- 1Department of Plant Protection, School of Agriculture, São Paulo State University (UNESP), Botucatu, Brazil
- 2Embrapa Environment, Laboratory of Environmental Microbiology, Jaguariúna, Brazil
- 3Department of Phytopathology, Federal University of Lavras, Lavras, Brazil
- 4Crop Bioprotection Research Unit, National Center for Agricultural Utilization Research, United States Department of Agriculture, Peoria, IL, United States
Microbial crop protection products based on Trichoderma have the ability to display multifunctional roles in plant protection, such as pathogen parasitism, enhance nutrient availability and stimulate plant growth, and these traits can be used to enhance the overall agronomic performance of a variety of crops. In the current study, we explored the multifunctional potential of two indigenous Brazilian strains of Trichoderma (T. asperelloides CMAA 1584 and T. lentiforme CMAA 1585) for their capability of controlling Sclerotinia sclerotiorum, a key plant pathogen of cotton, and for their ability of growth promotion in cotton plants (Gossypium hirsutum). Both strains were able to solubilize mineral phosphorus (CaHPO4), to release volatile organic compounds that impaired the mycelial growth of S. sclerotiorum, and to promote the growth of cotton plants under greenhouse conditions. In dual culture, Trichoderma strains reduced the growth rate and the number of sclerotia formed by S. sclerotiorum. By treating sclerotia with conidial suspensions of these Trichoderma strains, a strong inhibition of the myceliogenic germination was observed, as a result of the marked mycoparasitic activity exerted on the sclerotia. The parasitism over S. sclerotiorum was more effective with T. asperelloides CMAA 1584, whilst the biostimulant effects on cotton growth were more pronounced with T. lentiforme CMAA 1585, which also showed a higher capacity of phosphate solubilization. Thus, T. asperelloides CMAA 1584 displays higher efficiency in controlling S. sclerotiorum, while T. lentiforme CMAA 1585 is more suitable as a biostimulant due to its ability to promote growth in cotton plants. Overall, these Trichoderma strains may be used in mixture to provide both pathogen control and promotion of plant growth, and this strategy will support growers in minimizing the use of synthetic fertilizers and fungicides against white mold in cotton crops.
Introduction
Cotton (Gossypium hirsutum L.) is the most important source of natural fibers in the world (Sivakumar et al., 2021; Wu et al., 2022). According to Tarazi et al. (2019), approximately 150 countries are directly involved in the cotton industrial chain, being an income source for more than 100 million families worldwide. Brazil stands out as the fourth largest producer of cotton worldwide, attaining a cultivated area of 1.6 million hectares with an estimated crop production of 6.7 million tons in 2021/22 (CONAB - Companhia Nacional de Abastecimento, 2022).
Since 1990s, the cotton growing area has dramatically expanded throughout the savannah Central-West region of Brazil (known as biome ‘Cerrado’), mainly due to breeding efforts for developing locally adapted high-yielding cultivars and improving agronomic practices (Morello et al., 2015; Silva Neto et al., 2016; Barroso et al., 2017; Silva et al., 2019). As a result, the cultivated area in the Mato Grosso State has increased by approximately 1,500% in the last 30 years (ABRAPA - Associação Brasileira dos Produtores de Algodão, 2021). However, the high incidence of pests and diseases remain inflicting high productivity losses, accounting for approximately 35% of production costs (IMEA - Instituto Mato-Grossense de Economia Agropecuária, 2019).
Among several diseases that limit cotton growth and yield, white mold, also known as Sclerotinia stem rot, caused by the ascomycete fungus Sclerotinia sclerotiorum (Lib.) de Bary (Ascomycota: Sclerotiniaceae), is one of the most devastating and yield-limiting diseases. This plant pathogen cause billions of dollars of crop losses and is of great economic importance to several agricultural and vegetable crops worldwide, notably including cotton and soybean (Boland and Hall, 1994; O’Sullivan et al., 2021). According to the Brazilian Ministry of Agriculture, Livestock and Food Supply (MAPA), S. sclerotiorum is considered one of the eight diseases/pests with the highest phytosanitary risk for Brazil (Brazil, 2015). This disease poses a serious threat to cotton plants at all phenological stages, and pathogen forms a dark-pigmented and hardened mycelial threads known as sclerotium capable of surviving for several years in soil (Tourneau, 1979; Schwartz and Singh, 2013). Symptoms associated with the white mold in cotton include wilt, necrosis and rotting of stems, bolls, petioles and leaves (Charchar et al., 1999; Suassuna et al., 2019).
Currently, the Mato Grosso State is responsible for approximately 71% of the Brazilian cotton production, following an integrated cropping system with soybean and corn (Silva et al., 2019; IMEA - Instituto Mato-Grossense de Economia Agropecuária, 2021). According to Meyer et al. (2020), S. sclerotiorum is endemic in approximately 27% of soybean production areas in Brazil, and 87% of cotton growing areas in Mato Grosso, in which cotton is cultivated as a second crop after soybean crop (IMEA - Instituto Mato-Grossense de Economia Agropecuária, 2021). Thus, the succession of susceptible crops to S. sclerotiorum has been responsible for the continuous increase of the incidence of white mold, leading to an overuse of chemical fungicides as a means to alleviate crop yield losses. However, over-reliance of broad-spectrum chemical fungicides poses a serious risk to the environment (Komárek et al., 2010), health of growers (Kniss, 2017), and accelerate the selection of resistant strains of S. sclerotiorum (Zhou et al., 2014), all of which require urgent alternative measures that include the development bio-rational solutions for the integrated management of white mold disease.
Despite the fact that chemical fungicides are effective in protecting plants from the white mold, their stand-alone use has inconsistent and unsatisfactory results. This is mainly due to difficulties in achieving adequate application coverage of the target pathogen, coupled with the best timing of application when ascospores are discharged (Meyer et al., 2014). Additionally, the lack of genetically resistant plants and the adoption of intensive management strategies based solely on synthetic fungicides have led to the development of resistant S. sclerotiorum strains to many chemical active ingredients (Liang et al., 2015; Mao et al., 2018). In this sense, it is imperative to explore alternative measures such as biological control strategies against this cosmopolitan plant pathogen (Bettiol et al., 2021).
Among biological control agents, Trichoderma spp. are considered effective in controlling S. sclerotiorum across several crops (Li et al., 2005; Sharma and Sain, 2010; Elias et al., 2016; Sumida et al., 2018), including cotton. The efficacy of using the necrotrophic mycoparasite Trichoderma in the management of S. sclerotiorum is related to its ability to parasitize and degrade sclerotia, resulting in an inversely proportional relationship between the frequency of Trichoderma application and the viability of sclerotia in the soil (Ferraz et al., 2011; Geraldine et al., 2013; Smolińska and Kowalska, 2018). Notably, Trichoderma spp. antagonize a myriad of plant pathogens by distinct mechanisms of action (Lorito et al., 2010; Druzhinina et al., 2011; Hermosa et al., 2012; Monte et al., 2019; Monte and Hermosa, 2021). Furthermore, due to the plasticity of their genomes in expressing multiple ecological functions and diverse biochemical machinery, several Trichoderma species promote plant growth (Rubio et al., 2017; Monte et al., 2019; Monte and Hermosa, 2021) and induce plant defenses against biotic and abiotic stresses (Brotman et al., 2012; Hermosa et al., 2012; Rubio et al., 2017; Monte et al., 2019).
The effectiveness of plant pathogen suppression as well as growth promotion mediated by Trichoderma are species and strain dependent (Harman et al., 2004; Verma et al., 2007; Atanasova et al., 2013; Haddad et al., 2017; Sumida et al., 2018). Owing to the lack of studies exploring the biocontrol and biostimulant abilities of Trichoderma strains in association with cotton plants, this study aimed to investigate the potential of two novel indigenous Brazilian strains, Trichoderma asperelloides CMAA 1584 and Trichoderma lentiforme CMAA 1585, against S. sclerotiorum along with their role as cotton growth promoters.
Material and methods
Microorganisms
Trichoderma asperelloides CMAA 1584 (BRM 065723, GenBank accession ON542481) and Trichoderma lentiforme CMAA 1585 (BRM 065775, GenBank accession ON542480), both isolated from soil in Jaguariúna, SP, Brazil (22°43’43” S and 47°01’04” W), and deposited in the Collection of Microorganisms of Agricultural and Environmental Importance (CMAA) from Embrapa Environment (Jaguariúna, SP, Brazil), were used in these studies. These strains were reactivated and grown on potato-dextrose-agar medium (PDA; Acumedia Manufacturers®, Michigan, USA) in Petri dishes (9 × 1.5 cm) for 14 days at 25 ± 2 °C and 12:12 hours photoperiod. For preservation, 7-day-old sporulated colonies grown on PDA were cut into 5 mm pieces, placed in cryovials containing 1.5 mL of sterile solution of 20% (v/v) glycerol (Dinâmica®, São Paulo, SP, Brazil) prepared with double deionized water, and stored at –80 °C as stock cultures. Five-day-old PDA-grown cultures of these two Trichoderma strains were morphologically characterized based on colony growth aspects, conidiophores, and conidia size. Conidia size measurements were recorded with a light phase-contrast microscope (Olympus CS43 microscope and Olympus EP50 camera). The strains were identified phylogenetically using the translation elongation factor 1-α gene through direct comparison with data from reference type strains.
The plant pathogen Sclerotinia sclerotiorum CMAA 1105 (GenBank accession OM348513) was cultured on PDA in Petri dishes through myceliogenic germination from surface-sterilized sclerotia, and the newly-formed sclerotia were stored at 4 °C. This S. sclerotiorum strain was isolated in Jaguariúna, SP, Brazil (22°43’43” S and 47°01’04” W) in 1992, and was then deposited in the Collection of Microorganisms of Agricultural and Environmental Importance (CMAA) from Embrapa Environment (Jaguariúna, SP, Brazil). All fungal strains used in this study are registered under the Brazilian genetic heritage – SisGen – protocol A135E26.
