- 1Department of Agronomy (DAUCO-María de Maeztu Unit of Excellence), Campus de Excelencia Internacional Agroalimentario, Universidad de Córdoba, Córdoba, Spain
- 2Department of Botany, Ecology and Plant Physiology, Campus de Excelencia Internacional Agroalimentario, Universidad de Córdoba, Córdoba, Spain
When plants suffer from Fe deficiency, they develop morphological and physiological responses, mainly in their roots, aimed to facilitate Fe mobilization and uptake. Once Fe has been acquired in sufficient quantity, the responses need to be switched off to avoid Fe toxicity and to conserve energy. Several hormones and signaling molecules, such as ethylene, auxin and nitric oxide, have been involved in the activation of Fe deficiency responses in Strategy I plants. These hormones and signaling molecules have almost no effect when applied to plants grown under Fe-sufficient conditions, which suggests the existence of a repressive signal related to the internal Fe content. The nature of this repressive signal is not known yet many experimental results suggest that is not related to the whole root Fe content but to some kind of Fe compound moving from leaves to roots through the phloem. After that, this signal has been named LOng-Distance Iron Signal (LODIS). Very recently, a novel family of small peptides, “IRON MAN” (IMA), has been identified as key components of the induction of Fe deficiency responses. However, the relationship between LODIS and IMA peptides is not known. The main objective of this work has been to clarify the relationship between both signals. For this, we have used Arabidopsis wild type (WT) Columbia and two of its mutants, opt3 and frd3, affected, either directly or indirectly, in the transport of Fe (LODIS) through the phloem. Both mutants present constitutive activation of Fe acquisition genes when grown in a Fe-sufficient medium despite the high accumulation of Fe in their roots. Arabidopsis WT Columbia plants and both mutants were treated with foliar application of Fe, and later on the expression of IMA and Fe acquisition genes was analyzed. The results obtained suggest that LODIS may act upstream of IMA peptides in the regulation of Fe deficiency responses in roots. The possible regulation of IMA peptides by ethylene has also been studied. Results obtained with ethylene precursors and inhibitors, and occurrence of ethylene-responsive cis-acting elements in the promoters of IMA genes, suggest that IMA peptides could also be regulated by ethylene.
Introduction
Iron (Fe) participates in many important processes of plants, such as photosynthesis, respiration and nitrogen metabolism (Marschner, 2012). Iron is abundant in most soils, but its availability for plants is low, especially in calcareous soils, where the incidence of Fe chlorosis is frequent (Briat et al., 2015). Since calcareous soils represent more than 30% of arable soils, Fe chlorosis is one of the most important deficiencies caused by a micronutrient worldwide. Under Fe-deficient conditions, Strategy I plants (all plants but grasses) induce morphological and physiological changes in their roots, known as Fe deficiency responses (Kobayashi and Nishizawa, 2012; Brumbarova et al., 2015; Lucena et al., 2015), which favor Fe mobilization and acquisition. Among the main physiological responses are: an enhanced ferric reductase activity (FRA; determined by FRO genes), an enhanced Fe(II) uptake capacity (determined by IRT genes) and the acidification of the rhizosphere (Kobayashi and Nishizawa, 2012; Brumbarova et al., 2015). Among the morphological responses are: development of root hairs, of cluster roots and of transfer cells, all of them aimed to increase the surface of contact with soil (Lucena et al., 2015; Romera et al., 2017).
In the last years, the knowledge of the regulatory network controlling Fe deficiency responses and Fe acquisition by plants has increased considerably. In Arabidopsis (Strategy I), the master regulator of most Fe acquisition genes is FIT (bHLH29), a basic-helix–loop–helix transcription factor (TF), homologous of the tomato FER (Ling et al., 2002; Colangelo and Guerinot, 2004). For regulating key Fe acquisition genes, like FRO2 and IRT1, FIT interacts with another bHLH TFs of the Ib subgroup, such as bHLH38, bHLH39, bHLH100 and bHLH101. All of them are induced in roots in response to Fe deficiency and have redundant function (Yuan et al., 2008; Wang et al., 2013; Brumbarova et al., 2015). The expression of FIT, bHLH38, bHLH39, bHLH100 and bHLH101 is induced by homo- and heterodimers formed by IVc subgroup bHLH TFs: bHLH105 (ILR3) and its closest homologs bHLH34, bHLH104 and bHLH115 (Zhang et al., 2015; Li et al., 2016; Liang et al., 2017; Gao et al., 2019). Upstream of the IVc subgroup there are other bHLH TFs, like bHLH121 (URI), and the BRUTUS (BTS) and BTS-LIKE (BTSL) proteins (Zhang et al., 2015; Hindt et al., 2017; Kim et al., 2019; Rodríguez-Celma et al., 2019; Gao et al., 2020). BTS and BTSL proteins are E3 ligases which act as potential Fe sensors that interact with IVc bHLH TFs and FIT, targeting them for proteasomal degradation (Zhang et al., 2015; Liang et al., 2017; Rodríguez-Celma et al., 2019). Since FIT and the IVc bHLH TFs act as positive regulators of Fe deficiency responses, BTS and BTSL proteins would act as negative regulators (Zhang et al., 2015; Hindt et al., 2017; Rodríguez-Celma et al., 2019; Li et al., 2021).
Several hormones and signaling substances, whose production increases in Fe-deficient roots, have been implicated in the activation of Fe deficiency responses. Among them, ethylene plays a prominent role by up-regulating up to 19 Fe deficiency induced genes, including main regulatory genes, like FIT, bHLH38 and bHLH39, as well as essential genes for Fe acquisition (FRO2 and IRT1) and distribution (FRD3, NAS1 and NAS2; Lucena et al., 2006, 2015; Waters et al., 2007; García et al., 2010, 2011, 2018; Lingam et al., 2011; Romera et al., 2011, 2017; Yang et al., 2014; Li and Lan, 2017). Ethylene has also been involved in the regulation of morphological responses to Fe deficiency (Romera et al., 2011, 2017; Lucena et al., 2015) and in restricting the suberization of the endodermis under Fe deficiency (Barberon et al., 2016). Ethylene, auxin and other signaling substances, like NO (nitric oxide), greatly activate the expression of Fe acquisition genes in plants grown with low levels of Fe but weakly in those grown with high levels of Fe (Lucena et al., 2006; Graziano and Lamattina, 2007; Chen et al., 2010; Bacaicoa et al., 2011; García et al., 2011). This suggests the existence of a repressive signal related to the internal Fe content. The nature of this repressive signal is not yet known but many experimental results suggest that is not related to the whole root Fe content but to some kind of Fe compound moving from leaves to roots through the phloem. After that, this signal has been named LOng-Distance Iron Signal (LODIS; García et al., 2018). Although the nature of LODIS is not known, its consequences have already been investigated by comparing LODIS-deficient mutants, such as Arabidopsis frd3 and opt3, with the wild type (WT) cultivar Columbia, and by comparing plants treated or not with foliar application of Fe.
The Arabidopsis frd3 and opt3 mutants present constitutive activation of Fe acquisition genes (Rogers and Guerinot, 2002; Stacey et al., 2008; García et al., 2013). The frd3 mutant is affected in xylem Fe transport, being chlorotic even when grown in a Fe-sufficient medium (Durrett et al., 2007). However, it becomes green, and its Fe acquisition genes are down-regulated, when treated with Fe in the leaves, which indicates that LODIS can move from leaves to roots through its phloem (Lucena et al., 2006). However, the foliar application of Fe does not down-regulate Fe acquisition genes in opt3 plants, indicating that the opt3 mutation impairs LODIS translocation from leaves to roots (García et al., 2013, 2018). The Arabidopsis mutant opt3 is impaired in the loading of Fe and Cu into the phloem (Stacey et al., 2008; García et al., 2013, 2018; Chia et al., 2021). OPT3, whose expression, mainly in shoots, is enhanced under Fe or Cu deficiency, belongs to the oligopeptide transporter (OPT) family (Stacey et al., 2008; Chia et al., 2021). The nature of the substrate transported through OPT3 is not yet clear. Some authors, such as Zhai et al. (2014) and Chia et al. (2021), have found that OPT3 can transport Fe2+ and Cu2+ ions when expressed in Xenopus oocytes. However, other authors, like Mendoza-Cózatl et al. (2014), have found that OPT3 is unable to rescue the fet3fet4 strain of yeast, impaired in Fe uptake. In any case, even if OPT3 transports Fe ions, these ones should be chelated in the phloem sap to avoid their precipitation (Gutiérrez-Carbonell et al., 2015). GSH-derived compounds, proteins and peptides are among the proposed chelating agents (Krüger et al., 2002; Ramírez et al., 2011; Darbani et al., 2013; García et al., 2013).
Recently, a family of small peptides named IRON MAN/FE-UPTAKE-INDUCING PEPTIDE (IMA/FEP) has been shown to play a key role in the regulatory network of Fe acquisition and cellular homeostasis in plants (Grillet et al., 2018; Hirayama et al., 2018; Gautam et al., 2021; Kobayashi et al., 2021; Li et al., 2021). The Arabidopsis genome harbours eight IMA genes, all of them responsive to Fe supply. IMA1, IMA2 and IMA3 are highly expressed in both leaves and roots of Fe-deficient plants (Grillet et al., 2018). IMA1, IMA2 and IMA3 expression is under the transcriptional control of bHLH TFs, like bHLH105, bHLH115 or bHLH121 (see above; Gao et al., 2020; Li et al., 2021). Moreover, IMA peptides are ubiquitinated by BTS or BTSL proteins and degraded via the proteasome (Li et al., 2021; Lichtblau et al., 2022). These authors propose that IMA peptides could impair the interaction of BTS and BTSL proteins with IVc bHLH TFs, thus diminishing their proteasomal degradation and, consequently, favoring the activation of Fe deficiency responses in Arabidopsis roots (Li et al., 2021; Lichtblau et al., 2022).
IMA peptides function is redundant: ima8x plants are very small, chlorotic and died few days when grown under Fe sufficient conditions; however, the overexpression of IMA1 in ima8x plants restored the growth, chlorophyll content and the ferric reductase activity (Grillet et al., 2018). The results obtained by RNAseq experiments carried out in IMA overexpressing lines (IMA1Ox) showed that most of the main regulators of Fe deficiency responses, such as bHLH38, bHLH39, bHLH100 and bHLH101, and genes involved in Fe uptake (FRO2 and IRT1) or distribution (NAS1, NAS2 and FRD3) are strongly induced in roots of Fe-sufficient IMA1Ox plants. Genes encoding important proteins for Fe storage, such as the ferritins FER1 and FER3, and the vacuolar Fe transporters VTL1, VTL2 and VTL5, are also upregulated in IMA1Ox plants (Grillet et al., 2018). The ectopic expression of IMA1 and IMA2 also induces the biosynthesis and secretion of fraxetin, a coumarin, through the induction of the MYB72 TF and the S8H gene, encoding the Scopoletin 8-Hydroxylase enzyme (Gautam et al., 2021). Taken together, all these results clearly support a role for IMA peptides as activators of Fe deficiency responses in Arabidopsis roots.
Besides Arabidopsis, two IMA/FEP genes have been described in rice (Kobayashi et al., 2021). The expression of OsIMA1 and OsIMA2 is strongly induced under Fe deficiency, and the overexpression of OsIMA1 or OsIMA2 in rice confers tolerance to Fe deficiency and accumulation of Fe in leaves and seeds. The OsIMA-overexpressing lines exhibit enhanced expression of most of the known Fe deficiency-inducible genes involved in Fe uptake and translocation (Kobayashi et al., 2021). As occurs in Arabidopsis, OsIMA1 and OsIMA2 expression is also regulated by bHLH TFs, such as OsbHLH058 and OsbHLH059, and IMA proteins are degraded upon interaction with HRZ proteins (BTS homologous; Kobayashi et al., 2021; Peng et al., 2022).
