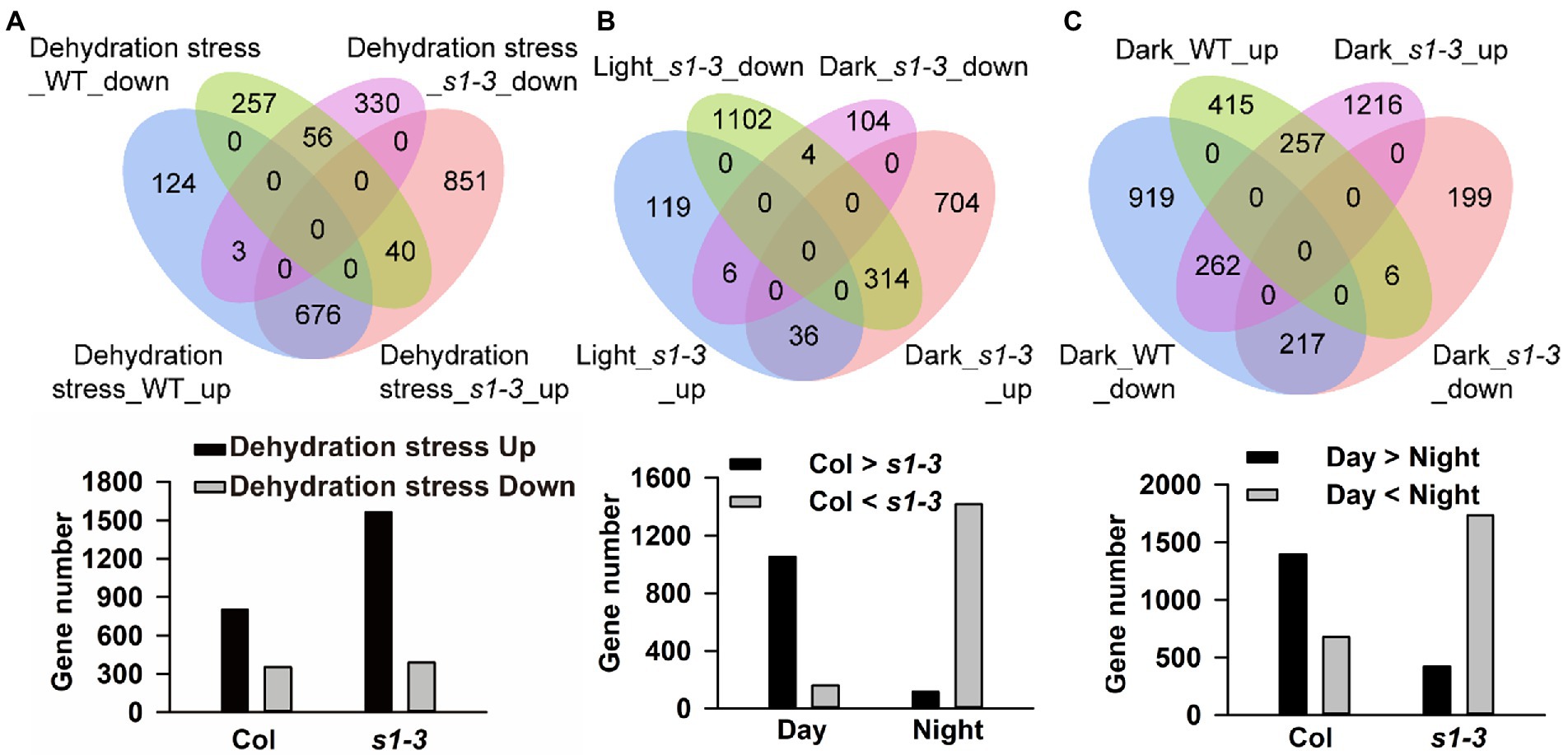
Figure 3. Differentially expressed gene (DEG) integration of each data set. DEGs of each data set are overlapped and presented as a Venn plot, including total, upregulated, and downregulated genes. DEGs, differently expressed genes. (A) Venn diagram of gene expression and gene number of up- or downregulated in dehydration stress; (B) up- or downregulated in dark conditions; and (C) up- or downregulated between WT and slac1-3 mutant.
A heat map, representing the above mentioned 891 genes, was created based on FPKM of RNA-sequencing data to visualize the quantitative differences in the expression of these genes in slac1-3 mutants under control and dehydration stress (Figure 4A). The level of expression of DEGs was divided into various classifications in the bar. The red and green regions are indicative of high and low expression, respectively. A color change from red to green, showing that the values of log10 (FPKM+1) change from high to low. Based on GO enrichment analysis the genes were classified into 30 functional categories (Figure 4B). The highest number of DEGs was related to plasma membrane function, followed by the genes related to plant hypersensitive response, response of chitin, defense response to fungus, protein targeting to membrane, response to water deficiency, and systemic acquired resistance (SAR). Based on enrichment factor, the major pathways significantly enriched in DEGs were cell communication pathway, innate immune response pathway, and salicylic acid biosynthesis and signaling pathways. To sum up, these data reveal the specific transcriptomic changes undertaken by the slac1-3 mutant under dehydration stress to cope up with defective stomatal closure.
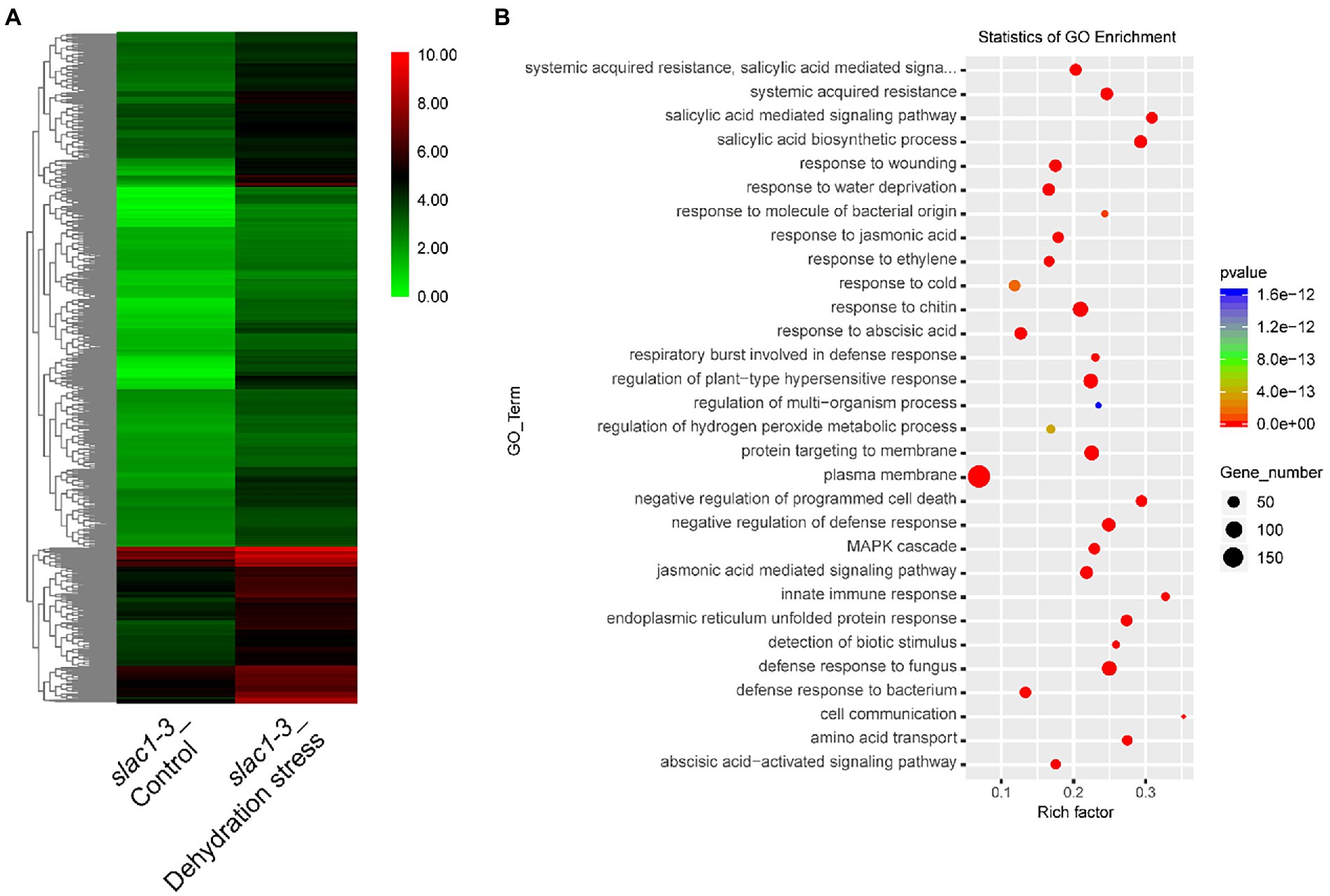
Figure 4. RNA-seq analysis of dehydration stress induced upregulated genes in slac1-3 mutant. (A) Heat map of DEGs (891 in Figure 3A) in slac1-3 mutants between control and dehydration stress (B) GO enrichment analysis of DEGs in slac1-3 mutants in control and dehydration stress.