Ability of Trichoderma strains to solubilize phosphate
The ability of Trichoderma strains to solubilize inorganic phosphate (P) was evaluated by quantifying the solubilized P in liquid NBRIP (National Botanical Research Institute’s Phosphate) medium, which contained per liter: 10.0 g glucose, 5.0 g MgCl2.6H2O, 0.25 g MgSO4.7H2O, 0.2 g KCl and 0.1 g (NH4)2SO4 (Nautiyal, 1999). In the medium, 50 mL of K2HPO4 (10%) and 100 mL of CaCl2 (10%) were added to form an insoluble calcium phosphate (CaHPO4) precipitate. For inoculum production, 7-day-old sporulated cultures of each Trichoderma strain were rinsed with 10 mL of a sterile solution containing 0.04% polyoxyethylene sorbitan mono-oleate (Tween® 80, Synth, SP, Brazil) and calibrated using a hemocytometer (improved Neubauer chamber, 400× magnification) under a microscope (DM 500, Leica Microsystems GmbH®, Germany) to provide a final inoculum size of 5 × 106 conidia mL-1 in the medium. These liquid cultures were then incubated at 28 ± 1°C in an orbital rotary shaker (TE-1401, Tecnal®, Piracicaba, SP, Brazil) at 180 rpm for 5 days with a 12:12 hours photoperiod. The amount of calcium phosphate in the medium before inoculation of Trichoderma strains were approximately 150 µg mL-1. Aliquots of 1 mL were taken at the 5th day and centrifuged at 7,000 rpm and 22 °C for 5 minutes to determine the concentration of soluble phosphorus, according to the colorimetric method described by Murphy and Riley (1962). The concentration of solubilized P in the supernatant was calibrated based on a standard curve of CaHPO4 (Sigma-Aldrich®, St. Louis, MO, USA) at concentrations of 0.5, 1.0, 2.0, 2.5, and 5.0 mg mL-1. The experiments were carried out with four biological repetitions to each fungal strain. Untreated control group (blank) was performed without the presence of microorganisms, whose values obtained were subtracted from those obtained in the presence of the fungal inoculum as a means to normalize the absorbance reads.
Antifungal activity of Trichoderma strains against S. sclerotiorum
The ability of Trichoderma strains to antagonize S. sclerotiorum was evaluated by dual culture tests. Mycelial plugs (5 mm diameter) from the colony margin of an actively growing Trichoderma culture in PDA were placed on the edge of the Petri dish, and another plug of 7-day-old colony of S. sclerotiorum cultured on PDA was placed on the opposite side, maintaining 7 cm apart from colony discs. The plates were incubated at 25 ± 2 °C and the mycelial growth of both fungi was measured daily until Trichoderma strains have overgrown or surrounded the S. sclerotiorum colony. After 14 days of incubation under dual culturing, the antagonistic potential of Trichoderma strains inhibiting the pathogen’s growth was measured and the development of sclerotia was also evaluated. Furthermore, a diagrammatic scale proposed by Bell et al. (1982) was used to score the antagonistic capacity, where: 1 - Trichoderma overcomes the pathogen and grows in 100% of the plate; 2 - Trichoderma grows on at least 75% of the plate; 3 - Trichoderma and the pathogen colonize approximately 50% of the plate; 4 - The pathogen colonizes at least 75% of the plate and resists to Trichoderma; 5 - The pathogen completely overlaps Trichoderma and occupies the entire surface of the plate. As a control, Petri dishes inoculated only with the pathogen served as the reference to calculate the percent inhibition of pathogen’s colony growth exerted by Trichoderma strains. The experiment was performed with five biological replicates for each strain.
To assess the effect of volatile organic compounds (VOCs) released by Trichoderma strains on S. sclerotiorum mycelial growth, two Petri dish bottoms, one containing the pathogen and the other with a Trichoderma strain, all plated in the center and grown on PDA, were superimposed (Muthukumar et al., 2011). As a control, Petri dishes containing the pathogen were overlaid with another containing only PDA. These paired cultures were maintained in a growth chamber under the same environmental conditions described above. After 2 days of incubation, due to the rapid mycelial growth of S. sclerotiorum, the percentage of inhibition of the pathogen was assessed and further calculated by the equation: Inhibition (%) = (D1 – D2)/D1 × 100, where D1 represents the radial diameter of the pathogen in the control treatment, and D2 the radial diameter of the pathogen confronted with Trichoderma. The experiment was performed with five biological replicates for each strain.
Parasitism of sclerotia of S. sclerotiorum by Trichoderma strains
The ability of both Trichoderma strains in parasitizing S. sclerotiorum sclerotia was evaluated in polypropylene boxes (11 cm × 11 cm × 3.5 cm) (Gerbox®) containing 200 g of a dystroferric dark red latosol, collected at Embrapa Environment and autoclaved at 121 °C for 60 minutes on three consecutive days. Dark-pigmented mature S. sclerotiorum sclerotia were produced in 500 mL Erlenmeyer flasks containing carrot and cornmeal, according to Garcia et al. (2012). Each autoclaved flask received three 5-mm-PDA discs of S. sclerotiorum mycelium, taken from the edge of a 7-day-old colony and incubated at 25 ± 2 °C. After 30 days of growth on carrot-cornmeal substrate, mature sclerotia were removed, placed on absorbent paper inside a laminar flow chamber, left drying for 24 hours, and then kept in a refrigerator at 4°C prior to using in bioassays. In each polypropylene box, 12 sclerotia were randomly distributed on the soil surface, and 10 mL suspensions containing 1 × 106, 1 × 107, and 1 × 108 conidia mL-1 of each Trichoderma strain were evenly applied with a pipette over the soil surface. All groups were incubated for 15 days at 25 ± 2 °C with a photoperiod of 12:12 hours (Geraldine et al., 2013). A control group was set up with sterile distilled water. After 15 days of incubation, all sclerotia were removed from the soil, surface-sterilized with ethanol (70%) and sodium hypochlorite (2%) for 2 minutes, and subsequently rinsed three times in sterile distilled water prior to plating them on a selective media. Soft and disintegrated sclerotia due to colonization by Trichoderma strains were counted after slight pressure with a tweezer (Henis et al., 1983). Sclerotia viability was evaluated by incubating them on Neon medium (Napoleão et al., 2006) for 7 days at 25 ± 2 °C, then observing for the formation of a yellow halo around the sclerotia, which were deemed to be viable. The experiment was performed in a completely randomized design for each strain, with three treatments (inoculum size) and four biological replicates, in addition to a mock control treated only with water.
Germination and vigor of cotton seeds treated with Trichoderma
Seeds of cotton cv. FM 975 WS® provided by Instituto Mato-Grossense do Algodão (IMA, Mato Grosso, Brazil) were used in the interaction studies involving Trichoderma strains and cotton plants. The seeds were surface disinfected in 70% ethanol followed by 2% sodium hypochlorite solution for 2 minutes and washed in sterile distilled water three times. Surface-sterilized cotton seeds were soaked in an aqueous Trichoderma suspension containing 1 × 106, 1 × 107, and 1 × 108 conidia mL-1 for 60 minutes, and then layered on a Petri dish to air-dry for 1 hour inside a laminar flow hood. Trichoderma-treated cotton seeds were sown in germitest® paper (Cienlab Equipamentos Científicos Ltda, Campinas, SP, Brazil) using a roller system moistened with distilled water and incubated at 25 ± 2 °C. The experiment was set up in a completely randomized design with three treatments (inoculum concentrations) and four independent biological replicates, with 20 seeds each (i.e., total of 80 seeds per treatment). The number of germinated seeds was determined on the 4th day after sowing, being expressed as a percentage of germinated seeds. Afterwards, the seedlings were dried in an oven at 105 °C until constant weight, and the vigor index was determined according to Abdul-Baki and Anderson (1973) by the equation: Vigor Index = Germination (%) × Seedling dry weight (g).
Effect of Trichoderma on cotton growth
Cotton seeds cv. FM 975 WS® were treated with crescent concentrations of Trichoderma conidia as described above and sown in rhizotron made of polyvinyl chloride (PVC) half-longitudinal tubes (100 cm height × 17.5 cm diameter), containing a mixture of a dystroferric dark red latosol and sand in a ratio of 2:1 (v/v). The soil exhibited the following chemical and physical attributes analyzed at 0 - 20 cm depth: pH in H2O = 4.3; OM = 32.3 g kg-1; P = 9.36 mg dm-3; Ca = 3.09 cmolc dm-3; Mg = 1.48 cmolc dm-3; K = 128.55 mg dm-3; SB = 4.95 cmolc dm-3; H + Al = 6.10 cmolc dm-3; t = 4.99 cmolc dm-3; V% = 44.54. In addition to seed treatment, 10 mL of the same conidial suspensions were applied in the planting furrow via drench at 15, 30 and 45 days after sowing (DAS). The experiment was set up in a randomized block design with three treatments (inoculum concentrations) and five independent biological replicates, in addition to mock cotton seeds as a control. The assay was carried out in a greenhouse for 60 days and the following growth parameters of the cotton plants were evaluated: height (14, 24, 31 and 55 DAS), root length (at 7, 14, 24 and 60 DAS) and leaf area of the first non-cotyledonary leaf (at 24 DAS), as described by Grimes and Carter (1969). At 60 DAS the following parameters were determined: stem diameter (2 cm above the soil surface), fresh and dry weights for both the aboveground portion and roots of the plants.
Statistical analysis
Homogeneity of variances and normality tests were performed by Bartlett’s and Shapiro-Wilk tests. Data were fitted to linear models and analyzed by analysis of variance (ANOVA) using original data sets to identify significant differences between means of the treatments (Tukey’s test, P < 0.05). Statistical analyses were performed using Minitab® software version 19.1.
Results
Morphological characterization of indigenous Trichoderma spp. strains
According to the phylogenetic analysis based on tef-alpha 1 gene, the strain CMAA 1584 was confirmed to be Trichoderma asperelloides, while the strain CMAA 1585 was identified as Trichoderma lentiforme. Purified monosporic cultures of these two Trichoderma spp. strains were very divergent from each other in terms of growth, color, conidial size, and conidiophores. As depicted in Figure 1, cultures of T. asperelloides CMAA 1584 exhibited profuse growth on PDA with dark green color when fully sporulated and forming ovoid conidia averaging 3.60 × 3.59 µm (length and width) with a resultant area estimated in 10.10 µm2 (standard error: ± 0.17 µm2, n = 20). When looking at T. lentiforme CMAA 1585 cultures, its sporulated colony assumed pale greenish color and produced conidia averaging 2.54 × 2.44 µm (length and width) with an estimated average area of 4.88 µm2 (standard error: ± 0.18 µm2, n = 20). The area size of T. asperelloides was noted to be twice larger than conidia of T. lentiforme. The morphological phenotypes and conidia sizes are consistent with values previously reported for these species (Samuels et al., 2010; Chaverri et al., 2015).
