- 1School of Agriculture Science and Biotechnology, Faculty of Bioresources and Food Industry, Universiti Sultan Zainal Abidin, Terengganu, Malaysia
- 2Department of Biology, College of Science, Taif University, Taif, Saudi Arabia
- 3School of Food Industry, Faculty of Bioresources and Food Industry, Universiti Sultan Zainal Abidin, Terengganu, Malaysia
- 4Department of Crop Science, Faculty of Agriculture, Universiti Putra Malaysia, Seri Kembangan, Malaysia
- 5Plant Production Department, College of Food and Agricultural Sciences, King Saud University, Riyadh, Saudi Arabia
Growth improvement of the medicinal plant, Ficus deltoidea (Mas Cotek) under drought conditions is a vital issue in Malaysia since it is a slow-growing plant and disposed to leaf damage under the stresses of drought. Therefore, investigation was done to examine the outcomes of hydrogen peroxide (H2O2) application on Rubisco gene expression and metabolites accumulation of stressed F. deltoidea plants, and thereby to record the changes in leaf histology, photosynthesis, biochemical properties, and the growth of the plant. H2O2 at the rates of 0, 5, 10, 15, and 20 mM were foliar sprayed biweekly on the drought stressed plants using a hand sprayer. The application of 20 mM H2O2 amplified leaf number, tallness, stomatal conductance, and photosynthetic yield by 143, 24, 88, and 18%, respectively, over the control plant. A reduced transpiration rate and improved chlorophyll fluorescence were also noted in H2O2-treated plants. The treatment produced a greater amount of chlorophyll a, total phenols, total flavonoids, sugar content, and antioxidant activities by 1.61-, 1.30-, 1.98-, 1.92-, and 1.53-fold, respectively. Application of 15 mM H2O2 enhanced net photosynthetic rate and internal CO2 concentrations by 1.05- and 1.25-fold, respectively. Additionally, H2O2 treatments promoted stomatal closure, increased stomata size, the number of stomata, improved vein structure, and reduced the damage of the leaf margin and mesophyll cells of drought stressed plants. The application of H2O2 also accumulated significantly higher contents of sodium (Na+), calcium (Ca2+), potassium (K+), magnesium (Mg+), and iron (Fe2+) in stressed plants. Although the amount of Arsenic (As+) and Antimony (Sb3+) increased to some extent, the increases were not at a toxic level. The use of H2O2 enhanced the Rubisco gene expression to a greater level and the ratio of Rubisco expression increased up to 16-fold. Finally, thirteen (13) identified and five (5) unmatched volatile compounds with a quality score above 70% were identified by gas chromatography-mass spectrometry (GCMS). The GCMS analysis showed that the foliar application of H2O2 accumulates a higher percentage of volatile components in plants which helps to mitigate the negative effects of drought stress. It is concluded that under drought stressed conditions the F. deltoidea plants should be treated with 10–15 mM of H2O2 twice a week to improve leaf histology, photosynthesis, the level of Rubisco gene expression and volatile compounds accumulation, and plant growth and development.
Introduction
An important medicinal plant in Malaysia, Ficus deltoidea (Mas Cotek) is a C3 plant of the Moraceae family that can be found in Malaysia, Indonesia, Thailand, The Philippines, South Asia, and Africa (Starr et al., 2003; Hasham et al., 2013; Badron et al., 2014). The plant is grown as a house plant in cooler regions and is also cultivated in various parts of the world in abundance along the peat soil, beaches, and forest areas up to 3,000 m above sea level (Starr et al., 2003; Musa, 2005). It is used in traditional medicinal practices due to its various medical properties and functions. It is effective for healing, is used as a palliative agent, antinociceptive, antioxidant, anti-proliferative, and as preventive medicine which treats postpartum depression and assists in contracting and healing the muscles of the uterus and vaginal canal (Fasihuddin and Din, 2002). Tea from the leaves of F. deltoidea contain a considerable amount of magnesium, manganese, potassium, sodium, iron, and zinc, which are used to fulfill human daily nutrient requirements (Nihayah et al., 2012). F. deltoidea extracts are used to reduce diabetes complications, increase HDL levels, decrease LDL levels, improve sex life, assist the contraction of the vagina after delivery in addition to having anti-cancer and antimicrobial properties (Aris et al., 2009; Dramant et al., 2012). The plant contains phenols, flavonoids, tannins, triterpenoids and proanthocyanins (Aris et al., 2009; Nazarni et al., 2018). All these phenols and flavonoids display high antioxidant properties, with bioactive compounds vitexin and isovitexin from F. deltoidea displaying α-glucosidase inhibition (Nazarni et al., 2018; Nurdiana et al., 2018).
Currently the pharmaceutical industry uses plant-based sources to produce bioactive compounds and secondary metabolites. F. deltoidea as a medicinal herb is gaining popularity in local markets for the preparation of herbal tea and capsules (Misbah et al., 2013). This is a slow growing medicinal herb with low photosynthetic capacity since direct sunlight does not enhance its growth resulting in low biomass accumulation. The biomass accumulation in plants is very important for its commercialization. This herb also cannot bear drought stress as it needs plenty of water (6–8% soil moisture) to ensure its optimum growth and development. F. deltoidea plant is prone to an unexpected loss of leaves under drought conditions. Drought stress reduces cell division and enlargement, root differentiation, leaf area expansion, plant height, altered stomatal opening and closing, water use efficiency, and decreased plant yield and quality (Kumawat and Sharma, 2018). Due to the closing of the stomata, the activity of photosynthesis decreases, increasing production of reactive oxygen species (ROS), inducing membrane injury and altered function of several enzymes which are associated with ATP synthesis (Kumawat and Sharma, 2018; Sharma et al., 2020). To overcome all these problems in the F. deltoidea plant, this study proposes to use H2O2 to increase photosynthetic capacity, nutrients absorption, improve leaf histology, metabolites accumulation, and improve drought tolerance.
Drought stresses create interruption in plant growth, reduce plant productivity, and in severe cases, lead to plant death. The plant under drought stress produces signals for new structural and metabolic capabilities through gene expression for immediate survival and acclimatization (Bohnert and Sheveleva, 1998). Hydrogen peroxide (H2O2) may act as a messenger molecule to adapt signaling which triggers anti-susceptibility under abiotic stresses when it orchestrates a programmed cell death (Cheeseman, 2007; Ismail et al., 2015). Kar (2011) reported that the drought stress generates reactive oxygen species (ROS) leading to cellular damage. But H2O2 is a highly stable ROS at optimum concentration, which acts as a second messenger and sends signals to molecules for the promotion of plant growth, enhancement of photosynthetic activity, and increase in sugar content (Ozaki et al., 2009; Khandaker et al., 2012). Ozaki et al. (2009) also stated that H2O2 (<50 mM concentration) produced stimulatory effects on plant growth and development of melon plants. A higher concentration of H2O2 initiates programmed cell death, damage chloroplast and the photosynthesis process, however, findings of Orabi et al. (2015) determined that a lower level of H2O2 stimulated growth regulators (auxins, gibberellin, and ABA) and enzyme activity. The foliar application of H2O2 maintains the leaf water content in soybean plants and enables them to avoid drought stress (Ishibashi et al., 2011). Many physiological processes in plants are controlled by H2O2 such as ABA-induced stomatal opening and closing, stomatal movement, hypersensitive responses, gene expression, programmed cell death, etc. (Neill et al., 2002).
H2O2 is produced in different enzymatic reactions in plants, which is transported through liquid flow in the plant body. It acts as a growth regulator in several selected plants. It was used in this study as the chemical promoter to overcome the early phase slow growth problem of F. deltoidea and to counteract the drought stress effects introduced to F. deltoidea plants. Attention was given through investigation to the regulatory effects of H2O2 in Rubisco (rbcL) gene expression and metabolite accumulation in F. deltoidea under drought stressed conditions to improve biochemical properties, leaf histology, photosynthesis, and plant growth. It was proposed that under drought stress conditions, foliar spraying of H2O2 could modulate the plant's growth and could mitigate the unfriendly effects of drought in this experimental plant, F. deltoidea. The findings of this study will be beneficial in mitigating the negative effects of drought stress in F. deltoidea as well as other medicinal herb plants.
Materials and methods
Experimental site and preparation of experimental plants
The study was conducted at Universiti Sultan Zainal Abidin, Terengganu, Malaysia during the period from December 2014 to February 2020. The experimental plant Ficus deltoidea var. deltoidea (FD 156) was collected from Kampung Sungai Nibong, Batu Pahat, Malaysia. The plants were propagated first in the university farm using stem cutting 8.0 inches in size and were used later for treatment application. For preparation of experimental plants, 25 stem cuttings were transplanted into an prepared planting media, each polybag contained 15 kg planting media. Cocoa peat, paddy husk, and perlite were mixed in the ratio of 2:1:1 to prepare the media. Side dressing with 2.5 g of NPK fertilizer (10:10:20) was done once a month. To simulate drought stress conditions in all experimental plants, less water (about 1.5 liters of water for 25 plants per spray) was applied three times per week using a watering can maintaining the moisture content at 40% of field capacity. One hundred eighty (180) ml of water was applied per plant to maintain drought stress of the experimental plants. The experimental plants were grown under a hoop house which provided the plants with direct sunlight and an average temperature about 30–35°C. The maximum PAR was between 300–700 μEm−2s−1 and relative humidity was 30–60% (Figure 2A).
Treatments application
The plants were sprayed twice a week manually with 10 ml of hydrogen peroxide (H2O2) at the concentrations of 5, 10, 15, 20 mM and 0 (water as control). The H2O2 concentration was selected based on previous studies reported by Ozaki et al. (2009) and Khandaker et al. (2012). The spraying was done at the dry leaf stage around 11:00 am, from the vegetative to flowering stage completing 15 foliar sprays during the experimental period. The treatments were applied following a completely randomized design (CRD) with five replications.
Data collection
Growth and physiological parameters
The number of leaves, the tallness of the plant, and the leaf area were recorded. A Leaf Area Meter (Model Portable Laser CI-202, CID Bio-science, USA) was used to quantify leaf area. A measuring tape was used to measure plant height (from base of plant to top of youngest shoot). The SPAD 502 Plus Meter of Konica Minolta; Opti-science, USA was used to record chlorophyll content. A Handy PEA meter (Hansatech-Instrument, UK) was used to record the data on chlorophyll fluorescence in three levels, F0, Fv, and Fv/Fm on sunny days, once a month. The CI-340 handheld Photosynthesis System (Bio-Science, USA) was used to record other physiological parameters, e.g., net photosynthetic rate (Pn), transpiration rate (tr), internal CO2 concentration, and stomatal conductance (gsw). As per Khandaker et al. (2012), growth and physiological parameters of treated and untreated plants were recorded eight (8) times and, for each time, data was recorded 2 days after the treatment application. A series of experiments were carried out for the repeated measurements of physiological and growth parameters, nutrient elements and biochemical analysis, gene expression studies, and major compound identifications from leaf extracts of drought stressed plants.
Biochemical properties and mineral nutrient analysis
First, the methanolic extract was developed using treated and untreated leaves of F. deltoidea for biochemical analysis. Harvested leaves (3 days after the treatment application) were dried for 3 days at 45°C in a dryer. The dried leaves were ground properly in a grinding machine and 10 g of leaf powder were soaked in 70% methanol extract for 3 days and then transferred to an orbitary shaker for mixing. Filtration of the contents was done using Whatsman No. 1 filter paper. The crude methanolic extract was prepared by evaporating the filtrate into a rotary evaporator.
Dubois et al. (1956) method was referred to when measuring the sugar content in the crude methanolic extract. The standard method of Singleton and Rossi (1965) was used to estimate the total phenolic content of the extract. Gallic acid, as a standard chemical was used to express the phenolic content as mg/GAE/g [milligram gallic acid equivalents (GAE) per gram] of dry extract. The Aluminum Chloride colorimetric analysis (Chang et al., 2002) was used to determine the total flavonoid content as mg/QE/g [milligram quercetin equivalents (QE) per gram] dry weight since quercetin was used as a standard chemical. The antioxidant properties of the plants were ascertained by carrying an ABTS assay following the guidelines of the ABTS kit's manual (Sigma-aldrich). The formulae of Arnon (1949) were used to determine the contents of chlorophyll a, b and carotenoid. The leaves and syconium of treated and untreated plants were collected at 1 day after the treatment application for mineral nutrients analysis. The Neutron Activation Analysis (NAA) method was used to analyze the mineral accumulation in leaves and syconium, which was described by Nashriyah et al. (2006). The Sodium (Na+), Potassium (K+), Calcium (Ca2+), Magnesium (Mg+), Iron (Fe2+), Arsenic (As+), and Antimoni (Sb3+) were analyzed at the Malaysian Nuclear Agency, Bangi, Kajang, Selangor.
Leaf histology
A histological examination was carried out at the Electron Microscope Scanning laboratory, Institute of Oceanography and Environment (INOS), Universiti Malaysia Terengganu (UMT), Terengganu with the help of a Scanning Electron Microscopy (SEM) (Model JEOL JSm-636OLA) as per methodology of Rohini et al. (2016). The prepared samples were arranged accordingly on the stub for gold coating and the samples were ready to be observed under the SEM. The parts of the leaves that were focused on in this study were the leaf cross section, leaf margin, stomata at lower and upper surfaces of the leaves, as well as stomatal size, and leaf vascular bundle.
Rubisco gene expression
The RNA extraction was done according to the procedure described by Wang et al. (2015) in the manual of SV RNA Isolation System kit (Promega) and the centrifugation was used at 12,000–14,000 rpm. Yield of RNA obtained was determined by using a Spectrophotometer at 260 nm wavelength. The manual of Takara Prime Script 1st strand cDNA synthesis kit was consulted to synthesize the cDNA. The reaction tubes were incubated in heat-block at 70°C for 15 mins to inactivate the reverse transcriptase before doing the PCR and amplification. Aliquots of the single strand cDNA of the treated plants either at 0, 15, or 20 mM were subjected to quantitative RT-PCR analysis on the rbcL gene. The expression of the Co-GAPDH (glyceraldehydes-3-phosphate-dehydrogenase) gene was considered as the internal control. Using a Rotor-Gene 6000 Series Software 1.7 (Build 65), the expression data were evaluated.
Gas chromatography-mass spectrometry
Agilent Technologies 7890A/5975C MSD Gas Chromatograph Mass spectrometry system, equipped with HP-5MS Ultra inert capillary column (30 m × 0.25 mm inner diameter, 0.25 μm film thickness) was used to identify the major compounds in the leaf extracts of H2O2 treated and untreated F. deltoidea. Helium was used as the carrier gas and conditioned at 8.2317 psi pressure with a flow rate at 1 ml min−1. The samples were injected into the GCMS system with the injector and transfer line temperature conditioned at 250°C. The oven temperature was programmed at 60°C (10 min), 60°C to 180°C at the rate of 3°C/min and 180°C (15 min). The sample was injected into a split mode of front inlet with split ratio 50:1. Compounds present in the samples with quality of the probability score above 70% were selected and identified using the Wiley7Nist05.L and HPCH2205.L Mass Spectral Library.