Transcriptomic response of slac1-3 mutant was variable than WT under light as well as dark
To determine the molecular response of two genotypes under day-night transition, we set to compare the pattern of gene expression in WT and mutants under light and dark. Under light conditions, 1,581 DEGs (161 upregulated and 1,420 downregulated) were identified between slac1-3 mutant and WT (Figure 3B). On the other hand, 1,168 DEGs (1,054 upregulated and 114 downregulated) were identified between mutant and WT under dark conditions (Figure 3B). These findings point to a highly variable response of slac1-3 mutant as compared with WT, under light as well as dark.
Further, we identified 314 DEGs which, compared with WT, had higher expression in mutant under dark but lower expression under light (Figure 3B).
Next, we set to get further insight into the transcriptome profiling of both genotypes under dark. The number of upregulated and downregulated genes was 678 and 1,398, respectively, in WT while it was 1,735 and 422, respectively, in slac1-3 mutant under dark (Figure 3C). These data highlight the contrasting pattern of gene expression in the genotypes; under dark, whereas majority of DEGs in WT were found to be downregulated, in slac1-3 majority were upregulated. Further, 262 DEGs were identified which were downregulated in WT but upregulated in mutant under dark. Since the dark-induced stomatal closure was defective in slac1-3 mutants, the higher number of upregulated DEGs could represent a compensatory response of the mutant.
We created a heat map of 314 DEGs shown in Figure 3B, to show the quantitative differences in the DEGs (Figure 5A). At daytime, these DEGs were downregulated in slac1-3 mutant while, under dark, these were upregulated in the mutant, compared with WT (Figure 5A).
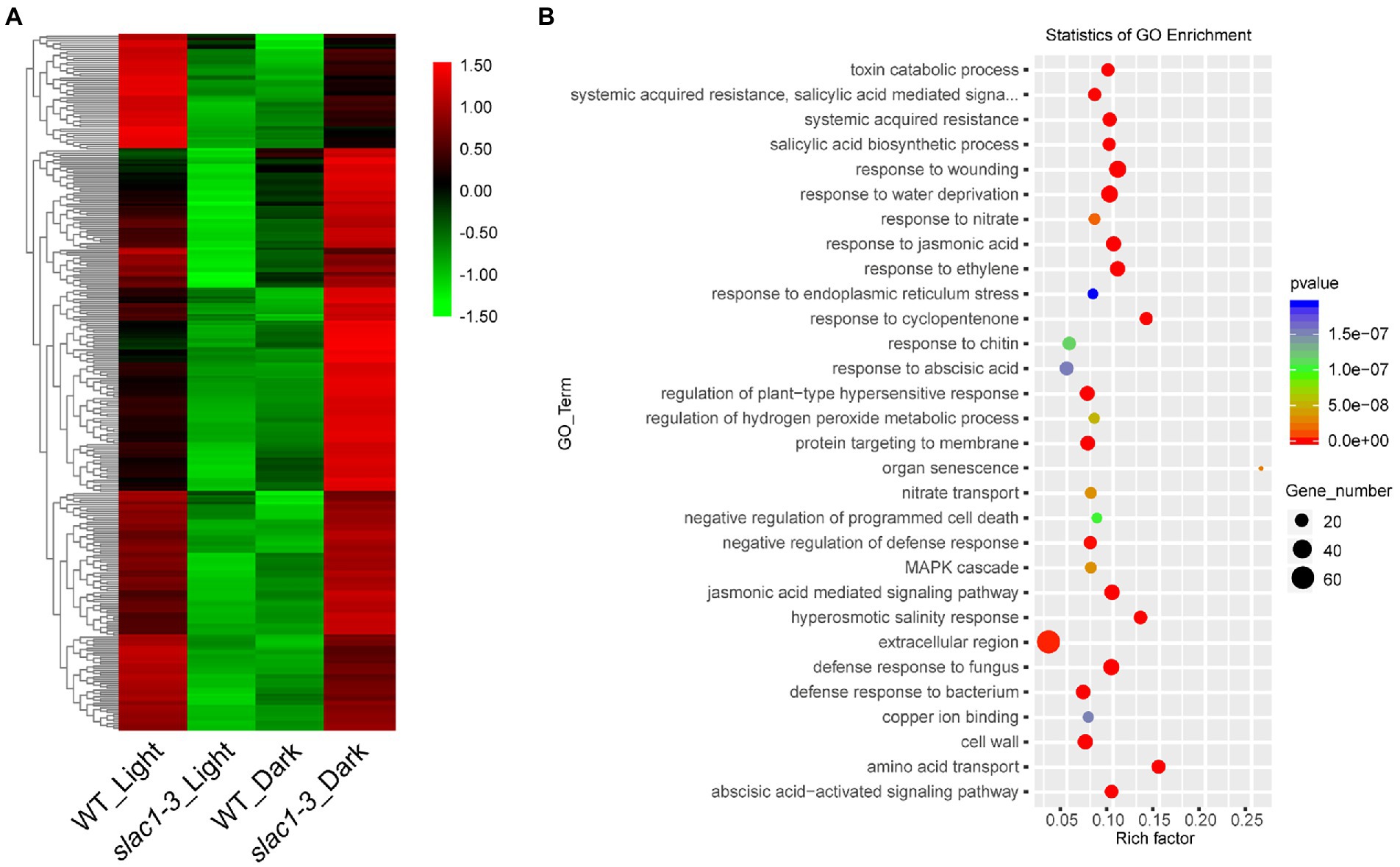
Figure 5. RNA-seq analysis of DEGs (314 in Figure 3B) at daytime or night. (A) Heat map of DEGs in slac1-3 mutants in dark and light conditions (B) GO enrichment analysis of DEGs in slac1-3 mutants in dark and light conditions.
GO enrichment analysis was performed, and the genes were assorted into 30 functional categories (Figure 5B). The highest number of DEGs was related to extracellular region, wounding response, dehydration stress response, jasmonic acid and ethylene signaling pathways, and defense response to fungus. The major pathways significantly enriched with DEGs included the one associated with organ senescence, amino acid transport, hyperosmotic salinity response, and response to cyclopentenone.