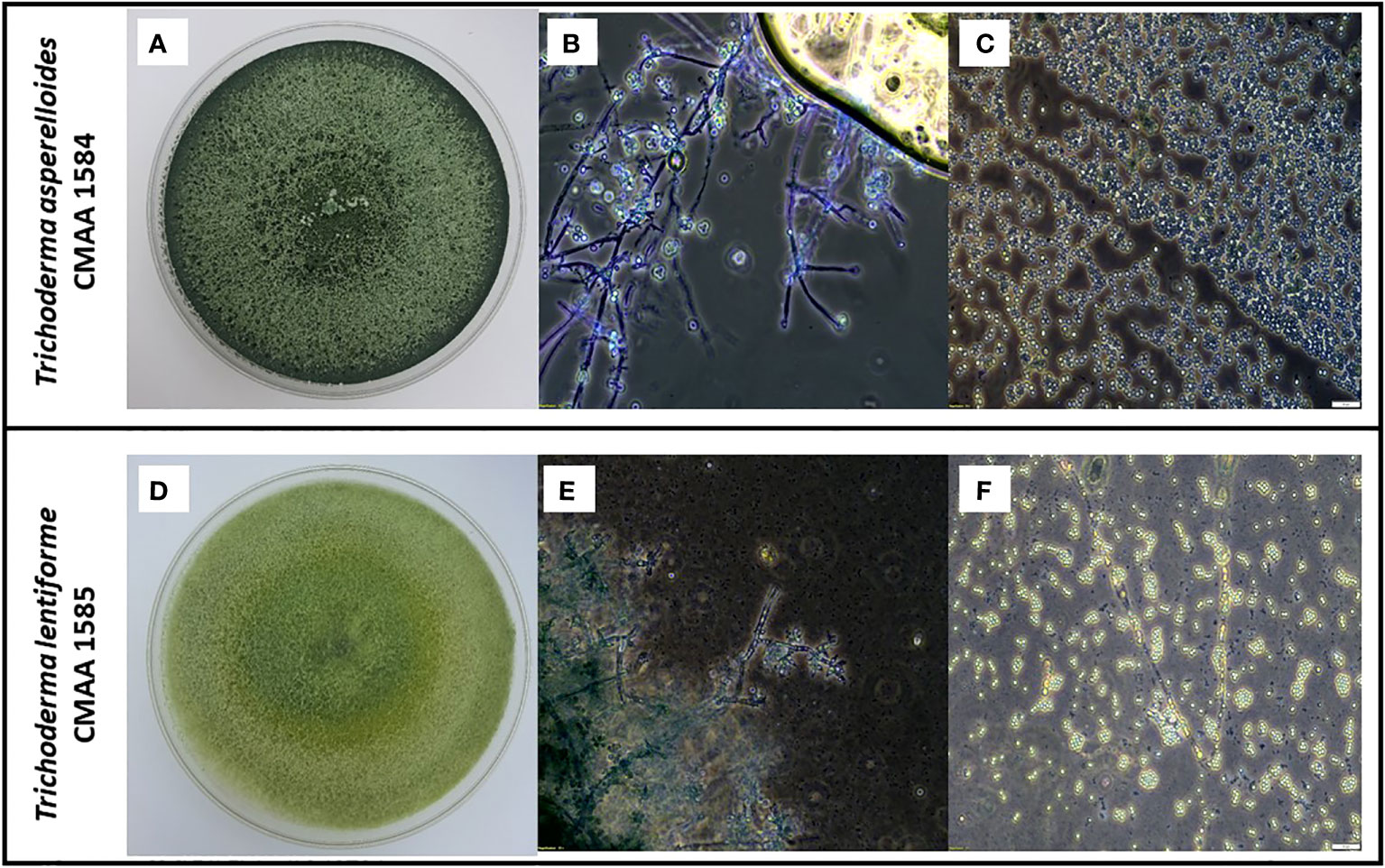
Figure 1 Morphological characterization of indigenous Trichoderma asperelloides, and Trichoderma lentiforme strains. (A) Five-day-old PDA-grown cultures of T. asperelloides (CMAA 1584); (B) Microscopic images showing conidiophores of CMAA 1584 (magnification at 200×); (C) Typical conidia of CMAA 1584 (magnification at 200×, scale bar = 20 µm); (D) Five-day-old PDA-grown cultures of T. lentiforme (CMAA 1585); (E) Microscopic images showing conidiophores of CMAA 1585 (magnification at 200×); (F) Typical conidia of CMAA 1585 (magnification at 200×, scale bar = 20 µm).
Phosphate solubilization
Trichoderma lentiforme CMAA 1585 and T. asperelloides CMAA 1584 were both capable of solubilizing inorganic phosphate, resulting in about 31.7% and 5.2% of CaHPO4 remaining in the medium, respectively, in comparison to control (Table 1). Phosphate solubilization was significantly (P < 0.05) higher in NBRIP medium, in which T. lentiforme solubilized significantly more phosphate than T. asperelloides (Table 1).
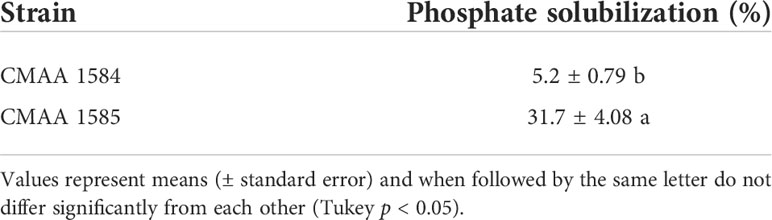
Table 1 Phosphate solubilization by Trichoderma asperelloides CMAA 1584 and Trichoderma lentiforme CMAA 1585.
Antifungal activity of Trichoderma strains against S. sclerotiorum
In general, volatile organic compounds (VOCs) released by Trichoderma strains significantly reduced (P < 0.05) the mycelial growth of S. sclerotiorum (Table 2). Compared to the control, VOCs emitted by T. lentiforme CMAA 1585 and T. asperelloides CMAA 1584 significantly reduced the growth rate of S. sclerotiorum by 55% and 53%, respectively (Table 2), albeit there was no difference between these Trichoderma strains in their ability to inhibit this pathogen by means of released VOCs.
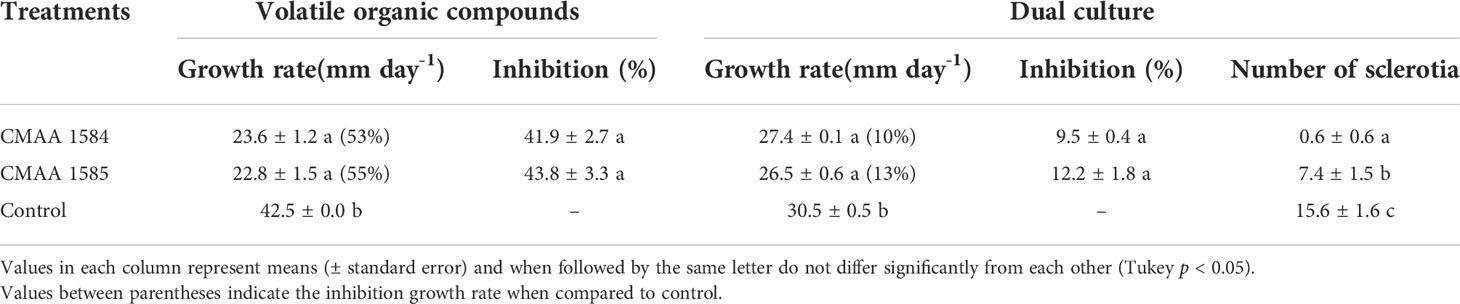
Table 2 Antagonistic activity of Trichoderma asperelloides CMAA 1584 and Trichoderma lentiforme CMAA 1585 against Sclerotinia sclerotiorum by dual culture test and production of volatile organic compounds (VOCs).
In dual culture assay for direct confrontation, T. asperelloides CMAA 1584 and T. lentiforme CMAA 1585 reduced the growth rate (mm day-1) of S. sclerotiorum in 10% and 13%, respectively, when compared to control (Table 2) (P < 0.05). The inhibition of mycelial growth was 9.5% and 12.2% for T. asperelloides CMAA 1584 and T. lentiforme CMAA 1585, respectively (Table 2). Notably, T. asperelloides CMAA 1584 and T. lentiforme CMAA 1585 remarkably decreased by 96% and 47% the number of sclerotia formed by S. sclerotiorum colony in comparison to control, respectively (Table 2, P < 0.05). Trichoderma lentiforme CMAA 1585 received higher scores (3.6) when compared to T. asperelloides CMAA 1584 (2.2) according to Bell’s diagrammatic scale, indicating that the former was less aggressive in parasitizing sclerotia than the latter (Figure 2).
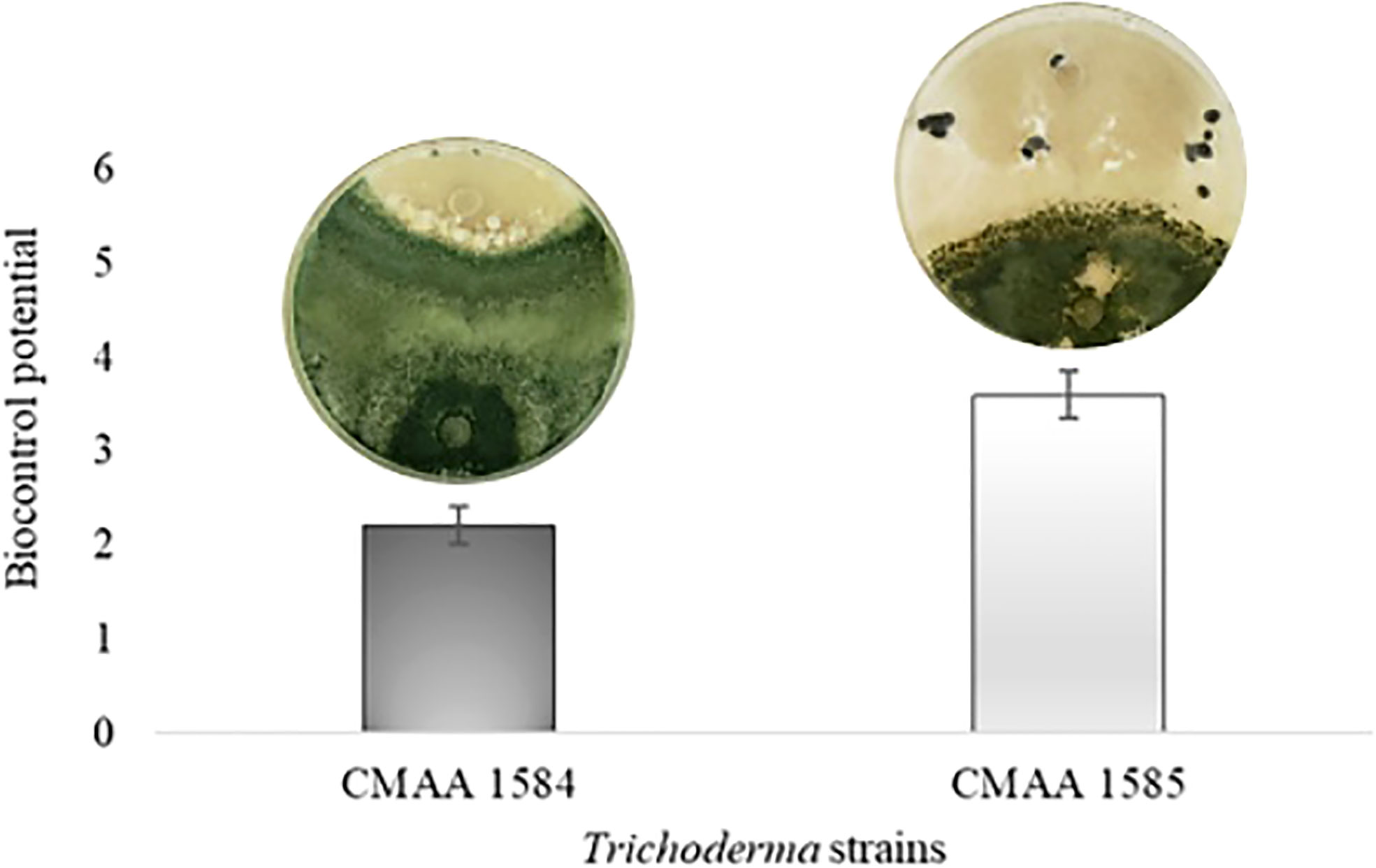
Figure 2 Biocontrol potential of Trichoderma asperelloides CMAA 1584, and Trichoderma lentiforme CMAA 1585 strains against Sclerotinia sclerotiorum according to Bell scale (Bell et al., 1982). Bars indicate means (± standard error).
