- 1Department of Biotechnology, University of Verona, Verona, Italy
- 2Dipartimento Politecnico di Ingegneria e Architettura, University of Udine, Udine, Italy
The application of synthetic Fe-chelates stands for the most established agronomical practice to alleviate lime-induced chlorosis, which still constitutes a major agronomic problem. However, the percolation through the soil profile due to the negative charge of the most deployed molecules results in agronomical and environmental problems. H2bpcd/Fe3+ complex features distinctive chemical characteristics, including moderate stability of the Fe(bpcd)+ species (logβML = 20.86) and a total positive charge, and we studied its behavior in soil and regreening effects on cucumber plants. Soil column experiments have underlined that H2bpcd/Fe3+ is retained in more amounts than EDDHA/Fe3+. The new ligand was not proven to be toxic for the cucumber and maize seedlings. A concentration of 20 μM H2bpcd/Fe3+ attained regreening of Fe-deficient cucumber plants grown in the hydroponic solution supplied with CaCO3, similar to that shown by EDDHA/Fe3+. Experiments with a 2 μM concentration of 57Fe showed that cucumber roots absorbed H2bpcd/57Fe3+ at a slower rate than EDTA/57Fe3+. The high kinetic inertness of H2bpcd/Fe3+ may explain such behavior.
Introduction
Among all metal micronutrients, iron (Fe) is a metal that is required in the largest amount by plants and plays a key role in the fundamental metabolic processes, such as respiration, photosynthesis, and chlorophyll biosynthesis (Broadley et al., 2012). Albeit its relatively high abundance in the Earth’s cultivated soils, Fe bioavailability is often scarce, particularly in calcareous soils, which cover over 30% of the Earth’s land surface (Chen and Barak, 1982). The alleviation of Fe chlorosis and lime-induced chlorosis still stands as a major agronomic problem. The main approaches to alleviate Fe deficiency include the increase of soil’s indigenous Fe availability, the improvement of plant efficiency in Fe uptake and translocation, and the supply to plants with external sources of Fe (Shenker and Chen, 2005). This last technique is the most widely used. Fe fertilizers are commonly applied either to the soil or to the foliage. Fe fertilizers comprise Fe inorganic salts, synthetic Fe3+-chelates (synthetic products of medium-high stability), and a selected number of Fe complexes with low stability (López-Rayo et al., 2019). The usage of Fe salts is deterred by their scarce uptake in leaves, further re-translocation, and rapid precipitation under neutral–alkaline pH conditions featuring in calcareous soils. Though the usage of nanosized materials has recently changed the view about the application of Fe salts to plants (Sánchez-Alcalá et al., 2012; Sega et al., 2019), their adoption is yet to be widespread. Since the 1950s, plants have been treated with Fe3+-chelates (Weinstein et al., 1954; Hill-Cottingham and Lloyd-Jones, 1957). A chelate consists of a hybrid organic–inorganic molecule that is formed when a polydentate ligand bonds to a central metal ion. Fe-chelating agents used in agriculture are generally derived from polyaminocarboxylic acids. Well-known examples feature ethylenediamine tetraacetic acid (EDTA) and ethylenediamine-N,N′-bis(o-hydroxyphenylacetic) acid (o,o-EDDHA) (Figure 1), with the latter being the most effective even in calcareous soils (Lucena, 2003; Abadía et al., 2011).
However, these molecules present several problems: percolation through the soil profile due to their total negative charge, little biodegradability, high cost, and synthesis of by-products whose identity is sometimes unknown. Their noteworthy scarce residual effect often demands repeated treatments, thereby not representing a sustainable solution for Fe chlorosis in plants (Rombolà and Tagliavini, 2006). Hence, the number of studies regarding the synthesis of new ligands and application in plant Fe nutrition has increased in the last 5 years (Santos et al., 2016; Martín-Fernandez et al., 2017; López-Rayo et al., 2019; Valverde et al., 2021).
Engineering a Fe-chelate to act as a reservoir can overcome the percolation issues, since a slow-release fertilizer increases the presence of Fe in the soil solution in the long term. This feature is strongly related to the sorption mechanisms of Fe-chelates on soil surfaces, which has been well-reviewed by Lucena (2006). A slow Fe release seems possible whenever the chelate features moderate stability allowing it to “react” with the treated soil. Indeed, less stable chelates can lose some of their links with Fe3+ more easily (i.e., the ones with the carboxyl groups) and subsequently interact with the soil surface (Hernández-Apaolaza and Lucena, 2001). While the highly stable o,o-EDDHA/Fe3+ chelate (logβML = 35.09)1 (Yunta et al., 2003) interacts weakly with the soil and concurrently lies in the soil solution in high concentrations, the less stable EDTA/Fe3+ chelate (logβML = 25.1) represents a more ideal candidate. Nevertheless, this chelate is not able to hold the Fe3+ ion available to the plant in calcareous soils, in which all the Fe precipitates as Fe(OH)3 due to the Ca displacement from the Fe-chelate (Lucena, 2006).
In this work, we propose a new Fe-chelate based on the racemic form of the ligand N,N′-bis(2-pyridylmethyl)-trans-1,2-diaminocyclohexane N,N′-diacetate (H2bpcd; Figure 2) as Fe-EDTA-like molecule capable to interact effectively with the soil, thanks to both its moderate stability (logβML = 20.86) and a total positive charge. Hence, it is expected that [Fe(bpcd)]+ should be strongly retained by the negatively charged soil particles. These particles prevail in neutral alkaline soils, thereby enabling a progressive Fe release at the rhizosphere via desorption and/or Fe3+ reduction (Strategy I plants) or ligand exchange (Strategy II plants). Therefore, we studied the behavior and performance of [Fe(bpcd)]+ in simplified systems, such as soil columns and hydroponic culture.
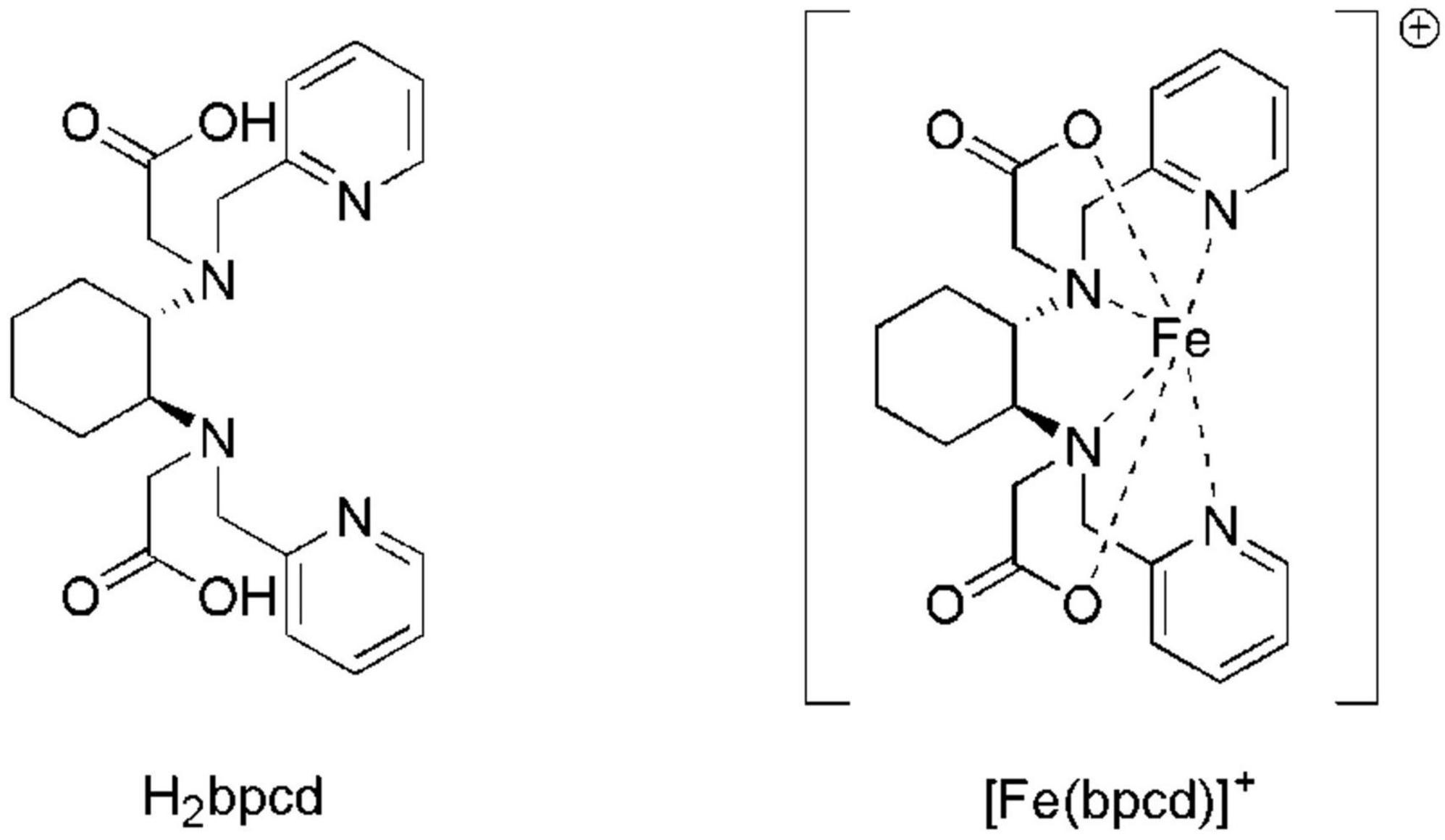
Figure 2. Molecular structures of H2bpcd and [Fe(bpcd)]+, the molecules synthesized and discussed in the present contribution. The racemic form of the ligand has been employed.
Materials and methods
N,N′-bis(2-pyridylmethyl)-trans-1,2-diaminocyclohexane N,N′-diacetate (H2bpcd) synthesis
(±)-N,N′-bis(2-pyridylmethyl)-trans-1,2-diaminocyclohexane N,N′-diacetate (H2bpcd) was synthesized as described by a previous study (Oddon et al., 2012).