Statistical analysis
A completely randomized design was followed to assign the treatments replicated five times. The SPSS-17 statistical software was referred to analyze the data as per the procedure of one-way ANOVA to know the significant difference between the parameters and the Tukey's test (HSD) was used to compare different concentrations of H2O2 in their effects on the parameters studied at p = 0.05.
Results
Impacts of H2O2 on growth and physiological parameters
The regulatory effects of various concentrations of liquid H2O2 and water were observed on drought-stressed Ficus deltoidea plants. The number of leaves were significantly influenced by the treatments, with the highest number of leaves recorded in the 20 mM treated plants. The plant height was 143% higher with the 20 mM H2O2 treatment as compared to the control plants, meaning that H2O2 application stimulated plant height of F. deltoidea in drought conditions although the differences were insignificant (Table 1). The leaf area was also not affected significantly with different treatments of H2O2.
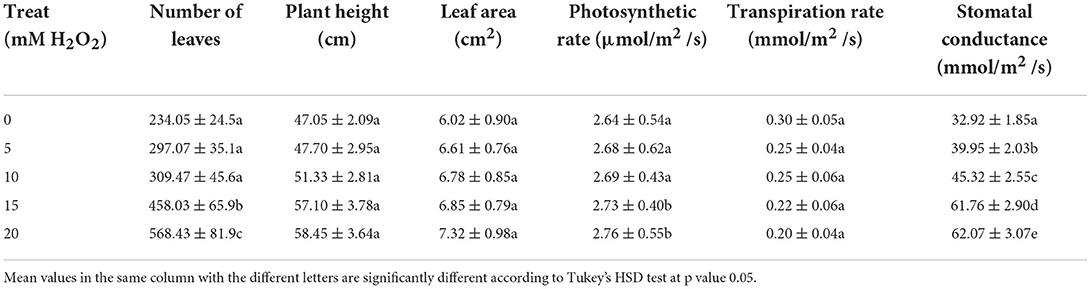
Table 1. The effects of H2O2 treatment on plant growth and photosynthetic characteristics of drought stressed F. deltoidea plants.
The net photosynthetic rate was significantly higher in all H2O2 treatments under drought stress. The application of H2O2 at 15- and 20-mM concentrations produced higher photosynthesis rates which were 1.05- and 1.03-fold higher than the control plant. The lowest photosynthesis rate was found in the untreated plants at 2.64 μmolCO2/m2/s. Therefore, the results indicated that H2O2 treatment could be a factor that promotes better photosynthetic rate in drought conditions (Table 1). For stressed plants, the highest transpiration rate was observed in the control plants with 0.3 μmolCO2/m2/s and the lowest transpiration rates were noted in the treatments of 20 and 15 mM H2O2. These were 33% and 27% lower than the control plants. These results signify that stomatal conductance was influenced differently between the treated and untreated plants under drought stressed conditions. Therefore, application of H2O2 is a method to reduce transpiration rate in stressed plants under drought conditions (Table 1).
The lowest stomatal conductance was observed in untreated plants at 32 mmol CO2/m2/s (Table 1) but it was 1.88- and 1.87-fold higher in the 20 mM and 15 mM H2O2 treated plants, respectively (Table 1). The internal CO2 was also influenced by different treatments of H2O2 (Table 2). The lowest internal CO2 was detected in control plants at 618 ppm, ambient CO2. Plants under the treatments of 15 mM and 20 mM H2O2 had significantly higher internal CO2 levels, which were 1.24- and 1.28-fold respectively, greater than the control plants. Leaf chlorophyll content (estimated by SPAD value) increased by at least 10% due to the different treatments of H2O2 (Table 2).
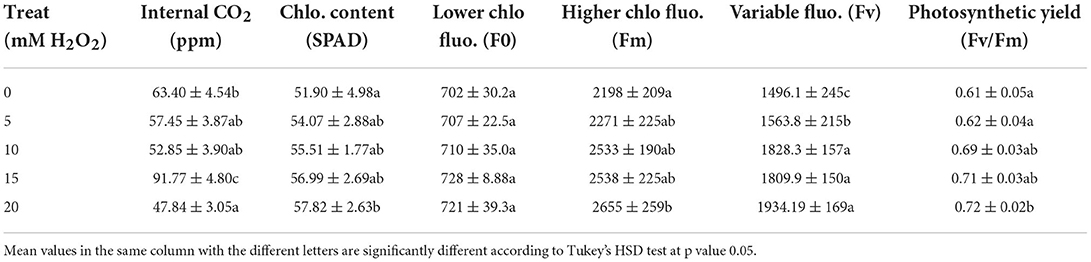
Table 2. The effects of H2O2 treatment on leaf chlorophyll content (SPAD) and chlorophyll fluorescence of F. deltoidea plants under drought conditions.
The chlorophyll fluorescence value (F0) and relative variable fluorescence (Fv) were significantly increased when H2O2 was applied to drought-stressed F. deltoidea plants. Plants treated with 20 mM of H2O2 produced a 20% higher Fm value compared to the control group. The photosynthetic yield increased 1.18-fold due to the application of 20 mM H2O2 (Table 2). The growth and plant physiological parameters of H2O2 treated plants were significantly positively correlated to each other except for the internal CO2 concentration (Figure 1). Additionally, the results show that the number of leaves, plant height, and leaf area were positively correlated with net photosynthetic rate, transpiration rate, stomatal conductance, chlorophyll content, and photosynthetic yield of H2O2 treated F. deltoidea plants under drought stressed conditions (Figure 1).
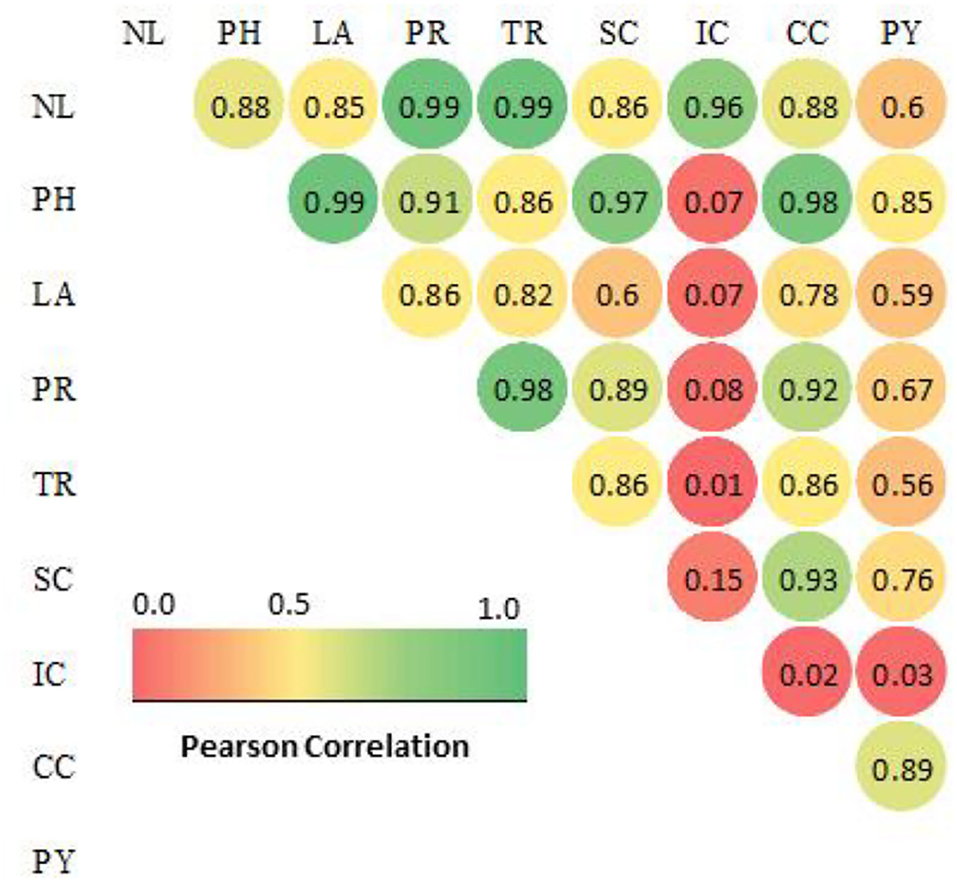
Figure 1. Correlation matrix of plant growth and physiological characteristics in H2O2 treated F. deltoidea plants under drought conditions[[Inline Image]]. The labels of data are similar in Tables 1, 2. Nl, number of leaves; PH, plant height; LA, leaf area; PR, photosynthetic rates; TR, transpiration rates; SC, stomatal conductance; IC, internal CO2 concentration; CC, chlorophyll content (SPAD); PY, photosynthetic yield (Fv/Fm). The colored gradient legends represent coefficients of correlation r values from 1.0 (green), 0.5 (yellow) and 0.0 (red). The value >0.9 a strong correlation, >0.8 a fair strong correlation and >0.6 a moderately strong correlation at p value 0.05.
Effects of H2O2 on biochemical properties
The chlorophyll content of leaves increased in the treated plants (Table 3) and this was highest for the treatment of 20 mM. Plants treated with 20 mM and 15 mM H2O2 yielded 1.67- and 1.59- fold higher chlorophyll b content (Table 3). The lowest carotenoid content was recorded in fresh leaves extract in control (22% lower) compared to the 20 mM treated plants (Table 3). Therefore, application of H2O2 on drought-stressed Mas Cotek plants had a significant effect to increase carotenoid content of the plants (Table 3).
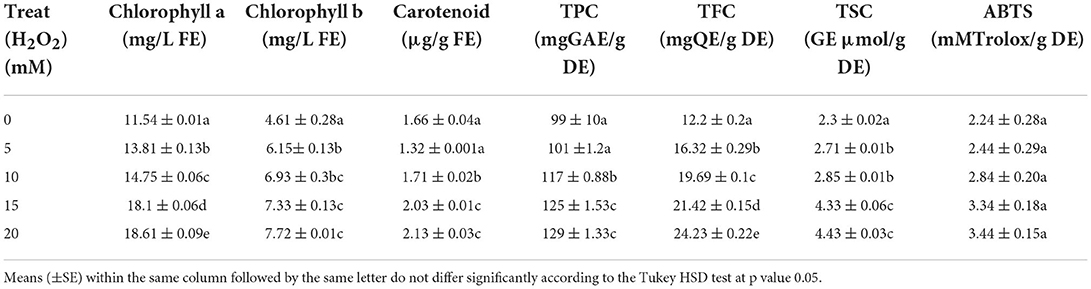
Table 3. The effects of H2O2 on chlorophyll content, biochemical properties, and antioxidant activities of drought stressed Ficus deltoidea plants.
The content of total phenolic (TPC) and total flavonoid (TFC) also increased with the treatment of H2O2 (Table 3). An amount of 1.30- and 1.26- fold higher TPC and TFC content were produced with the application of 15 mM and 20 mM H2O2 to the drought-stressed plants (Table 3). The production of TFC under the treatment of 20 mM H2O2 was 98% higher than in the control plants (Table 3). The lowest TFC was determined in control plants with 12.2 mg quercetin equivalent (QE) per gram of dry leaf extract. Similarly, the total sugar content (TSC) also increased in the leaf extract of treated plants of F. deltoidea (Table 3). The lowest TSC was determined in untreated plants at 2.1 μmol glucose equivalent (GE) per gram of dried leaf extract. The increment of TSC in 15 mM and 20 mM H2O2 treated plants was 1.88- and 1.92-fold higher, respectively (Table 3). The results of ABTS assay test indicates an increment of 49 and 54%, respectively, antioxidant activity in a F. deltoidea methanolic leaf extract under the treatments of 15 and 20 mM in comparison to quercetin as a positive control. However, the differences between the treatments were immaterial (Table 3).
Effects of H2O2 on nutrient uptakes
Mineral uptake and accumulation in the H2O2-treated plants under stressed conditions was positively influenced. The results of Neutron Activation Analysis (NAA) showed that the application of H2O2 promoted sodium (Na) intake into the plants. The highest Na content in a leaf was detected in the 15 mM H2O2 treated plants with 95 μg per gram of dried leaf extract. The lowest Na content was detected in the 20 mM H2O2 treated plants with a value of 70 μg per gram of dried leaf extract, which was significantly less than the 15 mM treated plants (Table 4). The Na content in the treated F. deltoidea syconium was different from the untreated plants. The lowest Na content was observed on control plants with only 260 μg per gram syconium dried extract, meanwhile the highest Na content was detected in the 10 mM H2O2 treated plants with 810 μg per gram syconium dried extract. Hence, the application of H2O2 showed stimulative effects on Na uptake by the plants as all treated plants had higher Na content than the control plants (Table 4). On the other hand, the trend of potassium (K) content in F. deltoidea leaves was different from the Na content, with the highest found in the control and 10 mM H2O2 treated plants (2.37 and 2.04% K) (Table 4). The lowest K content in F. deltoidea leaves was in the 20 mM H2O2 treated plants. The opposite pattern was observed in K content of F. deltoidea syconium. It was marked that the lowest K content was in the control and 5 mM H2O2 treated plants, but the highest K content was observed in the plants treated with the highest concentration of H2O2. Hence, it can be said that H2O2 promoted K uptake especially in syconium rather than in F. deltoidea leaves (Table 4).
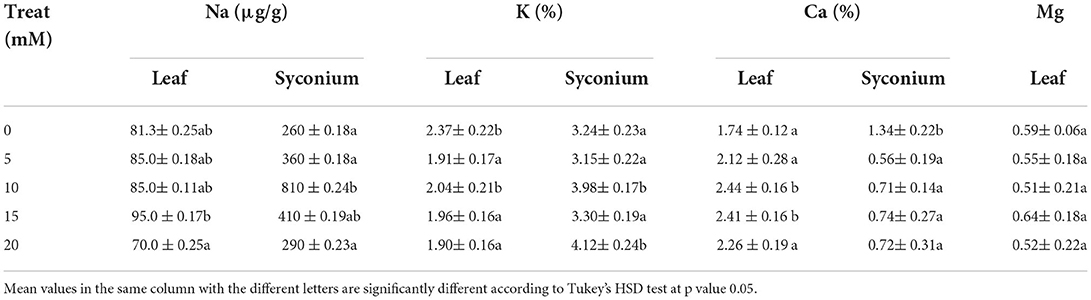
Table 4. The effects of H2O2 on macro mineral uptake and accumulation of F. deltoidea plants under drought stressed conditions.
