- 1College of Agronomy, Hunan Agricultural University, Changsha, China
- 2Hunan Provincial Key Laboratory of Rice and Rapeseed Breeding for Disease Resistance, Changsha, China
- 3Hunan Hybrid Rice Research Center, Hunan Academy of Agricultural Sciences, Changsha, China
- 4State Key Laboratory of Hybrid Rice, Changsha, China
Plant cell wall is a complex and changeable structure, which is very important for plant growth and development. It is clear that cell wall polysaccharide synthases have critical functions in rice growth and abiotic stress, yet their role in plant response to pathogen invasion is poorly understood. Here, we describe a dwarf and narrowed leaf in Hejiang 19 (dnl19) mutant in rice, which shows multiple growth defects such as reduced plant height, enlarged lamina joint angle, curled leaf morphology, and a decrease in panicle length and seed setting. MutMap analysis, genetic complementation and gene knockout mutant show that cellulose synthase-like D4 (OsCSLD4) is the causal gene for DNL19. Loss function of OsCSLD4 leads to a constitutive activation of defense response in rice. After inoculation with rice blast and bacterial blight, dnl19 displays an enhanced disease resistance. Widely targeted metabolomics analysis reveals that disruption of OsCSLD4 in dnl19 resulted in significant increase of L-valine, L-asparagine, L-histidine, L-alanine, gentisic acid, but significant decrease of L-aspartic acid, malic acid, 6-phosphogluconic acid, glucose 6-phosphate, galactose 1-phosphate, gluconic acid, D-aspartic acid. Collectively, our data reveals the importance of OsCSLD4 in balancing the trade-off between rice growth and defense.
Introduction
The growth of plants under natural conditions is constantly threatened by pathogens. Plant cell wall is a highly controlled and dynamic physiological structure, which is crucial to plant growth and development, and it is also the first front to fight against pathogens (Underwood, 2012; Bacete et al., 2018). Plant cell wall is mainly composed of cellulose, hemicellulose, pectin, lignin and glycoprotein (Somerville et al., 2004). As the most widely distributed and abundant polysaccharide, cellulose is produced by cellulose synthase (CESA) complexes (CSCs) on the plasma membrane (Lampugnani et al., 2019). The CESA superfamily also includes nine cellulose synthase-like (CSL) families, from CSLA to CSLH, and CSLJ (Yin et al., 2009). The CSLA family has been demonstrated to possess mannan and glucomannan synthase activity (Dhugga et al., 2004; Liepman et al., 2005; Goubet et al., 2009), while CSLC family members participate in the xyloglucan backbone synthesis (Cocuron et al., 2007; Kim et al., 2020). CSLF and CSLH exist only in grasses and are responsible for mixed linkage beta-glucan synthesis (Burton et al., 2006; Doblin et al., 2009; Jobling, 2015). CSLD gene family is the most similar CSL gene family to CESA family and also shows mannan synthase activity (Richmond and Somerville, 2000; Yin et al., 2011). In rice, a total of 45 CESA and CSL genes are identified, of which 11 were predicted to be OsCESA and 34 were predicted to be OsCSL (Wang et al., 2010). Except for a few genes, the role of most putative OsCESA and OsCSL genes in cell wall biosynthesis and remodelling is still elusive. OsCESA4, OsCESA7 and OsCESA9 are not functionally redundant in deposition of cellulose in the secondary wall (Tanaka et al., 2003). CslF6 mediates the biosynthesis of mixed linkage glucan in rice (Vega-Sánchez et al., 2012). OsCSLD1, ortholog of Arabidopsis gene AtCSLD3, is indispensable for root hair elongation and shows root-specific expression (Kim et al., 2007). OsCSLD4 plays an essential role in plant architecture of rice by regulating cell wall polysaccharide synthesis, and also participates in plant response to salt stress (Li et al., 2009; Hu et al., 2010; Wu et al., 2010; Luan et al., 2011; Yoshikawa et al., 2013; Ding et al., 2015; Zhao et al., 2022).
Alterations in plant cell wall component have been proven to impose a significant influence on disease resistance. By screening mutants with changed disease resistance, many mutants are turn out to be impaired with genes involved in cell wall biosynthesis (Zhao and Dixon, 2014). For instance, Arabidopsis mutants procuste1/isoxaben resistant 1/constitutive expression of VSP1 (prc1/ixr1/cev1), irregular xylem1/cesa8 (irx1/cesa8), irx3/cesa7 and irx5/cesa4, are defective in the biosynthesis of primary or secondary cell wall, in accompaniment with enhanced resistance to pathogens (Ellis et al., 2002; Hernández-Blanco et al., 2007). The rice loss-of-function mutant cslf6 displays strengthened resistance to a virulent isolate of Xanthomonas oryzae pv. oryzae (Xoo; Vega-Sánchez et al., 2012).
In plants, enhanced defense is usually accompanied by reduced costs of growth and reproduction, which is called the growth-defense trade-off and is a subtle and adaptive mechanism under adverse conditions (Figueroa-Macías et al., 2021). There is no doubt that phytohormones play a crucial role in the potential conflict between immune and growth signals. The resistance phenotype of prc1/ixr1/cev1 is related to the activation of ethylene (ET) and jasmonic acid (JA) signaling, while irx1/irx3/irx5-mediated resistance is partly attributed to constitutively activated abscisic acid (ABA) signaling pathway, not dependent on salicylic acid (SA), ET, and JA signaling (Ellis et al., 2002; Hernández-Blanco et al., 2007). Rice plants overexpressing OsWRKY45 are strongly resistant to both Magnaporthe oryzae (M. oryzae) and Xoo, due to a synergistic interaction between the cytokinin and SA signalling pathways (Jiang et al., 2013; Akagi et al., 2014). OsNPR1-induced growth inhibition is caused by disrupting the auxin pathway through promoting IAA-amido synthase expression (Li et al., 2016). OsBIHD1, as a critical molecular switch, balances growth and immunity in rice by coordinating ethylene-brassinosteroid pathway (Liu et al., 2017). The application of trade-off genes in crop breeding is confronted with the challenge of improving both disease resistance and yield potential. However, some progress has been made in optimizing the trade-off between yield and resistance. Manipulation of OsWRKY45 or IPA1 expression by different promoters develops disease-resistant rice without growth penalty (Goto et al., 2015; Liu et al., 2019).
OsCSLD4 is on the same allele as the reported gene NRL1/ND1/SLE1/DNL1/OsCD1 (Li et al., 2009; Hu et al., 2010; Wu et al., 2010; Luan et al., 2011; Yoshikawa et al., 2013; Ding et al., 2015). Recently, OsCSLD4 has been found to participates in rice salt stress response by mediating abscisic acid biosynthesis (Zhao et al., 2022). However, the function of OsCSLD4 in rice response to biotic stress remains unclear. In this study, we described a dwarf and narrowed leaf mutant dnl19 and found that OsCSLD4 was the candidate for DNL19. Our observation indicated that loss of function of OsCSLD4 compromised rice morphology, but greatly enhanced its resistance to pathogen. Therefore, OsCSLD4 was suggested to be an important regulator of the growth–defense trade-off in rice.
Materials and methods
Plant materials and growth conditions
The dnl19 mutant, initially identified from a T-DNA-inserted mutant pool, was in the japonica background of rice variety Hejiang 19 (Oryza sativa L. ssp. japonica). To construct OsCSLD4 knockout transgenic plants, the rice variety Nipponbare (Oryza sativa L. ssp. japonica) was used for transformation. The dnl19, as the pollen acceptor, was crossed with wild type Hejiang 19. The first generation (F1) plants were self-pollinated, and the second generation (F2) was used for genetic analysis. All plants were grown in a phytotron at 24-32°C with 12 h light/12 h dark, or in an experimental field under natural open-air condition.
Genetic transformation in rice
To complement the dnl19 mutant, the coding sequences (CDS) of OsCSLD4 was amplified and inserted into a binary vector pCAMBIA1300, under control of a maize ubiquitin promoter. The recombinant plasmid pCAMBIA1300-proUbi-OsCSLD4 was introduced into the dnl19 mutant. The positive transformants were identified by screening resistance to Hygromycin B (31282-04-9, Roche).
The OsCSLD4 knockout mutant was generated by Biorun Bio-tech. Co., Ltd. (Wuhan, China). The online tool DSDecodeM was used to analyze the sequencing results of oscsld4 mutant lines (Liu et al., 2015b).
Cross section and microscopy
Rice leaf blades and internodes were fully immersed in FAA fixative solution, dehydrated through a series of alcohol (30%, 50%, 75%, 85%, 90%, 95%, 100%) for one hour at each concentration, and then soaked in xylene for 3 times, 20 min each time, and finally embedded in paraffin. Tissues were sliced into 5 µm thick sections using a microtome (HistoCore AUTOCUT, Leica). Sections were put into the toluidine blue staining solution for about 2 minutes and observed under optical microscopy (Eclipse E100, Nikon).
MutMap analysis
Rice leaf genomic DNA was extracted, according to the CTAB method. Four different DNA samples, including two parents and two bulked DNA pools, were used for whole-genome resequencing analysis. The DNA pool was composed of equal DNA of 25 F2 plants with dwarf or normal morphology. To obtain high quality data, raw data was filtered by Phred score (Qphred) to remove adapter and low-quality reads. Then clean data was compared to the Nipponbare reference genome (https://rapdb.dna.affrc.go.jp/) using BWA software (http://bio-bwa.sourceforge.net/). Duplicates of the blast result was removed by picard (https://broadinstitute.github.io/picard/). Variation in single nucleotide polymorphism (SNP) and insertion-deletion (InDel) was investigated using GATK HaplotypeCaller tool (https://gatk.broadinstitute.org/hc/en-us). SNP-index was calculated, taking 500 kb as window and 50 kb as step.
RNA analysis
For transcriptome analysis, rice flag leaves of dnl19 and WT at heading stage were used for total RNA extraction, with CTAB-PBIOZOL reagent. The quality and concentration of total RNA were checked by a Nano Drop (Thermo Fisher Scientific, USA). Three biological replicates per sample were sent to BGI Shenzhen Co., Ltd. (Shenzhen, China) for mRNA library construction and sequencing. The RNA-seq data was analyzed on the online Dr. Tom system from BGI (https://biosys.bgi.com/) and has been deposited in National Center for Biotechnology Information (NCBI) Sequence Read Archive (SRA) database with accession number PRJNA853163.
