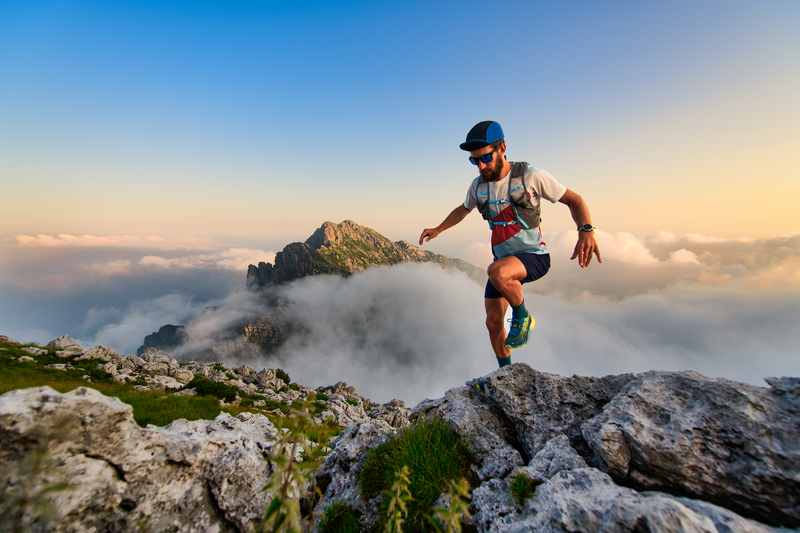
95% of researchers rate our articles as excellent or good
Learn more about the work of our research integrity team to safeguard the quality of each article we publish.
Find out more
ORIGINAL RESEARCH article
Front. Plant Sci. , 07 November 2022
Sec. Plant Abiotic Stress
Volume 13 - 2022 | https://doi.org/10.3389/fpls.2022.982610
This article is part of the Research Topic Subcellular Compartmentalization of Plant Antioxidants and ROS Generating Systems - Volume II View all 6 articles
The reactive oxygen species singlet oxygen, 1O2, has an extremely short half-life, yet is intimately involved with stress signalling in the cell. We previously showed that the effects of 1O2 on the transcriptome are highly correlated with 80S ribosomal arrest due to oxidation of guanosine residues in mRNA. Here, we show that dysregulation of chlorophyll biosynthesis in the flu mutant or through feeding by δ-aminolevulinic acid can lead to accumulation of photoactive chlorophyll intermediates in the cytoplasm, which generates 1O2 upon exposure to light and causes the oxidation of RNA, eliciting 1O2-responsive genes. In contrast, transcriptomes derived from DCMU treatment, or the Ch1 mutant under moderate light conditions display commonalties with each other but do not induce 1O2 gene signatures. Comparing 1O2 related transcriptomes to an index transcriptome induced by cycloheximide inhibition enables distinction between 1O2 of cytosolic or of plastid origin. These comparisons provide biological insight to cases of mutants or environmental conditions that produce 1O2.
Reactive oxygen species (ROS) were previously thought to be by-products of cellular dysfunction, and their release exacerbated damage to cellular components, hastening cellular demise. In recent years, ROS have also been found to be produced in a regulated fashion and participate in multifarious cell signalling pathways. H2O2 is the most stable ROS and is regarded for its ability to take part in redox reactions [reviewed in (Foyer, 2018)]. The superoxide ROS radical () has been shown to be produced in significant quantities in both chloroplasts and mitochondria, as a result of electron transport in photosynthesis and respiration, respectively. Additionally, it is produced by NADPH oxidases in various organelle membranes in response to pathogenic attack or during development [reviewed in (Kadota et al., 2015)], Such activity can trigger an organism-wide ‘ROS wave’ reminiscent of systemic signalling in the nervous system in animals (Miller et al., 2009). In contrast, singlet oxygen (1O2) has an extremely short half-life of 4 µs (Redmond & Kochevar, 2006), and was thought to be restricted to the action of light-driven photosensitization reactions typically occurring in the chloroplast (Hideg et al., 1998; Flors et al., 2006). Photosynthetically produced 1O2 was shown to damage photosynthetic machinery. In particular, the D1/2 reaction centre proteins appear to be particularly sensitive to 1O2 damage, and its de novo synthesis in response to damage is a critical repair mechanism to maintain chloroplast function [reviewed in (Li et al., 2018)]. Intriguingly, 1O2 was recently also shown to be produced in dark reactions (Mor et al., 2014; Prasad et al., 2017) and intimately related to osmotic stress responses in the root due to the activity of lipoxygenases (Chen and Fluhr, 2018; Chen et al., 2021).
1O2 signalling has been studied in a variety of systems, ranging from exogenous photosensitisers like Rose Bengal (RB) and Acridine Orange (AO), to genetic mutants such as FLUORESCENT IN BLUE LIGHT (flu), CHLORINA 1 (Ch1) and to inhibitors of photosynthesis such as 3-(3,4-dichlorophenyl)-1,1-dimethylurea (DCMU). As 1O2 is an extremely short-lived molecule, and is highly reactive towards electron-rich biomolecules such as unsaturated fatty acids, the effects of the different treatments are restricted to the locality where they are generated. A recent study using chemooptogenetic methods to target photosensitisers to specific subcellular compartments highlighted the differential apoptotic and necrotic outcomes of 1O2 localization (Liang et al., 2020). In Arabidopsis, we previously showed that RB and AO localizes 1O2 production to the plasma membrane and vacuole respectively. AO, but not RB, resulted in the stimulation of vacuolar-driven cell death via the leakage of vacuolar proteases (Koh et al., 2016). Similarly, DCMU is an inhibitor of photosynthesis which blocks the electron transport chain of Photosystem II, preventing the conversion of light energy to ATP. Light energy remains trapped in the excited chlorophyll pigments, which then transfer this energy to nearby molecular oxygen, forming 1O2. This 1O2 can cause damage to the nearby photosynthetic apparatus, and prolonged exposure to light can ultimately lead to chlorophyll bleaching and cell death (Ridley, 1977).
The flu mutant was one of the earliest mutants used to characterise the effects of 1O2 production in the cell. This mutant bears a defect in the feedback regulation of δ-aminolevulinic acid (ALA) synthesis, which allows for uncontrolled protochlorophyllide (Pchd) accumulation in the dark (Meskauskiene et al., 2001). Normally in the dark, Pchd is sequestered in the chloroplast complexed with protochlorophyllide oxidoreductase proteins responsible for the safe phototransformation of Pchd to chlorophyllide (Masuda and Takamiya, 2004). However, excess unbound Pchd can act as a natural photosensitizer upon transition from dark to light. It transfers light energy to O2 via Type II reactions, producing copious amounts of 1O2 that promote cell death (op den Camp et al., 2003). Thus, the release of 1O2 is proportional to the amount of Pchd accumulation, as well as the intensity of light exposure (Wang and Apel, 2018). It was shown that a set of 1O2 sensitive genes were upregulated in response to dark/light transition in the flu mutant, which was distinct from that produced by another sources of ROS e.g. methyl viologen (MV), an inducer of superoxide formation (op den Camp et al., 2003). It was hypothesized that the response required cellular signal transduction as further second site mutants were discovered (executer mutants; ex1, ex2) which exhibited attenuation of cellular death and diminished stress gene expression (Wagner et al., 2004; Lee et al., 2007). It was also shown recently that oxidative modification of a specific tryptophan residue in EX1 was responsible for propagating the cell death response (Dogra et al., 2019). Similarly, the protease FtSH2 and grana localized protein SAFEGUARD1 (SAFE1) was also shown to play critical roles in regulating the flu/ex1 response (Wang et al., 2016; Wang et al., 2020). The components of this regulatory pathway (Pchd, flu, ex1/ex2, FtSH2, SAFE1) have all been shown to reside within the confines of the chloroplast. Thus, it was proposed that some yet uncharacterised component was responsible for eliciting a nuclear-generated transcriptome response and provide for retrograde signalling.
Another source of 1O2 that occurs during photosynthesis was investigated in the Ch1 mutant that lacks chlorophyll a oxygenase, resulting in plants deficient in chlorophyll b (Espineda et al., 1999). As a consequence, it is devoid of Photosystem II (PSII) chlorophyll-protein antenna complexes (Havaux et al., 2007). This results in the improper assembly of the normal architecture of the PSII system which renders the mutant extremely sensitive to photooxidative stress (Havaux et al., 2007). The exposure of the mutant to a combination of HL/cold stress for 2 days resulted in production of 1O2, coupled with an increase in measured lipid peroxidation and stress gene transcript levels. The sensitivity of this mutant to photooxidative stress can be exacerbated by reduction of cellular ROS scavengers, which was demonstrated by crossing the Ch1 mutants with scavenger deficient mutants such as vte2 (tocopherol) and pdx1.2 (vitamin B6) (Havaux et al., 2007; Havaux et al., 2009). Interestingly, the crossing of Ch1 with the ex1 mutant did not appear to yield any significant effect on the attenuation of the 1O2 cell death response (Ramel et al., 2013). This was attributed to the two 1O2 generation systems differing in their signalling mechanisms.
It was found that cold/HL stress led to the accumulation of several oxidation products of β-carotene, a key carotenoid involved in the quenching of reactive oxygen species produced during photosynthesis (Ramel et al., 2012). Further in vitro studies showed that 1O2 was the key molecule involved in this oxidative process. Among the various oxidation products found, β-cyclocitral (BCC), a volatile organic compound, was observed to stimulate the induction of various 1O2 sensitive genes. Pre-application of BCC to WT Arabidopsis plants was able to acclimate them to a future cold/HL stress treatment (Ramel et al., 2013). BCC is thought to act as a possible signalling molecule in chloroplast-to-nuclear signalling. Putative transducers of the BCC signal include the zinc finger protein MBS1, that are important for BCC-induced acclimation to HL stress (Shumbe et al., 2017); and the TGAII/scarecrow like-14 (SCL14) transcription factors which regulate detoxification related genes for HL stress acclimation (D'Alessandro et al., 2018). Further investigations of the Ch1 mutant have also implicated the OXI1 kinase/MAPK and salicylic acid/H2O2 signalling pathways (Shumbe et al., 2016; Beaugelin et al., 2019). These pathways appear to modulate the endoplasmic reticulum mediated stress (ER stress) and unfolded protein responses (UPR), as part of the cellular responses to high light stress (Beaugelin et al., 2020), but do not appear to involve BCC signalling.