Next, we focused on the 262 DEGs (shown in Figure 3C) with opposite expression pattern in day/night between WT/mutant and developed a heatmap to represent quantitative differences in expression pattern (Figure 6A). These DEGs exhibited downregulation in WT but upregulation in slac1-3 mutant under dark while in light these DEGs were up-regulated in WT but down-regulated in slac1-3. The DEGs were further subjected to GO enrichment analysis, and the genes were assigned a particular functional category (Figure 6B). Among these, DEGs belonging to hormonal response (jasmonic acid, ethylene, and abscisic acid), wounding response, defense to fungal disease, salt stress response, and jasmonic acid signaling pathway were over-represented. The major pathways significantly enriched with DEGs were related to hyperosmotic salinity response, and responses to cyclopentenone, jasmonic acid, ethylene, and fungus infection.
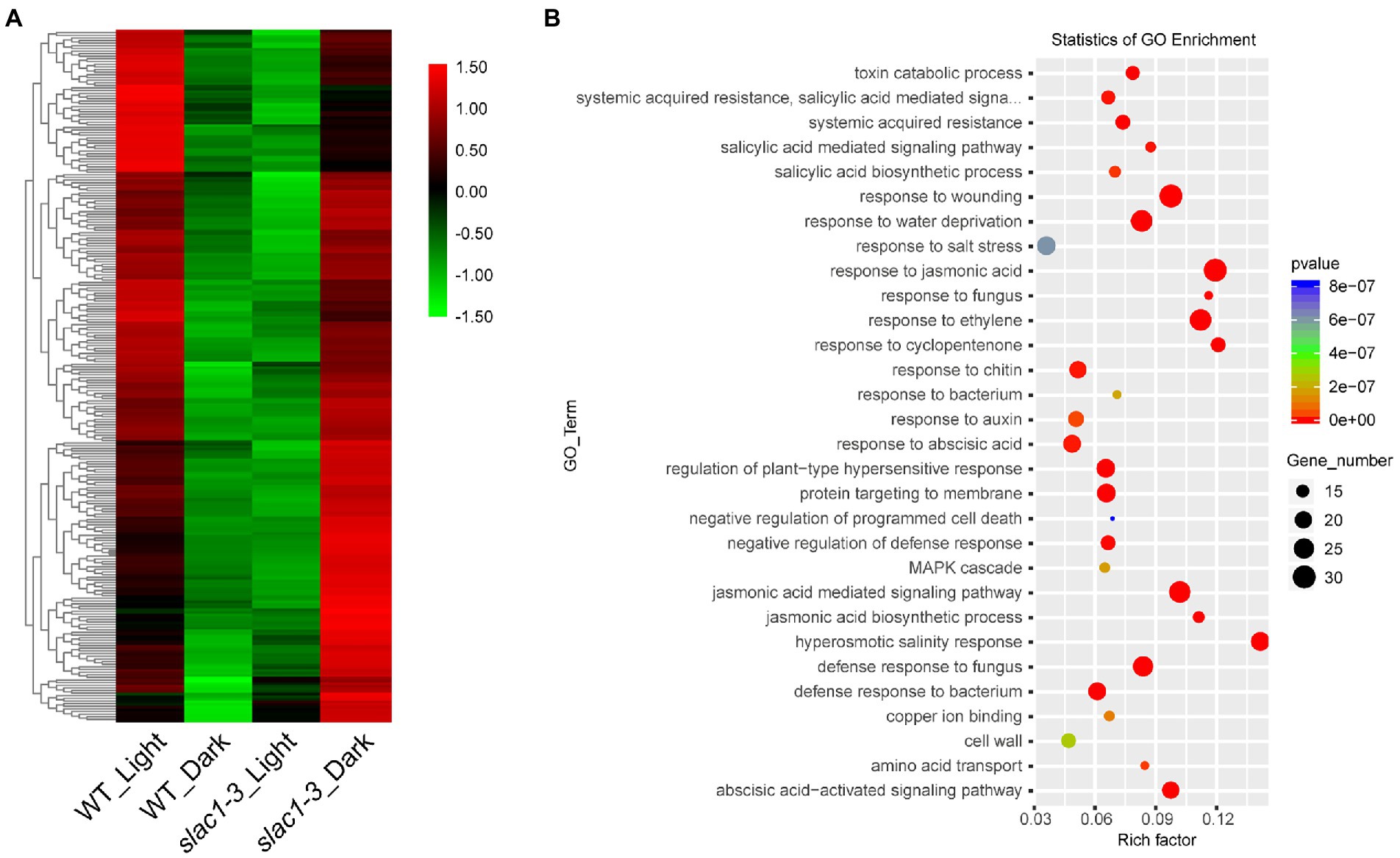
Figure 6. RNA-seq analysis of DEGs (262 in Figure 3C) between day and night. (A) Heat map of DEGs from day to night between slac1-3 mutant and WT. (B) GO enrichment analysis of DEGs from day to night between slac1-3 mutant and WT.
Analysis of DEGs under light, dark, and dehydration stress
We further analyzed the DEGs shown in Figures 3A–C. The focus was on 40 DEGs upregulated in slac1-3 mutant under dehydration stress but downregulated in WT (Figure 3A), 314 DEGs downregulated in slac1-3 mutant under light, but upregulated in the mutant under dark, and 262 DEGs (shown in Figure 3C) with opposite expression pattern in day/night between WT/mutant. Table 1 shows a list of 18 unique DEGs with opposite expression pattern in slac1-3 mutant and WT, under the above-mentioned conditions. These DEGs include drought-induced 21 (DI21), senescence related gene (SRG1), nitrilase 2 (NIT2), Kunitz trypsin inhibitor 1, plant invertase/pectin methyl esterase inhibitor superfamily, and lactoylglutathione lyase/glyoxalase I family protein.
