- Guangxi Key Laboratory of Functional Phytochemicals Research and Utilization, Guangxi Institute of Botany, Guangxi Zhuang Autonomous Region and Chinese Academy of Sciences, Guilin, China
Polygala fallax Hemsl. (Polygalaceae), a traditional Chinese medicinal species, requires optimal growth conditions for artificial cultivation. Irradiance is one of the primary environmental factors that affects the growth and survival of P. fallax Hemsl. plants, which seemingly grow better under weak irradiance conditions. However, the optimum light intensity for growing P. fallax Hemsl. is not clear. To determine the optimum light intensity for cultivating this medicinal plant species, P. fallax Hemsl. plants from two different habitats were grown and exposed to three shade treatments (50% shade, 70% shade and 90% shade, which resulted in photosynthetically active radiation amounts equal to 662 μmol m−2 s−1, 401 μmol m−2 s−1, and 131 μmol m−2 s−1, respectively) to evaluate survival, growth, leaf photosynthesis, and the main pharmacological active ingredients (saponins) in response to shade. Our results revealed that the P. fallax Hemsl. plants in the different habitats consistently exhibited relatively high photosynthesis rates, biomass, survival rates and saponins under 662 μmol m−2 s−1 created by the 50% shade treatment. We concluded that photosynthetically active radiation of approximately 662 μmol m−2 s−1 is suitable for the cultivation of P. fallax Hemsl. plants.
Introduction
Polygala fallax Hemsl., a species of the Polygalaceae family, is a shrub or small tree distributed mainly in southern China (Xu et al., 2003; Zhang et al., 2012). In traditional Chinese medicine, the roots of P. fallax Hemsl. have been used in the treatment of acute and chronic hepatitis (Zhang et al., 1997; Lin et al., 2005), as they have certain pharmacological effects, such as anti-HBV, immune regulation and antioxidant activities (Hong et al., 2014; Yao et al., 2020). Pharmaceutical analysis shows that there are several natural active ingredients, particularly saponins, which have great pharmacological activities, in P. fallax Hemsl. plants (Ma et al., 2003; Podolak et al., 2010). Additionally, P. fallax Hemsl. is edible and has been used for both medicine and food in the local folklore of Guangxi. Thus, P. fallax Hemsl. has great medicinal and economic value, and there is great utilization potential that warrants further exploration for improving global food security and public health (Fahad et al., 2021).
In recent years, the amount of wild resources of P. fallax Hemsl. has sharply declined due to overexcavation. Thus, there is an urgent need to cultivate P. fallax Hemsl. plants to meet the increasing demand and protect wild resources. However, low survival rates were frequently observed when the wild P. fallax Hemsl. plants were transplanted to smallholder farms, and the biomass yield of the surviving plants was relatively unstable and generally low under natural field conditions (Wang et al., 2016). Zhang (2013) reported that wild P. fallax Hemsl. populations were found to be mainly distributed in the shaded and humid environments of valley forests. As such, the growth of P. fallax Hemsl. plants requires relatively harsh environmental conditions.
Recently, P. fallax Hemsl. plants were reported to grow better under weak light conditions than under high light conditions (Wang et al., 2016). We thus speculated that irradiance is one of the primary environmental factors that affects the survival and growth of P. fallax Hemsl. plants. However, the optimum light intensity for the growth of P. fallax Hemsl plants is not clear. Light is crucial not only for leaf photosynthesis, plant growth, and biomass accumulation but also for the production of secondary metabolites in medicinal plants (Fahad et al., 2013; Müller et al., 2013; Townsend et al., 2018). However, few studies have evaluated the effects of light intensity on the growth and active ingredients of this traditional Chinese medicinal plant species (Zhang, 2013; Wang et al., 2016). The objectives of the present study were to (i) evaluate the effects of different light intensities on plant growth and the active ingredients in plant tissues of P. fallax Hemsl. plants and (ii) determine the optimum light intensity for the production of P. fallax Hemsl. plants.
Materials and methods
Plant material and growth conditions
Pot experiments were conducted at Guangxi Institute of Botany, Chinese Academy of Sciences, Guilin, China (25°4′N, 110°18′E, 175 m above sea level) from 2019 to 2021. The materials included two P. fallax Hemsl. plants collected from two locations, i.e., Lingchuan (LC) county in Guiling city (25°25′N, 110°20′E) and Zhaoping (ZP) county in Hezhou city(24°11′N, 110°48′E), which are the original habitats for wild P. fallax Hemsl. plants in Guangxi Province, China. The seeds of P. fallax Hemsl. collected from the two habitats (LC, ZP) were sown in nursery beds at the Guangxi Institute of Botany on 16 Sept. 2019. Individual seedlings were transplanted to cylindrical pots (height, 12 cm; diameter, 8 cm) filled with 1.2 kg of clay soil on 10 March 2020 and grown under natural ambient conditions. The soil of the experimental field was a clay loam with a pH of 6.23, a total nitrogen content of 1.15 mg kg−1, an available potassium content of 117 mg kg−1, and an available phosphorus content of 11.45 mg kg−1.