Recently, we showed that cytosolic sources of 1O2 stimulated stress transcriptomes were correlated with cycloheximide (CHX) transcriptomes (Koh et al., 2021). CHX is a potent and irreversible inhibitor of 80S ribosomal translation (Schneider-Poetsch et al., 2010). Under CHX treatment cytoplasmic translation ceases. In the absence of continual replacement to maintain a steady state, proteins will degrade according to their respective half-lives (Li et al., 2017). Transcripts previously kept repressed by short-lived transcription factors are then induced. Using a variety of 1O2 generating systems such as Rose Bengal (RB) and DCMU, we demonstrated that 1O2 generated in the cytosol but not within the chloroplast, showed CHX-like transcriptomic elements. This could be explained by the oxidation of guanosine residues to 8-hydroxyguanosine (8-oxoG) by 1O2. The oxidized guanosine stymies 80S ribosomal translation and mimicked the action of CHX (Tanaka et al., 2007). The effect was exacerbated in proteins regulated by the proteosome that have a high turnover rate. Thus, a wave of stress transcripts that are regulated by such proteins are freed from repression and accumulate as a 1O2 -specific stress signature. For example, jasmonic acid signalling genes that are repressed by short half-life JAZ repressors were stimulated via 1O2 mediated translational attenuation in the coi1 mutant, which is normally insensitive to JA (Koh et al., 2021). It is important to note that the great majority of genes do not change their expression so that a degree of specificity is achieved by limiting the response to rapidly turning over transcriptional repressors or activators without reprogramming of the entire transcriptome.
Here, we show that Pchd accumulates in both the chloroplast and cytosol of the flu mutant under dark incubation. Prolonged incubation in the dark led to increased Pchd accumulation in a time-dependent manner, which led to the formation of 1O2 upon exposure to light. RNA oxidation was monitored via the formation of oxidized guanosine (8-oxoG), and was found to accumulate proportionally with Pchd levels and light intensity. The translational competence of luciferase reporter transcripts in flu x IAA-LUC transgenic plants were analysed under these conditions. The results demonstrated that there was a corresponding decrease in translatability with increasing levels of 8-oxoG. Plants supplemented with ALA exhibited cytosolic accumulation of Pchd in a manner similar to the flu mutant, and also resulted in a strong CHX-like transcriptomic signature. In order to evaluate the contribution of 1O2 -mediated translational attenuation in various 1O2 -signalling systems such as RB, DCMU and the flu and Ch1 mutants, we developed a bioinformatic method for distinguishing between cytosolic and chloroplastic sources of 1O2. We then further apply our method to analyse various stress transcriptomes and gain biological insight into the nature of 1O2 signalling in these systems.
Arabidopsis thaliana (ecotype Columbia) seedlings (2-week-old) were grown under white light in a 16-h light (120 µEm-2s-1)/8-h dark cycle at 21°C on Murashige and Skoog medium, supplemented with 1% sucrose and 0.8% (w/v) phytoagar (Invitrogen). Plants were pre-equilibrated in double-distilled water (DDW) in a Petri dish for 1 h before being transferred to 12 well plates for the various chemical treatments. For treatment of flu seedlings, they were placed in the dark in DDW for the time points indicated and re-exposed to light as indicated.
Confocal microscopy analysis was carried out on 5-day old Arabidopsis seedlings. All images were taken with a Nikon A1 confocal microscope. For chlorophyll fluorescence, excitation was at 630 nm and emission was at 690 nm. All images were acquired using a 60x objective lens. Seedlings were equilibrated in DDW for 1 h in the light and subjected to the various treatments described. For WT, flu and ch1 mutants, seedlings were incubated in the dark for the time points indicated, then visualized under the microscope. For ALA treatment, seedlings were incubated with 1 mM ALA (in half-strength MS + 1% sucrose, unless indicated) for the time points indicated. The laser settings were constant for all treatments and the post processing look up table was adjusted for each treatment to eliminate background fluorescence.
Confocal measurements for spectral imaging were performed using the Leica TCS SP8 with an Acousto Optical Tunable Filter (Leica microsystems CMS GmbH, Germany). A representative relevant slice was scanned using 458 nm excitation (5% power) and collection carried out in 5 nm width windows in the range of (600-700 nm). Images were acquired at a scanning speed of 8000 pixels per second with 63X oil immersion objective and image analysis was performed using Leica Application Suite software (Leica microsystems CMS GmbH).
Arabidopsis seedlings (2-week-old, 7 whole seedlings per biological replicate, 3 replicates) were used for each treatment. Samples were harvested by flash freezing in liquid nitrogen and were homogenized in a shaker using glass beads. RNA was extracted from frozen tissues using a standard TRIzol extraction method (Sigma-Aldrich). DNase I (Sigma-Aldrich)-treated RNA was reverse transcribed using a high-capacity complementary DNA reverse transcription kit according to the manufacturer’s instructions (Quanta Biosciences). For qRT-PCR analysis, the SYBR Green method (KAPA Biosystems) was used on a Step One Plus platform (Applied Biosystems) with a standard fast program. qRT-PCR primers were designed in Snapgene software. All qRT-PCR primer sequences are listed in Supplemental Dataset S1.
Arabidopsis seedlings (2-week-old, 7 whole seedlings per biological replicate, 3 replicates) were used for each treatment. In all downstream processing steps from plants, 4 mM of 4-hydroxy-TEMPO (Sigma-Aldrich) was used as an antioxidant to prevent spurious oxidation of RNA (Hofer & Möller, 1998). Samples were subjected to RNA digestion (2 h at 37°C with 30 units Nuclease S1 in 20 mM sodium acetate, pH 5.2, followed by 1 h at 37°C with 10 units Shrimp alkaline phosphatase in 100 mM Tris-HCl, pH 8. The reaction mixture was then filtered through a 10 kDa filtration column (Amicon) for 15 min at 14,000 rpm, 4°C, and the filtrate was collected for 8-oxoG determination. For standard curves concentrations 0, 1, 5, 10, 25 and 50 ng/mL of 8-oxoG, and 0, 1, 5, 10, 25 and 50 μg/mL of G were prepared and measured. The chromatographic separation was performed on an Acquity UPLC system (I-Class, Waters, Milford, MA, USA) with a Cortecs UPLC C18+ column (1.6 μm, 2.1×100 mm). The mobile phase was (A) 0.1% acetic acid in water, and (B) methanol. Full peak separation was achieved (RTG=4.07 min and RT8-oxo-G=5.35 min) to avoid ion-suppression of 8-oxoG by G with the following gradient program %B (min): 2(0-4), 100(7-7.5), 2(8-10). MS detection was performed on a TQ-S triple quadrupole mass spectrometer (Waters), equipped with an ESI ion source operated in the positive mode. Detections were performed in MRM mode. The MS/MS transitions selected for 8-oxoG were 300.06→168.09 (collision energy CE=17eV) and 300.06→140.05 (CE=33eV) m/z. The transitions for G were 284.1→135.2 (CE=25eV) and 284.1→152.2 (CE=65eV) m/z. The collision energies were chosen to enable simultaneous measurement of 8-oxo-G and G in the same run. MassLynx and TargetLynx software (v.4.1, Waters) were applied for the acquisition and analysis of data.
Arabidopsis seedlings (2-week-old, 7 whole seedlings per biological replicate, 3 replicates) were used for each treatment. In vitro translation was performed with the Rabbit Reticulocyte Assay (Promega) according to the manufacturer’s instructions using 10 µg of RNA supplemented with 1 µl each of RNAasin Inhibitor (Promega) and Plant Protease inhibitor cocktail (Sigma) in a 50 µl final volume. The reactions were run at 30°C for 2 h in a thermocycler, and stopped by cooling the reaction to 4°C and further addition of 2 µl of 10 mM cycloheximide. Luciferase activity was measured using a luminometer (Turner Biosystems) in conjunction with Luciferase Assay Reagent (Luciferase Assay System, Promega). For luciferase measurements after in vitro translation the reaction was used directly without further processing.
Protochlorophyllide (Pchd) accumulation was measured by imaging live plants using a fluorimeter. Arabidopsis seedlings (14-day-old) were placed in individual wells of a 24-well plate in DDW, supplemented with ALA at the concentrations and times indicated. Fluorescence was measured using a fluorimeter scanning a 3x3 array of points per well and the highest value of each well taken for analysis (Ex/Em: 440/630 nm). The means and SE of 24 whole seedlings per time point are shown.
Libraries were prepared with the MARS-seq protocol (Jaitin et al., 2014) and sequenced using Illumina NextSeq 500 High Output v2 Kit (75 cycles). Reads were trimmed using ‘cutadapt’ (Martin, 2011) and mapped to the Araport 11 reference genome using STAR v2.4.2a (https://github.com/alexdobin/STAR/). Counting was done using HTSeq-count (Anders et al., 2015). Further analysis is done for genes having minimum 5 reads in at least one sample. Normalization of the counts and differential expression analysis was performed using DESeq2 (Love et al., 2014). Raw P values were adjusted for multiple testing using the procedure of Benjamini and Hochberg (Benjamini and Hochberg, 1995). The test samples were always compared to their respective 0 h, or untreated controls.
