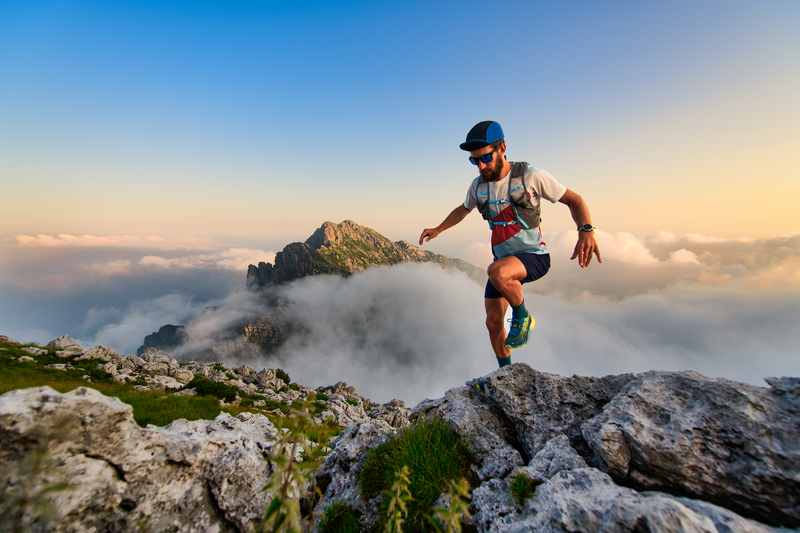
94% of researchers rate our articles as excellent or good
Learn more about the work of our research integrity team to safeguard the quality of each article we publish.
Find out more
ORIGINAL RESEARCH article
Front. Plant Sci. , 01 August 2022
Sec. Crop and Product Physiology
Volume 13 - 2022 | https://doi.org/10.3389/fpls.2022.967031
This article is part of the Research Topic Physiological, Molecular and Genetic Mechanisms of Abiotic Stress Tolerance in Tropical Crops View all 8 articles
Phytohormone auxin plays a vital role in plant development and responses to environmental stresses. The spatial and temporal distribution of auxin mainly relies on the polar distribution of the PIN-FORMED (PIN) auxin efflux carriers. In this study, we dissected the functions of OsPIN9, a monocot-specific auxin efflux carrier gene, in modulating chilling tolerance in rice. The results showed that OsPIN9 expression was dramatically and rapidly suppressed by chilling stress (4°C) in rice seedlings. The homozygous ospin9 mutants were generated by CRISPR/Cas9 technology and employed for further research. ospin9 mutant roots and shoots were less sensitive to 1-naphthaleneacetic acid (NAA) and N-1-naphthylphthalamic acid (NPA), indicating the disturbance of auxin homeostasis in the ospin9 mutants. The chilling tolerance assay showed that ospin9 mutants were more tolerant to chilling stress than wild-type (WT) plants, as evidenced by increased survival rate, decreased membrane permeability, and reduced lipid peroxidation. However, the expression of well-known C-REPEAT BINDING FACTOR (CBF)/DEHYDRATION-RESPONSIVE ELEMENT-BINDING PROTEIN 1 (DREB)-dependent transcriptional regulatory pathway and Ca2+ signaling genes was significantly induced only under normal conditions, implying that defense responses in ospin9 mutants have probably been triggered in advance under normal conditions. Histochemical staining of reactive oxygen species (ROS) by 3′3-diaminobenzidine (DAB) and nitroblue tetrazolium (NBT) showed that ospin9 mutants accumulated more ROS than WT at the early stage of chilling stress, while less ROS was observed at the later stage of chilling treatment in ospin9 mutants. Consistently, antioxidant enzyme activity, including catalase (CAT), peroxidase (POD), and superoxide dismutase (SOD), improved significantly during the early chilling treatments, while was kept similar to WT at the later stage of chilling treatment, implying that the enhanced chilling tolerance of ospin9 mutants is mainly attributed to the earlier induction of ROS and the improved ROS scavenging ability at the subsequent stages of chilling treatment. In summary, our results strongly suggest that the OsPIN9 gene regulates chilling tolerance by modulating ROS homeostasis in rice.
Due to their sessile nature, plants have to cope with various abiotic stresses, such as chilling stress. Chilling stress affects plant growth and development and restricts geographical distribution (Shi et al., 2018; Ding et al., 2020). Rice (Oryza sativa L.) is sensitive to chilling stress (Wang et al., 2003), and extreme temperature is a crucial factor limiting rice plant distribution and impairing rice quality and yield (Imin et al., 2004; Zeng et al., 2017). Therefore, improving rice chilling tolerance for maintaining rice yield is an urgent target by molecular genetic tools (Ma et al., 2015).
In recent years, the mechanism of plant adaption to cold stress has been extensively studied. Ca2+ influx, triggered by chilling stress, plays a crucial role in inducing temperature-responsive gene expression (Knight et al., 1996; Gong et al., 1998; Zhu, 2016; Ding et al., 2020). COLD1, a G-protein regulator, interacts with RGA1 (RICE G-PROTEIN α SUBUNIT 1) to activate the Ca2+ channel to improve chilling tolerance (Ma et al., 2015). Additionally, CBL-INTERACTING PROTEIN KINASE 7 (OsCIPK7) and CYCLIC NUCLEOTIDE-GATED CHANNELS 9 (OsCNGC9) were also shown to mediate Ca2+ influx under chilling stress in rice (Zhang et al., 2019a; Wang et al., 2021b). Growing evidence shows that the well-known C-REPEAT BINDING FACTOR (CBF)/DEHYDRATION-RESPONSIVE ELEMENT-BINDING PROTEIN 1 (DREB)-dependent transcriptional regulatory genes are dramatically induced by low temperature and may play a vital role in plant chilling tolerance (Stockinger et al., 1997; Liu et al., 1998; Ding et al., 2019; Sun et al., 2021), whereas reports also showed that there is no direct connection between the transcriptional abundance of these transcription factors and the degree of cold tolerance (Mao and Chen, 2012; Zhao et al., 2015; Zhang et al., 2016). By contrast, reactive oxygen species (ROS) homeostasis, including ROS induction and scavenging, was demonstrated to play a crucial role in chilling tolerance (Lee et al., 2002; Dong et al., 2009; Du et al., 2013b; Zhang et al., 2016).
The phytohormone auxin (indole-3-acetic acid, IAA) is essential in many plant developmental processes, including organ formation, vascular differentiation, apical dominance, flowering, and many other physiological processes (Benkova et al., 2003; Friml, 2003; Mattsson et al., 2003; Leyser, 2006; Zhao et al., 2013). In addition, auxin also participates in response to many environmental signals, such as light, gravity, and cold (Friml, 2003; Kimura and Kagawa, 2006; Palme et al., 2006; Shibasaki et al., 2009; Du et al., 2013a). Auxin mainly synthesizes in aerial organs (Woodward and Bartel, 2005; Zhao, 2008, 2010), such as leaf primordial and young leaves, and is transported basipetally by several transporters, which at least including the AUXIN1 (AUX1)/LIKE AUX1 (LAX) influx carriers, the PHOSPHOGLYCOPROTEIN (PGP/MDR/ABCB) efflux/influx transporters, and the PIN-FORMED (PIN) auxin efflux carriers (Michniewicz et al., 2007). Among which, PIN family proteins were reported to play a vital role in this process (Petrasek et al., 2006).