IMA peptides have been described few years ago and, although some details about their regulation are known, there is still a long way to go. The relationship between IMA peptides and LODIS, as well as the relationship between IMA peptides and other signals involved in the regulation of Fe deficiency responses, such as ethylene, has not been studied yet. To clarify their possible interactions is the main objective of this work.
Materials and methods
Plant materials, growth conditions and treatments
Arabidopsis thaliana seeds from (L.) Heynh ecotype ‘Columbia’, and from its LODIS-deficient mutants opt3-2 and frd3-3, were germinated and grown under controlled conditions as previously described (Lucena et al., 2006; García et al., 2018). The opt3-2 mutant is impaired in the loading of Fe2+ ions into the phloem (Stacey et al., 2008; Zhai et al., 2014; Chia et al., 2021). The frd3-3 mutant is impaired in xylem Fe transport (Rogers and Guerinot, 2002) but, as a consequence, less Fe gets to leaves to enter the phloem (Lucena et al., 2006; García et al., 2018). Briefly, seeds were germinated in black peat and, when appropriate, seedlings were transferred to individual containers (70 mL volume) with complete nutrient solution continuously aerated. The nutrient solution without Fe had the following composition: macronutrients, 2 mM Ca(NO3)2, 0.75 mM K2SO4, 0.65 mM MgSO4, 0.5 mM KH2PO4; and micronutrients, 50 μM KCl, 10 μM H3BO3, 1 μM MnSO4, 0.5 μM CuSO4, 0.5 μM ZnSO4, 0.05 μM (NH4)6Mo7O24. Fe-EDDHA was added to the nutrient solution at different concentrations (10 or 40 μM Fe-EDDHA) depending on the experiments. Plants were grown in a growth chamber at 22°C day/20°C night, with relative humidity between 50 and 70%, and an 8 h photoperiod (to postpone flowering) at a photosynthetic irradiance of 300 μMol m−2 s−1 provided by white fluorescent light (10,000 lux).
The treatments imposed were: Fe40: nutrient solution with 40 μM Fe-EDDHA; Fe40 + FeSO4: Fe40 treatment with FeSO4 application to leaves; Fe10: nutrient solution with 10 μM Fe-EDDHA; Fe10 + ACC1: Fe10 treatment with ACC addition, at 1 μM final concentration, during the last 6 or 24 h; -Fe: nutrient solution without Fe for 6, 12, 24, 48 or 72 h depending on the experiments; -Fe + Co: -Fe treatment 48 h with CoSO4 addition at 50, 75 or 100 μM final concentration, during the last 24 h; and-Fe + FeSO4: -Fe treatment during 2 days with FeSO4 application to leaves. For treatments with FeSO4, leaves were sprayed until total moistening once 24 h before harvest. FeSO4 was dissolved in deionized water (0.05% w/v) and Tween 20 was added as surfactant. After treatments, root ferric reductase activity (FRA) was determined as described in the next section. Finally, the roots were collected in liquid nitrogen and kept at −80°C to later analyze gene expression. Each treatment consists of six biological replications.
Ferric reductase activity determination
Ferric reductase activity (FRA) was determined as previously described (Lucena et al., 2006). In brief, intact plants were pretreated for 30 min in plastic vessels with 50 mL of a nutrient solution without micronutrients, pH 5.5, and then placed into 20 mL of a Fe (III) reduction assay solution for 1 h. This assay solution consisted of nutrient solution without micronutrients, 100 μM Fe(III)-EDTA and 300 μM Ferrozine, pH 5.0 (adjusted with 0.1 N KOH). The environmental conditions during the measurement of Fe (III) reduction were the same as the growth conditions described above. FRA was determined spectrophotometrically by measuring the absorbance (562 nm) of the Fe(II)-Ferrozine complex and by using an extinction coefficient of 29,800 M−1 cm−1. After the reduction assay, roots were excised and weighed, and the results were expressed on a root fresh weight basis. The values represent the mean ± SE of six replicates.
Real-time PCR analysis
PCR analysis was performed as previously described (García et al., 2018). In brief, once total RNA was extracted using the Tri Reagent solution (Molecular Research Center, Inc., Cincinnati, OH, United States), cDNA synthesis was done by using M-MLV reverse transcriptase (Promega, Madison, WI, United States) from 3 μg of DNase-treated root RNA as the template and random hexamers as the primers. The gene expression study by qRT-PCR was performed in a qRT-PCR Bio-Rad CFX connect thermal cycler and the following amplification profile: initial denaturation and polymerase activation (95°C for 3 min), amplification and quantification repeated 40 times (90°C for 10 s, 57°C for 15 s and 72°C for 30 s), and a final melting curve stage of 65 to 95°C with increment of 0.5°C for 5 s, to ensure the absence of primer dimer or non-specific amplification products. PCR reactions were set up in 20 μl of SYBR Green Bio-RAD PCR Master Mix, following the manufacturer’s instructions. Controls containing water instead of cDNA were included to check for contamination in the reaction components. Primer pairs designed by García et al. (2018) were used to amplify FRO2, IRT1 and FIT cDNA. Standard dilution curves were performed for each primer pair to confirm appropriate efficiency of amplification (E = 100 ± 10%). IMA1, IMA2 and IMA3 genes were amplified by using the primers previously described by Hirayama et al. (2018). Constitutively expressed SAND1 and YLS8 genes, which do not respond to Fe supply (Han et al., 2013), were used as reference genes to normalize qRT-PCR results. The relative expression levels were calculated from the threshold cycles (Ct) values and the primer efficiencies by the Pfaffl method (Pfaffl, 2001). Each PCR analysis was conducted on three biological replicates (each biological replicate was the mixture of the roots of two plants) and each PCR reaction repeated twice.
Occurrence of ethylene-responsive cis-acting elements in the promoters of IMA genes
The determination of ethylene-responsive cis-acting elements in the promoters of IMA genes was performed using the PLACE (Plant Cis-acting Regulatory DNA Elements) database (Higo et al., 1999).1 The sequences of the promoter regions of the tested genes were obtained from the EnsemblPlant database2 and the TAIR database.3
Statistical analyses
All experiments were repeated at least twice and representative results are presented. The values of qRT-PCR represent the mean ± SE of three independent biological replicates. The values of FRA represent the mean ± SE of six replicates. Depending on the experiment, different tests were used. When comparing different treatments with a control (Figures 1–3), *, ** or *** indicate significant differences (p < 0.05, p < 0.01 or p < 0,001, respectively) using one-way analysis of variance (ANOVA) followed by a Dunnett’s test. In the foliar Fe and Co experiments (Figures 4, 5) different letters indicate significant differences (p < 0.05) using one-way analysis of variance (ANOVA) followed by a Tukey’s test. In ACC experiments (Figure 6) *, ** or *** indicates significant differences (p < 0.05, p < 0.01 or p < 0,001, respectively) in relation to their respective control (Fe10 6 h or Fe10 24 h), using a Student’s test t.
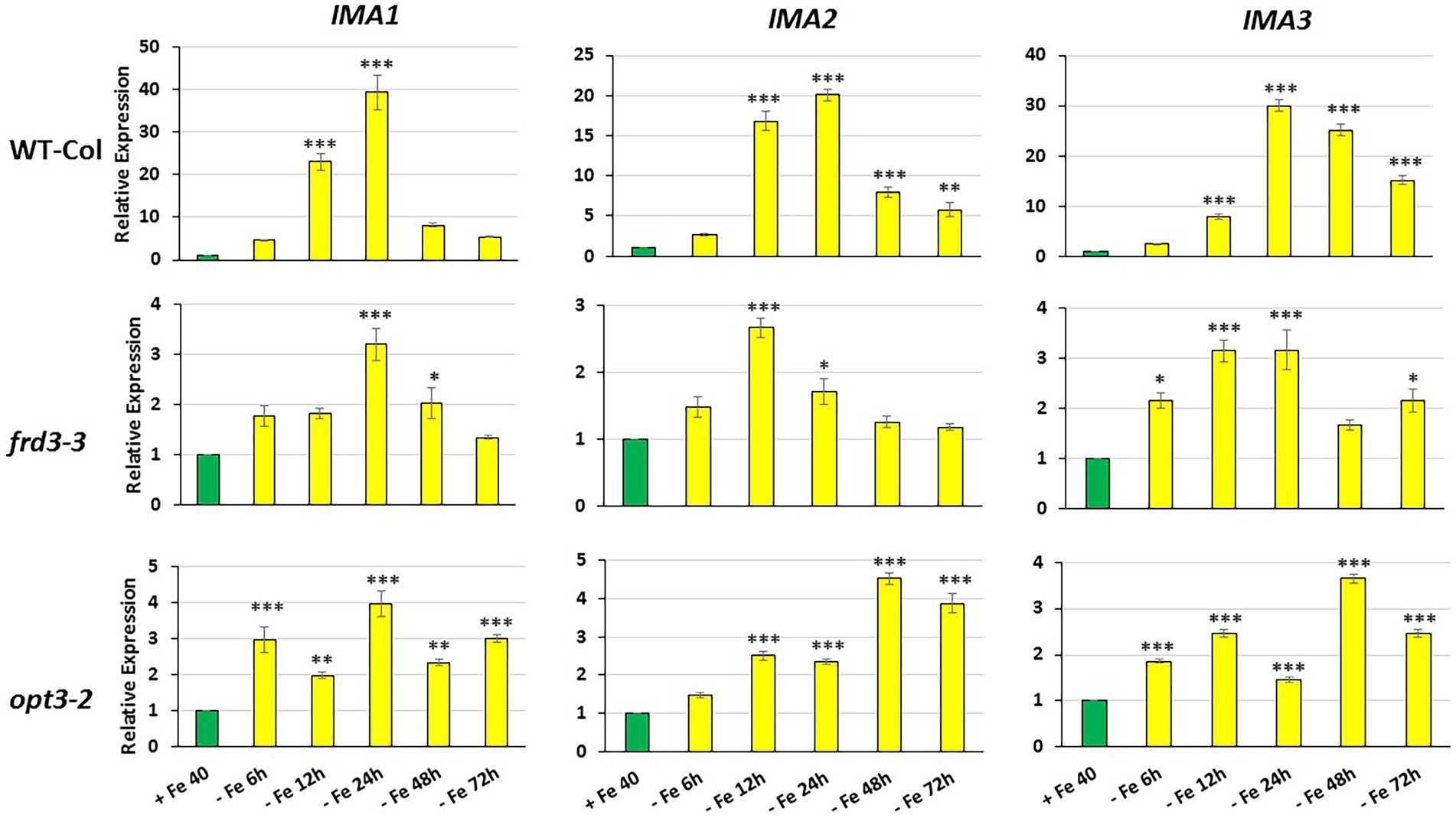
Figure 1. Time course of IMA1, IMA2 and IMA3 expression in roots of Arabidopsis WT Columbia and its LODIS defective mutants frd3-3 and opt3-2 grown under Fe-deficient conditions. Plants were grown in complete nutrient solution. When appropriate, some of them were transferred to complete nutrient solution with 40 μM Fe-EDDHA (+Fe40) or without Fe (–Fe) for 6, 12, 24, 48 or 72 h. IMA1, IMA2 and IMA3 relative expression was determined by qRT-PCR. Relative expression was calculated in relation to the +Fe40 treatment. Data represent the mean of 3 independent biological replicates ± S.E. Bars with *, ** or *** indicate significant differences (p < 0.05, p < 0.01 or p < 0.001, respectively) in relation to the +Fe40 treatment according to the Dunnett’s test.