The spraying of H2O2 produced higher (1.74%) calcium (Ca) content in F. deltoidea leaves than in the control plants (1.74%), while the highest amount was determined in the 10 and 15 mM H2O2-treated plants (2.44 and 2.41%) (Table 4). The opposite trend of Ca content was observed in the syconium. The untreated plants produced the highest Ca content (1.34%) in the syconium while the lowest Ca content was found in the 5 mM H2O2 treated plants. Generally, calcium content is higher in plant leaves than in the syconium of the plants, but the study indicates different results (Table 4). The same pattern of stimulative effect was observed in magnesium (Mg) uptake in treated plants under drought-stressed conditions. It was determined that the highest Mg content was in the 15 mM H2O2 treated plants with 0.64 μg per gram of dried leaf extract while the control plants contained a lower Mg content of 0.59 μg per gram of dried leaf extract (Table 4). On the other hand, the control plants had the highest Mg content in F. deltoidea syconium with 0.41 μg per gram of syconium dried extract (Table 4). Our results confirmed that the 15 mM H2O2-treated plants gave positive impacts on Mg uptake in plant leaves, but Mg uptake and accumulation was higher in the syconium of control plants (Table 5).
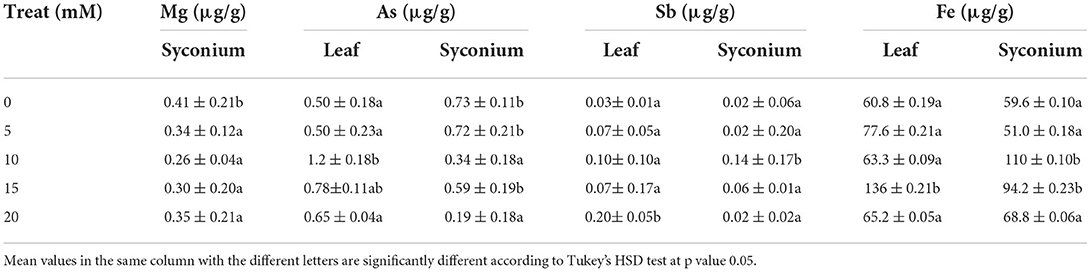
Table 5. The effects of H2O2 on micro mineral uptake and accumulation of F. deltoidea plants under drought stressed conditions.
The lowest arsenic (As) content in F. deltoidea leaves was detected in the control and 5 mM treated plants with 0.5 μg per gram of dried leaf extract, and the highest As content was noticed in the plants when treated with 10 mM H2O2 with 1.2 μg per gram of dried leaf extract (Table 5). On the other hand, the higher arsenic content in the syconium were detected in the control and 5 mM H2O2 treated plants and the lowest As content in the plant syconium was determined in the 20 mM H2O2 treated plants with only 0.19 μg per gram syconium dried extract. So, it can be said that the exogenous H2O2 application promoted arsenic accumulation in plant leaves, while it suppressed the As uptake in the plant syconium (Table 5). Antimony (Sb) content was present in F. deltoidea leaves only at trace amount. The Sb content in leaves was 6.67-fold higher in 20 mM H2O2 treated plants compared to the control plants and their differences were statistically significant (Table 5). The same increasing trend of Sb content was found in the syconium. This shows that 10–20 mM H2O2 were the best concentrations to promote Sb intake in both plant leaves and syconium (Table 5).
The iron (Fe) content in F. deltoidea leaves was observed at the lowest level in the control plants with 60.8 μg per gram of dried leaf extract, meanwhile the highest Fe content was detected in the 15 mM H2O2 treated plants with 136 μg per gram of dried leaf extract, which was significantly greater than the untreated plants (Table 5). In the syconium, the Fe content was also 1.58 times higher in treated plants compared to the control plants and their difference was statistically significant (Table 5). Therefore, H2O2 at the concentration of 15 mM was the best dose in promoting iron uptake in both plant leaves and syconium under drought stressed conditions (Table 5).
Regulatory effects of hydrogen peroxide on leaf histology
Several parts of leaves were examined under SEM to determine H2O2's effect on leaf histology of stressed F. deltoidea plants. H2O2 treatments under drought stress promoted stomatal closure of F. deltoidea leaves in a dose-dependent manner. The control treatment (H2O2 at 0 mM) had the widest leaf stomatal closure which was 2.7 μm. The smallest stomatal closure was observed in the highest H2O2 treated leaves (20 mM H2O2) which was 1.1 μm (Figure 2). It could be speculated that the application of exogenous H2O2 acts as an intermediary to promote guard cell closure under severe drought conditions in the presence of ABA.
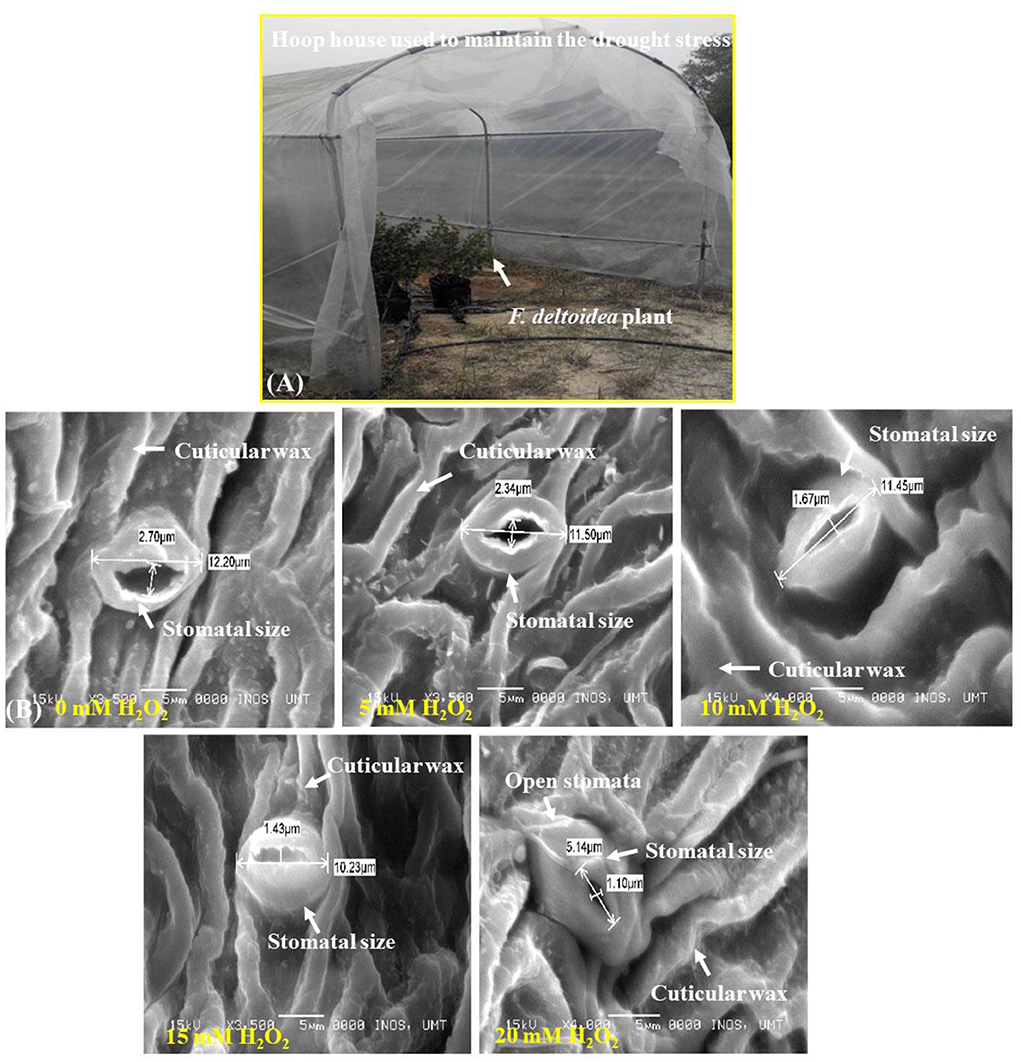
Figure 2. (A) A hoop house was used to maintain drought conditions to the experimental plants. (B) Scanning Electron Microscopy (SEM) photographs of stomatal closure at lower surface of treated and untreated leaves of F. deltoidea plants. Stomatal closure was the widest in control plants whereas the smallest stomatal closure was recorded in the 20 mM H2O2 treated plants. Deformed stoma and guard cell are visible at the leaf of control plants.
The application of H2O2 produced significant changes on stomatal size in the upper and lower surfaces of the leaf (Figures 3, 4). The stomatal opening found on the upper surface was smaller. The control plant had 1.6–3.1 μm of a stomatal opening but the size increased when applied with H2O2 (Figure 3). Plants treated with 20 mM H2O2 had the lowest stomatal size of 0.8–1.5, which were significantly different from the untreated plants. Therefore, it is clear that the stomatal opening or closure is stimulated by H2O2 under stressed field conditions (Figure 3).
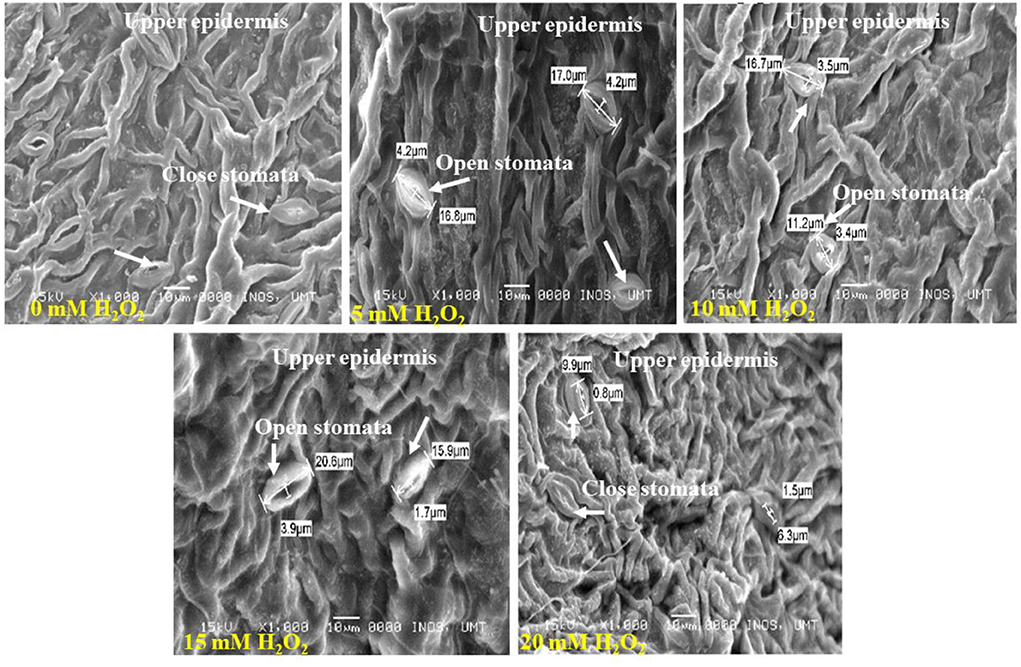
Figure 3. SEM photographs of stomatal opening at leaf's upper surface of H2O2 treated and untreated plants. Stomata are closed at control treatment, but the stomatal opening or size increased with H2O2 treatment (5 mM−15 mM H2O2). Stomatal size starts to decrease again with a higher concentration of H2O2. Less number of stomata contained at the upper surface of the leaves.
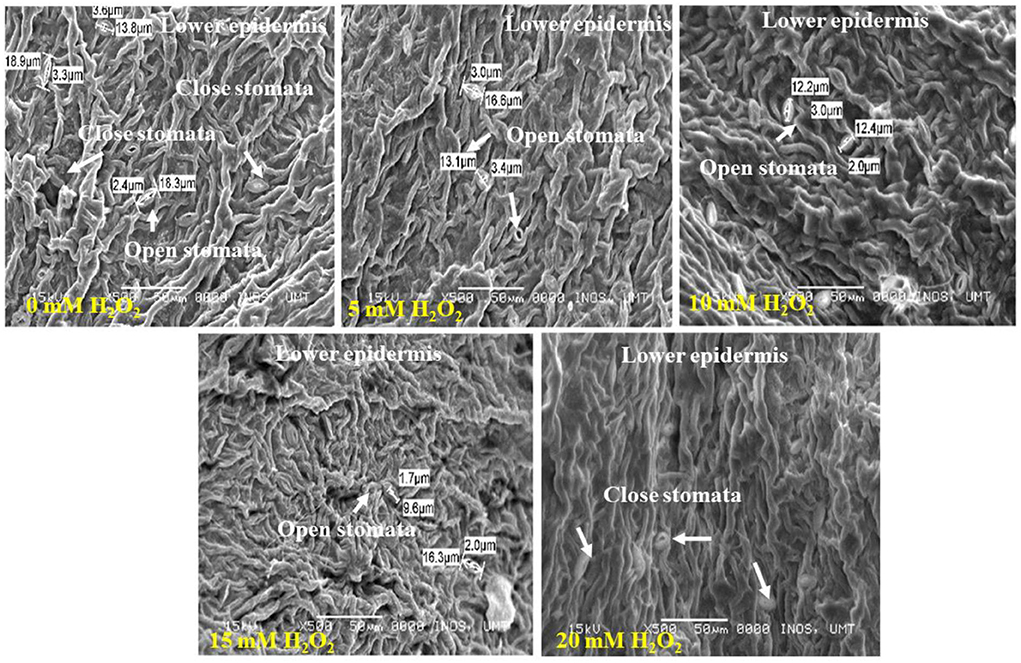
Figure 4. SEM photographs of stomatal closure at lower surface of the F. deltoidea leaves. Lower leaf surface contained a higher number of stomata than the upper surface. The smallest stoma size was observed in the 15 mM H2O2 treatment and stoma size started to decrease with an H2O2 concentration increase under drought stress.
The lower leaf surface contained more numbers of stomata, and the largest stomatal size (1.14–4.1 μm) was recorded with the 20 mM H2O2 treatment (Figure 4). The H2O2 application also had a stimulatory effect on the stomatal size under stressed conditions. Treated plants with 5 and 10 mM of H2O2 had stomatal sizes of 3.0–3.4 μm and 2.0–3.0 μm, respectively. The smallest stomatal sizes (1.7–2.0 μm) were observed in the 15 mM H2O2 treated leaves (Figure 4), with the possibility that 15 mM H2O2 concentration is the best concentration to promote stomatal closure under drought stressed field conditions. Although slight damage appeared on the leaf margin of the control plants and the plants treated with a low dose, 5 mM H2O2, under drought-stressed conditions (Figure 5), no damage was spotted on the leaf margin of F. deltoidea plants treated with higher doses, e.g., 10, 15, and 20 mM H2O2. The results confirm that spraying H2O2 could protect the leaf margin of F. deltoidea plants from damage caused by drought stress (Figure 5).