Shade treatment
After seedling recovery, 150 pots of seedlings with a uniform plant architecture for each P. fallax Hemsl. plant from each habitat were randomly arranged into three groups, with fifty pots in each group, and subjected to one of three different shade treatments, i.e., 50% shade treatment, 70% shade treatment, and 90% shade treatment, on 11 Apr. 2020. A shade net (deep colored nylon mesh) was used to reduce the full light amount by 50, 70, and 90%, which provided 50, 30 and 10% of the original light intensity, respectively. The photosynthetically active radiation (PAR) under full light averaged 1,339 ± 71 μmol m−2 s−1 (n = 10, the light input peaked at 1806 μmol m−2 s−1 at midday), and it was 662 ± 47 μmol m−2 s−1, 401 ± 34 μmol m−2 s−1, and 131 ± 12 μmol m−2 s−1 after the 50, 70, and 90% shade treatments, respectively. The plants were grown under the shade treatments for 6 months, after which the plants were sampled (five replications each).
Leaf photosynthesis
Measurements of the diurnal net photosynthetic rate were performed on the youngest fully expanded mature leaves on the same side of the canopy using a portable photosynthesis system (LI-COR 6400, Lincoln, NE, United States) equipped with light and CO2 control modules. The block temperature and CO2 concentration in the cuvette were set to those of ambient conditions. The flow rate of the air was set to 500 μmol s−1. To prevent the influence of external interference, the air inlet of the LI-6400 was connected to a plastic tube (2 ~ 3 m in length), and the end of the tube was placed away from the operator. The artificial photosynthetic photon flux density (PPFD) was set to 0, 20, 50, 100, 150, 200, 400, 600, 800, 1,000, 1,200, 1,500, 1800, and 2000 μmol m−2 s−1 in sequence to generate photosynthetic light response curves. To promote stomatal opening, sampled leaves were exposed to natural light conditions for 20 min before measurements.
Chlorophyll content
The fully expanded mature leaves of five plants randomly selected from each shade treatment were used to determine the chlorophyll content. Leaf discs were collected, ground to a fine powder and extracted with 10 ml of 80% acetone (v/v). The homogenate was then centrifuged at 4,000× g at 4°C for 10 min, after which the supernatant was separated and used for the chlorophyll assay. The amounts of chlorophyll a, b and carotenoids were determined spectrophotometrically by reading the absorbance at 663 (W663) nm, 645 (W645) nm and 480 (W470), respectively (Lichtenthaler and Wellburn, 1983). The chlorophyll content was expressed as milligrams per unit leaf area (mg dm−2). The calculations were as follows:
Extraction and determination of saponins
The total saponins in the roots and stems were extracted and quantified using a Test Kit (QYS-239346, Qiyi biological technology (Shanghai) CO., LTD., China) according to the instructions, which is similar to the method of Yu et al. (2017) with minor differences. Briefly, the sampled roots and stems were crushed and sieved by an 80 mesh sieve after drying to a constant weight at 60°C. A total of 0.05 g of powder was weighed and extracted in methanol (1 ml) with ultrasonication for 1 h. The extract solution was centrifuged at 8,000× g for 10 min at 25°C, after which 0.5 ml of the supernatant was collected and freeze-dried. Next, 0.2 ml of fresh 5% (W/V) vanillin-acetic acid solution and 0.8 ml of perchloric acid were added, and the mixtures were incubated at 55°C for 20 min. Finally, 0.2 ml of the cooled mixture was collected in a dark-colored tube, and 1.0 ml ethyl acetate was added. After mixing thoroughly, the absorbance was measured by an ultraviolet spectrophotometer (Shanghai Spectrum Instruments Co., Ltd.) at 589 nm, with a blank solution as a reference. The content of total saponins was expressed in units of mg per g DW.
Growth measurements
The P. fallax Hemsl. plants from the two habitats (LC, ZP) were collected from each shade treatment on 27 Oct. 2020. The height, basal diameter, crown width and length and width of the fully expanded mature leaves of each plant were measured with a ruler. The root length was measured after the whole plants were rinsed with tap water. Next, the roots, shoots, and leaves were separated to determine their dry weight (DW, g), which was used to express the biomass (g plant−1) of the plants.
Statistical analysis
Analysis of variance (ANOVA) for all the variables was carried out using Statistix 8.0 (Analytical Software, Tallahassee, FL, United States). The treatment means were compared using the least significant difference (LSD) test at the p < 0.05 level.
Results
Effects of light intensity on the survival of Polygala fallax Hemsl.