Parasitism of sclerotia of S. sclerotiorum by Trichoderma strains
The myceliogenic germination of sclerotia was significantly (P < 0.05) reduced by both Trichoderma strains (Figure 3). All concentrations of T. asperelloides CMAA 1584 colonized 100% of sclerotia and thus strongly inhibited the myceliogenic germination of all sclerotia (Figure 3A). Trichoderma lentiforme CMAA 1585, despite colonizing 100% of sclerotia, only 69% sclerotia were found ungerminated or non-viable based on the Neon selective medium test. The degradation of sclerotia did not reveal significant differences (P > 0.05) between Trichoderma strains for all concentrations tested (Figure 3B).
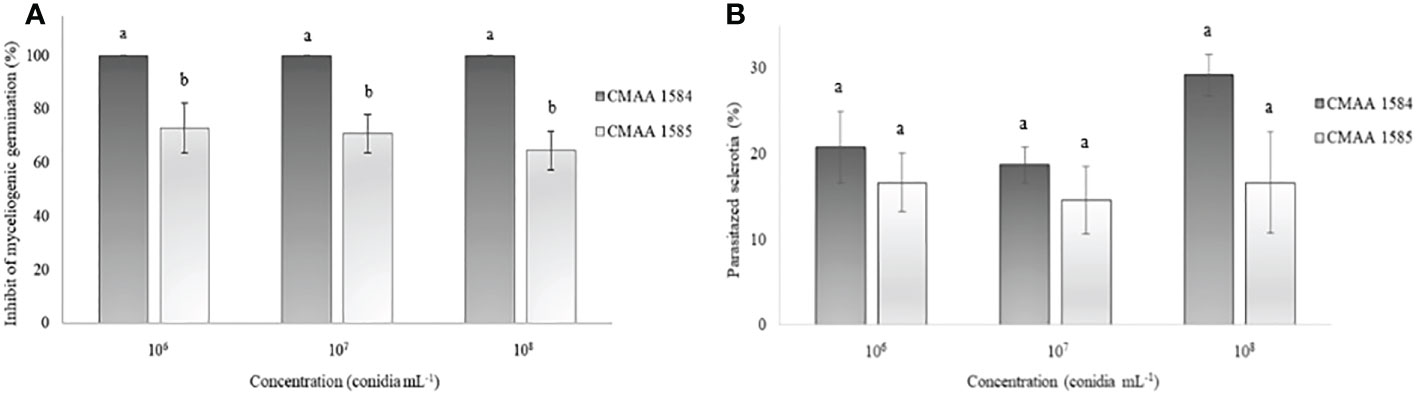
Figure 3 Ability of Trichoderma asperelloides CMAA 1584 and Trichoderma lentiforme CMAA 1585 strains to inhibit myceliogenic germination (A) and to parasitize (B) Sclerotinia sclerotiorum sclerotia by direct antagonism at different inoculum concentrations. *Bars indicate mean (± standard error). Means followed by the same letters do not differ from each other (Tukey test, p < 0.05).
Germination and vigor of cotton seeds treated with Trichoderma
Trichoderma asperelloides CMAA 1584 and T. lentiforme CMAA 1585 applied in cotton seeds, at 1 × 106, 1 × 107, and 1 × 108 conidia mL-1, did not show any significant differences (P > 0.05) for seed germination and initial vigor of cotton seedlings, when compared to control (Table 3).
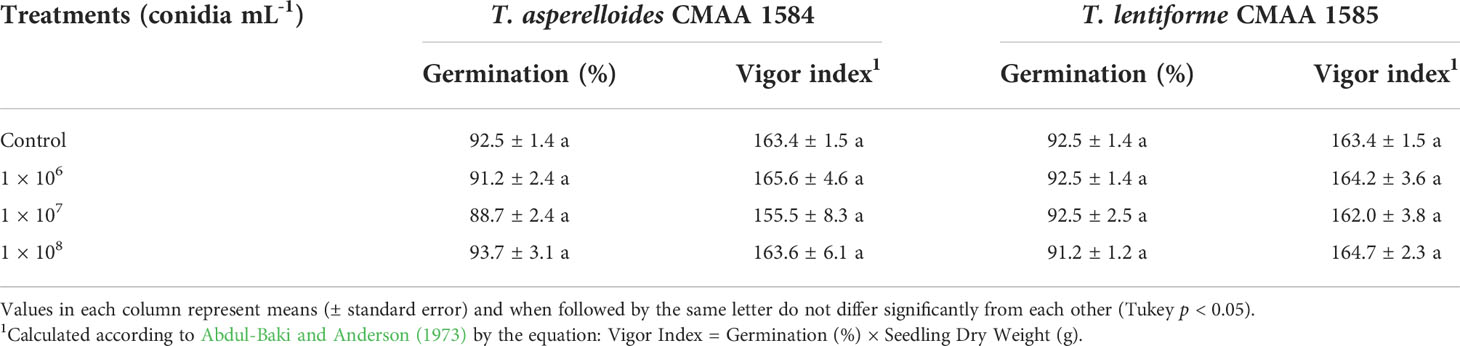
Table 3 Effect of Trichoderma asperelloides CMAA 1584 and Trichoderma lentiforme CMAA 1585 in cotton seeds germination and vigor index.
Effect of Trichoderma strains on cotton growth promotion
Under greenhouse conditions, growth of cotton plants derived from seeds coated with spores of T. asperelloides CMAA 1584 and T. lentiforme CMAA 1585 strains were compared with mock control plants. Notably, T. lentiforme CMAA 1585 outperformed T. asperelloides CMAA 1584 in promoting growth of cotton plants (Tables 4, 5; Figures 4, 5). Looking at T. asperelloides CMAA 1584, this strain incited significant cotton growth promotion only for leaf area (P < 0.05), inducing 98.9% and 42.0% larger leaf area than the mock control plants, when the fungus was applied to seeds at 1 × 107 and 1 × 108 conidia mL-1, respectively (Table 4, Figures 5A, B). Plant height, stem diameter, aboveground and root fresh and dry weights increased (P < 0.05) with the application of T. lentiforme CMAA 1585 at 1 × 108 conidia mL-1 (Table 5, Figure 4). Notably, cotton plants derived from seeds treated with T. lentiforme CMAA 1585 at 1 × 108 conidia mL-1 increased stem diameter, height, aboveground and root fresh and dry weights of 23.7%, 35.2%, 69.3%, 86.7%, 46.0%, and 30.4% (Table 5; Figures 4, 5), when compared to control plants (P < 0.05), respectively.
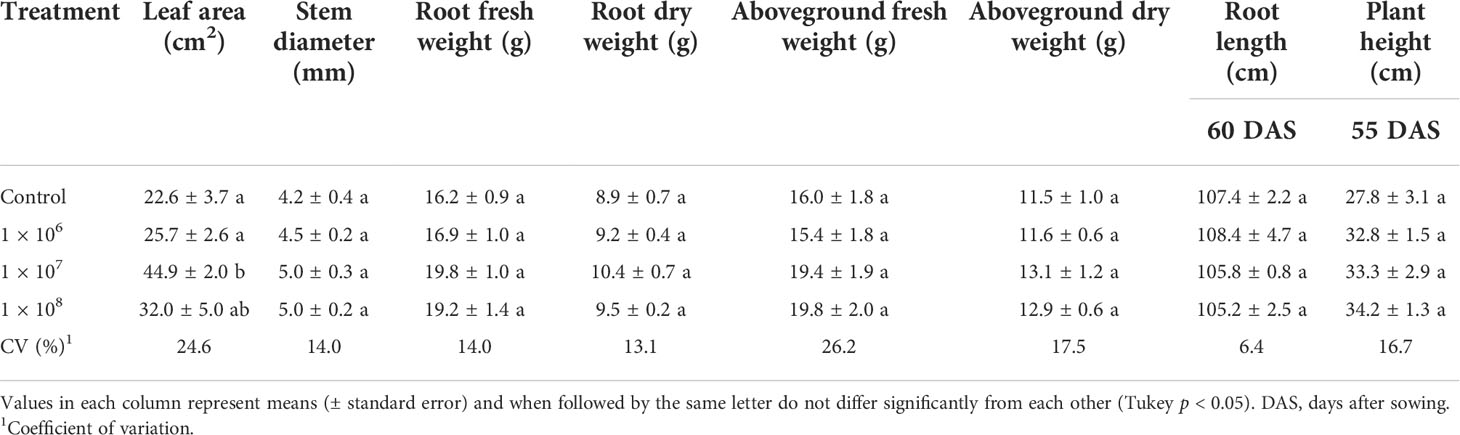
Table 4 Leaf area, stem diameter, root length, plant height and fresh and dry weight of the root and aboveground of cotton plants treated with different concentrations of Trichoderma asperelloides CMAA 1584.
