- 1Key Laboratory of Vegetation Restoration and Management of Degraded Ecosystems, South China Botanical Garden, Chinese Academy of Sciences, Guangzhou, China
- 2Southern Marine Science and Engineering Guangdong Laboratory, Guangzhou, China
- 3Graduate School of Agriculture, Hokkaido University, Sapporo, Japan
- 4Sarawak Tropical Peat Research Institute, Kota Samarahan, Malaysia
- 5Department of Environmental and Biological Sciences, University of Eastern Finland, Joensuu, Finland
Melting permafrost mounds in subarctic palsa mires are thawing under climate warming and have become a substantial source of N2O emissions. However, mechanistic insights into the permafrost thaw-induced N2O emissions in these unique habitats remain elusive. We demonstrated that N2O emission potential in palsa bogs was driven by the bacterial residents of two dominant Sphagnum mosses especially of Sphagnum capillifolium (SC) in the subarctic palsa bog, which responded to endogenous and exogenous Sphagnum factors such as secondary metabolites, nitrogen and carbon sources, temperature, and pH. SC's high N2O emission activity was linked with two classes of distinctive hyperactive N2O emitters, including Pseudomonas sp. and Enterobacteriaceae bacteria, whose hyperactive N2O emitting capability was characterized to be dominantly pH-responsive. As the nosZ gene-harboring emitter, Pseudomonas sp. SC-H2 reached a high level of N2O emissions that increased significantly with increasing pH. For emitters lacking the nosZ gene, an Enterobacteriaceae bacterium SC-L1 was more adaptive to natural acidic conditions, and N2O emissions also increased with pH. Our study revealed previously unknown hyperactive N2O emitters in Sphagnum capillifolium found in melting palsa mound environments, and provided novel insights into SC-associated N2O emissions.
Introduction
Arctic permafrost soils store ample nitrogen (N) reservoirs that may be subject to remobilization due to climate warming (Christensen et al., 2013), that leads to permafrost degradation and thawing (Borge et al., 2017). After permafrost thaws, increased nitrous oxide (N2O) emissions are observed in arctic permafrost peatlands (Voigt et al., 2017a,b). N2O is a potent greenhouse gas and contributes to the disruption of the ozone layer (IPCC, 2007; Ravishankara et al., 2009). Therefore, urgency to understand the primary source of N2O emissions in this arctic environment is crucial.
Peatlands store one-third of global soil carbon, and boreal peatlands account for 83% of the global peatland area (Eurola et al., 1984; Savolainen et al., 1994). Bare peat in permafrost peatlands has been identified as a hot spot for N2O emissions due to low availability nitrogen (N) competition in subarctic tundra (Repo et al., 2009; Marushchak et al., 2011). Sphagnum-dominated bogs have low nutrient content, low primary production, low-quality plant litter, low litter decomposition rates, and low mineral content combined with a low pH (<4.5) environment, which is vital for carbon (C) sequestration (Chronáková et al., 2019). Mineral N deposition to Sphagnum bogs has progressed, with ammonification, ammonia oxidation, and denitrification playing a critical role in the emission of N2O (Van Cleemput, 1998; Francis et al., 2007). In addition, the water table level also affects N2O emissions in northern peatland, as lowering the water table leads to increased N2O production (Regina et al., 1996). Once the peatlands are drained, Sphagnum vegetation and surface peat layers are exposed to the atmosphere, activating nitrification due to ammonium (-N) release in aerobic peat degradation, followed by denitrifier stimulation in N-enriched conditions to emit N2O (Martikainen et al., 1995; Regina et al., 1999; Minkkinen et al., 2020). Palmer and Horn (2012) reported that palsa peatlands in the northwestern Finnish Lapland showed N2O emissions in situ from −0.02 to 0.01 μmol N2O m−2 h−1. Emissions of N2O may rise considerably during the thaw of permafrost, representing another ongoing change in northern peatlands. It was reported that a five-fold increase in N2O flux from palsa mire peat in a permafrost thaw experiment (Voigt et al., 2017b). However, determining which active N2O emitters in these northern ecosystems contribute to high emissions remains largely elusive.
Sphagnum mosses (non-vascular plants) dominate the vegetation of many northern mire ecosystems and harbor a high diversity of nitrifiers and denitrifiers (Dedysh et al., 2006; Gilbert et al., 2006; Opelt et al., 2007). In these moss communities, N2O gas is mainly produced via nitrification, nitrifier denitrification, and denitrification pathways (Wrage et al., 2001). High hummocks in bogs and palsa mire permafrost mounds have relatively thick aerobic acrotelm layers and are the most potential microhabitats to N2O emissions. These microhabitats are characteristically dominated by Sphagnum fuscum (SF) and Sphagnum capillifolium (SC) (Markham, 2009; Novak et al., 2015; Zhong et al., 2020), which are widely distributed throughout European and North American peat bogs. These keystone species develop climax-type, raised bog hummock vegetation. Upon exposure to high N inputs, polyphenol secondary metabolites produced by these Sphagnum mosses, such as caffeic acid, are often reduced (Bragazza and Freeman, 2007; Montenegro et al., 2009). These secondary metabolites may impact the activity and community composition of the microbiota within the holobiont and the associated N2O emission rates (Wang and Cernava, 2020).
Our previous work has demonstrated that the N2O source in southeastern Finland was mainly from Sphagnum moss rather than peat soil. However, this previous study only focused on the single keystone and dominant species of SF in Finnish temperate marine climate areas (Nie et al., 2015). The different contributions of N2O emissions between several dominant Sphagnum species, especially in a typical subarctic permafrost peatland [hot-spots of N2O emission (Voigt et al., 2017b)] in Finland, is largely unknown. This study uses SF as the control plants and aim to answer three questions: (1) Are the N2O emission potentials between the two dominant Sphagnum species (SC and SF) similar or different in the subarctic palsa bog? (2) How does the culture-based N2O assay for the bacterial community composition of the two Sphagnum species influence the N2O emission potential? (3) What is the dominant process of N2O production by active N2O emitters under aerobic conditions of peat bogs? By investigating N2O emission potential in SF and SC grown in drained palsa peat bogs of northwestern Finland, we aim to characterize the dominant N2O emitters hidden in the microbiota of SF and SC in association with their N2O emission traits in response to major holobiont factors.
Materials and methods
Sampling Sphagnum mosses
Composite samples of SF and SC (photos of them at one site are shown in Supplementary Figure S1) were collected from a plateau of a permafrost mound of a palsa mire near Kilpisjärvi (68°43′; 21°25′), northwestern Finland (Figure 1A). Each sample of SC/SF was formed from three random sampling sites with three replicates in August–September, 2014. SC and SF were collected from the same patch (within 50–100 cm) and the sampling sites were 50 to 100 m away from each other. From each sampling site, random 533 to 565 individual plants of either SC or SF were collected and mixed for each sample in order to guarantee the sample's representation. Both SC and SF were collected from large homogenous stands with a 40 cm thaw layer above the permafrost surface. The region has a low annual mean temperature (−2.3°C) and moderate mean annual precipitation (487 mm). The growing season is one of the shortest in continental Europe (~100 d when the mean daily temperature is ≥5°C). The Sphagnum samples stored in Ziploc® bags at 4°C were used for further culture-based N2O emission measurements.
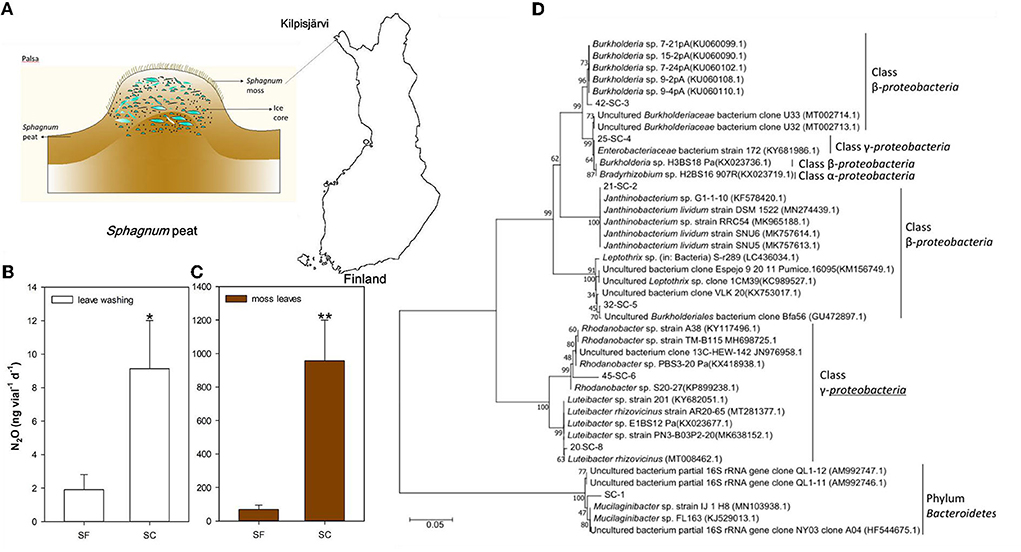
Figure 1. N2O emission potential and microbial communities in the Sphagnum mosses grown in Finland's plateau of a permafrost mound. Sample site of Sphagnum mosses (A). 100 μL Sphagnum leaves washing (100 mg/10 ml) as inoculants (B). Sphagnum mosses as the inoculants (C). The community structure of bacteria revealed by the PCR-DGGE profile was subjected to phylogenetic analysis of SC (D). Incubation conditions of (B,C): pH = 5, incubated at 15°C, 7 days, n = 3, with 0.05% sucrose. *P< 0.05 and **P< 0.01.