As shown in Figure 1, the survival rate of P. fallax Hemsl. plants significantly differed among the shade treatments and between the locations, and nonsignificant interactions occurred between the shade treatments and locations. The survival rates were 88.0, 88.0 and 69.3% under the 50% shade treatment, 70% shade treatment, and 90% shade treatment, respectively, in P. fallax Hemsl. plants from the LC habitat. However, the survival rates of P. fallax Hemsl. from the ZP habitat were 64.0, 82.7 and 53.3% under the 50% shade treatment, 70% shade treatment, and 90% shade treatment, respectively. Generally, the P. fallax Hemsl. plants from the two habitats exhibited relatively high survival under the 70% shade treatment.
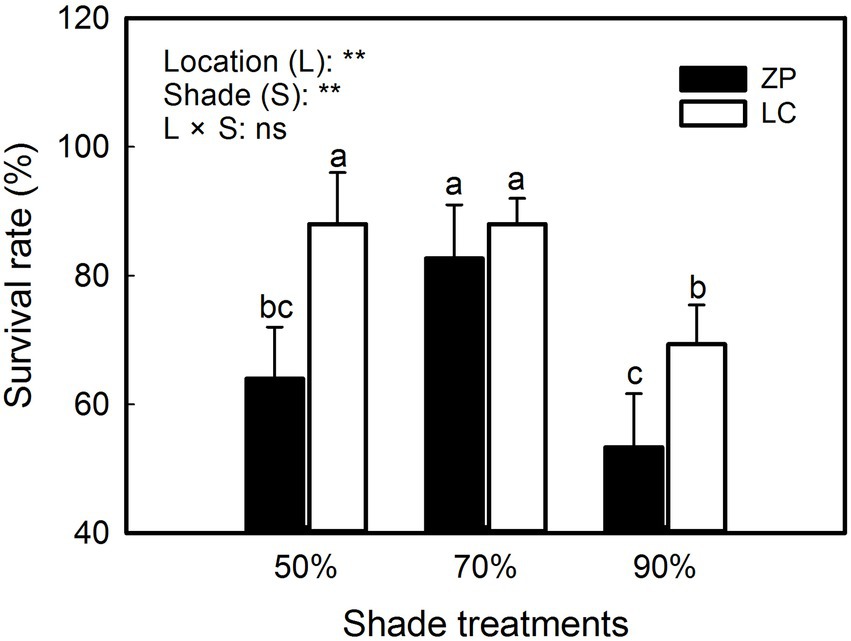
Figure 1. Effects of shade treatments on the survival of Polygala fallax Hemsl. plants. The data are presented as the mean ± SD (n = 5). The different letters indicate significant differences according to an LSD test at p < 0.05.
Effects of light intensity on the biomass of Polygala fallax Hemsl.
Table 1 shows that the biomass and the root-shoot ratio of P. fallax Hemsl. plants differed significantly among the shade treatments but not between locations, and significant interactions in terms of root biomass and the root-shoot ratio occurred between the shade treatments and locations. For the P. fallax Hemsl. plants from the LC habitat, the root biomass, aboveground biomass and total biomass were the highest under the 70% shade treatment, followed by the 50% shade treatment, and were the lowest under the 90% shade treatment. The root biomass, aboveground biomass and total biomass of P. fallax Hemsl. plants from the ZP habitat and the root-shoot ratio of P. fallax Hemsl. plants from the ZP and LC habitats were the highest under the 50% shade treatment, followed by the 70% shade treatment, and they were the lowest under the 90% shade treatment. However, no significant differences in these biomass parameters of the P. fallax Hemsl. plants from the ZP and LC habitats were observed between the 70% shade treatment and the 50% shade treatment.
Effects of light intensity on plant characteristics of Polygala fallax Hemsl.
Table 2 shows that, except for leaf width, the plant characteristics (plant height, basal diameter, root length, length and width of leaves) differed significantly among the shade treatments but not between the locations, and no significant interactions occurred between the shade treatments and locations. The P. fallax Hemsl. plants from the LC habitat exhibited the highest values for these characteristics under the 70% shade treatment, followed by the 50% shade treatment, and showed the lowest values under the 90% shade treatment. Similar trends in plant characteristics were observed for P. fallax Hemsl. plants from the ZP habitat, except for root length, which was the highest under the 50% shade treatment, followed by the 70% shade treatment. However, there were no significant differences in root length for the P. fallax Hemsl. plants from the ZP habitat between the two shade (70 and 50%) treatments.
Effects of light intensity on the chlorophyll content of Polygala fallax Hemsl.
Table 3 shows that the chlorophyll content (chlorophyll a, chlorophyll b, carotenoids) differed significantly among the shade treatments but not between locations, but there was a significant interaction between shade treatment and location, except for the chlorophyll b and carotenoid contents. The chlorophyll contents in the P. fallax Hemsl. plants from the two habitats (LC, ZP) exhibited a response trend similar to that found under the shade treatments. Generally, the chlorophyll a, chlorophyll b, carotenoid, and total chlorophyll contents were the highest under the 90% shade treatment, followed by the 70% shade treatment, and they were lowest under the 50% shade treatment.