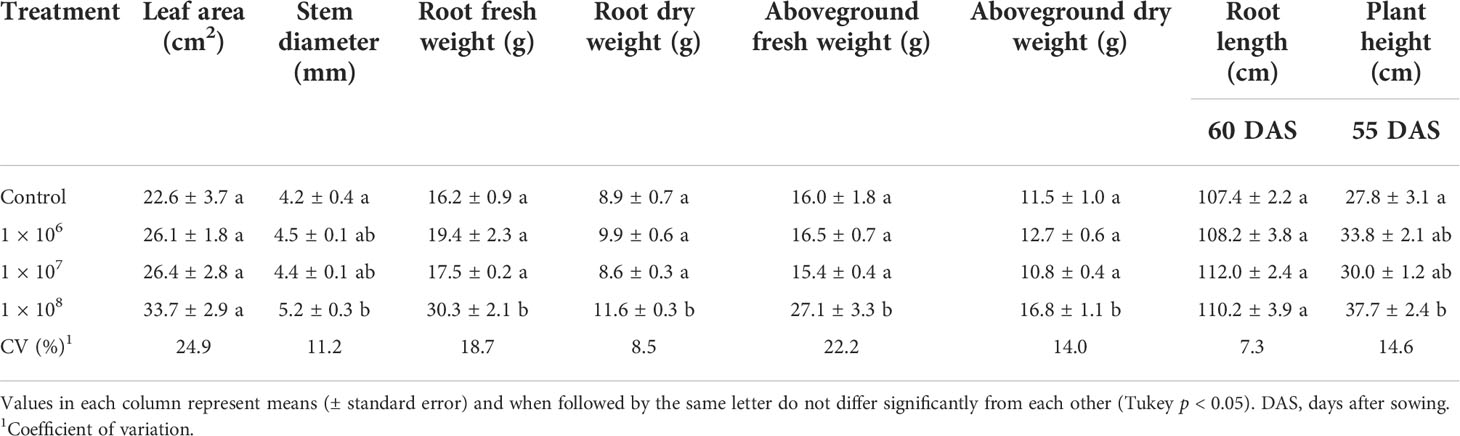
Table 5 Leaf area, stem diameter, root length, height and fresh and dry weight of the root and aboveground of cotton plants treated with different concentrations of Trichoderma lentiforme CMAA 1585.
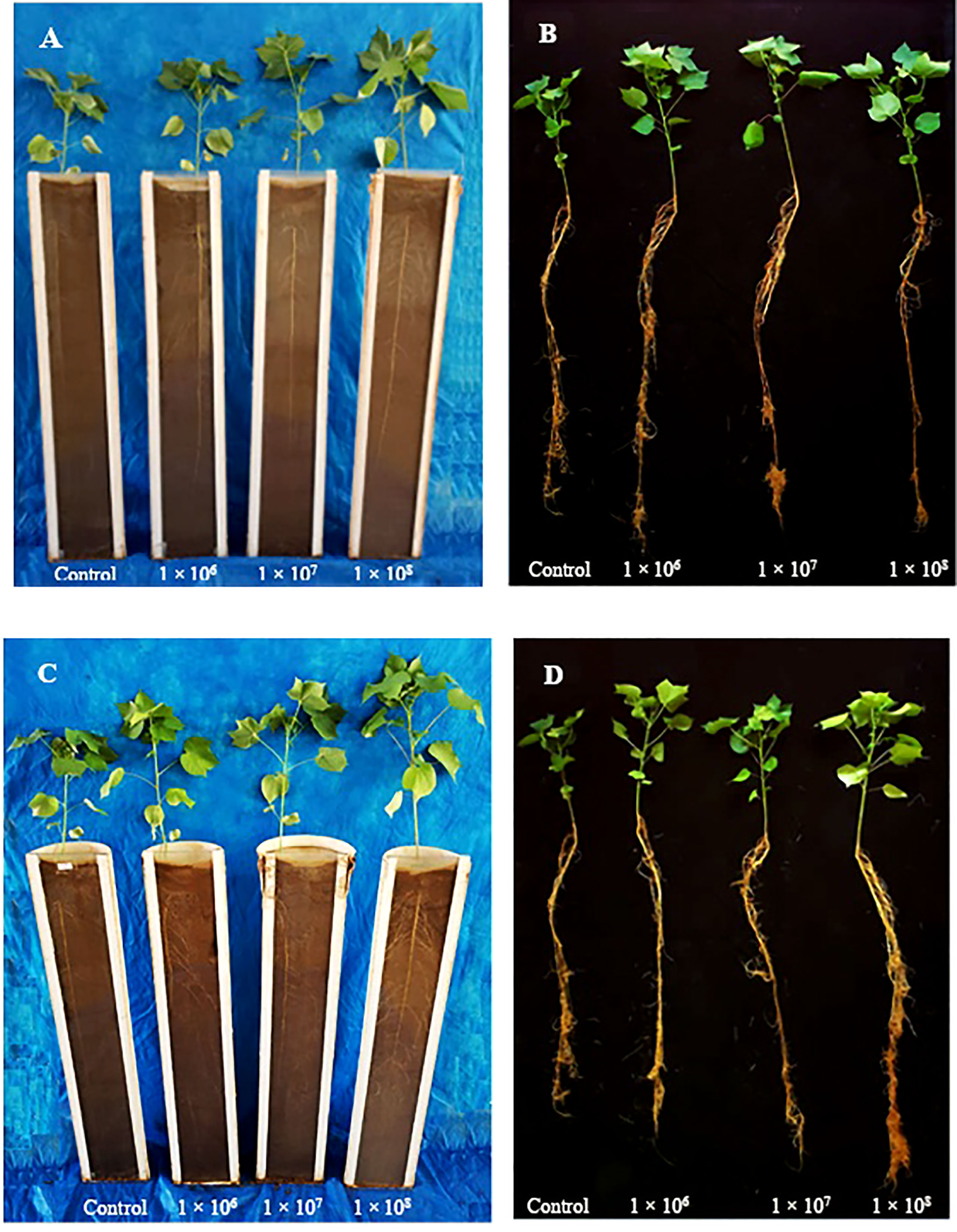
Figure 4 Cotton plants treated with Trichoderma asperelloides CMAA 1584 (A, B) and Trichoderma lentiforme CMAA 1585 (C, D) after 60 days of sowing in rhizotron.
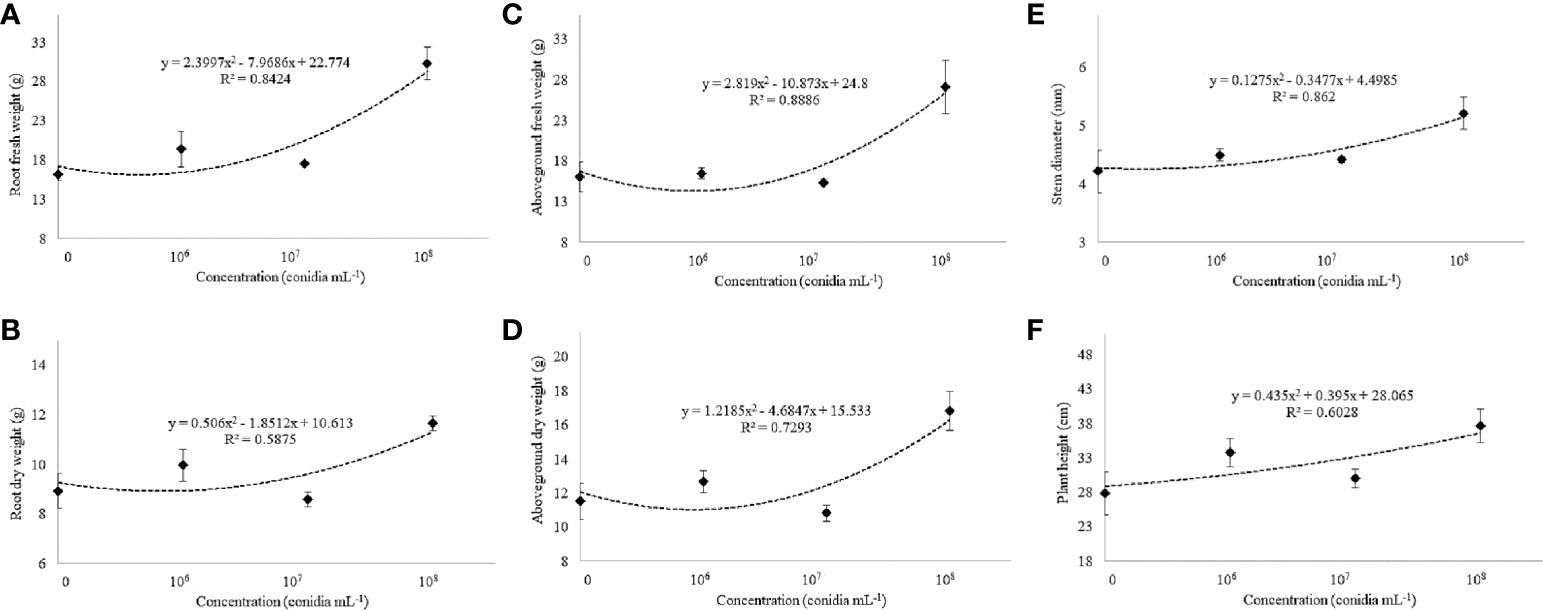
Figure 5 Fresh and dry weight of the root (A, B) and aboveground (C, D), stem diameter (E), and plant height (F) of cotton cultivar FM 975 WS® with Trichoderma lentiforme CMAA 1585. Black diamond symbol represents means (± standard error) and the dashed line represents the fitted quadratic curve.
Discussion
The present study reveals the ability of two indigenous Brazilian strains, T. lentiforme CMAA 1585 and T. asperelloides CMAA 1584 to solubilize inorganic phosphorus, a macronutrient of low availability in tropical soils. Furthermore, these strains are capable of emanating VOCs which inhibit the mycelial growth of S. sclerotiorum. In addition, these strains reduce the growth rate and total number of sclerotia of S. sclerotiorum in dual culture assay, and they inhibit the myceliogenic germination due to degradation of sclerotia through direct parasitism.
The difference in biocontrol performance between the T. lentiforme CMAA 1585 and T. asperelloides CMAA 1584 lies in their ability to suppress the myceliogenic germination of sclerotia, as reported in the present study (Figure 2), which may be related to secondary metabolites, including antifungal compounds, and the direct capacity of parasitism using an arsenal of well-known cuticle-degrading enzymes, where both mechanisms have been correlated with the virulence strategies employed by Trichoderma species (Harman et al., 2004; Geraldine et al., 2013; Monte et al., 2019). Previous studies have shown that T. harzianum, T. koningii, T. pseudokoningii, T. koningiopsis, T. asperellum, T. atroviride, and T. virens displayed excellent inhibitory effect on the myceliogenic germination of S. sclerotiorum in the range of 62% to 100%, when applied directly to the sclerotia (Haddad et al., 2017; Sumida et al., 2018). As noted in our study, evidence of interspecific variation in biocontrol efficacy among Trichoderma spp. is common and should be a key criterion to be incorporated into screening studies for biocontrol of plant pathogens.
There is a tremendous diversity among Trichoderma species and strains in their ability to produce and release biogenic volatile organic compounds (BVOCs) with remarkable roles in mediating plant growth and antagonism towards plant pathogens (Siddiquee et al., 2012; Contreras-Cornejo et al., 2014; Li et al., 2018). In this study, we noted that both of our Trichoderma strains imposed similar detrimental effects on S. sclerotiorum growth under in vitro conditions through emission of VOCs, whose compounds remain elusive. Given the importance of some VOCs emitted by Trichoderma playing pivotal roles in plant growth and biocontrol activity against plant pathogens, further research is needed to elucidate the emission profiles of VOCs by these T. asperelloides and T. lentiforme strains in view of providing new insights and applications of their metabolites in cotton growth enhancement and protection against white mold disease.