Although auxin has been proved to play an essential role in modulating almost all aspects of plant growth and development, its underlying mechanism under cold stress is elusive. Increasing evidence implied that cold stress is linked to auxin homeostasis. Transcript profiling showed that many auxin-responsive genes were differentially expressed under cold stress (Jain and Khurana, 2009), and cold treatment significantly increased IAA content in rice leaves (Du et al., 2013a). Auxin homeostasis is closely related to GH3 genes encoding auxin-conjugating enzymes. In Arabidopsis, overproduction of a GH3 gene WES1 by insertion mutation resulted in decreased auxin content and enhanced cold tolerance (Park et al., 2007). Consistently, overexpression of a rice GH3 family gene, OsGH3-2, and disruption of ABA and IAA biosynthesis gene also caused decreased IAA content and increased cold tolerance in rice (Du et al., 2012, 2013b), indicating that auxin homeostasis plays a potential role in regulating cold tolerance. In addition, auxin transport was shown to implicate in chilling response. An earlier report showed that the velocity of exogenous auxin transport was affected by temperature in some plant species (Morris, 1979). Cold treatment inhibited auxin transport basipetally, while room temperature restored it (Nadella et al., 2006). Further research demonstrated that cold stress affected auxin homeostasis by inhibition of intracellular trafficking of auxin efflux carriers (Shibasaki et al., 2009), and GNOM, a SEC7 containing ARF-GEF, which links to endosomal trafficking of auxin efflux carriers, was reported to positively modulate cold tolerance in Arabidopsis (Ashraf and Rahman, 2019). Collectively, these reports demonstrated that auxin homeostasis and transport play a potential role in regulating plant chilling tolerance. However, there is limited information on the role of auxin homeostasis and transport in chilling stress and the underlying molecular mechanisms.
Previous reports have shown that there are 12 PIN genes in the rice genome according to their relationship with PIN proteins reported in Arabidopsis, among which, OsPIN9, OsPIN10a/3a, and OsPIN10b/3b were identified as three monocot-specific PIN genes (Wang et al., 2009). It was demonstrated that OsPIN9 is a plasma membrane protein and robustly expressed in the vascular tissue of the roots and junctions; modulating the expression of OsPIN9 can change the transport and distribution of auxin (Hou et al., 2021). Our previous research showed that mutation of OsPIN9 by CRISPR/Cas9 significantly decreased the shoot height and adventitious root number at the seedling stage (Wu et al., 2021). However, little is known about the role of OsPIN9 in chilling stress response and tolerance in plants. Here, we examined the function of OsPIN9 in modulating chilling tolerance by CRISPR/Cas9 technology. Our results showed that chilling stress quickly and dramatically decreased the expression of OsPIN9, and mutation of OsPIN9 led to enhancement of chilling tolerance in rice. The ROS, accumulated at the early chilling stage in ospin9 mutants, probably as a signal to trigger the antioxidant enzyme system to quench the subsequent oxidative burst at the later chilling stage and contribute to the improved chilling tolerance. These results proved that mutation of OsPIN9 can substantially improve chilling tolerance and shed light on the relationship between OsPIN9-mediated polar auxin transport and chilling tolerance in rice.
Rice (O. sativa L.) seeds of Nipponbare were sterilized with 3% NaOCl for 30 min, washed with distilled water for 3–5 times, and incubated in Petri dishes with wetted filter papers at 30°C for 2–3 days in the dark. Then, germinated seeds were transferred to Kimura B complete nutrient solution (Xu et al., 2006) under greenhouse conditions (temperature of 30/25°C (day/night), relative humidity 60%–80%, light intensity 300 μmol/m2/s, and photoperiod of 12 h day/12 h night).
Fourteen-day-old seedlings cultured in the Kimura B solution were employed for all stress treatments. For drought treatment, seedlings were treated with 20% PEG; for salt treatment, seedlings were cultured in 10 g/L (w/v) NaCl; for chilling treatment, seedlings were transferred to 4°C conditions for 4 or 6 days, and then transferred back to the greenhouse at 28°C and grow for another 4 days to analyze the recovery and survival rate. Leaves from Wild-type (WT) and ospin9 mutants were collected at the indicated time points and used for physiological analysis.
The specific primer pairs were designed for the quantitative RT-PCR (qRT-PCR) analysis (Supplementary Table 1). Total RNA isolation was performed using RNAiso Plus (TAKARA BIO INC). RNA concentration, purity, and integrity were assessed with a NanoDrop 2000 spectrophotometer (Thermo Fisher Scientific, United States), and electrophoretic separation in 1% agarose gel. cDNA synthesis was finished using HiScript III RT SuperMix for qRT-PCR (+gDNA wiper; Nanjing Vazyme Biotech Company, Ltd.). qRT-PCR was conducted using AceQ Universal SYBR qPCR Master Mix (Nanjing Vazyme Biotech Company, Ltd.) and Lightcycler® 96 system. Three biological replicates and three technical repetitions were carried out to detect the gene expression. The specificity of amplified PCR products was verified by melting curve analysis. The data were normalized to the amplification of the OsACTIN1 gene (Os03g0718100). For abiotic stress treatments, the expression level of the OsPIN9 gene in CK was defined as 1.
The gene-editing vector CRISPR-RICE was used for the creation of ospin9 mutants in our previous report (Wu et al., 2021). Three homozygous ospin9 mutants, ospin9-1 ospin9-2, and ospin9-3, were identified according to the previous report (He et al., 2019). For off-target analysis, a toolkit for CRISPR-based genome editing CRISPR-GE1 was employed to analyze the potential off-target sites, and the genotype of targeted mutation was confirmed by sequencing of the PCR products directly (Liu et al., 2015). All primers used in this study were listed in Supplementary Table 1.
Seven-day-old and 14-day-old seedlings were used for phenotype analysis at the seedling stage. The agronomic traits, such as plant height, tiller number, and panicle length, were collected at the mature stage. For NAA or NPA treatment, germinated seeds were transferred to a nutrient solution containing 0.1 μM NAA or 0.5 μM NPA for 7 days, and then, the shoot height and root length were analyzed. WT and ospin9 mutants cultured under normal conditions were used as a control in this study.
Trypan blue staining was performed according to Choi et al. (2007). Electrolyte conductivity and malondialdehyde (MDA) contents were measured as described previously (Hu et al., 2020). 3′3-diaminobenzidine (DAB) staining and nitroblue tetrazolium (NBT) staining were conducted as previously reported (Zhang et al., 2016). Catalase (CAT), peroxidase (POD), and superoxide dismutase (SOD) activities were measured by the methods of Wu et al. (2003). Protein content was tested by Coomassie Brilliant Blue G-250 staining method (Bradford, 1976).
All experiments were repeated at least three times with consistent results. All data were given as means ± SD and analyzed by one-way analysis of variance (ANOVA) method in GraphPad PRISM (8.0.2) at the significance levels of p < 0.05 (*), p < 0.01 (**) and p < 0.001 (***).
To dissect the role of OsPIN9 in abiotic stress, we performed qRT-PCR to analyze the expression of OsPIN9 under drought, salt, and chilling treatments. OsPIN9, which showed a similar expression profile under drought and salt treatments, was firstly induced greatly and then repressed rapidly in rice roots (Figures 1A,B). In contrast to drought and salt treatments, OsPIN9 transcriptional abundance dramatically decreased by about 67% in rice roots after being transferred to chilling conditions for 3 h, and the abundance progressively decreased by about 90% after chilling for 12 h (Figure 1C). To determine whether OsPIN9 is an early chilling-regulated gene, we further investigated the expression of OsPIN9 at a shorter chilling treatment time. The time-course analyses of chilling treatment indicated that chilling treatment rapidly repressed the expression of OsPIN9 even after being transferred to chilling conditions only for half an hour (Figure 1D). These results indicate that OsPIN9 is rapidly inhibited by chilling treatment, implying a potential role of OsPIN9 in regulating chilling tolerance.
Figure 1. Expression profile analysis of OsPIN9 under various abiotic stresses. (A) 20% PEG; (B) 10 g/L NaCl; (C) 4°C treated for 0–12 h. (D) 4°C treated for 0–3 h.