Synthesis of the Fe3+ complex, H2bpcd/Fe3+
H2bpcd trifluoroacetate salt (300 mg, 0.7278 mmol) was dissolved in ethanol (60 mL) under an inert atmosphere (Ar). FeCl3⋅6H2O (196.7 mg, 1 eq) was added to the ligand solution under vigorous stirring. Then, potassium acetate (357.2 mg, 5 eq) dissolved in 30 mL of ethanol was added to the solution. The solution was left for 30 min at 95°C under reflux and later was allowed to cool down. The solution was stored for 2 h at −20°C and then centrifuged at 4,500 rcf for 15 min at 0°C, to separate the inorganic salts. The resulting supernatant was dried under a vacuum. The obtained brownish solid was triturated with diethyl ether several times to remove all of the residual trifluoroacetic acid and water. The resulting yellowish solid was left overnight under a vacuum. The obtained product weighed 467.7 mg with a yield of 82%. Elemental Anal. Calc. for C24H29FeN4O6 (MW 525,36): C, 54,87; H, 5.56; N, 10.66; O, 18.27. Found C, 54.20; H, 5.48; N, 10.50; O, 18.52. ESI-MS (Scan ES+; m/z) in methanol: 520.30 (100%), 521.25 (26%); [Fe(bpcd) (OCH3)Na]+; 498.47 (100%), 499.14 (26%); [Fe(bpcd) (OCH3)H]+.
In the case of the synthesis of H2bpcd/57Fe3+, a slight excess of 6M hydrochloric acid (HCl) was added to 57Fe(III) oxide(57Fe2O3) to produce 57Fe(III) chloride (57FeCl3) according to the following reaction:
The solution was then heated slightly to eliminate excess HCl. Then the compound was freeze-dried for a day to remove the excess water, and 57FeCl3⋅2H2O was finally isolated. To obtain [57Fe(bpcd)](CH3COO) complex, we used the same synthetic protocol adopted for [Fe(bpcd)](CH3COO) molecule. A similar chemical yield was obtained. The complex was analyzed by ICP-MS to determine the purity (82.4% of [57Fe(bpcd)](CH3COO) and 17.6% of potassium acetate).
Synthesis of EDTA/57Fe3+
EDTA/57Fe3+ was synthesized as described by Loots et al. (2007).
Speciation in solution
The standard solutions of Fe3+were prepared by dissolving the required amount of chloride salt (Sigma) in Milli-Q water and adding a stoichiometric amount of HCl to prevent hydrolysis. The NaOH and HCl stock solutions were prepared with Fixanal (Fluka Analytical) and Milli-Q water (>18 MΩ cm) in a Milli-Q system (ELGA Purelab UHQ). The Fe3+ stock solution was standardized by spectrophotometric determination of the DFO (Desferal) complex (Cappai et al., 2018). Spectrophotometric data were collected (220–360 nm) for a series of solutions in the pH range of 0–2. Spectrophotometric data were analyzed using the HypSpec program (Gans et al., 1996). Ligand protonation constants were taken from Leonzio et al. (2017) and kept constant during the refinement procedure.
The potentiometric data were also collected at pH > 2 to determine the presence of additional equilibrium. Before each titration, the electrode was calibrated by acid–base titrations with standard HCl and NaOH solutions. Electromotive force (emf) values were measured using a combined glass electrode (MetrohmUnitrode 6.0259.100) and collected by a computer-controlled device (Amel Instruments, 338 pH Meter). The temperature in the titration cell was maintained at 25.0 ± 0.1 °C by means of a circulatory bath. Carbon dioxide content in the solution was checked by Gran’s method (Gran, 1952). Complex formation constants were obtained by processing the experimental data with the Hyperquad program (Gans et al., 1996). Hydrolysis constants for Fe3+ at T = 25°C and I = 0.1 M (NaCl) were taken from the literature (Stefánsson, 2007). Speciation calculations were done by means of the software ChemEQL v3.22. The speciation model regarded the ligand protonation and metal (Fe3+, Ca2+) complex formation constant; they were determined in this work or taken from the literature (Lacoste et al., 1965; Delgado et al., 1997; Lucena, 2003). The model comprised the presence of a CaCO3 solid phase (calcite). Equilibrium constants for metal hydrolysis, hydroxide precipitation, carbonate acid–base, and complexation equilibria for the speciation model were also taken from the literature (Stefánsson, 2007) and the internal databases of ChemEQL (Smith and Martell, 1976; Morel and Hering, 1993; Stumm and Morgan, 1995).
HPLC analysis
The [(Fe(bpcd)](CH3COO) complex was analyzed with a Shimadzu HPLC, using a C18, 00G-4053-E0 Jupiter is: 5 μM 300 Å New column 250 × 4.6 mm2 671,529-9. The mobile phases were H20 + 0.1% TFA (phase A) and ACN + 0.1% TFA (phase B). Mobile phase flux constituted 1 mL min–1 with a ramping from 5% phase B to 95% phase B within 20 min, and 95% phase B was kept until 25 min. The acquired wavelengths were 300, 280, 260, 263, 265, and 250 nm. The retention time of the complex was 23 min (Supplementary Figure 1), and no extra peaks belonging to impurity have been detected.
Elemental analysis
Elemental analyses were carried out by using an EACE 1110 CHNOS analyzer.
ESI-MS
Complex purity was assessed via ESI-MS analysis of H2bpcd [(Fe(bpcd)+(CH3COO) –] performed with a Finnigan LXQ Linear Ion Trap (ThermoScientific, San Jose, CA, United States) and an electrospray ionization source (ESI) operating in positive ion mode. Data acquisition was performed with Xcalibur software (Thermo Scientific). H2bpcd and [(Fe(bpcd)+(CH3COO) –] were dissolved in methanol and injected into the ion source at a rate of 10 μL min–1, with the temperature of the transfer line being 275 °C and 4.70 kV He pressure in the ionic trap was 1 mTorr.
Soil leaching experiments
The study of the interaction between H2bpcd/Fe3+ and soil particles was carried out using a soil column. A silt-loam soil was utilized, with the following chemical characteristics: pH (in water) 7.0, organic matter 2.21%, C.E.C. 23.7 meq/100 g, total limestone 10.9%, active limestone 2.3%, organic carbon 1.28%, total nitrogen 0.15%, available phosphorous 3.9 mg/kg, soluble boron 0.09 mg/kg, and C/N ratio 8.8.
A glass column (height: 43 cm, diameter: 4 cm, and soil weight: 284.61 g) was filled with soil as follows: the dry soil was sieved at 2 mm, loaded into the column from above and pounded to obtain a homogeneous profile, and the column was then supersaturated from above with deionized water. A 1-cm layer of silica sand was put on top of the column to avoid column digging. One milliliter of 7.7 mM Fe complex (EDDHA/Fe3+ or H2bpcd/Fe3+) solution (7.7 μmol of total Fe) was applied to the column and eluted with Milli-Q water. After a dead volume leakage of 50 mL, the eluate was collected in 5-mL fractions. Elution fractions were used to quantify Fe by ICP-MS analysis. The soil leaching experiment was also conducted with a 0.5 M CaCl2 solution for the elution instead of water.
Phytotoxicity test
To evaluate the potential toxicity of H2bpcd for plants, germination inhibition and root seedling elongation were evaluated for Cucumis sativus (Viridis F1 hybrid seeds) and Zea mays (inbred line P0423, Pioneer). Forty seeds of Cucumis sativus and 45 seeds of Zea mays were germinated in boxes (23.50 × 18 cm2 for cucumber; 24.50 × 30 cm2 for maize) on filter paper moistened with a solution (20 mL for cucumber; 32 mL for maize) of H2bpcd or NaEDTA at the following concentrations: 0, 10, 50, and 100 μM. The boxes were closed with plastic film and aluminum foil and incubated at 25°C in the dark. Germination time consisted of 4 days for cucumber and 3 days for maize. The experiment was repeated four times.
Plant materials and treatments
Cucumber seeds (Cucumis sativus L. and Viridis F1 hybrid) were germinated for 6 days in a closed box with the filter paper moistened with 1 mM CaSO4 solution. The seeds were incubated in a dark chamber at 25°C. Growth experiments were carried out in hydroponics. After the germination, six seedlings were grown for 7 days in one black pot with 1.8 L of nutrient solution featuring the following composition: 0.7 mM K2SO4, 2 mM Ca(NO3)2, 0.5 mM MgSO4, 0.1 mM KH2PO4, 0.1 mM KCl, 10 μM H3BO3, 0.5 μM MnSO4, 0.5 μM ZnSO4, 0.2 μM CuSO4, and 0.01 μM (NH4)6Mo7O24 in the presence of 1 g/L CaCO3. Fe as Fe(EDDHA) with the final concentration equal to 50 μM (positive control) was added purposely to one pot. The growth parameters were as follows: temperature of 25°C, 16/8 h light/dark regime, relative humidity of 50%, and light intensity of 200–250 μmol m–2 s–1 PPFD (photosynthetic photon flux density). The nutrient solution was kept aerated by means of a pump system. After 7 days, the Fe-deficient seedings (previously grown without Fe) were supplied with the aforementioned nutrient solution at three different concentrations (0.2, 2, and 20 μM) of two Fe sources, such as Fe(bpcd)]+ and Fe(EDDHA). One pot (six seedlings) was maintained without Fe (negative control). The positive control was grown for further 7 days in similar conditions. Three growth and treatment experiments (biological replicates) with six plants each (technical replicates) were independently repeated, for a total of 18 plants.
The fresh and dry weight of shoots and roots, and the leaf count were determined for each plant. In addition, soil and plant analyzer development (SPAD) chlorophyll indices were measured by using a chlorophyll meter (Konica-Minolta). The average size of each leaf was determined based on five measurements. Throughout each experiment, shoots and roots of three plants per each condition were washed for three times with Milli-Q water. Thereafter, tissue samples were dried for 3 days at 60°C, homogeneously ground with mortar and pestle, and used for the determination of macro- and micronutrient content by ICP-MS analysis.
The root apparatus was scanned with an Epson WIA scanner. Acquired images were then analyzed with WinRHIZO™ software, 2015a Pro version (Regent Instruments Inc.), using the “root morphology” mode.