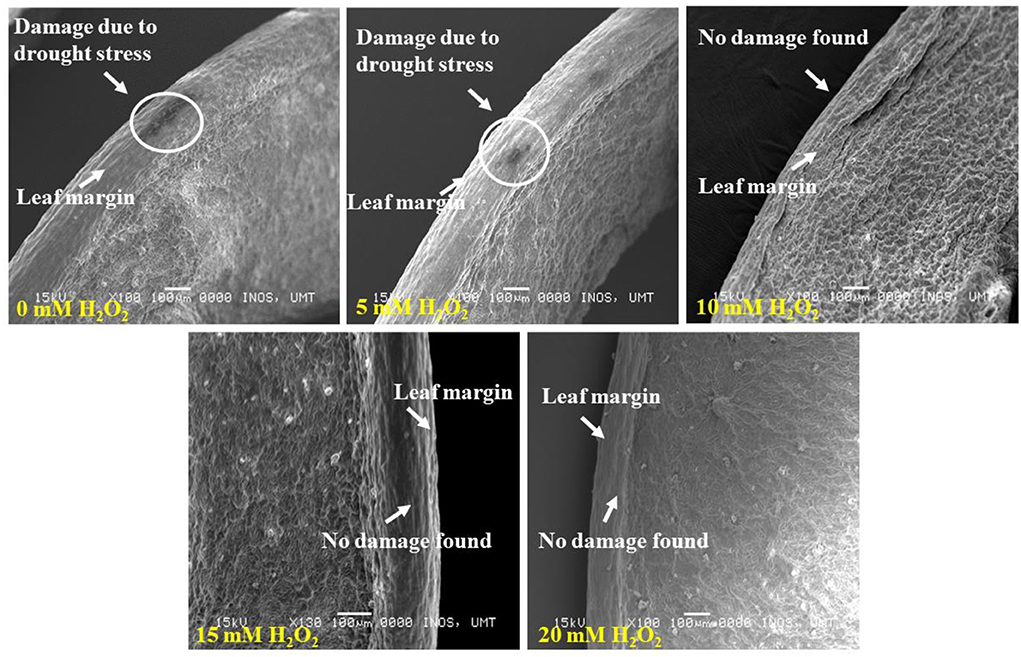
Figure 5. SEM photographs showing the leaf margin of F. deltoidea plants. Damaged appeared in the leaf margin of the control and 5 mM H2O2 treated plants. No damage was found in the 10–20 mM H2O2 treated plants and it is proven that exogenous H2O2 can protect leaf structure from oxidative damage induced by drought stress.
Figure 5 demonstrates the cross section of treated and untreated leaves of F. deltoidea plants. It was noticed that the application of H2O2 reduced the damage to mesophyll cells in the stressed plants. Treated leaves with 5, 10, 15, and 20 mM H2O2 had finer spongy and palisade mesophyll layers than the control plants. This might be related to the function of H2O2 in alleviating stress in the plants (Figure 6). When the cross section of the leaf vein was examined, a similar kind of positive effect, as noticed in mesophyll cells, was observed. All the treated plants had better vein structure than the untreated plants (Figure 7). It was observed that untreated plants had more or less a compact structure of xylem and phloem, whereas H2O2, treated plants had more turgid cells of tracheid in xylem and well-developed phloem tissue. It was also detected that the tracheid and vessel's cells in the vascular bundle were well developed and organized in the treated plants (Figure 7).
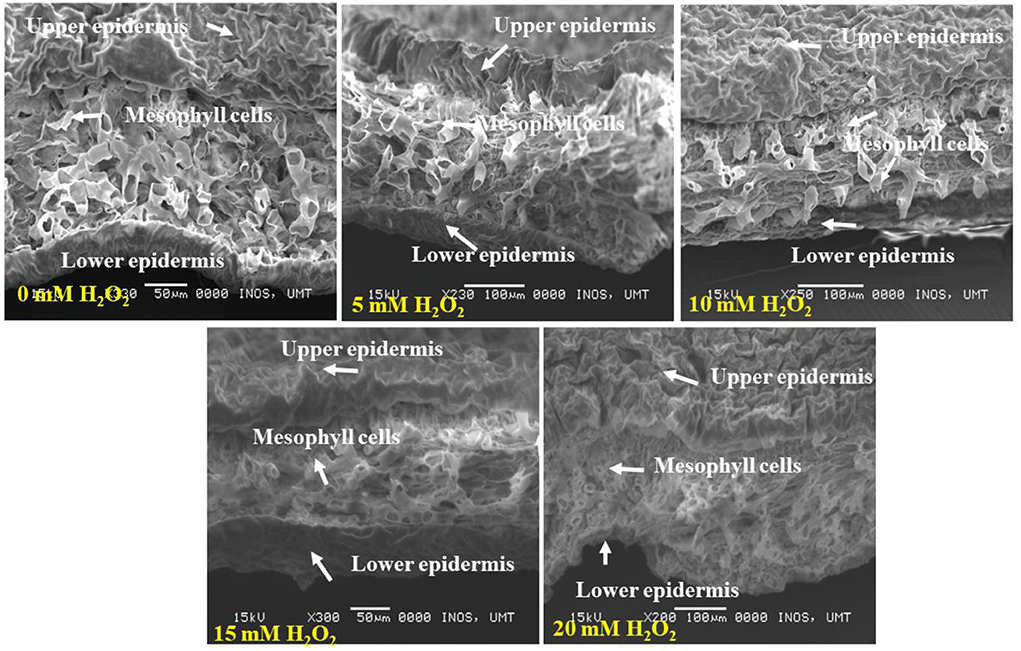
Figure 6. SEM photographs show the cross section of F. deltoidea leaves under drought stressed condition. The photographs show upper and lower leaf surfaces, each surface consists of an epidermis layer, cuticular wax, and mesophyll cells. Well-developed mesophyll cells are visible in 5 mM and 10 mM H2O2 treated plant leaves.
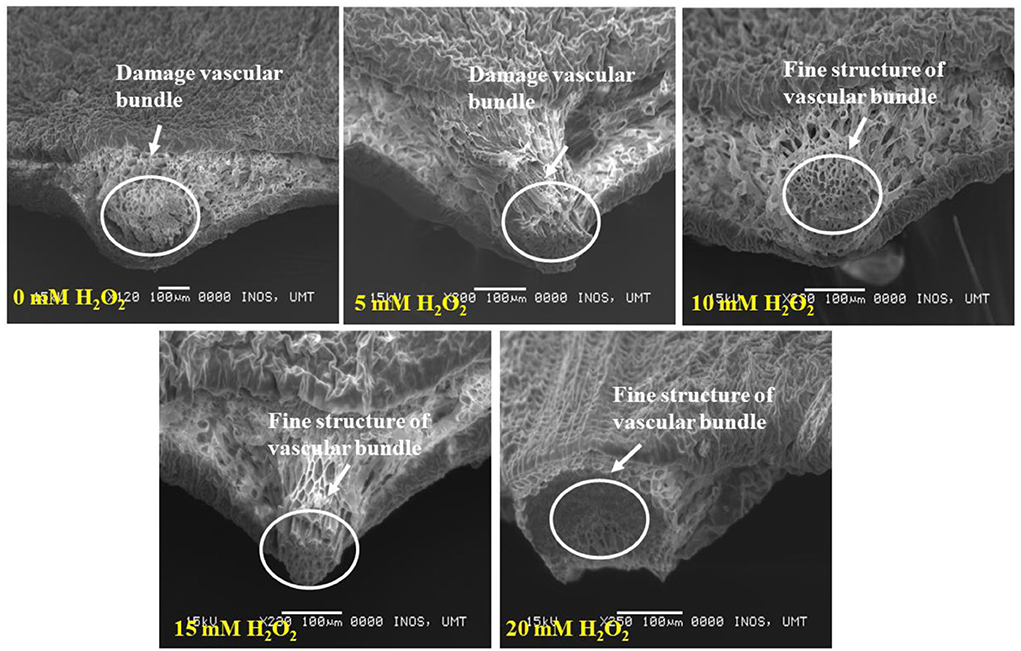
Figure 7. SEM photographs show the cross section of drought stressed F. deltoidea leaf veins. Fine structure of the vascular bundle (xylem and phloem tissues) is visible in the 10–20 mM H2O2 treated plant leaves. Damaged leaf vascular tissues are found in the control and 5 mM H2O2 treated plants.
Effect of hydrogen peroxide on Rubisco gene expression
The effects of H2O2 on Rubisco gene expression in F. deltoidea plants were more visible in the study. H2O2 also regulated the rbcL gene expression in drought-stressed plants of F. deltoidea. In earlier studies, it was reported that the spraying of H2O2 at the concentrations of 15 and 20 mM produced the highest stimulative effects on a leaf histology, photosynthesis, biochemical properties, and plant growth, under drought-stressed conditions. In this study, 0 (control), 15, and 20 mM H2O2 treatments allow us to see the H2O2 effects on the rubisco gene expression and fold change, and determine the best treatment compared to the control. Based on plant growth and physiological performance we selected these three treatments for the rbcL gene expression study. The Real-Time PCR (RT-PCR) test confirmed the stimulative effects on the rbcL gene expression observed in a gel electrophoresis. Figure 8A displays the presence of the rbcL gene in both the leaves treated with 15 mM and 20 mM H2O2 and also in the leaves of control plants when compared with the housekeeping gene (Co-GAPDH). The fold change of rbcL gene was also calculated following Pfaffl and 2−ΔΔCT methods in comparison to 0 mM H2O2 as the control gene. Quantitative analysis indicated that the rbcL gene expression ratio was the highest 0.8649- (Pfaffl method) and 10.23-fold higher (2−ΔΔCTmethod) in the treatment of 15 mM H2O2 when compared to the control plants (Figure 8B). However, the plants treated with 20 mM of H2O2 had a slightly lower fold change of rbcL gene in comparison to the 15 mM H2O2 treatment, but it was still higher than the control. Therefore, application of 15 mM H2O2 promotes the expression of the rbcL gene at a better rate than 20 mM H2O2 treatments under drought stressed field conditions (Figure 8). The rbcL gene fold change was the lowest in the control gene (0 mM H2O2).
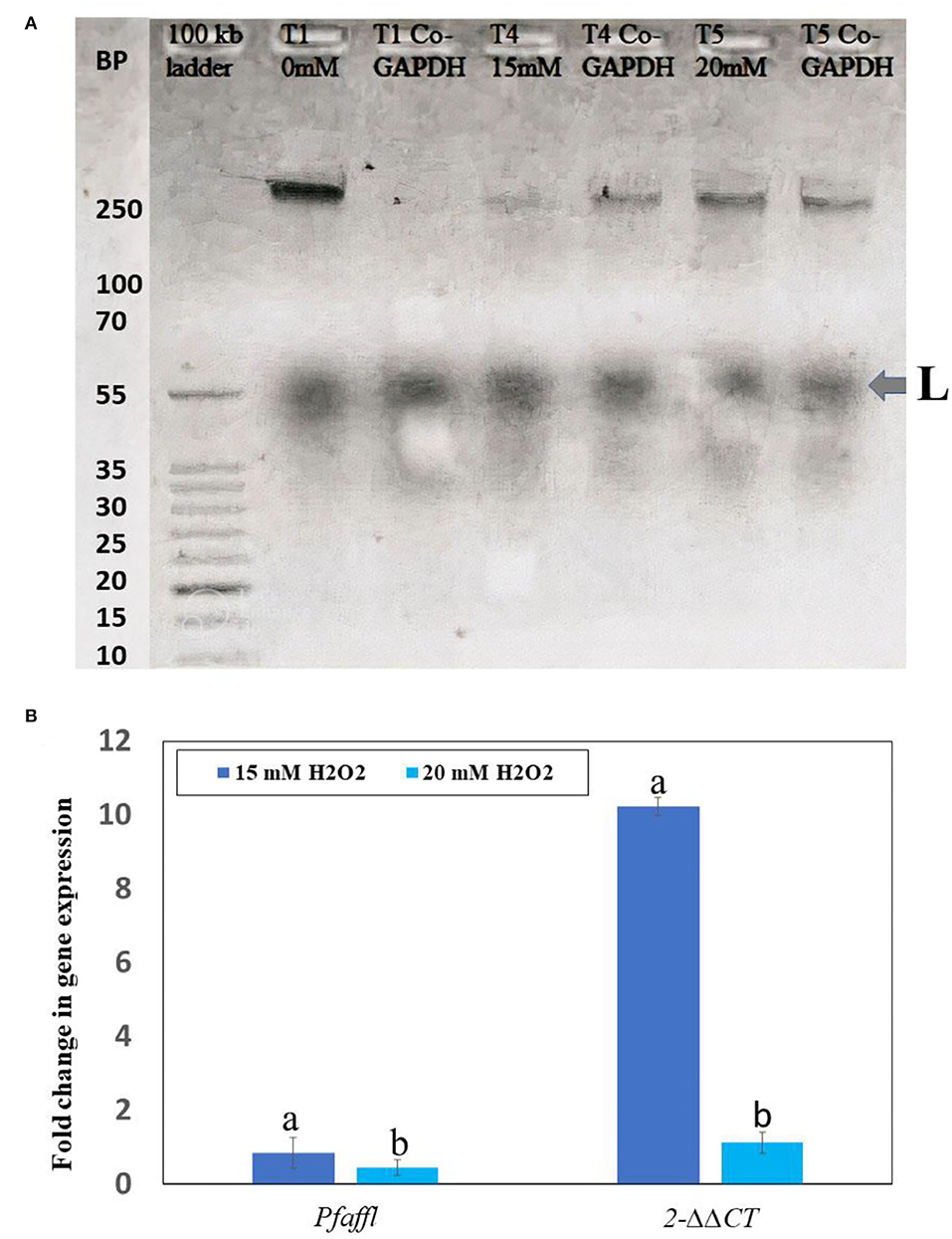
Figure 8. The result of RT-PCR when viewed under gel electrophoresis (A). The Pfaffi and 2−ΔΔCT methods were used to analyze the fold change in rubisco (rbcL) gene expression in the control, 15 mM and 20 mM H2O2 treated plants (B). Bars indicate (± S. E.). Means followed by the same lower case letters within the column are not significantly different according to Tukey's HSD-test (n = 3, p ≤ 0.05).