Microarray data was processed using the affy, oligo or open source Bioconductor marray R packages and normalized using mas5 and associated packages. Transcripts were filtered for at least 2-fold up or down regulation, with a p-value cutoff of 0.05. Datasets are available in Supplemental Dataset S2. ROSMETER analyses were performed using the ROSMETER tool by providing fold-change and P values of the respective transcriptomes (http://app.agri.gov.il/noa/ROSMETER.php) (Rosenwasser et al., 2013). Venn diagrams were generated by: (Venny 21; http://bioinfogp.cnb.csic.es/tools/venny/). P-values were obtained by using Fisher’s Exact Test of 2x2 contingency table for each individual comparison and are represented by the numbers in parentheses.
The reference CHX transcriptome used was derived from GSE111284. The material used to prepare the transcriptome were 14-day old Arabidopsis WT (Col-0) seedlings that had been treated with 100 µM CHX for 2 h under low light (30 µE m-2 s-1) conditions. For any gene list, we queried its respective genes against the CHX reference transcriptome. The steps of TAI analysis are the following: Up-regulated genes (>2-fold, p < 0.05) from any transcriptome are searched within the CHX transcriptome and their fold change under CHX obtained. The corresponding fold change values of the CHX genes (log2) are then arranged in a plot in the order of largest to smallest. The value of TAI was calculated in the following way. TAI = [Number of up-regulated genes]/[Total number of genes]. For example: A gene list of 100 genes are queried against the CHX transcriptome. If 70 genes had a log2 fold change value > 0, then TAI = 70/100 = 0.7.
The reference databases used here CHX, RB, flu, DCMU, Ch1 are GSE111284, GSE111285, GSE111286, GSE111287, and GSE205861 respectively. The flu, flu/ex1, flu/ex2 and flu/ex1/ex2 transcriptomes were obtained from GEO GSE10509 (Lee et al., 2007). The flu/ex1 and flu/ex1/safe1 transcriptomes were obtained from GEO GSE131610 (Wang et al., 2020). The drought microarray data was obtained from ArrayExpress: E-MEXP-2377 (Mizoguchi et al., 2010). The Ch1 (Ramel) microarray data done after dual light stress and cold treatment was obtained from Project CEA10-02_Light (Ramel et al., 2013). The other microarray data were obtained from the respective reference databases. fc1, fc2 – GEO GSE71764 (Woodson et al., 2015); FR treatment – GEO GSE6169 (Page et al., 2017); vte2 - GEO GSE4847 (Sattler et al., 2006).
The flu and Ch1 mutants have been extensively studied as 1O2 generating systems although it is understood that 1O2 generation in both systems stem from different sources. The former from protochlorophyllide (Pchd) accumulation in the dark, and the latter from improperly assembled light harvesting complexes. Transcriptomes for flu were obtained upon transfer of plants to light from the dark (GSE111286) and for Ch1 after dual application of light stress and cold (Ramel et al., 2013). They were compared to CHX and RB transcriptomes (Koh et al., 2021). Genes that were at least 2-fold upregulated, showed significant overlap of 49.2% (P = 2.9E-70) and 46.6% (P = 3.3E-118) with CHX and 39.9% (1.1E-106) and 29.1% (P = 1.2E-119) with RB, in flu and Ch1 respectively (Figure 1A). Relatively, down regulated transcripts showed less but highly significantly overlap, of 31.5% (P = 2.9E-98) and 34.0% (P = 2.1E-70) with CHX and 13.5% (P = 1.7E-37) and 8.7% (P = 4.5E-14) with RB, in flu and Ch1 respectively (Figure 1A, Supplemental Table S1A). The ROSMeter tool compares transcriptomes to a database of known ROS-based transcriptomes (Rosenwasser et al., 2013; Mor et al., 2014). Each has its own distinct signature; while the flu, and RB transcriptomes are most similar they all contain with CHX and Ch1 mutant a common 1O2 signature. The comparisons suggest that both flu and Ch1 transcriptomes contain elements of the RNA oxidation and translational arrest mechanism associated with the oxidation of RNA (Figure 1B). Exogenous application of H2O2 also showed similarity and such treatments have been shown to stimulate RNA oxidation as well (Hofer et al., 2005). Interestingly, some of the tested transcriptomes appeared to exhibit a negative correlation to mitochondria superoxide and H2O2 accumulation in organelles; where AS-AOX and TDNA-AOX are mitochondrial knockdown and knockout mutants of alternative oxidase, respectively (Figure 1B). In contrast, the chloroplast superoxide and H2O2 associated with KO-APX mechanisms appeared to be unrelated to the conditions examined.
Figure 1 Comparison of transcriptomes of seedlings treated by RB, DCMU, cycloheximide (CHX) and from the flu mutant. Arabidopsis seedlings were treated with either CHX (100 µM, 2 h, 30 µE m-2 s-1) or RB (400 µM, 2 h, 30 µE m-2 s-1). The flu mutant was incubated in the dark for 4 h, before light exposure (30 µE m-2 s-1) for 30 min. The Ch1 transcriptome was treated under HL/cold conditions (1200 µE m-2 s-1, 10°C, 48 h, see Ramel, 2013) Further details and the source of transcriptomes are referenced in the Methods. (A) Venn diagram overlaps of 2-fold induced genes of Up-regulated (left) and Down-regulated genes (right). (B) ROSMETER transcriptomic analysis. Transcriptomes of all significant up and down-regulated genes were analysed using the ROSMETER tool showing the correlation of gene activity with the ROSMETER databases. Red represents a positive correlation of +1, and green represents a negative correlation of -1.
Chlorophyll biosynthesis, including the critical photoconversion of Pchd, takes place in the chloroplast, where the majority of chlorophyll biosynthetic enzymes reside. Regulated accumulation in conjunction with other components of the photosynthetic machinery is crucial as an excess of free Pchd or chlorophyll would act as disruptive photosensitizers. Pchd accumulation in the flu mutant was previously shown to occur only in the chloroplast (op den Camp et al., 2003). However, Pchd fluorescence of dark-incubated flu mutant showed cytosolic accumulation that is confirmed here [Figure 2; (Koh et al., 2021)]. This finding may be due to the current advances in the sensitivity of confocal technology. In comparison, dark incubated WT or Ch1 mutant seedlings show chlorophyll localized only to the chloroplast. A precise fluorescence emission spectrum was carried out in various subcellular localizations (Figure 2A). It showed accumulation of two major peaks corresponding to Pchd and chlorophyll in the plastids and cytosol of the flu mutant, but only a single chlorophyll peak in the plastids of WT and Ch1 (Figure 2B).
Figure 2 Comparison of chlorophyll fluorescence and localization in WT, flu and Ch1 seedlings under dark incubation. (A) Spectral imaging of WT, flu or Ch1 seedlings during dark incubation. WT, flu or Ch1 seedlings were incubated in the dark for 4 h, then mounted in DDW for imaging as described in the Methods. Scale bar represents 5 µm. (B) Spectral analysis of chlorophyll fluorescence. Areas indicated by boxes in (A) were analyzed and the emission spectrum was plotted.
The levels of Pchd can be manipulated by increasing the dark incubation time of mutant flu seedlings (Figure 3A). Guanosine residues are highly reactive to 1O2 (Wilkinson et al., 1995). To monitor this, the degree of guanosine oxidation to 8-oxoG was measured relative to guanosine (G) where the latter serves as an internal quantitative control. This ratio rises upon dark to high light transition to about 20 oxidation events for every 105 residues (Figure 3B). Assuming an average transcript length of about 1500 bp for Arabidopsis, this seemingly low rate would still lead to approximately one oxidation event in 7.5% of the transcripts. That level can have profound effects on rapidly turning over proteins that need constant replenishment by transcript translation to maintain their steady state.
Figure 3 RNA oxidation in the flu mutant is correlated with dark incubation time and light intensity. (A) Protochlorophyllide (Pchd) accumulation in flu x UBIQ10:IAA-LUC seedlings. Fluorescence was measured in dark incubated flu x UBIQ10:IAA-LUC seedlings using a fluorimeter (Ex/Em: 440/630 nm). The means and SE of 24 whole seedlings per time point are shown. (B) RNA oxidation analysis. Arabidopsis (Col-0, flu x UBIQ10:IAA-LUC) seedlings were incubated in the dark for 0, 8, 12, 24 h in the dark before 30 min exposure to low light (LL, 30 µE m-2 s-1) or high light (HL, 1000 µE m-2 s-1). RNA was processed for RNA oxidation as described in the Methods. (C) RNA translatability analysis. RNA as in (B) was used for in vitro translation and luciferase activity analysis as described in the Methods. Values were normalized against luciferase transcript levels, and Student’s t-test performed against LL 0 h. (D) RNA as in (B) was processed as described in the Materials and Methods for qRT-PCR analysis to determine fold change in luciferase mRNA levels in the various samples using luciferase specific primers. (E) and (F) RNA as in (B) was extracted and processed as described in the Materials and Methods for qRT-PCR analysis. The means and SE of three replicates is shown. Student’s t-test was performed against their respective controls for significance (* - P<0.05; ** - P<0.01; *** - P<0.001). 1O2 sensitive genes: At3g61190 – Bonsai associated protein (BAP1), At5g64870 - nodulin-related protein, At3g01830 - calmodulin-related protein, At5g13200 - GRAM domain-containing protein.