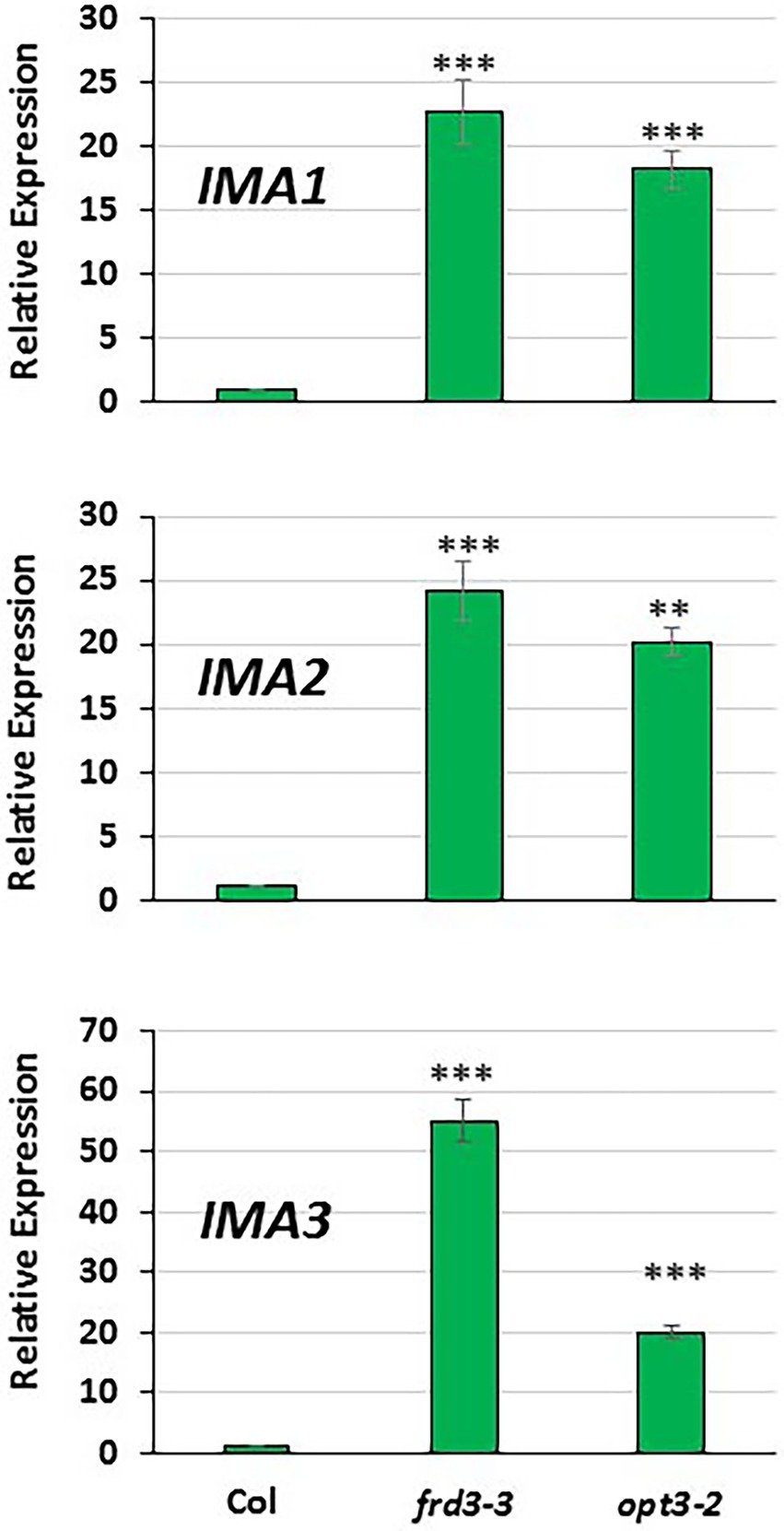
Figure 2. Comparison of IMA1, IMA2 and IMA3 expression in roots of Arabidopsis WT Columbia and its LODIS defective mutants frd3-3 and opt3-2 grown under Fe-sufficient conditions. Plants were grown in complete nutrient solution. When appropriate, were transferred to complete nutrient solution with 40 μM Fe-EDDHA (+Fe40) during 48 h. IMA1, IMA2 and IMA3 expression was determined by qRT-PCR. Relative expression was calculated in relation to the WT Columbia. Data represent the mean of 3 independent biological replicates ± S.E. Bars with ** or *** indicate significant differences (p < 0.01 or p < 0.001, respectively) in relation to the WT Colombia +Fe40 treatment according to the Dunnett’s test.
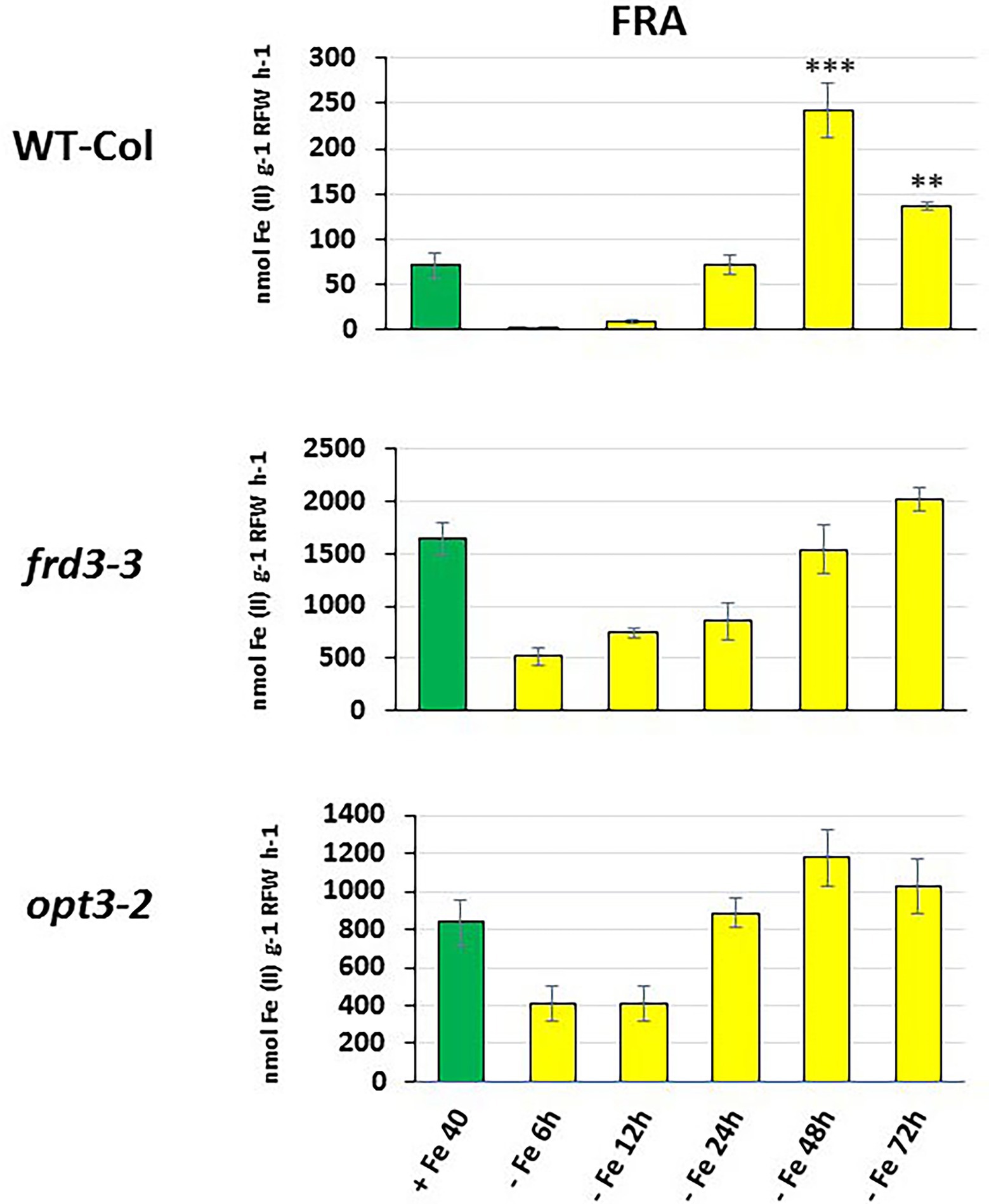
Figure 3. Time course of ferric reductase activity (FRA) in roots of Arabidopsis WT Columbia and its LODIS defective mutants frd3-3 and opt3-2 grown under Fe-deficient conditions. Treatments as in Figure 1. After treatments, FRA was determined along time. Data represent the mean of 6 replicates ± S.E. Bars with ** or *** indicate significant differences (p < 0.01 or p < 0.001, respectively) in relation to the +Fe40 treatment according to the Dunnett’s test.