For defense-responsive gene expression analysis, rice leaves at seedling and tillering stages were collected and used for total RNA extraction. To investigate the influence of Xoo infection on OsCSLD4 gene expression, leaves of Nipponbare at tillering stages were used for inoculation, and 2 cm leaf fragments next to cutting site were used for RNA extraction. RNA samples were reverse-transcribed with HiScript® II Q RT SuperMix for qPCR (R223-01, Vazyme). The Hieff® qPCR SYBR Green Master Mix (11201ES03, Yeasen) was used to perform real time-quantitative PCR (RT-qPCR) with the Real-Time System CFX96™ C1000 Thermal Cycler (BioRad), and gene expression was analysed by BioRad CFX Manager.
Pathogen inoculation
The fourth leaf blades of rice seedlings at five leaf stage were used for inoculation by M. oryzae isolate 110-2 strain from Hunan. A punch inoculation method was used for rice blast inoculation (Liu et al., 2015a). The M. oryzae isolate was cultured on oatmeal agar for 15 days at 28°C before sporulation. Spores were collected and spore suspension was adjusted to 5×105 spores/ml. The two injured dots on each leaf were dipped by 5 μl spore suspension. At 7 days post-inoculation (dpi), the disease lesion length was measured and DNA was extracted for analyzing the relative fungal biomass. Primers for the pot2 gene of M. oryzae and the ubiquitin (OsUbi) gene of rice genome were previously described (Li et al., 2017).
Xoo PXO99 was cultured on PSA medium plates (10 g/L peptone, 10 g/L sucrose, 1 g/L glutamic acid and 15 g/L agar) at 28°C for 2 days before they were suspended in sterilized water (OD600 = 0.5). A leaf-clipping method was used to inoculate rice leaves as previously described (Xu et al., 2019). The disease symptom was documented at 2 weeks after inoculation.
Metabonomic analysis
The aboveground tissues of rice were collected at tillering stage and sent to Biotree (https://www.biotree.cn) for metabolomic profiling. All samples were performed on an ultra-high-performance liquid chromatography (UHPLC) system with a Waters ACQUITY UPLC HSS T3 column coupled to a SCIEX QTRAP 6500+ mass spectrometer (AB Sciex, USA). The detailed experimental procedures and analytical methods were described (Zou et al., 2022).
Results
Pleiotropic phenotypes of rice dnl19 mutant
We isolated a rice mutant (dnl19) displaying stunted growth from T-DNA insertion mutant seeds. During the whole developmental period, the dnl19 mutant was shorter than the wild type (WT) (Figure 1; Supplementary Figure 1). At the seedling stage, dnl19 plants were easily distinguished from WT because of their short stature, narrow leaves and enlarged lamina joint angle (Supplementary Figures 1A–D). At the heading and mature stages, the height of dnl19 mutant was about half of that of the WT plants (Figure 1A; Supplementary Figure 1E). The flag leaf length and width, panicle length and internode elongation in dnl19 mutant were also investigated. We found that flag leaf length was shortened in mutant, and leaf width was reduced to approximately 50% of normal leaf width (Figures 1B, F). Panicle length and seed setting rate of dnl19 plants were obviously decreased (Figures 1C, G). The length of the first (1st), second (2nd), and third (3rd) internodes in mutant was decreased to 57%, 66%, and 80% of that of the WT plants, respectively (Figures 1D, H). These results indicated that the dwarfism of dnl19 was attributed to inhibited internode elongation, but not to fewer internodes. In addition, grain size was also affected in dnl19 mutant. Compared with wild type plants, the dnl19 plants had a significant decrease in grain width and 1000-grain weight (Figures 1E, I).
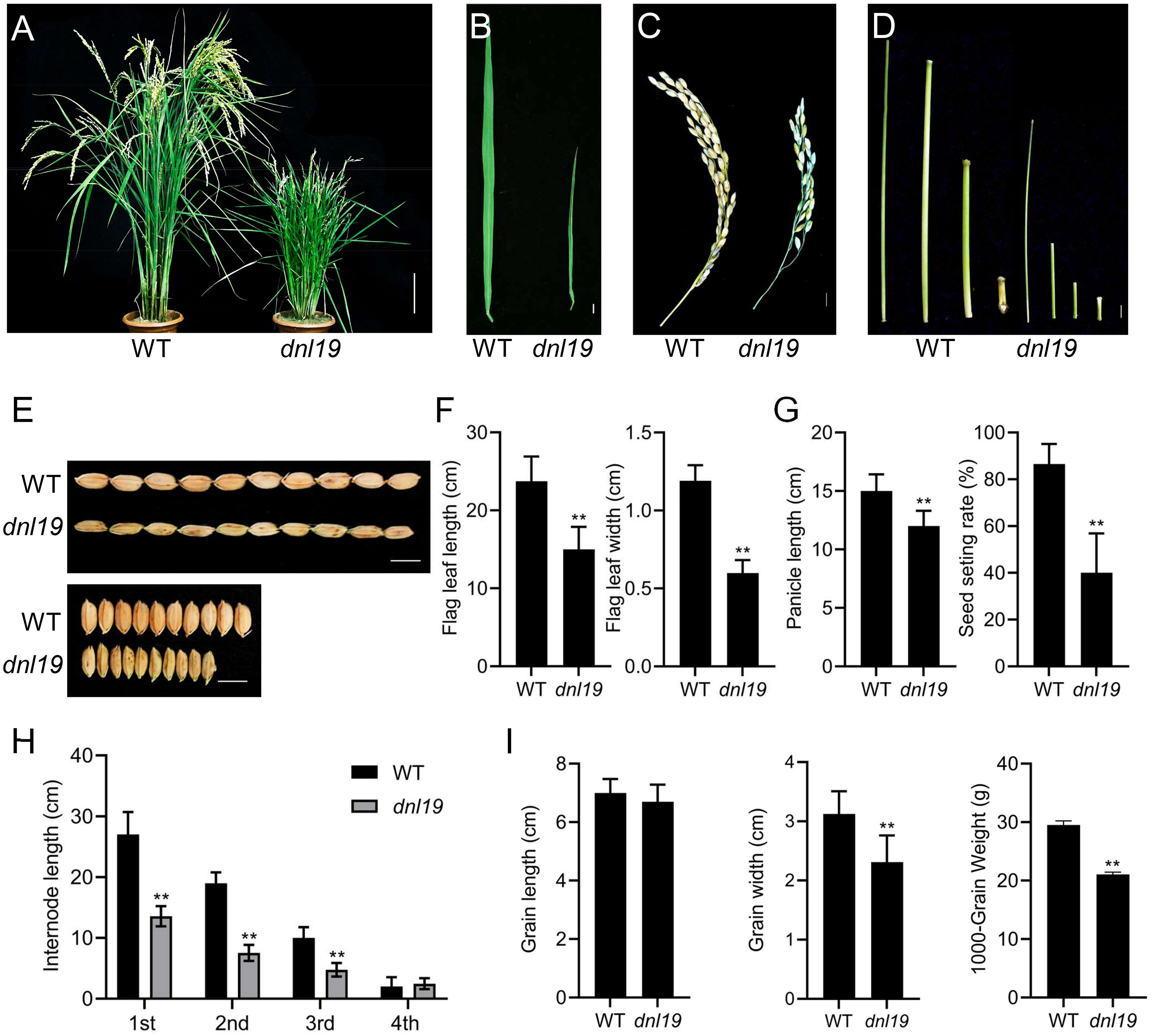
Figure 1 Characteristics of dnl19 mutant. (A) Dwarfism was observed in dnl19 plants at mature stage. Bar = 10 cm. (B) Flag leave of dnl19 was slenderer than that of wild type (WT). Bar = 1 cm. (C) Panicle morphology was affected in dnl19. Bar = 1 cm. (D) The culm of dnl19 was shorter than that of WT. Bar = 1 cm. (E) The grain shape was affected in dnl19 plants. Bar = 1 cm. (F) Both the flag leaf length and width were reduced in dnl19 mutant. Data are means ± SD (n ≥13, **P < 0.01, Student’s t-test). (G) The dnl19 plants displayed a decrease in panicle length and seed setting rate. Data are means ± SD (n≥10, **P < 0.01, Student’s t-test). (H) The 1st, 2nd and 3rd internodes of dnl19 were shortened. Data are means ± SD (n≥29, **P < 0.01, Student’s t-test). (I) Grain length, grain width and 1000-grain weight of WT and dnl19 plants were statistically analyzed. Data are means ± SD (**P < 0.01, Student’s t-test).
Altered leaf vein patterning and cell size of internode in dnl19
To describe the dnl19 mutant in detail, we analyzed the histology of leaf blade and internode sections in dnl19 mutant. Leaf veins are important components of leaf morphology. We found that the number of large veins was not affected in mutant, while the number of small veins was decreased by 27%, resulting in the narrow-leaf phenotypes (Figures 2A, D). Two large locules, called clear cells, are located in middle area of the midrib (Yamaguchi et al., 2004). The size of clear cells in dnl19 was strikingly reduced, mostly contributing to a decrease in midrib thickness (Figures 2B, D). Bulliform cells are mainly located on the upper epidermis of rice leaves and play a key role in regulating leaf rolling. We found that the dnl19 mutant possessed significantly smaller bulliform cells in leaf vein areas than the wild type (Figure 2C), which could explain the easily-curled morphology of dnl19 leaves. The examination of the longitudinal sections of the 2nd internodes revealed that the parenchymal cells were irregularly arranged and the number of horizontal cell layers were decreased in dnl19, and the cell size was enlarged compared with the wild type (Figure 2E). Therefore, these results suggested that the shortened length in the 2nd internode was due to a reduction in cell number, not in cell size.