IAA-LUC fusions produce constitutive levels of IAA-luciferase mRNA and can be used to precisely measure changes in mRNA functionality by comparing the translational competence of its RNA. To precisely measure the effect of 8-oxoG accumulation in the flu mutant, a flu x UBIQ10:IAA-LUC transgenic line was constructed. The effect of dark incubation time and subsequent exposure to light on the translational competence of constitutively expressed luciferase transcripts was then measured in a reticulocyte translation system. We observed that the relative translational competence of luciferase transcripts showed a significant decrease at 12 and 24 h dark of incubation time and was sensitive to higher light intensity (Figure 3C). The levels of LUC mRNA as measured by qPCR were used to normalise the luciferase activity levels. They remained relatively unchanged in all treatments (Figure 3D). Using a 1O2 -specific gene set previously validated to be responsive to RNA oxidation and translational arrest, we observed that increased dark incubation time and light intensity led to a general increase in expression of these genes (Figures 3E, F). Figure 3E shows that at later times the gene activity of the 1O2 marker genes is decreasing (i.e. at 24 h, rather than continuing to increase. This could be due to cross regulation by compensatory mechanisms e.g. increased RNA synthesis in LL (low light) conditions; whereas these mechanisms are not sufficient to compensate under (HL) high light intensities (Figure 3F). Thus, we show that Pchd accumulates proportionally to the period of dark incubation, and this Pchd then generates 1O2 and RNA oxidation in a manner dependent on light intensity. Following from this, the induction of 1O2-signature gene expression in the flu mutant is correlated with the oxidation of RNA and the level of its translational competence.
δ-aminolaevulinic acid (ALA) is an early precursor of chlorophyll (Granick, 1959). As the flu mutant is defective in the negative feedback regulation of ALA synthesis in the dark, we examined to what extent supplementation of the media with ALA could phenocopy the flu mutant. WT Arabidopsis seedlings incubated with ALA in the dark were observed to accumulate cytosolic fluorescence in a time dependent manner (Figures 4A, C). Analysis of the fluorescence emission spectrum of ALA treated plants showed twin peaks of Pchd and chlorophyll in the plastids as well as the cytosol. The untreated plants subject to the same period of dark incubation had only a single chlorophyll peak and no fluorescence in the cytosol could be detected (Figure 4B). qPCR analysis of ALA treated plants exposed to light showed a strong time-dependent induction of transcripts from 1O2 sensitive genes, but not from superoxide () sensitive genes (Figure 4D). The results indicate that dark incubation with ALA mimics the flu mutant in showing accumulation of chlorophyll precursors in the cytosol and the induction of a 1O2 transcriptome upon exposure to light.
Figure 4 ALA feeding leads to extrachloroplastic accumulation of chlorophyll. (A) Accumulation of chlorophyll in extrachloroplastic regions under ALA treatment. Arabidopsis (WT, Col-0) seedlings were incubated with 1 mM ALA (in MS) in the dark for 12 h, and then mounted for visualisation as described in the Methods. Scale bar represents 50 µm. (B) (Left) Spectral imaging of Arabidopsis (WT, Col-0) seedlings were treated with DDW or 1 mM ALA (in DDW) and incubated in the dark for 2 h, and then mounted for visualisation by confocal microscopy as described in the Methods. Scale bar represents 50 µm. (Right) The areas indicated by boxes were scanned for spectral analyses as shown on the right. (C) Protochlorophyllide (Pchd) accumulation in ALA treated seedlings. Fluorescence was measured in WT seedlings (WT, Col-0) treated with 1 mM ALA (in MS) and incubated in the dark for 0, 4, 8, 12 h. Pchd fluorescence was measured using a fluorimeter (Ex/Em: 440/630 nm). The means and SE of 24 whole seedlings per time point are shown. (D) Arabidopsis (WT, Col-0) seedlings were incubated with 1 mM ALA (in MS) in the dark for 0, 4, 8, 12 h in the dark before 30 min exposure to low light (30 µE m-2 s-1). RNA was extracted and processed as described in the Materials and Methods for qRT-PCR analysis. The means and SE of three replicates is shown. Student’s t-test was performed against their respective controls for significance (** - P<0.01; *** - P<0.001). 1O2 sensitive genes as in Figure 3E. sensitive genes: At5g01600 – Ferritin 1 (FER1), At4g21870 - heat shock protein family, At1g71030 - myb family transcription factor, At2g40300 - ferritin-related (FER4).
The executor (Ex) pathway was previously established as a genetic model that abrogated the cell death phenotype of the flu mutant. Under 16 h light/8 h dark growth conditions, the flu mutant develops necrotic lesions and severely impaired growth. In contrast, the flu/ex1 mutant displayed normal growth similar to WT and a distinct lack of lesions (Wagner et al., 2004). Another mutation, ex2, was observed to be unable to abrogate cell death in the flu/ex2 mutant, but was able to enhance the protective effect of ex1 in the flu/ex1/ex2 triple mutant (Lee et al., 2007). However, Pchd and 1O2 accumulation was reported to be similar in the flu, flu/ex1, flu/ex2 and flu/ex1/ex2 mutants (Kim et al., 2012).
In order to investigate the role of the executor pathway in the cytosolic accumulation of Pchd, ALA treatment was used to generate chlorophyll precursors in the WT, ex1, ex2 and ex1/ex2 mutants. We noted that the rate of Pchd accumulation in bulk tissue was similar between WT, ex1, ex2 and ex1/ex2 mutants under ALA treatment (Supplemental Figure S1). Next, we observed that in both the WT or in the ex1 mutant significant Pchd fluorescence accumulated in the cytosol under ALA treatment (Figure 5A). Furthermore, 1O2 signature genes were all similarly induced in the WT and ex1 mutants after transition to light (Figure 5B). It is also important to note that in the longer dark incubation times the induction of these genes was much greater than in the flu mutant (compare Figures 3E, 4D, 5B). This is likely due to greater accumulation of Pchd in the cytoplasm (compare images in Figure 2A, flu and Figure 4A, ALA). We examined published transcriptomes of flu, flu/ex1, flu/ex2 and flu/ex1/ex2 mutants subjected to dark-light shifts by comparing them to the CHX transcriptome. Both flu and flu/ex2 mutants showed significant overlap with CHX, that was reduced in the flu/ex1 and especially in the flu/ex1/ex2 combinations (Figure 6A). In contrast, feeding by ALA leads to more substantial accumulation that cannot be inhibited by the executor mutations as shown in Figures 5A, B.
Figure 5 Extrachloroplastic accumulation of chlorophyll is not regulated by the executor pathway under ALA treatment. (A) Accumulation of chlorophyll in extrachloroplastic regions under ALA treatment. Arabidopsis WT and ex1 seedlings were incubated with 1 mM ALA (in MS) in the dark for 12 h, and then mounted for visualisation by confocal microscopy. Ex/Em: 630/690 nm. Scale bar represents 50 µm. (B) Arabidopsis WT and ex1 seedlings were treated with 1 mM ALA (in MS) and incubated in the dark for 0, 4, 8, 12 h in the dark before 30 min exposure to low light (30 µE m-2 s-1). RNA was extracted and processed as described in the Materials and Methods for qRT-PCR analysis. The means and SE of three replicates is shown. Student’s t-test was performed against their respective controls for significance (* - P<0.05; ** - P<0.01; *** - P<0.001).
Figure 6 Venn diagram and Translational Attenuation Index analysis of flu, flu/ex1, flu/ex2, flu/ex1/ex2 transcriptomes. (A) Venn diagram overlaps of 2-fold up-regulated (p < 0.05) genes of CHX, flu, flu/ex1, flu/ex2, flu/ex1/ex2. (B) Translational Attenuation Index analysis of CHX, flu, flu/ex1, flu/ex2 and flu/ex1/ex2 induced transcripts. Genes from flu, flu/ex1, flu/ex2, flu/ex1/ex2 transcriptomes as in (A) were screened against a dataset of cycloheximide treatment and their expression values under cycloheximide treatment are displayed accordingly. The data from CHX, flu, flu/ex1, flu/ex2 and flu/ex1/ex2 were obtained from public databases. (CHX – GSE111284; flu, flu/ex1, flu/ex2 and flu/ex1/ex2 – GSE10509).
A recent study described a grana localised protein, SAFEGUARD1 (SAFE1), that appeared to play an important role in protecting the chloroplast against 1O2-mediated toxicity in the flu mutant, but independently of the EX1 pathway (Wang et al., 2020). The absence of this protein in the flu/ex1/safe1 triple mutant led to significant symptoms of 1O2-mediated toxicity that is produced in the flu mutant, but is typically attenuated in the flu/ex1 mutant. We compared published transcriptomes of the flu/ex1 and flu/ex1/safe1 mutants subject to dark-light shifts against the CHX and RB transcriptomes. We observed that the flu/ex1 transcriptomes showed approximately 32.5% (p = 3.9 E-11) and 24.2% (p = 1 E-17) overlap with CHX and RB respectively, which was further increased to 77.7% (p = 0) and 46% (p = 0) respectively in the flu/ex1/safe1 mutant (Supplemental Figure S2). Furthermore, it was also reported that the dark-light shift led to chloroplast leakage in this mutant, whereas plastid integrity was maintained in the flu/ex1 mutant under the same conditions (Wang et al., 2020). These results support the notion that SAFE1 is an important component for the maintenance of plastid integrity preventing leakage of photodynamic components to the cytoplasm.
Comparison of experimental transcriptomes to the CHX transcriptome can provide an estimation of the degree of translational repression due to 1O2 production in the cytoplasm. To carry this out, genes from various transcriptomic experiments were subjected to a 2-fold expression threshold and p<0.05 significance cut-off. The selected genes were compared to CHX fold changes that is used as a common reference for all comparisons. The percentage of genes that are upregulated and the relative amplitude of their fold-change provides insight into the 1O2-responsiveness of the experimental transcriptome. We can use these properties to assign a simple quantitative index which we will term here as the Translational Attenuation Index (TAI), where the highest theoretical value that occurs in complete overlap would be 1 (See Methods).