Trichoderma asperelloides CMAA 1584 showed a great potential for use in biological control of S. sclerotiorum as it inhibited 100% myceliogenic germination of all sclerotia exposed to their conidia, as well as decreasing the sclerotia formation by 96.1%, when compared to the mock control. According to Atanasova et al. (2013), Trichoderma spp. craft distinct strategies to combat and outcompete other host fungi. These authors observed host sensing in T. atroviride and T. virens through expression of genes involved in the attack, whereas T. reesei was keener to outcompete the pathogen for nutrients. Thus, screening studies for potential biocontrol candidates of Trichoderma can reveal interesting phenotypical traits between species and strains and differential pattern of gene expression linked to biocontrol during the parasitism process of targeted hosts (Atanasova et al., 2013; Troian et al., 2014). Our results strengthen the need to select the antagonist strain according to the desired targeted pathogen taking into account its biology and epidemiology in the crop system (Köhl et al., 2011; Bettiol et al., 2021).
Sclerotia that failed myceliogenic germination and were colonized by Trichoderma were classified as unviable, as this was the same criterion employed by Abdullah et al. (2008) and Görgen et al. (2009). Such mycotrophic lifestyle is one of the most remarkable antagonistic mechanisms expressed by Trichoderma spp. and is implicated in the direct attack of one fungal species to another (Sood et al., 2020). In this sequential process, the first step involves recognition by chemical cues of the targeted pathogenic fungus by Trichoderma, which then its hyphae attach and coil around the prey fungal hyphae (Harman et al., 2004), followed by the onset production of lytic enzymes that cause the dissolution of fungal cell walls (Druzhinina et al., 2011). Hence, it may be expected that antagonists with increased secretion of extracellular enzymes should be responsible for a more pronounced decline in the S. sclerotiorum inoculum levels in soil (Woo et al., 2006).
Among 20 strains of Trichoderma spp. evaluated for management of S. sclerotiorum in common beans, T. asperellum (cryptic sister species of T. asperelloides) exhibited the highest secretion of cell wall-degrading enzymes (CWDE) activity (Lopes et al., 2012). These data are consistent with those observed by Qualhato et al. (2013), where T. asperellum was effective against Fusarium solani, Rhizoctonia solani and S. sclerotiorum, and its antagonistic activity was associated with high activity of chitinase, β-1,3-glucanase and acid phosphatases. In our study, T. asperelloides CMAA 1584 displayed a great ability to inhibit myceliogenic germination and further degrade sclerotia of S. sclerotiorum by direct parasitism, outperforming T. lentiforme CMAA 1585 in this particular attribute. According to Geraldine et al. (2013), NAGase (N-β-acetylglucosaminidase) and β-1,3-glucanase enzymes play a key role in reducing the number of apothecia and the chain of events in the field that account for white mold severity, underlining the importance of these CWDEs in the control of white mold.
On the other hand, T. lentiforme CMAA 1585 demonstrates to be more suitable as a biostimulant due to its ability to boost growth of cotton plants (Table 5, Figures 4 and 5). The high phosphate solubilization in the soil (Table 1) displayed by this strain and better development of cotton roots are possible mechanisms associated with plant growth enhancement. Many authors have detailed the ability of Trichoderma spp. to modulate physiological, biochemical, and molecular mechanisms in a wide assortment of plants under various growth conditions (Hermosa et al., 2013; Rubio et al., 2014; Rubio et al., 2017; Elkelish et al., 2020), by the production phytohormones and a plethora of secondary metabolites (Jaroszuk-Ściseł et al., 2019).
Using in vitro bioassay, Contreras-Cornejo et al. (2009) showed that T. virens Gv29.8 and T. atroviride IMI206040 can synthesize indole-acetic acid [IAA] (and some of its derivatives), and suggests that the higher lateral root development observed in Arabidopsis wildtype plants is mediated by auxins. IAA synthesized by plant root-associated microorganisms can interfere with plant development by disturbing the auxin balance in plants, which can modify root architecture, increase root mass, and consequently, increase nutrient uptake by well-developed root system (Contreras-Cornejo et al., 2009). Sofo et al. (2011) reported that cherry rootstocks treated with T. harzianum commercial strain T-22 resulted in increased root and shoot growth by 76% and 61%, respectively. Furthermore, in mass spectrometry analyses these authors found that IAA and gibberellic acid (GA) levels were significantly increased by 40% and 143% in the roots, and by 49% and 71% in leaves, respectively.
Gravel et al. (2007) suggested that growth promotion, in tomato seedling, is associated with the reduced ethylene (ET) production resulting from a decrease in its precursor 1-aminocyclopropane-1-carboxylic acid (ACC), and/or through the ACC deaminase (ACCD) activity present in the microorganism. Another possible mechanism arises from increased plant tolerance to abiotic stresses and/or by mitigation of damages caused by the accumulation of reactive oxygen species (ROS) in stressed plants (Mastouri et al., 2010). Thus, it can be hypothesized that the absence of significant results (P > 0.05) in seed germination and seedling vigor index in our assay under laboratory conditions, using the germitest paper method, the growth promotion may be related to the absence of environmental stresses. When evaluating the germination and vigor of wheat seedlings (Triticum aestivum L.) after seed treatment with different strains of Trichoderma spp., Anjum et al. (2020) obtained results that corroborate this scenario, because in a greenhouse trial, the positive outcomes were significantly more expressive than those observed in in vitro test conducted in the laboratory. In the present study, although the germination index was similar for these Trichoderma strains in both growth conditions, cotton plants from the mock control group exhibited less growth in the greenhouse trial when compared with plants derived from the Trichoderma treatments, most likely due to the exposition of mock plants to suboptimal environmental conditions in contrast to higher resilience and improved growth promotion afforded by Trichoderma as a biological inoculant.
Both Trichoderma strains may be further tested under field conditions, but with different purposes. As such, we propose that T. asperelloides CMAA 1584 should be designated to control sclerotia of S. sclerotiorum, while T. lentiforme CMAA 1585 would assume a role as a biostimuant due to its ability to promote better growth of cotton plants. Overall, these selected Trichoderma strains are suitable for application in consortium targeting both pathogen control and growth promotion in cotton crops with a consequent contribution to diminishing the reliance on chemical fertilizers and fungicides.
Data availability statement
The datasets presented in this study can be found in online repositories. The names of the repository/repositories and accession number(s) can be found in the article/supplementary material.
Author contributions
WB, GM, and LG conceived and designed the laboratory and greenhouse experiments. LG, RC, and PS performed the laboratory and greenhouse experiments, and analyzed the data. WB and GM contributed with reagents/materials/analysis tools. CD provided critical analysis and editorial enhancements. All authors wrote the manuscript. All authors read and approved the final manuscript.
Funding
This study was supported by Empresa Brasileira de Pesquisa Agropecuária (Embrapa SEG 20.19.02.006.00.00) and Coordenação de Aperfeiçoamento de Pessoal de Nível Superior - Brasil (CAPES) - Finance Code 001. Wagner Bettiol (CNPq 307855/2019-8) acknowledges Conselho Nacional de Desenvolvimento Científico e Tecnológico – CNPq for the productivity fellowship. This work was supported in part by the U.S. Department of Agriculture, Agricultural Research Service (Project Number: 5010-22410-024-00-D).
Conflict of interest
The authors declare that the research was conducted in the absence of any commercial or financial relationships that could be construed as a potential conflict of interest.
Publisher’s note
All claims expressed in this article are solely those of the authors and do not necessarily represent those of their affiliated organizations, or those of the publisher, the editors and the reviewers. Any product that may be evaluated in this article, or claim that may be made by its manufacturer, is not guaranteed or endorsed by the publisher.
Author disclaimer
Any opinions, findings, conclusions, or recommendations expressed in this publication are those of the author(s) and do not necessarily reflect the view of the U.S. Department of Agriculture. The mention of firm names or trade products does not imply they are endorsed or recommended by the USDA over other firms or similar products not mentioned. USDA is an equal opportunity provider and employer.
References
Abdul-Baki, A. A., Anderson, J. D. (1973). Vigor determination in soybean seed by multiple criteria 1. Crop Sci. 13, 630–633. doi: 10.2135/cropsci1973.0011183X001300060013x
Abdullah, M. T., Ali, N. Y., Suleman, P. (2008). Biological control of Sclerotinia sclerotiorum (Lib.) de bary with Trichoderma harzianum and Bacillus amyloliquefaciens. Crop Prot. 27, 1354–1359. doi: 10.1016/j.cropro.2008.05.007
ABRAPA - Associação Brasileira dos Produtores de Algodão (2021) Algodão no brasil. Available at: https://www.abrapa.com.br/Paginas/Dados/Algod%C3%A3o%20no%20Brasil.aspx (Accessed February 13, 2021).
Anjum, Z. A., Hayat, S., Ghazanfar, M. U., Ahmad, S., Adnan, M., Hussian, I. (2020). Does seed priming with Trichoderma isolates have any impact on germination and seedling vigor of wheat. Int. J. Bot. Stud. 5, 65–68. Available at: https://www.google.com/url?sa=t&rct=j&q=&esrc=s&source=web&cd=&ved=2ahUKEwjM94Trqb36AhWmRDABHbiuCaYQFnoECAUQAQ&url=http%3A%2F%2Fwww.botanyjournals.com%2Fdownload%2F542%2F5-1-38-125.pdf&usg=AOvVaw3zV2o5XvyfkcMOg4tYnTiF
Atanasova, L., Le Crom, S., Gruber, S., Coulpier, F., Seidl-Seiboth, V., Kubicek, C. P., et al. (2013). Comparative transcriptomics reveals different strategies of Trichoderma mycoparasitism. BMC Genomics 14, 121. doi: 10.1186/1471-2164-14-121
Barroso, P. A. V., Suassuna, N. D., Pedrosa, M. B., Morello, C., de, L., da Silva Filho, J. L., et al. (2017). BRS 368RF: A glyphosate tolerant, midseason upland cotton cultivar for northeast and north Brazilian cerrado. Crop Breed. Appl. Biotechnol. 17, 399–402. doi: 10.1590/1984-70332017v17n4c59
Bell, D. K., Wells, W. D., Markham, C. R. (1982). In vitro antagonism of Trichoderma species against six fungal plant pathogens. Phytopathology 72, 379. doi: 10.1094/Phyto-72-379
Bettiol, W., de Medeiros, F. H. V., Barros Chiaramonte, J., Mendes, R. (2021). “Advances in screening approaches for the development of microbial bioprotectants to control plant diseases,” in Microbial bioprotectants for plant disease management. Eds. Köhl, J., Ravensberg, W. (London: Burleigh Dodds Science Publishing), 33–86. doi: 10.19103/AS.2021.0093.02
Boland, G. J., Hall, R. (1994). Index of plant hosts of Sclerotinia sclerotiorum. Can. J. Plant Pathol. 16, 93–108. doi: 10.1080/07060669409500766
Brazil (2015) Portaria n 5, de 21 de agosto de 2015 - diário oficial da união no 161 (Brasília). Available at: https://www.in.gov.br/web/guest/materia/-/asset_publisher/Kujrw0TZC2Mb/content/id/32414403/do1-2015-08-24-portaria-n-5-de-21-de-agosto-de-2015-3241423 (Accessed February 15, 2021). DF.