Comparison of N2O emission potentials in two Sphagnum mosses
To evaluate the potential for N2O emission of the two Sphagnum mosses under an experimental nitrogen load, we took three Sphagnum mosses plants (~0.1 g in dry weight) randomly from the respectively, composite sample of SC and SF using sterilized tweezers. At the same time, we standardized the dry weight for the N2O assay. Either 100 μL of Sphagnum moss leaf extract (100 mg/10 ml) or 3 plants were added to N2O assay medium [10 ml of modified Winogradsky's Gellan (MWG) medium containing 0.005% yeast extract and solidified with 3% gellan gum with 22.6 ml of headspace in each vial (30 ml gas-chromatographic vial with a butyl rubber plug) (Nichiden-Rika Glass Co., Kobe, Japan)] with 0.05% sucrose diluted with sterilized Milli-Q water (the solution was adjusted to pH = 5.0 with 2 M H2SO4) (three replicates in each case) (Hashidoko et al., 2008). After incubation at 15°C (according to the mean value of summer temperature of Finland) for 7 days in the dark, an N2O assay was carried out by using an electron capture detector(ECD)-gas chromatograph (Shimadzu GC-14B, 125 Kyoto, Japan) connected to a Porapak N column (1 m long, Waters, Milford, MS, USA). In another treatment, 0.1 g L−1 of caffeic acid instead of 0.05% sucrose was added as the carbon source to the vials with three plants (~0.1 g in dry weight) randomly taken from the above composite samples (pH 5). A control for the assay, without any carbon source, was also performed simultaneously (three replicates in each case). After incubation at 15°C in the dark for 4, 8, and 15 days, an assay of N2O was performed as mentioned above.
DGGE profiling of the bacterial communities in two Sphagnum species
Polymerase chain reaction-denatured gradient gel electrophoresis (PCR-DGGE) was performed to observe the culture-based bacterial communities on the leaves of the two Sphagnum mosses. First, genomic DNA was extracted from the medium after the N2O assay using an Isoplant II DNA Extraction kit (Nippon Gene, Toyama, Japan). The PCR steps and conditions were as follows: PCR denaturation for 5 min at 95°C, and 30 cycles of amplification (15 s at 95°C, 30 s at 55°C, 30 s at 72°C), and 10 min elongation at 72°C. Then PCR products for DGGE were obtained by using the common 16S rRNA primers GC-341F (CGC CCG CCG CGC CCC GCG GGG GTC CCG CCG CCC CCG CCC GCC T AC GGG AGG CAG CAG) and 907R (CCG TCA ATT CCT TTR AGT TT) (Ferris et al., 1996) and run on a 30–70% denatured gradient gel (6% w/v). The sequences of DGGE-cutting bands were obtained using an ABI prismTM 310 Genetic Analyzer and retained in the NCBI (BioProject No. PRJNA681491).
Culture-dependent screening and identification of N2O emitters
100 μl of medium with three Sphagnum mosses (after incubation for 7 days) was diluted 1× 104- and 106-Fold and inoculated onto MWG plates to screen N2O emitters. After incubation for 5 days at 20°C in the dark, 13 distinguishable bacterial colonies characterized by colony characteristics were selected for streak cultivation on MWG plates and transferred to potato dextrose agar (PDA) plates until purified. Each Pure strain [a total of 108 isolates (13 bacterial colonies with 8 replicates), with 100 μl of each bacterial cell suspension (OD660nm = 0.9–1.0)] was inoculated into an N2O assay vial with 10 ml of modified MWG medium to test their N2O emission ability. The three pure strains SC-K1, SC-L1, and SC-H2 (from SC) showed relatively higher N2O production and were active N2O emitters (Supplementary Table S2, data collected from six top active N2O emission-bacterial colonies). The genomic DNA of each strain was extracted, and the 16S RRNA gene was amplified through PCR by using a series of primers 27F, 338R, 341F, 907R, 1080R, 1380R, 1492R, 1112F, and 1525R. Sequencing was performed with an ABI PrismTM 310 Genetic Analyzer (Applied Biosystems, USA) (Nie et al., 2015). All the resulting 16S RRNA gene sequencing datasets were deposited in the NCBI database (accession nos. MW301596–MW301598) and compared with sequences in the nucleotide basic local alignment search tool (BLASTN) database program provided by NCBI (National Center of Biotechnology Information, Bethesda, MD, USA; http://Blast.Ncbi.nlm.nih.gov/Blast.cgi).
N2O emitters response to nitrogen sources, pH, and temperature
The pure isolates (SC-K1, SC-L1, and SC-H2) pre-cultured on PDA for 4 days at 15°C were separately scraped with a nichrome wire loop and suspended into 1.5 ml Milli-Q water (equal amounts of each pure strain was guaranteed). A 20 μl portion of the inoculant that showed an optical density of OD660nm 0.9–1.0 was added to the N2O assay vial and then was thoroughly vortexed for 30s. 1 mM NH4NO3, KNO3, and NH4Cl were tested and incubated at 15°C for 5 days with 0.05% sucrose (pH = 5.0) to determine the optimal nitrogen substrates for pure N2O emitters. The pH was adjusted with 1 M H2SO4 and 1 M KOH solutions to 4.6, 5.0, 5.7, 6.8, and 7.3 before autoclaving and incubated at 15°C for 5 days with 0.05% sucrose to determine the optimal pH for N2O emitters. Different temperatures (4, 10, 15, 20, 25, and 30°C) were set in separate incubators and incubated for 5 days with 0.05% sucrose to find the appropriate temperature. All experiments were performed with three replicates.
Carbon source- and polyphenol-supplementation assays
Sucrose and E-caffeic acid were applied as carbon sources and secondary metabolites (polyphenols), respectively, for the microbiota inhabiting Sphagnum moss (Nie et al., 2015). The inoculants were prepared as described in Nie et al. (2015). To observe the responses of the N2O emitters (SC-K1, SC-L1, SC-H2) to sucrose, 0 (control), 0.05, and 0.5% sucrose were used for the separated/cultivated bacterial strains. To determine the optimal concentrations of E-caffeic acid for N2O emitters (SC-K1, SC-L1, SC-H2), 0 (control), 0.005, 0.01, 0.05, 0.1, 0.5, and 1 g L−1 E-caffeic acid were used. Each treatment contained three analytical replicates incubated at 15°C for 5 days with inoculants for N2O assays. Their N2O emissions were separately measured.
Analysis of denitrification rates of N2O emitters
We applied the acetylene inhibition assay, which is widely used to measure denitrification rates (Sørensen, 1978). The activity of N2O reductase was inhibited by adding acetylene (C2H2) at pH 5.0 and 7.0, and 10% C2H2 gas was injected into the headspace of vials inoculated with N2O emitters (the same with above inoculation method) (Bollmann and Conrad, 1997). At the same time, treatments without injected C2H2 gas were carried out as controls to compare the N2O reductase activity (three replicates in each case). Incubation conditions were the same as described above.
Detection of nitrogen cycling functional genes in N2O emitters
Functional genes of nitrogen cycling, including narG, nirK, nirS, and nosZ (Supplementary Figure S4), were detected by using the PCR method. The target genes were amplified by using the primers narGF (TCG GGC AAG GGC CAT GAG TAC) and narGR (TTT CGT ACC AGG TGG CGG TCG), nirSCd3Af (AAC GYS AAG GAR ACS GG) (Nie et al., 2015) and nirSR3cd (GAS TTC GGR TGS GTC T) (Throbäck et al., 2004), nirK-1F (GGM ATG GTK CCS TGG CA) and nirK-5R (GCC TCG ATC AGR TTR TGG) (Braker et al., 1998), nosZ-1111F (STA CAA CWC GGA RAA SG), nosZ-661F (CGG CTG GGG GCT GAC CAA), nosZ-1527R (CTG RCT GTC GAD GAA CAG), and nosZ-1773R (ATR TCG ATC ARC TGB TCG TT) (Scala and Kerkhof, 1998). The exact reaction conditions of the PCR amplifications are presented in Supplementary Table S1.
Statistical analysis
The data were expressed as mean with standard error (SE). The data were examined for normality and homoscedasticity using the Shapiro-Wilk's and Levene's tests, respectively (SPSS, version 23.0). All data was found to fit the normal distribution and homogeneity of variances. Comparisons were made using a one-way analysis of variance (ANOVA) among two or more groups. One-way ANOVA was used to compare differences in N2O emission with different inoculants (Sphagnum mosses or their leaves washing), physicochemical factors [pH, temperature, sucrose, nitrogen types, and secondary metabolite (E-caffeic acid)], and C2H2 inhibition assay. Using the Fisher's Least Significant Difference(LSD) method, multiple comparisons were carried out using IBM SPSS 23.0 software (Chicago, Illinois, USA).