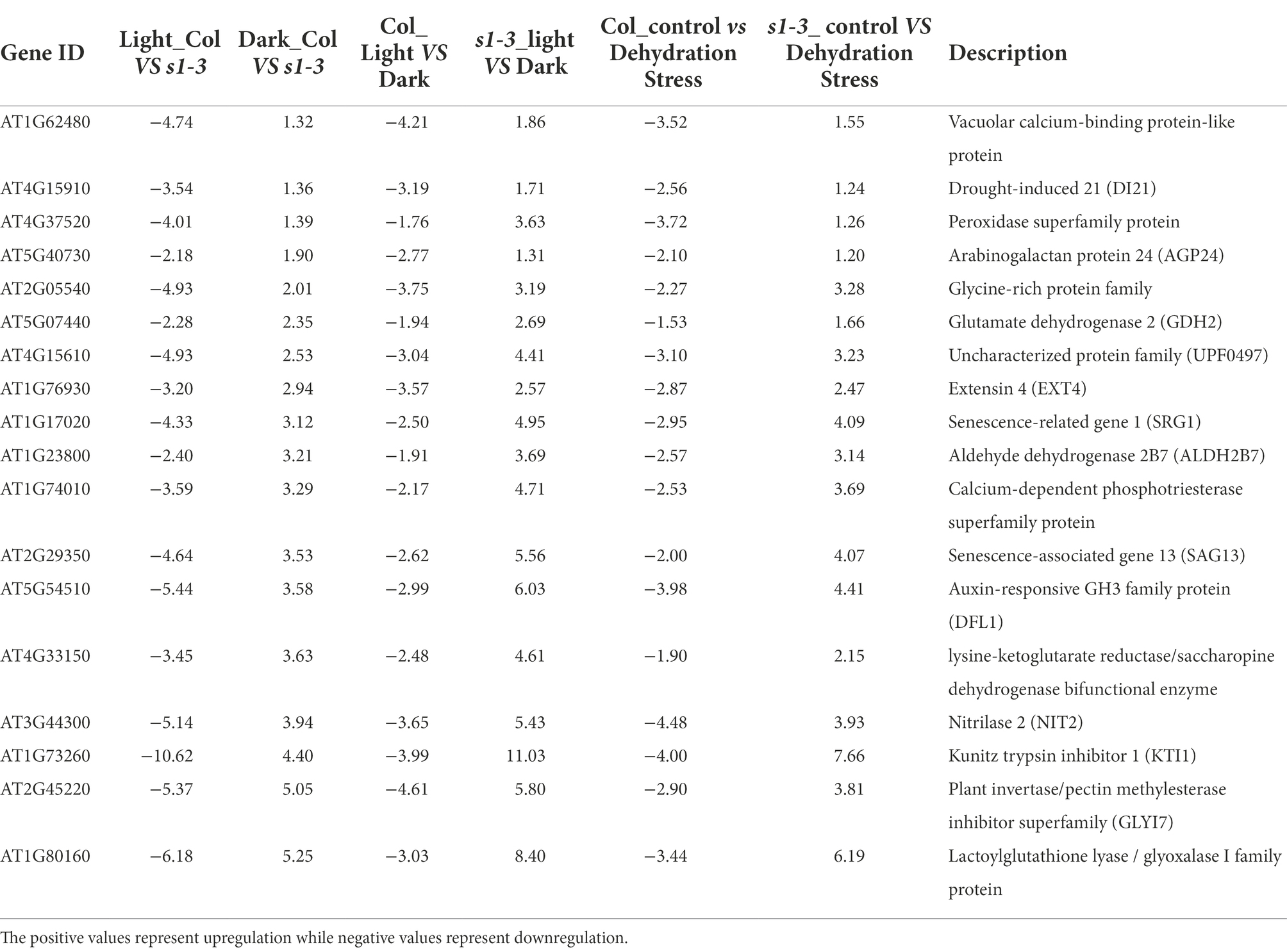
Table 1. List of 18 DEGs common between dehydration stress (40, Figure 3A), slac1-3 mutant (314, Figure 3B), and day and night (262, Figure 3C) showing opposite expression pattern in WT and mutant under different conditions.
Furthermore, in slac1-3 mutants, six DEGs were identified showing higher expression in WT (and lower expression in slac1-3 mutant) under light but lower expression in WT (and higher expression in slac1-3 mutant) under dark (Table 2). These included RING/FYVE/PHD zinc finger superfamily protein, three hypothetical proteins, and a transmembrane protein.
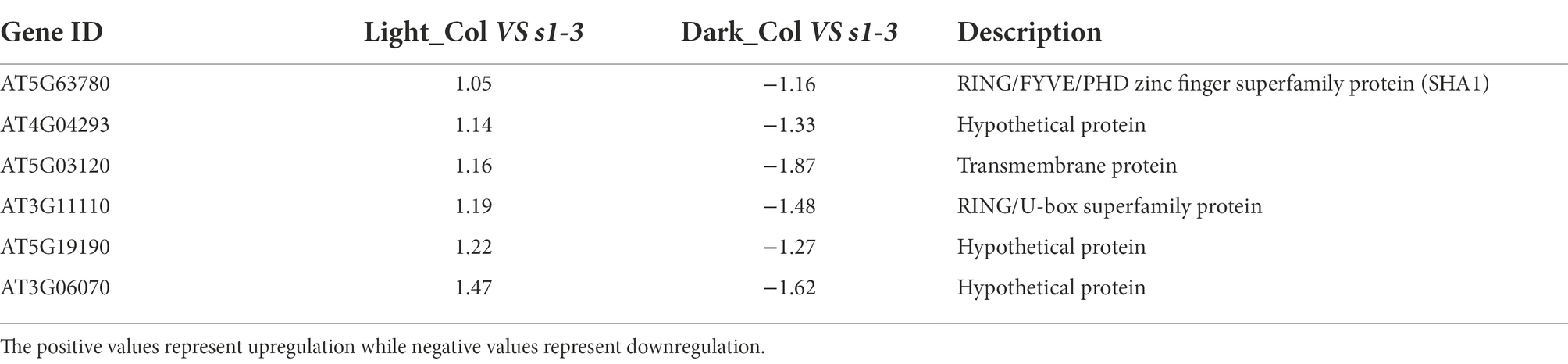
Table 2. List of six DEGs (Figure 3B) showing higher expression in WT (and lower expression in slac1-3 mutant) under light but lower expression in WT (and higher expression in slac1-3 mutant) under dark.
As shown in Table 3, six DEGs were identified with opposite pattern of expression in WT and mutant under light–dark transition. These include an ovate family protein 16, a HXXXD-type acyl-transferase family protein, and a myo-inositol oxygenase 1 protein. We also identified three DEGs which, under dehydration stress, were upregulated in slac1-3 mutant but downregulated in WT. One of these proteins was phloem protein 2-A13 while the other was nine-cis-epoxycarotenoid dioxygenase 4 (NCED4; Table 4).
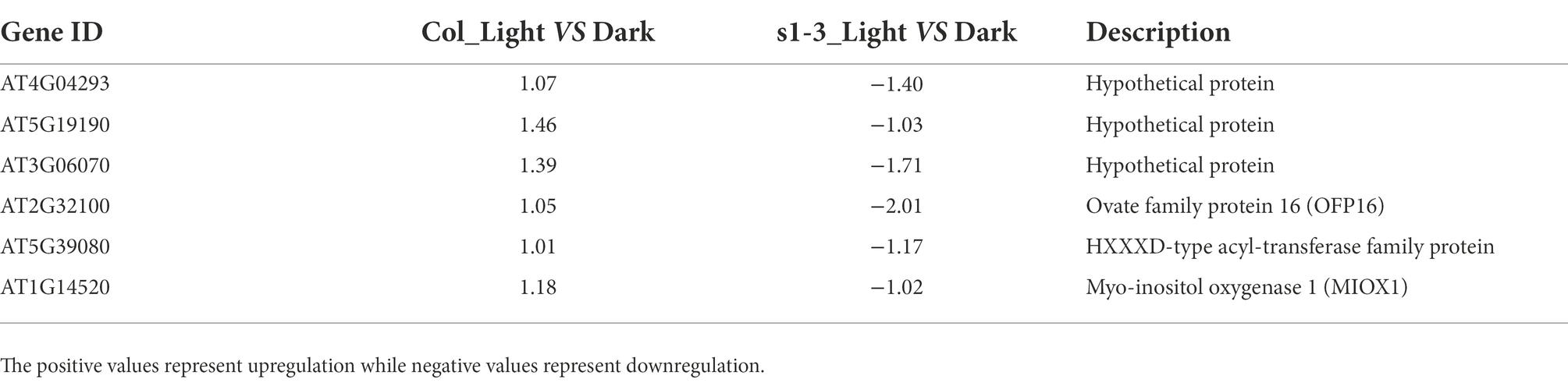
Table 3. List of six DEGs (Figure 3C) with opposite expression pattern in mutant and WT in light–dark transition (in light upregulation in WT but in dark upregulation in slac1-3 mutant).

Table 4. List of three DEGs (Figure 3A) with opposite expression pattern in mutant and WT (downregulated in WT but upregulated in slac1-3 mutant under dehydration stress).