The TAI values were computed for the flu (0.81); flu/ex1(0.73); flu/ex2 (0.93) and flu/ex1/ex2 (0.73) transcriptomes (Figure 6B). While the flu/ex1 and flu/ex1/ex2 mutants showed a decreased TAI (Figure 6B), the flu/ex2 mutant showed a higher TAI than even the flu mutant. These values are consistent with previous analyses showing that ex1 can mitigate the flu stress phenotype, while ex2 alone appears to enhance it (Lee et al., 2007). In any case, the executor mutations appear to only attenuate the effect of flu, suggesting that the presence of cytosolic 1O2 or its effect may be reduced but not completely abrogated by the executor pathway, in agreement with the results of the application of ALA. In addition, TAI analysis for the flu/ex1 and flu/ex1/safe1 transcriptomes obtained by a separate group showed a TAI value of 0.57 for flu/ex1, while the TAI value of the flu/ex1/safe1 transcriptome was further increased to 0.90 (Supplemental Figure S2), consistent with the observation that plastid integrity was compromised in this mutant under a dark-light shift (Wang et al., 2020), which may lead to leakage of chlorophyll metabolites into the cytoplasm.
Previous observations showed that the Ch1 mutant under combined cold/HL stress conditions produced significantly greater amounts of 1O2 and lipid peroxidation compared to WT plants (Ramel et al., 2013). The Ch1 mutant under combined cold/HL stress showed a strong 1O2 response, with significant overlaps of Ch1 with CHX, RB and the flu mutant (Figure 1). It was established that the Ch1 mediated photooxidative stress response did not appear to be dependent on the executor pathway, as Ch1/ex1 double mutant plants were indistinguishable from Ch1 plants under cold/HL stress (Ramel et al., 2013). Venn diagram analysis using publicly available Ch1 transcriptomes show a strong overlap of the Ch1 mutant to CHX (46.6%, p = 3.3E-118) and RB (29.1%, p = 1.2E-119), suggesting the possibility of a significant common effect on cytosolic translation (Supplemental Figure S3, Supplemental Table S1A). Consistent with the overlap, the Ch1 mutants under cold/HL showed a relatively high TAI score (TAI = 0.69, Supplemental Figure S3); indicating that the combined cold/HL stress likely impacted on translational activity.
Interestingly, observations showed that cold/HL treatment caused chloroplast rupture and leakage of chloroplast contents into the cytosol (Kim et al., 2012). Therefore, under those conditions, the source of the 1O2 effect may stem from released photodynamic chlorophyll pigments. For example, green sections of the var2 mutant manifested chloroplast rupture and cell death whereas albino sections do not (Kim et al., 2012). In a similar manner, the ferrochelatase 2 (FC2) protein is responsible for the insertion of iron into protoporphyrin IX to form protoheme. In its absence (i.e. fc2), chlorophyll biosynthesis is disrupted and chloroplasts showed progressive degradation as a function of 1O2 stress (Fisher et al., 2021).
The mutant Ch1 was also examined in moderate conditions without cold for 0, 1, 2, 4 h in LL and HL. Interestingly in this case, 1O2 responsive genes were not induced; indeed, they appear to be repressed (Figure 7A). In contrast, superoxide () responsive genes were induced in a light and time dependent manner (Figure 7A). To extend these observations, RNAseq analysis was carried out. In the absence of cold, only a small overlap of less than 10% between Ch1 LL/HL transcriptomes and that of Ch1 under cold/HL stress was obtained. Furthermore, in the absence of cold, moderate overlaps of 20-30% with DCMU and Ch1 were observed (Supplemental Tables S2, S3) and low TAI values were obtained (LL TAI = 0.29; HL TAI = 0.28; Figure 7B).
Figure 7 Ch1 seedlings do not present cytosolic 1O2 signatures under low and high light treatment. (A) Arabidopsis Ch1 seedlings were exposed to low light (30 µE m-2 s-1) or high light (1000 µE m-2 s-1) for 0, 1, 2, 4 h). RNA was extracted and processed as described in the Materials and Methods for qRT-PCR analysis. The means and SE of three replicates is shown. Student’s t-test was performed against their respective controls for significance (* - P<0.05; ** - P<0.01; *** - P<0.001). (B) Translational Attenuation Index analysis of Ch1 induced transcripts under low light (30 µE m-2 s-1) or high light (1000 µE m-2 s-1) conditions after 4 h.
As β-cyclocitral (BCC) is also thought to be a signalling molecule involved in transducing 1O2 signalling in the Ch1 mutant, we performed Venn diagram analysis using publicly available Ch1 (cold/HL) and BCC transcriptomes showed that the Ch1 mutant when carried out with cold treatment had a strong overlap with CHX (46.6%, p = 3.3E-118) and RB ((29.1%, p = 1.2E-119), indicating a significant effect on cytosolic translation (Supplemental Table S1A). Application of BCC showed a similar but slightly weaker overlap with CHX (31.4%, p = 4.4E-23) and RB (24.2%, p = 1.2E-41), while demonstrating a strong overlap with Ch1 (48.8%, p = 1.1E-164) (Supplemental Table S1A). In contrast, when compared to Ch1 LL and HL transcriptomes carried out here without additional cold treatment, the BCC transcriptome showed significantly weaker overlaps of between 4-7% for LL and 10-15% for HL (Supplemental Table S4). Thus, the increased light intensity with cold treatment stimulates a certain level of BCC production. These results suggest that in the case of Ch1, application of multiple stresses such as cold/HL treatment may result in leakage from chloroplasts causing relatively high TAI correlations and elevated BCC levels (compare Supplemental Figure S3 and Figure 7B). In contrast, under conditions where chloroplast integrity is maintained and 1O2 production limited to the chloroplast, 1O2 can elicit some form of BCC-mediated retrograde signalling, but does not affect cytosolic translation and thus does not stimulate 1O2 sensitive genes.
The transcriptome of CHX was compared to other 1O2 generating systems known to be localized to the cytoplasm or to the chloroplast. For example, the herbicide diuron (DCMU) blocks the electron transfer between QA and QB of PSII causing photoinhibition via production of 1O2 in the chloroplast (Fufezan et al., 2002). RB was shown previously to localize mainly to the plasma membrane, and elicit detectable cytosolic 1O2 production (Koh et al., 2016). As shown in Figure 8, the ordering of the various transcriptomes based on their TAI values: RB, flu, DCMU, Ch1, are consistent with their putative cellular locations; placing RB and flu in one class (cytosol) and DCMU and Ch1 in another (chloroplast).
Figure 8 Cytosolic but not chloroplastic 1O2 production are correlated with CHX-induced transcriptomic responses. Translational Attenuation Index analysis of RB, flu, DCMU and Ch1 transcripts. Genes were obtained from various transcriptomic experiments in the literature for the specific treatments and subjected to a 2-fold expression threshold and p<0.05 significance cutoff. Each list of >100 genes were then screened against a dataset of cycloheximide treatment and their expression values under cycloheximide treatment are displayed accordingly. The data here were obtained from public databases (CHX – GSE111284; RB -GSE111285; flu - GSE10509; DCMU - GSE111287; Ch1 - GSE205861).
It was of interest to analyse additional mutations known to cause 1O2 production and examine their localization based on similarity to the CHX transcriptome. The fc2 mutant, in a manner similar to flu, accumulates Pchd in the dark and displays a flu-like phenotype (Woodson et al., 2015). Interestingly, its light stress transcriptome shows significant overlaps with CHX, RB and flu transcriptomes (25-30%; TAI = 0.66; Supplemental Table S1A and Supplemental Figure S4). The weaker mutant fc1, that is also a ferrochelatase mutant, accumulates predominantly Protoporphyrin IX in the dark that is a less potent photosensitiser (Scharfenberg et al., 2015). The mutant fc1 shows a weaker yet significant overlap with CHX, RB and flu compared to fc2, (TAI = 0.51; Supplemental Table S1A and Supplemental Figure S4). As noted above, progressive chloroplast degradation occurred in the light as a function of 1O2 stress in fc2 (Fisher et al., 2021). Thus, the origin of some components of nuclear signalling in ferrochelatase mutants may emanate from photosensitizers stimulating direct cytosolic oxidation in a manner similar to the flu mutant.
Growth in Far-Red (FR) light has been shown to cause the accumulation of Pchd through its ability to stimulate protochlorophyllide biosynthesis, but not allowing for its efficient photoconversion to chlorophyll (Page et al., 2017). Analysis shows that transcriptomes of WT plants exposed to FR treatment and then exposed to white light overlap with CHX (28.1%, p-value = 1.3E-25) and RB (25.3%, p-value = 8.4E-68), respectively (Supplemental Table S1A). TAI analysis yields values of TAI = 0.62, suggestive of the generation of cytosolic 1O2 features in this case as well (Supplemental Figure S4).
1O2 is a potent ROS. Due to the ease of quantitative measurement, the oxidation of unsaturated fatty acids by 1O2 is well established. However, compared to unsaturated fatty acids, the oxidation of guanosine (G) residues is about 100-fold more reactive towards 1O2 (Wilkinson et al., 1995). When produced in the cytoplasm, 1O2 causes the oxidation of RNA and attenuation of cytoplasmic ribosomal translation. This results in decreased levels of short-lived repressor proteins, and stimulates the de-repression of the genes that they control (Koh et al., 2021). From our analysis here and previously, we observed that many 1O2 signalling transcripts were highly correlated with CHX treatment, supporting the hypothesis of 1O2 promoting translational attenuation (Koh et al., 2021). Here, we compare various light-dependent stress transcriptomes to that of the CHX transcriptome, so as to discern if cytoplasmic production of 1O2 is involved.