Effects of light intensity on the photosynthetic rate of Polygala fallax Hemsl.
Light intensity significantly affected the photosynthetic rate of P. fallax Hemsl. plants. The response trends of the photosynthetic rate of P. fallax Hemsl. plants from the two habitats (LC, ZP) were similar under the three shade treatments. The photosynthetic rates of P. fallax Hemsl. plants from the two habitats were highest under the 70% shade treatment, followed by the 50% shade treatment, but no significant difference existed between the 70% shade treatment and the 50% shade treatment. The lowest photosynthetic rates were observed under the 90% shade treatment, and these values were significantly lower than the values under the 70% shade treatment and the 50% shade treatment (Figure 2).
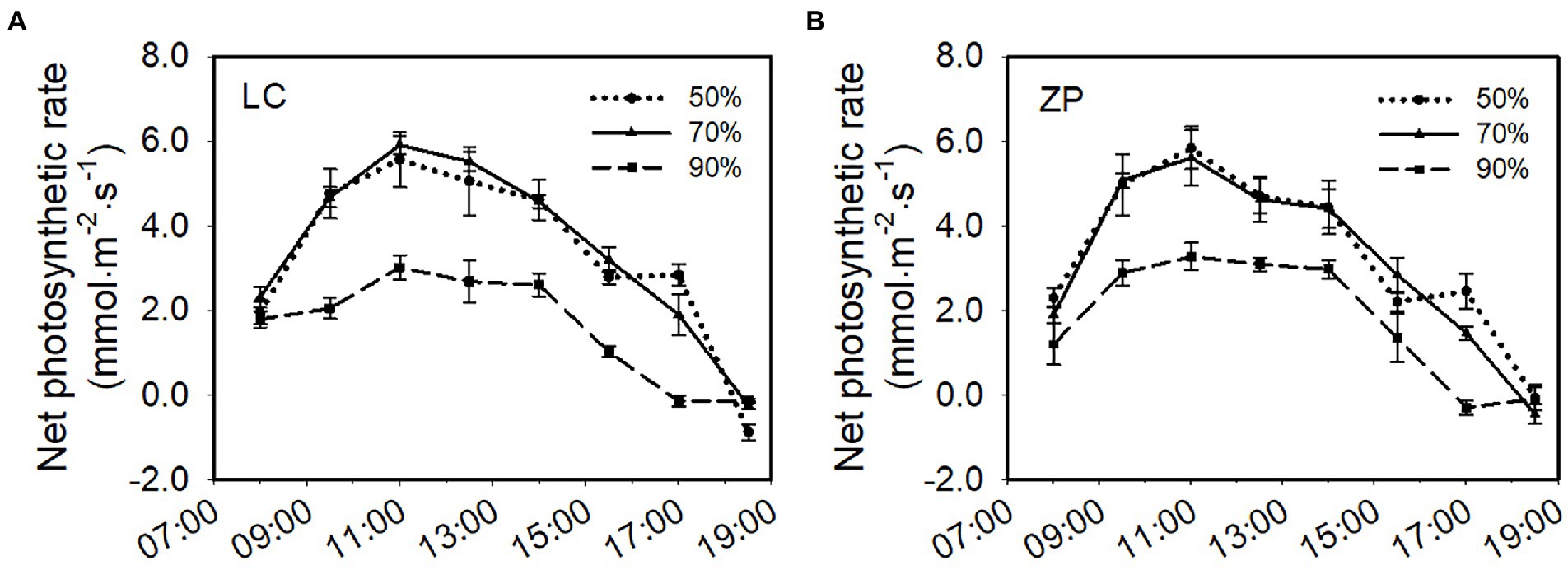
Figure 2. Effects of shade treatments on the net photosynthetic rate of P. fallax Hemsl. plants. The data are presented as the mean ± SD (n = 5). (A) Net photosynthesis rate of the P. fallax Hemsl. plants from the LC habitat; (B) Net photosynthesis rate of the P. fallax Hemsl. plants from the ZP habitat.
Effects of light intensity on the saponin concentration of Polygala fallax Hemsl.
Figure 3 shows that the concentration of total saponins in P. fallax Hemsl. plants differed significantly among the shade treatments but not between locations, and significant interactions occurred between the shade treatments and locations in the concentration of saponins in roots but not in stems. The concentration of saponins in roots and stems of P. fallax Hemsl. plants from the ZP and LC habitats were significantly higher under the 50% shade treatment than under the 70 and 90% shade treatments. However, the differences in the concentration of saponins in P. fallax Hemsl. plants were small between the 70 and 90% shade treatments.