Results
N2O emission potential and microbial communities
After incubation for 7 days, we found that the average N2O emissions of SF were 1.9 ng vial−1 d−1 in the leaf extract and 69.9 ng vial−1 d−1 in the leaf samples. The SC sample showed N2O emissions of 9.1 in the leaf extract and 956.2 ng vial−1 d−1 in the leaf samples (Figures 1B,C).
The PCR-DGGE profile showed that the major culture-based bacterial communities in these Sphagnum mosses were similar. However, the SC sample harbored the family Enterobacteriaceae (Figure 1D, Supplementary Figure S2), while the SF sample contained the genus Dyella of Gammaproteobacteria (Supplementary Figure S2). N2O production increased with 0.1 g L−1 caffeic acid addition in both samples, and the effect was significantly larger in the SC sample than in the SF sample (p < 0.05) (Figures 2A,B).
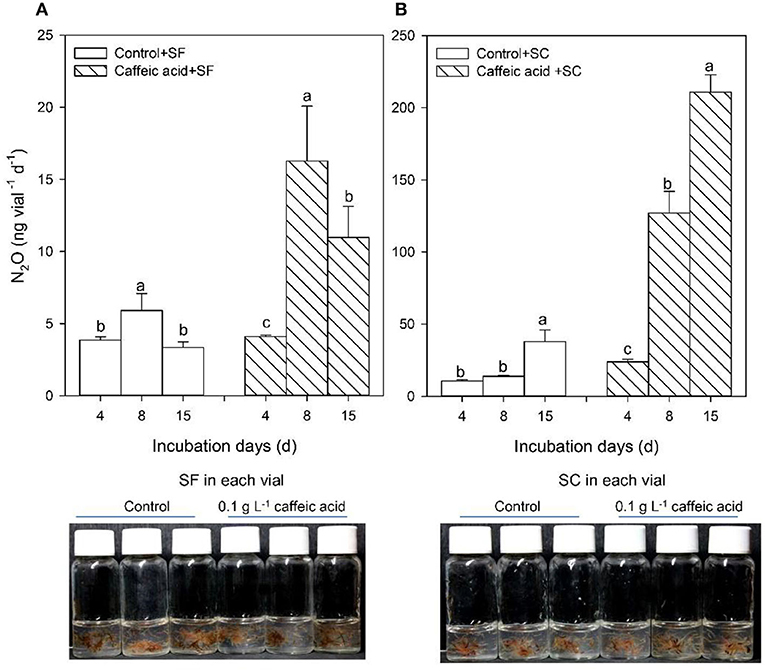
Figure 2. Response of N2O production of Sphagnum fuscum (SF) and Sphagnum capillifolium (SC) to caffeic acid. Response of N2O production of Sphagnum fuscum (SF) to caffeic acid (A). Response of N2O production of Sphagnum capillifolium (SC) to caffeic acid (B). Incubation conditions: pH = 5, incubated at 15°C, 4, 8, 15 days, n = 3, without sucrose. Values are means ± s.e. (shown as error bars).
Major N2O emitters in Sphagnum mosses
Compared to PCR-DGGE, the culture-based approach revealed distinctive profiles of N2O emitters (Supplementary Figure S2). Two Burkholderia spp. were isolated from the SF sample, while three Gammaproteobacteria (one Pseudomonas sp., one Serratia sp., and an unidentified Enterobacteriaceae bacterium) and one Burkholderia sp. were isolated from the SC sample. Among them, Serratia sp. SC-K1, Enterobacteriaceae bacterium SC-L1, and Pseudomonas sp. SC-H2 showed the most efficient N2O emissions, and the activity of N2O emissions was the greatest in Pseudomonas sp. SC-H2, then Enterobacteriaceae bacterium SC-L1, and then Serratia sp. SC-K1 (pH 5) (Table 1, Supplementary Table S2).
Effects of substrate type, temperature and pH on microbial N2O emissions
According to the N2O production responses to different nitrogen sources, KNO3 was the most efficient substrate for N2O emission, followed by NH4NO3, while almost no N2O emissions were found with NH4Cl as the substrate. Active N2O emissions from KNO3 indicated that the three N2O emitters were nitrate reducers (Figure 3). N2O emissions increased as the pH increased from 4.6 to 7.3. Enterobacteriaceae bacterium SC-L1 and Serratia sp. SC-K1 showed a temporary increase at a pH value of 5 but no drastic increase in N2O emissions, indicating adaptation to acidic environments (Figures 4A,B). At pH values over 6, Pseudomonas sp. SC-H2 emissions increased sharply, making it the most likely N2O emitter (Figure 4C). For the three strains used, N2O emissions also increased with increasing temperature from 4 to 30°C (Figures 4D–F).
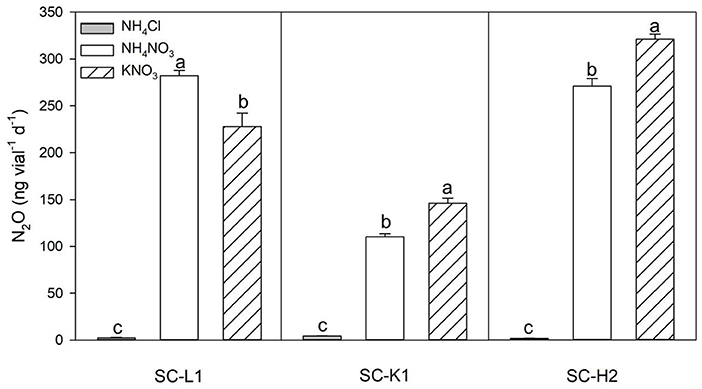
Figure 3. N2O emission by three pure N2O emitters (SC-L1, SC-K1, SC-H2) upon exposure to different nitrogen substrates (1 mM NH4Cl, NH4NO3, KNO3). Incubation conditions: pH = 5, incubated at 15°C for 5 days with 0.05% sucrose (n = 3). Values are means ± s.d. (shown as error bars).
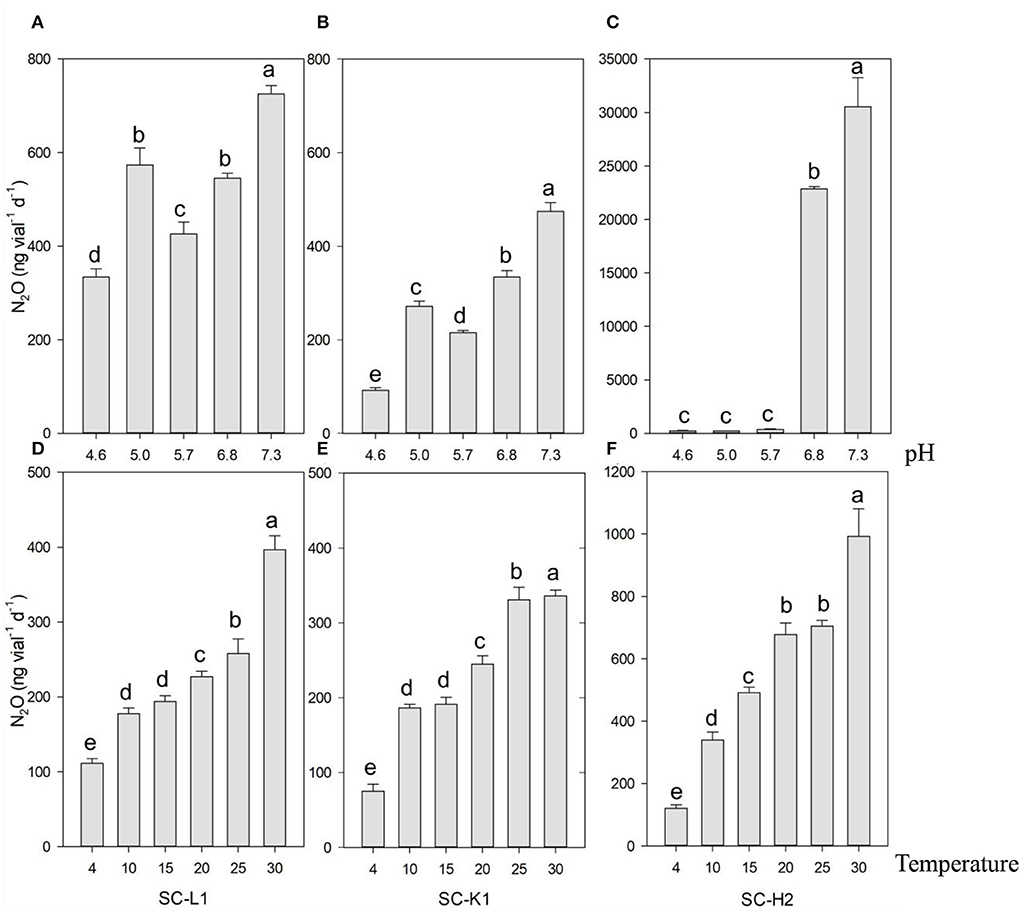
Figure 4. N2O emission by three pure N2O emitters (SC-L1, SC-K1, SC-H2) upon the gradient pH and temperature. N2O emission by SC-L1 (A,D), SC-K1 (B,E), SC-H2 (C,F) upon exposure to different pH from 4.6 to 7.3 (A–C), and different temperatures from 4 to 30 °C (D–F) was analyzed. For the impact of pH on N2O emission, the N2O emitters were incubated at 15°C for 5 days with 0.05% sucrose (n = 3). For the impact of temperature on N2O, the N2O emitters were incubated for 5 days with 0.05% sucrose (n = 3 and pH = 5).