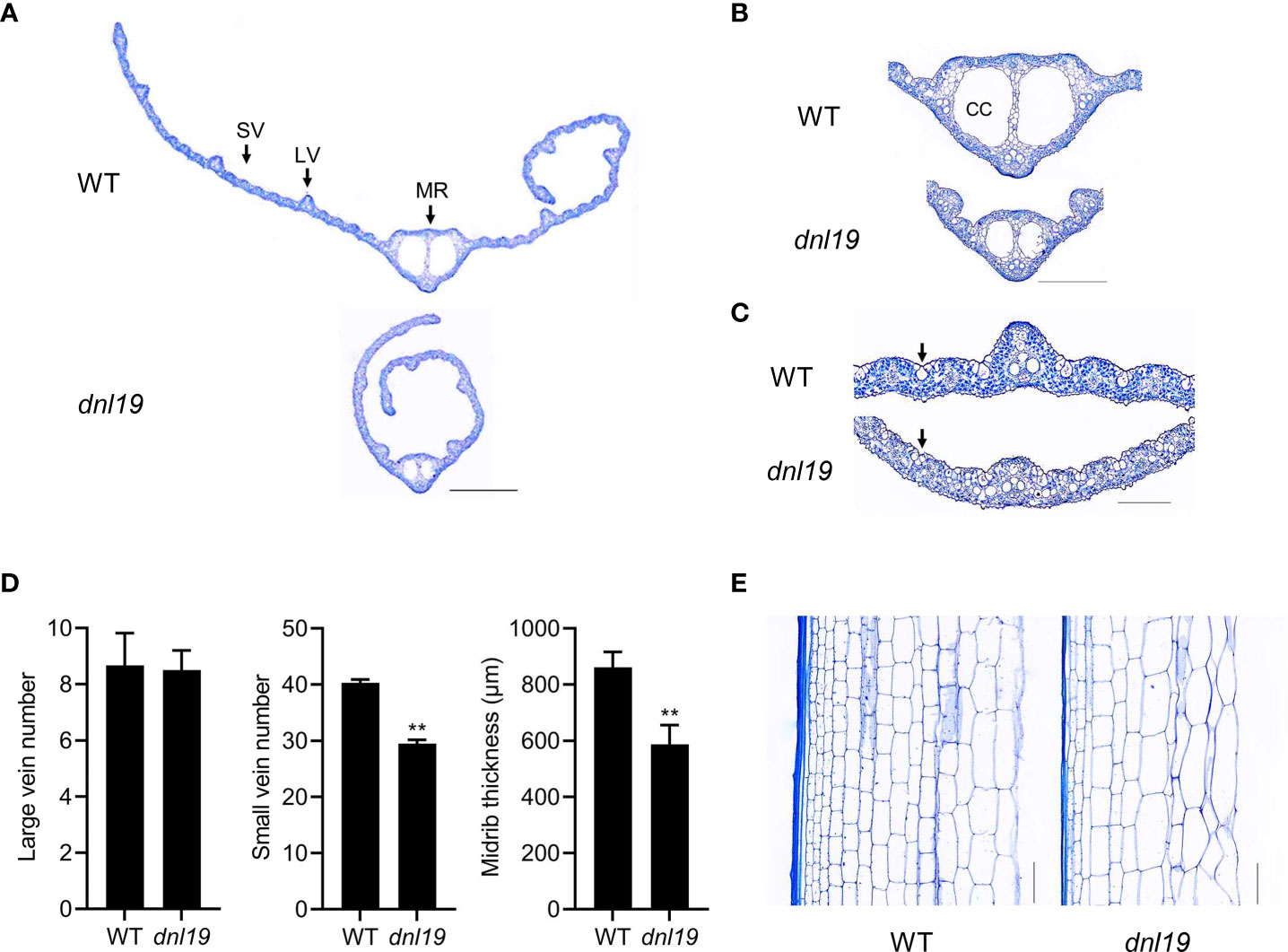
Figure 2 Effects of the dnl19 mutation on leaf and culm structure. (A) Cross section of flag leaf blades in WT and dnl19 plants. SV: small vein, LV: large vein, MR: midrib. Bar = 1 mm. (B, C) Magnified images of the leaf vein shown in (A). CC: clear cell, Arrow: bulliform cell. Bar = 0.5 mm in (B) and 0.2 mm in (C). (D) Number of large and small veins, and midrib thickness in leaf blades of WT and dnl19 plants. Data are means ± SD (n=10, **P < 0.01, Student’s t-test). (E) Longitudinal section of the 2nd internodes at the mature stage from WT and dnl19. Bar = 0.1 mm.
Isolation of genomic flanking sequences of T-DNA insertion in dnl19 mutant
The dnl19 mutant was obtained from the progeny of transgenic plants transformed with the pCAMBIA1300 binary vector. To identify the T-DNA insertion site of dnl19 mutant, high-efficiency thermal asymmetric interlaced PCR (hiTAIL-PCR) procedure was applied (Liu and Chen, 2007). The result showed that the T-DNA element was inserted in the intron region of CHR702 (LOC_Os06g08480) (Supplementary Figure 2A), whose protein product belonged to Chromodomain-Helicase/ATPase-DNA-binding domain (CHD) family. However, it is well documented that T-DNA insertion mutant and knockdown of CHR702 did not cause any morphological differences (Hu et al., 2012). Besides, it was observed that the transgene-free dnl19, also had a dwarf morphology (Supplementary Figure 2B). Therefore, we concluded that CHR702 was not the causal gene of dnl19 mutant.
Identification of the candidate region and the causal SNPs of dnl19 mutant by MutMap analysis
When dnl19 was crossed with the original strain (Hejiang 19), the F1 plants showed normal plant height relative to the WT (Supplementary Figure 3A). In F2 generation, the segregation model of normal stature and short stature was in good agreement with the expected single genetic ratio of 3:1, indicating that the dnl19 phenotype was controlled by a single recessive gene (Supplementary Table 1). A MutMap approach was employed to identify the DNL19 gene. Samples of Hejiang 19, dnl19, normal pool and dwarf pool were prepared for whole-genome resequencing analysis with 30×sequencing depth. The clean data was compared to the Nipponbare reference sequence and covered at least 97% of the rice genome. Taking 500 kb as window and 50 kb as step, SNP-index was calculated. According to SNP-index ≥0.6 of sliding window, the candidate interval was obtained on chromosome 9, 11 and 12 (Supplementary Figure 3B).
There were 13 SNPs in the candidate region with ΔSNP-index (SNP-index difference of two pools) >0.6, most of which are located in the non-coding region (Table 1). We found that the SNP at nucleotide position 22,606,187 was in the first exon of the gene OsCSLD4 (LOC_Os12g36890), previously reported to function in regulating cell wall polysaccharide synthesis (Luan et al., 2011). A C base deletion at codon 302, leading to a frame-shift and introducing a premature stop codon at nucleotide 985 of OsCSLD4 CDS (Figure 3A). Sequencing analysis confirmed the mutation site and the C deletion could be the reasons for the abnormal phenotype of dnl19 mutant (Figure 3B).
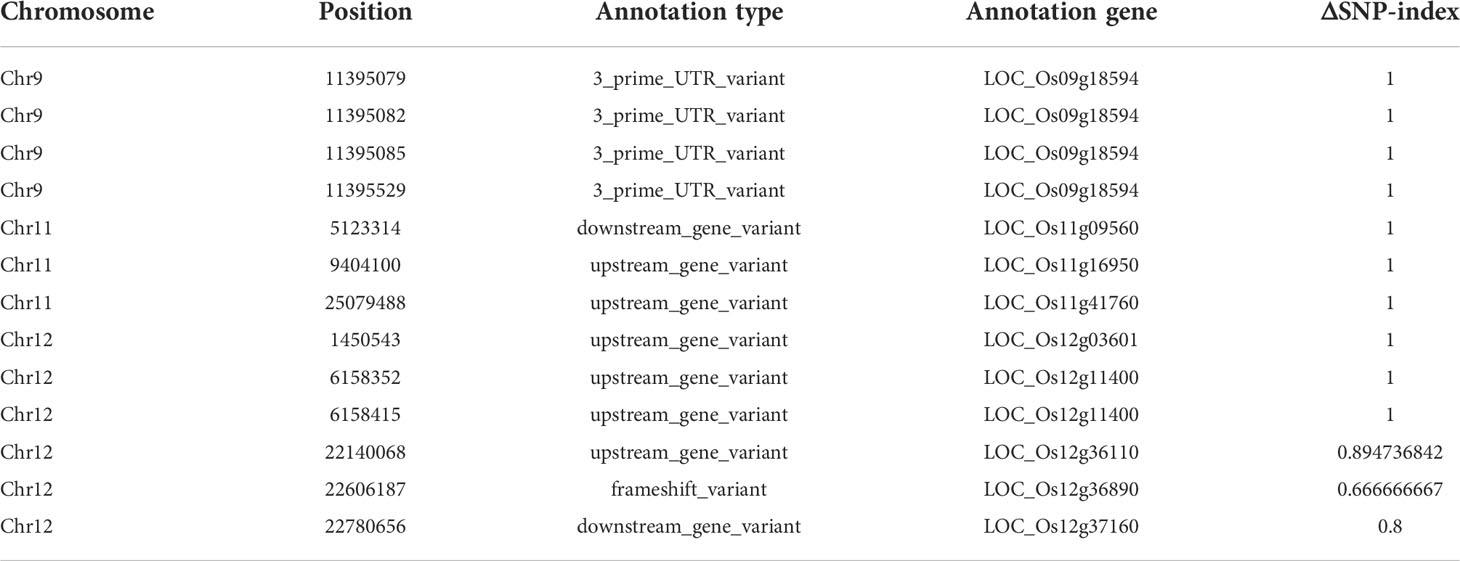
Table 1 Summary of candidate genes containing SNPs with ΔSNP-index>0.6 on genomic region of chromosomes.