Activity of ferric-chelate reductase
Three plant roots were washed for three times with Milli-Q water to remove any residues of nutrient solution. The root apparatus was incubated in a 0.1 mM disodium EDTA (Na2EDTA) solution to remove chelate-free and apoplastic Fe. The roots were then washed three times with Milli-Q water to remove EDTA and then incubated in 20 mL of assay solution for 20 min at 25°C in the darkness. The assay solution had the following composition: 0.5 mM CaSO4, 500 μM 3-(2-pyridyl)-5,6-bis(4-phenylsulfonic acid)-1,2,4-triazine also called ferrozine (PDT), 250 μM ferric sodium EDTA (FeNaEDTA), 10 mM 2-(N-morpholino) ethanesulfonic acid sodium hydroxide (MES NaOH), and pH 6.0. The formation of the complex between chromophore PDT and its ligand Fe2+, produced by the root ferric-chelate reductase (FCR) activity, was determined spectrophotometrically by measuring the absorbance at 562 nm (Stookey, 1970). The activity of the enzyme was calculated as nmol Fe2+g–1 root FW h–1.
57Fe absorption experiments
The cucumber seeds were germinated as previously described and hydroponically grown in pots with nutrient solution for only 7 days, without the addition of any Fe source. The composition of the nutrient solution was the same as detailed in section “Plant Materials and Treatments” but without the addition of CaCO3. Two assay solutions for the 57Fe absorption experiment were prepared by using the nutrient solution with H2bpcd/57Fe3+ or EDTA/57Fe3+, with the concentration of Fe reaching 2 μM. The root apparatus of each plant was incubated in 50 mL of the assay solution. The experiment was performed at 25°C by maintaining the root apparatus in the dark. The nutrient solution was kept aerated by means of a pump system. The assay was carried out for 1 and 24 h. The assay was performed for both H2bpcd/57Fe3+ and EDTA/57Fe3+ chelates. The root apparatus of each cucumber plant was washed with Milli-Q water and incubated in the assay solution. After 1 or 24 h, the roots were sequentially washed once with a 0.1 mM Na2EDTA solution and twice with Milli-Q water. Samples were dried for 3 days at 60°C and homogeneously ground with mortar and pestle. Thereafter, they were digested and analyzed at ICP-MS.
ICP-MS analysis
The ICP-MS analysis was used to establish the Fe concentration of each fraction eluted from the columns in the soil leaching experiment and the macro- and micronutrient content of plant tissues. Moreover, the content of 57Fe in the shoots and roots was defined throughout the isotope ratio measurements carried out with the same instrument (Agilent 7500ce ICP-MS detection system, Agilent technologies). In the case of eluted fractions, samples were diluted (1:50) with 2% HNO3 (prepared using ultrapure 68% HNO3 in water and 18.2 MΩ⋅cm at 25 °C) and filtered (0.45 μm). For the analysis of the plant tissues, about 10 mg of samples was mineralized in a 3-mL TFM microsampling insert (Milestone) using 350 μL of ultrapure 68% HNO3. Three inserts were put into a 100-mL TFM vessel with 11 mL of Milli-Q water (18.2 MΩ⋅cm at 25°C) and 1 mL of ultrapure grade 30% H2O2. The digestion lasted for 20 min at 180°C in a StartD microwave digestion system (Milestone Srl). The digested samples were diluted up to a concentration of 2% HNO3 and then analyzed using an Agilent 7500ce ICP-MS detection system (Agilent technologies). Calibration curves were attained by dilution of a custom-made multielement standard (Romil LTD), with a stock solution containing K (20,000 ppm), Ca (10,000 ppm), Mg and P (2,000 ppm), Na (400 ppm), Fe (50 ppm), Mn (40 ppm), B and Zn (20 ppm), Cu (5 ppm), Co, Mo, and Se (1 ppm). Measurement accuracy and matrix effect errors were assessed using a standard reference material (NIST® SRM® 1515 Apple leaves). The measurement accuracy for the analysis of 57Fe was conducted using a certified isotopic reference material (IRMM-634).
Results
Speciation of H2bpcd/Fe3+ complex in aqueous solution
Figure 3A exhibits the spectral changes recorded during/alongside the titration of the ligand/FeCl3 solution from pH∼0 to 2. By increasing the pH, the maximum located at λmax = 259 nm (blue) shifts slightly (final λmax = 253 nm) and increases in intensity (Figure 3A). The fitting of spectrophotometric data at low pH provided a quite high formation constant for the 1:1 cationic complex with bpcd ([Fe(bpcd)]+; logβ = 20.86; Figure 3C). Protonated species [e.g., Fe(bpcd)H+] were discarded by the fitting program.
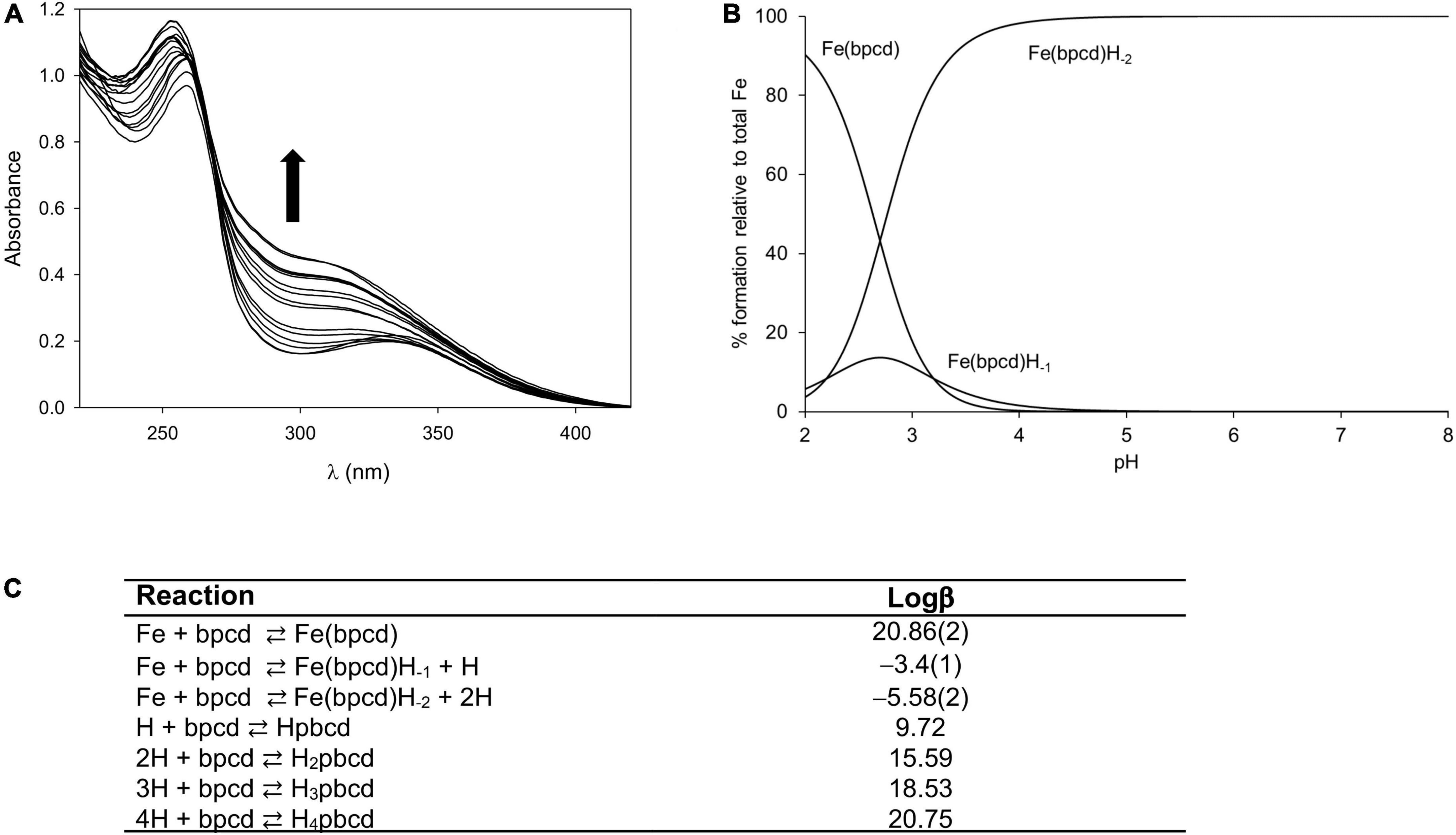
Figure 3. Spectral changes observed when increasing the pH from 0.0 to 2.0 in solutions containing equimolar amounts of the bpcd ligand (0.088 mM) and FeCl3 (A). Speciation for the Fe3+/bpcd system (CFe = 1.0 mM, 1:1 metal/ligand molar ratio) calculated on the basis of the fitted constants in panel (C) (B). Protonation (Leonzio et al., 2017) and formation constants for Fe(bpcd) species (standard deviation in parenthesis) (C). Charges are omitted for clarity.
Incidentally, according to the speciation derived from the formation constants herein obtained (Figure 3B), the dominant species above pH = 4 is a negatively charged hydroxo complex ([Fe(bpcd)H–2] –; Figure 4). The speciation of o,o-EDDHA/Fe3+ and EDTA/Fe3+ as a function of the pH are shown in Supplementary Figure 2. At a level near pH 8, the dominant species are the negatively charged [Fe(EDDHA)]- (100%) and the hydroxo complex [Fe(EDTA)H–1]2– (60%), respectively.