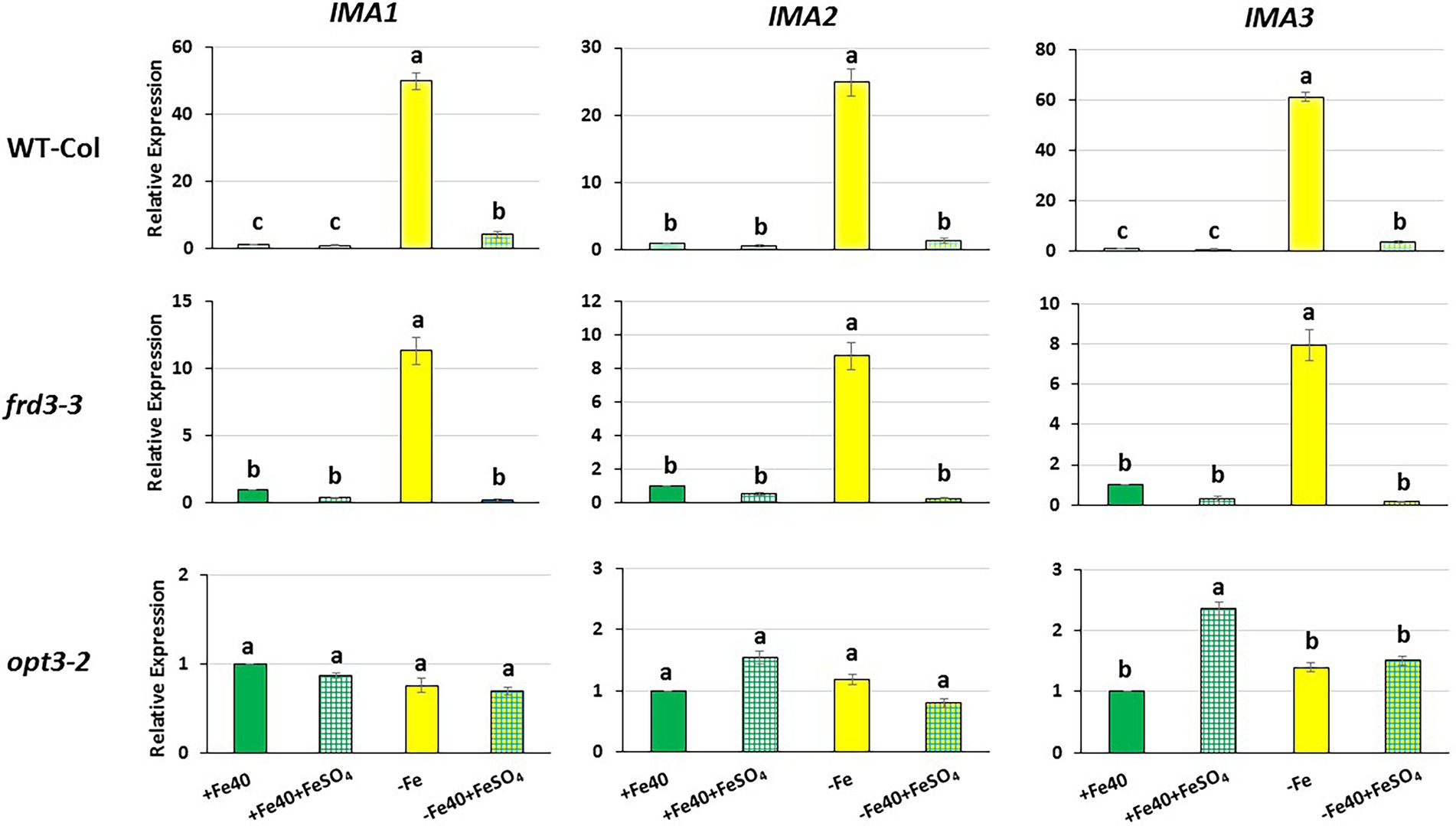
Figure 4. Effect of the foliar application of Fe on IMA1, IMA2 and IMA3 expression in roots of Arabidopsis WT Columbia and its LODIS defective mutants frd3-3 and opt3-2 grown under Fe-sufficient and Fe-deficient conditions. Plants were grown in complete nutrient solution. When appropriate, some of them were transferred to complete nutrient solution with 40 μM Fe-EDDHA (+Fe40) or without Fe (–Fe). 24 h later, half of the plants of each treatment (+Fe 40 and –Fe) were sprayed with FeSO4 (0.05% w/v) on their leaves (+Fe40 + FeSO4; –Fe + FeSO4). 24 h after the foliar treatments, roots were collected and IMA1, IMA2 and IMA3 expression was determined by qRT-PCR. Relative expression was calculated in relation to the +Fe40 treatment. Data represent the mean of 3 independent biological replicates ± S.E. Bars with different letters indicate significant differences (p < 0.05) using one-way analysis of variance (ANOVA) followed by a Tukey’s test.
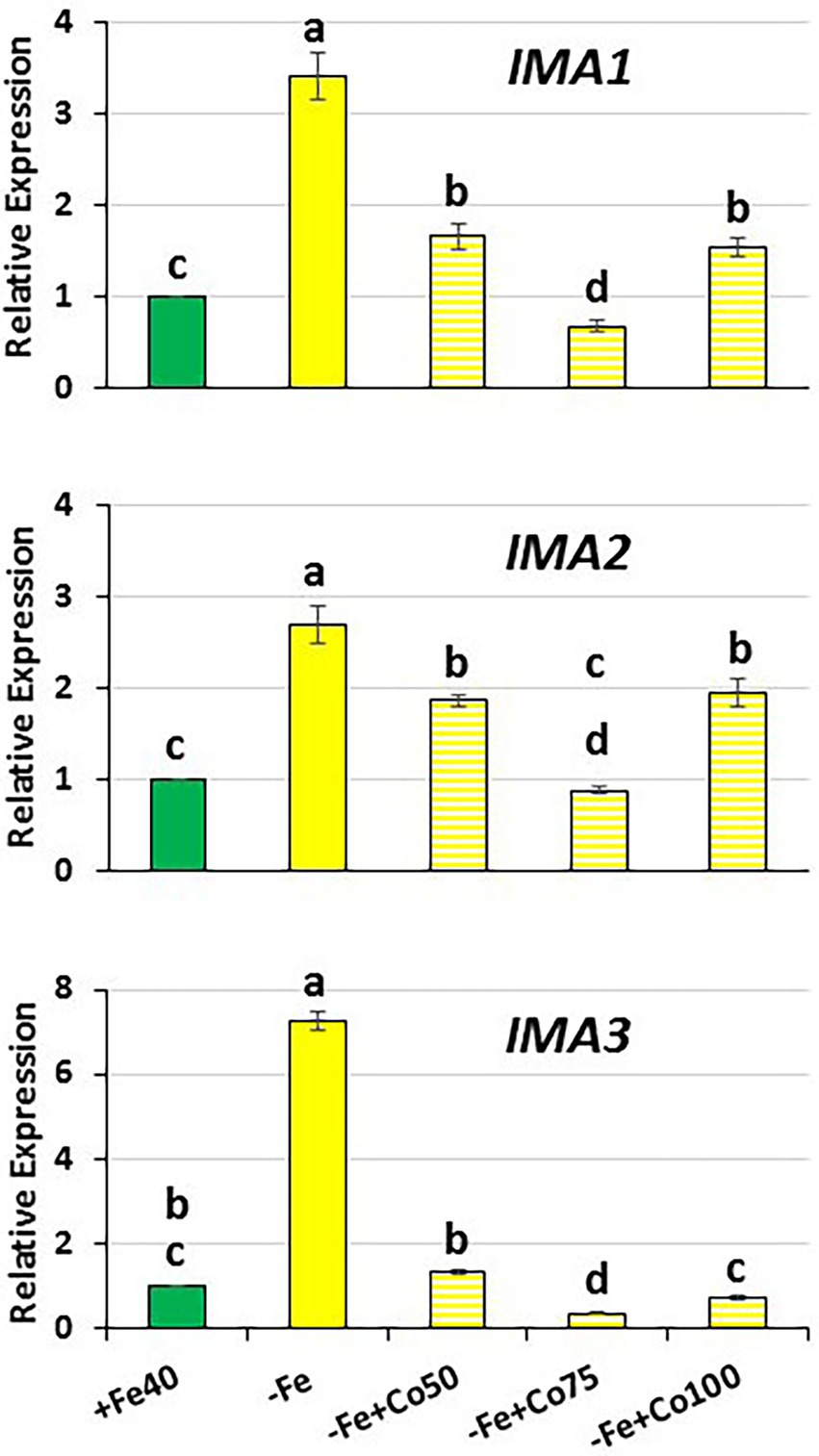
Figure 5. Effect of Fe deficiency and cobalt on IMA1, IMA2 and IMA3 expression in roots of Arabidopsis WT Columbia. Plants were grown in complete nutrient solution. When appropriate, some of them were transferred during 48 h to complete nutrient solution with 40 μM Fe-EDDHA (+Fe40) or without Fe (–Fe). Co, at different final concentrations (50, 75 or 100 μM), was added to the nutrient solution without Fe during the last 24 h. IMA1, IMA2 and IMA3 expression was determined by qRT-PCR. Relative expression was calculated in relation to the +Fe40 treatment. Data represent the mean of 3 independent biological replicates ± S.E. Bars with different letters indicate significant differences (p < 0.05) using one-way analysis of variance (ANOVA) followed by a Tukey’s test.
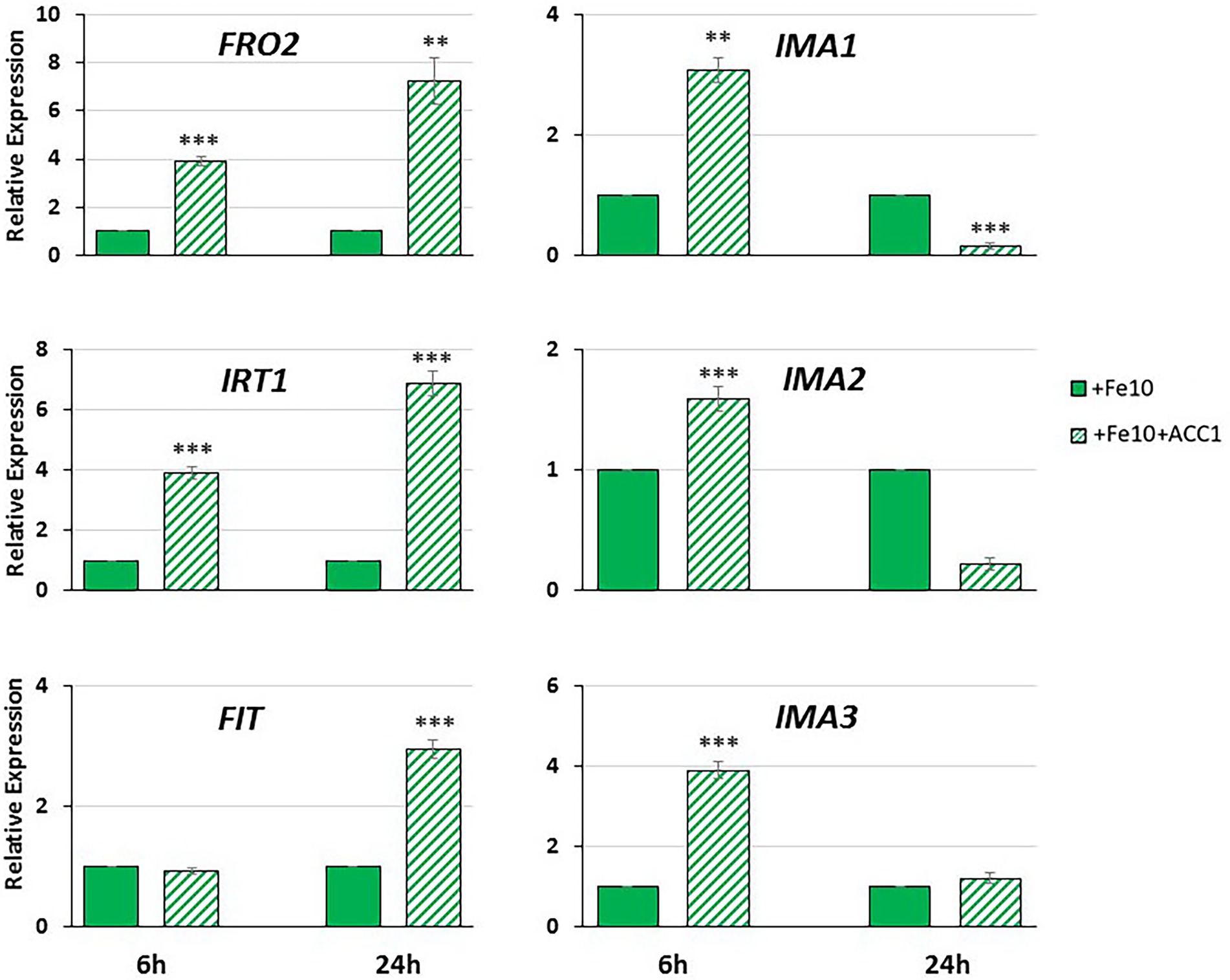
Figure 6. Effect of ACC treatment on the expression of the Fe acquisition genes FRO2, IRT1 and FIT, and of the IMA peptide genes IMA1, IMA2 and IMA3, in roots of Arabidopsis WT Columbia grown under Fe-sufficient conditions. Plants were grown in complete nutrient solution with 10 μM Fe-EDDHA (+Fe10). ACC at 1 μM, final concentration, was added to the nutrient solution of half of the plants during the last 6 h or 24 h. IMA1, IMA2 and IMA3 expression was determined by qRT-PCR. Relative expression was calculated in relation to the +Fe10 treatment. Data represent the mean of 3 independent biological replicates ± S.E. ** or *** indicates significant differences (p < 0.01 or p < 0,001, respectively) in relation to their respective control (+Fe10 6 h or + Fe10 24 h), using a Student’s test t.