Disparate responses of N2O emitters to caffeic acid and sucrose
The three microbial strains exhibited disparate responses to sucrose and E-caffeic acid (Figure 5). In the absence of added sucrose (control treatment), Serratia sp. SC-K1 emitted more N2O than Enterobacteriaceae bacterium SC-L1 and Pseudomonas sp. SC-H2, while these last two strains emitted N2O at higher levels with 0.05% sucrose supplementation (Figures 5A,B). Notably, the response of Pseudomonas sp. SC-H2 to 0.05% sucrose was very drastic, resulting in emission ~2x103 times higher than without sucrose (Figure 5C). This result demonstrated that Serratia sp. SC-K1 is an oligotrophic bacterium, whereas Enterobacteriaceae bacterium SC-L1 and Pseudomonas sp. SC-H2 are eutrophic bacteria.
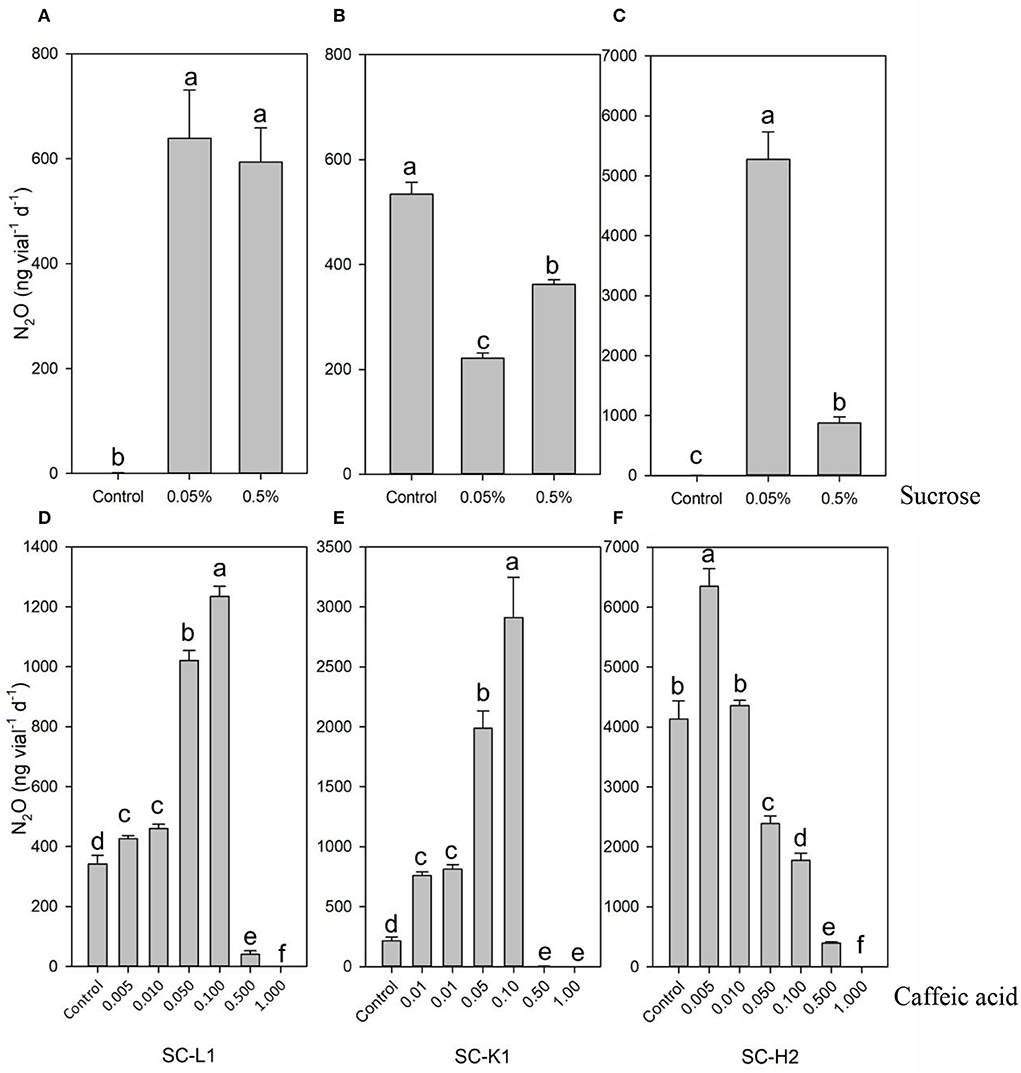
Figure 5. (A) N2O emission by three pure N2O emitters (SC-L1, SC-K1, SC-H2) exposure to the different concentrations of sucrose (A–C) and caffeic acid (D–F). N2O emission by SC-L1 (A,D), SC-K1 (B,E), SC-H2 (C,F) upon exposure to different concentration of sucrose from 0 to 0.5% (A–C) and different concentration of caffeic acid from 0 to 0.1 g L−1 (D–F) was analyzed. For the impact of sucrose on N2O emission, the N2O emitters were incubated at pH = 7 for 5 days (n = 3), and the control was without supplemented sucrose. For the impact of caffeic acid on N2O emission, the N2O emitters were incubated at pH = 7 for 5 days with 0.05% sucrose (n = 3), and the control was without supplemented caffeic acid.
For the pure strains of Enterobacteriaceae bacterium SC-L1 and SC-K1, a relatively lower concentration of E-caffeic acid ( ≤ 0.1 g L−1) increased N2O emissions of these two strains, and the optimum concentration was 0.1 g L−1 (Figures 5D,E). Among them, Serratia sp. SC-K1 was very sensitive to 0.1 g L−1, and 13-fold higher N2O production was found than without E-caffeic acid (Figure 5E). For Pseudomonas sp. SC-H2, when the concentration of E-caffeic acid was above 0.01 g L−1, N2O emissions decreased significantly (p < 0.01) (Figure 5F).
Modest responses of N2O emitters to acetylene
There was no detectable difference between the 10% C2H2 and control treatment emissions at a pH value of 5.0. However, in Pseudomonas sp. SC-H2 cultured at a pH value of 7.0, N2O emissions upon exposure to C2H2 were drastically increased to four-fold higher than that of the control. Without 10% C2H2, the production level of N2O at a pH value of 7.0 was higher than that at a pH value of 5.0 (Figure 6). This result suggested that the peat ecosystem was highly disturbed at a pH value of 7.0, denitrification was greatly accelerated, and the final denitrification step to reduce N2O to N2 was driven by N2O reductase.
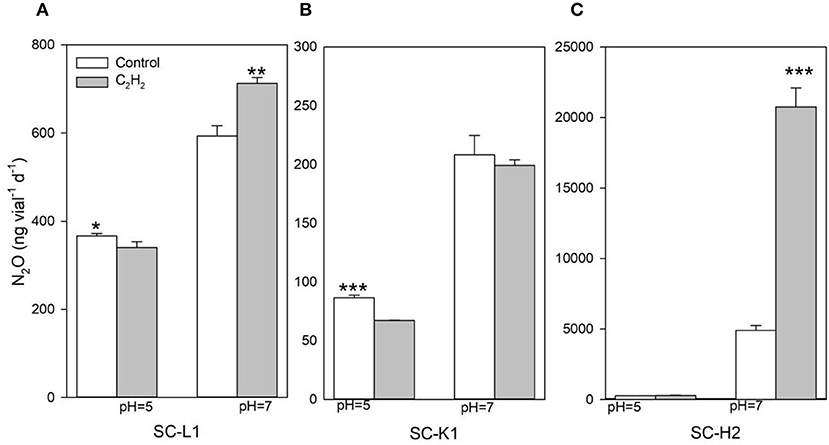
Figure 6. N2O emission by three N2O emitters (SC-L1, SC-K1, SC-H2) exposure to 10% C2H2 gas (A–C). The three N2O emitters were incubated at pH 5 and 7, under 15°C with 0.05% sucrose for 5 days (n = 3). Without C2H2 gas was used as a control. *P< 0.05, **P< 0.01, and ***P< 0.001.
Functional genes involved in N2O emission
PCR assays detected the narG gene in the three N2O emitter strains, but only Pseudomonas sp. SC-H2 contained nirS and nosZ genes (Table 2; Supplementary Figure S3). In combination with the results of the C2H2 assay, these results suggested that Pseudomonas sp. SC-H2 is a complete denitrifier. The nirK gene was not detected within Enterobacteriaceae bacterium SC-L1 and Serratia sp. SC-K1.
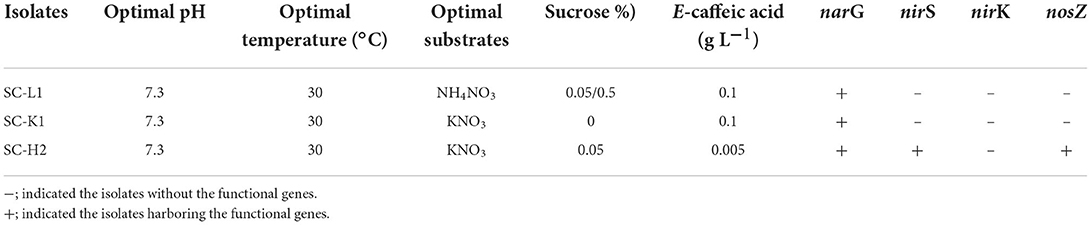
Table 2. Characteristics of the three active N2O emitters isolated from SC and PCR assay to detect denitrification-related genes.