To investigate the function of OsPIN9, we have employed the CRISPR/Cas9 technology to generate two ospin9 mutants, ospin9-1 and ospin9-2 (Wu et al., 2021). The identities of amino acids of OsPIN9 proteins in these two mutants predicted based on the nucleotide sequences was up to 99% and only one amino acid is different (Wu et al., 2021). To confirm that the phenotype of ospin9 mutants was indeed caused by the mutation of OsPIN9, we further obtained another ospin9 homozygous mutant (ospin9-3), which contained an A base insertion at the 18th base of the target sequence and caused premature termination of translation (Figure 2). ospin9-3 owned a shorter OsPIN9 protein (39 aa) compared with ospin9-1 and ospin9-2 (172 aa). The potential off-target sequence of OsPIN9 was further predicted in the rice genome and no nucleotide change was detected (data not shown). The homozygous mutants of ospin9-1 and ospin9-3 were used for further experiments.
Figure 2. Generation of ospin9 mutants. (A) Gene structure of WT and ospin9 mutants. Blue box indicates the OsPIN9 gene region. (B) One base-pair nucleotide A was inserted in the ospin9-3 mutant.
The previous study showed that mutation of OsPIN9 affects rice architecture (Hou et al., 2021). We evaluated the phenotype of ospin9-1 and ospin9-3 at the seedling stage. Consistent with previous report (Hou et al., 2021), mutation of OsPIN9 significantly decreased shoot height, root length, and adventitious root number at the seedling stage (Supplementary Figure 1), suggesting that OsPIN9 plays a vital role in regulating plant architecture.
1-Naphthaleneacetic acid can enter into cells by passive diffusion and be employed to study the sensitivity to auxin in various auxin-related mutants (Delbarre et al., 1996; Li et al., 2019). N-1-naphthylphthalamic acid is a classical inhibitor of polar auxin transport in plants (Abas et al., 2021). We assayed the effects of mutation of OsPIN9 on auxin homeostasis with NAA or NPA treatment in WT and ospin9 mutants. Germinated seeds were transferred to NAA or NPA solution for 7 days, and then, the shoot height and root length were assessed. 1-Naphthaleneacetic acid and NPA treatment significantly increased the primary root length and shoot height of ospin9 mutants compared with WT plants, respectively (Figure 3), indicating that the roots and shoots of ospin9 were less sensitive to NAA and NPA treatment, respectively. These results demonstrate that auxin homeostasis in ospin9 mutants is disrupted.
Figure 3. Response of WT and ospin9 mutants to NAA and NPA treatment. (A) Phenotype of WT and ospin9 mutants under normal, NAA or NPA treatment. Bar = 5 cm. (B) Shoot height and root length of WT and ospin9 mutants under normal, NAA or NPA treatment. Values are means ± standard deviation (SD; n = 15). Data were analyzed by ANOVA and Tukey’s tests at p < 0.05 significant level.
Since OsPIN9 was inhibited quickly by chilling treatment (Figures 1C,D), the role of OsPIN9 in chilling tolerance was evaluated in ospin9 mutants and WT plants. After chilling for 4 days, almost all of the leaves of WT plants showed rolling and wilting, while part of ospin9 leaves remained normal (Figure 4A), and ospin9-1 and ospin9-3 showed 62.5% and 67.4% survival rates, respectively, compared to 31.3% in WT plants after another 4 days of recovery at 28°C (Figure 4B). After 6 days of chilling treatment, there was no visual difference between WT and ospin9 mutant leaves (Figure 4C), and the survival rate of ospin9 mutants was still remarkably higher than that of WT after another 4 days of recovery (Figure 4D). These results suggest that OsPIN9 is involved in the modulation of chilling stress alleviation.
Figure 4. Mutation of OsPIN9 enhanced chilling tolerance in rice. (A) Phenotype of WT and ospin9 mutants before chilling treatment, after chilling treatment for 4 days and recovery for another 4 days. Bar = 4 cm. (B) Statistical presentation of survival rate after chilling 4 days and recovery for another 4 days. (C) Phenotype of WT and ospin9 mutants before chilling treatment, after chilling treatment for 6 days and recovery for another 4 days. Bar = 4 cm. (D) Statistical presentation of survival rate after chilling 6 days and recovery for another 4 days. Values are means ± standard deviation (SD; n ≥ 30). Data were analyzed by ANOVA and Tukey’s tests at p < 0.05 significant level.
Cold stress causes cell damage (Ding et al., 2019). We further evaluated the cell damage by trypan blue staining for cell death, electrolyte leakage, and MDA contents for membrane integrity. There was no apparent difference in trypan blue staining between WT and ospin9 mutant leaves under normal growth conditions, while staining with trypan blue was more intense in leaves from WT plants compared to the ospin9 mutants after chilling for 4 days (Figure 5A), indicating that the cell death in ospin9 is less than that in WT plants. Cold stress often damages cell membranes, and electrolyte leakage is usually a representative of damage to cell membranes by cold stress (Du et al., 2012); therefore, electrolyte leakage was assessed. The electrolyte leakage of ospin9 mutants was consistent with WT before chilling treatment. However, it was significantly lower in ospin9 mutants than that in WT plants after chilling for 4 days (Figure 5B). Malondialdehyde contents, derived from lipid peroxidation of polyunsaturated fatty acid, were similar to WT before chilling treatment while markedly decreased in ospin9 leaves than WT plants after chilling treatment (Figure 5C). Taken together, these results strongly demonstrate that ospin9 mutants are more resistant to chilling stress than WT plants and the mutation of OsPIN9 contributes to the enhanced chilling tolerance in ospin9 mutants.
Figure 5. Mutation of OsPIN9 altered cell damage, cell membrane stability and lipid peroxidation in rice seedling leaves. (A) Trypan blue staining; Bar = 1 mm. (B) Electrolyte leakage; (C) Malondialdehyde contents. The samples were taken randomly from the leaves of at least six plants of each independent (WT, ospin9-1, and ospin9-3) line after different time points of chilling stress. Values are means ± standard deviation (SD; n = 6). Data were analyzed by ANOVA and Tukey’s tests at p < 0.05 significant level.
Over the past two decades, a series of components have been identified in cold-stress signaling pathways, such as the well-known CBF/DREB regulon and Ca2+ signaling (Zhang et al., 2016; Ding et al., 2020). To further investigate the underlying mechanism of OsPIN9 in chilling stress, we firstly performed qRT-PCR to evaluate whether the CBF/DREB genes were related to the improved chilling tolerance in ospin9 mutants. OsDREB1A, OsDREB1B, and OsDREB1C expressions were analyzed due to the early induction by cold in rice (Dubouzet et al., 2003; Mao and Chen, 2012). Under normal conditions, these three OsDREB1 genes in ospin9 mutants showed a significantly higher accumulation than WT plants, while the induction level of these OsDREB1 genes in ospin9 mutants changed to a similar even lower level compared to WT plants after chilling for 8 h (Figure 6A). In addition, it was demonstrated that MITOGEN-ACTIVATED PROTEIN KINASE3 (OsMAPK3) phosphorylates OsbHLH002/INDUCER of CBF EXPRESSION1 (OsICE1) and promotes TREHALOSE-6-PHOSPHATE PHOSPHATASE1 (OsTPP1) expression to improve chilling tolerance in rice (Zhang et al., 2017). Consistent with the expression of OsDREB1 genes, OsTPP1 was markedly induced under normal conditions rather than under chilling conditions (Figure 6B). Besides the well-known CBF/DREB regulon, Ca2+ signaling-related genes, such as COLD1 and OsCNGC9, also play a vital role in regulating plant cold tolerance (Ma et al., 2015; Wang et al., 2021b). Similar to the expression of OsDREB1 and OsTPP1 genes, the expression of COLD1 and OsCNGC9 was also significantly increased in ospin9 mutants compared with WT plants under normal conditions. After being transferred to low-temperature conditions for 8 h, the expression showed a similar or decreased level than that in WT plants (Figure 6C). Collectively, these results indicated that mutation of OsPIN9 probably triggers the induction of plant defense response in advance under normal conditions, which may contribute to the enhanced chilling tolerance under chilling stress, while these genes did not show a striking increase after chilling for 8 h, implying that CBF/DREB regulon might play a minor role in modulating ospin9 chilling tolerance under chilling conditions. Considering that Ca2+ signaling plays a role during short-term cold treatments (Ma et al., 2015; Wang et al., 2021b), the role of these genes in regulating ospin9 chilling responses still needs further investigation.