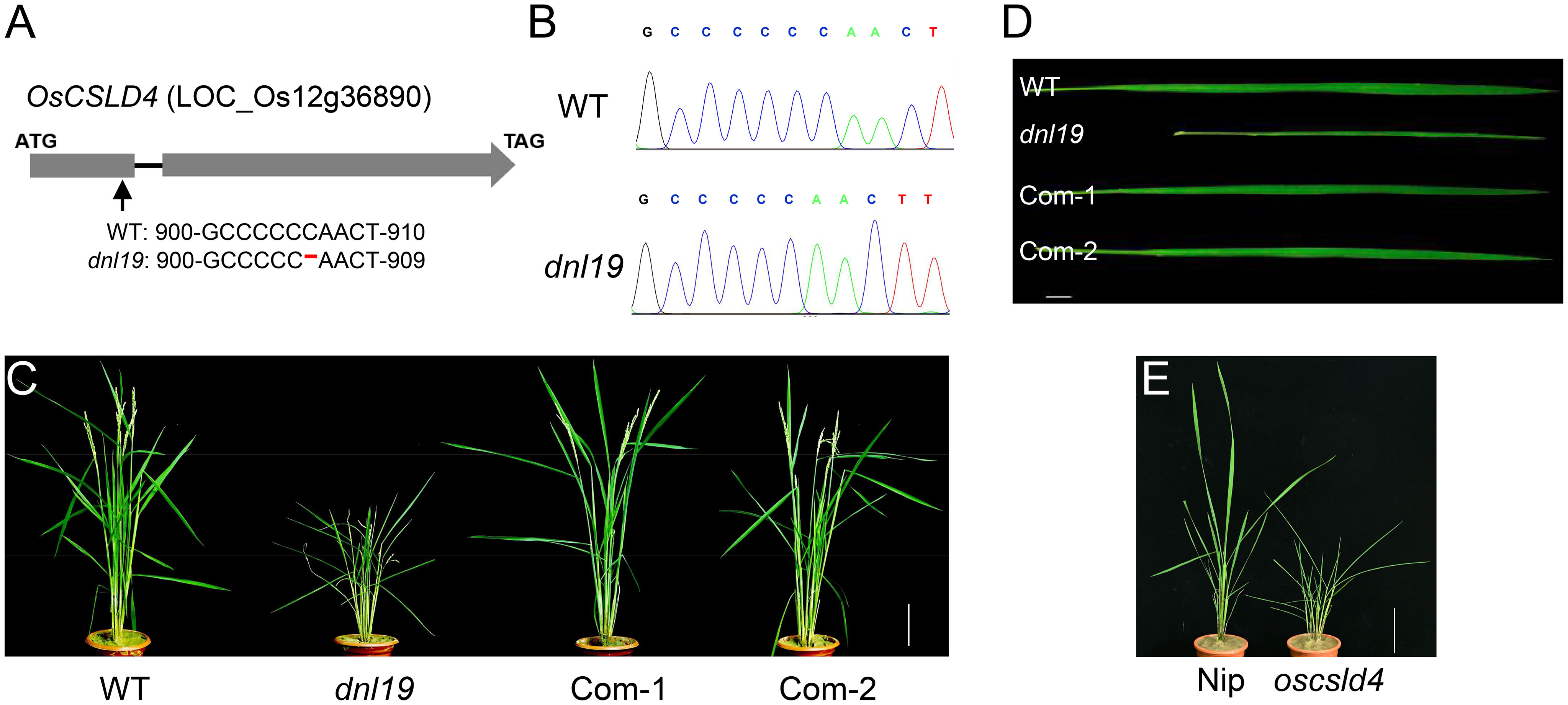
Figure 3 OsCSLD4 is the causal gene of dnl19 mutant. (A) Schematic diagram (not in scale) illustrates the InDel site in OsCSLD4. The arrow indicates the position of the dnl19 mutation, and the red dash indicates a C base deletion. (B) Sequencing results of the OsCSLD4 in wild type and dnl19 mutant. (C) Leaf morphology of the wild plant, dnl19 and functionally complemented plants (Com-1, Com-2). Bar = 1 cm. (D) Gross morphology of WT, dnl19, Com-1 and Com-2 at the maturing stage. Bar = 10 cm. (E) Gross morphology of Nipponbare (Nip) and oscsld4 at the tillering stage. Bar = 10 cm.
To rescue the various developmental defects of dnl19 mutant, we expressed OsCSLD4 in dnl19 plants under the control of the maize ubiquitin promoter. After the gene was successfully introduced into the mutant dnl19, the phenotype of dnl19 was completely inhibited. As for plant height and the morphological features of leaves, there is no difference between complementary plants and wild type plants (Figures 3C, D). In addition, the CRISPR/Cas9 system was employed to generate mutant named oscsld4 in the Nipponbare background. Similar to dnl19, the oscsld4 in T0 generation also produced a dwarf plant with altered leaves (Figure 3E; Supplementary Figure 3C).
Transcriptome analysis of dnl19 mutant by RNA-seq
In plants, it is common that impaired growth and development is accompanied by enhanced defense. To clarify whether the OsCSLD4 was involved in rice defense response, transcriptome of dnl19 and WT flag leaves at the heading stage were studied by RNA-seq analysis. 27,518 expressed genes were detected in WT and 27,664 expressed genes in dnl19, among which 26,076 genes were common ones (Figure 4A). Based on the threshold values of fold change ≥1.0 and q-value <0.05, a total of 867 genes were differentially expressed between dnl19 and WT flag leaves, including 684 upregulated and 183 downregulated ones (Figure 4B; Supplementary Table 3). Besides cell wall organization, gene ontology (GO) classification of biological process of the DEGs were abundantly enriched in defense response (Figure 4C). And among those DEGs, we found that the transcripts of pathogenesis-related (PR) gene, chitinase gene, Gns6 (Wang et al., 2021), OsMBL1 (Han et al., 2019), and Xa21 (Song et al., 1995), were highly upregulated in dnl19 mutant (Figure 4D). The reported narrow leaf and dwarf1 (nd1) was a OsCSLD4 functional disruption mutant in Zhongxian 3037 indica variety (Li et al., 2009). Two-week old seedlings of nd1 were treated with water (mock) or NaCl (150 mM NaCl) for two days, and sampled for RNA-seq analysis (Zhao et al., 2022). According to the available data, we found that lots of PR genes were identified in upregulated DEGs of nd1 under mock treatment; Gns6, OsMBL1, and Xa21 showed an increased transcript accumulation in nd1 (Supplementary Figure 4). We checked the relative expression of some defense-responsive genes in dnl19 and WT at both seedling stage and tillering stage using qPCR (Figure 4E). The PR genes, including OsPR1a, OsPR1b, OsPR2, OsPR5, OsPR10a and OsPR10b, were strikingly upregulated in 14-day-old plants of dnl19. And their expression, except for OsPR1a and OsPR2, was differently increased at tillering stage compared to control plants. We also found that transcripts of CHITs, other than CHIT13, were highly accumulated in dnl19 at both seedling and tillering stage. Taken together, these results suggested that disruption of OsCSLD4 in dnl19 activated the expression of defense-responsive genes.
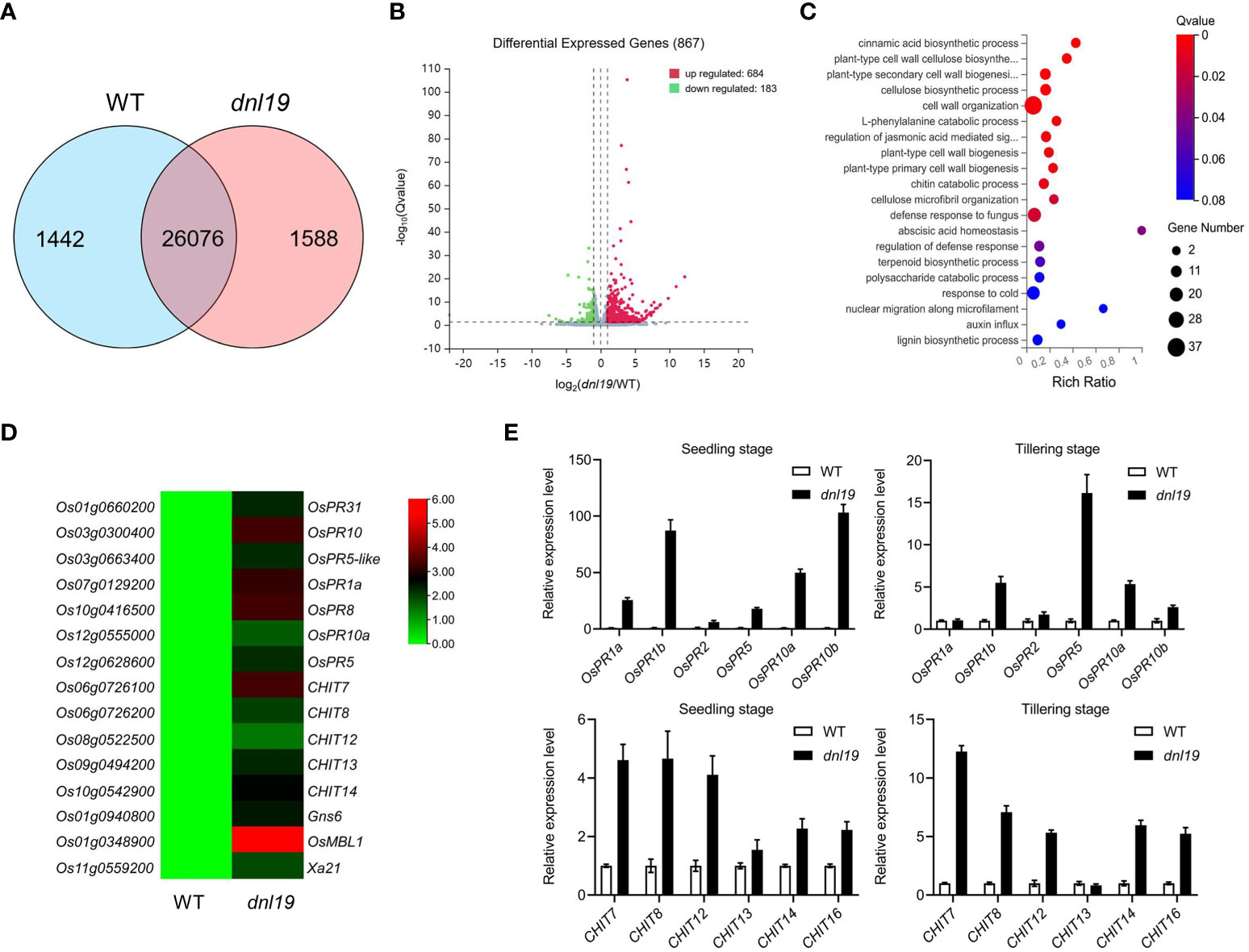
Figure 4 Global transcriptome analysis of dnl19 mutant. (A) Venn diagram of common genes in dnl19 and WT. (B) Volcano map of differentially expressed genes (DEGs) between dnl19 and WT. (C) Significantly enriched GO terms of DEGs between dnl19 and WT. GO terms belong to biological processes. (D) Heatmap of pathogen-associated genes transcription in dnl19 and WT. (E) Investigation of the relative expression of pathogen-associated genes by qRT-PCR. The expression of OsActin1 was used as an internal control.