Results
Relationship between LODIS and IMA peptides
To look further in the possible interaction between LODIS and IMA peptides, we carried out a time course experiment to compare the relative IMA1, IMA2 and IMA3 expression in Arabidopsis WT Columbia and LODIS-deficient mutants frd3-3 and opt3-2 plants subjected to Fe deficiency. Complementarily, we studied the effect of the foliar application of Fe on the relative IMA1, IMA2 and IMA3 expression in the WT Columbia and the two mutants named above.
As shown in Figure 1, the relative IMA1, IMA2 and IMA3 expression greatly increased in roots of WT Columbia plants after 12 h of Fe starvation and reached their maximum expression after 24 h of the deficiency. Expression levels were up to 20–30 fold higher in Fe-deficient conditions compared to the Fe-sufficient control (Figure 1). In the mutants frd3-3 and opt3-2, the differences observed in IMA expression under Fe-deficient conditions, relative to their control with Fe, were much lower (3–4 fold higher) than the observed in the WT Columbia (Figure 1). It should be noted that these mutants already display a constitutive Fe deficiency response and IMA expression under Fe-sufficient conditions could also be constitutively up-regulated. To confirm this possibility, we compared IMA expression under Fe-sufficient conditions between WT Columbia and the two mutants. Just as expected, we found that all the IMA genes were up-regulated in both the frd3-3 and opt3-2 mutants (Figure 2). These results would explain why both mutants present constitutively induced ferric reductase activity (Figure 3) and expression of the Fe acquisition genes FRO2, IRT1 and FIT (García et al., 2013, 2018), since all of them are up-regulated by the IMA peptides (Grillet et al., 2018).
Previous results of our group showed that the application of FeSO4 to leaves of Fe-deficient WT Columbia and frd3-3 plants greatly decreased their ferric reductase activity as well as the expression of the Fe acquisition genes FRO2, IRT1 and FIT (Lucena et al., 2006; García et al., 2013, 2018). However, the foliar FeSO4 treatment did not significantly affect either ferric reductase activity or gene expression in Arabidopsis opt3-2 plants (García et al., 2013), suggesting that the repressive “long-distance iron signal (LODIS)” can not move adequately from leaves to roots in the opt3 mutant (García et al., 2018).
In this work, we studied the effect of the foliar application of FeSO4 on IMA1, IMA2 and IMA3 expression in the WT Columbia and in its LODIS-deficient mutants frd3-3 and opt3-2. As occurred with the Fe acquisition genes (García et al., 2013), foliar application of Fe drastically inhibited IMA gene expression in WT and in frd3-3 mutant plants, not restricted in the movement of LODIS from shoots to roots (Figure 4). However, it had no effect on IMA gene expression in opt3-2 mutant plants, restricted in such a movement (Figure 4). These results clearly suggest that LODIS is involved in the regulation of IMA1, IMA2 and IMA3 expression.
Ethylene involvement in the regulation of IMA gene expression
To investigate the possible relationship between ethylene and IMA peptides, both of them positive regulators of Fe deficiency responses, we carried out several assays in which Arabidopsis WT Columbia plants were subjected to ACC (ethylene precursor) or CoSO4 (Co, an inhibitor of ethylene synthesis) treatments (Lucena et al., 2006).
As shown in Figure 6, ACC treatment had a positive effect in the expression of the Fe acquisition genes FRO2 and IRT1, at 6 and 24 h. However, in the case of FIT, the key TF that regulates FRO2 and IRT1, the induction of its expression could only be observed at 24 h after ACC treatment (Figure 6).
IMA1, IMA2 and IMA3 expression experimented a fast induction after 6 h of ACC treatment (Figure 6). However, the positive effect of ACC treatment on IMA expression disappeared after 24 h (Figure 6).
To further verify the possible role of ethylene in the regulation of IMA genes, we studied the effect of Co treatment on their expression in Fe-deficient WT Columbia plants. We found that Co inhibited IMA1, IMA2 and IMA3 expression at all doses used: 50, 75 and 100 μM (Figure 5). Taken together, all these results clearly show that ethylene could participate in the regulation of IMA1, IMA2 and IMA3 genes.
Occurrence of ethylene-responsive cis-acting elements in the promoters of IMA genes
The occurrence of ethylene-responsive cis-acting elements in the promoters of the IMA genes was investigated as additional evidence of their regulation by ethylene. The 8 bp sequence AWTTCAAA, is a well-known ethylene-responsive cis-acting element (ERELEE4 motif) that has been reported to mediate the ethylene regulation of genes for fruit ripening and leaf senescence (Montgomery et al., 1993; Itzhaki et al., 1994).
All eight Arabidopsis IMA genes, excepting IMA1, displayed at least one conserved ERELEE4 motif (Figure 7). Nevertheless, semi-conserved ERELEE4 motifs (one mismatch allowed) are present 11 times in the promoter of IMA1 (Figure 7).
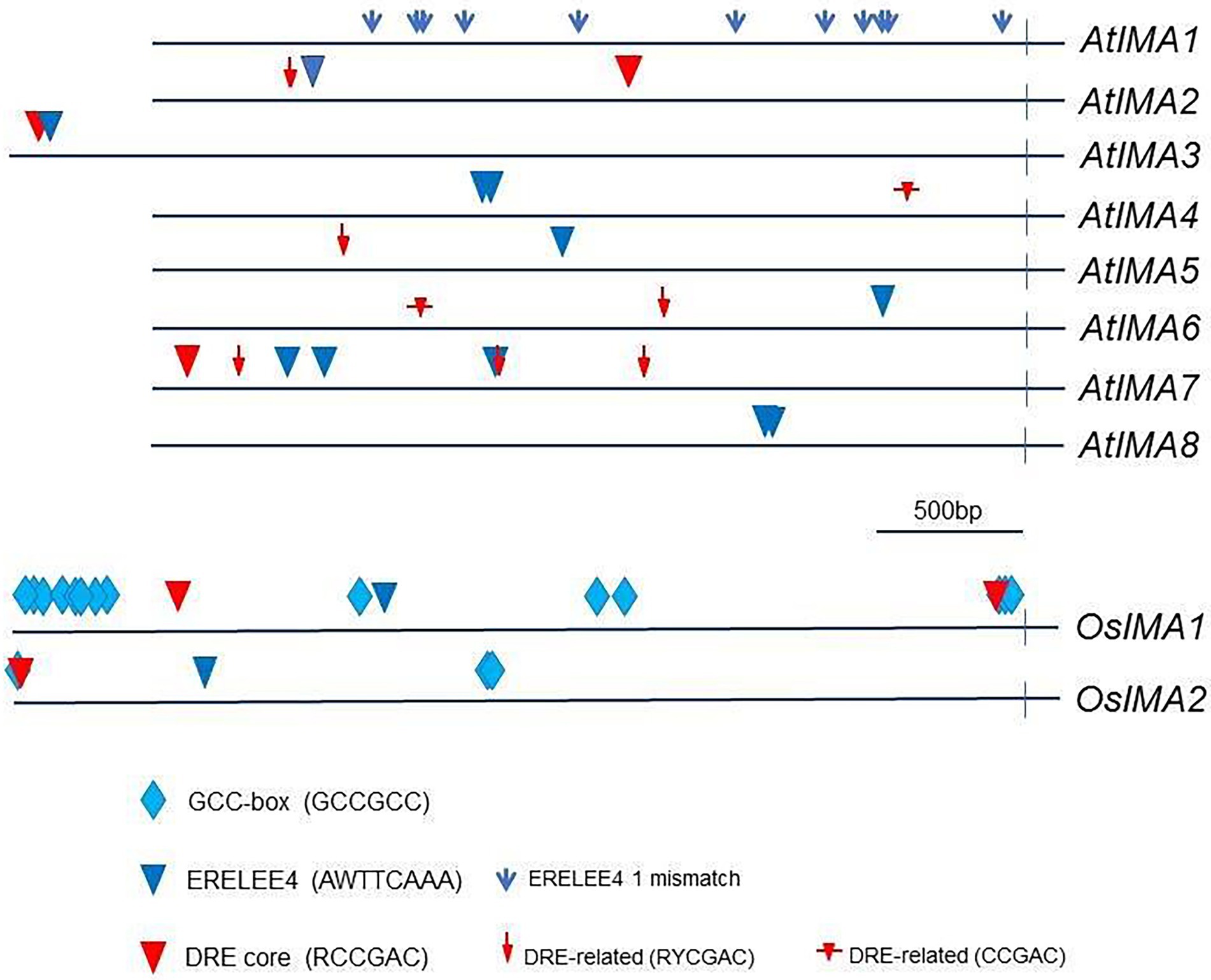
Figure 7. Location of ethylene-responsive motifs in the promoter regions of IMA genes. Ethylene-responsive motifs in the promoters of IMA genes were located by using the PLACE (Plant Cis-acting Regulatory DNA Elements) database. A stretch of 3,000 bp upstream of the transcription initiation site was examined in Arabidopsis (AtIMA) and in rice (OsIMA) sequences. For AtIMA3 and OsIMA1 and OsIMA2, the surveyed stretch was extended to 4,000 bp. The sequences of the promoter regions of the IMA genes were obtained from the EnsemblPlant and the TAIR database. AtIMA1 (AT1G47400), AtIMA2 (AT1G47395), AtIMA3 (AT2G30766), AtIMA4 (AT1G07367), AtIMA5 (AT1G09505), AtIMA6 (AT1G07373), AtIMA7 (AT2G00920), AtIMA8 (AT1G47401), OsIMA1 (Os01g0647200), OsIMA2 (Os07g0142100).
ERF1 is a transcription factor from the AP2/ERF family and is a fundamental part of the ethylene signaling pathway (Solano et al., 1998). The DRE core motif, RCCGAC, has been identified as a target sequence for ERF1 in Arabidopsis. By means of the DRE core motif, ethylene regulates gene expression in response to drought, salt, and thermal stresses (Cheng et al., 2013).
Cis-acting elements RYCGAC and CCGAC are close related to the DRE core (RCCGAC) and have also been identified as target sequences for AP2/ERF transcription factors (Hao et al., 2002; Sakuma et al., 2002; Xue, 2003; Lata and Prasad, 2011). Those DRE core-like sequences control gene expression in response to drought and cold in barley (Xue, 2003) and Arabidopsis (Sakuma et al., 2002; Lata and Prasad, 2011).
The DRE core motif and related elements were detected in all Arabidopsis IMA genes except in IMA1 and IMA8 (Figure 7). Moreover, six out of eight Arabidopsis IMA genes, including IMA2 and IMA3, contain both types of ethylene-responsive elements, ERELEE4 and DRE (Figure 7).
IMA orthologues in rice, OsIMA1 and OsIMA2, have been recently characterized by Kobayashi et al. (2021). Both genes are strongly induced under Fe deficiency and play key roles in enhancing the Fe deficiency responses. However, whether ethylene can regulate OsIMA1 or OsIMA2 expression or not, is yet unknown.
We have extended our identification of ethylene responsive cis-acting elements to OsIMA1 and OsIMA2. The promoters of each of them contain both ERELEE4 and DRE core motifs (Figure 7). Moreover, both OsIMA1 and OsIMA2 also display multiple copies of another well-characterized ethylene responsive cis-acting element called the GCC box (GCCGCC; Hao et al., 1998). This motif is especially abundant in OsIMA1, which contain 19 copies (Figure 7).