Discussion
Cultured bacterial communities in the leaves distinguishable between two Sphagnum species
Increased atmospheric N deposition can reduce the growth of some Sphagnum species, such as Sphagnum magellanicum (Aerts et al., 2001; Limpens and Berendse, 2003). In contrast, the production of SF increased with elevated N deposition but decreased as N deposition reached 14.0 kg ha−1 yr−1 as reported by Vitt et al. (2003). SC can also tolerate a high N supply (Bonnett et al., 2010). Our study offered evidence that individual samples of the latter two Sphagnum species had N2O emission potential reasonably associated with their bacterial communities. In particular, the SC sample harbored specific bacterial communities associated with high N2O emission. Surprisingly, the N2O emission of the SC sample was significantly greater than that of the SF sample (Figure 1B) (p < 0.01). Such a large difference in N2O emission between the SF and SC species gives precedence to the hypothesis of potential N2O emission differences in different Sphagnum species.
Based on the analysis of bacterial communities using culture-based PCR-DGGE and isolation of N2O emitters, the major Sphagnum-associated bacterial communities of our samples were consistent with boreal mire and tropical peat forest and included Burkholderia, Mucilaginibacter, Rhodanobacter, and Janthinobacterium but their N2O emission activity was different in varied sites due to differences in climate and habitat environments (Hashidoko et al., 2008; Sun et al., 2014). Janthinobacterium spp. did not show high N2O emission potential in subarctic palsa bog unlike in the tropical peatland soil, which suggested that the N2O emission functions of N2O emitters were changing in different climate zones. Previous experimentation has shown that the Sphagnum microbiota supported the host plant and the entire ecosystem under environmental changes (Bragina et al., 2014). Burkholderia spp. were N2O emitters, but their N2O emission functions were significantly lower than the acid-tolerant Janthinobacterium sp. in a deforested tropical peatland soil, which was previously determined by soil pH (Hashidoko et al., 2010). The Burkholderia spp. isolates in SF were similar to another climate zone in Finland, showing the same species of Sphagnum although in a different climate zone (Nie et al., 2015). Within this study, some unique bacterial strains were found in the leaves of SC, including a Pseudomonas sp. and two Enterobacteriaceae family members. In numerous previous studies, Pseudomonas species (P. denitrificans, P. perfectomarinus, P. fluorescens, P. stutzeri, P. aeruginosa, and P. nautica) were found performing denitrification (Delwiche, 1959; Payne et al., 1971; Balderston et al., 1976; Sørensen et al., 1980; Dooley et al., 1987; Viebrock and Zumft, 1988; SooHoo and Hollocher, 1991; Prudêncio et al., 2000). The isolated Pseudomonas sp. was not found in the bands of PCR-DGGE, possibly due to relatively low abundance under acidic conditions (pH 5) (Figure 4C). Anderson and Levine (1986) offered evidence that Enterobacteriaceae and Serratia sp.'s nitrate respiration produces N2O, which was also found in our SC sample. Enterobacter sp. was also found as dissimilatory nitrate reduction to ammonium (DNRA) bacteria in agricultural soils (Heo et al., 2020). Pseudomonas sp. SC-H2, Enterobacteriaceae bacterium SC-L1, and Serratia sp. SC-K1 were responsible for N2O emissions in our Sphagnum samples (SC). These findings suggest that the variation in the N2O emission potential of Sphagnum found in peatlands is associated with species-specific bacterial communities, which are variable under different species and environments.
Complex environmental factors also impact N2O production of active N2O emitters
The top three active N2O emitters (Pseudomonas sp. SC-H2, Enterobacteriaceae bacterium SC-L1, and Serratia sp. SC-K1) from SC increased N2O production with increasing temperature up to 30°C (Figures 4D–F), illustrating a potential rise in N2O emissions following global warming (Pfenning and McMahon, 1997; Voigt et al., 2017a; Chen et al., 2020). For the three active N2O emitters, N2O production was relatively high at a pH value of 7.0 (Figures 4A–C), which is much higher than the naturally low pH of Sphagnum microhabitats (Tahvanainen and Tuomaala, 2003). Although N2O reduction to N2 by Pseudomonas sp. SC-H2 was obvious, the N2O production was still high after 5 days of incubation (Figure 6). This result indicated that N2O emission hotspots are inclined to be in neutral peatlands, as supported by Palmer and Horn (2015). Combining these results with acetylene inhibition assays at pH value of 5.0 and 7.0 showed that N2O reduction to N2 was almost negligible at a pH value of 5 for these three active N2O emitters. This result is consistent with a previous study of the lack of N2O reductase (nos) function at low pH (Liu et al., 2014). This result also suggested that N2O reduction was inhibited in the acidic environment in the peat bogs. Since the Sphagnum microhabitats are very acidic, N2O reductase activity is repressed, supporting that N2O reduction is not a pathway decreasing N2O emissions in the pristine Sphagnum bog system. Under low-pH conditions, N2O production by Pseudomonas sp. SC-H2 was small, but N2O could be accumulated. However, the palsa mounds are formed due to the ice core under the Sphagnum peat layer in the subarctic climate, and once they collapse after permafrost thawing, the peat acidity will be neutralized to some extent by mixing with mineral material and minerogenic water flow (Seppälä, 2011; Takatsu et al., 2022).
Sphagnum mosses are important for peat accumulation and form a carbon pool of global significance. Increasing atmospheric N deposition can activate phenol oxidase in peat bogs and destabilize peat carbon (Bragazza et al., 2006). Phenol oxidase requires bimolecular oxygen for its activity (Freeman et al., 2004), and drying increases aerobic conditions in peatlands (Swindles et al., 2019) and can degrade recalcitrant phenolic materials. Tahvanainen and Haraguchi (2013) showed that this phenolic mechanism is affected by pH. Such changes may reduce the generally high C:N ratio, which increases net N mineralization, nitrification, and denitrification rates, while subsequently increasing the potential of N2O production in peat bogs, while lower C:N ratios ( ≤ 25–30) stimulate N2O emissions (Huang et al., 2004; Klemedtsson et al., 2005; Maljanen et al., 2012). Connected mechanisms and the release of ice-trapped N2O are further impacted by thawing permafrost (Voigt et al., 2017b). Our findings indicate that N2O emissions are not exceptionally high under the naturally cold temperatures and low pH of Sphagnum habitats; rather, substantially high pH and temperatures, and perhaps a connected imbalance of microbial communities in such conditions, induced the highest N2O emissions. The results warrant caution in interpretation and against unexpected emission potential under rapidly changing conditions. It also calls for a need to monitor the in situ N2O emissions from different permafrost Sphagnum species in the permafrost in future studies.
Responses of N2O emitters to primary metabolites and secondary metabolites of Sphagnum mosses
Without sucrose, the N2O emitters Enterobacteriaceae bacterium SC-L1 and Pseudomonas sp. SC-H2 could not emit N2O because of their low growth. This result indicated that these two strains were heterotrophic microorganisms that needed to gain C sources from Sphagnum moss and form plant-microbial symbionts between plants and microbes. Interestingly, Serratia sp. SC-K1 grew well without sucrose and emitted much more N2O; meanwhile, it could be significantly inhibited by adding a low concentration of sucrose (0.05%). This result indicated that this strain is an autotrophic microorganism adapted to nutrient-poor environments, using carbon dioxide (CO2) as a C source. These autotrophic microorganisms contribute to CO2 uptake and carbon sequestration. Drained peatland ecosystems have an immense potential for C sinks to maintain the C balance, even though droughts are occasionally caused by decreasing photosynthesis (Minkkinen et al., 2018).
Our study showed that N2O emitters (Serratia sp. SC-K1 and Enterobacteriaceae bacterium SC-L1) could resist relatively higher concentrations of caffeic acid ( ≤ 0.1 g L−1), while the N2O emitter (Pseudomonas sp. SC-H2) had low resistance to caffeic acid ( ≤ 0.005 g L−1) (Figures 5D–F). These results could explain why we could not find the Pseudomonas spp. using DGGE band sequencing. Polyphenol (caffeic acid) from Sphagnum moss inhibits growth and results in a low relative abundance of Pseudomonas spp. The more abundant Serratia sp. SC-K1 and Enterobacteriaceae bacterium SC-L1 were the dominant N2O emitters due to their higher resistance to polyphenolic compounds. The stimulated N2O production in the Sphagnum moss-microbe vial with 0.1 g L−1 caffeic acid confirmed Serratia sp. SC-K1 and Enterobacteriaceae bacterium SC-L1 were the dominant N2O emitters. Serratia spp. are gram-negative bacilli and belong to the family Enterobacteriaceae. The interaction of polyphenolic compounds and Enterobacteriaceae bacteria might directly influence N2O emissions in peatland ecosystems. High concentrations of polyphenols are likely to lower N2O emissions. The response of phenol oxidase to N deposition differs by ecosystem type. In peat bogs, elevated N deposition decreased polyphenols' contents and decreased the polyphenol ratio to N, which may increase N2O production due to an inverse relationship between N2O emissions and the polyphenol to nitrogen ratio (Pimentel et al., 2015).