Utilizing well known models that generate 1O2 signalling such as RB, DCMU and the flu and Ch1 mutants, TAI analyses provide a unified basis for examining their properties. For instance, RB preferentially localizes to the plasma membrane, while DCMU is a competitive analogue of plastoquinone targeted to the chloroplast. In each case 1O2, due to its short half-life, is generated locally and will biologically interact in its specific locality. RB and flu scored high in the TAI scale indicative of cytoplasmic ribosomal attenuation, while DCMU and Ch1 (without dual stress of cold treatment) had the lowest scores (Figure 8). While the bioinformatic results of RB, DCMU and Ch1 were anticipated, the cytoplasmic accumulation of flu and its concomitant high TAI score as shown here were not. Indeed, as Pchd was thought to localize only to the chloroplast its influence via photodynamic production of 1O2 on cellular expression was thought to occur exclusively through a retrograde signalling system from the chloroplast to the nucleus (op den Camp et al., 2003; Wagner et al., 2004). However, the observation that Pchd accumulates in the cytoplasm as well, illustrates how 1O2 is produced in the cytoplasm and can oxidize mRNA. Thus, while parallel retrograde pathways may exist, the oxidation of mRNA provides a mechanistic approach. A graphical summary of the key concepts discussed in the paper is provided in Figure 9.
Figure 9 Plastid and cytoplasmic origins of 1O2. The flu mutant in the dark, or application of ALA, leads to the accumulation of chlorophyll metabolites and production of 1O2 in the cytoplasm in the light. It is currently unknown if the export of these metabolites occurs via specialized transport proteins or via membrane leakage. In the cytoplasm, 1O2 efficiently and selectively oxidizes guanosine residues in RNA that then inhibit translation lowering the levels of rapidly turning over repressors of transcription. The decrease in repressors will initiate the 1O2 transcriptome signature that resembles the transcriptome of CHX, an inhibitor of 80S ribosome translation. The EX1 mutant pathway has been shown to attenuate flu-mediated cell death, but how EX1 signals are transported outside the chloroplast and elicits nuclear signaling is currently unknown. In the light, the ch1 mutant or application of DCMU will induce 1O2 locally in the chloroplast and initiate a transcriptome response that is distinct from cytoplasmic 1O2 (area in grey). It has been shown that BCC can serve as a signaling intermediate in the Ch1 mutant and HL stress, but its mechanism of action is currently unknown. However, under conditions of high light and cold the increased levels of 1O2 initiate chloroplast leakage and production of 1O2 in the cytoplasm leading to a 1O2 transcriptome signature of cytoplasmic origin.
Another system that stimulates Pchd accumulation is FR treatment (Sperling et al., 1997; McCormac and Terry, 2004). Continual exposure to FR light leads to an increase in Pchd. This is consistent with analysis of the FR light treatment transcriptome that exhibits a significant overlap with CHX (Supplemental Table S1A and Figure S4). Likewise, the fc2 mutant conditionally accumulates photodynamic protoporphyrin IX and Pchd in the dark (Woodson et al., 2015). The mutant was found to rapidly bleach and die under a 16 h light/8 h dark light cycle, but not under continuous light, similar to the flu mutant. The fc2 mutant upon dark-light transition showed similarity with the CHX transcriptome (Supplemental Table S1A and Supplemental Figure S4). Based on this analysis, one may expect that Pchd accumulates in the cytoplasm in these cases as well.
Mutants of EX1 and EX2 attenuate effects of the flu mutant. They localize to the grana margins of the thylakoid stacks in the chloroplast (Wang et al., 2016), and were found to interact with several members of the chloroplast quality control pathway, as well as the import or export machinery (Fang et al., 2022). The 1O2-mediated oxidation under dark-light transition, and subsequent proteolysis of the EX1 protein by FtSH2 was proposed to produce a retrograde signal in the nucleus (Dogra et al., 2019). The executor pathway was shown to attenuate, but not negate, the effects of the dark-light shift in the flu mutant (Wagner et al., 2004). Indeed as was shown, the inhibitory effect of executor was overwhelmed by longer periods of dark incubation or high light intensity (Wang and Apel, 2018). A scenario whereby the EX1 pathway impacts on the cytoplasmic proteasome may be one way in which EX imparts retrograde signalling. Indeed, inhibition of the proteasome was found to attenuate the 1O2-induced transcriptome response (Koh et al., 2021).
Feeding of the chlorophyll precursor, ALA, in plants with the ex1 mutations had no effect on the accumulation of Pchd; either in bulk tissue measurements or by visualization of Pchd in the cytoplasmic region (Figure 5A, Supplemental Figure S1). Also, the stimulation of 1O2 signature genes showed no significant difference between the ALA treated WT or ex1 (Figure 5B). Thus, the executor mutations are likely to regulate 1O2 signalling only under limiting conditions of Pchd accumulation as demonstrated in Figure 6. With higher levels of Pchd, such as occurs in application of ALA or longer dark incubation of the flu mutant ex1 is less effective (Figure 3 and Figure 5). The grana localized protein SAFE1 was found to be important in preventing the degradation of the grana margins under conditions where 1O2 is produced, and thus acts as an important buffer under physiological levels of 1O2 toxicity (Wang et al., 2020) Degradation of the thylakoids could lead to the release of photodynamic molecules, that would generate 1O2 under light.
When bound by the photosynthetic machinery chlorophyll is protected by proximal antioxidants such as carotenoids. However, in the event in which photodynamic chlorophyll intermediates accumulate (as in the flu mutant or ALA treatment) or where photosynthesis is disturbed (as in the Ch1 mutant or DCMU application), potent and dangerous 1O2 is produced. This is especially exacerbated in the presence of additional stresses e.g. cold or high light or their combinations (Mittler, 2006; Bratt et al., 2016). The discovery of extraplastidic RNA targets for 1O2 and the possibility of chlorophyll leakage or export from the chloroplast adds direct mechanistic understanding to its effect on gene induction (Koh et al., 2021).
Bioinformatic comparisons of the Ch1 mutant are a case of interest as it shows a transition from a transcriptomic character solely defined by a chloroplastic localization to one which is commiserate with cytoplasmic localization. When conditions were moderate, 1O2 production was limited to the chloroplast and its integrity was maintained. Under these conditions, TAI scores were low and comparable to DCMU as both are localized to the chloroplast (Figure 7 and 8). Indeed, 1O2 signature transcripts in the Ch1 mutant appeared to be repressed, while the superoxide/hydrogen peroxide signature transcripts, indicative of perturbation in photosynthetic flux, increased with the light intensity and its duration (Figure 7A). In contrast, when the Ch1 mutant was challenged with simultaneous cold and HL conditions they showed significant lipid peroxidation (Ramel et al., 2013); and as shown here the transcriptome yielded a significant TAI score. The latter conditions may stimulate chloroplast rupture and leakage of chloroplastic photodynamic metabolites into the cytoplasm and stimulate 1O2 production in a manner similar to flu and RB. Alternatively, the activity of lipoxygenases on free fatty acids has been shown to be an additional source of 1O2 (Chen et al., 2021); these elements may be freed from the chloroplast during stress.
Chloroplast leakage in dehydration stress conditions in the light was also shown to occur concomitantly to a rise in RNA oxidation and 1O2 signature gene expression (Koh et al., 2021). Examination of published transcriptomes from dehydration treated leaves yielded a 58.3% overlap with CHX and a TAI value of 0.90 (Supplemental Figure S3C, D). Thus, once photodynamic material is released to the cytoplasm, 1O2 can disrupt ribosomal translation stimulating signature genes for translational arrest (Koh et al., 2021). Furthermore, chlorophyll degradation products can accumulate under conditions of starvation, senescence and pathogenesis (Pružinská et al., 2007; Hörtensteiner and Kräutler, 2011) that can also serve as a source of 1O2. Interestingly, the infestation of Arabidopsis by the beet cyst nematode Heterodera schachtii was found to lead to decreased chlorophyll levels, and an increase in 8-oxoG content (Labudda et al., 2018). In this manner, 1O2 generating systems that are normally sequestered in the plastid are converted to a system that disrupts processes in the cytoplasm and yield a transcriptome with CHX-like commonalties.
In addition to stresses or mutations that lead to the accumulation of photodynamic molecules, it is expected that plants deficient in the scavenging of basal levels of 1O2 production may show diagnostic 1O2 transcriptome signatures. For example, non-enzymatic lipid peroxidation was shown to activate stress signalling genes in the tocopherol-deficient mutant vte2. (Sattler et al., 2006). Inspection of the basal state of the vte2 up-regulated transcriptome grown under moderate light conditions (120 µE m-2 s-1 under 16 h light/8 h dark cycle for 3 days) detects significant overlaps (33%, p-value = 7.4E-19) and (27%, p-value = 4.5E-36) with the transcriptomes of CHX and RB, respectively (Supplemental Table S1A). The TAI value was calculated to be TAI = 0.60 (Supplemental Figure S4), This suggests that a component of the cellular reaction brought about by the loss of scavenging capacity in the vte2 mutant could be explained by its inability to detoxify 1O2 that initiates the attenuation of translation. Interestingly, it was observed that Ch1 mutants lacking either tocopherol or zeaxanthin in the Ch1vte2 and Ch1npq1 mutants were more susceptible to cold/HL treatment than the Ch1 mutant alone (Havaux et al., 2007). Hence, 1O2 confined to the chloroplast will lead to one set of transcriptomic responses while stresses that impact on chloroplast leakage may lead to another. The analyses presented here show the importance of localizing the source of photodynamic molecules in the cell that will contribute to their specific modes of action.
The original contributions presented in the study are publicly available. This data can be found here: NCBI, GSE205861.
EK and RF designed the experiments. EK and AB executed the experiments. All authors participated in the interpretation and discussion of results. EK and RF wrote the manuscript. All authors contributed to the article and approved the submitted version.
This work was supported by a grant from the Israel Science Foundation to RF, Grant number 2106/21.
The authors would like to thank Tevi Mehlman, Dr. Hadas Keren-Shaul, Dr. Yosef Addadi and Dr. Bareket Dassa of the Weizmann Life Sciences Core Facility for excellent technical assistance. We would also like to thank Dr. Tomer Chen for invaluable advice and discussions in the course of this work.
The authors declare that the research was conducted in the absence of any commercial or financial relationships that could be construed as a potential conflict of interest.