Brotman, Y., Lisec, J., Méret, M., Chet, I., Willmitzer, L., Viterbo, A. (2012). Transcript and metabolite analysis of the Trichoderma-induced systemic resistance response to Pseudomonas syringae in Arabidopsis thaliana. Microbiology 158, 139146. doi: 10.1099/mic.0.052621-0
Charchar, M. J. D., dos Anjos, J. R. N., Ossipi, E. (1999). Ocorrência de nova doença do algodoeiro irrigado, no brasil, causada por Sclerotinia sclerotiorum. Pesqui. Agropecu. Bras. 34, 1100–1106. doi: 10.1590/S0100-204X1999000600024
Chaverri, P., Branco-Rocha, F., Jaklitsch, W., Gazis, R., Degenkolb, T., Samuels, G. J. (2015). Systematics of the Trichoderma harzianum species complex and the re-identification of commercial biocontrol strains. Mycologia 107, 558–590. doi: 10.3852/14-147
CONAB - Companhia Nacional de Abastecimento (2022) Acompanhamento da safra brasileira de grãos - 11° levantamento. Available at: https://www.conab.gov.br/info-agro/safras/graos/boletim-da-safra-de-grao (Accessed August 13, 2022).
Contreras-Cornejo, H. A., Macías-Rodríguez, L., Cortés-Penagos, C., López-Bucio, J. (2009). Trichoderma virens, a plant beneficial fungus, enhances biomass production and promotes lateral root growth through an auxin-dependent mechanism in Arabidopsis. Plant Physiol. 149, 1579–1592. doi: 10.1104/pp.108.130369
Contreras-Cornejo, H. A., Macias-Rodriguez, L., Herrera-Estrella, A., Lopez-Bucio, J. (2014). The 4-phosphopantetheinyl transferase of Trichoderma virens plays a role in plant protection against Botrytis cinerea through volatile organic compound emission. Plant Soil 379, 261–274. doi: 10.1007/s11104-014-2069-x
Druzhinina, I. S., Seidl-Seiboth, V., Herrera-Estrella, A., Horwitz, B. A., Kenerley, C. M., Monte, E., et al. (2011). Trichoderma: the genomics of opportunistic success. Nat. Rev. Microbiol. 9, 749–759. doi: 10.1038/nrmicro2637
Elias, L. M., Domingues, M. V. P. F., de Moura, K. E., Harakava, R., Patricio, F. R. A. (2016). Selection of Trichoderma isolates for biological control of Sclerotinia minor and S. sclerotiorum in lettuce. Summa Phytopathol. 42, 216–221. doi: 10.1590/0100-5405/2147
Elkelish, A. A., Alhaithloul, H. A. S., Qari, S. H., Soliman, M. H., Hasanuzzaman, M. (2020). Pretreatment with Trichoderma harzianum alleviates waterlogging-induced growth alterations in tomato seedlings by modulating physiological, biochemical, and molecular mechanisms. Environ. Exp. Bot. 171, 103946. doi: 10.1016/j.envexpbot.2019.103946
Ferraz, L., de, C. L., Nasser, L. C. B., Café-Filho, A. C. (2011). Viabilidade de escleródios de Sclerotinia sclerotiorum e incidência de fungos antagonistas em solo de cerrado. Summa Phytopathol. 37, 208–210. doi: 10.1590/S0100-54052011000400009
Garcia, R. A., Juliatti, F. C., Cassemiro, T. A. (2012). Production of sclerotia on Sclerotinia sclerotiorum (Lib.) de bary in culture media. Biosci. J. 28, 1–7. Available at: https://www.researchgate.net/publication/285674900_Production_of_sclerotia_on_sclerotinia_sclerotiorum_LIB_De_Bary_in_culture_media
Geraldine, A. M., Lopes, F. A. C., Carvalho, D. D. C., Barbosa, E. T., Rodrigues, A. R., Brandão, R. S., et al. (2013). Cell wall-degrading enzymes and parasitism of sclerotia are key factors on field biocontrol of white mold by trichoderma spp. Biol. Control 67, 308–316. doi: 10.1016/j.biocontrol.2013.09.013
Görgen, C. A., da Silveira Neto, A. N., Carneiro, L. C., Ragagnin, V., Lobo Junior, M. (2009). Controle do mofo-branco com palhada e Trichoderma harzianum 1306 em soja. Pesqui. Agropecu. Bras. 44, 1583–1590. doi: 10.1590/S0100-204X2009001200004
Gravel, V., Antoun, H., Tweddell, R. J. (2007). Growth stimulation and fruit yield improvement of greenhouse tomato plants by inoculation with Pseudomonas putida or Trichoderma atroviride: Possible role of indole acetic acid (IAA). Soil Biol. Biochem. 39, 1968–1977. doi: 10.1016/j.soilbio.2007.02.015
Grimes, D. W., Carter, L. M. (1969). A linear rule for direct nondestructive leaf area measurements 1. Agron. J. 61, 477–479. doi: 10.2134/agronj1969.00021962006100030048x
Haddad, P. E., Leite, L. G., Lucon, C. M. M., Harakava, R. (2017). Selection of Trichoderma spp. strains for the control of Sclerotinia sclerotiorum in soybean. Pesqui. Agropecu. Bras. 52, 1140–1148. doi: 10.1590/s0100-204x2017001200002
Harman, G. E., Howell, C. R., Viterbo, A., Chet, I., Lorito, M. (2004). Trichoderma species - opportunistic, avirulent plant symbionts. Nat. Rev. Microbiol. 2, 43–56. doi: 10.1038/nrmicro797
Henis, Y., Adams, P. B., Lewis, J. A., Papavizas, G. C. (1983). Penetration of sclerotia of Sclerotium rolfsii by Trichoderma spp. Phytopathology 73, 1043–1046. doi: 10.1094/Phyto-73-1043
Hermosa, R., Rubio, M. B., Cardoza, R. E., Nicolás, C., Monte, E., Gutiérrez, S. (2013). The contribution of Trichoderma to balancing the costs of plant growth and defense. Internat. Microbiol. 16, 69–80. doi: 10.2436/20.1501.01.181
Hermosa, R., Viterbo, A., Chet, I., Monte, E. (2012). Plant-beneficial effects of Trichoderma and of its genes. Microbiology 158, 17–25. doi: 10.1099/mic.0.052274-0
IMEA - Instituto Mato-Grossense de Economia Agropecuária (2019) Custos de produção do algodoeiro no mato grosso. Available at: https://www.imea.com.br/imea-site/relatorios-mercado-detalhe?c=1&s=3 (Accessed January 2, 2020).
IMEA - Instituto Mato-Grossense de Economia Agropecuária (2021) 5a estimativa da safra 2020/21: Algodão. Available at: http://www.imea.com.br/imea-site/relatorios-mercado-detalhe?c=1&s=9 (Accessed January 2, 2021).
Jaroszuk-Ściseł, J., Tyśkiewicz, R., Nowak, A., Ozimek, E., Majewska, M., Hanaka, A., et al. (2019). Phytohormones (auxin, gibberellin) and ACC deaminase in vitro synthesized by the mycoparasitic Trichoderma DEMTkZ3A0 strain and changes in the level of auxin and plant resistance markers in wheat seedlings inoculated with this strain conidia. Int. J. Mol. Sci. 20, 4923. doi: 10.3390/ijms20194923
Kniss, A. R. (2017). Long-term trends in the intensity and relative toxicity of herbicide use. Nat. Commun. 8, 14865. doi: 10.1038/ncomms14865
Köhl, J., Postma, J., Nicot, P., Ruoco, M., Blum, B. (2011). Stepwise screening of microorganisms for commercial use in biological control of plant-pathogenic fungi and bacteria. Biol. Control 57, 1–12. doi: 10.1016/j.biocontrol.2010.12.004
Komárek, M., Čadková, E., Chrastný, V., Bordas, F., Bollinger, J.-C. (2010). Contamination of vineyard soils with fungicides: A review of environmental and toxicological aspects. Environ. Int. 36, 138–151. doi: 10.1016/j.envint.2009.10.005
Li, N., Alfiky, A., Wang, W., Islam, M., Nourollahi, K., Liu, X., et al. (2018). Volatile compound-mediated recognition and inhibition between Trichoderma biocontrol agents and Fusarium oxysporum. Front. Microbiol. 9. doi: 10.3389/fmicb.2018.026
Liang, H.-J., Di, Y.-L., Li, J.-L., Zhu, F.-X. (2015). Baseline sensitivity and control efficacy of fluazinam against Sclerotinia sclerotiorum. Eur. J. Plant Pathol. 142, 691–699. doi: 10.1007/s10658-015-0644-5
Li, G. Q., Huang, H. C., Acharya, S. N., Erickson, R. S. (2005). Effectiveness of Coniothyrium minitans and Trichoderma atroviride in suppression of Sclerotinia blossom blight of alfalfa. Plant Pathol. 54, 204–211. doi: 10.1111/j.1365-3059.2005.01119.x
Lopes, F. A. C., Steindorff, A. S., Geraldine, A. M., Brandão, R. S., Monteiro, V. N., Lobo Júnior, M., et al. (2012). Biochemical and metabolic profiles of Trichoderma strains isolated from common bean crops in the Brazilian cerrado, and potential antagonism against Sclerotinia sclerotiorum. Fungal Biol. 116, 815–824. doi: 10.1016/j.funbio.2012.04.015
Lorito, M., Woo, S. L., Harman, G. E., Monte, E. (2010). Translational research on Trichoderma : From ‘omics to the field. Annu. Rev. Phytopathol. 48, 395–417. doi: 10.1146/annurev-phyto-073009-114314
Mao, X. W., Li, J. S., Chen, Y. L., Song, X. S., Duan, Y. B., Wang, J. X., et al. (2018). Resistance risk assessment for fluazinam in Sclerotinia sclerotiorum. Pestic. Biochem. Phys. 144, 27–35. doi: 10.1016/j.pestbp.2017.10.010
Mastouri, F., Björkman, T., Harman, G. E. (2010). Seed treatment with Trichoderma harzianum alleviates biotic, abiotic, and physiological stresses in germinating seeds and seedlings. Phytopathology 100, 1213–1221. doi: 10.1094/PHYTO-03-10-0091
Meyer, M. C., Campos, H. D., Godoy, C. V., Utiamada, C. M. (2014). Ensaios cooperativos de controle químico de mofo branco na cultura da soja: safras 2009 a 2012 (Londrina:Embrapa Soybean). Available at: https://ainfo.cnptia.embrapa.br/digital/bitstream/item/101371/1/Ensaios-cooperativos-de-controle-quimico-de-mofo-branco-na-cultura-da-soja-safras-2009-a-2012.pdf.