N2O production of active N2O emitters
The three N2O emitters preferred KNO3 as a substrate over NH4Cl. This result suggested that these three isolates mainly use DNRA or denitrification to produce N2O gas. For the Enterobacteriaceae bacterium SC-L1 and Serratia sp. SC-K1, the nirS, nirK, and nosZ genes were not detected, but the narG gene was, suggesting that they do not have nitrite reductase and are non-denitrifiers consistent with other Enterobacteriaceae bacteria emitting N2O as a final product (Arkenberg et al., 2011). Enterobacter species are often reported as producing N2O by DNRA (Smith and Zimmerman, 1981). This result indicated that they are also important sources for N2O emissions in SC dominant bogs. Pseudomonas sp. SC-H2 harbored nosZ, nirS, and narG. Therefore, Pseudomonas sp. SC-H2 was a typical denitrifier. Microbial heterotrophic denitrification and DNRA compete for shared resources (Jia et al., 2020).
Although the N2O potential was relatively high in the SC sample, the N2O emissions in situ in the peat bogs were generally low in northern Finland, which might be impacted by the complexity of environmental conditions (Dinsmore et al., 2017). The potential N2O emissions in the field (Repo et al., 2009; Voigt et al., 2017b) and laboratory incubations (Elberling et al., 2010) increase with increasing mineral N availability, permafrost thawing, and drainage. A previous study suggested that drainage of bogs alters nutrient cycling and microbial communities to increase N2O emissions (Frolking et al., 2011). Unvegetated (free of vascular plants) peat surfaces resulting from wind erosion and frost action were hot spots for N2O emission in subarctic permafrost peatlands due to the absence of plant nitrogen uptake, a low C:N ratio, and sufficient drainage (Marushchak et al., 2011; Voigt et al., 2017b). Pseudomonas sp. SC-H2 had negligible N2O emissions at low pH (<4.5), while the other two N2O-emitting Enterobacteriaceae bacteria from SC exhibited contrasting patterns in the Sphagnum bogs. Therefore, the contribution of denitrification and DNRA to N2O emissions in boreal peat bogs should be considered in future studies.
Conclusion
In summary, our study identified several N2O emitters in microbial communities of Sphagnum samples from the subarctic permafrost habitat of palsa mires. A composite sample of SC showed high potential to emit N2O, and a composite of SF showed moderate potential to emit N2O. The N2O emission potential was attributed to distinctive bacterial communities inhabiting moss leaves in both cases. Two classes of hyperactive N2O emitters hidden in the SC holobiont were revealed. Pseudomonas sp. SC-H2 was found to harbor narG, nirS, and nosZ genes. N2O reduction to N2 catalyzed by N2O reductase was noteworthy in the neutral pH microenvironment. The other hyperactive N2O emitters, Enterobacteriaceae bacterium SC-L1 and Serratia sp. SC-K1 lacked the nirS, nirK, and nosZ genes but contained the narG gene and emitted NO/N2O as the final product, possibly via the DNRA pathway. These findings provided some theoretical evidence for the future N2O emission study of the in situ subarctic palsa under elevated N availability and global warming.
Data availability statement
The datasets presented in this study can be found in online repositories. The names of the repository/repositories and accession number(s) can be found in the article/Supplementary material.
Author contributions
YH and YN designed the research, experiments, and acquired the funds. YH, RI, and TT collected the samples in Finland. YN performed experiments and analyzed data. YN, SYL, XT, XL, SL, TT, RI, and QY wrote and edited the paper. All authors read and approved the final manuscript.
Funding
This research was supported by the National Natural Science Foundation of China (32071596 to YN), the Key Special Project for Introduced Talents Team of Southern Marine Science and Engineering Guangdong Laboratory (Guangzhou) (GML2019ZD0408), Grants-in-Aid A (20255002 and 26252058 to YH) and B (26304042 to YH) by JSPS (Japan Society for the Promotion of Science). Kilpisjärvi Biological Station of the University of Helsinki supported our fieldwork. We sincerely appreciate the Chinese Scholarship Council for a scholarship to YN (CSC 201204910200).
Acknowledgments
We are particularly grateful to Professor Ryusuke Hatano for the GC instruments used in the N2O assay (Soil Science Laboratory, Research Faculty of Agriculture, Hokkaido University, Japan). We thank Professor Akira Haraguchi for their assistance and advice. We thank Hiroaki Nishizuka for their sampling assistance.
Conflict of interest
The authors declare that the research was conducted in the absence of any commercial or financial relationships that could be construed as a potential conflict of interest.
Publisher's note
All claims expressed in this article are solely those of the authors and do not necessarily represent those of their affiliated organizations, or those of the publisher, the editors and the reviewers. Any product that may be evaluated in this article, or claim that may be made by its manufacturer, is not guaranteed or endorsed by the publisher.
Supplementary material
The Supplementary Material for this article can be found online at: https://www.frontiersin.org/articles/10.3389/fpls.2022.974251/full#supplementary-material
References
Aerts, R., Wallén, B., Malmer, N., and De Caluwe, H. (2001). Nutritional constraints on Sphagnum-growth and potential decay in northern peatlands. J. Ecol. 89, 292–299. doi: 10.1046/j.1365-2745.2001.00539.x
Anderson, I. C., and Levine, J. S. (1986). Relative rates of nitric oxide and nitrous oxide production by nitrifiers, denitrifiers, and nitrate respirers. Appl. Environ. Microbiol. 51, 938–945. doi: 10.1128/aem.51.5.938-945.1986
Arkenberg, A., Runkel, S., Richardson, D. J., and Rowley, G. (2011). The production and detoxification of a potent cytotoxin, nitric oxide, by pathogenic enteric bacteria. Biochem. Soc. Trans. 39, 1876–1879. doi: 10.1042/BST20110716
Balderston, W. L., Sherr, B., and Payne, W. (1976). Blockage by acetylene of nitrous oxide reduction in Pseudomonas perfectomarinus. Appl. Environ. Microbiol. 31, 504–508. doi: 10.1128/aem.31.4.504-508.1976
Bollmann, A., and Conrad, R. (1997). Acetylene blockage technique leads to underestimation of denitrification rates in oxic soils due to scavenging of intermediate nitric oxide. Soil Biol. Biochem. 29, 1067–1077. doi: 10.1016/S0038-0717(97)00007-2
Bonnett, S. A. F., Ostle, N., and Freeman, C. (2010). Short-term effect of deep shade and enhanced nitrogen supply on Sphagnum capillifolium morphophysiology. Plant Ecol. 207, 347–358. doi: 10.1007/s11258-009-9678-0
Borge, A. F., Westermann, S., Solheim, I., and Etzelmuller, B. (2017). Strong degradation of palsas and peat plateaus in northern Norway during the last 60 years. Cryosphere 11, 1–16. doi: 10.5194/tc-11-1-2017
Bragazza, L., and Freeman, C. (2007). High nitrogen availability reduces polyphenol content in Sphagnum peat. Sci. Total Environ. 377, 439–443. doi: 10.1016/j.scitotenv.2007.02.016
Bragazza, L., Freeman, C., Jones, T., Rydin, H., Limpens, J., Fenner, N., et al. (2006). Atmospheric nitrogen deposition promotes carbon loss from peat bogs. Proc. Natl. Acad. Sci. U. S. A. 103, 19386–19389. doi: 10.1073/pnas.0606629104
Bragina, A., Oberauner-Wappis, L., Zachow, C., Halwachs, B., Thallinger, G. G., Müller, H., et al. (2014). The Sphagnum microbiome supports bog ecosystem functioning under extreme conditions. Mol. Ecol. 23, 4498–4510. doi: 10.1111/mec.12885
Braker, G., Fesefeldt, A., and Witzel, K.-P. (1998). Development of PCR primer systems for amplification of nitrite reductase genes (nirK and nirS) to detect denitrifying bacteria in environmental samples. Appl. Environ. Microbiol. 64, 3769–3775. doi: 10.1128/AEM.64.10.3769-3775.1998
Chen, M., Chang, L., Zhang, J., Guo, F., Vymazal, J., He, Q., et al. (2020). Global nitrogen input on wetland ecosystem: the driving mechanism of soil labile carbon and nitrogen on greenhouse gas emissions. Environ. Sci. Ecotechnol. 4:100063. doi: 10.1016/j.ese.2020.100063
Christensen, J. H., Kanikicharla, K. K., Aldrian, E., An, S.-I., Cavalcanti, I. F. A., de Castro, M., et al. (2013) “Climate phenomena and their relevance for future regional climate change supplementary material,” in Climate Change 2013: The Physical Science Basis. Contribution of Working Group I to the Fifth Assessment Report of the Intergovernmental Panel on Climate Change, eds T. F. Stocker, D. Qin, G.-K. Plattner, M. Tignor, S. K. Allen, J. Boschung, A. Nauels, Y. Xia, V. Bex, and P. M. Midgley. Available online at: www.climatechange2013.org; www.ipcc.ch
Chronáková, A., Barta, J., Kaštovská, E., Urbanová, Z., and Picek, T. (2019). Spatial heterogeneity of belowground microbial communities linked to peatland microhabitats with different plant dominants. FEMS Microbiol. Ecol. 95:fiz130. doi: 10.1093/femsec/fiz130
Dedysh, S. N., Pankratov, T. A., Belova, S. E., Kulichevskaya, I. S., and Liesack, W. (2006). Phylogenetic analysis and in situ identification of bacteria community composition in an acidic Sphagnum peat bog. Appl. Environ. Microbiol. 72, 2110–2117. doi: 10.1128/AEM.72.3.2110-2117.2006