Gas chromatography-mass spectrometry analysis
GC-MS analysis was carried out to analyze the effects of hydrogen peroxide (H2O2) treatments (T1, 5 mM; T2, 10 mM; T3, 15 mM; T4, 20 mM) on drought stressed F. deltoidea for the major compounds in the leaf extracts compared to the untreated (T0) plants. The retention time (RT) and relative abundance (%) were recorded in this study. The comparison of total ion chromatograms of the control and treated groups (Figure 9) revealed the differences in percentages of 18 volatile compounds in the leaves following F. deltoidea treatment with H2O2. Thirteen (13) identified compounds with a quality score above 70% were identified, while five unmatched compounds were labeled as U1–U5 (Table 6). In this study, the aromatic volatile components detected in the native leaf extracts (T0) were mainly (E)-Caryophyllene (38.88%) at minute (min) 34.72, followed by α-Humulene (12.70%) at min 36.28, while the least amount was (E)-β-Ocimene (0.50%) at min 14.66. Other compounds detected were α-trans-Bergamotene (5.42%), α-Ylangene (3.38%), (E, E)-α-farnesene (2.94%), α-copaene (2.37%), and Germacrene D (1.26%). The volatile components detected in the leaves of the 5 mM H2O2 (T1) treated plants were mainly (E)-β-Ocimene (23.08%) at minute 14.66, followed by Germacrene D (15.86%) at minute 37.52, (E)-Caryophyllene (15.36) at minute 34.72, δ-Selinene (6.73%) at minute 37.75, and α-Copaene (1.96%) at minute 32.69, respectively (Table 6). On the other hand, the volatile components detected in the leaves of the 10 mM H2O2 (T2) treated plants were mainly Germacrene D (32.90%) at minute 37.52, followed by (E)-Caryophyllene (20.21%) at minute 34.72, α-Copaene (5.07%) at minute 32.69, δ-Cadinene (3.07%) at minute 39.38, and α-Guaiene (2.04%) at minute 38.15, respectively (Table 6). There were five (5) unidentified compounds which were also detected in the H2O2 treated plants under drought stressed conditions.
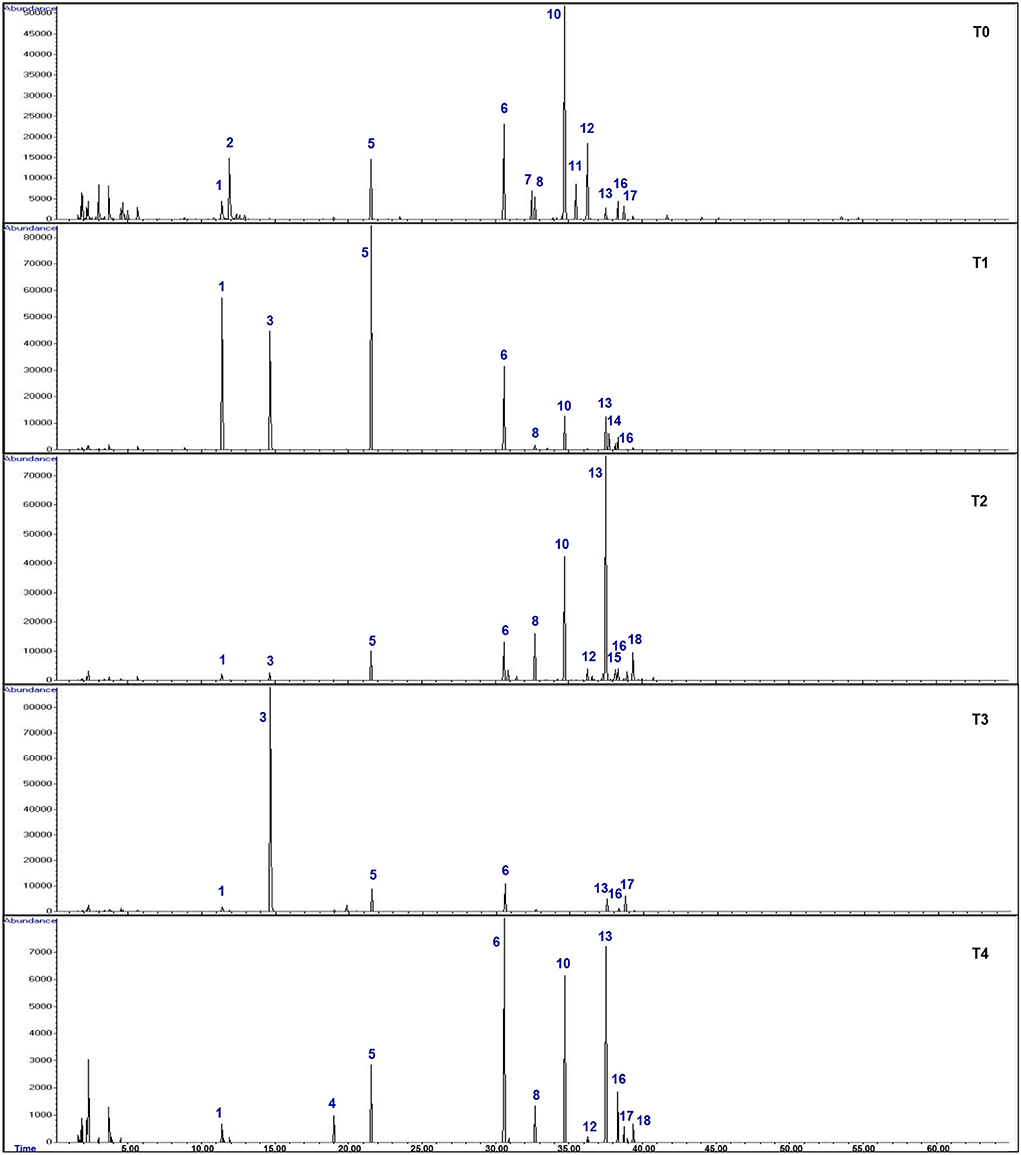
Figure 9. Gas chromatography mass spectrometry (GCMS) total ion chromatograms (TIC) of the leaf extracts of H2O2 treated and untreated F. deltoidea plants under drought stress. Thirteen (13) identified and five (5) unmatched volatile compounds with a quality score above 70% were identified, which are responsible for drought stress mitigation.
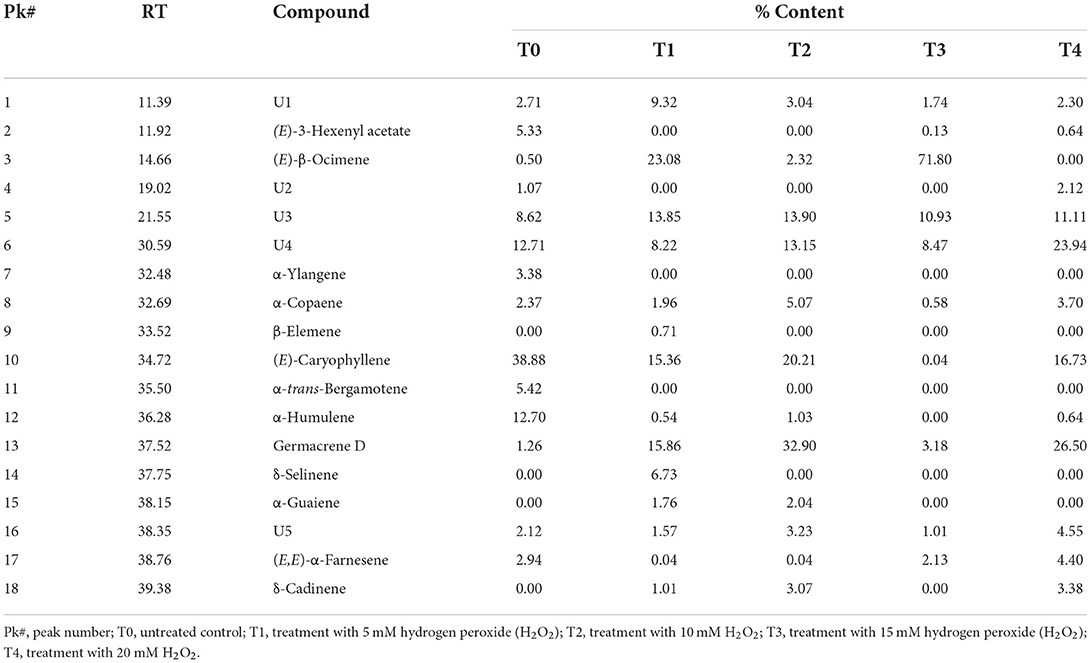
Table 6. Retention time (RT) and relative abundance (%) of the volatile compounds (identified and unknown) in the leaf extracts of drought stressed F. deltoidea plants treated and untreated with H2O2.
Heat map clustering analysis showed increasing average percentages of certain compounds (pattern; red > white > blue). These compounds were found to occur, be absent and/ or increase in F. deltoidea leaf extracts corresponding to H2O2 treatments (Figure 10). As a result, a significant increase of (E)-β-Ocimene was clearly shown after the plant underwent T1 (23.08%) and T3 (71.80%) treatments, but the percentage was lessened to trace amounts for the T4 application. Besides, T1 and T2 treatments resulted in a higher abundance of Germacrene D with respective 15.86% and 32.90% compared to the native (control) plant. Following T4, the Germacrene D amount was found to be slightly less (26.50%). T1 also ensued the occurrence of several other volatile compounds including δ-Selinene (6.73%), while T2 resulted in a higher amount of α-Guaiene (2.04%), and δ-Cadinene (3.07%). Unidentified compound abundances also occur more intensely after T1 such as presented by U1 and U3. However, some compounds including (E)-Caryophyllene, α-Humulene, α-Ylangene, and ethyl acetate were found to have decreased. Following T4, δ-Cadinene (3.38%), (E, E)-α-Farnesene (4.40%), and U4 (23.94%) relative abundance were found to be higher than the control plant without treatment.
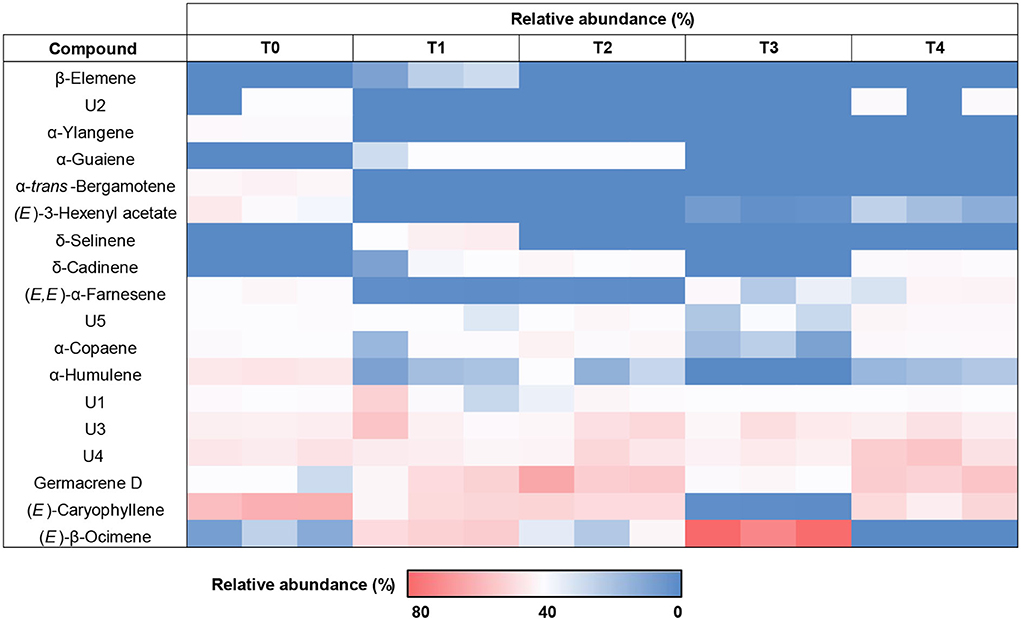
Figure 10. Clustered heat map based on increasing average relative abundance (%) of the volatile compounds (identified and unknown) in the leaf extracts of H2O2 treated F. deltoidea and untreated plants under drought stress.
Discussion
Drought stress induces negative effects in plants including morphological, physiological, molecular, and metabolic changes which need to be improved by different means for better growth and development. Our results indicate that the number of leaves increased significantly with the application of H2O2 during drought conditions. This was due to the role of H2O2 in protein initiation, as well as in plant signaling and development (Barba-Espín et al., 2011; Ralmi et al., 2021). The treatments produced enhancing effects on plant height and leaf area of the F. deltoidea, although the results were not significant. H2O2 plays a role in increasing endogenous levels or to regulate relative gene expression. Liu et al. (2013) reported that the application of H2O2 positively influenced the plant metabolism and antioxidant enzyme production leading to better plant growth. It can be noted that drought stress leads to a decrease in photosynthetic activity due to stomatal closure. This study proved that the rate of photosynthesis was significantly improved with the application of H2O2 on stressed F. deltoidea plants. H2O2 is a signaling molecule, which creates a positive response under abiotic stress, such as drought (Ashraf et al., 2015). It is obvious in this study that exogenous H2O2 enhanced drought resistance by protecting the structures of organelle under drought stress conditions. In addition, H2O2 protects chloroplast/mesophyll ultra-structure, modulates the expression of the rubisco gene, and increases the actions of antioxidant enzymes to preserve photosynthesis during drought stress conditions (Liao et al., 2012).
H2O2 application reduces the transpiration rate in stressed F. deltoidea plants to some extent, which has been confirmed from this study. The reduced transpiration rate is an indicator of high water-use efficiency. Sofo et al. (2005) reported that H2O2, being an important signaling molecule, may increase water use efficiency, the recovery of net transpiration rate, and the net photosynthetic rate of plants. Increase in stomatal conductance with the application of H2O2 is a tool to mitigate drought stress since it helps to control adaptive transpiration as reported by Hessini et al. (2008). Lawlor (1995) stated that when increasing the stress when the stomata is closed the presence of inner leaf CO2 also declines. The opening and closing of the stomata is a mechanism in plants which regulates the water loss by sacrificing CO2 uptake, especially when the environmental conditions are disparaging as reported by Arve et al. (2011). However, the results obtained from this study opposed these statements, and noted that the spraying of H2O2 at the concentrations of 15 and 20 mM could significantly improve the CO2 content in stressed F. deltoidea plants. H2O2 also plays a vital role in brassinosteroid-regulated stomatal movement (Shi et al., 2015).
As per reports of others (Santos, 2004) under abiotic stresses the leaf chlorophyll content decreases due to an inhibited synthesis of 5-aminolaevulinic acid, but this study indicates a significant increase of chlorophyll content with H2O2 treatment in the F. deltoidea plants. It has been proclaimed by Steffens et al. (2013) that the withdrawal of the negative impacts of stresses through the application of H2O2 promoted plant chlorophyll content. Improved chlorophyll content might be due to the non-enzymatic defense mechanism especially due to activity of ROS-scavenging proteins under stress conditions. It is also speculated that this might be caused by the role of H2O2 itself as a signal of oxidative stress since it changed the redox status to antioxidative status of the surrounding cells (Sairam and Srivastava, 2000). Our study indicates that relative variable fluorescence and photosynthetic yield were significantly higher in treated plants under drought field conditions. This higher photosynthetic yield is also an indication of stress removal in the presence of exogenous H2O2. Our results showed that all the plant growth and physiological parameters were positively correlated with each other in H2O2 treated F. deltoidea plants. Improved plant physiological parameters create significant effects on plant growth and development under drought-stressed conditions.