Discussion
IMA peptides were found few years ago in Arabidopsis and described as key positive regulators of Fe deficiency responses in Strategy I plants (Grillet et al., 2018; Hirayama et al., 2018). This finding has been further supported by other authors (Gautam et al., 2021; Li et al., 2021; Lichtblau et al., 2022), representing a great advance in the knowledge about the complex regulatory system of the Fe deficiency responses. Recently, the IMA peptides have also been described in rice (Kobayashi et al., 2021; Peng et al., 2022).
Previously to the discovery of IMA peptides, different hormones and signaling substances, such as ethylene, auxin, GSH and NO, have also been involved in the induction of Fe deficiency responses through the activation of FIT and other TFs (Lucena et al., 2006, 2015; Graziano and Lamattina, 2007; Waters et al., 2007; Chen et al., 2010; García et al., 2010, 2011, 2018; Lingam et al., 2011; Meiser et al., 2011; Romera et al., 2011, 2017; Koen et al., 2012; Yang et al., 2014; Shanmugam et al., 2015; Li and Lan, 2017). Besides these activating signals, inhibitory signals, like cytokinins and Fe itself, have also been described as necessary to switch off the responses once sufficient Fe has been acquired (Lucena et al., 2006; Séguéla et al., 2008; García et al., 2013, 2018). In relation to Fe itself, the repressing signal has been associated with some Fe compound circulating from shoots to roots through the phloem and related to the OPT3 transporter (García et al., 2013, 2018; Mendoza-Cózatl et al., 2014; Zhai et al., 2014). Probably, this Fe repressing signal is either a substrate of the OPT3 transporter or is derived from it (García et al., 2018). This long-distance inhibitory iron signal has been named “LODIS” and integrated with activating signals, such as ethylene, in a recent model about regulation of Fe deficiency responses (García et al., 2018). After the discovery of the IMA peptides, our group have been working in trying to fit them in the previous model (García et al., 2013, 2018). To this end, it is necessary to decipher the possible relationship between LODIS and IMA peptides, and between IMA peptides and ethylene.
To look further in the LODIS-IMA relationship, we carried out several experiments with the Arabidopsis WT Columbia and its LODIS defective mutants opt3-2 and frd3-3 (see Materials and Methods). First, we compared the expression profiles of IMA1, IMA2 and IMA3 in roots of WT Columbia and its two LODIS defective mutants subjected to Fe-deficient conditions. The results obtained showed a great increment of the expression of IMA genes in WT Columbia after 12 h of Fe deficiency, reaching their maximum expression level at 24 h, up to 30–40 fold (Figure 1). This increment was associated with an enhanced ferric reductase activity, one of the Fe deficiency responses and a target of IMA peptides (Grillet et al., 2018), at 48 h (Figure 3). On the other side, Fe deficiency did not induce either a great increase of the expression of IMA genes (only about 3–4 fold), nor of ferric reductase activity, in the frd3-3 and opt3-2 mutants (Figures 1, 3). These results could be explained by taking into account that the frd3-3 and opt3-2 mutants present constitutive expression of Fe deficiency responses (García et al., 2013, 2018), like the ferric reductase activity (Figure 3), and also constitutive expression of the IMA genes (Figure 2). In supporting these latter results, Chia et al. (2021) have also found up-regulation of IMA2 expression in Fe-sufficient roots of opt3-2 plants. Taking into account that frd3-3 and opt3-2 are LODIS defective mutants (see Material and Methods), the results depicted above suggest that LODIS is involved in the regulation of IMA expression. To further confirm the role of LODIS on IMA regulation, we performed several experiments in which WT Columbia, frd3-3 and opt3-2 plants were subjected to foliar Fe treatment, to provide leaves with enough LODIS to export to the roots. The results obtained showed that the induction of IMA expression was drastically abolished by the foliar Fe treatment in WT Columbia and in frd3-3 mutant plants grown under Fe-deficient conditions (Figure 4). This further supports an inhibition of IMA expression by LODIS. However, the foliar application of Fe had no effect in the mutant opt3-2 (Figure 4), just as occurred with Fe acquisition genes (FRO2, IRT1, and FIT) in previous works (García et al., 2013, 2018). It should be noted that the opt3-2 mutation impairs LODIS exportation from leaves to roots while the frd3-3 mutation does not (García et al., 2018). All these results clearly suggest that LODIS is involved in the negative regulation of IMA expression.
The second objective of this work was to determine the possible relationship between two activating signals of the Fe deficiency responses: IMA peptides and ethylene. Previous results of our group showed that ACC (ethylene precursor) requires low Fe concentration in the medium to activate Fe deficiency responses (Lucena et al., 2006). In this work, we have found that ACC application to WT Columbia plants grown on low Fe conditions (Fe 10 μM) had a positive effect on the Fe acquisition genes (FRO2 and IRT1) at both times studied (6 and 24 h; Figure 6). However, a significant induction of FIT expression could only be observed at 24 h after ACC treatment (Figure 6). The fact that FIT expression increase takes place later than that of their target genes FRO2 and IRT1 may seem puzzling. Nevertheless, Balparda et al. (2020) have recently proposed that FRO2 and IRT1 expression could be also directly controlled by the ERF1 TF (an essential part of the ethylene signaling) in a FIT independent way.
The expression of the IMA genes studied in this work increased in a significant manner 6 h after ACC treatment (Figure 6). This positive effect of ACC on the IMA expression disappeared quickly, in such a way that 24 h after ACC treatment no effect was observed (Figure 6). These results clearly suggest that ethylene positively regulates IMA expression, at least transitorily. To corroborate this, we further studied the expression of IMA genes in WT Columbia Fe-deficient plants subjected to an ethylene synthesis inhibitor, like cobalt (CoSO4; Lucena et al., 2006). The results showed that Co, at all doses used, inhibits the induction IMA expression in Fe-deficient plants (Figure 5), thus corroborating a possible role for ethylene in the up-regulation of IMA expression.
Finally, as and additional proof of ethylene involvement on IMA regulation, we investigated the occurrence of ethylene-responsive cis-acting elements in the promoters of IMA genes in both Arabidopsis and rice. Interestingly, the eight Arabidopsis IMA genes, excepting IMA1, displayed at least one conserved ERELEE4 motif (Figure 7). On the other side, in the promoter of IMA1, semi-conserved ERELEE4 motifs (only one mismatch allowed) are present 11 times (Figure 7). The ERELEE4 motif has also been found in the promoters of 14 Fe-related genes regulated by ethylene (García et al., 2010). Some of them are essential genes for the regulation of Fe deficiency responses (FIT, bHLH38), Fe acquisition (IRT1) and Fe distribution (FRD3, NAS1 and NAS2). The DRE core motif, RCCGAC, is another ethylene-responsive cis-acting element (Cheng et al., 2013). DRE core or related motifs occur in all Arabidopsis IMA genes but IMA1 and IMA8 (Figure 7). Moreover, six out of eight Arabidopsis IMA genes, including the highly expressed IMA2 and IMA3 (Grillet et al., 2018), contain both types of ethylene-responsive cis-acting elements, ERELEE4 and DRE core (Figure 7). Taken together, all these results clearly support a role for ethylene in the regulation of IMA expression and provide a way to know how ethylene could regulate them. It is interesting to consider that, since IMA genes seem to play redundant roles in triggering Fe deficiency responses (Grillet et al., 2018), the regulation by ethylene of just one of them, especially the highly expressed IMA1, IMA2 or IMA3, will be enough to establish a control by ethylene of the IMA gene function. Ethylene-responsive cis-acting elements are also found in the promoters of rice IMA genes (Figure 7), which further supports the role of ethylene in the regulation of IMA peptides.
In conclusion, the results obtained in this work suggest that LODIS could act upstream of IMA peptides and ethylene as depicted in the Working Model of Figure 8. According to this model, modification of a previous one presented by García et al. (2018), once Fe gets to shoots, LODIS is formed and moves to roots through the phloem. In roots, LODIS could negatively affect IMA expression and ethylene synthesis and signaling, either directly or through BTS. In a previous work, it was found that LODIS can affect ethylene production in roots since several Arabidopsis LODIS defective mutants, such as op3-2, frd3-3 and nas4x-1, present higher ACC (ethylene precursor) accumulation in Fe-sufficient roots than the WT (García et al., 2018). Since BTS is a putative sensor of Fe (probably of LODIS), then it is also possible that BTS could influence ethylene production. In supporting this view, it should be noted that the SAM1 gene, encoding a SAM synthetase (involved in ethylene synthesis), is up-regulated in the bts-3 mutant (Hindt et al., 2017). In addition, the phenotype of the bts-3 mutant could also be related to ethylene since it presents shorter roots and small shoots (Hindt et al., 2017), as plants treated with ethylene. Ethylene itself can also affect IMA expression as well as the expression of FIT and that of the bHLHs of the Ib subgroup (García et al., 2010; Lucena et al., 2015). Both IMAs and BTS interact to regulate IVc-bHLHs activity, which activate Ib-bHLHs and FIT, and consequently Fe acquisition genes (Li et al., 2021; Lichtblau et al., 2022).
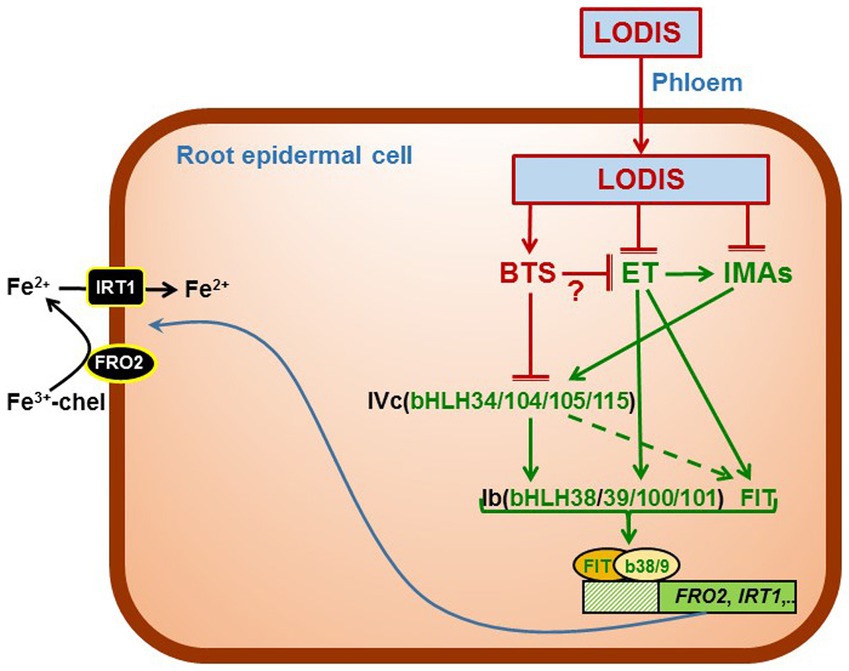
Figure 8. Working model to explain the role of LODIS, IMA peptides and ethylene on the regulation of Fe acquisition genes. Once in shoots, some Fe (either as free ions or in chelated form) can enter the phloem through the OPT3 transporter and move back to roots bound to a chelating agent (forming LODIS). In roots, LODIS could negatively affect IMA expression and ethylene synthesis and signaling, either directly or through BTS. Ethylene can affect IMA expression as well as the expression of FIT and Ib-bHLH expression. Both IMAs and BTS interact to regulate IVc-bHLH activity, which influence Ib-bHLHs and FIT and, consequently, Fe acquisition genes. ET (ethylene), IMAs (IMA peptides). (→: promotion; ⊥: inhibition).