Delwiche, C. (1959). Production and utilization of nitrous oxide by Pseudomonas denitrificans. J. Bacteriol. 77, 55–59. doi: 10.1128/jb.77.1.55-59.1959
Dinsmore, K. J., Drewer, J., Levy, P. E., George, C., Lohila, A., Aurela, M., et al. (2017). Growing season CH4 and N2O fluxes from a subarctic landscape in northern Finland; from chamber to landscape scale. Biogeosciences 14, 799–815. doi: 10.5194/bg-14-799-2017
Dooley, D. M., Moog, R. S., and Zumft, W. G. (1987). Characterization of the copper sites in Pseudomonas perfectomarina nitrous oxide reductase by resonance Raman spectroscopy. J. Am. Chem. Soc. 109, 6730–6735. doi: 10.1021/ja00256a029
Elberling, B., Christiansen, H. H., and Hansen, B. U. (2010). High nitrous oxide production from thawing permafrost. Nat. Geosci. 3, 332–335. doi: 10.1038/ngeo803
Eurola, S., Hicks, S. T., and Kaakinen, E. (1984). “Key to finnish mire types,” in European Mires, ed P. D. Moore (London, Great Britain: Academic Press), 1–117. doi: 10.1016/b978-0-12-505580-2.50006-4
Ferris, M., Muyzer, G., and Ward, D. (1996). Denaturing gradient gel electrophoresis profiles of 16S rRNA-defined populations inhabiting a hot spring microbial mat community. Appl. Environ. Microbiol. 62, 340–346. doi: 10.1128/aem.62.2.340-346.1996
Francis, C. A., Beman, J. M., and Kuypers, M. M. (2007). New processes and players in the nitrogen cycle: the microbial ecology of anaerobic and archaeal ammonia oxidation. ISME J. 1, 19–27. doi: 10.1038/ismej.2007.8
Freeman, C., Ostle, N. J., Fenner, N., and Kang, H. (2004). A regulatory role for phenol oxidase during decomposition in peatlands. Soil Biol. Biochem. 36, 1663–1667. doi: 10.1016/j.soilbio.2004.07.012
Frolking, S., Talbot, J., Jones, M. C., Treat, C. C., Kauffman, J. B., Tuittila, E. S., et al. (2011). Peatlands in the Earth's 21st century climate system. Environ. Rev. 19, 371–396. doi: 10.1139/a11-014
Gilbert, D., Mitchell, E., Martini, I., Martínez-Cortizas, A., and Chesworth, W. (2006). “Microbial diversity in Sphagnum peatlands,” in Peatlands: Evolution and Records of Environmental and Climate Changes (Elsevier: Amsterdam), 287–318. doi: 10.1016/S0928-2025(06)09013-4
Hashidoko, Y., Takakai, F., Toma, Y., Darung, U., Melling, L., Tahara, S., et al. (2008). Emergence and behaviors of acid-tolerant Janthinobacterium sp. that evolves N2O from deforested tropical peatland. Soil Biol. Biochem. 40, 116–125. doi: 10.1016/j.soilbio.2007.07.014
Hashidoko, Y., Takeda, H., Hasegawa, S., Hara, S., Wijaya, H., Darung, U., et al. (2010). “Screening of N2O-emitting bacteria from acidic soils and their characteristics under acidic conditions,” in Proceedings of Bogor Symposium and Workshop on Tropical Peatland Management, (Bogor) 14–15 July 2009, 52–56.
Heo, H., Kwon, M., Song, B., and Yoon, S. (2020). Involvement of in ecophysiological regulation of dissimilatory nitrate/nitrite reduction to ammonium (DNRA) is implied by physiological characterization of soil DNRA bacteria isolated via a colorimetric screening method. Appl. Environ. Microbiol. 86:e01054-20. doi: 10.1128/AEM.01054-20
Huang, Y., Zou, J., Zheng, X., Wang, Y., and Xu, X. (2004). Nitrous oxide emissions as influenced by amendment of plant residues with different C:N ratios. Soil Biol. Biochem. 36, 973–981. doi: 10.1016/j.soilbio.2004.02.009
IPCC (2007). “Climate change 2007. Mitigation of climate change,” in Working Group III Contribution to the Fourth Assessment Report of the Intergovernmental Panel on Climate Change, eds B. Metz, O. Davidson, P. Bosch, R. Dave, L. Meyer (Cambridge University Press: Cambridge).
Jia, M., Winkler, M. K. H., and Volcke, E. I. P. (2020). Elucidating the competition between heterotrophic denitrification and DNRA using the resource-ratio theory. Environ. Sci. Technol. 54, 13953–13962. doi: 10.1021/acs.est.0c01776
Klemedtsson, L., Von Arnold, K., Weslien, P., and Gundersen, P. (2005). Soil CN ratio as a scalar parameter to predict nitrous oxide emissions. Glob. Chang. Biol. 11, 1142–1147. doi: 10.1111/j.1365-2486.2005.00973.x
Limpens, J., and Berendse, F. (2003). Growth reduction of Sphagnum magellanicum subjected to high nitrogen deposition: the role of amino acid nitrogen concentration. Oecologia 135, 339–345. doi: 10.1007/s00442-003-1224-5
Liu, B., Frostegård, Å., and Bakken, L. R. (2014). Impaired reduction of N2O to N2 in acid soils is due to a posttranscriptional interference with the expression of nosZ. mBio 5:e01383-14. doi: 10.1128/mBio.01383-14
Maljanen, M., Shurpali, N., Hytönen, J., Mäkiranta, P., Aro, L., Potila, H., et al. (2012). Afforestation does not necessarily reduce nitrous oxide emissions from managed boreal peat soils. Biogeochemistry 108, 199–218. doi: 10.1007/s10533-011-9591-1
Markham, J. H. (2009). Variation in moss-associated nitrogen fixation in boreal forest stands. Oecologia 161, 353–359. doi: 10.1007/s00442-009-1391-0
Martikainen, P. J., Nykänen, H., Alm, J., and Silvola, J. (1995). Change in fluxes of carbon dioxide, methane and nitrous oxide due to forest drainage of mire sites of different trophy. Plant Soil 168, 571–577. doi: 10.1007/BF00029370
Marushchak, M. E., Pitkamaki, A., Koponen, H., Biasi, C., Seppala, M., and Martikainen, P. J. (2011). Hot spots for nitrous oxide emissions found in different types of permafrost peatlands. Glob. Chang. Biol. 17, 2601–2614. doi: 10.1111/j.1365-2486.2011.02442.x
Minkkinen, K., Ojanen, P., Koskinen, M., and Penttilä, T. (2020). Nitrous oxide emissions of undrained, forestry-drained, and rewetted boreal peatlands. For. Ecol. Manage. 478:118494. doi: 10.1016/j.foreco.2020.118494
Minkkinen, K., Ojanen, P., Penttila, T., Aurela, M., Laurila, T., Tuovinen, J. P., et al. (2018). Persistent carbon sink at a boreal drained bog forest. Biogeosciences 15, 3603–3624. doi: 10.5194/bg-15-3603-2018
Montenegro, G., Portaluppi, M. C., Salas, F. A., and Diaz, M. F. (2009). Biological properties of the Chilean native moss Sphagnum magellanicum. Biol. Res. 42, 233–237. doi: 10.4067/S0716-97602009000200012
Nie, Y., Li, L., Wang, M., Tahvanainen, T., and Hashidoko, Y. (2015). Nitrous oxide emission potentials of Burkholderia species isolated from the leaves of a boreal peat moss Sphagnum fuscum. Biosci. Biotechnol. Biochem. 79, 2086–2095. doi: 10.1080/09168451.2015.1061420
Novak, M., Veselovsky, F., Curik, J., Stepanova, M., Fottova, D., Prechova, E., et al. (2015). Nitrogen input into Sphagnum bogs via horizontal deposition: an estimate for N-polluted high-elevation sites. Biogeochemistry 123, 307–312. doi: 10.1007/s10533-015-0076-5
Opelt, K., Chobot, V., Hadacek, F., Schonmann, S., Eberl, L., and Berg, G. (2007). Investigations of the structure and function of bacterial communities associated with Sphagnum mosses. Environ. Microbiol. 9, 2795–2809. doi: 10.1111/j.1462-2920.2007.01391.x
Palmer, K., and Horn, M. (2015). Denitrification activity of a remarkably diverse fen denitrifier community in Finnish lapland is N-oxide limited. PLoS ONE 10:e0123123. doi: 10.1371/journal.pone.0123123
Palmer, K., and Horn, M. A. (2012). Actinobacterial nitrate reducers and proteobacterial denitrifiers are abundant in N2O-metabolizing palsa peat. Appl. Environ. Microbiol. 78, 5584–5596. doi: 10.1128/AEM.00810-12
Payne, W. J., Riley, P., and Cox, C. (1971). Separate nitrite, nitric oxide, and nitrous oxide reducing fractions from Pseudomonas perfectomarinus. J. Bacteriol. 106, 356–361. doi: 10.1128/jb.106.2.356-361.1971
Pfenning, K., and McMahon, P. (1997). Effect of nitrate, organic carbon, and temperature on potential denitrification rates in nitrate-rich riverbed sediments. J. Hydrol. 187, 283–295. doi: 10.1016/S0022-1694(96)03052-1
Pimentel, L. G., Weiler, D. A., Pedroso, G. M., and Bayer, C. (2015). Soil N2O emissions following cover-crop residues application under two soil moisture conditions. J. Plant Nutr. Soil Sci. 178, 631–640. doi: 10.1002/jpln.201400392
Prudêncio, M., Pereira, A. S., Tavares, P., Besson, S., Cabrito, I., Brown, K., et al. (2000). Purification, characterization, and preliminary crystallographic study of copper-containing nitrous oxide reductase from Pseudomonas nautica 617. Biochemistry 39, 3899–3907. doi: 10.1021/bi9926328
Ravishankara, A. R., Daniel, J. S., and Portmann, R. W. (2009). Nitrous Oxide (N2O): the dominant ozone-depleting substance emitted in the 21st century. Science 326, 123–125. doi: 10.1126/science.1176985
Regina, K., Nykänen, H., Silvola, J., and Martikainen, P. J. (1996). Fluxes of nitrous oxide from boreal peatlands as affected by peatland type, water table level and nitrification capacity. Biogeochemistry 35, 401–418. doi: 10.1007/BF02183033
Regina, K., Silvola, J., and Martikainen, P. J. (1999). Short-term effects of changing water table on N2O fluxes from peat monoliths from natural and drained boreal peatlands. Glob. Chang. Biol. 5, 183–189. doi: 10.1046/j.1365-2486.1999.00217.x
Repo, M. E., Susiluoto, S., Lind, S. E., Jokinen, S., Elsakov, V., Biasi, C., et al. (2009). Large N2O emissions from cryoturbated peat soil in tundra. Nat. Geosci. 2, 189–192. doi: 10.1038/ngeo434
Savolainen, I., Hillebrand, K., Nousiainen, I., and Sinisalo, J. (1994). Greenhouse Impacts of the Use of Peat and Wood for Energy. Espoo: Technical Research Centre of Finland.