All claims expressed in this article are solely those of the authors and do not necessarily represent those of their affiliated organizations, or those of the publisher, the editors and the reviewers. Any product that may be evaluated in this article, or claim that may be made by its manufacturer, is not guaranteed or endorsed by the publisher.
The Supplementary Material for this article can be found online at: https://www.frontiersin.org/articles/10.3389/fpls.2022.982610/full#supplementary-material
Anders, S., Pyl, P. T., Huber, W. (2015). HTSeq-a Python framework to work with high-throughput sequencing data. Bioinformatics 31 (2), 166–169. doi: 10.1093/bioinformatics/btu638
Beaugelin, I., Chevalier, A., D'Alessandro, S., Ksas, B., Havaux, M. (2020). Endoplasmic reticulum-mediated unfolded protein response is an integral part of singlet oxygen signalling in plants. Plant J. 102 (6), 1266–1280. doi: 10.1111/tpj.14700
Beaugelin, I., Chevalier, A., D'Alessandro, S., Ksas, B., Novák, O., Strnad, M., et al. (2019). OXI1 and DAD regulate light-induced cell death antagonistically through jasmonate and salicylate levels. Plant Physiol. 180 (3), 1691–1708. doi: 10.1104/pp.19.00353
Benjamini, Y., Hochberg, Y. (1995). Controlling the false discovery rate - a practical and powerful approach to multiple testing. J. R Stat. Soc. B 57 (1), 289–300. doi: 10.1111/j.2517-6161.1995.tb02031.x
Bratt, A., Rosenwasser, S., Meyer, A., Fluhr, R. (2016). Organelle redox autonomy during environmental stress. Plant Cell Environ. 39 (9), 1909–1919. doi: 10.1111/pce.12746
Chen, T., Cohen, D., Itkin, M., Malitsky, S., Fluhr, R. (2021). Lipoxygenase functions in 1O2 production during root responses to osmotic stress. Plant Physiol. 185 (4), 1638–1651. doi: 10.1093/plphys/kiab025
Chen, T., Fluhr, R. (2018). Singlet oxygen plays an essential role in the root’s response to osmotic stress. Plant Physiol. 177 (4), 1717–1727. doi: 10.1104/pp.18.00634
D'Alessandro, S., Ksas, B., Havaux, M. (2018). Decoding β-Cyclocitral-Mediated retrograde signaling reveals the role of a detoxification response in plant tolerance to photooxidative stress. Plant Cell 30 (10), 2495–2511. doi: 10.1105/tpc.18.00578
Dogra, V., Li, M., Singh, S., Li, M., Kim, C. (2019). Oxidative post-translational modification of EXECUTER1 is required for singlet oxygen sensing in plastids. Nat. Commun. 10 (1), 2834. doi: 10.1038/s41467-019-10760-6
Espineda, C. E., Linford, A. S., Devine, D., Brusslan, J. A. (1999). The AtCAO gene, encoding chlorophyll a oxygenase, is required for chlorophyll b synthesis in arabidopsis thaliana. Proc. Natl. Acad. Sci. U.S.A. 96 (18), 10507–10511. doi: 10.1073/pnas.96.18.10507
Fang, J., Li, B., Chen, L. J., Dogra, V., Luo, S., Wu, W., et al. (2022). TIC236 gain-of-function mutations unveil the link between plastid division and plastid protein import. Proc. Natl. Acad. Sci. U.S.A. 119 (11), e2123353119. doi: 10.1073/pnas.2123353119
Fisher, K. E., Krishnamoorthy, P., Joens, M. S., Chory, J., Fitzpatrick, J. A. J., Woodson, J. D. (2021). Singlet oxygen leads to structural changes to chloroplasts during their degradation in the arabidopsis thaliana plastid ferrochelatase two mutant. Plant Cell Physiol. 63 (2), 248–264. doi: 10.1093/pcp/pcab167
Flors, C., Fryer, M. J., Waring, J., Reeder, B., Bechtold, U., Mullineaux, P. M., et al. (2006). Imaging the production of singlet oxygen in vivo using a new fluorescent sensor, singlet oxygen sensor green. J. Exp. Bot. 57 (8), 1725–1734. doi: 10.1093/jxb/erj181
Foyer, C. H. (2018). Reactive oxygen species, oxidative signaling and the regulation of photosynthesis. Environ. Exp. Bot. 154, 134–142. doi: 10.1016/j.envexpbot.2018.05.003
Fufezan, C., Rutherford, A. W., Krieger-Liszkay, A. (2002). Singlet oxygen production in herbicide-treated photosystem II. FEBS Lett. 532 (3), 407–410. doi: 10.1016/s0014-5793(02)03724-9
Granick, S. (1959). Magnesium porphyrins formed by barley seedling treated with δ-aminolevulinic acid. Plant Physiol. 34, XVIII.
Havaux, M., Dall'Osto, L., Bassi, R. (2007). Zeaxanthin has enhanced antioxidant capacity with respect to all other xanthophylls in arabidopsis leaves and functions independent of binding to PSII antennae. Plant Physiol. 145 (4), 1506–1520. doi: 10.1104/pp.107.108480
Havaux, M., Ksas, B., Szewczyk, A., Rumeau, D., Franck, F., Caffarri, S., et al. (2009). Vitamin B6 deficient plants display increased sensitivity to high light and photo-oxidative stress. BMC Plant Biol. 9, 130–130. doi: 10.1186/1471-2229-9-130
Hideg, E., Kálai, T., Hideg, K., Vass, I. (1998). Photoinhibition of photosynthesis in vivo results in singlet oxygen production detection via nitroxide-induced fluorescence quenching in broad bean leaves. Biochemistry 37 (33), 11405–11411. doi: 10.1021/bi972890+
Hofer, T., Badouard, C., Bajak, E., Ravanat, J.-L., Mattsson, Å., Cotgreave, I. A. (2005). Hydrogen peroxide causes greater oxidation in cellular RNA than in DNA. Biol. Chem. 386 (4), 333–337. doi: 10.1515/BC.2005.040
Hofer, T., Möller, L. (1998). Reduction of oxidation during the preparation of DNA and analysis of 8-hydroxy-2'-deoxyguanosine. Chem. Res. Toxicol. 11 (8), 882–887. doi: 10.1021/tx980041x
Hörtensteiner, S., Kräutler, B. (2011). Chlorophyll breakdown in higher plants. Biochim. Biophys. Acta 1807 (8), 977–988. doi: 10.1016/j.bbabio.2010.12.007
Jaitin, D. A., Kenigsberg, E., Keren-Shaul, H., Elefant, N., Paul, F., Zaretsky, I., et al. (2014). Massively parallel single-cell RNA-seq for marker-free decomposition of tissues into cell types. Science 343 (6172), 776–779. doi: 10.1126/science.1247651
Kadota, Y., Shirasu, K., Zipfel, C. (2015). Regulation of the NADPH oxidase RBOHD during plant immunity. Plant Cell Physiol. 56 (8), 1472–1480. doi: 10.1093/pcp/pcv063
Kim, C., Meskauskiene, R., Zhang, S., Lee, K. P., Lakshmanan Ashok, M., Blajecka, K., et al. (2012). Chloroplasts of arabidopsis are the source and a primary target of a plant-specific programmed cell death signaling pathway. Plant Cell 24 (7), 3026–3039. doi: 10.1105/tpc.112.100479
Koh, E., Carmieli, R., Mor, A., Fluhr, R. (2016). Singlet oxygen-induced membrane disruption and serpin-protease balance in vacuolar-driven cell death. Plant Physiol. 171 (3), 1616–1625. doi: 10.1104/pp.15.02026
Koh, E., Cohen, D., Brandis, A., Fluhr, R. (2021). Attenuation of cytosolic translation by RNA oxidation is involved in singlet oxygen-mediated transcriptomic responses. Plant Cell Environ. 44 (11), 3597–3615. doi: 10.1111/pce.14162
Labudda, M., Różańska, E., Czarnocka, W., Sobczak, M., Dzik, J. M. (2018). Systemic changes in photosynthesis and reactive oxygen species homeostasis in shoots of arabidopsis thaliana infected with the beet cyst nematode heterodera schachtii. Mol. Plant Pathol. 19 (7), 1690–1704. doi: 10.1111/mpp.12652
Lee, K. P., Kim, C., Landgraf, F., Apel, K. (2007). EXECUTER1- and EXECUTER2-dependent transfer of stress-related signals from the plastid to the nucleus of arabidopsis thaliana. Proc. Natl. Acad. Sci. U.S.A. 104 (24), 10270–10275. doi: 10.1073/pnas.0702061104
Liang, P., Kolodieznyi, D., Creeger, Y., Ballou, B., Bruchez, M. P. (2020). Subcellular singlet oxygen and cell death: Location matters. Front. Chem. 8. doi: 10.3389/fchem.2020.592941
Li, L., Aro, E. M., Millar, A. H. (2018). Mechanisms of photodamage and protein turnover in photoinhibition. Trends Plant Sci. 23 (8), 667–676. doi: 10.1016/j.tplants.2018.05.004
Li, L., Nelson, C. J., Trösch, J., Castleden, I., Huang, S., Millar, A. H. (2017). Protein degradation rate in arabidopsis thaliana leaf growth and development. Plant Cell 29 (2), 207–228. doi: 10.1105/tpc.16.00768
Love, M. I., Huber, W., Anders, S. (2014). Moderated estimation of fold change and dispersion for RNA-seq data with DESeq2. Genome Biol. 15 (12). doi: 10.1186/s13059-014-0550-8
Martin, M. (2011). Cutadapt removes adapter sequences from high-throughput sequencing reads. EMBnet.journal 17, 10–12. doi: 10.14806/ej.17.1.200
Masuda, T., Takamiya, K.-I. (2004). Novel insights into the enzymology, regulation and physiological functions of light-dependent protochlorophyllide oxidoreductase in angiosperms. Photosynth Res. 81 (1), 1–29. doi: 10.1023/B:PRES.0000028392.80354.7c