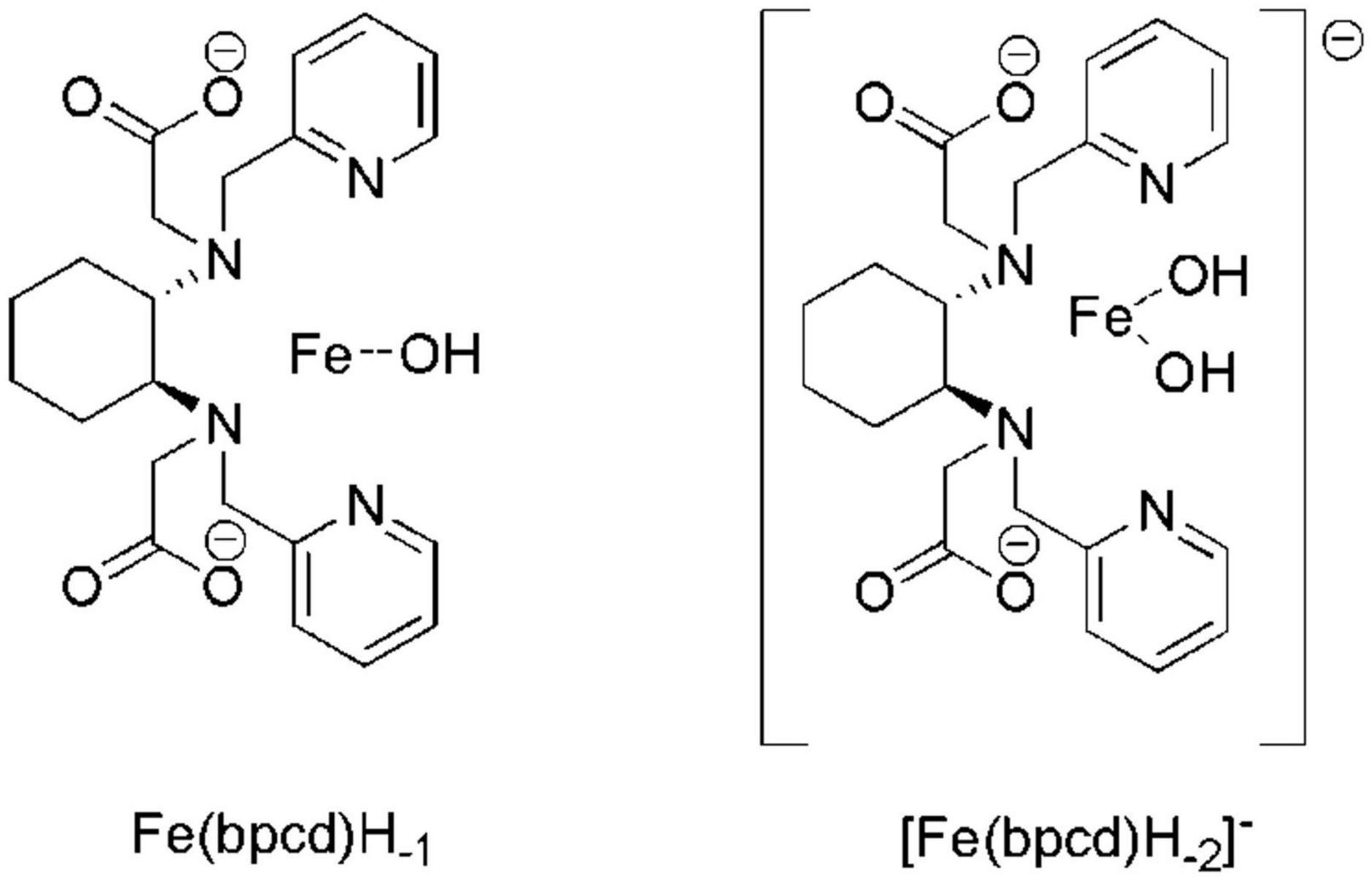
Figure 4. Molecular structure of the different Fe3+-based species observed in aqueous solution above pH 3. In Fe(bpcd)H–1 and [Fe(bpcd)H–2] – species, the ligand/Fe3+ dative bonds are not depicted.
Speciation of H2bpcd/Fe3+, o,o-EDDHA/Fe3+, and EDTA/Fe3+ complexes (2 μM) in solution, considering the presence of dissolved CO2, solid CaCO3, and Fe(OH)3
By virtue of the ChemEQL software, the speciation of the three chelates was predicted in more realistic conditions, miming those existing in calcareous soils, in which solids, such as CaCO3 and Fe(OH)3, and dissolved CO2 are present together with the species in the solution. The analysis of the data in Table 1 and the speciation plots of solutions containing a 2 μM total concentration of Fe3+ and H2bpcd, and o,o-EDDHA and EDTA (ligand/metal ratio = 1) in the 2-11 pH range (Supplementary Figure 3) lead to the following findings: (i) in the 2-11 pH range, we observed precipitation of Fe(OH)3 only in the case of EDTA ligand (above pH 5.4); (ii) at the pH recorded in our experiments in hydroponic culture (around 8), the most abundant species containing the ligand are the negatively charged bis-hydroxo complex ([Fe(bpcd)H–2]– (10–6 M) (Figure 4) in the case of H2bpcd/Fe3+, the negatively charged [Fe(EDDHA)] – (2⋅10–6 M) in the case of o,o-EDDHA/Fe3+, and the negatively charged [Ca(EDTA)]2– (8⋅10–7 M) in the case of EDTA/Fe3+; (iii) at pH around 8, EDTA is less effective in chelating Fe3+ ion, as the concentration of Fe-chelate species is by one order of magnitude lower than the other two ligands; (iv) contrary to EDTA, the less affinity for Ca2+ and the presence of soluble Fe-hydroxo complexes (Fe(bpcd)H–1 and [Fe(bpcd)H–2]–), in the case of H2bpcd ligand, prevented the precipitation of Fe(OH)3, at pH > 7.
Leaching experiment in soil column
The simulation of the leaching of chelates through the soil profile was carried out using a glass column filled with silt-loam soil. The amount of eluted Fe was determined by ICP-MS for each 5-mL collected fraction. As shown in Figure 5A, EDDHA/Fe3+ starts to exit from the column at the 65th milliliter, and at an eluted volume equal to 95 mL, Fe reaches its maximum concentration. After around 170 mL, almost all Fe loaded as EDDHA/Fe3+ flowed through the column. In the case of H2bpcd/Fe3+, Fe concentration in the eluate raised significantly slowly, reaching the maximum concentration at about 140 mL. By observing the amount of eluted Fe (Figure 5B), it can be inferred that almost 93% of applied EDDHA/Fe3+ was recovered. Conversely, the recovery of H2bpcd/Fe3+ reached only 5.4%, thereby suggesting a stronger interaction of this chelate with soil particles. H2bpcd/Fe3+ was hardly displaced from the soil even when 0.5 M CaCl2 occurred in the solution used for elution (Figure 5C). Only 9% of Fe was recovered at the end of the experiment.
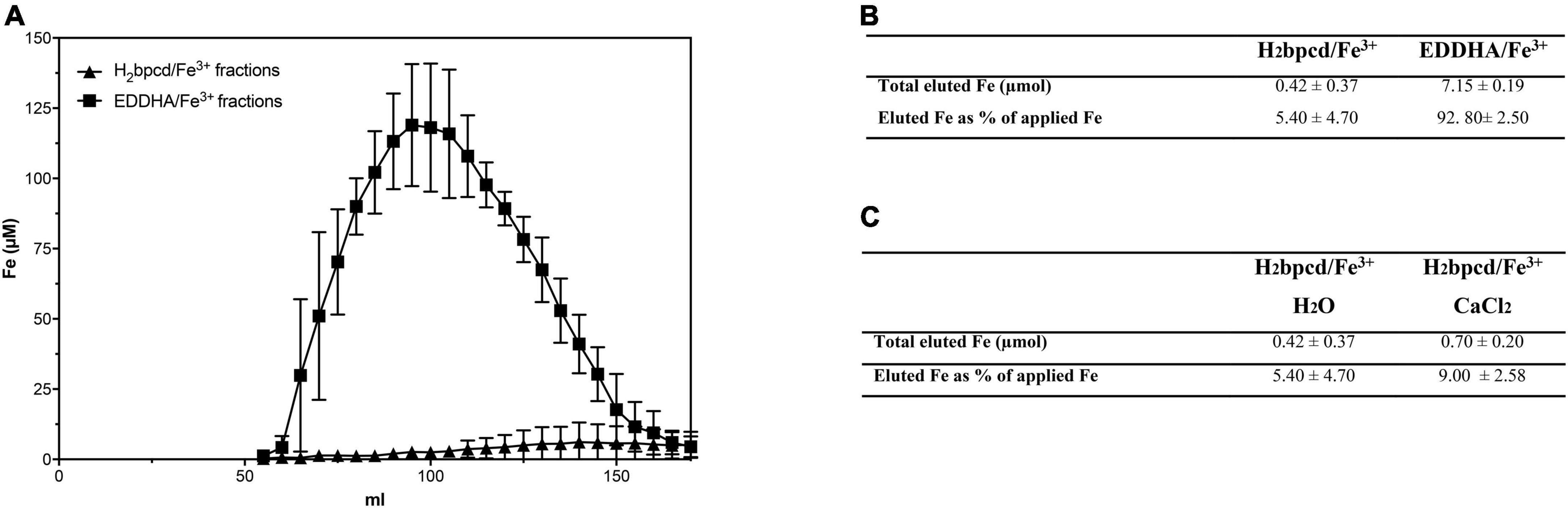
Figure 5. Leaching through the soil columns: (A) profile of eluted Fe after the application of H2bpcd/Fe3+ and EDDHA/Fe3+ and (B) comparison of the total amount of eluted Fe after the application of both Fe sources when elution was carried out with H2O, and (C) comparison of the total amount of eluted Fe after the application H2bpcd/Fe3+ between elution with H2O and 0.5 M CaCl2. Data are expressed as mean ± S.D. of three independent replicates.
Phytotoxicity assays
Germination tests were performed on cucumber and maize seedlings to assess the presence of toxicity effects of H2bpcd ligand on plants. The results (Table 2) show that the application of H2bpcd or Na2EDTA up to 100 μM did not affect maize and cucumber germination, and morphological traits. Furthermore, they did not differ from the results of the control.