Scala, D. J., and Kerkhof, L. J. (1998). Nitrous oxide reductase (nosZ) gene-specific PCR primers for detection of denitrifiers and three nosZ genes from marine sediments. FEMS Microbiol. Lett. 162, 61–68. doi: 10.1111/j.1574-6968.1998.tb12979.x
Seppälä, M. (2011). Synthesis of studies of palsa formation underlining the importance of local environmental and physical characteristics. Quatern. Res. 75, 366–370. doi: 10.1016/j.yqres.2010.09.007
Smith, M. S., and Zimmerman, K. (1981). Nitrous oxide production by nondenitrifying soil nitrate reducers. Soil Sci. Soc. Am. J. 45, 865–871 doi: 10.2136/sssaj1981.03615995004500050008x
SooHoo, C. K., and Hollocher, T. (1991). Purification and characterization of nitrous oxide reductase from Pseudomonas aeruginosa strain P2. J. Biol. Chem. 266, 2203–2209. doi: 10.1016/S0021-9258(18)52229-8
Sørensen, J. (1978). Denitrification rates in a marine sediment as measured by the acetylene inhibition technique. Appl. Environ. Microbiol. 36, 139–143. doi: 10.1128/aem.36.1.139-143.1978
Sørensen, J., Tiedje, J., and Firestone, R. (1980). Inhibition by sulfide of nitric and nitrous oxide reduction by denitrifying Pseudomonas fluorescens. Appl. Environ. Microbiol. 39, 105–108. doi: 10.1128/aem.39.1.105-108.1980
Sun, H., Terhonen, E., Koskinen, K., Paulin, L., Kasanen, R., and Asiegbu, F. O. (2014). Bacterial diversity and community structure along different peat soils in boreal forest. Appl. Soil Ecol. 74, 37–45. doi: 10.1016/j.apsoil.2013.09.010
Swindles, G. T., Morris, P. J., Mullan, D. J., Payne, R. J., Roland, T. P., Amesbury, M. J., et al. (2019). Widespread drying of European peatlands in recent centuries. Nat. Geosci. 12, 922–928. doi: 10.1038/s41561-019-0462-z
Tahvanainen, T., and Haraguchi, A. (2013). Effect of pH on phenol oxidase activity on decaying Sphagnum mosses. Eur. J. Soil Biol. 54, 41–47. doi: 10.1016/j.ejsobi.2012.10.005
Tahvanainen, T., and Tuomaala, T. (2003). The reliability of mire water pH measurements–A standard sampling protocol and implications to ecological theory. Wetlands 23, 701–708. doi: 10.1672/0277-5212(2003)0230701:TROMWP2.0.CO;2
Takatsu, Y., Miyamoto, T., Tahvanainen, T., and Hashidoko, Y. (2022). Nitrous oxide emission in response to pH from degrading palsa mire peat due to permafrost thawing. Curr. Microbiol. 79:56. doi: 10.1007/s00284-021-02690-8
Throbäck, I. N., Enwall, K., Jarvis, Å., and Hallin, S. (2004). Reassessing PCR primers targeting nirS, nirK and nosZ genes for community surveys of denitrifying bacteria with DGGE. FEMS Microbiol. Ecol. 49, 401–417. doi: 10.1016/j.femsec.2004.04.011
Van Cleemput, O. (1998). Subsoils: chemo-and biological denitrification, N2O and N2 emissions. Nutr. Cycling Agroecosyst. 52, 187–194. doi: 10.1023/A:1009728125678
Viebrock, A., and Zumft, W. (1988). Molecular cloning, heterologous expression, and primary structure of the structural gene for the copper enzyme nitrous oxide reductase from denitrifying Pseudomonas stutzeri. J. Bacteriol. 170, 4658–4668. doi: 10.1128/jb.170.10.4658-4668.1988
Vitt, D. H., Wieder, K., Halsey, L. A., and Turetsky, M. (2003). Response of Sphagnum fuscum to nitrogen deposition: a case study of ombrogenous peatlands in Alberta, Canada. Bryologist 106, 235–245. doi: 10.1639/0007-2745(2003)1060235:ROSFTN2.0.CO;2
Voigt, C., Lamprecht, R. E., Marushchak, M. E., Lind, S. E., Novakovskiy, A., Aurela, M., et al. (2017a). Warming of subarctic tundra increases emissions of all three important greenhouse gases-carbon dioxide, methane, and nitrous oxide. Glob. Chang. Biol. 23, 3121–3138. doi: 10.1111/gcb.13563
Voigt, C., Marushchak, M. E., Lamprecht, R. E., Jackowicz-Korczynski, M., Lindgren, A., Mastepanov, M., et al. (2017b). Increased nitrous oxide emissions from Arctic peatlands after permafrost thaw. Proc. Natl. Acad. Sci. U. S. A. 114, 6238–6243. doi: 10.1073/pnas.1702902114
Wang, M., and Cernava, T. (2020). Overhauling the assessment of agrochemical-driven interferences with microbial communities for improved global ecosystem integrity. Environ. Sci. Ecotechnol. 4:100061. doi: 10.1016/j.ese.2020.100061
Wrage, N., Velthof, G., Van Beusichem, M., and Oenema, O. (2001). Role of nitrifier denitrification in the production of nitrous oxide. Soil Biol. Biochem. 33, 1723–1732. doi: 10.1016/S0038-0717(01)00096-7
Keywords: Sphagnum moss, bacteria, N2O emitters, N2O-related genes, pH, permafrost peat
Citation: Nie Y, Lau SYL, Tan X, Lu X, Liu S, Tahvanainen T, Isoda R, Ye Q and Hashidoko Y (2022) Sphagnum capillifolium holobiont from a subarctic palsa bog aggravates the potential of nitrous oxide emissions. Front. Plant Sci. 13:974251. doi: 10.3389/fpls.2022.974251
Received: 21 June 2022; Accepted: 08 August 2022;
Published: 07 September 2022.
Edited by:
Mengcen Wang, Zhejiang University, ChinaReviewed by:
Haruna Matsumoto, Zhejiang University, ChinaKou Yongping, Chinese Academy of Sciences (CAS), China
Copyright © 2022 Nie, Lau, Tan, Lu, Liu, Tahvanainen, Isoda, Ye and Hashidoko. This is an open-access article distributed under the terms of the Creative Commons Attribution License (CC BY). The use, distribution or reproduction in other forums is permitted, provided the original author(s) and the copyright owner(s) are credited and that the original publication in this journal is cited, in accordance with accepted academic practice. No use, distribution or reproduction is permitted which does not comply with these terms.
*Correspondence: Yanxia Nie, bmlleWFueEBzY2JnLmFjLmNu
†Deceased