Altered resistance to M. oryzae and Xoo in dnl19 mutant
Rice blast and bacterial blight are common and highly destructive diseases of rice. To investigate the ability against rice blast, dnl19 was evaluated by punch inoculation at seedling stages. The results showed that the dnl19 leaves had much less lesions after inoculation with M. oryzae isolate 110-2 strain (Figure 5A). The relative fungal biomass of each lesion was studied, and there were fewer fungi growing in the mutant leaves (Figure 5B).
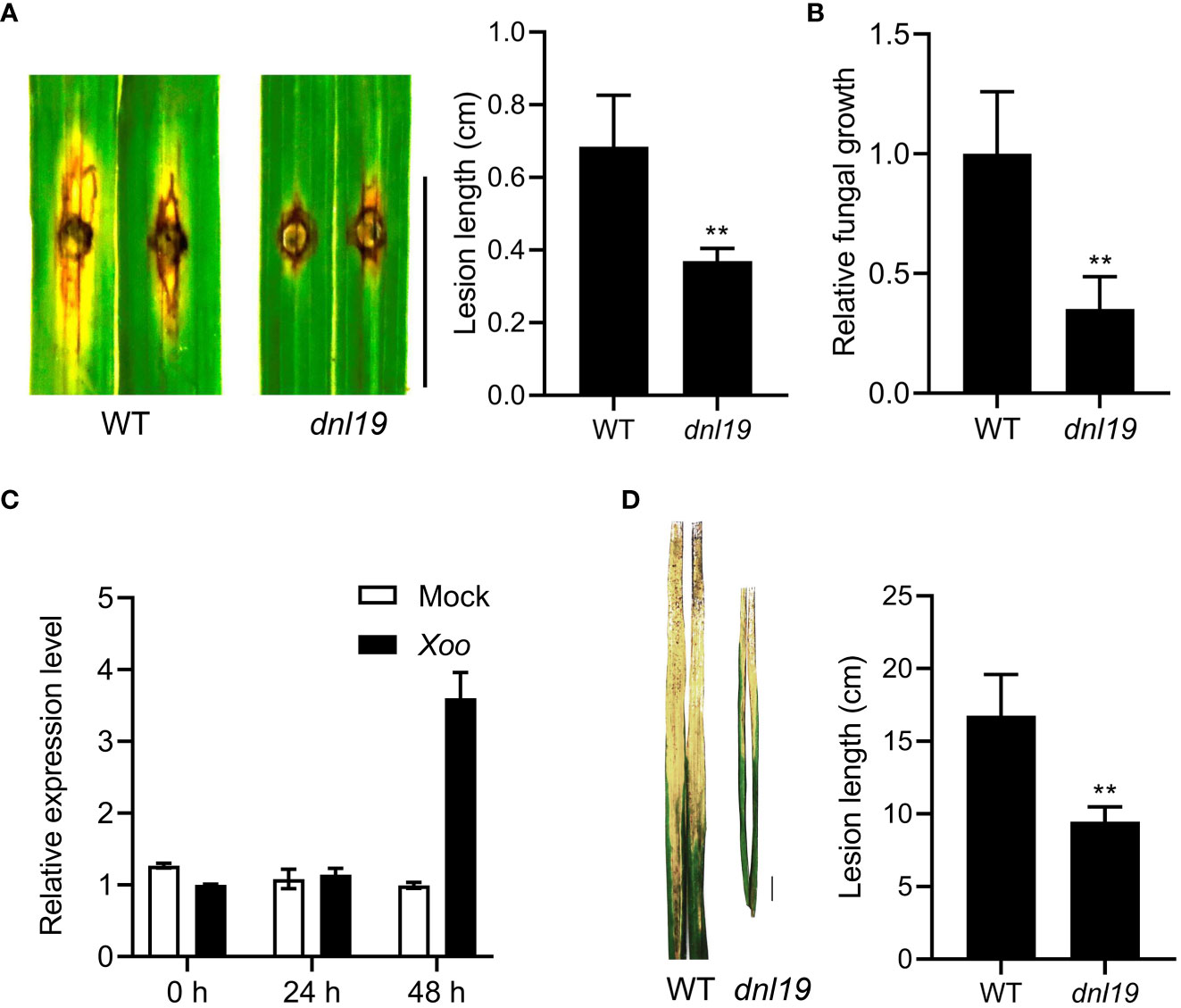
Figure 5 Phenotypical characterization of dnl19 and wild type against M. oryzae and Xoo. (A) The dnl19 mutant was inoculated with the M. oryzae isolate. Blast resistance was evaluated by punch inoculation. Lesion length were determined on leaves at 7 days after inoculation. Bar = 1 cm. Data are means ± SD (n=6, **P < 0.01, Student’s t-test). (B) Fungal growth was quantified by qPCR. Data are means ± SD (n=6, **P < 0.01, Student’s t-test). (C) The change of relative expression level of OsCSLD4 in response to Xoo strain PXO99 treatment. The leaves of Nipponbare plants at tillering stage were used for inoculation. The expression of OsActin1 was used as an internal control. (D) Phenotypes of disease reactions in dnl19 flag leaves after inoculation with Xoo strain PXO99. Bar = 1 cm. Disease lesion length of wild type and mutant was measured at 2 weeks after inoculation. Data are means ± SD (n≥10, **P < 0.01, Student’s t-test).
In order to study the resistance of dnl19 mutant to bacterial blight, we first tested whether OsCSLD4 was responsive to Xoo strain PXO99 infection. We analyzed the expression of OsCSLD4 in Nipponbare leaves inoculated with PXO99 at tillering stage. The result showed that the expression of OsCSLD4 was markedly increase after 48 hours of treatment (Figure 5C). Then PXO99 was inoculated on the flag leaf of the dnl19 mutant by tip-cutting method. At 2 weeks after inoculation, the dnl19 showed significantly shorter lesion length than WT (Figure 5D). Taken together, these results suggested that loss of OsCSLD4 gene function enhanced rice disease resistance.
Metabolic profiling analysis of dnl19 mutant
To understand the metabolic effect of the OsCSLD4 mutation, we applied widely targeted metabolomics using UHPLC-MS to investigate the chemical change of dnl19 mutant. 678 metabolites were identified in total, including 118 flavonoids, 64 alkaloids, 64 terpenes, 58 phenols, 43 amino acids and derivatives, 27 fatty acyls, 24 steroids and steroid derivatives, 22 nucleotides and derivatives, 22 phenylpropanoids, 19 coumarins, and others (Supplementary Table 4). The dnl19 group and the WT group were clearly separated by score plots of orthogonal partial least-squares discriminant analysis (OPLS-DA) (Figure 6A). The quality and validity of the OPLS-DA model were evaluated through 7-fold cross validation and permutation tests, respectively (Figure 6B). Any metabolite with variable importance in the projection (VIP) values ≥1, and P-value<0.05, was selected as differential metabolites. A total of 69 differential metabolites were identified; among them, there were 26 upregulated and 43 downregulated metabolites in dnl19 mutant (Figure 6C; Supplementary Figure 5A; Supplementary Table 5). The upregulated metabolites included L-valine, L-asparagine, L-histidine, L-alanine, gentisic acid and other important metabolites. Meanwhile, downregulated metabolites included L-aspartic acid, malic acid, 6-phosphogluconic acid, glucose 6-phosphate, galactose 1-phosphate, gluconic acid, D-aspartic acid and so on. Based on the KEGG metabolic pathway analysis, the differential metabolites were enriched in 15 differentially metabolic pathways (Supplementary Figure 5B). Differential abundance score (DA Score) analysis was applied to reflect the overall change of all differential metabolites in a pathway. The pathways, including vitamin B6 metabolism, cyanoamino acid metabolism, aminoacyl-tRNA biosynthesis, biosynthesis of amino acids and D-amino acid metabolism, tend to be upregulated. And cysteine and methionine metabolism, pentose phosphate pathway, carbon fixation and carbon metabolism tend to be downregulated (Figure 6D).