Discussion
Owing to their central role in regulating CO2 uptake and transpiration in plants, changes in stomatal opening and development exhibit adaptive relationships to the environmental conditions in which the plant is growing (Richardson et al., 2017). Being sessile in nature, plants adjust their physiological processes like stomatal movements in response to external factors. The guard cells perceive and respond to various environmental cues to optimize the gas exchange, and at the same time avoiding drought stress (Pillitteri and Torii, 2012). Being a central player in mediating stimuli-induced stomatal closure, SLAC1 seems to be a converging point for different stomata closure pathways.
The regulation of stomatal movements could also be governed through transcriptional changes (Sirichandra et al., 2009) as demonstrated by the fact that the transcriptional inhibitors negatively affected stomatal opening, and that two RNA-binding proteins were involved in ABA-induced stomatal closure (Hugouvieux et al., 2001; Li et al., 2002). Furthermore, studies focusing on ABA-induced transcriptomic changes in the guard cell have led to the identification of transcription factors (TFs) involved in regulating the stomatal movements. The TFs involved in stomatal closure include AtMYB15, AtMYB44, AtMYB61, and SNAC1 etc. (Cominelli et al., 2010). Hence it seems that gene expression also plays important role in stomatal movements.
It is evident that failure in proper stomatal closure and/or opening could lead to changes in gene expression. We identified many differentially modulated genes between slac1-3 mutant and WT under different stimuli like light, dark, and dehydration stress (Figures 3A–C), showing that mutation in SLAC1 results in larger rearrangements at transcriptome level. So, what could be the link between SLAC1 disruption and alterations in gene expression? The failure of stomata to close normally results in water loss, changes in leaf temperature, osmotic changes, and pathogen invasion etc. All these factors can affect the expression of many genes. Moreover, the altered gene expression could be plant’s compensatory response due the failure in properly opening and closing the stomatal pore. It is already known that aberrations in stomatal movements lead to compensatory alterations in gene expression, or reorganization of the signaling pathways to compensate for the WT function of the gene (Webb and Baker, 2002).
It is well established that stomatal movements affect the transpiration rate which results in changes in osmotic potential and leaf temperature (Kaňa and Vass, 2008). Similarly, stomatal movements induced by day-night transition also result in changes in osmotic potential and temperature. As shown in Figure 2, the leaves of slac1-3 mutants were cooler than WT in dark, which could have led to differences in the expression pattern of genes between WT and the mutant. These data showed that stomatal switch could be linked to changes in gene expression. Moreover, it has been demonstrated that guard cells of slac1-3 mutants exhibit higher resting Ca2+ level (Laanemets et al., 2013). As Ca2+ is a ubiquitous signaling molecule, therefore, perturbations in Ca2+ level could also be associated with differential gene expression in slac1-3 mutants.
When challenged with drought stress, plants adopt few urgent measures alongside few long-term responses. The former, known as drought avoidance response, includes immediate stomatal closure to conserve water (Dobra et al., 2010) while the long-term response includes induction of drought stress responsive genes. As demonstrated in our study, dehydration stress-induced stomatal closure was defective in slac1-3 mutants (Figure 1) and therefore, these mutants lost more water than WT from detached leaves. As the extent of dehydration stress was more pronounced in mutants, therefore, a significantly higher number of genes were upregulated in dehydration stress only in the mutant but not in WT, possibly to initiate compensatory responses.
We identified few DEGs in mutant which could represent such compensatory mechanisms. For example, a gene encoding for nine-cis-epoxycarotenoid dioxygenase 4 (NCED4) was about 2.5-fold upregulated in slac1-3 mutant under dehydration stress (Table 4). NCED is involved in de novo ABA synthesis and catalyzes the first committed step in synthesizing ABA by converting 9-cis-neoxanthin to xanthoxin (Tan et al., 1997; Huang et al., 2018). It was observed that detached leaves of slac1 mutants lost water significantly faster than WT, showing defects in transpiration regulation under drought stress (Negi et al., 2008; Vahisalu et al., 2008). Since the mutant is unable to properly close stomata under dark and dehydration stress, the upregulation of NCED could reflect a strategy by slac1-3 mutant to promote ABA biosynthesis and thus induce stomatal closure to avoid water loss. Similarly, another gene encoding a member of ovate family had two-fold higher expression in slac1-3 mutants than WT (Table 3). Ovate family proteins (OFPs) are plant-specific transcription factors, which regulate the content of epicuticular wax, a coating of wax covering the outer surface of the plant cuticle in land plants to reduce the water loss via surfaces (Tang et al., 2018). It is possible that lack of normal stomatal closure, could be linked with deploying other strategies like increasing epicuticular waxes for reducing water loss from plant surface. However, further investigations will be needed to experimentally prove if mutant deposit more wax over their surfaces.
In current study, we found that a lactoylglutathione lyase family protein (also known as Glyoxalase I) had lower expression in slac1-3 mutants in dark and dehydration stress (Table 1). Under abiotic stress, methylglyoxal (MG), a toxic molecule, accumulates in plants. It has been reported that at a certain optimum concentration MG induces stomatal closure, involving oxidative burst and [Ca2+]cyt oscillations but in ABA-independent manner (Hoque et al., 2012a,b). MG also inhibits Kin channel currents in Arabidopsis guard cells and interferes with light-induced stomatal opening (Hoque et al., 2012a,b, 2016). Glyoxalase I (also known as lactoylglutathione lyase) is involved in MG detoxification (Norton et al., 1990; Maiti et al., 1997). It seems that by keeping glyoxalase I expression at low level, mutant could be attempting to increase MG level and thereby promote stomatal closure under dehydration stress and darkness. However, further experimentation is needed to prove whether differential expression of Glyoxalase I had any impact on MG level by directly determining the latter’s level in both genotypes.
Interestingly, under both stimuli for stomatal closure (i.e., dark and dehydration stress), a major chunk of DEGs was found to be associated with abiotic and biotic stress responses (e.g., response to bacterial and fungal infections, wounding, chilling, salinity, dehydration stress etc.) and stress related-hormonal pathways (e.g., abscisic acid, salicylic acid, jasmonic acid, and ethylene pathways). This shows that defects in stimulus-induced stomatal closure may result in the induction of stress response pathways.
To conclude, in current study, we have identified specific changes in gene expression underlying slac1-3 mutation and resultant defects in stomatal closure in Arabidopsis. The large-scale changes in gene expression in slac1-3 mutants could be attributed to changes in osmotic potential, leaf temperature, and/or higher resting Ca2+ level in mutant. Furthermore, the induction of stress responsive pathways in mutant could be suggestive of the role of stomata as a switch in triggering abiotic and biotic stress responsive genes, which could help the plant to survive under stressful conditions. The conclusions of current research are presented as a model in Figure 7.