Carotenoid content of pea seedlings was reduced when they were subjected to drought conditions (Moran et al., 1994). Our results show that carotenoid content was significantly improved with the spraying of 15 and 20 mM of H2O2 on F. deltoidea stressed plants. Total phenolic and flavonoid contents were also significantly improved with the application of H2O2 in F. deltoidea plants. The possible reason for higher phenolic and flavonoid content in treated plants was probably due to the fact that H2O2 acted as a signaling molecule in phenolic synthesis (Zhang et al., 2013; Jamaludin et al., 2020). Chen et al. (2007) reported that the accumulation of proline, sugars, and polyols are responsible for plant survival under abiotic stresses. Soluble sugars also take part in signaling to regulate molecules in numerous plant growth and developmental processes. Our results demonstrate that the total sugar content of stressed F. deltoidea plants was significantly amended with the application of H2O2. The sucrose phosphate synthase (SPS) activity might be improved due to the regulatory effects of H2O2, and it regulated the manufacture of sucrose from triose phosphates during photosynthesis (Uchida et al., 2002).
We found that the spraying of H2O2 improved antioxidant activity in F. deltoidea plants under drought stress conditions. H2O2 may alleviate the level of antioxidants in plants by activating PAL, CHS, and stilbene enzymes (Nyathi and Baker, 2006). Zhang et al. (2001) suggested that under stressed conditions, when guard cells are amended with ABA, it may close the stomata through the production of H2O2, and H2O2 plays a role as intermediate in ABA signaling. This is in parallel with our results which indicate that the application of H2O2 promoted stomatal closure of F. deltoidea plants. The stomatal closure during drought stress is also related to the maintenance of xylem integrity (Jones and Sutherland, 1991).
The application of H2O2 improved the sodium (Na+) content in both leaves and syconium under drought stress. The beneficial effects of Na+ are the same as nitrogen ( and ) or K+, which regulates plant growth by improving leaf chlorophyll content, net photosynthetic rates, water use efficiency, nitrate reductase activity and relative growth rate (Brownell and Jackman, 1966; Smith et al., 1980; Redondo-Gómez et al., 2010; Gattward et al., 2012). Nitrate reductase enzyme catalyzes the reduction of nitrate N to organic forms and reflects the level of nitrogen (N) activity in plant leaves (Lane et al., 1975). Na+ also enhances nitrate uptake by roots and increases assimilation of nitrate in leaves (Ohta et al., 1989). The improved level of Na content may increase the activity of N in plants which stimulates plant growth and development in drought conditions. The photosynthetic capacity of leaves is interconnected with the nitrogen content, predominantly because the proteins of the Calvin cycle and thylakoids exemplify most of the leaf nitrogen (John, 1989). The treatment of 20 mM H2O2 produced the highest amount of K content in the plant syconium. The elevated level of K is essential in regulating the osmotic potential and water uptake in water-stressed plants. Since H2O2 is related to stomatal closure, it helps to adjust to drought stress in escalating plant resistance (WHO Regional Office for Europe, 2000). Moreover, the nutrient K, helps in galvanizing different enzyme systems and thereby regulates water movement and water use efficiency, nitrogen uptake and protein building and photosynthesis [Risk Assessment Studies (RAS), 2004]. In a study with broad beans, Kazerouni et al. (2001) observed that potassium improved the water content in the plant leaves, and it increased the tolerance to water stress. Hence, under environmental stress conditions the enhancement of K content of plants is important for the survival of crops (Cakmak, 2005).
The higher amount of Ca is a good indicator of improved plant condition under stressed conditions. The elevated level of Ca2+ in a cell acts as a signal carrier and transporter into appropriate biological responses by downstream effectors. In this study, the amount of Ca content in the leaves was found to be greater in 10 mM H2O2 treated plants. The higher amount of Ca in plants helps to alleviate the adverse effects of drought stress. The exogenous application of Ca2+ and H2O2 also recovered the physiological damage of Brassica seedlings under drought stress (Khan et al., 2017). Pei et al. (2000) reported that, H2O2 production by induction of ABA and the H2O2-activated Ca2+ channels are significant for ABA-induced stomatal closing under stress conditions. Our results indicate that magnesium (Mg) content in the leaves was significantly higher in 15 mM H2O2 treated plants. The leaf magnesium plays a decisive role in the process of photosynthesis and is one of the major contributing factors of biomass formation (Tränkner et al., 2016). Liu and Porterfield (2014) explained the role of H2O2 in alleviating the effects of stresses as they demonstrated the use of magnesium peroxide for flooded corn as an effective oxygen buffer, which generated H2O2 and released biological oxygen for the stressed plants.
Our results indicate that arsenic (As) content was increased in the 10 mM H2O2 treated leaves while the highest As content in the syconium was present in the control plants. Higher doses of arsenate reduce seed germination, plant biomass accumulation, and produce oxidative damage (Mascher et al., 2002; Shri et al., 2009). But our results showed a positive result in plant growth and development, it was suggested that the application of foliar H2O2 promoted As uptake but not to a harmful level. The antimony (Sb) content in leaves was the highest in the 20 mM H2O2 treatment, while the 10 mM H2O2 treated plants had the highest Sb content in the plant syconium. Kabata and Pendias (2001) suggested that 5–10 mg kg−1 of Sb in plant tissue are phytotoxic, but as per reports of Eikmann and Kloke (1993) 5 mg kg−1 Sb in plants is at a tolerable level. Pan et al. (2010) also noticed the reduced plant growth and biomass accumulation due to Sb pollution. Thus, although the application of H2O2 improved Sb uptake, it was not up to its toxicity level in the plants.
Our results indicate that iron (Fe) accumulation was significantly improved in the leaves and syconium with the application of H2O2. Fe deficiency leads to severe chlorosis and reduction in yield and also the nutritional content of the plants (Marschner, 2002; Curie and Briat, 2003). Additionally, Fe is helpful in many physiological processes such as photosynthesis, plant respiration, sulfate assimilation, synthesis of hormones, nitrogen fixation, DNA synthesis (Hell and Stephan, 2003; Puig et al., 2007). The improved physiological activities of treated plants may be due to the higher level of Fe in the plants. Ravet et al. (2009) demonstrated that ferritin protein in plants is responsible for controlling the levels of ROS, enzymatic activity is involved in ROS detoxification, which is a crucial role for the protection against oxidative damage in plants.
The distribution of stomata was found more frequent on the abaxial surface of leaves in treated F. deltoidea plants in comparison to the adaxial surface of the leaves, which is located on the epidermal layer of the leaf. Fu et al. (2013) testified that under drought stress conditions, stomatal density in eggplants at the abaxial surface increased by 20.39% compared to well-watered plants. As per reports of Liu et al. (2009) usually the stomatal numbers and pore aperture numbers would decrease during the periods of water stress, which is a common occurrence in Arabidopsis and grass species. Nonetheless, in some plant species it was disclosed that a decreased water level led to an increased stomatal density (Fraser et al., 2009). Moderate drought stress enhances the leaf thickness which may produce more guard cells for a given leaf area and increase stomatal density. Other than that, we suggest that the application of H2O2 could protect the leaf's margin from damage. This observation might be correlated to the function of H2O2 as a protective and signaling molecule which mediates the negative effects of drought stress in plants. Asad et al. (1998) suggested that H2O2 induced safety against lethal and mutagenic effects in Escherichia coli cells. Rose and Swift (2014) described the scorching of leaves which could appear as a spot or a burn along the leaf margin under drought stress. Hence, spraying the right amount of H2O2 could reduce the damage caused by water deficiency as shown in our results.
Thicker palisade parenchyma cells and a higher number of stomata make plants resistant to drought conditions. Moreover, our results indicate that the application of H2O2 reduced the damage of mesophyll cells in stressed plants by causing thicker mesophyll cells when compared to the untreated plants. In opposition, Sun et al. (2012) stated that tomato plants under mild stress conditions displayed a reduced palisade and spongy tissue thickness. Baum et al. (2000) noticed that abiotic stress affects the thickness of xylem and phloem cells, which regulates the xylem and phloem transport. The application of H2O2 in this study improved the leaf vein structure under drought stress field conditions. It is expected that the treated plants work better at carbon and water transportation as in plants with an improved structure of phloem and xylem. Nasir et al. (2021) also reported that a lower concentration of H2O2 improved the tracheary elements and vascular cell of Mas Cotek leaves. Additionally, treated F. deltoidea plants might lead to better cross-linking of the cells and tissue of xylem and phloem. Hamann (2012) recorded that the strength and rigidity of the cell walls are improved by the cross-linking process which is an important mechanism in plants to mitigate environmental stresses.
Rubisco is a major enzyme which assimilates atmospheric CO2 into the biosphere and is the most imperative target for improving the efficiency of photosynthesis in vascular plants (Parry et al., 2013). The progressive down-regulation of rubisco directs to a reduced RuBP content in plants during severe drought thereby inhibiting photosynthetic CO2 assimilation (Flexas and Medrano, 2002). Our results indicate that the Rubisco gene expression was upregulated because of exogenous H2O2, and we suggest that it contributed to better adaptation of the F. deltoidea var. deltoidea plants by increasing photosynthetic rates and photosynthates accumulation under drought-stress. The results also show that the rbcL gene expression ratio was the highest in the 15 mM treatment compared to the 20 mM and 0 mM H2O2 treatments. Better rbcL gene expression in the 15 mM H2O2 treatment may trigger plant growth and development in drought stress conditions. It can be speculated that the exogenous H2O2 improved the levels of gene expression leading to better relative stability in the plants (Liu et al., 2010). This indication is supported by this study, that the net photosynthetic rate of all H2O2-treated plants increased in drought stress conditions. H2O2 as a ROS growth promoting chemicals induced the largest changes in the gene expression levels in various plants and this was probably due to its relative stability (Li et al., 2009).
In this study a total of 18 volatile compounds were detected from H2O2 treated and untreated F. deltoidea plants under drought conditions. These compounds were reported to be responsible for the aroma of F. deltoidea as well as other Ficus species (Grison-Pigé et al., 2002). Among the unidentified compounds, U4 peak at min 30.59 with a significantly high relative abundance (12.71%) is suggested to be the linalool which is reported as among the major essential oils in F. deltoidea. U4 is also less volatile, and thus detected later than ocimene which corresponds to the reported linalool (Grison-Pigé et al., 2002; Lee et al., 2011). The results of heat map clustering analysis showed that the percentage of certain compounds in treated leaves increasingly corresponded to H2O2 treatment under drought conditions. This finding indicates that treatment of F. deltoidea with H2O2 influences the accumulation of the volatile oils. Such similar occurrence was reported focusing on accumulation of volatile oils mediated by H2O2 production (Wang et al., 2011).
Drought stress affects synthesis of photosynthetic pigments and protein content, electron transfer chain (ETC), rubisco and sucrose phosphate synthase (SPS) enzyme activity, and the percentage of aromatic or volatile oils of plants (Bakshi et al., 2019). Our results show that exogenous H2O2 improved plant growth processes, protected cellular structure of leaves from damage, increased mineral accumulation and the level of Rubisco gene expression under drought stress. The percentage of volatile oils content in leaves of treated plants increased significantly compared to the control plants. The reduction of volatile oils in the control plants in this study may be due to the disturbance of the photosynthesis process and reduction of photosynthates accumulation in plants under drought stress, which suppresses plant growth and development (Flexas and Medrano, 2002). The disrupted function of the Calvin cycle and depletion of leaf area due to drought stress reduces the biosynthesis of volatile oils. The reduction of volatile oils in plant parts is a consequence of drought stress (Razmjoo et al., 2008). H2O2 is a non-radicle reactive oxygen species, which due to its stability and diffusion through the plasma membrane acts as a secondary messenger and regulates the flow of Ca+2, protein synthesis and gene expression (Bienert et al., 2006).
Volatile oils belong to the group of terpenoids and are synthesized via the cytosolic mevalonic acid (MVA) pathway or methylerythritol phosphate (MEP) plasticity pathway (Perreca et al., 2020). Monoterpenes (synthesis by MEP pathway) and Sesquiterpenes (synthesis by MVA pathway) are the main constituents of volatile oils that play a role in synthesis of photosynthetic pigments, aroma, flavor, plant defense system and antioxidant activities (Pavela et al., 2021). H2O2 elevates the level of expression of saponin synthesis (SS), squalene epoxidase (SE) and β-amyrin synthase (β-AS) enzymes which regulate the biosynthesis of volatile oils (Hu et al., 2002, 2004; Kim et al., 2006). H2O2 also acts as a signaling molecule which mediates the biosynthesis of saponin in response to oligogalacturonic acid (OGA) and jasmonic acid (JA) (Zhao et al., 2010). In this study, the optimum concentrations of H2O2 (10–15 mM) may mediate the biosynthesis of volatile oils in F. deltoidea plants under drought conditions. The improved level of volatile oils plays a significant role in mitigating the negative effects of drought as well as other abiotic stresses of plants.
Conclusion
It is concluded that foliar applications of 10–15 mM of H2O2 should be applied biweekly to increase plant growth, photosynthesis, stomatal conductance, and chlorophyll fluorescence in F. deltoidea plants grown in drought stress conditions. In addition, chlorophyll, carotene, total phenols, total flavonoids, sugar content, and antioxidant activities of stressed plants are increased significantly with the H2O2 treatments leading to sustainable plant growth under drought-stressed conditions. The enhancement of macro nutrients content in the treated plants alleviates the negative effects of drought stress by improving plant physiological activities. A slight increase of arsenic and antimony at safe levels in the treated plants contributes to stress mitigation as well. Furthermore, foliar applications of H2O2 improved the cellular and vein structure of the leaves and reduced the damage in leaves caused by drought stress. Similarly, the application of 15 mM H2O2 should be done to up-regulate the expression of the Rubisco gene in stressed F. deltoidea plants to catalyze the assimilation of CO2 and increase net photosynthesis. Foliar application of H2O2 increased the accumulation of volatile oils in the plants, and the improved level of volatile oils mitigates the negative effects of drought stress. All these effects and influences help to alleviate the undesirable effects of drought stress. Our findings suggest that a foliar application of H2O2 is a novel technique to reduce the negative effects of drought stress in plants.
Data availability statement
The original contributions presented in the study are included in the article/supplementary material, further inquiries can be directed to the corresponding author.
Author contributions
MK: conceptualization, funding acquisition, supervision, data curation, and writing—original draft and editing. RJ: data curation, writing—original draft, and statistical analysis. AM, ZR, and SK: review and language editing. HA-Y, NB, MA, and KM: review, editing, and validation. All authors contributed to the article and approved the submitted version.
Funding
This research was funded by the Kementerian Pengajian Tinggi (KPT), Fundamental Research Grant Scheme (Project Code: FRGS/2/2014/SG03/UNISZA/02/1).
Acknowledgments
The authors wish to thank the Center for Research Excellence and Incubation Management (CREIM), Universiti Sultan Zainal Abidin, Campus Gong Badak, Terengganu, Malaysia, and FRGS/1/2019/WAB01/UNISZA/02/2 for the publication support. We also kindly acknowledge Taif University researchers supporting project numbers (TURSP-2020/199), Taif University, Taif, Saudi Arabia for providing partial publication support.