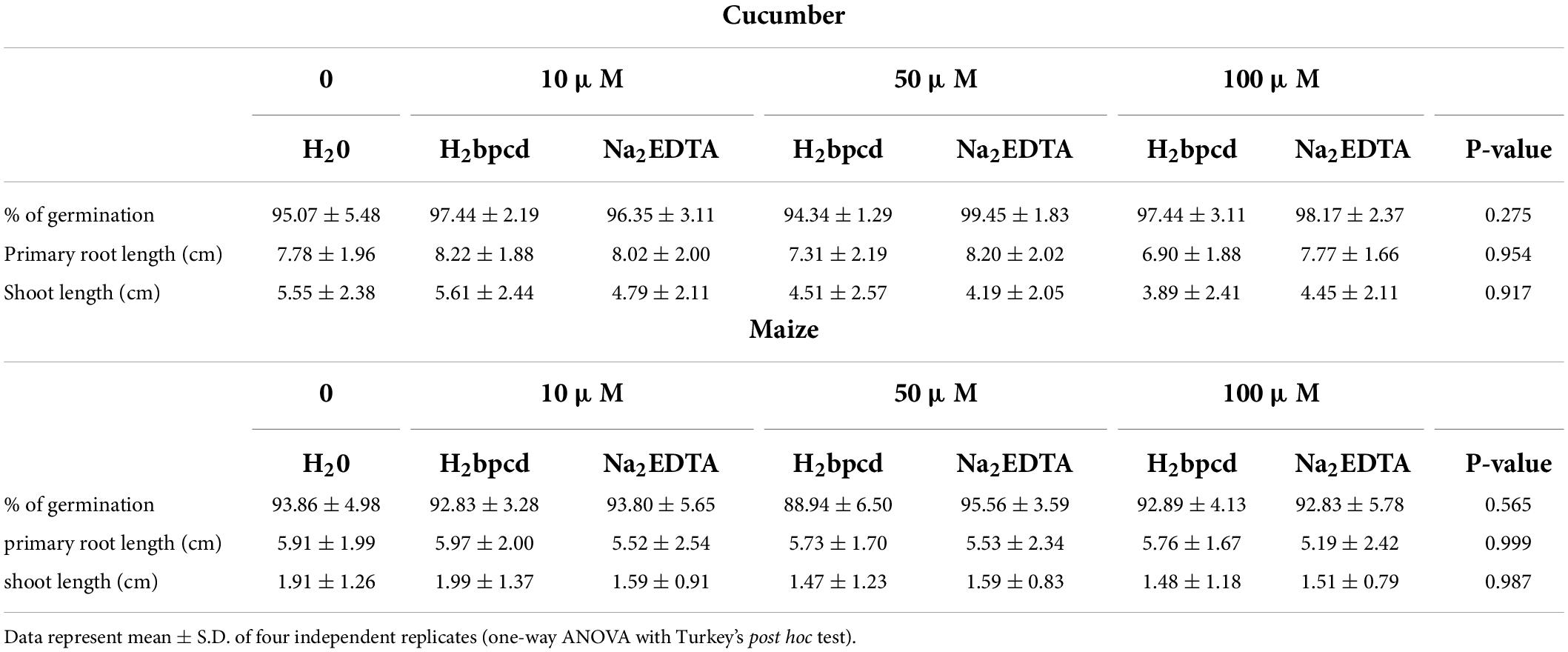
Table 2. Comparison of germination parameters for cucumber and maize seedlings when treated with different doses (10, 50, and 100 μM) of H2bpcd or Na2EDTA.
Recovery of cucumber plants from Fe deficiency
To assess the capability of H2bpcd/Fe3+ to supply Fe to Fe-deficient plants (Supplementary Table 1), we compared the recovery of hydroponically grown plants when supplied for 7 days with H2bpcd/Fe3+or EDDHA/Fe3+ at three different suboptimal concentrations (0.2 μM, 2 μM, and 20 μM) in the presence of CaCO3 at 1g/L. The capability of H2bpcd/Fe3+ to supply Fe was evaluated (Supplementary Figure 5) through the analysis of leaf SPAD index, root and shoot dry weight (DW), root development, and root ferric chelate reductase (FCR) activity (Figure 6).
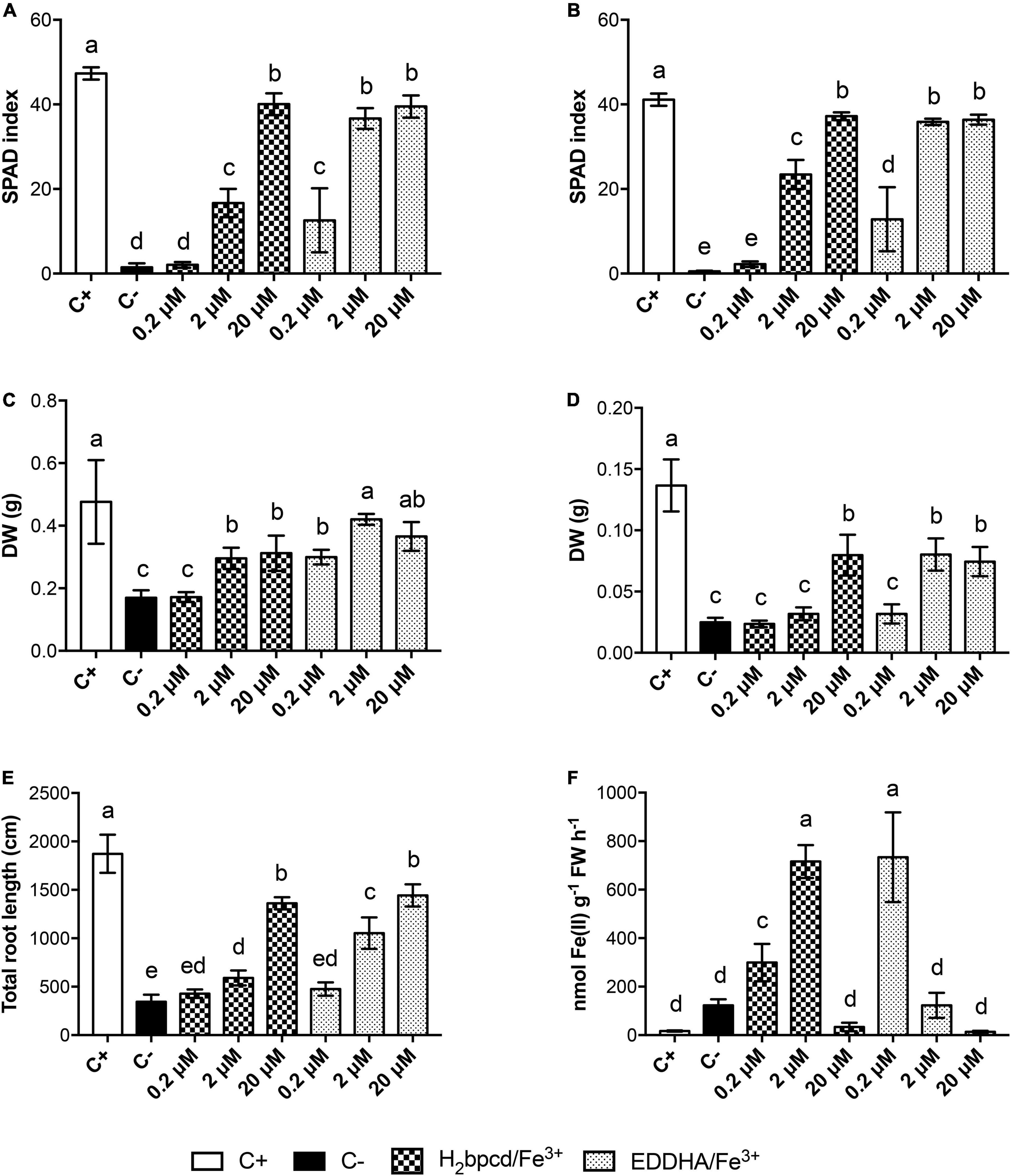
Figure 6. Morpho-physiological parameters of Fe-deficient cucumber plants supplied with H2bpcd/Fe3+ and EDDHA/Fe3+ for 7 days. (A) SPAD index of the first leaf, (B) SPAD index of the second leaf, (C) shoot DW, (D) root DW, (E) total root length (F), and FCR activity. Data represent mean ± S.D. of three independent replicates with four plants each (n = 12) for panels (A,B) and two plants each (n = 6) for panels (C–F) (one-way ANOVA with Turkey’s post hoc test, p < 0.05, significant differences are indicated by different letters).
The trend of the SPAD index was found to be quite similar in the first and the second leaves (Figures 6A,B and Supplementary Table 2). H2bpcd/Fe3+ supplied at 0.2 μM did not prompt a significant increase in the SPAD index. A partial regreening started at 2 μM of H2bpcd/Fe3+ (Figures 6A,B) and reached values similar to those of the positive control at 20 μM (40 vs. 47, Supplementary Table 2). EDDHA/Fe3+ proved to be more effective, since the regreening started even at 0.2 μM. Conversely, at the highest concentration assayed, the SPAD values did not differ significantly from those of the plants supplied with the two Fe-chelates (Supplementary Table 2). Data concerning the shoot, root biomass, and root length (Figures 6C–E) confirmed that EDDHA/Fe3+ can start the restoration of the plant nutritional status of Fe at concentrations lower than those of H2bpcd/Fe3+. However, even these parameters did not show significant differences at 20 μM (Supplementary Table 2).
We also measured the capacity of cucumber roots to reduce Fe. As expected, the lowest values of FCR were recorded for the positive controls (Figure 6F). The highest activity for H2bpcd/Fe3+-treated plants was recorded at 2 μM, while that of EDDHA/Fe3+ was found at 0.2 μM.
Ionomic analysis
The content of Fe and other macro- and micronutrients in shoots (Figure 7 and Supplementary Table 4) and root tissues (Supplementary Tables 3, 5) was investigated by ICP-MS analysis. As foreseen, Fe-deficient plants had significantly lower Fe concentration in shoots with respect to the positive control (Figure 7F). The treatments with the two chelates exhibited trends similar to those described for the growth parameters (Figure 6). It is remarkable that the concentration of 20 μM H2bpcd/Fe3+ could restore Fe shoot content similar to the positive control, but when EDDHA/Fe3+ was resupplied at the same rate, the plants accumulated Fe two times as much as the positive control (Supplementary Table 4).