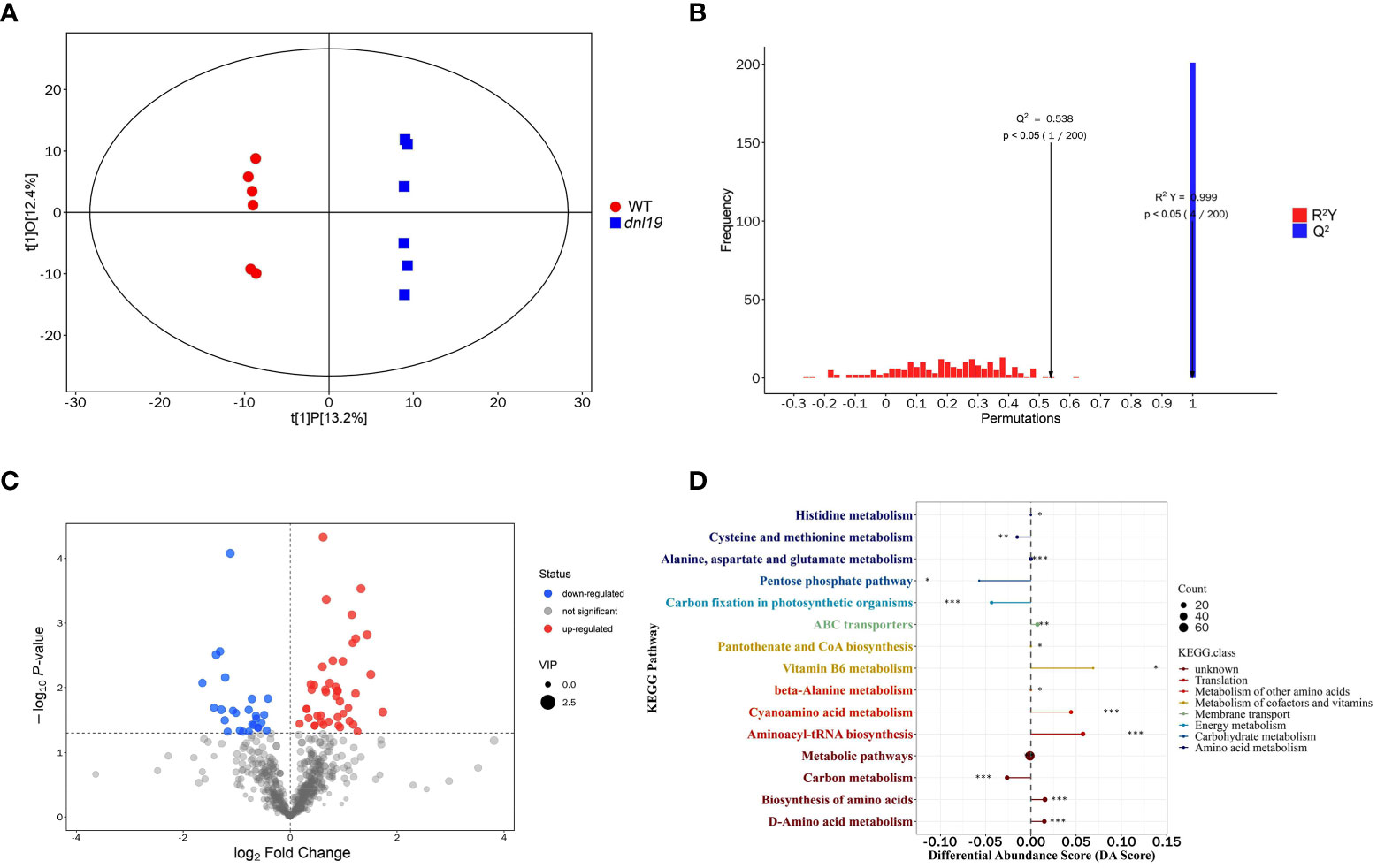
Figure 6 Widely targeted metabolomics analysis of dnl19 mutant. (A) Scatter plot of OPLS-DA scores for WT group versus dnl19 group. (B) Permutation tests of OPLS-DA model. The X-axis indicates the accuracy of the OPLS-DA model, and the Y-axis indicates the accuracy frequency of 200 models in 200 permutation tests. R2(Y) = 0.999, Q2 = 0.538. (C) Volcano map of differential metabolites with VIP value ≥1 and P-value<0.05. The abscissa represents the fold change of each metabolite, and the ordinate represents the P-value by student’s t-test. (D) Analysis of overall changes of KEGG metabolic pathway. Each dot represents a metabolic pathway. The X-axis was the differential abundance (DA) score, and the Y-axis was the ID number of KEGG metabolic pathway. * represents significance. * P < 0.05, ** P < 0.01, *** P < 0.001.
Discussion
Plants have evolved a precise mechanism to fine-tune the balance between growth and defense, which facilitates to ensure survival under adverse conditions. Cell wall biosynthesis has complex crosstalk with plant growth and defense. A significant body of genetic studies have shown that alteration of plant cell wall components affects the defense against pathogens (Zhao and Dixon, 2014). However, the detailed characterization of cell wall polysaccharide synthesis enzymes in rice disease response is still obscure. Previous studies have demonstrated that OsCSLD4 not only plays important roles in rice growth and development, but also participates in rice salt stress response by mediating abscisic acid biosynthesis (Li et al., 2009; Hu et al., 2010; Wu et al., 2010; Luan et al., 2011; Yoshikawa et al., 2013; Ding et al., 2015; Zhao et al., 2022). Here, we describe in detail the characteristics of dnl19 mutant, cloning of DNL19 with MutMap approach, gene transcriptome analysis, pathogen inoculation and metabolomic analysis. All the data indicate that OsCSLD4 is an important trade-off gene between rice growth and defense.
As we have illustrated, the dnl19 mutant displayed a series of serious developmental defects (Figure 1; Supplementary Figure 1). Leaf morphology is one of the decisive factors of ideal plant architecture of rice. Moderate leaf curling is beneficial to photosynthesis and increase crop yield. The identified genes regulating leaf rolling are divided into five categories, among which, the changes of bulliform cell are considered to be the major cause (Xu et al., 2018). The leaf width of dnl19 was significantly reduced, and its bulliform cells were smaller than those of wild type (Figure 2). Plant height is an important agronomic trait, which is closely related to lodging resistance. Most rice mutants with altered plant stature are identified to be associated with plant hormones gibberellins (GAs) and brassinosteroids (BRs) (Wang and Li, 2008). However, unlike GA deficient or BR deficient mutants, such as sdg721, sdg705, d61 and brd1-1 (Yamamuro et al., 2000; Hong et al., 2002; Jiang et al., 2018), there was no decrease in cell length in the internode region of dnl19 mutant (Figure 2). It is speculated that the pathway related to OsCSLD4 may be another way to control plant height, different from the plant hormone signal transduction pathway (Luan et al., 2011).
Many genes, which serve as the master regulators of the growth-defense trade-off, have been identified in rice. It was observed in transgenic rice overexpressing OsWRKY45 and IPA1 or knockout of OsDOF11 and OsALDH2B1, that compromised plant morphology often brings about defense activation (Jiang et al., 2013; Akagi et al., 2014; Wu et al., 2018; Liu et al., 2019; Ke et al., 2020). We found that the expression levels of defense-related genes were markedly upregulated both in OsCSLD4 knockout mutants dnl19 and nd1, even if these two mutants were in distinct genetic background and samples for RNA-seq analysis were different (Figure 4; Supplementary Figure 4). In accordance with a constitutively activated defense response, the dnl19 mutant had an enhanced resistance to rice blast and bacterial blight, two major biotic constraints that limit rice productivity (Figure 5).
The growth-defense trade-off process is considered to involve readjusting the allocation of resources to different pathways. Energy supply is essential for plants to combat with pathogens, which is achieved through primary metabolic activities (Bolton, 2009). Energy production involves many physiological processes, such as glycolysis pathway, pentose phosphate pathway, tricarboxylic acid (TCA) cycle and respiratory electron transport chain pathway (Less et al., 2011). Glucose 6-phosphate is formed in the first step of glycolysis, and 6-phosphogluconic acid is an intermediate product in pentose phosphate pathway, which begins with glucose 6-phosphate; malic acid, a four-carbon dicarboxylic acid, is a key intermediate of the TCA cycle (Fernie et al., 2004). We found that the relative contents of glucose 6-phosphate, 6-phosphogluconic acid, and malic acid were decreased in dnl19 mutant (Supplementary Figure 5; Supplementary Table 5). It is possible that the energy saved by down regulating primary metabolism is transferred and used for defense response. Amino acids are closely related to plant defense, and the variation in amino acid levels is determined by pathogen species during plant-pathogen interaction (Ward et al., 2010). It has been reported that the level of asparagine was positively correlated with disease resistance in pepper and tomato (Hwang et al., 2011; Seifi et al., 2014). In dnl19 mutant, L-asparagine was among the upregulated amino acids (Supplementary Figure 5; Supplementary Table 5). Secondary metabolites are small molecular compounds with biological activities, which regulate the growth and development of rice and promote disease and insect resistance (Wang et al., 2018). According to our results, the differential metabolites are mainly enriched in secondary metabolic pathways, including 17 flavonoids, 4 alkaloids, 6 terpenes, 6 phenols, and 2 steroids and steroid derivatives, suggesting that the dysfunction of OsCSLD4 has a notable impact on the production of secondary metabolites.
OsCSLD4 was related with hemicellulose polysaccharides, and mutation in OsCSLD4 altered the content of xylose, cellulose, arabinose, homogalacturonan (Li et al., 2009). The nd1 with changed cell wall composition reduced ABA content and impaired expression of ABA synthesis and response genes in an unknown mechanism (Zhao et al., 2022). It seems that OsCSLD4 has opposite effects on the response of rice to abiotic and biological stresses, although the expression level of OsCSLD4 is upregulated under salt stress or after pathogen inoculation (Figure 5). In dnl19 mutant, transcripts of pathogenesis-related genes (OsPR1a, OsPR1b, OsPR2, OsPR5, OsPR10a and OsPR10b) and chitinase genes are highly accumulated, possibly supporting the notion that the basic energy of plants is more used for synthesis of defense responsive proteins. In addition, the enhanced disease resistance of dnl19 mutant with a dwarf phenotype may also involve the activation of cell wall integrity (CWI) signaling. The plant CWI monitors the state of cell wall and triggers cell wall metabolism in response to adaptive changes in cell wall damage (Vaahtera et al., 2019). The Arabidopsis dwarf mutants, which are defective in lignin biosynthetic enzymes hydroxycinnamoyl CoA:shikimate/quinate hydroxycinnamoyl transferase (HCT) and cinnamoyl CoA reductase 1 (CCR1), exhibit extensive cell wall remodeling and release pectic oligosaccharide elicitors to activate defense-related genes expression (Gallego-Giraldo et al., 2020). In general, specific oligosaccharides are produced as a major plant-derived damage-associated molecular pattern (DAMP) during pathogen infection and can activate the plant immune response, such as 31-β-D-Cellobiosyl-glucose and 31-β-D-Cellotriosyl-glucose, which are released from the hemicellulose of rice cell wall during M. oryzae infection (Yang et al., 2021). However, it is unclear whether the dnl19 or other OsCSLD4 null mutants, defective in cell wall biosynthesis, will also release or provide precursors for oligosaccharide signal molecules to constitutively trigger plant defense response, which needs to be further investigated.
Data availability statement
The datasets presented in this study can be found in online repositories. The names of the repository/repositories and accession number(s) can be found in the article/Supplementary Material.
Author contributions
XL, ZY and WY performed experiments; XL and ZY analyzed data and wrote original draft; YW, SC, JL, XDL, FW, GZ, and YX provided suggestions and methodologies; YX, HD and WT designed experiments, reviewed and edited draft. All authors contributed to the article and approved the submitted version.
Funding
This research was funded by the National Natural Science Foundation of China (32172078), the Science and Technology Innovation Program of Hunan Province (2021NK1001), Natural Science Foundation of Hunan Province (2021JJ40238 and 2022JJ30285), and Hunan Provincial Innovation Foundation for Postgraduate (CX20210665).
Conflict of interest
The authors declare that the research was conducted in the absence of any commercial or financial relationships that could be construed as a potential conflict of interest.
Publisher’s note
All claims expressed in this article are solely those of the authors and do not necessarily represent those of their affiliated organizations, or those of the publisher, the editors and the reviewers. Any product that may be evaluated in this article, or claim that may be made by its manufacturer, is not guaranteed or endorsed by the publisher.