Figure 6. OsDREB1 genes, OsTPP1, and Ca2+ signaling genes expression under normal and chilling conditions in WT and ospin9 mutants. (A) OsDREB1s gene expression analysis. (B) OsTPP1 expression analysis. (C) Ca2+ signaling genes expression analysis. Values were means ± standard deviation (SD; n = 3). Data were analyzed by ANOVA and Tukey’s tests at p < 0.05 significant level.
Cold stress induces ROS production (Zhang et al., 2016; Guo et al., 2018). Low-level ROS at the early stress stage can be signaled to trigger stress-responsive activities (Zhang et al., 2019b), so we investigated the ROS content by DAB staining for hydrogen peroxide (H2O2) and NBT staining for superoxide anion radicals ( ) under chilling conditions. After chilling for 36 h, the ospin9 leaves accumulated more ROS than WT (Figure 7A). In contrast, after chilling for 72 h, strong ROS accumulation was only observed in WT plants, whereas feeble levels of ROS were detected in ospin9 mutants (Figure 7B). The antioxidant enzyme system, such as SOD, CAT, and POD, has been proved to function in quenching ROS under various adverse conditions (Jaleel et al., 2009; Shah et al., 2021; Song et al., 2021). We then analyzed the activity of these three enzymes under normal and chilling conditions. The results showed that the activity of CAT, POD, and SOD was comparable with WT plants under normal conditions (Figure 7C), while it was significantly higher than that of WT plants after chilling for 24 h (Figure 7D), and after chilling for 36 h, SOD activity was comparable with WT, while the activity of CAT and SOD was still noticeably higher than that of WT (Figure 7E). This increase was completely abolished after chilling for 72 h between ospin9 mutants and WT plants (Figure 7F). These results indicate that ROS homeostasis, including ROS production and scavenging, plays a vital role in modulating chilling tolerance of ospin9 mutants.
Figure 7. ROS production and scavenging in WT and ospin9 mutants during chilling stress. (A) ROS accumulation in WT and ospin9 mutants was detected at 36 h with NBT and DAB staining. Bar = 1 mm. (B) DAB and NBT staining for WT and ospin9 mutants after chilling treatment for 72 h. Bar = 1 mm. (C–F) Antioxidant enzyme activities analysis after chilling for 0, 24, 36, and 72 h in WT and ospin9 mutants. Values were means ± standard deviation (SD; n = 6). Data were analyzed by ANOVA and Tukey’s tests at p < 0.05 significant level.
It was reported that NAA treatment can induce the production of H2O2, indicating that auxin probably plays a crucial role in ROS homeostasis (Du et al., 2012). Therefore, we detected the H2O2 content in WT plants under indole-3-acetic acid (IAA) treatment by DAB staining. As shown in Figure 8A, the application of 0.5 μM and 1 μM IAA obviously accelerated the production of H2O2. Since OsPIN9 functions in the transport of auxin from the main stem to the junction, and mutation of OsPIN9 causes the accumulation of auxin in the leaves (Hou et al., 2021), so we further detected the H2O2 content in ospin9 mutants under normal conditions. The results show that even under normal conditions, ospin9 leaves showed a slight accumulation of H2O2 than WT plants (Figure 8B).
Figure 8. Effects of auxin application on the production of H2O2 and the proposed model of OsPIN9-mediated chilling tolerance in rice. (A) H2O2 content analysis after auxin application. Bar = 1 mm. (B) H2O2 content in WT and ospin9 leaves under normal conditions. Bar = 1 mm. (C) Proposed model of OsPIN9-mediated chilling tolerance in rice.
Low temperature is a major factor affecting rice growth and development. Over the past decades, many genes have been identified and demonstrated to be functional in cold adaption. The phytohormones, such as brassinosteroid (BR), abscisic acid (ABA), ethylene, and jasmonic acid (JA) also play a critical role in plant cold tolerance (Ding et al., 2020). Auxin, the first hormone discovered in plants, is one of the most important compounds in modulating plant growth and development, its role in regulating plant chilling tolerance is still largely unknown. As one of the monocot-specific OsPIN genes, OsPIN9 is mainly expressed in adventitious root primordia and pericycle cells on stem bases (Wang et al., 2009; Miyashita et al., 2010), implying that OsPIN9 protein may function in regulating adventitious root development (Wang et al., 2009). Consistent with this speculation, Hou et al. (2021) reported that OsPIN9 is positively involved in regulating adventitious root number in rice, as well as rice tiller number (Hou et al., 2021). Additionally, OsPIN9 was reported to respond to heavy metal stresses, such as arsenic (Singh et al., 2017) and cadmium stress (Wang et al., 2021a). Despite recent progress in elucidating the biological function of OsPIN9, it is still at a beginning stage, especially for the function of OsPIN9 in the regulation of abiotic stresses. In this report, we revealed that OsPIN9 could be inhibited quickly by chilling stress and firstly demonstrated that OsPIN9 is involved in chilling stress alleviation in rice by CRISPR/Cas9 technology at the seedling stage.
Rice is mainly distributed in tropical and subtropical areas and is sensitive to chilling stress (Kovach et al., 2007; Sang and Ge, 2007; Saito et al., 2010; Ma et al., 2015). Chilling stress severely impacts rice growth and development, and severe chilling disasters significantly impair rice yield (Zhang et al., 2019b). Improving chilling tolerance has great potential for improving rice yield under low-temperature conditions (Zhang et al., 2019b). Previous studies have reported that auxin transport is associated with chilling stress (Nadella et al., 2006; Shibasaki et al., 2009; Du et al., 2013a; Ashraf and Rahman, 2019), but there was no report regarding the role of OsPIN proteins in the regulation of chilling stress. In the present study, we functionally characterized the role of OsPIN9 in chilling tolerance. Several lines of evidence support the result that ospin9 mutants are more tolerant to chilling stress than WT plants. First, the survival rate of ospin9 mutants was significantly higher than that of WT after chilling treatment (Figure 4). Second, as evidenced by trypan blue staining, the cell death in WT plants was more severe than that in ospin9 mutants under chilling treatment (Figure 5A). Third, the electrolyte leakage, an index of cell membrane integrity, was significantly lower in ospin9 mutants than in WT plants during chilling treatment (Figure 5B). Finally, MDA content, an indicator of lipid peroxidation, also showed a lower level in ospin9 mutants than in WT plants after chilling treatment (Figure 5C). Collectively, these results strongly demonstrate that mutation of OsPIN9 indeed increases the chilling tolerance in rice.
Plants have evolved multiple mechanisms to withstand chilling stress, among which cold-responsive transcription factors and their target genes, as well as ROS-dominated adaptation mechanism, have been demonstrated to play a critical role in the regulation of chilling stress (Yamaguchi-Shinozaki and Shinozaki, 2006; Zhang et al., 2016, 2019b). For example, it has been demonstrated that ICE1 and their target genes, such as CBF/DREB and TPP1, play a vital role in chilling resistance (Chinnusamy et al., 2003; Zhang et al., 2017). Although cold induces OsDREB1 homologs (Dubouzet et al., 2003; Mao and Chen, 2012), overexpressing OsDREB1A, OsDREB1B, and OsDREB1G enhanced cold tolerance (Dubouzet et al., 2003; Ito et al., 2006; Moon et al., 2019), other reports showed that the expression levels of these transcription factors were not in line with the degree of cold tolerance (Mao and Chen, 2012; Zhao et al., 2015; Zhang et al., 2016). Our results showed that the expressions of CBF/DREB (OsDREB1A, OsDREB1B, OsDREB1C), OsTPP1, and Ca2+ signaling-related genes (COLD1 and OsCNGC9) in ospin9 mutants were all significantly higher than that in WT plants under normal conditions (Figure 6), and ospin9 mutants accumulated more H2O2 than WT under normal conditions (Figure 8B). Since OsDREB1 homologs could be induced by various abiotic stresses (Dubouzet et al., 2003; Moon et al., 2019), and Ca2+ influx into the cytoplasm can be triggered by ROS (Kwak et al., 2003), it is reasonable to speculate that defense responses in ospin9 mutants have probably been triggered in advance even under normal conditions, and this probably partially alleviated the chilling stress in ospin9 mutants. By contrast, the expression of CBF/DREB genes and OsTPP1 in ospin9 mutants was similar to or even lower than that in WT under chilling stress (Figures 6A,B), indicating that the CBF/DREB regulon probably plays a minor role in improving chilling tolerance of ospin9 mutants, especially under chilling conditions. Although Ca2+ signaling genes COLD1 and OsCNGC9 were also decreased after chilling for 8 h in ospin9 mutants (Figure 6C), Ca2+ influx into the cytosol is an early signaling event in response to stress stimuli in plants, which can be activated within seconds or minutes (Ma et al., 2015; Wang et al., 2021b). So whether Ca2+ signaling contributes to the enhanced chilling tolerance in ospin9 mutants still needs further investigation.