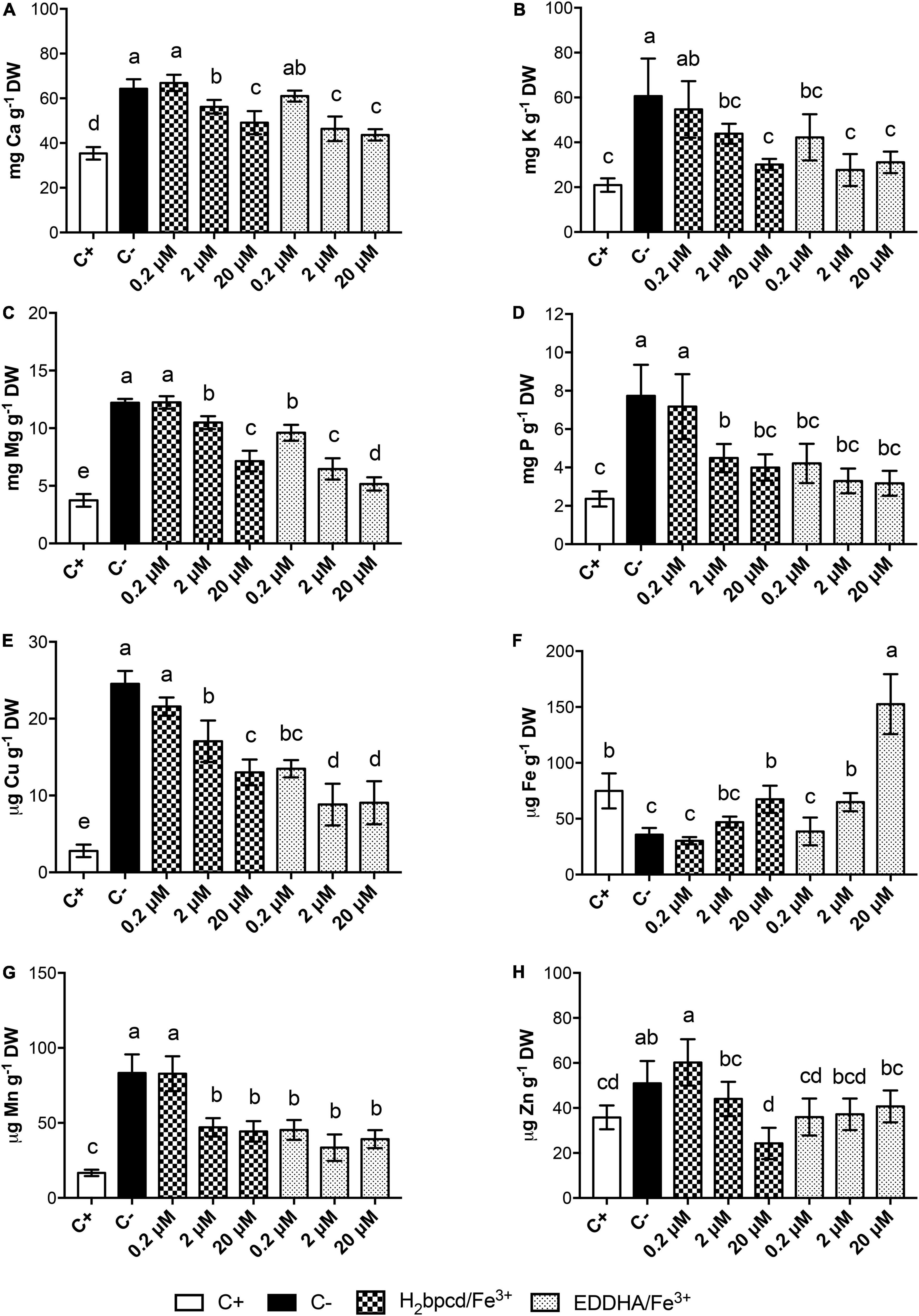
Figure 7. Macro- and micronutrient content in shoot tissues of Fe-deficient cucumber plants supplied with H2bpcd/Fe3+ and EDDHA/Fe3+ for 7 days. Shoot content of (A) Ca, (B) K, (C) Mg, (D) P, (E) Cu, (F) Fe, (G) Mn, and (H) Zn. Data represent mean ± S.D. of three independent replicates with two plants each (n = 6) (one-way ANOVA with Turkey’s post hoc test, p < 0.05, significant differences are indicated by different letters).
Data concerning other nutrients underlined that Fe deficiency determined higher content of divalent cations in shoot tissues when compared to the positive control (Figure 7).
Short-term 57Fe accumulation
The capability of Fe-deficient cucumber plants to utilize the complex of H2bpcd with Fe was evaluated after 1 and 24 h of treatment with 2 μM H2bpcd/57Fe3+. The EDTA/57Fe3+ at the same concentration was used as a benchmark. The experiment was run at pH 6.0 (without CaCO3).
H2bpcd/57Fe3+-treated roots showed an increase in 57Fe accumulation from 1 h to 24 h, whereas no differences were observed between 1 and 24 h in the case of EDTA/57Fe3+-treated roots (Figure 8A). In the case of shoot, 57Fe was detectable only after 24 h with higher significant values of accumulation for the plants treated with EDTA/57Fe3+ (Figure 8B). In contrast, the shoot/root Fe content ratio at 24 h exhibited similar values (0.47 for H2bpcd/57Fe3 vs. 0.53 for EDTA/57Fe3+) between the plants supplied with the two Fe-chelates.
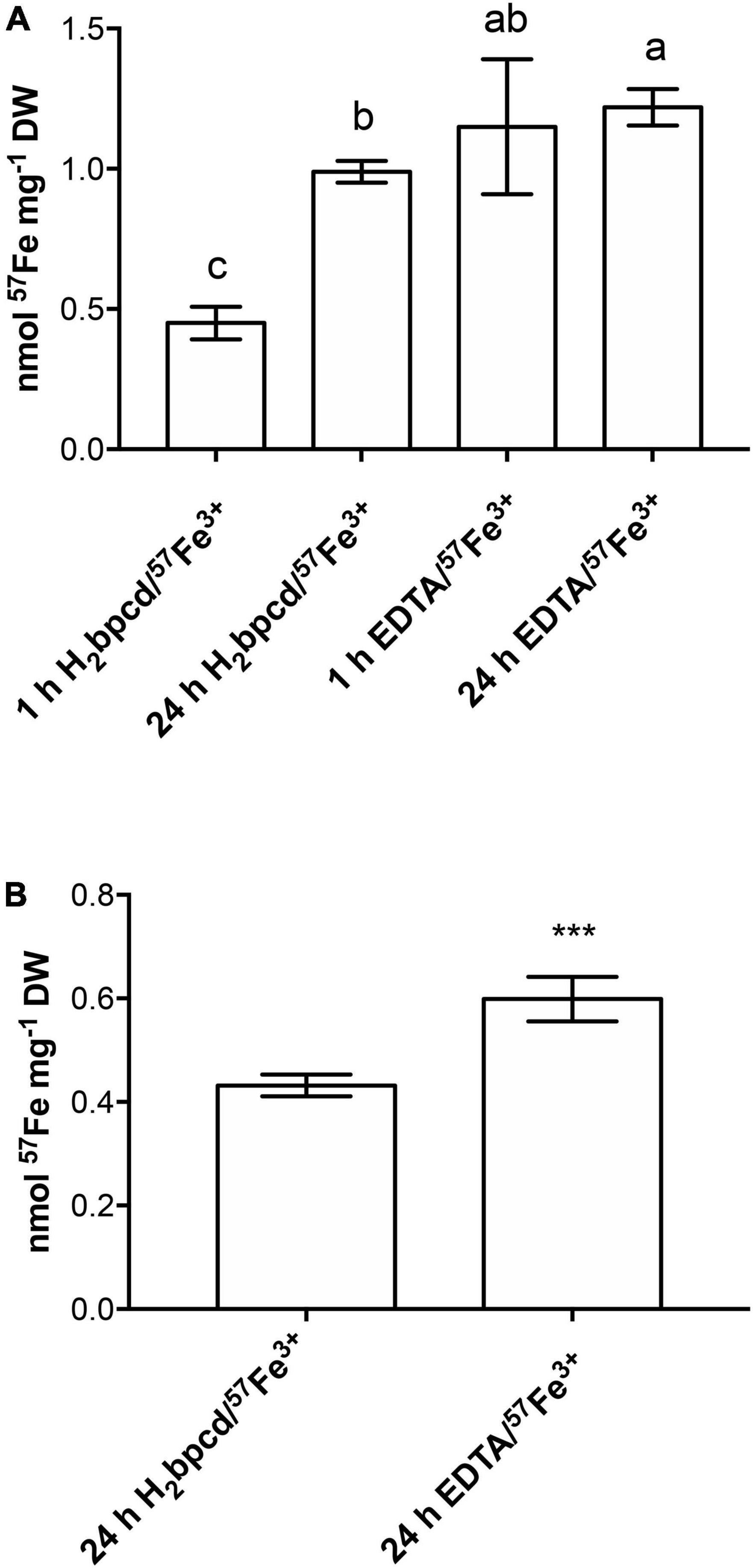
Figure 8. 57Fe uptake in (A) root of cucumber plants after 1 and 24 h of treatment with H2bpcd/57Fe3+ or EDTA/57Fe3+ and (B) in shoot after 24 h of the same treatments. Data represent mean ± S.D. of three independent replicates with two plants each (n = 6) (one-way ANOVA with Turkey’s post hoc test, p < 0.05, significant differences are indicated by different letters and Student’s t-test: ***p < 0.001).
Discussion
The high mobility in the soil of Fe-chelates hinders their use in agriculture, as they lead to a low persistence for the subsequent plant uptake and environmental risk. In the work detailed herein, we studied the behavior of a new Fe-chelate, H2bpcd/Fe3+, with respect to soil. Furthermore, we investigated how H2bpcd/Fe3+ can support the growth of Fe-deficient plants of cucumber and supply Fe in hydroponics in the presence of CaCO3.
We found that the complex of Fe with bpcd ([Fe(bpcd)]+; logβ = 20.86) features a lower stability than that with EDTA (logβ = 25.10) (Delgado et al., 1997), o,o-EDDHA(logβ = 35.09) (Yunta et al., 2003), and CDTA (logβ = 29.05, T = 25°C) (Brausam et al., 2009), and a higher one than that with dicarboxylic analog (ethylenediamine-N,N′-diacetic acid, EDDA logβ = 15.5) (Serratrice et al., 2001). This behavior indicates that all donor groups are involved in the binding of Fe3+ ion, yet with lower stability than that estimated by Lacoste et al. (1965) with the similar ligand ethylenebis- N,N′-(2-aminomethyl)- pyridine-N,N′-diacetic acid (EDAMPDA, logβ > 21.5).
The two H2bpcd/Fe3+ and o,o-EDDHA/Fe3+ systems, which were obtained in the presence of solid CaCO3, Fe(OH)3, and dissolved CO2, exhibited similar behavior, as per the speciation data (Supplementary Figure 3 and Table 1). Unlike EDTA, whose higher affinity for Ca2+ causes the precipitation of Fe hydroxide, both chelates can specifically maintain Fe3+ ion in solution in basic conditions.
The experiments conducted in columns containing soil with a total limestone level of 10.9% confirmed the high mobility of o,o-EDDHA/Fe3+ along the soil profile (Figure 5). In fact, the highly stable o,o-EDDHA/Fe3+ chelate, which should weakly interact with the soil, is highly concentrated in the soil solution and almost fully eluted (about 93%). Similar percentages for the elution of [59Fe(EDDHA)]– from the soil columns were reported by Cesco et al. (2000). Conversely, the less stable H2bpcd/Fe3+ chelate is retained by the soil and provides only a low quantity of Fe3+ (no more than 10% of the total applied Fe) after the elution of the same solution volume (Figure 5 and Table 1).