Supplementary material
The Supplementary Material for this article can be found online at: https://www.frontiersin.org/articles/10.3389/fpls.2022.980424/full#supplementary-material
Supplementary Table 4 | All metabolites identified in WT and dnl19 mutant.
Supplementary Table 5 | Differential metabolites in WT and dnl19 mutant.
References
Akagi, A., Fukushima, S., Okada, K., Jiang, C. J., Yoshida, R., Nakayama, A., et al. (2014). WRKY45-dependent priming of diterpenoid phytoalexin biosynthesis in rice and the role of cytokinin in triggering the reaction. Plant Mol. Biol. 86, 171–183. doi: 10.1007/s11103-014-0221-x
Bacete, L., Mélida, H., Miedes, E., Molina, A. (2018). Plant cell wall-mediated immunity: cell wall changes trigger disease resistance responses. Plant J. 93, 614–636. doi: 10.1111/tpj.13807
Bolton, M. D. (2009). Primary metabolism and plant defense-fuel for the fire. Mol. Plant Microbe Interact. 22, 487–497. doi: 10.1094/mpmi-22-5-0487
Burton, R. A., Wilson, S. M., Hrmova, M., Harvey, A. J., Shirley, N. J., Medhurst, A., et al. (2006). Cellulose synthase-like CslF genes mediate the synthesis of cell wall (1,3;1,4)-β-D-glucans. Science 311, 1940–1942. doi: 10.1126/science.1122975
Cocuron, J. C., Lerouxel, O., Drakakaki, G., Alonso, A. P., Liepman, A. H., Keegstra, K., et al. (2007). A gene from the cellulose synthase-like c family encodes a β-1,4 glucan synthase. Proc. Natl. Acad. Sci. U. S. A. 104, 8550–8555. doi: 10.1073/pnas.0703133104
Dhugga, K. S., Barreiro, R., Whitten, B., Stecca, K., Hazebroek, J., Randhawa, G. S., et al. (2004). Guar seed β-mannan synthase is a member of the cellulose synthase super gene family. Science 303, 363–366. doi: 10.1126/science.1090908
Ding, Z., Lin, Z., Li, Q., Wu, H., Xiang, C., Wang, J. (2015). DNL1, encodes cellulose synthase-like D4, is a major QTL for plant height and leaf width in rice (Oryza sativa l.). Biochem. Biophys. Res. Commun. 457, 133–140. doi: 10.1016/j.bbrc.2014.12.034
Doblin, M. S., Pettolino, F. A., Wilson, S. M., Campbell, R., Burton, R. A., Fincher, G. B., et al. (2009). A barley cellulose synthase-like CSLH gene mediates (1,3;1,4)- β-d-glucan synthesis in transgenic Arabidopsis. Proc. Natl. Acad. Sci. U. S. A. 106, 5996–6001. doi: 10.1073/pnas.0902019106
Ellis, C., Karafyllidis, I., Wasternack, C., Turner, J. G. (2002). The Arabidopsis mutant cev1 links cell wall signaling to jasmonate and ethylene responses. Plant Cell 14, 1557–1566. doi: 10.1105/tpc.002022
Fernie, A. R., Carrari, F., Sweetlove, L. J. (2004). Respiratory metabolism: glycolysis, the TCA cycle and mitochondrial electron transport. Curr. Opin. Plant Biol. 7, 254–261. doi: 10.1016/j.pbi.2004.03.007
Figueroa-Macías, J. P., García, Y. C., Núñez, M., Díaz, K., Olea, A. F., Espinoza, L. (2021). Plant growth-defense trade-offs: molecular processes leading to physiological changes. Int. J. Mol. Sci. 22, 693. doi: 10.3390/ijms22020693
Gallego-Giraldo, L., Liu, C., Pose-Albacete, S., Pattathil, S., Peralta, A. G., Young, J., et al. (2020). ARABIDOPSIS DEHISCENCE ZONE POLYGALACTURONASE 1 (ADPG1) releases latent defense signals in stems with reduced lignin content. Proc. Natl. Acad. Sci. U. S. A. 117, 3281–3290. doi: 10.1073/pnas.1914422117
Goto, S., Sasakura-Shimoda, F., Suetsugu, M., Selvaraj, M. G., Hayashi, N., Yamazaki, M., et al. (2015). Development of disease-resistant rice by optimized expression of WRKY45. Plant Biotechnol. J. 13, 753–765. doi: 10.1111/pbi.12303
Goubet, F., Barton, C. J., Mortimer, J. C., Yu, X., Zhang, Z., Miles, G. P., et al. (2009). Cell wall glucomannan in Arabidopsis is synthesised by CSLA glycosyltransferases, and influences the progression of embryogenesis. Plant J. 60, 527–538. doi: 10.1111/j.1365-313X.2009.03977.x
Han, Y., Song, L., Peng, C., Liu, X., Liu, L., Zhang, Y., et al. (2019). A Magnaporthe chitinase interacts with a rice jacalin-related lectin to promote host oolonization. Plant Physiol. 179, 1416–1430. doi: 10.1104/pp.18.01594
Hernández-Blanco, C., Feng, D. X., Hu, J., Sánchez-Vallet, A., Deslandes, L., Llorente, F., et al. (2007). Impairment of cellulose synthases required for Arabidopsis secondary cell wall formation enhances disease resistance. Plant Cell 19, 890–903. doi: 10.1105/tpc.106.048058
Hong, Z., Ueguchi-Tanaka, M., Shimizu-Sato, S., Inukai, Y., Fujioka, S., Shimada, Y., et al. (2002). Loss-of-function of a rice brassinosteroid biosynthetic enzyme, c-6 oxidase, prevents the organized arrangement and polar elongation of cells in the leaves and stem. Plant J. 32, 495–508. doi: 10.1046/j.1365-313x.2002.01438.x
Hu, Y., Liu, D., Zhong, X., Zhang, C., Zhang, Q., Zhou, D. X. (2012). CHD3 protein recognizes and regulates methylated histone H3 lysines 4 and 27 over a subset of targets in the rice genome. Proc. Natl. Acad. Sci. U. S. A. 109, 5773–5778. doi: 10.1073/pnas.1203148109
Hu, J., Zhu, L., Zeng, D., Gao, Z., Guo, L., Fang, Y., et al. (2010). Identification and characterization of NARROW AND ROLLED LEAF 1, a novel gene regulating leaf morphology and plant architecture in rice. Plant Mol. Biol. 73, 283–292. doi: 10.1007/s11103-010-9614-7
Hwang, I. S., An, S. H., Hwang, B. K. (2011). Pepper asparagine synthetase 1 (CaAS1) is required for plant nitrogen assimilation and defense responses to microbial pathogens. Plant J. 67, 749–762. doi: 10.1111/j.1365-313X.2011.04622.x
Jiang, C. J., Shimono, M., Sugano, S., Kojima, M., Liu, X., Inoue, H., et al. (2013). Cytokinins act synergistically with salicylic acid to activate defense gene expression in rice. Mol. Plant Microbe Interact. 26, 287–296. doi: 10.1094/mpmi-06-12-0152-r
Jiang, P., Wang, S., Ikram, A. U., Xu, Z., Jiang, H., Cheng, B., et al. (2018). SDG721 and SDG705 are required for rice growth. J. Integr. Plant Biol. 60, 530–535. doi: 10.1111/jipb.12644
Jobling, S. A. (2015). Membrane pore architecture of the CslF6 protein controls (1-3,1-4)-β-glucan structure. Sci. Adv. 1, e1500069. doi: 10.1126/sciadv.1500069
Ke, Y., Yuan, M., Liu, H., Hui, S., Qin, X., Chen, J., et al. (2020). The versatile functions of OsALDH2B1 provide a genic basis for growth-defense trade-offs in rice. Proc. Natl. Acad. Sci. U. S. A. 117, 3867–3873. doi: 10.1073/pnas.1918994117
Kim, S. J., Chandrasekar, B., Rea, A. C., Danhof, L., Zemelis-Durfee, S., Thrower, N., et al. (2020). The synthesis of xyloglucan, an abundant plant cell wall polysaccharide, requires CSLC function. Proc. Natl. Acad. Sci. U. S. A. 117, 20316–20324. doi: 10.1073/pnas.2007245117
Kim, C. M., Park, S. H., Je, B. I., Park, S. H., Park, S. J., Piao, H. L., et al. (2007). OsCSLD1, a cellulose synthase-like D1 gene, is required for root hair morphogenesis in rice. Plant Physiol. 143, 1220–1230. doi: 10.1104/pp.106.091546
Lampugnani, E. R., Flores-Sandoval, E., Tan, Q. W., Mutwil, M., Bowman, J. L., Persson, S. (2019). Cellulose synthesis - central components and their evolutionary relationships. Trends Plant Sci. 24, 402–412. doi: 10.1016/j.tplants.2019.02.011
Less, H., Angelovici, R., Tzin, V., Galili, G. (2011). Coordinated gene networks regulating Arabidopsis plant metabolism in response to various stresses and nutritional cues. Plant Cell 23, 1264–1271. doi: 10.1105/tpc.110.082867
Liepman, A. H., Wilkerson, C. G., Keegstra, K. (2005). Expression of cellulose synthase-like (Csl) genes in insect cells reveals that CslA family members encode mannan synthases. Proc. Natl. Acad. Sci. U. S. A. 102, 2221–2226. doi: 10.1073/pnas.0409179102
Liu, Y. G., Chen, Y. (2007). High-efficiency thermal asymmetric interlaced PCR for amplification of unknown flanking sequences. Biotechniques 43, 649–650. doi: 10.2144/000112601
Liu, H., Dong, S., Gu, F., Liu, W., Yang, G., Huang, M., et al. (2017). NBS-LRR protein pik-H4 interacts with OsBIHD1 to balance rice blast resistance and growth by coordinating ethylene-brassinosteroid pathway. Front. Plant Sci. 8. doi: 10.3389/fpls.2017.00127
Liu, J., Park, C. H., He, F., Nagano, M., Wang, M., Bellizzi, M., et al. (2015a). The RhoGAP SPIN6 associates with SPL11 and OsRac1 and negatively regulates programmed cell death and innate immunity in rice. PloS Pathog. 11, e1004629. doi: 10.1371/journal.ppat.1004629
Liu, M., Shi, Z., Zhang, X., Wang, M., Zhang, L., Zheng, K., et al. (2019). Inducible overexpression of Ideal plant Architecture1 improves both yield and disease resistance in rice. Nat. Plants 5, 389–400. doi: 10.1038/s41477-019-0383-2