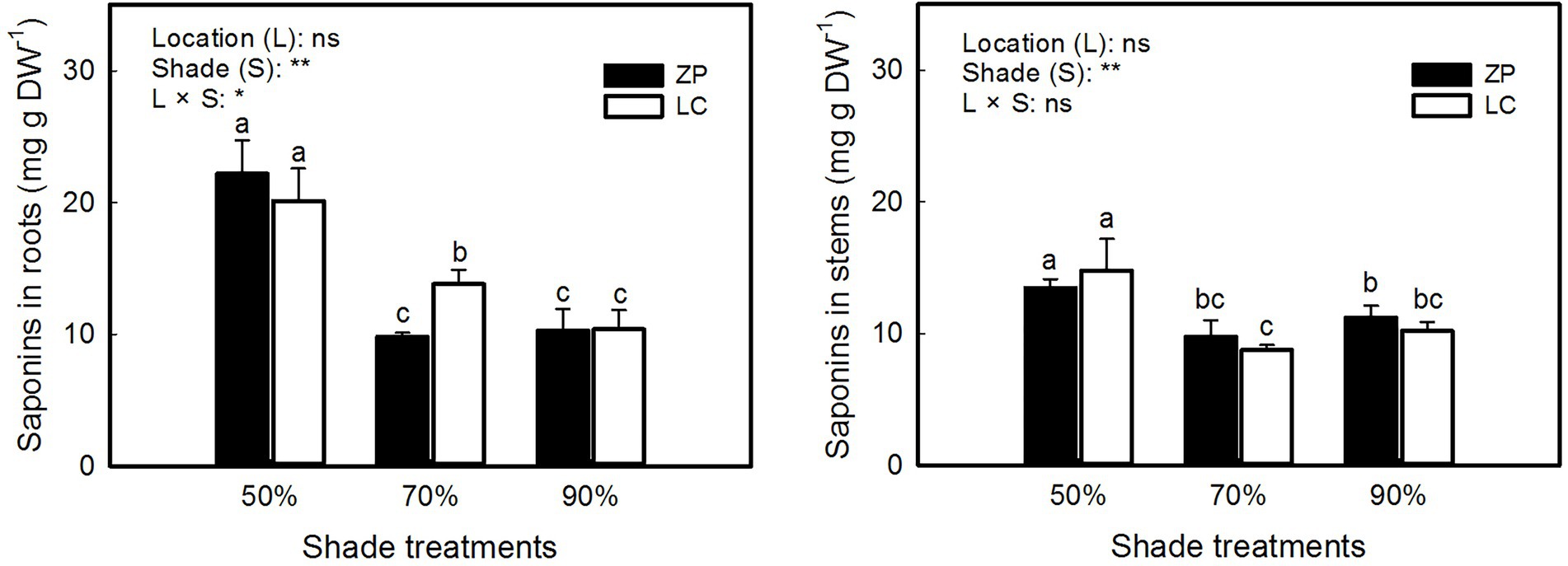
Figure 3. Effects of shade treatments on the saponin concentration of P. fallax Hemsl. plants. The data are presented as the mean ± SD (n = 3). *, ** and ns indicate significance at p < 0.05, p < 0.01 level and not significant, respectively. Different letters indicate significant differences among the three shade treatments for the P. fallax Hemsl. plants from the two habitats (LC, ZP) at the p < 0.05 level by a least significant difference test.
Discussion
Polygala fallax Hemsl., a traditional Chinese medicinal plant species, is highly sensitive to irradiance. Previously, Zhang (2013) cultivated P. fallax Hemsl. plants under fir and broadleaf mixed forests with different canopy densities and obtained high biomass production of P. fallax Hemsl. under a canopy density of 0.1 ~ 0.3, followed by a canopy density of 0.3 ~ 0.5, under which the plants could survive. The lowest amounts of biomass were produced under a canopy density of 0.7 ~ 0.9. P. fallax Hemsl. plants seemingly grew better under weaker light conditions. Thus, we believe that P. fallax Hemsl. is a typical shade loving plant. However, the optimum light intensity for ensuring the best growth of P. fallax Hemsl. plants remains unclear.
In the current study, P. fallax Hemsl. plants from different habitats presented high survival rates under the 50 and 70% shade treatments (PAR of 662 μmol m−2 s−1 and 401 μmol m−2 s−1, respectively; Figure 1), which is in accordance with previous results (Zhang, 2013). We also observed that P. fallax Hemsl. plants exhibited the highest values for biomass and plant characteristics under the 50 and 70% shade treatments (Tables 1, 2), which can be explained by the relatively high photosynthesis rate of the leaves under these treatments (Figure 2). Thus, the P. fallax Hemsl. plants preferentially grew under PARs of 662 μmol m−2 s−1 and 401 μmol m−2 s−1 resulting from the 50 and 70% shade treatments. In summary, a PAR of 401 μmol m−2 s−1 – 662 μmol m−2 s−1 ensured a high leaf photosynthesis rate, resulting in high biomass production, and was associated with a relatively high survival rate. Thus, this level of irradiance is optimal for the cultivation of P. fallax Hemsl. plants.