This work has been focused in the study of the possible relationship between IMA peptides and other known signals implicated in the regulation of Fe deficiency responses, like LODIS and ethylene. Evidence for a role of LODIS and ethylene in the regulation of IMA1, IMA2 and IMA3 expression in Arabidopsis is presented. Nevertheless, there is still a long way to go. We have studied the effect of LODIS and ethylene on IMA1, IMA2, and IMA3 expression, but further studies are necessary to clarify other possibilities, like this one: is ethylene influenced by IMA peptides? Another open question to be solved is the possible relationship between NO and IMA peptides. Ethylene and NO act in conjunction to regulate Fe deficiency responses (García et al., 2011), hence it is possible that NO could also participate in IMA regulation.
Data availability statement
The datasets presented in this study can be found in online repositories. The names of the repository/repositories and accession number(s) can be found in the article.
Author contributions
MG and FR designed the experiments after discussions with RP-V. MG, MA, and CL conducted the laboratory work. MA drew the figures and did the statistical data analyses. RP-V did the search for DNA motifs in IMA genes. MG, FR, and RP-V wrote the manuscript which was improved by the other authors. All authors contributed to the article and approved the submitted version.
Funding
This work was supported by the European Regional Development Fund from the European Union, the ‘Ministerio de Economía y Competitividad’ (Project RTI2018-097935-B-I00), and the ‘Junta de Andalucía’ (Research Groups AGR115 and BIO159).
Acknowledgments
We acknowledge financial support from the Spanish Ministry of Science and Innovation, the Spanish State Research Agency, through the Severo Ochoa and María de Maeztu Program for Centers and Units of Excellence in R&D (Ref. CEX2019-000968-M).
Conflict of interest
The authors declare that the research was conducted in the absence of any commercial or financial relationships that could be construed as a potential conflict of interest.
Publisher’s note
All claims expressed in this article are solely those of the authors and do not necessarily represent those of their affiliated organizations, or those of the publisher, the editors and the reviewers. Any product that may be evaluated in this article, or claim that may be made by its manufacturer, is not guaranteed or endorsed by the publisher.
Footnotes
References
Bacaicoa, E., Mora, V., Zamarreño, Á. M., Fuentes, M., Casanova, E., and García-Mina, J. M. (2011). Auxin: a major player in the shoot-to-root regulation of root Fe-stress physiological responses to Fe deficiency in cucumber plants. Plant Physiol. Biochem. 49, 545–556. doi: 10.1016/j.plaphy.2011.02.018
Balparda, M., Armas, A. M., Estavillo, G. M., Roschzttardtz, H., Pagani, M. A., and Gomez-Casati, D. F. (2020). The PAP/SAL1 retrograde signaling pathway is involved in iron homeostasis. Plant Mol. Biol. 102, 323–337. doi: 10.1007/s11103-019-00950-7
Barberon, M., Vermeer, J. E. M., De Bellis, D., Wang, P., Naseer, S., Andersen, T. G., et al. (2016). Adaptation of root function by nutrient-induced plasticity of endodermal differentiation. Cells 164, 447–459. doi: 10.1016/j.cell.2015.12.021
Briat, J. F., Dubos, C., and Gaymard, F. (2015). Iron nutrition, biomass production, and plant product quality. Trends Plant Sci. 20, 33–40. doi: 10.1016/j.tplants.2014.07.005
Brumbarova, T., Bauer, P., and Ivanov, R. (2015). Molecular mechanisms governing Arabidopsis iron uptake. Trends Plant Sci. 20, 124–133. doi: 10.1016/j.tplants.2014.11.004
Chen, W. W., Yang, J. L., Qin, C., Jin, C. W., Mo, J. H., Ye, T., et al. (2010). Nitric oxide acts downstream of auxin to trigger root ferric-chelate reductase activity in response to iron deficiency in Arabidopsis. Plant Physiol. 154, 810–819. doi: 10.1104/pp.110.161109
Cheng, M. C., Liao, P. M., Kuo, W. W., and Lin, T. P. (2013). The Arabidopsis ETHYLENE RESPONSE FACTOR1 regulates abiotic stress-responsive gene expression by binding to different cis-acting elements in response to different stress signals. Plant Physiol. 162, 1566–1582. doi: 10.1104/pp.113.221911
Chia, J. C., Yan, J., Ishka, M. R., Faulkner, M. M., Huang, R., Smieska, L., et al. (2021). OPT3 plays a central role in both copper and iron homeostasis and signaling due to its ability to deliver copper as well as iron to the phloem in Arabidopsis. bioRxiv [Preprint]. doi: 10.1101/2021.07.30.454504
Colangelo, E. P., and Guerinot, M. L. (2004). The essential basic helix-loop-helix protein FIT1 is required for the iron deficiency response. Plant Cell 16, 3400–3412. doi: 10.1105/tpc.104.024315
Darbani, B., Briat, J. F., Holm, P. B., Husted, S., Noeparvar, S., and Borg, S. (2013). Dissecting plant iron homeostasis under short and long-term iron fluctuations. Biotech. Adv. 31, 1292–1307. doi: 10.1016/j.biotechadv.2013.05.003
Durrett, T. P., Gassmann, W., and Rogers, E. E. (2007). The FRD3-mediated efflux of citrate into the root vasculature is necessary for efficient iron translocation. Plant Physiol. 144, 197–205. doi: 10.1104/pp.107.097162
Gao, F., Robe, K., Bettembourg, M., Navarro, N., Rofidal, V., Santoni, V., et al. (2020). The transcription factor bHLH121 interacts with bHLH105 (ILR3) and its closest homologs to regulate iron homeostasis in Arabidopsis. Plant Cell 32, 508–524. doi: 10.1105/tpc.19.00541
Gao, F., Robe, K., Gaymard, F., Izquierdo, E., and Dubos, C. (2019). The transcriptional control of iron homeostasis in plants: a tale of bHLH transcription factors? Front. Plant Sci. 10:6. doi: 10.3389/fpls.2019.00006
García, M. J., Corpas, F. J., Lucena, C., Alcántara, E., Pérez-Vicente, R., Zamarreño, Á. M., et al. (2018). A shoot Fe signaling pathway requiring the OPT3 transporter controls GSNO Reductase and ethylene in Arabidopsis thaliana roots. Front. Plant Sci. 9:1325. doi: 10.3389/fpls.2018.01325
García, M. J., Lucena, C., Romera, F. J., Alcántara, E., and Pérez-Vicente, R. (2010). Ethylene and nitric oxide involvement in the up regulation of key genes related to iron acquisition and homeostasis in Arabidopsis. J. Exp. Bot. 61, 3885–3899. doi: 10.1093/jxb/erl189
García, M. J., Romera, F. J., Stacey, M. G., Stacey, G., Villar, E., Alcántara, E., et al. (2013). Shoot to root communication is necessary to control the expression of iron-acquisition genes in Strategy I plants. Planta 237, 65–75. doi: 10.1007/s00425-012-1757-0
García, M. J., Suárez, V., Romera, F. J., Alcántara, E., and Pérez-Vicente, R. (2011). A new model involving ethylene, nitric oxide and Fe to explain the regulation of Fe-acquisition genes in Strategy I plants. Plant Physiol. Biochem. 49, 537–544. doi: 10.1016/j.plaphy.2011.01.019
Gautam, C. K., Tsai, H. H., and Schmidt, W. (2021). IRONMAN tunes responses to iron deficiency in concert with environmental pH. Plant Physiol. 187, 1728–1745. doi: 10.1093/plphys/kiab329
Graziano, M., and Lamattina, L. (2007). Nitric oxide accumulation is required for molecular and physiological responses to iron deficiency in tomato roots. Plant J. 52, 949–960. doi: 10.1111/j.1365-313X.2007.03283.x
Grillet, L., Lan, P., Li, W., Mokkapati, G., and Schmidt, W. (2018). IRON MAN, a ubiquitous family of peptides that control iron transport in plants. Nat. Plants. 4, 953–963. doi: 10.1038/s41477-018-0266-y
Gutiérrez-Carbonell, E., Lattanzio, G., Albacete, A., Rios, J. J., Kehr, J., Abadía, A., et al. (2015). Effects of Fe deficiency on the protein profile of Brassica napus phloem sap. Proteomics 15, 3835–3853. doi: 10.1002/pmic.201400464
Han, B., Yang, Z., Samma, M. K., Wang, R., and Shen, W. (2013). Systematic validation of candidate reference genes for qRT-PCR normalization under iron deficiency in Arabidopsis. Biometals 26, 403–413. doi: 10.1007/s10534-013-9623-5
Hao, D., Ohme-Takagi, M., and Sarai, A. (1998). Unique mode of GCC box recognition by the DNA-binding domain of ethylene-responsive element-binding factor (ERF domain). Plant J. Biol. Chem. 273, 26857–26861. doi: 10.1074/jbc.273.41.26857
Hao, D., Yamasaki, K., Sarai, A., and Ohme-Takagi, M. (2002). Determinants in the sequence specific binding of two plant transcription factors, CBF1 and NtERF2, to the DRE and GCC motifs. Biochemist 41, 4202–4208. doi: 10.1021/bi015979v
Higo, K., Ugawa, Y., Iwamoto, M., and Korenaga, T. (1999). Plant cis-acting regulatory DNA elements (PLACE) database: 1999. Nucleic Acids Res. 27, 297–300. doi: 10.1093/nar/27.1.297
Hindt, M. N., Akmakjian, G. Z., Pivarski, K. L., Punshon, T., Baxter, I., Salt, D. E., et al. (2017). BRUTUS and its paralogs, BTS LIKE1 and BTS LIKE2, encode important negative regulators of the iron deficiency response in Arabidopsis thaliana. Metallomics 9, 876–890. doi: 10.1039/c7mt00152e
Hirayama, T., Lei, G. J., Yamaji, N., Nakagawa, N., and Ma, J. F. (2018). The putative peptide gene FEP1 regulates iron deficiency response in Arabidopsis. Plant Cell Physiol. 59, 1739–1752. doi: 10.1093/pcp/pcy145
Itzhaki, H., Maxson, J. M., and Woodson, W. R. (1994). An ethylene responsive enhancer element is involved in the senescence-related expression of the carnation glutathione-S-transferase (GSTI) gene. Proc. Natl. Acad. Sci. U. S. A. 91, 8925–8929. doi: 10.1073/pnas.91.19.8925
Kim, S. A., LaCroix, I. S., Gerber, S. A., and Guerinot, M. L. (2019). The iron deficiency response in Arabidopsis thaliana requires the phosphorylated transcription factor URI. Proc. Natl. Acad. Sci. U. S. A. 116, 24933–24942. doi: 10.1073/pnas.1916892116
Kobayashi, T., Nagano, A. J., and Nishizawa, N. K. (2021). Iron deficiency-inducible peptide-coding genes OsIMA1 and OsIMA2 positively regulate a major pathway of iron uptake and translocation in rice. J. Exp. Bot. 72, 2196–2211. doi: 10.1093/jxb/eraa546