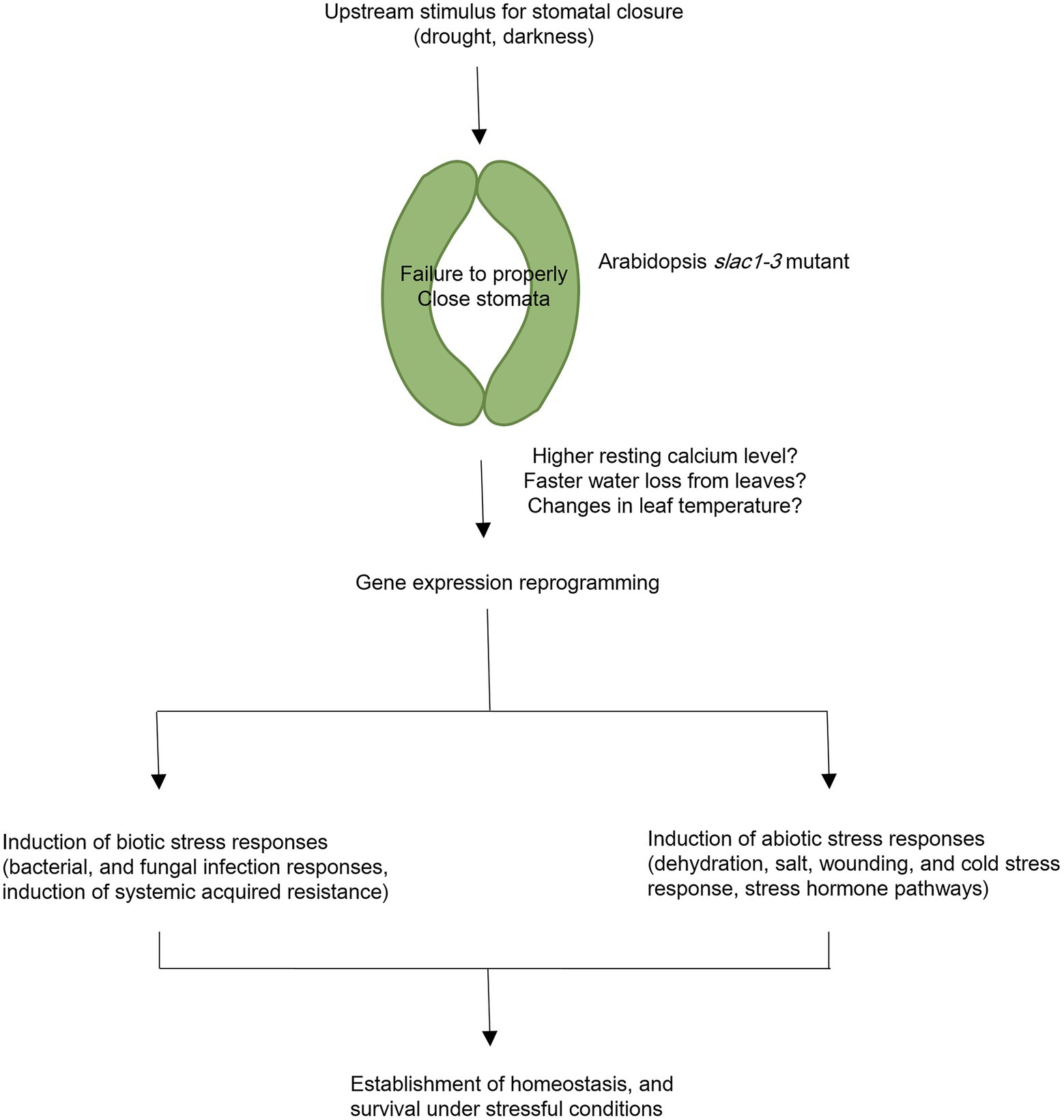
Figure 7. A model representing transcriptomic changes undertaken by Arabidopsis slac1-3 mutant in response to dehydration stress and dark treatment. Under these stimuli, slac1-3 mutant shows defective stomatal closure. Resultantly, plants lose more water and exhibit cooler leaf temperature. Mutation in Arabidopsis SLAC1 is also linked with higher resting calcium level in mutant. These factors could be among the possible links between slac1-3 mutation and differential transcriptomic response in mutant. To compensate for defective stomatal closure and its consequences, mutant undergo biotic and abiotic stress responses. The former involves induction of bacterial and fungal infection pathways, and systemic acquired resistance while the latter involves dehydration, salt, wounding, and cold stress responses, and induction of stress hormone pathways. However, it should be noted that few of these hormones may also be simultaneously associated with biotic stress response.
Data availability statement
The original contributions presented in the study are included in the article/Supplementary material, further inquiries can be directed to the corresponding authors.
Author contributions
ZW, SW, DX, and YX: methodology, formal analysis, investigation, and writing–review and editing. YO: methodology, formal analysis, investigation, and writing–original draft. HR: conceptualization, methodology, formal analysis, investigation, writing–original draft, writing–review and editing, and funding acquisition. JH: conceptualization and manuscript writing, review, and editing. GQ: conceptualization. All authors contributed to the article and approved the submitted version.
Funding
This study was supported by the Natural Science Foundation of Zhejiang Province (Grant No: LY20C020001), the Open Project Program of State Key Laboratory of Crop Stress Biology for Arid Areas, NWFU, Yangling, Shanxi, China (Grant No: CSBAAKF2021008), and the State Key Laboratory of Subtropical Silviculture (Grant No: ZY20190201).
Conflict of interest
The authors declare that the research was conducted in the absence of any commercial or financial relationships that could be construed as a potential conflict of interest.
Publisher’s note
All claims expressed in this article are solely those of the authors and do not necessarily represent those of their affiliated organizations, or those of the publisher, the editors and the reviewers. Any product that may be evaluated in this article, or claim that may be made by its manufacturer, is not guaranteed or endorsed by the publisher.
Supplementary material
The Supplementary material for this article can be found online at: https://www.frontiersin.org/articles/10.3389/fpls.2022.987606/full#supplementary-material
Supplementary Figure S1 | Venn diagram of DEGs between dehydration stressdrought (40, Figure 3A), slac1-3 mutant 698 (314, Figure 3B), and day and night (262, Figure 3C).
Footnotes
References
Brandt, B., Brodsky, D. E., Xue, S., Negi, J., Iba, K., Kangasjärvi, J., et al. (2012). Reconstitution of abscisic acid activation of SLAC1 anion channel by CPK6 and OST1 kinases and branched ABI1 PP2C phosphatase action. Proc. Natl. Acad. Sci. U. S. A. 109, 10593–10598. doi: 10.1073/pnas.1116590109
Chen, C., Chen, H., Zhang, Y., Thomas, H. R., Frank, M. H., He, Y., et al. (2020). TBtools: an integrative toolkit developed for interactive analyses of big biological data. Mol. Plant 13, 1194–1202. doi: 10.1016/j.molp.2020.06.009
Chen, Y. H., Hu, L., Punta, M., Bruni, R., Hillerich, B., Kloss, B., et al. (2010). Homologue structure of the SLAC1 anion channel for closing stomata in leaves. Nature 467, 1074–1080. doi: 10.1038/nature09487
Cheong, Y. H., Pandey, G. K., Grant, J. J., Batistic, O., Li, L., Kim, B. G., et al. (2007). Two calcineurin B-like calcium sensors, interacting with protein kinase CIPK23, regulate leaf transpiration and root potassium uptake in Arabidopsis. Plant J. 52, 223–239. doi: 10.1111/j.1365-313X.2007.03236.x
Cominelli, E., Galbiati, M., and Tonelli, C. (2010). Transcription factors controlling stomatal movements and drought tolerance. Transcription 1, 41–45. doi: 10.4161/trns.1.1.12064
Dobra, J., Motyka, V., Dobrev, P., Malbeck, J., Prasil, I. T., Haisel, D., et al. (2010). Comparison of hormonal responses to heat, drought and combined stress in tobacco plants with elevated proline content. J. Plant Physiol. 167, 1360–1370. doi: 10.1016/j.jplph.2010.05.013
Engineer, C. B., Hashimoto-Sugimoto, M., Negi, J., Israelsson-Nordström, M., Azoulay-Shemer, T., Rappel, W. J., et al. (2016). CO2 sensing and CO2 regulation of stomatal conductance: advances and open questions. Trends Plant Sci. 21, 16–30. doi: 10.1016/j.tplants.2015.08.014
Geiger, D., Scherzer, S., Mumm, P., Marten, I., Ache, P., Matschi, S., et al. (2010). Guard cell anion channel SLAC1 is regulated by CDPK protein kinases with distinct Ca2+ affinities. Proc. Natl. Acad. Sci. U. S. A. 107, 8023–8028. doi: 10.1073/pnas.0912030107
Geiger, D., Scherzer, S., Mumm, P., Stange, A., Marten, I., Bauer, H., et al. (2009). Activity of guard cell anion channel SLAC1 is controlled by drought-stress signaling kinase-phosphatase pair. Proc. Natl. Acad. Sci. U. S. A. 106, 21425–21430. doi: 10.1073/pnas.0912021106
Hedrich, R., and Geiger, D. (2017). Biology of SLAC1-type anion channels - from nutrient uptake to stomatal closure. New Phytol. 216, 46–61. doi: 10.1111/nph.14685
Hoque, T. S., Hossain, M. A., Mostofa, M. G., Burritt, D. J., Fujita, M., and Tran, L. S. (2016). Methylglyoxal: an emerging signaling molecule in plant abiotic stress responses and tolerance. Front. Plant Sci. 7:1341. doi: 10.3389/fpls.2016.01341
Hoque, T. S., Okuma, E., Uraji, M., Furuichi, T., Sasaki, T., Hoque, M. A., et al. (2012a). Inhibitory effects of methylglyoxal on light-induced stomatal opening and inward K+ channel activity in Arabidopsis. Biosci. Biotechnol. Biochem. 76, 617–619. doi: 10.1271/bbb.110885