Reactive oxygen species homeostasis is vital for plant growth and development. The plant needs to control ROS production whenever necessary precisely. Enzymatic and non-enzymatic antioxidant defense systems scavenge ROS and protect plant cells from oxidative damage (Mittler, 2002; Apel and Hirt, 2004). Chilling stress induces the production of H2O2 in the cell (Prasad et al., 1994b), and H2O2 level has dual effects during abiotic stress, i.e., low-level H2O2 at the early stress stage is considered as a signal to trigger stress-response, while high-level H2O2 at the later stress stage is considered as oxidative molecules to cause plant cell damage (Prasad et al., 1994b; Zhang et al., 2019b). The previous report showed that exogenous application of H2O2 can improve maize seedling chilling tolerance (Prasad et al., 1994a), and chilling acclimation indeed increased H2O2 content and chilling capacity in maize seedlings (Prasad et al., 1994b), indicating that proper H2O2 content can substantially enhance plant chilling tolerance. Consistent with this view, Zhang et al. (2016) demonstrated that japonica varieties with higher chilling tolerance accumulate more and less ROS at the middle and late chilling stages, respectively, while indica varieties which are more sensitive to chilling treatment performed inversely, indicating that ROS accumulation at different chilling stages plays different roles during chilling treatment. In line with these results, our study showed that ospin9 mutants accumulated more and less ROS than WT at the early (36 h) and later (72 h) chilling stages, respectively, which was similar to the observation in japonica and indica varieties. Furthermore, the antioxidant enzyme system, including CAT, POD, and SOD, was all significantly enhanced in ospin9 mutants than in WT plants at the early chilling stage (Figures 7D,E). Thus, we speculate that the ROS-dominated adaption mechanism plays a vital role in regulating ospin9 chilling tolerance. Whether selection has acted on OsPIN9, just like COLD1 (Ma et al., 2015), and contributed to the diversity of chilling tolerance between japonica and indica still needs further investigation.
Despite the crucial role of ROS homeostasis in modulating chilling tolerance in rice, the role of CBF/DREB regulon and Ca2+ signaling under chilling stress cannot be excluded in ospin9 mutants. Early signaling events, including cytosolic Ca2+ influx and burst of ROS, can be activated within a very short time (Baxter et al., 2014). The interconnection of Ca2+ signaling with ROS production complicates the underlying mechanism under different abiotic stresses in plants, which are intimately correlated with diverse abiotic stresses (Baxter et al., 2014; Dong et al., 2022). Previous data showed that Ca2+ signal formation and H2O2 accumulation are fine-tuned in a reciprocal manner (Steinhorst and Kudla, 2013). Ca2+ signaling genes were significantly induced under normal conditions in ospin9 mutants (Figure 6C), implying the probability of the enhanced cytosolic Ca2+ influx. Disruption of OsPIN9 obviously enhanced the accumulation of H2O2 in ospin9 mutant leaves (Figure 8B). The accumulated H2O2 and probably increased Ca2+ influx might trigger the defense response in advance in ospin9 mutants, as evidenced by the increased expression of OsDREB1s and OsTPP1 (Figures 6A,B), which may also contribute to the enhanced chilling tolerance in ospin9 mutants. While the interconnection of Ca2+ and H2O2 signaling involved in chilling stress response still needs further investigation.
Previously, we have reported that OsPIN9 is suppressed by chilling treatment, while induced by salt and drought treatments as well as ABA, gibberellin (GA), methyl jasmonate (MeJA), and salicylic acid (SA) treatments (Xu et al., 2022). Additionally, cis-element analysis of OsPIN9 promoter also showed that the OsPIN9 promoter contains many cis-elements that are predicted to be responsible to auxin, ABA, GA, MeJA, and SA (Xu et al., 2022), implying that OsPIN9 might have a role under diverse abiotic stresses and be implicated in the interconnection of diverse phytohormones by influencing auxin homeostasis.
Auxin transport from shoot to root is mainly mediated by OsPIN9 and mutation of OsPIN9 results in the accumulation of auxin in rice leaves (Hou et al., 2021). It is reasonable to speculate that more auxin is accumulated in ospin9 mutants, which was further verified by NAA and NPA treatment assay (Figure 3), and more H2O2 accumulation was induced by auxin in leaves (Figure 8A). We speculated that rice probably possesses a native PAT-related chilling tolerance mechanism (Figure 8C). In brief, OsPIN9 expression is quickly suppressed by low temperature (Figure 1), which results in the accumulation of auxin in leaves (Figure 3; Du et al., 2013a; Hou et al., 2021). The accumulated auxin promotes the production of H2O2 at the early chilling stress stage (Figure 7A), and H2O2 can be employed as a signal to trigger stress-responsive activities (Zhang et al., 2019b). After prolonged chilling treatment, improved antioxidant enzymes (Figures 7D,E) lead to reduced ROS (Figure 7B), which attenuates the damages caused by ROS and finally enhances chilling tolerance. Considering that mutation of OsPIN9 substantially perturbed auxin homeostasis, caused growth retardation (Supplementary Figure 1; Hou et al., 2021), as well as enhanced chilling tolerance, chilling-induced quickly suppression of OsPIN9 is likely to be an adaptive strategy that coordinately regulates growth rate and chilling tolerance in rice. In future, whether other cold-sensitive monocot plants, such as maize (Zea mays) and sorghum (Sorghum bicolor), also possess a similar chilling-resistant mechanism needs to be further investigated. Our results shed light on the relationship between PAT and chilling tolerance in rice.
Collectively, we investigated the role of OsPIN9 in the regulation of chilling stress in rice in the present study. Although ospin9 mutants show adverse effects on some agronomic traits, OsPIN9 substantially contributes rice to survival under low-temperature stress. Further, investigation on how OsPIN9 is rapidly inhibited after chilling treatment and ROS homeostasis is regulated by auxin homeostasis under normal and chilling conditions will improve our understanding of the mechanism of chilling stress in rice. Our discovery demonstrates that OsPIN9 expression is negatively regulated by chilling stress and OsPIN9 plays a critical role in chilling tolerance by modulating ROS homeostasis in rice.
The raw data supporting the conclusions of this article will be made available by the authors, without undue reservation.
HX and CZ designed the experiments. XY, YZ, HW, SW, ZZ, and HX carried out the experiments. HL, HX, and XY analyzed the data and took photographs. HX wrote the article. HL, GA, and CZ revised and approved the article. All authors contributed to the article and approved the submitted version.
This work was supported by the National Natural Science Foundation of China (3110019), the Natural Science Foundation of Henan province (182300410012), and the Student Research Training Program of Henan University of Science and Technology (2022452).
The authors thank Guozhang Kang (The National Key Laboratory of Wheat and Maize Crop Science, Henan Agricultural University) for constructive discussion.
The authors declare that the research was conducted in the absence of any commercial or financial relationships that could be construed as a potential conflict of interest.