Liu, W., Xie, X., Ma, X., Li, J., Chen, J., Liu, Y. G. (2015b). DSDecode: a web-based tool for decoding of sequencing chromatograms for genotyping of targeted mutations. Mol. Plant 8, 1431–1433. doi: 10.1016/j.molp.2015.05.009
Li, M., Xiong, G., Li, R., Cui, J., Tang, D., Zhang, B., et al. (2009). Rice cellulose synthase-like D4 is essential for normal cell-wall biosynthesis and plant growth. Plant J. 60, 1055–1069. doi: 10.1111/j.1365-313X.2009.04022.x
Li, X., Yang, D. L., Sun, L., Li, Q., Mao, B., He, Z. (2016). The systemic acquired resistance regulator OsNPR1 attenuates growth by repressing auxin signaling through promoting IAA-amido synthase expression. Plant Physiol. 172, 546–558. doi: 10.1104/pp.16.00129
Li, W., Zhu, Z., Chern, M., Yin, J., Yang, C., Ran, L., et al. (2017). A natural allele of a transcription factor in rice confers broad-spectrum blast resistance. Cell 170, 114–126. doi: 10.1016/j.cell.2017.06.008
Luan, W., Liu, Y., Zhang, F., Song, Y., Wang, Z., Peng, Y., et al. (2011). OsCD1 encodes a putative member of the cellulose synthase-like d sub-family and is essential for rice plant architecture and growth. Plant Biotechnol. J. 9, 513–524. doi: 10.1111/j.1467-7652.2010.00570.x
Richmond, T. A., Somerville, C. R. (2000). The cellulose synthase superfamily. Plant Physiol. 124, 495–498. doi: 10.1104/pp.124.2.495
Seifi, H., De Vleesschauwer, D., Aziz, A., Höfte, M. (2014). Modulating plant primary amino acid metabolism as a necrotrophic virulence strategy: the immune-regulatory role of asparagine synthetase in botrytis cinerea-tomato interaction. Plant Signal. Behav. 9, e27995. doi: 10.4161/psb.27995
Somerville, C., Bauer, S., Brininstool, G., Facette, M., Hamann, T., Milne, J., et al. (2004). Toward a systems approach to understanding plant cell walls. Science 306, 2206–2211. doi: 10.1126/science.1102765
Song, W. Y., Wang, G. L., Chen, L. L., Kim, H. S., Pi, L. Y., Holsten, T., et al. (1995). A receptor kinase-like protein encoded by the rice disease resistance gene, Xa21. Science 270, 1804–1806. doi: 10.1126/science.270.5243.1804
Tanaka, K., Murata, K., Yamazaki, M., Onosato, K., Miyao, A., Hirochika, H. (2003). Three distinct rice cellulose synthase catalytic subunit genes required for cellulose synthesis in the secondary wall. Plant Physiol. 133, 73–83. doi: 10.1104/pp.103.022442
Underwood, W. (2012). The plant cell wall: a dynamic barrier against pathogen invasion. Front. Plant Sci. 3. doi: 10.3389/fpls.2012.00085
Vaahtera, L., Schulz, J., Hamann, T. (2019). Cell wall integrity maintenance during plant development and interaction with the environment. Nat. Plants 5, 924–932. doi: 10.1038/s41477-019-0502-0
Vega-Sánchez, M. E., Verhertbruggen, Y., Christensen, U., Chen, X., Sharma, V., Varanasi, P., et al. (2012). Loss of cellulose synthase-like F6 function affects mixed-linkage glucan deposition, cell wall mechanical properties, and defense responses in vegetative tissues of rice. Plant Physiol. 159, 56–69. doi: 10.1104/pp.112.195495
Wang, L., Guo, K., Li, Y., Tu, Y., Hu, H., Wang, B., et al. (2010). Expression profiling and integrative analysis of the CESA/CSL superfamily in rice. BMC Plant Biol. 10, 282. doi: 10.1186/1471-2229-10-282
Wang, Y., Li, J. (2008). Molecular basis of plant architecture. Annu. Rev. Plant Biol. 59, 253–279. doi: 10.1146/annurev.arplant.59.032607.092902
Wang, W., Li, Y., Dang, P., Zhao, S., Lai, D. (2018). Rice secondary metabolites: structures, roles, biosynthesis, and metabolic regulation. Molecules 233098. doi: 10.3390/molecules23123098
Wang, Y., Liu, M., Wang, X., Zhong, L., Shi, G., Xu, Y., et al. (2021). A novel β-1,3-glucanase Gns6 from rice possesses antifungal activity against Magnaporthe oryzae. J. Plant Physiol. 265, 153493. doi: 10.1016/j.jplph.2021.153493
Ward, J. L., Forcat, S., Beckmann, M., Bennett, M., Miller, S. J., Baker, J. M., et al. (2010). The metabolic transition during disease following infection of Arabidopsis thaliana by Pseudomonas syringae pv. tomato. Plant J. 63, 443–457. doi: 10.1111/j.1365-313X.2010.04254.x
Wu, C., Fu, Y., Hu, G., Si, H., Cheng, S., Liu, W. (2010). Isolation and characterization of a rice mutant with narrow and rolled leaves. Planta 232, 313–324. doi: 10.1007/s00425-010-1180-3
Wu, Y., Lee, S. K., Yoo, Y., Wei, J., Kwon, S. Y., Lee, S. W., et al. (2018). Rice transcription factor OsDOF11 modulates sugar transport by promoting expression of Sucrose transporter and SWEET genes. Mol. Plant 11, 833–845. doi: 10.1016/j.molp.2018.04.002
Xu, P., Ali, A., Han, B., Wu, X. (2018). Current advances in molecular basis and mechanisms regulating leaf morphology in rice. Front. Plant Sci. 91528. doi: 10.3389/fpls.2018.01528
Xu, Z., Xu, X., Gong, Q., Li, Z., Li, Y., Wang, S., et al. (2019). Engineering broad-spectrum bacterial blight resistance by simultaneously disrupting variable TALE-binding elements of multiple susceptibility genes in rice. Mol. Plant 12, 1434–1446. doi: 10.1016/j.molp.2019.08.006
Yamaguchi, T., Nagasawa, N., Kawasaki, S., Matsuoka, M., Nagato, Y., Hirano, H. Y. (2004). The YABBY gene DROOPING LEAF regulates carpel specification and midrib development in Oryza sativa. Plant Cell 16, 500–509. doi: 10.1105/tpc.018044
Yamamuro, C., Ihara, Y., Wu, X., Noguchi, T., Fujioka, S., Takatsuto, S., et al. (2000). Loss of function of a rice brassinosteroid insensitive1 homolog prevents internode elongation and bending of the lamina joint. Plant Cell 12, 1591–1606. doi: 10.1105/tpc.12.9.1591
Yang, C., Liu, R., Pang, J., Ren, B., Zhou, H., Wang, G., et al. (2021). Poaceae-specific cell wall-derived oligosaccharides activate plant immunity via OsCERK1 during Magnaporthe oryzae infection in rice. Nat. Commun. 122178. doi: 10.1038/s41467-021-22456-x
Yin, Y., Huang, J., Xu, Y. (2009). The cellulose synthase superfamily in fully sequenced plants and algae. BMC Plant Biol. 9, 99. doi: 10.1186/1471-2229-9-99
Yin, L., Verhertbruggen, Y., Oikawa, A., Manisseri, C., Knierim, B., Prak, L., et al. (2011). The cooperative activities of CSLD2, CSLD3, and CSLD5 are required for normal Arabidopsis development. Mol. Plant 4, 1024–1037. doi: 10.1093/mp/ssr026
Yoshikawa, T., Eiguchi, M., Hibara, K. I., Ito, J. I., Nagato, Y. (2013). Rice slender leaf 1 gene encodes cellulose synthase-like D4 and is specifically expressed in m-phase cells to regulate cell proliferation. J. Exp. Bot. 64, 2049–2061. doi: 10.1093/jxb/ert060
Zhao, Q., Dixon, R. A. (2014). Altering the cell wall and its impact on plant disease: from forage to bioenergy. Annu. Rev. Phytopathol. 52, 69–91. doi: 10.1146/annurev-phyto-082712-102237
Zhao, H., Li, Z., Wang, Y., Wang, J., Xiao, M., Liu, H., et al. (2022). Cellulose synthase-like protein OsCSLD4 plays an important role in the response of rice to salt stress by mediating abscisic acid biosynthesis to regulate osmotic stress tolerance. Plant Biotechnol. J. 20, 468–484. doi: 10.1111/pbi.13729
Keywords: rice, cellulose synthase-like D, trade-off, disease resistance, widely targeted metabolomics
Citation: Liu X, Yin Z, Wang Y, Cao S, Yao W, Liu J, Lu X, Wang F, Zhang G, Xiao Y, Tang W and Deng H (2022) Rice cellulose synthase-like protein OsCSLD4 coordinates the trade-off between plant growth and defense. Front. Plant Sci. 13:980424. doi: 10.3389/fpls.2022.980424
Received: 18 July 2022; Accepted: 12 September 2022;
Published: 26 September 2022.
Edited by:
Yihua Zhou, State Key Laboratory of Plant Genomics (CAS), ChinaReviewed by:
Juan Du, Sichuan University, ChinaYiqin Wang, Institute of Genetics and Developmental Biology (CAS), China
Copyright © 2022 Liu, Yin, Wang, Cao, Yao, Liu, Lu, Wang, Zhang, Xiao, Tang and Deng. This is an open-access article distributed under the terms of the Creative Commons Attribution License (CC BY). The use, distribution or reproduction in other forums is permitted, provided the original author(s) and the copyright owner(s) are credited and that the original publication in this journal is cited, in accordance with accepted academic practice. No use, distribution or reproduction is permitted which does not comply with these terms.
*Correspondence: Wenbang Tang, dGFuZ3dlbmJhbmdAMTYzLmNvbQ==; Huabing Deng, ZGVuZ2h1YWJpbmdAMTI2LmNvbQ==
†These authors have contributed equally to this work