We tentatively rule out the involvement of the attractive coulombic interaction by the negatively charged clay surface, despite the complexity of the mechanisms at the basis of soil/chelate interaction. The analysis and review of these mechanisms exceed the scope of the present contribution, as the dominant species at pH ≥ 7 is bis-hydroxo complex ([Fe(bpcd) (OH)2] –; Figure 4), which is not cationic, yet anionic. Furthermore, no significant change in the eluted quantity of H2bpcd/Fe3+ was observed during the leaching experiment with added Ca2+ to the simulated elution solution (Figure 5C). Nevertheless, we may infer that the donating atoms of H2bpcd hold responsibility for the interaction with the metal cations present in the soil. Likewise, Hernández-Apaolaza and Lucena (2001) reported similar results for the complex montmorillonite-Ca-Fe-chelate. In addition, given the presence of two OH groups in the [Fe(bpcd)H–2] – complex, the participation of hydrogen bonds in the chelate/soil interaction cannot be indeed neglected.
The results detailed herein suggest that the H2bpcd/Fe3+ complex can be less leached out of the root zone and that Fe bioavailability might last longer. Such an attitude can avoid frequent Fe replenishment of the rhizosphere to correct the plant deficiency is less frequently required, thereby preventing extensive groundwater pollution and diminishing the concern associated with the use of Fe-chelates (Abadía et al., 2011).
The feasibility of H2bpcd/Fe3+ as an environmentally friendly alternative to alternative Fe chelator requires the evaluation of its effects on plants. Germination tests underlined that the H2bpcd ligand did not influence the seed germination more negatively than EDTA. Given that, we decided to evaluate the ability of H2bpcd/Fe3+ to supply Fe to Fe-deficient cumber plants in hydroponics. We select the harshest condition for the Strategy I plant, namely, high pH due to the CaCO3 presence. The effect of H2bpcd/Fe3+ treatment was compared to that of EDDHA/Fe3+ supplied at three different suboptimal Fe concentrations (0.2, 2, and 20 μM). As expected, the recovery from Fe deficiency was found to be concentration-dependent and faster at 20 μM Fe concentration (Figure 6). At a consistently lower concentration than that is optimal for Fe concentration in hydroponics solution (Agnolon et al., 2001), H2bpcd/Fe3+- and EDDHA/Fe3+-treated plants did not display statistically significant differences in leaf SPAD index, shoot and root dry biomass, and total root length (Supplementary Table 2). Santos et al. (2016) found that the complex between the ligand tris(3-hydroxy-4-pyridinonate) and Fe3+ improves the plant growth if compared to 20 μM commercial EDDHA/Fe3+ supplied to Fe-deficient Glycine max plants grown in hydroponics. However, these experiments were carried out at pH 5.5, a value lower than that of CaCO3-containing soils where lime-induced chlorosis frequently occurs. Differences between the two Fe sources were observed only at the lower concentrations (0.2 and 2 μM, Supplementary Table 2). In these conditions, EDDHA/Fe3+ exhibited an enhanced capacity in supplying the micronutrient, as demonstrated by all the parameters herein evaluated (Supplementary Tables 2, 4).
Comparable values of root FCR activity to those measured in the positive control (Fe-sufficient plants grown at 50 μM) (Figure 6F) confirm effective recovery from the deficiency at 20 μM of both Fe sources. The highest FCR activity was recorded at 0.2 and 2 μM for plants treated with H2bpcd/Fe3+ and 0.2 μM for EDDHA/Fe3+, respectively. Therefore, it can be inferred that the supply of these quantities of Fe was not sufficient for the plants, thereby maintaining an active FCR (Agnolon et al., 2001). Such values were even higher than those measured in the negative controls, which comprised plants grown for 14 days without Fe. The lower levels of FCR activity in the negative control could be linked to the complete absence of the micronutrient. It was reported that the presence of at least some amount of Fe is of the utmost importance to the functioning of FCR (Robinson et al., 1999). Furthermore, the evidence of higher FCR activity in the H2bpcd/Fe3+-treated plants than those supplied with EDDHA/Fe3+ at 2 μM of Fe (Figure 6F) reinforces the hypothesis that the regreening capacity of this new chelator occurs at a concentration as high as 2 μM. Conversely, the shoot Fe content of the plants supplied with 20 μM EDDHA/Fe3+ was found to be higher than that measured for the H2bpcd/Fe3+-treated plants, yet without significant differences in SPAD index (Figures 6A,B, 7F). In addition, we observed a general decrease in the concentration of Ca, K, Mg, Cu, Mn, and Zn after 7 days of supply with both Fe-chelates (Figure 7). A decrease in the leaf content of K, Ca, Cu, Mn, and Zn in cucumber plants was observed during the recovery from Fe deficiency when supplied with different natural Fe complexes (Tomasi et al., 2014). The explanation for the highest content of these nutrients in tissues may be twofold. First, an alteration in cation/anion uptake due to enhanced activity of FCR and Fe transporter which can also uptake other metals. Second, a concentration due to a reduction of shoot dry biomass under deficiency (Tomasi et al., 2014). The pattern of the concentrations of these cationic elements in H2bpcd/Fe3+- and EDDHA/Fe3+-treated plants further confirms that the recovery from Fe deficiency started earlier for the second chelate.
In the end, we discussed the possible differences occurring in the uptake of Fe by roots during the recovery in the suboptimal range of concentration (2 μM) using the 57Fe as a tracer chelated to H2bpcd and EDTA. When treated with EDTA/57Fe3+ rather than with H2bpcd/Fe3+ (Figure 8), roots exhibited a significantly higher content of 57Fe after 1 h and 24 h, and shoots after 24 h. The correlation between chelate characteristics (charge and logβ), FCR activity, and the subsequent Fe uptake has not been fully explained yet (Chaney, 1989; Lucena and Chaney, 2006). Lucena (2006) asserted that the weakest Fe3+-chelates consist in the best substrates for the FCR in Fe-deficient cucumber roots. H2bpcd/Fe3+ should, indeed, represent a better substrate than both EDTA/Fe3+ and EDDHA/Fe3+. The following points may support the rationale for such a seeming contradiction: (i) the lower availability for the substrate of the enzyme caused by a potential stronger interaction of the H2bpcd/Fe3+ molecule with the cell wall; (ii) the stability of the H2bpcd/Fe2+ counterparts, as the Fe uptake involves Fe2+, and (iii) the strong and likely crucial impact on the release of the metal ion in solution produced by the kinetic inertness of the metal complexes (quite often neglected). It is widely recognized that the presence of the trans-1,2-diaminocyclohexane ring in the ligand backbone can originate a rigidifying effect, thereby increasing the kinetic stability of the related metal complex. This holds true for lanthanide(III)-based complexes (Tircsó et al., 2016), which is also observed for Fe-chelates. Therefore, it can be speculated that the H2bpcd/Fe3+ kinetic stability is as high as slow as the Fe supply to plant is. Since the deepening of the biochemical reasons behind the differences in the rate of Fe uptake stretches beyond the scope of this work, we will discuss this aspect in a further paper.
Conclusion
The obtained data demonstrate that, as hypothesized, the H2bpcd/Fe3+ complex interacts with soil particles and is much less mobile than EDDHA/Fe3+. In hydroponics, the complex, which is even less effective in the regreening than EDDHA/Fe3+ at very low Fe concentrations, proved to be capable of restoring the physiological Fe concentration at 20 μM. These two characteristics suggest that the H2bpcd/Fe3+ complex is a good candidate as new environmentally friendly Fe fertilizer. The validation of the information obtained in simplified laboratory scale experiments demands further studies to be carried out in plant-soil microcosms or in fields.
Data availability statement
The original contributions presented in this study are included in the article/Supplementary material, further inquiries can be directed to the corresponding author.
Author contributions
ZV and FP conceived the research. AZ directed the experiments. DS, AM, SR, and MS performed the experiments. FP, DS, AZ, AM, and ZV analyzed and interpreted the data. FP, ZV, and AZ wrote the manuscript. FP, AM, ZV, and AZ revised the manuscript. ZV obtained the funding to carry out the investigation. All authors contributed to the article and approved the submitted version.
Funding
The work was funded by Joint Project 2019 developed by the University of Verona and Fabbrica Cooperativa Perfosfati Cerea.
Acknowledgments
We thank the Facility “Centro Piattaforme Tecnologiche” of the University of Verona for access to the Agilent 7500ce ICP-MS.
Conflict of interest
The authors declare that the research was conducted in the absence of any commercial or financial relationships that could be construed as a potential conflict of interest.
Publisher’s note
All claims expressed in this article are solely those of the authors and do not necessarily represent those of their affiliated organizations, or those of the publisher, the editors and the reviewers. Any product that may be evaluated in this article, or claim that may be made by its manufacturer, is not guaranteed or endorsed by the publisher.
Supplementary material
The Supplementary Material for this article can be found online at: https://www.frontiersin.org/articles/10.3389/fpls.2022.964088/full#supplementary-material
Footnotes
- ^ For the metal (M) – ligand (L) overall complex formation reaction, considering also protonation equilibria: iM + jL + kH ⇋ MiLjHk
- ^ https://www.eawag.ch/en/department/surf/projects/chemeql/
References
Abadía, J., Vázquez, S., Rellán-Álvarez, R., El-Jendoubi, H., Abadía, A., Álvarez-Fernández, A., et al. (2011). Towards a knowledge-based correction of iron chlorosis. Plant Physiol. Biochem. 49, 471–482. doi: 10.1016/j.plaphy.2011.01.026
Agnolon, F., Santi, S., Varanini, Z., and Pinton, R. (2001). Enzymatic responses of cucumber roots to different levels of Fe supply. Plant Soil 241, 35–41. doi: 10.1023/A:1016034631038
Brausam, A., Maigut, J., Meier, R., Szilágyi, P. Á, Buschmann, H.-J., Massa, W., et al. (2009). Detailed spectroscopic, thermodynamic, and kinetic studies on the protolytic equilibria of feiiicydta and the activation of hydrogen peroxide. Inorg. Chem. 48, 7864–7884. doi: 10.1021/ic900834z
Broadley, M., Brown, P., Cakmak, I., Rengel, Z., and Zhao, F. (2012). “Function of nutrients: micronutrients,” in Marschner’s Mineral Nutrition of Higher Plants, ed. P. Marschner (San Diego, CA: Academic Press), 191–248.