Meyer, M. C., Campos, H. D., Godoy, C. V., Utiamada, C. M., Sato, L. N., Dias, A. R., et al. (2020). Eficiência de fungicidas para controle de mofo-branco (Sclerotinia sclerotiorum) em soja, na safra 2019/20: Resultados sumarizados dos ensaios cooperativos (Londrina:Embrapa Soybean). Available at: http://ainfo.cnptia.embrapa.br/digital/bitstream/item/148978/1/CT-122.pdf.
Monte, E., Bettiol, W., Hermosa, R. (2019). “Trichoderma e seus mecanismos de ação para o controle de doenças de plantas,” in Trichoderma: Usos na agricultura. Eds. Meyer, M. C., Mazaro, S. M., da Silva, J. C. (Brasília: Embrapa Soja), 181–200.
Monte, E., Hermosa, R. (2021). “The use of trichoderma spp. to control plant diseases,” in Microbial bioprotectants for plant disease management. Eds. Köhl, J., Ravensberg, W.(London:Burleigh Dodds Science Publishing), 401–428. doi: 10.19103/AS.2021.0093.13
Morello, C. de L., Suassuna, N. D., Barroso, P. A. V., da Silva Filho, J. L., de Barcellos Ferreira, A. C., Lamas, F. M., et al. (2015). BRS 369RF and BRS 370RF: Glyphosate tolerant, high-yielding upland cotton cultivars for central Brazilian savanna. Crop Breed. Appl. Biotechnol. 15, 290–294. doi: 10.1590/1984-70332015v15n4c49
Murphy, J., Riley, J. P. (1962). A modified single solution method for the determination of phosphate in natural waters. Anal. Chim. Acta 27, 31–36. doi: 10.1016/S0003-2670(00)88444-5
Muthukumar, A., Eswaran, A., Sanjeevkumas, K. (2011). Exploitation of Trichoderma species on the growth of Pythium aphanidermatum in chilli. Braz. J. Microbiol. 42, 1598–1607. doi: 10.1590/S1517-83822011000400047
Napoleão, R., Nasser, L., Lopes, C., Café Filho, A. (2006). Neon-s, novo meio para detecção de Sclerotinia sclerotiorum em sementes. Summa Phytopathol. 32, 180–182. doi: 10.1590/S0100-54052006000200014
Nautiyal, C. S. (1999). An efficient microbiological growth medium for screening phosphate solubilizing microorganisms. FEMS Microbiol. Lett. 170, 265–270. doi: 10.1111/j.1574-6968.1999.tb13383.x
O’Sullivan, C. A., Belt, K., Thatcher, L. F. (2021). Tackling control of a cosmopolitan phytopathogen: Sclerotinia. Front. Plant Sci. 12, 1764. doi: 10.3389/fpls.2021.707509
Qualhato, T. F., Lopes, F. A. C., Steindorff, A. S., Brandão, R. S., Jesuino, R. S. A., Ulhoa, C. J. (2013). Mycoparasitism studies of Trichoderma species against three phytopathogenic fungi: evaluation of antagonism and hydrolytic enzyme production. Biotechnol. Let. 35, 1461–1468. doi: 10.1007/s10529-013-1225-3
Rubio, M. B., Hermosa, R., Vicente, R., Gómez-Acosta, F. A., Morcuende, R., Monte, E., et al. (2017). The combination of Trichoderma harzianum and chemical fertilization leads to the deregulation of pytohormone networking, preventing the adaptive responses of tomato plants to salt stress. Front. Plant Sci. 8. doi: 10.3389/fpls.2017.00294
Rubio, M. B., Quijada, N. M., Pérez, E., Domínguez, S., Monte, E., Hermosa, R. (2014). Identifying beneficial qualities of Trichoderma parareesei for plants. Appl. Environ. Microbiol. 80, 1864–1873. doi: 10.1128/AEM.03375-13
Samuels, G. J., Ismaiel, A., Bon, M. C., De Respinis., S., Petrini, O. (2010). Trichoderma asperellum sensu lato consists of two cryptic species. Mycologia 102, 4, 944–4, 966. doi: 10.3852/09-243
Schwartz, H. F., Singh, S. P. (2013). Breeding common bean for resistance to white mold: A review. Crop Sci. 53, 1832–1844. doi: 10.2135/cropsci2013.02.0081
Sharma, P., Sain, S. K. (2010). Induction of systemic resistance in tomato and cauliflower by Trichoderma spp. against stalk rot pathogen, Sclerotinia sclerotiorum (Lib) de bary. J. Biol. Control 18, 21–28. doi: 10.18311/jbc/2004/4042
Siddiquee, S., Cheong, B. E., Taslima, K., Kausar, H., Hasan, M. M. (2012). Separation and identification of volatile compounds from liquid cultures of Trichoderma harzianum by GC-MS using three different capillary columns. J. Chromatogr. Sci. 50, 358–367. doi: 10.1093/chromsci/bms012
Silva, J. C., Bettiol, W., Suassuna, N. D. (2019). Ramularia leaf spot: an emergent disease of cotton in Brazil. Trop. Plant Pathol. 44, 473–482. doi: 10.1007/s40858-019-00308-w
Silva Neto, S.P.da, Pereira, A. F., Morello, C.de L., Suassuna, N. D. (2016). “Melhoramento clássico e biotecnologia visando à superação de desafios,” in Desafios do cerrado: Como sustentar a expansão da produção com produtividade e competitividade. associação mato-grossense dos produtores de algodão. Eds. Piccoli, G. V., Endrigo, D., Maurício, A. L. (Cuiabá: Associação mato-grossense dos produtores de algodão), 215–252.
Sivakumar, S., Prem Kumar, G., Vinoth, S., Siva, G., Vigneswaran, M., Gurusaravanan, P., et al. (2021). Temporal expression profiling of GhNAC transcription factor genes in cotton cultivars under abiotic stresses. Plant Gene 28, 100334. doi: 10.1016/j.plgene.2021.100334
Smolińska, U., Kowalska, B. (2018). Biological control of the soil-borne fungal pathogen Sclerotinia sclerotiorum - a review. J. Plant Pathol. 100, 1–12. doi: 10.1007/s42161-018-0023-0
Sofo, A., Scopa, A., Manfra, M., De Nisco, M., Tenore, G., Troisi, J., et al. (2011). Trichoderma harzianum strain T-22 induces changes in phytohormone levels in cherry rootstocks (Prunus cerasus × P. canescens). Plant Growth Regul. 65, 421–425. doi: 10.1007/s10725-011-9610-1
Sood, M., Kapoor, D., Kumar, V., Sheteiwy, M. S., Ramakrishnan, M., Landi, M., et al. (2020). Trichoderma: The “secrets” of a multitalented biocontrol agent. Plants 9, 762. doi: 10.3390/plants9060762
Suassuna, N. D., Silva, J. C., Bettiol, W. (2019). “Uso do trichoderma na cultrua do algodão,” in Trichoderma: Usos na agricultura. Eds. Meyer, M. C., Mazaro, S. M., da Silva, J. C. (Brasília: Embrapa Soja), 361–379.
Sumida, C. H., Daniel, J. F. S., Araujod, A. P. C. S., Peitl, D. C., Abreu, L. M., Dekker, R. F. H., et al. (2018). Trichoderma asperelloides antagonism to nine Sclerotinia sclerotiorum strains and biological control of white mold disease in soybean plants. Biocontrol Sci. Technol. 28, 142–156. doi: 10.1080/09583157.2018.1430743
Tarazi, R., Jimenez, J. L. S., Vaslin, M. F. S. (2019). Biotechnological solutions for major cotton (Gossypium hirsutum) pathogens and pests. Biotechnol. Res. Innovation 3, 19–26. doi: 10.1016/j.biori.2020.01.001
Tourneau, D. (1979). Morphology, cytology, and physiology of sclerotinia species in culture. Phytopathology 69, 887–890. doi: 10.1094/Phyto-69-887
Troian, R. F., Steindorff, A. S., Ramada, M. H. S., Arruda, W., Ulhoa, C. J. (2014). Mycoparasitism studies of Trichoderma harzianum against Sclerotinia sclerotiorum: evaluation of antagonism and expression of cell wall-degrading enzymes genes. Biotechnol. Lett. 36, 2095–2101. doi: 10.1007/s10529-014-1583-5
Verma, M., Brar, S. K., Tyagi, R. D., Surampalli, R. Y., Valéro, J. R. (2007). Antagonistic fungi, Trichoderma spp.: Panoply of biological control. Biochem. Eng. J. 37, 1–20. doi: 10.1016/j.bej.2007.05.012
Woo, S. L., Scala, F., Ruocco, M., Lorito, M. (2006). The molecular biology of the interactions between Trichoderma spp., phytopathogenic fungi, and plants. Phytopathology 96, 181–185. doi: 10.1094/PHYTO-96-0181
Wu, N., Yang, J., Wang, G., Ke, H., Zhang, Y., Liu, Z., et al. (2022). Novel insights into water-deficit-responsive mRNAs and lncRNAs during fiber development in Gossypium hirsutum. BMC Plant Biol. 22, 1–16. doi: 10.1186/s12870-021-03382-y
Keywords: bioprotectant, biofungicide, white mold, biofertilizer, phosphate solubilization ability
Citation: Silva LG, Camargo RC, Mascarin GM, Nunes PSO, Dunlap C and Bettiol W (2022) Dual functionality of Trichoderma: Biocontrol of Sclerotinia sclerotiorum and biostimulant of cotton plants. Front. Plant Sci. 13:983127. doi: 10.3389/fpls.2022.983127
Received: 30 June 2022; Accepted: 20 September 2022;
Published: 07 October 2022.
Edited by:
Pablo Velasco, Biological Mission of Galicia (CSIC), SpainReviewed by:
Muthukrishnan Sathiyabama, Bharathidasan University, IndiaOswaldo Valdes-Lopez, National Autonomous University of Mexico, Mexico
Copyright © 2022 Silva, Camargo, Mascarin, Nunes, Dunlap and Bettiol. This is an open-access article distributed under the terms of the Creative Commons Attribution License (CC BY). The use, distribution or reproduction in other forums is permitted, provided the original author(s) and the copyright owner(s) are credited and that the original publication in this journal is cited, in accordance with accepted academic practice. No use, distribution or reproduction is permitted which does not comply with these terms.
*Correspondence: Gabriel Moura Mascarin, Z2FicmllbC5tYXNjYXJpbkBlbWJyYXBhLmJy