Hoque, T. S., Uraji, M., Ye, W., Hossain, M. A., Nakamura, Y., and Murata, Y. (2012b). Methylglyoxal-induced stomatal closure accompanied by peroxidase-mediated ROS production in Arabidopsis. J. Plant Physiol. 169, 979–986. doi: 10.1016/j.jplph.2012.02.007
Huang, Y., Guo, Y., Liu, Y., Zhang, F., Wang, Z., Wang, H., et al. (2018). 9-cis-epoxycarotenoid dioxygenase 3 regulates plant growth and enhances multi-abiotic stress tolerance in rice. Front. Plant Sci. 9:162. doi: 10.3389/fpls.2018.00162
Hugouvieux, V., Kwak, J. M., and Schroeder, J. I. (2001). An mRNA cap binding protein, ABH1, modulates early abscisic acid signal transduction in Arabidopsis. Cell J. 106, 477–487. doi: 10.1016/S0092-8674(01)00460-3
Jammes, F., Song, C., Shin, D., Munemasa, S., Takeda, K., Gu, D., et al. (2009). MAP kinases MPK9 and MPK12 are preferentially expressed in guard cells and positively regulate ROS-mediated ABA signaling. Proc. Natl. Acad. Sci. U. S. A. 106, 20520–20525. doi: 10.1073/pnas.0907205106
Jezek, M., and Blatt, M. R. (2017). The membrane transport system of the guard cell and its integration for stomatal dynamics. Plant Physiol. 174, 487–519. doi: 10.1104/pp.16.01949
Kaňa, R., and Vass, I. (2008). Thermoimaging as a tool for studying light-induced heating of leaves. Environ. Exp. Bot. 64, 90–96. doi: 10.1016/j.envexpbot.2008.02.006
Khokon, A. R., Okuma, E., Hossain, M. A., Munemasa, S., Uraji, M., Nakamura, Y., et al. (2011). Involvement of extracellular oxidative burst in salicylic acid-induced stomatal closure in Arabidopsis. Plant Cell Environ. 34, 434–443. doi: 10.1111/j.1365-3040.2010.02253.x
Khokon, M. A., Salam, M. A., Jammes, F., Ye, W., Hossain, M. A., Uraji, M., et al. (2015). Two guard cell mitogen-activated protein kinases, MPK9 and MPK12, function in methyl jasmonate-induced stomatal closure in Arabidopsis thaliana. Plant Biol. 17, 946–952. doi: 10.1111/plb.12321
Kim, T. H., Bohmer, M., Hu, H., Nishimura, N., and Schroeder, J. I. (2010). Guard cell signal transduction network: advances in understanding abscisic acid, CO2, and Ca2+ signaling. Annu. Rev. Plant Biol. 61, 561–591. doi: 10.1146/annurev-arplant-042809-112226
Kusumi, K., Hirotsuka, S., Kumamaru, T., and Iba, K. (2012). Increased leaf photosynthesis caused by elevated stomatal conductance in a rice mutant deficient in SLAC1, a guard cell anion channel protein. J. Exp. Bot. 63, 5635–5644. doi: 10.1093/jxb/ers216
Laanemets, K., Wang, Y. F., Lindgren, O., Wu, J., Nishimura, N., Lee, S., et al. (2013). Mutations in the SLAC1 anion channel slow stomatal opening and severely reduce K+ uptake channel activity via enhanced cytosolic [Ca2+] and increased Ca2+ sensitivity of K+ uptake channels. New Phytol. 197, 88–98. doi: 10.1111/nph.12008
Lee, S. C., Lan, W., Buchanan, B. B., and Luan, S. (2009). A protein kinase-phosphatase pair interacts with an ion channel to regulate ABA signaling in plant guard cells. Proc. Natl. Acad. Sci. U. S. A. 106, 21419–21424. doi: 10.1073/pnas.0910601106
Li, J., Kinoshita, T., Pandey, S., Ng, C. K. Y., Gygi, S. P., Shimazaki, K. I., et al. (2002). Modulation of an RNA-binding protein by abscisic-acid-activated protein kinase. Nature 418, 793–797. doi: 10.1038/nature00936
Maierhofer, T., Diekmann, M., Offenborn, J. N., Lind, C., Bauer, H., Hashimoto, K., et al. (2014). Site- and kinase-specific phosphorylation-mediated activation of SLAC1, a guard cell anion channel stimulated by abscisic acid. Sci. Signal. 7:ra86. doi: 10.1126/scisignal.2005703
Maiti, M. K., Krishnasamy, S., Owen, H. A., and Makaroff*, C. A. (1997). Molecular characterization of glyoxalase II from Arabidopsis thaliana. Plant Mol. Biol. 35, 471–481. doi: 10.1023/A:1005891123344
Melotto, M., Underwood, W., and He, S. Y. (2008). Role of stomata in plant innate immunity and foliar bacterial diseases. Annu. Rev. Phytopathol. 46, 101–122. doi: 10.1146/annurev.phyto.121107.104959
Meyer, S., Mumm, P., Imes, D., Endler, A., Weder, B., al-Rasheid, K. A. S., et al. (2010). AtALMT12 represents an R-type anion channel required for stomatal movement in Arabidopsis guard cells. Plant J. 63, 1054–1062. doi: 10.1111/j.1365-313X.2010.04302.x
Mori, I. C., Murata, Y., Yang, Y., Munemasa, S., Wang, Y. F., Andreoli, S., et al. (2006). CDPKs CPK6 and CPK3 function in ABA regulation of guard cell S-type anion- and ca(2+)-permeable channels and stomatal closure. PLoS Biol. 4:e327. doi: 10.1371/journal.pbio.0040327
Murata, Y., Pei, Z. M., Mori, I. C., and Schroeder, J. (2001). Abscisic acid activation of plasma membrane ca(2+) channels in guard cells requires cytosolic NAD(P)H and is differentially disrupted upstream and downstream of reactive oxygen species production in abi1-1 and abi2-1 protein phosphatase 2C mutants. Plant Cell 13, 2513–2523. doi: 10.1105/tpc.010210
Mustilli, A. C., Merlot, S., Vavasseur, A., Fenzi, F., and Giraudat, J. (2002). Arabidopsis OST1 protein kinase mediates the regulation of stomatal aperture by abscisic acid and acts upstream of reactive oxygen species production. Plant Cell 14, 3089–3099. doi: 10.1105/tpc.007906
Negi, J., Matsuda, O., Nagasawa, T., Oba, Y., Takahashi, H., Kawai-Yamada, M., et al. (2008). CO2 regulator SLAC1 and its homologues are essential for anion homeostasis in plant cells. Nature 452, 483–486. doi: 10.1038/nature06720
Nishimura, N., Sarkeshik, A., Nito, K., Park, S. Y., Wang, A., Carvalho, P. C., et al. (2010). PYR/PYL/RCAR family members are major in-vivo ABI1 protein phosphatase 2C-interacting proteins in Arabidopsis. Plant J. 61, 290–299. doi: 10.1111/j.1365-313X.2009.04054.x
Norton, S. J., Talesa, V., Yuan, W. J., and Principato, G. B. (1990). Glyoxalase I and glyoxalase II from Aloe vera: purification, characterization and comparison with animal glyoxalases. Biochem. Int. 22, 411–418.