McCormac, A. C., Terry, M. J. (2004). The nuclear genes lhcb and HEMA1 are differentially sensitive to plastid signals and suggest distinct roles for the GUN1 and GUN5 plastid-signalling pathways during de-etiolation. Plant J. 40 (5), 672–685. doi: 10.1111/j.1365-313X.2004.02243.x
Meskauskiene, R., Nater, M., Goslings, D., Kessler, F., Camp, R. O. D., Apel, K. (2001). FLU: A negative regulator of chlorophyll biosynthesis in arabidopsis thaliana. Proc. Natl. Acad. Sci. U.S.A. 98 (22), 12826–12831. doi: 10.1073/pnas.221252798
Miller, G., Schlauch, K., Tam, R., Cortes, D., Torres, M. A., Shulaev, V., et al. (2009). The plant NADPH oxidase RBOHD mediates rapid systemic signaling in response to diverse stimuli. Sci. Signal 2 (84), ra45. doi: 10.1126/scisignal.2000448
Mittler, R. (2006). Abiotic stress, the field environment and stress combination. Trends Plant Sci. 11 (1), 15–19. doi: 10.1016/j.tplants.2005.11.002
Mizoguchi, M., Umezawa, T., Nakashima, K., Kidokoro, S., Takasaki, H., Fujita, Y., et al. (2010). Two closely related subclass II SnRK2 protein kinases cooperatively regulate drought-inducible gene expression. Plant Cell Physiol. 51 (5), 842–847. doi: 10.1093/pcp/pcq041
Mor, A., Koh, E., Weiner, L., Rosenwasser, S., Sibony-Benyamini, H., Fluhr, R. (2014). Singlet oxygen signatures are detected independent of light or chloroplasts in response to multiple stresses. Plant Physiol. 165 (1), 249–261. doi: 10.1104/pp.114.236380
op den Camp, R. G. L., Przybyla, D., Ochsenbein, C., Laloi, C., Kim, C., Danon, A., et al. (2003). Rapid induction of distinct stress responses after the release of singlet oxygen in arabidopsis. Plant Cell 15 (10), 2320–2332. doi: 10.1105/tpc.014662
Page, M. T., McCormac, A. C., Smith, A. G., Terry, M. J. (2017). Singlet oxygen initiates a plastid signal controlling photosynthetic gene expression. N Phytol. 213 (3), 1168–1180. doi: 10.1111/nph.14223
Prasad, A., Sedlářová, M., Kale, R. S., Pospíšil, P. (2017). Lipoxygenase in singlet oxygen generation as a response to wounding: in vivo imaging in arabidopsis thaliana. Sci. Rep. 7 (1), 9831. doi: 10.1038/s41598-017-09758-1
Pružinská, A., Anders, I., Aubry, S., Schenk, N., Tapernoux-Lüthi, E., Müller, T., et al. (2007). In vivo participation of red chlorophyll catabolite reductase in chlorophyll breakdown. Plant Cell 19 (1), 369–387. doi: 10.1105/tpc.106.044404
Ramel, F., Birtic, S., Ginies, C., Soubigou-Taconnat, L., Triantaphylidès, C., Havaux, M. (2012). Carotenoid oxidation products are stress signals that mediate gene responses to singlet oxygen in plants. Proc. Natl. Acad. Sci. U.S.A. 109 (14), 5535–5540. doi: 10.1073/pnas.1115982109
Ramel, F., Ksas, B., Akkari, E., Mialoundama, A. S., Monnet, F., Krieger-Liszkay, A., et al. (2013). Light-induced acclimation of the arabidopsis chlorina1 mutant to singlet oxygen. Plant Cell 25 (4), 1445–1462. doi: 10.1105/tpc.113.109827
Redmond, R. W., Kochevar, I. E. (2006). Spatially resolved cellular responses to singlet oxygen. Photochem. Photobiol. 82 (5), 1178–1186. doi: 10.1562/2006-04-14-ir-874
Ridley, S. M. (1977). Interaction of chloroplasts with inhibitors: Induction of chlorosis by diuron during prolonged illumination in vitro. Plant Physiol. 59 (4), 724–732. doi: 10.1104/pp.59.4.724
Rosenwasser, S., Fluhr, R., Joshi, J. R., Leviatan, N., Sela, N., Hetzroni, A., et al. (2013). ROSMETER: a bioinformatic tool for the identification of transcriptomic imprints related to reactive oxygen species type and origin provides new insights into stress responses. Plant Physiol. 163 (2), 1071–1083. doi: 10.1104/pp.113.218206
Sattler, S. E., Mène-Saffrané, L., Farmer, E. E., Krischke, M., Mueller, M. J., DellaPenna, D. (2006). Nonenzymatic lipid peroxidation reprograms gene expression and activates defense markers in arabidopsis tocopherol-deficient mutants. Plant Cell 18 (12), 3706–3720. doi: 10.1105/tpc.106.044065
Scharfenberg, M., Mittermayr, L., Von Roepenack-Lahaye, E., Schlicke, H., Grimm, B., Leister, D., et al. (2015). Functional characterization of the two ferrochelatases in arabidopsis thaliana. Plant Cell Environ. 38 (2), 280–298. doi: 10.1111/pce.12248
Schneider-Poetsch, T., Ju, J., Eyler, D. E., Dang, Y., Bhat, S., Merrick, W. C., et al. (2010). Inhibition of eukaryotic translation elongation by cycloheximide and lactimidomycin. Nat. Chem. Biol. 6 (3), 209–217. doi: 10.1038/nchembio.304
Shumbe, L., Chevalier, A., Legeret, B., Taconnat, L., Monnet, F., Havaux, M. (2016). Singlet oxygen-induced cell death in arabidopsis under high-light stress is controlled by OXI1 kinase. Plant Physiol. 170 (3), 1757–1771. doi: 10.1104/pp.15.01546
Shumbe, L., D'Alessandro, S., Shao, N., Chevalier, A., Ksas, B., Bock, R., et al. (2017). METHYLENE BLUE SENSITIVITY 1 (MBS1) is required for acclimation of arabidopsis to singlet oxygen and acts downstream of β-cyclocitral. Plant Cell Environ. 40 (2), 216–226. doi: 10.1111/pce.12856
Sperling, U., van Cleve, B., Frick, G., Apel, K., Armstrong, G. A. (1997). Overexpression of light-dependent PORA or PORB in plants depleted of endogenous POR by far-red light enhances seedling survival in white light and protects against photooxidative damage. Plant J. 12 (3), 649–658. doi: 10.1046/j.1365-313x.1997.00649.x
Tanaka, M., Chock, P. B., Stadtman, E. R. (2007). Oxidized messenger RNA induces translation errors. Proc. Natl. Acad. Sci. U.S.A. 104 (1), 66–71. doi: 10.1073/pnas.0609737104
Wagner, D., Przybyla, D., Camp, R. O. D., Kim, C., Landgraf, F., Lee, K. P., et al. (2004). The genetic basis of singlet oxygen induced stress responses of arabidopsis thaliana. Science 306 (5699), 1183–1185. doi: 10.1126/science.1103178
Wang, L., Apel, K. (2018). Dose-dependent effects of 1O2 in chloroplasts are determined by its timing and localization of production. J. Exp. Bot. 70 (1), 29–40. doi: 10.1093/jxb/ery343
Wang, L., Kim, C., Xu, X., Piskurewicz, U., Dogra, V., Singh, S., et al. (2016). Singlet oxygen- and EXECUTER1-mediated signaling is initiated in grana margins and depends on the protease FtsH2. Proc. Natl. Acad. Sci. U.S.A. 113 (26), E3792–E3800. doi: 10.1073/pnas.1603562113
Wang, L., Leister, D., Guan, L., Zheng, Y., Schneider, K., Lehmann, M., et al. (2020). The arabidopsis SAFEGUARD1 suppresses singlet oxygen-induced stress responses by protecting grana margins. Proc. Natl. Acad. Sci. U.S.A. 117 (12), 6918–6927. doi: 10.1073/pnas.1918640117
Wilkinson, F., Helman, W. P., Ross, A. B. (1995). Rate constants for the decay and reactions of the lowest electronically excited singlet-state of molecular-oxygen in solution - an expanded and revised compilation. J. Phys. Chem. Ref Data 24 (2), 663–1021. doi: 10.1063/1.555965
Keywords: arabidopsis, singlet oxygen (1 O2), RNA oxidation, 8oxo-guanosine (8-oxoG), ROS - reactive oxygen species
Citation: Koh E, Brandis A and Fluhr R (2022) Plastid and cytoplasmic origins of 1O2-mediated transcriptomic responses. Front. Plant Sci. 13:982610. doi: 10.3389/fpls.2022.982610
Received: 30 June 2022; Accepted: 21 October 2022;
Published: 07 November 2022.
Edited by:
Christine Helen Foyer, University of Birmingham, United KingdomReviewed by:
Ralf Oelmüller, Friedrich Schiller University Jena, GermanyCopyright © 2022 Koh, Brandis and Fluhr. This is an open-access article distributed under the terms of the Creative Commons Attribution License (CC BY). The use, distribution or reproduction in other forums is permitted, provided the original author(s) and the copyright owner(s) are credited and that the original publication in this journal is cited, in accordance with accepted academic practice. No use, distribution or reproduction is permitted which does not comply with these terms.
*Correspondence: Eugene Koh, ZXVnZW5lQHRsbC5vcmcuc2c=
Disclaimer: All claims expressed in this article are solely those of the authors and do not necessarily represent those of their affiliated organizations, or those of the publisher, the editors and the reviewers. Any product that may be evaluated in this article or claim that may be made by its manufacturer is not guaranteed or endorsed by the publisher.
Research integrity at Frontiers
Learn more about the work of our research integrity team to safeguard the quality of each article we publish.