All claims expressed in this article are solely those of the authors and do not necessarily represent those of their affiliated organizations, or those of the publisher, the editors and the reviewers. Any product that may be evaluated in this article, or claim that may be made by its manufacturer, is not guaranteed or endorsed by the publisher.
The Supplementary material for this article can be found online at: https://www.frontiersin.org/articles/10.3389/fpls.2022.967031/full#supplementary-material
Supplementary Figure 1 | Comparison of phenotype between WT and ospin9 mutants in rice at the seedling stage. (A) Phenotype of WT and ospin9 mutants after germination for 7 days. Bar = 3 cm. (B) Phenotype of WT and ospin9 mutants after germination for 14 days. Bar = 3 cm. Values are means ± standard deviation (SD; n = 12). Data were analyzed by ANOVA and Tukey’s tests at p < 0.05 significant level.
Abas, L., Kolb, M., Stadlmann, J., Janacek, D. P., Lukic, K., Schwechheimer, C., et al. (2021). Naphthylphthalamic acid associates with and inhibits PIN auxin transporters. Proc. Natl. Acad. Sci. U. S. A. 118:e2020857118. doi: 10.1073/pnas.2020857118
Apel, K., and Hirt, H. (2004). Reactive oxygen species: metabolism, oxidative stress, and signal transduction. Annu. Rev. Plant Biol. 55, 373–399. doi: 10.1146/annurev.arplant.55.031903.141701
Ashraf, M. A., and Rahman, A. (2019). Cold stress response in Arabidopsis thaliana is mediated by GNOM ARF-GEF. Plant J. 97, 500–516. doi: 10.1111/tpj.14137
Baxter, A., Mittler, R., and Suzuki, N. (2014). ROS as key players in plant stress signalling. J. Exp. Bot. 65, 1229–1240. doi: 10.1093/jxb/ert375
Benkova, E., Michniewicz, M., Sauer, M., Teichmann, T., Seifertova, D., Jurgens, G., et al. (2003). Local, efflux-dependent auxin gradients as a common module for plant organ formation. Cell 115, 591–602. doi: 10.1016/s0092-8674(03)00924-3
Bradford, M. M. (1976). A rapid and sensitive method for the quantitation of microgram quantities of protein utilizing the principle of protein–dye binding. Anal. Biochem. 72, 248–254. doi: 10.1006/abio.1976.9999
Chinnusamy, V., Ohta, M., Kanrar, S., Lee, B. H., Hong, X., Agarwal, M., et al. (2003). ICE1: a regulator of cold-induced transcriptome and freezing tolerance in Arabidopsis. Genes Dev. 17, 1043–1054. doi: 10.1101/gad.1077503
Choi, H. W., Kim, Y. J., Lee, S. C., Hong, J. K., and Hwang, B. K. (2007). Hydrogen peroxide generation by the pepper extracellular peroxidase CaPO2 activates local and systemic cell death and defense response to bacterial pathogens. Plant Physiol. 145, 890–904. doi: 10.1104/pp.107.103325
Delbarre, A., Muller, P., Imhoff, V., and Guern, J. (1996). Comparison of mechanisms controlling uptake and accumulation of 2,4-dichlorophenoxy acetic acid, naphthalene-1-acetic acid, and indole-3-acetic acid in suspension-cultured tobacco cells. Planta 198, 532–541. doi: 10.1007/BF00262639
Ding, Y., Shi, Y., and Yang, S. (2019). Advances and challenges in uncovering cold tolerance regulatory mechanisms in plants. New Phytol. 222, 1690–1704. doi: 10.1111/nph.15696
Ding, Y., Shi, Y., and Yang, S. (2020). Molecular regulation of plant responses to environmental temperatures. Mol. Plant 13, 544–564. doi: 10.1016/j.molp.2020.02.004
Dong, Q., Wallrad, L., Almutairi, B. O., and Kudla, J. (2022). Ca2+ signaling in plant responses to abiotic stresses. J. Integr. Plant Biol. 64, 287–300. doi: 10.1111/jipb.13228
Dong, C. H., Zolman, B. K., Bartel, B., Lee, B. H., Stevenson, B., Agarwal, M., et al. (2009). Disruption of Arabidopsis CHY1 reveals an important role of metabolic status in plant cold stress signaling. Mol. Plant 2, 59–72. doi: 10.1093/mp/ssn063
Du, H., Liu, H., and Xiong, L. (2013a). Endogenous auxin and jasmonic acid levels are differentially modulated by abiotic stresses in rice. Front. Plant Sci. 4:397. doi: 10.3389/fpls.2013.00397
Du, H., Wu, N., Chang, Y., Li, X., Xiao, J., and Xiong, L. (2013b). Carotenoid deficiency impairs ABA and IAA biosynthesis and differentially affects drought and cold tolerance in rice. Plant Mol. Biol. 83, 475–488. doi: 10.1007/s11103-013-0103-7
Du, H., Wu, N., Fu, J., Wang, S., Li, X., Xiao, J., et al. (2012). A GH3 family member, OsGH3-2, modulates auxin and abscisic acid levels and differentially affects drought and cold tolerance in rice. J. Exp. Bot. 63, 6467–6480. doi: 10.1093/jxb/ers300
Dubouzet, J. G., Sakuma, Y., Ito, Y., Kasuga, M., Dubouzet, E. G., Miura, S., et al. (2003). OsDREB genes in rice, O. sativa L., encode transcription activators that function in drought-, high-salt-and cold-responsive gene expression. Plant J. 33, 751–763. doi: 10.1046/j.1365-313x.2003.01661.x
Friml, J. (2003). Auxin transport – shaping the plant. Curr. Opin. Plant Biol. 6, 7–12. doi: 10.1016/s1369526602000031
Gong, M., van der Luit, A. H., Knight, M. R., and Trewavas, A. J. (1998). Heat-shock-induced changes in intracellular Ca2+ level in tobacco seedlings in relation to thermotolerance. Plant Physiol. 116, 429–437. doi: 10.1104/pp.116.1.429
Guo, X., Liu, D., and Chong, K. (2018). Cold signaling in plants: insights into mechanisms and regulation. J. Integr. Plant Biol. 60, 745–756. doi: 10.1111/jipb.12706
He, Y., Cheng, J., He, Y., Yang, B., Cheng, Y., Yang, C., et al. (2019). Influence of isopropylmalate synthase OsIPMS1 on seed vigour associated with amino acid and energy metabolism in rice. Plant Biotechnol. J. 17, 322–337. doi: 10.1111/pbi.12979
Hou, M., Luo, F., Wu, D., Zhang, X., Lou, M., Shen, D., et al. (2021). OsPIN9, an auxin efflux carrier, is required for the regulation of rice tiller bud outgrowth by ammonium. New Phytol. 229, 935–949. doi: 10.1111/nph.16901
Hu, Z., Huang, X., Amombo, E., Liu, A., Fan, J., Bi, A., et al. (2020). The ethylene responsive factor CdERF1 from bermudagrass (Cynodon dactylon) positively regulates cold tolerance. Plant Sci. 294:110432. doi: 10.1016/j.plantsci.2020.110432
Imin, N., Kerim, T., Rolfe, B. G., and Weinman, J. J. (2004). Effect of early cold stress on the maturation of rice anthers. Proteomics 4, 1873–1882. doi: 10.1002/pmic.200300738
Ito, Y., Katsura, K., Maruyama, K., Taji, T., Kobayashi, M., Seki, M., et al. (2006). Functional analysis of rice DREB1/CBF-type transcription factors involved in cold-responsive gene expression in transgenic rice. Plant Cell Physiol. 47, 141–153. doi: 10.1093/pcp/pci230
Jain, M., and Khurana, J. P. (2009). Transcript profiling reveals diverse roles of auxin-responsive genes during reproductive development and abiotic stress in rice. FEBS J. 276, 3148–3162. doi: 10.1111/j.1742-4658.2009.07033.x