Pillitteri, L. J., and Torii, K. U. (2012). Mechanisms of stomatal development. Annu. Rev. Plant Biol. 63, 591–614. doi: 10.1146/annurev-arplant-042811-105451
Prodhan, M. Y., Munemasa, S., Nahar, M. N., Nakamura, Y., and Murata, Y. (2018). Guard cell salicylic acid signaling is integrated into Abscisic acid signaling via the ca(2+)/CPK-dependent pathway. Plant Physiol. 178, 441–450. doi: 10.1104/pp.18.00321
Qi, G. N., Yao, F. Y., Ren, H. M., Sun, S. J., Tan, Y. Q., Zhang, Z. C., et al. (2018). The S-type Anion Channel ZmSLAC1 plays essential roles in stomatal closure by mediating nitrate efflux in maize. Plant Cell Physiol. 59, 614–623. doi: 10.1093/pcp/pcy015
Ren, H., Su, Q., Hussain, J., Tang, S., Song, W., Sun, Y., et al. (2021). Slow anion channel GhSLAC1 is essential for stomatal closure in response to drought stress in cotton. J. Plant Physiol. 258-259:153360. doi: 10.1016/j.jplph.2020.153360
Richardson, F., Brodribb, T. J., and Jordan, G. J. (2017). Amphistomatic leaf surfaces independently regulate gas exchange in response to variations in evaporative demand. Tree Physiol. 37, 869–878. doi: 10.1093/treephys/tpx073
Scherzer, S., Maierhofer, T., Al-Rasheid, K. A., Geiger, D., and Hedrich, R. (2012). Multiple calcium-dependent kinases modulate ABA-activated guard cell anion channels. Mol. Plant 5, 1409–1412. doi: 10.1093/mp/sss084
Schroeder, J. I., Allen, G. J., Hugouvieux, V., Kwak, J. M., and Waner, D. (2001). Guard cell signal transduction. Annu. Rev. Plant Physiol. Plant Mol. Biol. 52, 627–658. doi: 10.1146/annurev.arplant.52.1.627
Sirichandra, C., Wasilewska, A., Vlad, F., Valon, C., and Leung, J. (2009). The guard cell as a single-cell model towards understanding drought tolerance and abscisic acid action. J. Exp. Bot. [J]. 60, 1439–1463. doi: 10.1093/jxb/ern340
Tan, B. C., Schwartz, S. H., Zeevaart, J. A., and McCarty, D. R. (1997). Genetic control of abscisic acid biosynthesis in maize. Proc. Natl. Acad. Sci. U. S. A. 94, 12235–12240. doi: 10.1073/pnas.94.22.12235
Tang, Y., Zhang, W., Yin, Y. L., Feng, P., Li, H. L., and Chang, Y. (2018). Expression of ovate family protein 8 affects epicuticular waxes accumulation in Arabidopsis thaliana. Bot. Stud. 59:12. doi: 10.1186/s40529-018-0228-8
Umezawa, T., Nakashima, K., Miyakawa, T., Kuromori, T., Tanokura, M., Shinozaki, K., et al. (2010). Molecular basis of the core regulatory network in ABA responses: sensing, signaling and transport. Plant Cell Physiol. 51, 1821–1839. doi: 10.1093/pcp/pcq156
Vahisalu, T., Kollist, H., Wang, Y. F., Nishimura, N., Chan, W. Y., Valerio, G., et al. (2008). SLAC1 is required for plant guard cell S-type anion channel function in stomatal signalling. Nature 452, 487–491. doi: 10.1038/nature06608
Webb, A. A. R., and Baker, A. J. (2002). Stomatal biology: new techniques, new challenges. New Phytol. 153, 365–369. doi: 10.1046/j.0028-646X.2001.00347.x
Yan, S., Mclamore, E. S., Dong, S., Gao, H., Taguchi, M., Wang, N., et al. (2015). The role of plasma membrane H(+) -ATPase in jasmonate-induced ion fluxes and stomatal closure in Arabidopsis thaliana. Plant J. 83, 638–649. doi: 10.1111/tpj.12915
Yoshida, R., Umezawa, T., MizoguchI, T., Takahashi, S., Takahashi, F., and Shinozaki, K. (2006). The regulatory domain of SRK2E/OST1/SnRK2.6 interacts with ABI1 and integrates abscisic acid (ABA) and osmotic stress signals controlling stomatal closure in Arabidopsis. J. Biol. Chem. 281, 5310–5318. doi: 10.1074/jbc.M509820200
Young, J. J., Mehta, S., Israelsson, M., Godoski, J., Grill, E., and Schroeder, J. I. (2006). CO(2) signaling in guard cells: calcium sensitivity response modulation, a ca(2+)-independent phase, and CO(2) insensitivity of the gca2 mutant. Proc. Natl. Acad. Sci. U. S. A. 103, 7506–7511. doi: 10.1073/pnas.0602225103
Zotova, L., Kurishbayev, A., Jatayev, S., Khassanova, G., Zhubatkanov, A., Serikbay, D., et al. (2018). Genes encoding transcription factors TaDREB5 and TaNFYC-A7 are differentially expressed in leaves of bread wheat in response to drought, dehydration and ABA. Front. Plant Sci. 9:1441. doi: 10.3389/fpls.2018.01441
Keywords: drought stress, abscisic acid, stomata, anion channel, transcriptome
Citation: Wang Z, Ouyang Y, Ren H, Wang S, Xu D, Xin Y, Hussain J and Qi G (2022) Transcriptome profiling of Arabidopsis slac1-3 mutant reveals compensatory alterations in gene expression underlying defective stomatal closure. Front. Plant Sci. 13:987606. doi: 10.3389/fpls.2022.987606
Edited by:
Pasala Ratnakumar, Indian Institute of Oilseeds Research (ICAR), IndiaReviewed by:
Prachi Pandey, National Institute of Plant Genome Research (NIPGR), IndiaCun Wang, Northwest A&F University, China
Copyright © 2022 Wang, Ouyang, Ren, Wang, Xu, Xin, Hussain and Qi. This is an open-access article distributed under the terms of the Creative Commons Attribution License (CC BY). The use, distribution or reproduction in other forums is permitted, provided the original author(s) and the copyright owner(s) are credited and that the original publication in this journal is cited, in accordance with accepted academic practice. No use, distribution or reproduction is permitted which does not comply with these terms.
*Correspondence: Guoning Qi, Z25xaUB6YWZ1LmVkdS5jbg==; Jamshaid Hussain, amFtc2hhaWRodXNzYWluQGN1aWF0ZC5lZHUucGs=
†These authors have contributed equally to this work and share first authorship