Conflict of interest
The authors declare that the research was conducted in the absence of any commercial or financial relationships that could be construed as a potential conflict of interest.
Publisher's note
All claims expressed in this article are solely those of the authors and do not necessarily represent those of their affiliated organizations, or those of the publisher, the editors and the reviewers. Any product that may be evaluated in this article, or claim that may be made by its manufacturer, is not guaranteed or endorsed by the publisher.
References
Aris, S. R. S., Mustafa, S., Ahmat, N., Jaafar, F. M., and Ahmad, R. (2009). Phenolic content and antioxidant activity of fruits of Ficus deltoidea var Angustifolia sp. Malay. J. Anal. Sci. 13, 146–150. Available online at: https://www.researchgate.net/publication/256089022_Phenolic_content_and_antioxidant_activity_of_fruits_of_Ficus_deltoidea_var_angustifolia_sp (accessed September 20, 2022).
Arnon, D. I. (1949). Copper enzymes in isolated chloroplasts, polyphenoxidase in Beta vulgaris. Plant Physiol. 24, 1–15. doi: 10.1104/pp.24.1.1
Arve, L. E., Torre, S., Olsen, J. E., and Tanino, K. K. (2011). Stomatal Responses to Drought Stress and Air Humidity. Biochemistry, Genetics and Molecular Biology. Abiotic Stress in Plants—Mechanisms and Adaptations. London: IntechOpen.
Asad, N. R., Asad, L. M. B. O., Silva, A. B., Felzenszwal, I., and Leitão, A. C. (1998). Hydrogen peroxide induces protection against lethal effects of cumene hydrogen peroxide in Escherichia coli cells: an Ahp independent but OxyR dependent system? Mutat. Res. 407, 253–259. doi: 10.1016/S0921-8777(98)00010-X
Ashraf, M. A., Rasheed, R., Hussain, I., Iqbal, M., Haider, M. Z., Parveen, S., et al. (2015). Hydrogen peroxide modulates antioxidant system and nutrient relation in maize (Zea mays L.) under water-deficit conditions. Arch. Agron. Soil Sci. 61, 507–523. doi: 10.1080/03650340.2014.938644
Badron, U. H., Talip, N., Mohamad, A. L., Affenddi, A. E. A., and Ahmad Juhari, A. A. (2014). Studies on leaf venation in selected taxa of the genus Ficus L. (Moraceae) in peninsular Malaysia. Trop. Life Sci. Res. 25, 111–125.
Bakshi, A., Moin, M., Madhav, M. S., and Kirti, P. B. (2019). Target of Rapamycin, a master regulator of multiple signalling pathways and a potential candidate gene for crop improvement. Plant Biol. (Stuttg) 21, 190–205. doi: 10.1111/plb.12935
Barba-Espín, G., Diaz-Vivancos, P., Job, D., Belghazi, M., Job, C., and Hernández, J. A. (2011). Understanding the role of H2O2 during pea seed germination: a combined proteomic and hormone profiling approach. Plant Cell Environ. 34, 1907–1919. doi: 10.1111/j.1365-3040.2011.02386.x
Baum, S. F., Phong, N. T., and Wendy, K. S. (2000). Effects of salinity on xylem structure and water use in growing leaves of sorghum. New Phytol. 146, 119–127. doi: 10.1046/j.1469-8137.2000.00625.x
Bienert, G. P., Schjoerring, J. K., and Jahn, T. P. (2006). Membrane transport of hydrogen peroxide'. Biochem. Biophys. Acta 1758, 994–1003. doi: 10.1016/j.bbamem.2006.02.015
Bohnert, H. J., and Sheveleva, E. (1998). Plant stress adaptations making metabolism move. Curr. Opin. Plant Biol. 1, 267–274. doi: 10.1016/S1369-5266(98)80115-5
Brownell, P. F., and Jackman, M. E. (1966). Changes during recovery from sodium deficiency in Atriplex. Plant Physiol. 41, 617–622. doi: 10.1104/pp.41.4.617
Cakmak, I. (2005). The role of potassium in alleviating detrimental effects of abiotic stresses in plants. J. Plant Nutri. Soil Sci. 168, 521–530. doi: 10.1002/jpln.200420485
Chang, C, Yang, M., Wen, H., and Chern, J. (2002). Estimation of total flavonoid content in propolis by two complementary colorimetric methods. J. Food Drug. Anal. 10, 178–182. doi: 10.38212/2224-6614.2748
Cheeseman, J. (2007). Hydrogen peroxide and plant stress: a challenging relationship. Plant Stress 1, 4–15. Available online at: https://www.life.illinois.edu/cheeseman/main/publications/peroxidePubs/PlStressH2O2review.pdf (accessed September 20, 2022).
Chen, Z., Cuin, T. A., Zhou, M., Twomey, A., Naidu, B. P., and Shabala, S. (2007). Compatible solute accumulation and stress-mitigating effects in barley genotypes contrasting in their salt tolerance. J. Exp. Bot. 58, 4245–4255. doi: 10.1093/jxb/erm284
Curie, C., and Briat, J. F. (2003). Iron transport and signaling in plants. Ann. Rev. Plant Biol. 54, 183–206. doi: 10.1146/annurev.arplant.54.031902.135018
Dramant, S., Aris, M. A. M., Akter, S. F. U., Azlina, H., Nor Azlina, A. R., and Norazlanshah, H. (2012). F. deltoidea: a possible supplement for type II diabetes: (a pilot study). Pertanika J. Trop. Agril. Sci. 35, 93–102. Available online at: http://www.pertanika.upm.edu.my/pjtas/browse/regular-issue?article=JTAS-0211-2009 (accessed September 20, 2022).
Dubois, M, K., Gilles, J., Hamilton, K., Robers, P. A., and Smith, F. (1956). A colorimetric method for the determination of sugar and related substances. Anal. Chem. 28, 350–356. doi: 10.1021/ac60111a017
Eikmann, T., and Kloke, A. (1993). “Nutzungs- und schutzgutbezogene Orientierungswerte fur (Schad-) stoffe in Boden,” in Bodenschutz. Ergänzbares Handbuch der Maßnahmen und Empfehlungen für Schutz, Pflege und Sanierung von Böden, Landschaft und Grundwasser-1, Band, 14 Lfg X/93, eds D. Rosenkranz, G. Bachmann, G. Einsele, H. M. Harress (Berlin: Erich Schmidt).
Fasihuddin, B. A., and Din, L. B. (2002). Medicinal Plants Used by Various Ethnic Groups in sabah. Paper Presented at the French Malaysian-Symposium on Natural Products. Dept. of Chemistry, University of Malaya, Kuala Lumpur, p. 85
Flexas, J., and Medrano, H. (2002). Drought-inhibition of photosynthesis in C3 plants: stomatal and non-stomatal limitations revisited. Anal. Bot. 89, 183–189. doi: 10.1093/aob/mcf027
Fraser, L. H., Carlyle, C., Turkington, R., and Friedman, C. R. (2009). Adaptive phenotypic plasticity of Pseudoroegneria spicata: response of stomatal density, leaf area and biomass to changes in water supply and increased temperature. Ann Bot. 103, 769–775. doi: 10.1093/aob/mcn252
Fu, Q. S., Yang, R. C., Wang, H. S., Zhao, B., Zhou, C. L., Ren, S. X., et al. (2013). Leaf morphological and ultrastructural performance of eggplant (Solanum melongena L.) in response to water stress. Photosynthetica 51, 109–114. doi: 10.1007/s11099-013-0005-6
Gattward, J. N., Almeide, A. A. F., Souza, J. O., Gomes, F. P., and Kronzucker, H. J. (2012). Sodium-potassium synergism in Theobroma cacao: stimulation of photosynthesis, water-use efficiency and mineral nutrition. Physiol. Plant. 146, 350–362. doi: 10.1111/j.1399-3054.2012.01621.x
Grison-Pigé, L., Hossaert-McKey, M., and Bessiere, J. M. (2002). Fig volatile compounds—a first comparative study. Phytochemistry 61, 61–71. doi: 10.1016/S0031-9422(02)00213-3
Hamann, T. (2012). Plant cell wall integrity maintenance as an essential component of biotic stress response mechanisms. Front. Plant Sci. 3, 77. doi: 10.3389/fpls.2012.00077
Hasham, R., Choi, H. K., Sarmidi, M. R., and Park, C. S. (2013). Protective effects of a Ficus deltoidea extract against UVB-induced photo ageing in skin cells. Biotechnol. Bioprocess Eng. 18, 185–193. doi: 10.1007/s12257-012-0353-2
Hell, R., and Stephan, U. W. (2003). Iron uptake, trafficking and homeostasis in plants. Planta. 216, 541–551. doi: 10.1007/s00425-002-0920-4
Hessini, K., Ghandour, M., Albouchi, A., Soltani, A., Werner, K. H., and Abdelly, C. (2008). Biomass production, photosynthesis, and leaf water relations of Spartina alterniflora under moderate water stress. J. Plant Res. 121, 311–318. doi: 10.1007/s10265-008-0151-2
Hu, X. Y., Fang, J. Y., Cai, W. M., and Tang, Z. C. (2004). Oxygen burst and saponin synthesis of Panax ginseng cells induced by chitosan mediated by mitogen-activated protein kinase. Sci. China Series C Life Sci. 34, 3l−40. doi: 10.1360/03yc0074
Hu, X. Y., Zhang, W. Q., Fang, J. Y., Cai, W. M., and Tang, Z. C. (2002). Chitosan treatment raises the accumulation of saponin and the transcriptional level of genes encoding the key enzymes of saponin synthesis in cultured Panax ginseng cells. J. Plant Physiol. Mol. Biol. 28, 485–490. Available online at: https://europepmc.org/article/cba/539300 (accessed September 20, 2022).
Ishibashi, Y., Yamaguchi, H., Yuasa, T., Iwaya-Inoue, M., Arima, S., and Zheng, S. H. (2011). Hydrogen peroxide spraying alleviate drought stress in soybean plants. J. Plant Physiol. 168, 1562–1567. doi: 10.1016/j.jplph.2011.02.003
Ismail, S. Z., Khandaker, M. M., Mat, N., and Boyce, A. N. (2015). Effects of hydrogen peroxide on growth, development and quality of fruits: a review. J. Agronomy 14, 331–336. doi: 10.3923/ja.2015.331.336
Jamaludin, R., Mat, N., Mohd, K. S., Badaluddin, N. A., Mahmud, K., Sajili, M. H., et al. (2020). Influence of exogenous hydrogen peroxide on plant physiology, leaf anatomy and rubisco gene expression of the Ficus deltoidea Jack var. Deltoidea. Agronomy 10, 497. doi: 10.3390/agronomy10040497
John, R. E. (1989). Photosynthesis and nitrogen relationships in leaves of C3 plants. Aecologia 78, 9–19. doi: 10.1007/BF00377192
Jones, H. G., and Sutherland, R. A. (1991). Stomatal control of xylem embolism. Plant Cell. Environ. 14, 607–612. doi: 10.1111/j.1365-3040.1991.tb01532.x
Kabata, P. A., and Pendias, H. (2001). Trace elements in Soils and Plants. 3rd edn. Boca Raton, FL: CRC Press.
Kar, R. K. (2011). Plant responses to water stress: role of reactive oxygen species. Plant Signal. Behav. 6, 1741–1745. doi: 10.4161/psb.6.11.17729
Kazerouni, N., Sinha, R., Che-Han, H., Greenberg, A., and Rothman, N. (2001). Analysis of 200 items for benzo[a]pyrene and estimation of its intake in an epidemiologic study. Food Chem. Toxicol. 39, 423–436. doi: 10.1016/S0278-6915(00)00158-7
Khan, A., Anwar, Y., Hasan, M. M., Iqbal, A., Ali, M., Alharby, H. F., et al. (2017). Attenuation of Drought Stress in Brassica Seedlings with Exogenous Application of Ca2+ and H2O2. Plants 6, 20. doi: 10.3390/plants6020020
Khandaker, M. M., Boyce, A. N., and Osman, N. (2012). The influence of hydrogen peroxide on the growth, development and quality of wax apple (Syzygium samarangense, [Blume] Merrill & L. M. Perry var. jambu madu) fruits. Plant Physiol. Biochem. 53, 101–110. doi: 10.1016/j.plaphy.2012.01.016
Kim, Y. S., Cho, J. H., Ahn, J., and Hwang, B. (2006). Upregulation of isoprenoid pathway genes during enhanced saikosaponin biosynthesis in the hairy roots of Bupleurum falcatum. Mol. Cells 22, 269–274.
Kumawat, K. R., and Sharma, N. K. (2018). Effect of drought stress on plants growth. Popular Kheti 6, 239–241.
Lane, H. C., Thompson, A. C., Hesketh, J. D., and Sloane, C. (1975). “Some observations on nitrate reduction in cotton. III,” in Proc. Beltwide Cotton Production Res. Conf. ed J. Brown (Memphis, TN: National Cotton Council)
Lawlor, D. W. (1995). “The effects of water deficit on photosynthesis,” in Environment and Plant Metabolism. Flexibility and Acclimation, ed N. Smirnoff (Oxford: BIOS Scientific Publishers).
Lee, S. W., Wee, W., Yong, J. F. S., and Syamsumir, D. F. (2011). Characterization of antioxidant, antimicrobial, anticancer property and chemical of Ficus deltoidea Jack leaf extract. J. Biol. Active Products Nat. 1, 1–6. doi: 10.1080/22311866.2011.10719067
Li, Z., Wakao, S., Fischer, B. B., and Niyogi, K. K. (2009). Sensing and responding to excess light. Ann. Rev. Plant Biol. 60, 239–260. doi: 10.1146/annurev.arplant.58.032806.103844
Liao, W. B., Huang, G. B., Yu, J. H., and Zhang, M. L. (2012). Nitric oxide and hydrogen peroxide alleviate drought stress in marigold explants and promote its adventitious root development. Plant Physiol. Biochem. 58, 6–15. doi: 10.1016/j.plaphy.2012.06.012
Liu, G., and Porterfield, D. M. (2014). Oxygen enrichment with magnesium peroxide for minimizing hypoxic stress of flooded corn. J. Plant Nutr. Soil Sci. 177, 733–740. doi: 10.1002/jpln.201300424
Liu, J., Macarisin, D., Wisniewski, M., Sui, Y., By, S., and Norelli, J., et al. (2013). Production of hydrogen peroxide and expression of ros-generating genes in peach flower petals in response to host and non-host fungal pathogens. Plant Pathol. 62, 820–828. doi: 10.1111/j.1365-3059.2012.02683.x
Liu, T., Ohashi-Ito, K., and Bergmann, D. C. (2009). Orthologs of Arabidopsis thaliana stomatal bHLH genes and regulation of stomatal development in grasses. Development 136, 2265–2276. doi: 10.1242/dev.032938
Liu, Y., Ye, N., Liu, R., Chen, M., and Zhang, J. (2010). H2O2 mediates the regulation of ABA catabolism and GA biosynthesis in Arabidopsis seed dormancy and germination. J. Exp. Bot. 61, 2979–2990. doi: 10.1093/jxb/erq125
Mascher, R., Lippmann, B., Holzinger, S., and Bergmann, H. (2002). Arsenate toxicity: effects on oxidative stress response molecules and enzymes in red clover plants. Plant Sci. 163, 961–969. doi: 10.1016/S0168-9452(02)00245-5
Misbah, H., Abdul Aziz, A., and Aminudin, N. (2013). Antidiabetic and antioxidant properties of F. deltoidea fruit extracts and fractions. BMC Complement. Altern. Med. 13, 118. doi: 10.1186/1472-6882-13-118
Moran, J. F., Manuel, B., Iñaki, I. O., Silvia, F., Robert, V. K., and Pedro, A. T. (1994). Drought induces oxidative stress in pea plants. Planta 194, 346–352. doi: 10.1007/BF00197534
Musa, Y. (2005). Variability in morphology and agronomy of mas cotek accessions found in Kelantan and Terengganu. Bul. Teknol. Tanam. 2, 35–48. Available online at: http://hari-inovasi.mardi.gov.my/2020/docu/Pemenang/New%20MSTE%20Extended%20Abstract%20.pdf (accessed September 20, 2022).