Jaleel, C. A., Riadh, K., Gopi, R., Manivannan, P., Ines, J., Al-Juburi, H., et al. (2009). Antioxidant defense responses: physiological plasticity in higher plants under abiotic constraints. Acta Physiol. Plant. 31, 427–436. doi: 10.1007/s11738-009-0275-6
Kimura, M., and Kagawa, T. (2006). Phototropin and light-signaling in phototropism. Curr. Opin. Plant Biol. 9, 503–508. doi: 10.1016/j.pbi.2006.07.003
Knight, H., Trewavas, A. J., and Knight, M. R. (1996). Cold calcium signaling in Arabidopsis involves two cellular pools and a change in calcium signature after acclimation. Plant Cell 8, 489–503. doi: 10.1105/tpc.8.3.489
Kovach, M. J., Sweeney, M. T., and McCouch, S. R. (2007). New insights into the history of rice domestication. Trends Genet. 23, 578–587. doi: 10.1016/j.tig.2007.08.012
Kwak, J. M., Mori, I. C., Pei, Z. M., Leonhardt, N., Torres, M. A., Dangl, J. L., et al. (2003). NADPH oxidase AtrbohD and AtrbohF genes function in ROS-dependent ABA signaling in Arabidopsis. EMBO J. 22, 2623–2633. doi: 10.1093/emboj/cdg277
Lee, B. H., Lee, H., Xiong, L., and Zhu, J. K. (2002). A mitochondrial complex I defect impairs cold-regulated nuclear gene expression. Plant Cell 14, 1235–1251. doi: 10.1105/tpc.010433
Leyser, O. (2006). Dynamic integration of auxin transport and signalling. Curr. Biol. 16, R424–R433. doi: 10.1016/j.cub.2006.05.014
Li, Y., Zhu, J., Wu, L., Shao, Y., Wu, Y., and Mao, C. (2019). Functional divergence of PIN1 paralogous genes in rice. Plant Cell Physiol. 60, 2720–2732. doi: 10.1093/pcp/pcz159
Liu, Q., Kasuga, M., Sakuma, Y., Abe, H., Miura, S., Yamaguchi-Shinozaki, K., et al. (1998). Two transcription factors, DREB1 and DREB2, with an EREBP/AP2 DNA binding domain separate two cellular signal transduction pathways in drought-and low-temperature-responsive gene expression, respectively, in Arabidopsis. Plant Cell 10, 1391–1406. doi: 10.1105/tpc.10.8.1391
Liu, W., Xie, X., Ma, X., Li, J., Chen, J., and Liu, Y. G. (2015). DSDecode: a web-based tool for decoding of sequencing chromatograms for genotyping of targeted mutations. Mol. Plant 8, 1431–1433. doi: 10.1016/j.molp.2015.05.009
Ma, Y., Dai, X., Xu, Y., Luo, W., Zheng, X., Zeng, D., et al. (2015). COLD1 confers chilling tolerance in rice. Cell 160, 1209–1221. doi: 10.1016/j.cell.2015.01.046
Mao, D., and Chen, C. (2012). Colinearity and similar expression pattern of rice DREB1s reveal their functional conservation in the cold-responsive pathway. PLoS One 7:e47275. doi: 10.1371/journal.pone.0047275
Mattsson, J., Ckurshumova, W., and Berleth, T. (2003). Auxin signaling in Arabidopsis leaf vascular development. Plant Physiol. 131, 1327–1339. doi: 10.1104/pp.013623
Michniewicz, M., Brewer, P. B., and Friml, J. I. (2007). Polar auxin transport and asymmetric auxin distribution. Arabidopsis Book 5:e0108. doi: 10.1199/tab.0108
Mittler, R. (2002). Oxidative stress, antioxidants and stress tolerance. Trends Plant Sci. 7, 405–410. doi: 10.1016/s1360-1385(02)02312-9
Miyashita, Y., Takasugi, T., and Ito, Y. (2010). Identification and expression analysis of PIN genes in rice. Plant Sci. 178, 424–428. doi: 10.1016/j.plantsci.2010.02.018
Moon, S. J., Min, M. K., Kim, J. A., Kim, D. Y., Yoon, I. S., Kwon, T. R., et al. (2019). Ectopic expression of OsDREB1G, a member of the OsDREB1 subfamily, confers cold stress tolerance in rice. Front. Plant Sci. 10:297. doi: 10.3389/fpls.2019.00297
Morris, D. A. (1979). The effect of temperature on the velocity of exogenous auxin transport in intact chilling-sensitive and chilling-resistant plants. Planta 146, 603–605. doi: 10.1007/BF00388839
Nadella, V., Shipp, M. J., Muday, G. K., and Wyatt, S. E. (2006). Evidence for altered polar and lateral auxin transport in the gravity persistent signal (gps) mutants of Arabidopsis. Plant Cell Environ. 29, 682–690. doi: 10.1111/j.1365-3040.2005.01451.x
Palme, K., Dovzhenko, A., and Ditengou, F. A. (2006). Auxin transport and gravitational research: perspectives. Protoplasma 229, 175–181. doi: 10.1007/s00709-006-0216-9
Park, J. E., Park, J. Y., Kim, Y. S., Staswick, P. E., Jeon, J., Yun, J., et al. (2007). GH3-mediated auxin homeostasis links growth regulation with stress adaptation response in Arabidopsis. J. Biol. Chem. 282, 10036–10046. doi: 10.1074/jbc.M610524200
Petrasek, J., Mravec, J., Bouchard, R., Blakeslee, J. J., Abas, M., Seifertova, D., et al. (2006). PIN proteins perform a rate-limiting function in cellular auxin efflux. Science 312, 914–918. doi: 10.1126/science.1123542
Prasad, T. K., Anderson, M. D., and Stewart, C. R. (1994a). Acclimation, hydrogen peroxide, and abscisic acid protect mitochondria against irreversible chilling injury in maize seedlings. Plant Physiol. 105, 619–627. doi: 10.1104/pp.105.2.619
Prasad, T. K., Anderson, M. D., and Stewart, C. R. (1994b). Evidence for chilling-induced oxidative stress in maize seedling and a regulatory role for hydrogen peroxide. Plant Cell 6, 65–74. doi: 10.1105/tpc.6.1.65
Saito, K., Hayano-Saito, Y., Kuroki, M., and Sato, Y. (2010). Map-based cloning of the rice cold tolerance gene Ctb1. Plant Sci. 179, 97–102. doi: 10.1016/j.plantsci.2010.04.004
Sang, T., and Ge, S. (2007). Genetics and phylogenetics of rice domestication. Curr. Opin. Genet. Dev. 17, 533–538. doi: 10.1016/j.gde.2007.09.005
Shah, F. A., Ni, J., Yao, Y., Hu, H., Wei, R., and Wu, L. (2021). Overexpression of Karrikins receptor gene Sapium sebiferum KAI2 promotes the cold stress tolerance via regulating the redox homeostasis in A. thaliana. Front. Plant Sci. 12:657960. doi: 10.3389/fpls.2021.657960
Shi, Y., Ding, Y., and Yang, S. (2018). Molecular regulation of CBF signaling in cold acclimation. Trends Plant Sci. 23, 623–637. doi: 10.1016/j.tplants.2018.04.002
Shibasaki, K., Uemura, M., Tsurumi, S., and Rahman, A. (2009). Auxin response in Arabidopsis under cold stress: underlying molecular mechanisms. Plant Cell 21, 3823–3838. doi: 10.1105/tpc.109.069906
Singh, P. K., Indoliya, Y., Chauhan, A. S., Singh, S. P., Singh, A. P., Dwivedi, S., et al. (2017). Nitric oxide mediated transcriptional modulation enhances plant adaptive responses to arsenic stress. Sci. Rep. 7, 3592. doi: 10.1038/s41598-017-03923-2
Song, J., Wu, H., He, F., Qu, J., Wang, Y., Li, C., et al. (2021). Citrus sinensis CBF1 functions in cold tolerance by modulating putrescine biosynthesis through regulation of arginine decarboxylase. Plant Cell Physiol. 63, 19–29. doi: 10.1093/pcp/pcab135