Irradiance is necessary for the survival, growth, and reproduction of higher plants during their lifespan (Franklin and Forster, 1997). Excessive or insufficient light intensity can be harmful to the photosynthetic system of medicinal plants. In the present study, P. fallax Hemsl. plants exhibited a higher photosynthesis rate under the 50% shade treatment and 70% shade treatment but a lower photosynthesis rate under the 90% shade treatment (Figure 2). High light stress reduced photosynthesis due to (i) the blocked electron transport during photosynthesis and (ii) the irreversible inactivation of the photosystem II (PSII) reaction center (Lu et al., 2017), while weak light limited photosynthesis due to the resulting dark-induced senescence (Spundova et al., 2005; Parlitz et al., 2011). We speculated that full light may induce high light stress injury to the photosynthetic system and that the serious shading (PAR of 131 μmol m−2 s−1) resulting from the 90% shade treatment induced weak light stress injury to the P. fallax Hemsl. plants.
Additionally, we observed that the chlorophyll content decreased in P. fallax Hemsl. plants from both the LC and ZP habitats as the light intensity increased (Table 3). Shade treatment was shown to reduce the chlorophyll content in soybean, rice, cotton, American grapevine and Chinese tea plants (Tian et al., 2017; Li et al., 2019; Silva et al., 2019; Hussain et al., 2020; Zhang et al., 2020). Moreover, the chlorophyll contents of Camellia japonica ‘Naidong’ and maize plants decreased under shade treatment, as was observed in other plant species (Ren et al., 2016; Liu et al., 2018). Xie et al. (2020) reported that chlorophyll a and chlorophyll b contents decreased in C3 turfgrasses but increased in C4 turfgrasses under shaded conditions. The responses of chlorophyll to light intensity seemingly vary among species. P. fallax Hemsl. plants prefer weaker light intensity and thus may accumulate more chlorophyll under shaded conditions. In tea plants, chlorophyll and carotenoid synthesis-related genes were downregulated in response to strong light but were upregulated in response to shade or reduced light intensity (Yue et al., 2021). Genes involved in the biosynthesis of chlorophyll in P. fallax Hemsl. plants under shade treatment should be further studied.
As one of the most important active ingredients in P. fallax Hemsl. plants, saponins were the highest under the 50% shade treatment (Figure 3). In Panax japonicus var. major, the total saponins were enhanced by shade treatment when compared with nonshade treatment (Huang et al., 2018). However, the total saponins in the roots and stems of P. fallax Hemsl. plants decreased as shade increased (Figure 3), which may be due to the expression of key enzymes involved in saponin biosynthesis being downregulated by more serious shading (Cheng et al., 2021). Thus, an optimum light intensity is crucial for saponin biosynthesis in this medicinal plant. In the present study, we found that a PAR of 662 μmol m−2 s−1 resulting from the 50% shade treatment contributed to the relatively high accumulation of saponins in P. fallax Hemsl. plants.
Conclusion
The P. fallax Hemsl. plants exhibited a high leaf photosynthesis rate, produced high biomass and presented a relatively high survival rate under a PAR of approximately 401 μmol m−2 s−1 – 662 μmol m−2 s−1. The greatest concentration of total saponins in P. fallax Hemsl. plants were achieved under a PAR of 662 μmol m−2 s−1. In conclusion, a PAR of approximately 662 μmol m−2 s−1 should be adopted for the cultivation of P. fallax Hemsl. plants for obtaining both high biomass production and a high yield of saponins.
Data availability statement
The original contributions presented in the study are included in the article/supplementary material, further inquiries can be directed to the corresponding author.
Author contributions
HL, MW, and HT designed experiments. HL, XH, LW, XZ, and BL performed experiments. CW and BL analyzed data and compiled figures. CW wrote the manuscript. CW and HT edited the final manuscript. All authors contributed to the article and approved the submitted version.
Funding
This work was supported by the Science and Technology Major Project of Guangxi, China (grant no. Guike AA22096020), the Guangxi Natural Science Foundation (grant no. 2021GXNSFBA220067), the Guangxi Key Laboratory of Functional Photochemical Research and Utilization lab’s projects (grant no. 20190213-2), and the Basic Research Fund of Guangxi Academy of Sciences (grant no. CQZ-C-1901).
Conflict of interest
The authors declare that the research was conducted in the absence of any commercial or financial relationships that could be construed as a potential conflict of interest.
Publisher’s note
All claims expressed in this article are solely those of the authors and do not necessarily represent those of their affiliated organizations, or those of the publisher, the editors and the reviewers. Any product that may be evaluated in this article, or claim that may be made by its manufacturer, is not guaranteed or endorsed by the publisher.
References
Cheng, Y., Liu, H., Tong, X., Liu, Z., and Yu, X. (2021). Effects of shading on triterpene saponin accumulation and related gene expression of Aralia elata (Miq.) seem. Plant Physiol. Biochem. 160, 166–174. doi: 10.1016/j.plaphy.2021.01.009
Fahad, S., Chen, Y., Saud, S., Wang, K., Xiong, D., Chen, C., et al. (2013). Ultraviolet radiation effect on photosynthetic pigments, biochemical attributes, antioxidant enzyme activity and hormonal contents of wheat. J. Food Agric. Environ. 11, 1635–1641.