Nashriyah, M., Zaini, H., Mazleha, M., and Abdul, K. W. (2006). Mineral uptake by taro (Colocasia esculenta) in swamp agroecosystem following gramoxone® (paraquat) herbicide spraying. J. Nuclear Related Technol. 3, 59–68. Available online at: https://www.researchgate.net/publication/235951060_MINERAL_UPTAKE_BY_TARO_COLOCASIA_ESCULENTA_IN_SWAMP_AGROECOSYSTEM_FOLLOWING_GRAMOXONE_R_PARAQUAT_HERBICIDE_SPRAYING (accessed September 20, 2022).
Nasir, N. N. N. M., Khandaker, M. M., Mohd, K. S., Badaluddin, N. A., Osman, N., and Mat, N. (2021). Effect of hydrogen peroxide on plant growth, photosynthesis, leaf histology and rubisco gene expression of the Ficus deltoidea Jack Var. deltoidea Jack. J. Plant Growth Regul. 40, 1950–1971. doi: 10.1007/s00344-020-10243-9
Nazarni, C. I. M., Ajit, A., Naila, A., and Sulaiman, A. Z. (2018). Effect of microwave assisted hydro-distillation extraction on extracts of Ficus deltoidea. Mater. Today Proc. 2018, 5, 21772–21779. doi: 10.1016/j.matpr.2018.07.031
Neill, S., Desikan, R., and Hancock, J. (2002). Hydrogen peroxide signalling. Curr. Opin. Plant Biol. 5, 388–395. doi: 10.1016/S1369-5266(02)00282-0
Nihayah, M., Yong, K. W., and Faizah, A. B. (2012). Determination of mineral content in the Ficus deltoidea leaves. J. Sci. Health Malaysia. 10, 25–29. Available online at: http://journalarticle.ukm.my/6365/1/25-29.pdf (accessed September 20, 2022).
Nurdiana, S., Goh, Y. M., Hafandi, A., Dom, S. M., Nur Syimal'ain, A., Noor Syaffinaz, N. M., et al. (2018). Improvement of spatial learning and memory, cortical gyrification patterns and brain oxidative stress markers in diabetic rats treated with Ficus deltoidea leaf extract and vitexin. J. Trad. Complement. Med. 8, 190–202. doi: 10.1016/j.jtcme.2017.05.006
Nyathi, Y., and Baker, A. (2006). Plant peroxisomes as a source of signaling molecules. Biochim. Biophys. Acta 1763,1478–1495. doi: 10.1016/j.bbamcr.2006.08.031
Ohta, D., Yasuoka, S., Matoh, T., and Takahashi, E. (1989). Sodium stimulates growth of Amaranthus tricolor L. plants through enhanced nitrate assimilation. Plant Physiol. 89, 1102–1105. doi: 10.1104/pp.89.4.1102
Orabi, S. A., Dawood, M. G., and Salman, S. R. (2015). Comparative study between the physiological role of hydrogen peroxide and salicylic acid in alleviating the harmful effect of low temperature on tomato plants grown under sand-ponic culture. Sci. Agric. 9, 49–59. doi: 10.15192/PSCP.SA.2015.1.9.4959
Ozaki, K., Uchida, A., Takabe, T., Shinagawa, F., Tanaka, Y., Takabe, T., et al. (2009). Enrichment of sugar content in melon fruits by hydrogen peroxide treatment. Plant Physiol. 166, 569–578. doi: 10.1016/j.jplph.2008.08.007
Pan, X., Zhang, D., Chen, X., Bao, A., and Li, L. (2010). Antimony accumulation, growth performance, antioxidant defense system and photosynthesis of zea mays in response to antimony pollution in soil. Water, Air Soil Poll. 215, 517–523. doi: 10.1007/s11270-010-0496-8
Parry, M. A. J., Andralojc, P. J., Scales, J. C., Salvucci, M. E., Carmo-Silva, A. E., Alonso, H., et al. (2013). Rubisco activity and regulation as targets for crop improvement. J. Exp. Bot. 64, 717–730. doi: 10.1093/jxb/ers336
Pavela, R., Maggic, F., Giordanid, C., Cappellaccic, L., Petrellic, R., Canalee, A., et al. (2021). Insecticidal activity of two essential oils used in perfumery (ylang and frankincense). Nat. Prod. Res. 108, 137–147. doi: 10.1080/14786419.2020.1715403
Pei, Z. M., Murata, Y., Benning, G., Thomine, S., Klusener, B., Allen, G. J., et al. (2000). Calcium channels activated by hydrogen peroxide mediate abscisic acid signalling in guard cells. Nature 406, 731–734. doi.10.1038/35021067doi: 10.1038/35021067
Perreca, E., Rohwer, J., González-Cabanelas, D., Loreto, F., Schmidt, A., Gershenzon, J., et al. (2020). Effect of drought on the methylerythritol 4-phosphate (MEP) pathway in the isoprene emitting conifer Picea glauca. Front. Plant Sci. 11, 546295. doi: 10.3389/fpls.2020.546295
Puig, S., Andres-Colas, N., Garcia-Molina, A., and Pefiarrubia, L. (2007). Copper and iron homeostasis in Arabidopsis: responses to metal defincies, interactions and biotechnological applications. Plant Cell Environ. 30, 27–290. doi: 10.1111/j.1365-3040.2007.01642.x
Ralmi, N. H. A. A., Khandaker, M. M., Mohd, K. S., Majrashi, A., Fallatah, A. M., Badaluddin, N. A., et al. (2021). Influence of rhizopheric H2O2 on growth, mineral absorption, root anatomy and nematode infection of Ficus deltoidea. Agronomy 11, 704. doi: 10.3390/agronomy11040704
Ravet, K., Touraine, B., Boucherez, J., Briat, J. F., Gaymard, F., and Cellier, F. (2009). Ferritins control interaction between iron homeostasis and oxidative stress in Arabidopsis. Plant J. 57, 400–412. doi: 10.1111/j.1365-313X.2008.03698.x
Razmjoo, K., Heydarizadeh, P., and Sabzalian, M. R. (2008). Effect of salinity and drought stresses on growth parameters and essential oil content of Matricaria chamomila. Int. J. Agric. Biol. 10, 451–454. doi: 10.63/ASB/2008/10-4-451-454
Redondo-Gómez, S., Mateos-Naranjo, E., Figueroa, M. E., and Davy, A. J. (2010). Salt stimulation of growth and photosynthesis in an extreme halophyte, Arthrocnemum macrostachyum. Plant Biol. 12, 79–87. doi: 10.1111/j.1438-8677.2009.00207.x
Risk Assessment Studies (RAS) (2004). Polycyclic Aromatic Hydrocarbons in Barbecued Meat. Report No., 14. Food and Environmental Hygiene Department, the Government of the Hong Kong Special Administrative Region.
Rohini, G. H. G., Hariprasad, P., Brijesh, S. S., and Niranjana, S. R. (2016). Biological control of Phomopsis leaf blight of brinjal (Solanum melongena L.) with combining phylloplane and rhizosphere colonizing beneficial bacteria. Biol. Cont. 101, 123–129. doi: 10.1016/j.biocontrol.2016.05.007
Rose, S., and Swift, C. E. (2014). Leaf Scorch—Colorado State University Extension. Available online at: https://extension.colostate.edu/docs/pubs/garden/02911.pdf (accessed May 24, 2022).
Sairam, R. K., and Srivastava, G. C. (2000). Induction of oxidative stress and antioxidant activity by hydrogen peroxide treatment in tolerant and susceptible wheat genotypes. Biol. Plant. 43, 381–386. doi: 10.1023/A:1026730008917
Santos, C. V. (2004). Regulation of chlorophyll biosynthesis and degradation by salt stressed in sunflower leaves. Sci. Hort. 103, 93–99. doi: 10.1016/j.scienta.2004.04.009
Sharma, A., Kumar, V., Shahzad, B., Ramakrishnan, M., Sidhu, G. P. S., Bali, A. S., et al. (2020). Photosynthetic response of plants under different abiotic stresses: a review. J. Plant Growth Regul. 39, 509–531. doi: 10.1007/s00344-019-10018-x
Shi, K., Li, X., Zhang, H., Zhang, G., Liu, Y., Zhou, Y., et al. (2015). Guard cell hydrogen peroxide and nitric oxide mediate elevated CO2-induced stomatal movement in tomato. New Phytol. 208, 342–353. doi: 10.1111/nph.13621
Shri, M., Kumar, S., Prabodh, D. C., Trivedi, K., Mallick, S., Misra, P., et al. (2009). Effect of arsenic on growth, oxidative stress, and antioxidant system in rice seedlings. Ecotoxicol. Environ. Safety 72, 1102–1110. doi: 10.1016/j.ecoenv.2008.09.022
Singleton, V., and Rossi, J. A. J. (1965). Colorimetry of total phenolic with phosphomolybdic—phosphotungstic acid reagents. Am. J. Enol. Viticult. 16, 144–158.
Smith, G. S., Middleton, K. R., and Edmonds, A. S. (1980). Sodium nutrition of pasture plants. II. Effects of sodium chloride on growth, chemical composition and reduction of nitrate nitrogen. New Phytol. 84, 613–622. doi: 10.1111/j.1469-8137.1980.tb04775.x
Sofo, A., Tuzio, A. C., Dichio, B., and Xiloyannis, C. (2005). Influence of water deficit and rewatering on the components of the ascorbate-glutathione cycle in four interspecific Prumus hybrids. Plant Sci. 169, 403–412. doi: 10.1016/j.plantsci.2005.04.004
Starr, F., Starr, K., and Loope, L. (2003). Ficus deltoidea. Maui, HI: United States Geological Survey-Biological Resources Division, Haleakala Field Station.
Steffens, B., Steffen-Heins, A., and Sauter, M. (2013). Reactive oxygen species mediate growth and death in submerged plants. Front. Plant Sci. 4, 1–7. doi: 10.3389/fpls.2013.00179
Sun, S. J., Li, J. M., Zong, W. J., Yao, Y. Z., and Chen, K. L. (2012). Effects of sub-low temperature and drought stress on root morphology and leaf structure of tomato seedlings. Ying Yong Sheng Tai Xue Bao. 23, 3027–3032.
Tränkner, M., Jákli, B., Tavakol, E., Geilfus, C. M., Cakmak, I., Dittert, K., et al. (2016). Magnesium deficiency decreases biomass water-use efficiency and increases leaf water-use efficiency and oxidative stress in barley plants. Plant Soil 406, 409–423. doi: 10.1007/s11104-016-2886-1
Uchida, A., Jagendorf, A. T., Hibino, T., Takabe, T., and Takabe, T. (2002). Effects of hydrogen peroxide and nitric oxide on both salt and heat stress tolerance in rice. Plant Sci. 163, 515–523. doi: 10.1016/S0168-9452(02)00159-0
Wang, B., Chen, J., Chen, L., Wang, X., Wang, R., Ma, L., et al. (2015). Combined drought and heat stress in Camellia oleifera cultivars: leaf characteristics, soluble sugar and protein contents, and Rubisco gene expression. Trees 29,1483–1492. doi: 10.1007/s00468-015-1229-9
Wang, Y., Dai, C. C., Zhao, Y. W., and Peng, Y. (2011). Fungal endophyte-induced volatile oil accumulation in Atractylodes lancea plantlets is mediated by nitric oxide, salicylic acid and hydrogen peroxide. Process Biochem. 46, 730–735. doi: 10.1016/j.procbio.2010.11.020
Zhang, F., Wan, X., and Zhong, Y. (2013). Nitrogen as an important detoxification factor to cadmium stress in poplar plants. J. Plant Interact. 9, 249–258. doi: 10.1080/17429145.2013.819944
Zhang, X., Lin, Z., Facai, D., Junfeng, G., David, W. G., and Chun-Peng, S. (2001). Hydrogen peroxide is involved in abscisic acid-induced stomatal closure in Vicia faba. Plant Physiol. 126, 1438–1448. doi: 10.1104/pp.126.4.1438
Keywords: Ficus deltoidea, H2O2, drought, growth, physiology, Rubisco, metabolites
Citation: Khandaker MM, Jamaludin R, Majrashi A, Rashid ZM, Karim SMR, Al-Yasi HM, Badaluddin NA, Alenazi MM and Mohd KS (2022) Enhancing Rubisco gene expression and metabolites accumulation for better plant growth in Ficus deltoidea under drought stress using hydrogen peroxide. Front. Plant Sci. 13:965765. doi: 10.3389/fpls.2022.965765
Received: 10 June 2022; Accepted: 02 August 2022;
Published: 30 September 2022.
Edited by:
Mohammad Anwar Hossain, Bangladesh Agricultural University, BangladeshReviewed by:
Hesham Alharby, King Abdulaziz University, Saudi ArabiaShyamal Krishna Talukder, Texas A&M University, United States
Copyright © 2022 Khandaker, Jamaludin, Majrashi, Rashid, Karim, Al-Yasi, Badaluddin, Alenazi and Mohd. This is an open-access article distributed under the terms of the Creative Commons Attribution License (CC BY). The use, distribution or reproduction in other forums is permitted, provided the original author(s) and the copyright owner(s) are credited and that the original publication in this journal is cited, in accordance with accepted academic practice. No use, distribution or reproduction is permitted which does not comply with these terms.
*Correspondence: Mohammad Moneruzzaman Khandaker, bW9uZXJ1enphbWFuQHVuaXN6YS5lZHUubXk=