Cappai, R., Chand, K., Lachowicz, J. I., Chaves, S., Gano, L., Crisponi, G., et al. (2018). A new tripodal-3-hydroxy-4-pyridinone for iron and aluminium sequestration: synthesis, complexation and in vivo studies. New J. Chem. 42, 8050–8061. doi: 10.1039/C8NJ00116B
Cesco, S., Römheld, V., Varanini, Z., and Pinton, R. (2000). Solubilization of iron by water-extractable humic substances. J. Plant Nutr. Soil Sci. 163, 285–290.
Chaney, R. (1989). of ferric chelate reduction by deficient peanut (Arachis species. Acta Bot. Neerl. 38, 155–163.
Chen, Y., and Barak, P. (1982). Iron nutrition of plants in calcareous soils. Adv. Agron. 35, 217–240. doi: 10.1016/S0065-2113(08)60326-0
Delgado, R., do Carmo Figueira, M., and Quintino, S. (1997). Redox method for the determination of stability constants of some trivalent metal complexes. Talanta 45, 451–462. doi: 10.1016/S0039-9140(97)00157-4
Gans, P., Sabatini, A., and Vacca, A. (1996). Investigation of equilibria in solution. Determination of equilibrium constants with the HYPERQUAD suite of programs. Talanta 43, 1739–1753. doi: 10.1016/0039-9140(96)01958-3
Gran, G. (1952). Determination of the equivalence point in potentiometric titrations. Analyst 77, 661–671.
Hernández-Apaolaza, L., and Lucena, J. J. (2001). Fe(III)-EDDHA and -EDDHMA Sorption on Ca-Montmorillonite, Ferrihydrite, and Peat. J. Agric. Food Chem. 49, 5258–5264. doi: 10.1021/jf010313t
Hill-Cottingham, D., and Lloyd-Jones, C. (1957). Behaviour of iron chelates in calcareous soils. Plant Soil 8, 263–274. doi: 10.1007/BF01666161
Lacoste, G., Christoffers, G. V., and Martell, A. E. (1965). New multidentate ligands. II. Amino acids containing α-pyridyl groups. J. Am. Chem. Soc. 87, 2385–2388. doi: 10.1021/ja01089a015
Leonzio, M., Melchior, A., Faura, G., Tolazzi, M., Zinna, F., Di Bari, L., et al. (2017). Strongly circularly polarized emission from water-soluble Eu(III)- and Tb(III)-based complexes: a structural and spectroscopic study. Inorg. Chem. 56, 4413–4422. doi: 10.1021/acs.inorgchem.7b00430
Loots, D. T., Lieshout, M. V., and Lachmann, G. (2007). Sodium iron (III) ethylenediaminetetraacetic acid synthesis to reduce iron deficiency globally. Eur. J. Clin. Nutr. 61, 287–289. doi: 10.1038/sj.ejcn.1602499
López-Rayo, S., Sanchis-Pérez, I., Ferreira, C. M. H., and Lucena, J. J. (2019). [S,S]-EDDS/Fe: a new chelate for the environmentally sustainable correction of iron chlorosis in calcareous soil. Sci. Total Environ. 647, 1508–1517. doi: 10.1016/j.scitotenv.2018.08.021
Lucena, J. J. (2003). Fe chelates for remediation of Fe chlorosis in strategy I plants. J. Plant Nutr. 26, 1969–1984. doi: 10.1081/PLN-120024257
Lucena, J. J. (2006). “Synthetic iron chelates to correct iron deficiency in plants,” in Iron Nutrition in Plants and Rhizospheric Microorganisms, eds J. Barton and J. Abadia (Dordrecht: Springer), 103–120.
Lucena, J. J., and Chaney, R. L. (2006). Synthetic iron chelates as substrates of root ferric chelate reductase in green stressed cucumber plants. J. Plant Nutr. 29, 423–439. doi: 10.1080/01904160500524886
Martín-Fernandez, C., Solti, A., Czech, V., Kovacs, K., Fodor, F., Garate, A., et al. (2017). Response of soybean plants to the application of synthetic and biodegradable Fe chelates and Fe complexes. Plant Physiol. Biochem. 118, 579–588. doi: 10.1016/j.plaphy.2017.07.028
Morel, F., and Hering, J. (1993). Principles and Applications of Aquatic Chemistry. Hoboken, NJ: Wiley & Sons.
Oddon, F., Girgenti, E., Lebrun, C., Marchi-Delapierre, C., Pécaut, J., and Ménage, S. (2012). Iron coordination chemistry of N2Py2 ligands substituted by carboxylic moieties and their impact on alkene oxidation catalysis. Eur. J. Inorg. Chem. 2012, 85–96. doi: 10.1002/ejic.201100785
Robinson, N. J., Procter, C. M., Connolly, E. L., and Guerinot, M. L. (1999). A ferric-chelate reductase for iron uptake from soils. Nature 397, 694–697. doi: 10.1038/17800
Rombolà, A. D., and Tagliavini, M. (2006). “Iron nutrition of fruit tree crops,” in Iron Nutrition in Plants and Rhizospheric Microorganisms, eds L. Barton and J. Abadia (Dordrecht: Springer), 61–83.
Sánchez-Alcalá, I., del Campillo, M. C., Barrón, V., and Torrent, J. (2012). Pot evaluation of synthetic nanosiderite for the prevention of iron chlorosis. J. Sci. Food Agric. 92, 1964–1973. doi: 10.1002/jsfa.5569
Santos, C., Carvalho, S., Leite, A., Moniz, T., Roriz, M., Rangel, A., et al. (2016). Effect of tris(3-hydroxy-4-pyridinonate) iron(III) complexes on iron uptake and storage in soybean (Glycine max L.). Plant Physiol. Biochem. 106, 91–100. doi: 10.1016/j.plaphy.2016.04.050
Sega, D., Ciuffreda, G., Mariotto, G., Baldan, B., Zamboni, A., and Varanini, Z. (2019). FePO4 nanoparticles produced by an industrially scalable continuous-flow method are an available form of P and Fe for cucumber and maize plants. Sci. Rep. 9:11252. doi: 10.1038/s41598-019-47492-y
Serratrice, G., Galey, J. B., Aman, E. S., and Dumats, J. (2001). Iron(III) complexation by new aminocarboxylate chelators - Thermodynamic and kinetic studies. Eur. J. Inorg. Chem. 2001, 471–479.
Shenker, M., and Chen, Y. (2005). Increasing iron availability to crops: fertilizers, organo-fertilizers, and biological approaches. Soil Sci. Plant Nutr. 51, 1–17. doi: 10.1111/j.1747-0765.2005.tb00001.x
Smith, R. M., and Martell, A. E. (1976). Inorganic Ligands BT - Critical Stability Constants: Inorganic Complexes. Boston, MA: Springer US, 1–129.
Stefánsson, A. (2007). Iron(III) hydrolysis and solubility at 25°C. Environ. Sci. Technol. 41, 6117–6123. doi: 10.1021/es070174h
Stookey, L. L. (1970). Ferrozine - a new spectrophotometric reagent for iron. Anal. Chem. 42, 779–781. doi: 10.1021/ac60289a016
Stumm, W. J., and Morgan, J. (1995). Aquatic Chemistry: Chemical Equilibria and Rates in Natural Waters, 3rd Edn. Hoboken, NJ: Wiley.
Tircsó, G., Regueiro-Figueroa, M., Nagy, V., Garda, Z., Garai, T., Kálmán, F. K., et al. (2016). Approaching the kinetic inertness of macrocyclic gadolinium(III)-based MRI contrast agents with highly rigid open-chain derivatives. Chem. A Eur. J. 22, 896–901. doi: 10.1002/chem.201503836
Tomasi, N., Mimmo, T., Terzano, R., Alfeld, M., Janssens, K., Zanin, L., et al. (2014). Nutrient accumulation in leaves of Fe-deficient cucumber plants treated with natural Fe complexes. Biol. Fertil. Soils 50, 973–982. doi: 10.1007/s00374-014-0919-6
Valverde, S., Arcas, A., López-Rayo, S., and Lucena, J. J. (2021). Fast determination of a novel iron chelate prototype used as a fertilizer by liquid chromatography coupled to a diode array detector. J. Agric. Food Chem. 69, 15746–15754. doi: 10.1021/acs.jafc.1c05943
Weinstein, L. H., Robbins, W. R., and Perkins, H. F. (1954). Chelating agents and plant nutrition. Science 120, 41–43. doi: 10.1126/science.120.3106.41
Yunta, F., García-Marco, S., Lucena, J. J., Gómez-Gallego, M., Alcázar, R., and Sierra, M. A. (2003). Chelating agents related to ethylenediamine bis(2-hydroxyphenyl)acetic acid (EDDHA): synthesis, characterization, and equilibrium studies of the free ligands and their Mg2+, Ca2+, Cu2+, Fe 3+ chelates. Inorg. Chem. 42, 5412–5421. doi: 10.1021/ic034333j
Keywords: cucumber, Fe-chelate, Fe leaching, N,N′-bis(2-pyridylmethyl)-trans-1,2-diaminocyclohexane N,N′-diacetate, plant regreening
Citation: Piccinelli F, Sega D, Melchior A, Ruggieri S, Sanadar M, Varanini Z and Zamboni A (2022) Regreening properties of the soil slow-mobile H2bpcd/Fe3+ complex: Steps forward to the development of a new environmentally friendly Fe fertilizer. Front. Plant Sci. 13:964088. doi: 10.3389/fpls.2022.964088
Received: 08 June 2022; Accepted: 14 July 2022;
Published: 04 August 2022.
Edited by:
Rongli Shi, Leibniz Institute of Plant Genetics and Crop Plant Research (IPK), GermanyReviewed by:
Sayed Mohammad Mohsin, Sher-e-Bangla Agricultural University, BangladeshBrigitta Lantos, Eötvös Loránd University, Hungary
Copyright © 2022 Piccinelli, Sega, Melchior, Ruggieri, Sanadar, Varanini and Zamboni. This is an open-access article distributed under the terms of the Creative Commons Attribution License (CC BY). The use, distribution or reproduction in other forums is permitted, provided the original author(s) and the copyright owner(s) are credited and that the original publication in this journal is cited, in accordance with accepted academic practice. No use, distribution or reproduction is permitted which does not comply with these terms.
*Correspondence: Zeno Varanini, emVuby52YXJhbmluaUB1bml2ci5pdA==