Fahad, S., Sonmez, O., Saud, S., Wang, D., Wu, C., Adnan, M., et al. (2021). Developing Climate-Resilient Crops Improving Global Food Security and Safety. CRC Press, Boca Raton.
Franklin, L. A., and Forster, R. M. (1997). The changing irradiance environment: consequences for marine macrophyte physiology, productivity and ecology. Eur. J. Phycol. 32, 207–232. doi: 10.1080/09670269710001737149
Hong, D., Bai, Y. P., Shi, R. Z., Tan, G. S., Hu, C. P., and Zhang, G. G. (2014). Inhibitory effect of reinioside C on vascular smooth muscle cells proliferation induced by angiotensin II via inhibiting NADPH oxidase-ROS-ENK1/2-NF-kappaB-AP-1 pathway. Pharmazie 69, 698–703. doi: 10.1691/ph.2014.4556
Huang, W. J., Sun, X. C., Shi, X., Yue, Z. G., and Cai, X. H. (2018). Effects of shading on key enzyme genesexpression and accumulation of saponins in Panax japonicus var. major. China J. Chin. Mat. Med. 43, 3855–3861. doi: 10.19540/j.cnki.cjcmm.20180528.007
Hussain, S., Pang, T., Iqbal, N., Shafiq, I., Skalicky, M., Brestic, M., et al. (2020). Acclimation strategy and plasticity of different soybean genotypes in intercropping. Funct. Plant Biol. 47, 592–610. doi: 10.1071/FP19161
Li, T., Dai, J., Zhang, Y., Kong, X., Li, C., and Dong, H. (2019). Topical shading substantially inhibits vegetative branching by altering leaf photosynthesis and hormone contents of cotton plants. Field Crop Res. 238, 18–26. doi: 10.1016/j.fcr.2019.04.019
Lichtenthaler, H. K., and Wellburn, A. R. (1983). Determinations of total carotenoids and chlorophylls a and b of leaf extracts in different solvents. Biochem. Soc. Trans. 11, 591–592. doi: 10.1042/bst0110591
Lin, L. L., Huang, F., Chen, S. B., Yang, D. J., Chen, S. L., Yang, J. S., et al. (2005). Chemical constituents in roots of Polygala fallax and their anti-oxidation activities in vitro. China J. Chin. Mat. Med. 30, 827–830. doi: 10.3321/j.issn:1001-5302.2005.11.008
Liu, C., Guo, X., Wang, K., Liu, Q., Sun, Y., Jiang, X. Q., et al. (2018). Ecophysiological responses of Camellia japonica (Naidong) to different light and water conditions. Chin. J. Appl. Ecol. 29, 1125–1132. doi: 10.13287/j.1001-9332.201804.005
Lu, T., Meng, Z., Zhang, G., Qi, M., Sun, Z., Liu, Y., et al. (2017). Sub-high temperature and high light intensity induced irreversible inhibition on photosynthesis system of tomato plant (Solanum lycopersicum L.). Frontiers. Plant Sci. 8:365. doi: 10.3389/fpls.2017.00365
Ma, W., Wei, X., Ling, T., Xie, H., and Zhou, W. (2003). New phenolics from Polygala fallax. J. Nat. Prod. 66, 441–443. doi: 10.1021/np020467i
Müller, V., Albert, A., Winkler, J. B., Lankes, C., Noga, G., and Hunsche, M. (2013). Ecologically relevant UV-B dose combined with high PAR intensity distinctly affect plant growth and accumulation of secondary metabolites in leaves of Centella asiatica L. Urban. J. Photochem. Photobiol. B Biol. 127, 161–169. doi: 10.1016/j.jphotobiol.2013.08.014
Parlitz, S., Kunze, R., Mueller-Roeber, B., and Balazadeh, S. (2011). Regulation of photosynthesis and transcription factor expression by leaf shading and re-illumination in Arabidopsis thaliana leaves. J. Plant Physiol. 168, 1311–1319. doi: 10.1016/j.jplph.2011.02.001
Podolak, I., Galanty, A., and Sobolewska, D. (2010). Saponins as cytotoxic agents: a review. Phytochem. Rev. 9, 425–474. doi: 10.1007/s11101-010-9183-z
Ren, B., Cui, H., Camberato, J. J., Dong, S., Liu, P., Zhao, B., et al. (2016). Effects of shading on the photosynthetic characteristics and mesophyll cell ultrastructure of summer maize. Sci. Nat. 103:67. doi: 10.1007/s00114-016-1392-x
Silva, P. S. O., Oliveirajunior, L. F. G., Mattos, E. C., Maciel, L. B. S., Santos, M. P. F., de Sena, E. O. A., et al. (2019). Calcium particle films promote artificial shading and photoprotection in leaves of American grapevines (Vitis labrusca L.). Sci. Hortic. 252, 77–84. doi: 10.1016/j.scienta.2019.03.041