Steinhorst, L., and Kudla, J. (2013). Calcium – a central regulator of pollen germination and tube growth. Biochim. Biophys. Acta 1833, 1573–1581. doi: 10.1016/j.bbamcr.2012.10.009
Stockinger, E. J., Gilmour, S. J., and Thomashow, M. F. (1997). A. thaliana CBF1 encodes an AP2 domain-containing transcriptional activator that binds to the C-repeat/DRE, a cis-acting DNA regulatory element that stimulates transcription in response to low temperature and water deficit. Proc. Natl. Acad. Sci. U. S. A. 94, 1035–1040. doi: 10.1073/pnas.94.3.1035
Sun, Q., Huang, R., Zhu, H., Sun, Y., and Guo, Z. (2021). A novel Medicago truncatula calmodulin-like protein (MtCML42) regulates cold tolerance and flowering time. Plant J. 108, 1069–1082. doi: 10.1111/tpj.15494
Wang, J. R., Hu, H., Wang, G. H., Li, J., Chen, J. Y., and Wu, P. (2009). Expression of PIN genes in rice (O. sativa L.): tissue specificity and regulation by hormones. Mol. Plant 2, 823–831. doi: 10.1093/mp/ssp023
Wang, J., Ren, Y., Liu, X., Luo, S., Zhang, X., Liu, X., et al. (2021b). Transcriptional activation and phosphorylation of OsCNGC9 confer enhanced chilling tolerance in rice. Mol. Plant 14, 315–329. doi: 10.1016/j.molp.2020.11.022
Wang, W., Vinocur, B., and Altman, A. (2003). Plant responses to drought, salinity and extreme temperatures: towards genetic engineering for stress tolerance. Planta 218, 1–14. doi: 10.1007/s00425-003-1105-5
Wang, H. Q., Xuan, W., Huang, X. Y., Mao, C., and Zhao, F. J. (2021a). Cadmium Iihibits lateral root emergence in rice by disrupting OsPIN-mediated auxin distribution and the protective effect of OsHMA3. Plant Cell Physiol. 62, 166–177. doi: 10.1093/pcp/pcaa150
Woodward, A. W., and Bartel, B. (2005). Auxin: regulation, action, and interaction. Ann. Bot. 95, 707–735. doi: 10.1093/aob/mci083
Wu, S. Y., Yang, X. Y., Zhang, Y. W., Hou, D. Y., and Xu, H. W. (2021). Generation of ospin9 mutants in rice by CRISPR/Cas9 genome editing technology. Sci. Agric. Sin. 54, 3805–3817. doi: 10.3864/j.issn.0578-1752.2021.18.002
Wu, F. B., Zhang, G. P., and Dominy, P. (2003). Four barley genotypes respond differently to cadmium: lipid peroxidation and activities of antioxidant capacity. Environ. Exp. Bot. 50, 67–78. doi: 10.1016/S0098-8472(02)00113-2
Xu, H. W., Ji, X. M., He, Z. H., Shi, W. P., Zhu, G. H., Niu, J. K., et al. (2006). Oxalate accumulation and regulation is independent of glycolate oxidase in rice leaves. J. Exp. Bot. 57, 1899–1908. doi: 10.1093/jxb/erj131
Xu, H. W., Zhang, Y. W., Yang, X. Y., Wang, H. H., and Hou, D. Y. (2022). Tissue specificity and responses to abiotic stresses and hormones of PIN genes in rice. Biologia 77, 1459–1470. doi: 10.1007/s11756-022-01031-9
Yamaguchi-Shinozaki, K., and Shinozaki, K. (2006). Transcriptional regulatory networks in cellular responses and tolerance to dehydration and cold stresses. Annu. Rev. Plant Biol. 57, 781–803. doi: 10.1146/annurev.arplant.57.032905.105444
Zeng, Y., Zhang, Y., Xiang, J., Uphoff, N. T., Pan, X., and Zhu, D. (2017). Effects of low temperature stress on spikelet-related parameters during anthesis in indica-japonica hybrid rice. Front. Plant Sci. 8:1350. doi: 10.3389/fpls.2017.01350
Zhang, D., Guo, X., Xu, Y., Li, H., Ma, L., Yao, X., et al. (2019a). OsCIPK7 point-mutation leads to conformation and kinase-activity change for sensing cold response. J. Integr. Plant Biol. 61, 1194–1200. doi: 10.1111/jipb.12800
Zhang, Z., Li, J., Li, F., Liu, H., Yang, W., Chong, K., et al. (2017). OsMAPK3 phosphorylates OsbHLH002/OsICE1 and inhibits its ubiquitination to activate OsTPP1 and enhances rice chilling tolerance. Dev. Cell 43:e735. doi: 10.1016/j.devcel.2017.11.016
Zhang, J., Li, X. M., Lin, H. X., and Chong, K. (2019b). Crop improvement through temperature resilience. Annu. Rev. Plant Biol. 70, 753–780. doi: 10.1146/annurev-arplant-050718-100016
Zhang, J., Luo, W., Zhao, Y., Xu, Y., Song, S., and Chong, K. (2016). Comparative metabolomic analysis reveals a reactive oxygen species-dominated dynamic model underlying chilling environment adaptation and tolerance in rice. New Phytol. 211, 1295–1310. doi: 10.1111/nph.14011
Zhao, Y. (2008). The role of local biosynthesis of auxin and cytokinin in plant development. Curr. Opin. Plant Biol. 11, 16–22. doi: 10.1016/j.pbi.2007.10.008
Zhao, Y. (2010). Auxin biosynthesis and its role in plant development. Annu. Rev. Plant Biol. 61, 49–64. doi: 10.1146/annurev-arplant-042809-112308
Zhao, C., Lang, Z., and Zhu, J. K. (2015). Cold responsive gene transcription becomes more complex. Trends Plant Sci. 20, 466–468. doi: 10.1016/j.tplants.2015.06.001
Zhao, Z., Zhang, Y., Liu, X., Zhang, X., Liu, S., Yu, X., et al. (2013). A role for a dioxygenase in auxin metabolism and reproductive development in rice. Dev. Cell 27, 113–122. doi: 10.1016/j.devcel.2013.09.005
Keywords: auxin efflux carrier, CRISPR/Cas9, Oryza sativa, OsPIN9, chilling tolerance
Citation: Xu H, Yang X, Zhang Y, Wang H, Wu S, Zhang Z, Ahammed GJ, Zhao C and Liu H (2022) CRISPR/Cas9-mediated mutation in auxin efflux carrier OsPIN9 confers chilling tolerance by modulating reactive oxygen species homeostasis in rice. Front. Plant Sci. 13:967031. doi: 10.3389/fpls.2022.967031
Received: 12 June 2022; Accepted: 05 July 2022;
Published: 01 August 2022.
Edited by:
Zhijian Chen, Chinese Academy of Tropical Agricultural Sciences, ChinaReviewed by:
Babar Usman, Kyung Hee University, South KoreaCopyright © 2022 Xu, Yang, Zhang, Wang, Wu, Zhang, Ahammed, Zhao and Liu. This is an open-access article distributed under the terms of the Creative Commons Attribution License (CC BY). The use, distribution or reproduction in other forums is permitted, provided the original author(s) and the copyright owner(s) are credited and that the original publication in this journal is cited, in accordance with accepted academic practice. No use, distribution or reproduction is permitted which does not comply with these terms.
*Correspondence: Huawei Xu, eGh3Y3luQDE2My5jb20=
Disclaimer: All claims expressed in this article are solely those of the authors and do not necessarily represent those of their affiliated organizations, or those of the publisher, the editors and the reviewers. Any product that may be evaluated in this article or claim that may be made by its manufacturer is not guaranteed or endorsed by the publisher.
Research integrity at Frontiers
Learn more about the work of our research integrity team to safeguard the quality of each article we publish.