Kobayashi, T., and Nishizawa, N. K. (2012). Iron uptake, translocation, and regulation in higher plants. Annu. Rev. Plant Biol. 63, 131–152. doi: 10.1146/annurev-arplant-042811-10552
Koen, E., Szymańska, K., Klinguer, A., Dobrowolska, G., Besson-Bard, A., and Wendehenne, D. (2012). Nitric oxide and glutathione impact the expression of iron uptake-and iron transport-related genes as well as the content of metals in A. thaliana plants grown under iron deficiency. Plant Signal. Behav. 7, 1246–1250. doi: 10.4161/psb.21548
Krüger, C., Berkowitz, O., Stephan, U. W., and Hell, R. (2002). A metal-binding member of the late embryogenesis abundant protein family transports iron in the phloem Ofricinus communis L. J. Biol. Chem. 277, 25062–25069. doi: 10.1074/jbc.M201896200
Lata, C., and Prasad, M. (2011). Role of DREBs in regulation of abiotic stress responses in plants. J. Exp. Bot. 62, 4731–4748. doi: 10.1093/jxb/err210
Li, W., and Lan, P. (2017). The understanding of the plant iron deficiency responses in Strategy I plants and the role of ethylene in this process by omic approaches. Front. Plant Sci. 8:40. doi: 10.3389/fpls.2017.00040
Li, Y., Lu, C. K., Li, C. Y., Lei, R. H., Pu, M. N., Zhao, J. H., et al. (2021). IRON MAN interacts with BRUTUS to maintain iron homeostasis in Arabidopsis. Proc. Natl. Acad. Sci. U. S. A. 118:39. doi: 10.1073/pnas.2109063118
Li, X., Zhang, H., Ai, Q., Liang, G., and Yu, D. (2016). Two bHLH transcription factors, bHLH34 and bHLH104, regulate iron homeostasis in Arabidopsis thaliana. Plant Physiol. 170, 2478–2493. doi: 10.1104/pp.15.01827
Liang, G., Zhang, H., Li, X., Ai, Q., and Yu, D. (2017). bHLH transcription factor bHLH115 regulates iron homeostasis in Arabidopsis thaliana. J. Exp. Bot. 68, 1743–1755. doi: 10.1093/jxb/erx043
Lichtblau, D. M., Schwarz, B., Baby, D., Endres, C., Sieberg, C., and Bauer, P. (2022). The iron deficiency-regulated small protein effector FEP3/IRON MAN1 modulates interaction of BRUTUS-LIKE1 with bHLH subgroup IVc and POPEYE transcription factors. Front. Plant Sci. 13:930049. doi: 10.3389/fpls.2022.930049
Ling, H. Q., Bauer, P., Bereczky, Z., Keller, B., and Ganal, M. (2002). The tomato fer gene encoding a bHLH protein controls iron-uptake responses in roots. Proc. Natl. Acad. Sci. U. S. A. 99, 13938–13943. doi: 10.1073/pnas.212448699
Lingam, S., Mohrbacher, J., Brumbarova, T., Potuschak, T., Fink-Straube, C., Blondet, E., et al. (2011). Interaction between the bHLH transcription factor FIT and the ETHYLENE INSENSITIVE3/ETHYLENE INSENSITIVE3-LIKE1 reveals molecular linkage between the regulation of iron acquisition and ethylene signaling in Arabidopsis. Plant Cell 23, 1815–1829. doi: 10.1105/tpc.111.084715
Lucena, C., Romera, F. J., García, M. J., Alcántara, E., and Pérez-Vicente, R. (2015). Ethylene participates in the regulation of Fe deficiency responses in Strategy I plants and in rice. Front. Plant Sci. 6:1056. doi: 10.3389/fpls.2015.01056
Lucena, C., Waters, B. M., Romera, F. J., García, M. J., Morales, M., Alcántara, E., et al. (2006). Ethylene could influence ferric reductase, iron transporter and H+-ATPase gene expression by affecting FER (or FER-like) gene activity. J. Exp. Bot. 57, 4145–4154. doi: 10.1093/jxb/erl189
Marschner, P. (2012). Marschner’s Mineral Nutrition of Higher Plants. 3rd Edn. London: Academic Press.
Meiser, J., Lingam, S., and Bauer, P. (2011). Posttranslational regulation of the iron deficiency basic helix-loop-helix transcription factor FIT is affected by iron and nitric oxide. Plant Physiol. 157, 2154–2166. doi: 10.1104/pp.111.183285
Mendoza-Cózatl, D. G., Xie, Q., Akmakjian, G. Z., Jobe, T. O., Patel, A., Stacey, M. G., et al. (2014). OPT3 is a component of the iron-signaling network between leaves and roots and misregulation of OPT3 leads to an over-accumulation of cadmium in seeds. Mol. Plant 7, 1455–1469. doi: 10.1093/mp/ssu067
Montgomery, J., Goldman, S., Deikman, J., Margossian, L., and Fischer, R. L. (1993). Identification of an ethylene-responsive region in the promoter of a fruit ripening gene. Proc. Natl. Acad. Sci. U. S. A. 90, 5939–5943. doi: 10.1073/pnas.90.13.5939
Peng, F., Li, C., Lu, C., Li, Y., Xu, P., and Liang, G. (2022). IRONMAN interacts with OsHRZ1 and OsHRZ2 to maintain Fe homeostasis. bioRxiv [Preprint]. doi: 10.1101/2022.03.11.483574
Pfaffl, M. W. (2001). A new mathematical model for relative quantification in real-time RT-PCR. Nucleic Acids Res. 29:e45. doi: 10.1093/nar/29.9.e45
Ramírez, L., Simontacchi, M., Murgia, I., Zabaleta, E., and Lamattina, L. (2011). Nitric oxide, nitrosyl iron complexes, ferritin and frataxin: a well-equipped team to preserve plant iron homeostasis. Plant Sci. 181, 582–592. doi: 10.1016/j.plantsci.2011.04.006
Rodríguez-Celma, J., Connorton, J. M., Kruse, I., Green, R. T., Franceschetti, M., Chen, Y. T., et al. (2019). Arabidopsis BRUTUS-LIKE E3 ligases negatively regulate iron uptake by targeting transcription factor FIT for recycling. Proc. Natl. Acad. Sci. U. S. A. 116:17584–17591. doi: 10.1073/pnas.1907971116
Rogers, E. E., and Guerinot, M. L. (2002). FRD3, a member of the multidrug and toxin efflux family, controls iron deficiency responses in Arabidopsis. Plant Cell 14, 1787–1799. doi: 10.1105/tpc.001495
Romera, F. J., García, M. J., Alcántara, E., and Pérez-Vicente, R. (2011). Latest findings about the interplay or auxin, ethylene and nitric oxide in the regulation of Fe deficiency responses by Strategy I plants. Plant Signal. Behav. 6, 167–170. doi: 10.4161/psb.6.1.14111
Romera, F. J., Lucena, C., García, M. J., Alcántara, E., and Pérez-Vicente, R. (2017). “The role of ethylene and other signals in the regulation of Fe deficiency responses by dicot plants,” in Stress Signaling in Plants: Genomics and Proteomics Perspectives. Vol. 2. ed. M. Sarwat, (Dordrecht: Springer), 277–300.
Sakuma, Y., Liu, Q., Dubouzet, J. G., Abe, H., Shinozaki, K., and Yamaguchi-Shinozaki, K. (2002). DNA-binding specificity of the ERF/AP2 domain of Arabidopsis DREBs, transcription factors involved in dehydration- and cold-inducible gene expression. Biochem. Biophys. Res. Commun. 290, 998–1009. doi: 10.1006/bbrc.2001.6299
Séguéla, M., Briat, J. F., Vert, G., and Curie, C. (2008). Cytokinins negatively regulate the root iron uptake machinery in Arabidopsis through a growth-dependent pathway. Plant J. 55, 289–300. doi: 10.1111/j.1365-313X.2008.03502.x
Shanmugam, V., Wang, Y. W., Tsednee, M., Karunakaran, K., and Yeh, K. C. (2015). Glutathione plays an essential role in nitric oxide-mediated iron-deficiency signaling and iron-deficiency tolerance in Arabidopsis. Plant J. 84, 464–477. doi: 10.1111/tpj.13011
Solano, R., Stepanova, A., Chao, Q., and Ecker, J. R. (1998). Nuclear events in ethylene signaling: a transcriptional cascade mediated by ETHYLENEINSENSITIVE3 and ETHYLENE-RESPONSE-FACTOR1. Genes Dev. 12, 3703–3714. doi: 10.1101/gad.12.23.3703
Stacey, M. G., Patel, A., McClain, W. E., Mathieu, M., Remley, M., Rogers, E. E., et al. (2008). The Arabidopsis AtOPT3 protein functions in metal homeostasis and movement of iron to developing seeds. Plant Physiol. 146, 589–601. doi: 10.1104/pp.107.108183
Wang, N., Cui, Y., Liu, Y., Fan, H., Du, J., Huang, Z., et al. (2013). Requirement and functional redundancy of Ib subgroup bHLH proteins for iron deficiency responses and uptake in Arabidopsis thaliana. Mol. Plant 6, 503–513. doi: 10.1093/mp/sss089
Waters, B. M., Lucena, C., Romera, F. J., Jester, G. G., Wynn, A. N., Rojas, C. L., et al. (2007). Ethylene involvement in the regulation of the H+-ATPase CsHA1 gene and of the new isolated ferric reductase CsFRO1 and iron transporter CsIRT1 genes in cucumber plants. Plant Physiol. Biochem. 45, 293–301. doi: 10.1016/j.plaphy.2007.03.011
Xue, G. P. (2003). The DNA-binding activity of an AP2 transcriptional activator HvCBF2 involved in regulation of low-temperature responsive genes in barley is modulated by temperature. Plant J. 33, 373–383. doi: 10.1046/j.1365-313X.2003.01630.x
Yang, Y., Ou, B., Zhang, J., Si, W., Gu, H., Qin, G., et al. (2014). The Arabidopsis mediator subunit MED16 regulates iron homeostasis by associating with EIN3/EIL1 through subunit MED25. Plant J. 77, 838–851. doi: 10.1111/tpj.12440
Yuan, Y., Wu, H., Wang, N., Li, J., Zhao, W., Du, J., et al. (2008). FIT interacts with AtbHLH38 and AtbHLH39 in regulating iron uptake gene expression for iron homeostasis in Arabidopsis. Cell Res. 18, 385–397. doi: 10.1038/cr.2008.26
Zhai, Z., Gayomba, S. R., Jung, H. I., Vimalakumari, N. K., Piñeros, M., Craft, E., et al. (2014). OPT3 is a phloem-specific iron transporter that is essential for systemic iron signaling and redistribution of iron and cadmium in Arabidopsis. Plant Cell 26, 2249–2264. doi: 10.1105/tpc.114.123737
Keywords: dicotyledonous, ethylene, Fe deficiency responses, IMA peptides, Long Distance Iron Signal (LODIS), Strategy I plants
Citation: García MJ, Angulo M, Romera FJ, Lucena C and Pérez-Vicente R (2022) A shoot derived long distance iron signal may act upstream of the IMA peptides in the regulation of Fe deficiency responses in Arabidopsis thaliana roots. Front. Plant Sci. 13:971773. doi: 10.3389/fpls.2022.971773
Edited by:
Peng Wang, Humboldt University of Berlin, GermanyReviewed by:
José López-Bucio, Universidad Michoacana de San Nicolás de Hidalgo, MexicoOlena Vatamaniuk, Cornell University, United States
Copyright © 2022 García, Angulo, Romera, Lucena and Pérez-Vicente. This is an open-access article distributed under the terms of the Creative Commons Attribution License (CC BY). The use, distribution or reproduction in other forums is permitted, provided the original author(s) and the copyright owner(s) are credited and that the original publication in this journal is cited, in accordance with accepted academic practice. No use, distribution or reproduction is permitted which does not comply with these terms.
*Correspondence: Francisco Javier Romera, YWcxcm9ydWZAdWNvLmVz