Spundova, M., Sloukova, K., Hunkova, M., and Naus, J. (2005). Plant shading increases lipid peroxidation and intensifies senescence-induced changes in photosynthesis and activities of ascorbate peroxidase and glutathione reductase in wheat. Photosynthetica 43, 403–409. doi: 10.1007/s11099-005-0064-4
Tian, Y., Zhang, L., Zhang, Z., Qiao, M., and Fan, Y. (2017). Effects of shading in summer and autumn on physiological and biochemical characteristics of ‘Huangjinya’ in Shandong Province, China. Chin. J. Appl. Ecol. 28, 789–796. doi: 10.13287/j.1001-9332.201703.004
Townsend, A. J., Retkute, R., Chinnathambi, K., Randall, J. W. P., Foulkes, J., Carmo-Silva, E., et al. (2018). Suboptimal acclimation of photosynthesis to light in wheat canopies. Plant Physiol. 176, 1233–1246. doi: 10.1104/pp.17.01213
Wang, Z., He, Z., and Liu, J. (2016). Research summary of cultivation and utilization of Polygala fallax. Chin. Wild Plant Res. 35, 48–52. doi: 10.3969/j.issn.1006-9690.2016.04.013
Xie, F. C., Shi, Z. J., Zhang, G. Y., Zhang, C. T., Sun, X. Y., Yan, Y., et al. (2020). Quantitative leaf anatomy and photophysiology systems of C3 and C4 turfgrasses in response to shading. Sci. Hortic. 274:109674. doi: 10.1016/j.scienta.2020.109674
Xu, H., Xu, Z., and Zhu, D. (2003). Resources investigation and determination of total saponin content in Polygala fallax from Guangxi. J. Plant Resour. Environ. 12, 47–49. doi: 10.3969/j.issn.1674-7895.2003.01.011
Yao, Z. R., Li, Y., Wang, Z. W., Lan, Y. Y., Zeng, T. X., Gong, H., et al. (2020). Research on anti-hepatocellular carcinoma activity and mechanism of Polygala fallax Hemsl. J. Ethnopharmacol. 260:113062. doi: 10.1016/j.jep.2020.113062
Yu, M., Xiang, H., Zhang, S., Huang, Z., and Liu, Y. (2017). Contrastive study on content and anti-oxidation activity of total saponins from cultivated and wild Paris plants in Southwest China. Med. Plant 8, 7–11.
Yue, C., Wang, Z., and Yang, P. (2021). Review: the effect of light on the key pigment compounds of photosensitive etiolated tea plant. Bot. Stud. 62, 1–15. doi: 10.1186/s40529-021-00329-2
Zhang, H. (2013). Fir and broad forest interplanted Polygala fallax growth effect analysis. J. Fujian For. Sci. Technol. 40, 113–116. doi: 10.3969/j.issn.1002-7351.2013.03.26
Zhang, D., Miyase, T., Kuroyanagi, M., Umehara, K., and Noguchi, H. (1997). Oligosaccharide polyesters from roots of Polygala glomerata. Phytochemistry 45, 733–741. doi: 10.1016/S0031-9422(97)00490-1
Zhang, J., Song, Y., Zhang, Q., Chen, Z., and Zhang, H. (2012). Community structure and species diversity of precious herbs Polygala fallax Hemsl. J. Plant Genet. Res. 13, 819–824. doi: 10.13430/j.cnki.jpgr.2012.05.001
Keywords: Polygala fallax Hemsl., light intensity, photosynthesis, biomass, survival rate, saponin
Citation: Liang H, Liu B, Wu C, Zhang X, Wang M, Huang X, Wan L and Tang H (2022) Effects of light intensity on the growth of Polygala fallax Hemsl. (Polygalaceae). Front. Plant Sci. 13:985628. doi: 10.3389/fpls.2022.985628
Edited by:
Iftikhar Ali, Institute of Genetics and Developmental Biology (CAS), ChinaReviewed by:
Li Liangbo, Guangxi University of Chinese Medicine, ChinaQing Liu, South China Agricultural University, China
Copyright © 2022 Liang, Liu, Wu, Zhang, Wang, Huang, Wan and Tang. This is an open-access article distributed under the terms of the Creative Commons Attribution License (CC BY). The use, distribution or reproduction in other forums is permitted, provided the original author(s) and the copyright owner(s) are credited and that the original publication in this journal is cited, in accordance with accepted academic practice. No use, distribution or reproduction is permitted which does not comply with these terms.
*Correspondence: Chao Wu, d3VjaGFvQGd4aWIuY24=; Hui Tang, dGhAZ3hpYi5jbg==
†These authors have contributed equally to this work