- 1Institute of Geographic Sciences and Natural Resources Research, Chinese Academy of Sciences (CAS), Beijing, China
- 2Department of Botany, Aligarh Muslim University, Aligarh, Uttar Pradesh, India
- 3Incharge Medical Officer, Basic Health Unit Munday Key District Kasur, Kasur, Pakistan
- 4Department of Forestry, College of Agriculture, University of Sargodha, Sargodha, Pakistan
- 5School of Resource and Environmental Science, Wuhan University, Wuhan, China
- 6Department of Botany, University of Sargodha, Sargodha, Pakistan
- 7Department of Plant Pathology, Institute of Agricultural Sciences, University of the Punjab, Lahore, Punjab, Pakistan
- 8Senior Superintendent Gardens, RO-II Wing, University of the Punjab, Lahore, Punjab, Pakistan
- 9Department of Biology, College of Science, United Arab Emirates University, Al Ain, United Arab Emirates
Environmental pollutants and climate change are the major cause of abiotic stresses. Hexachlorobenzene (HCB) is an airborne and aero-disseminated persistent organic pollutants (POP) molecule causing severe health issues in humans, and temperature extremes and HCB in combination severely affect the growth and yield of crop plants around the globe. The higher HCB uptake and accumulation by edible plants ultimately damage human health through the contaminated food chain. Hence, confining the passive absorbance of POPs is a big challenge for researchers to keep the plant products safer for human consumption. BioClay functional layered double hydroxide is an effective tool for the stable delivery of acidic molecules on plant surfaces. The current study utilized gibberellic acid (GA3) impregnated BioClay (BioClayGA) to alleviate abiotic stress in Brassica alboglabra plants. Application of BioClayGA mitigated the deleterious effects of HCB besides extreme temperature stress in B. alboglabra plants. BioClayGA significantly restricted HCB uptake and accumulation in applied plants through increasing the avoidance efficacy (AE) up to 377.61%. Moreover, the exogenously applied GA3 and BioClayGA successfully improved the antioxidative system, physiochemical parameters and growth of stressed B. alboglabra plants. Consequently, the combined application of BioClay and GA3 can efficiently alleviate low-temperature stress, heat stress, and HCB toxicity.
Introduction
Persistent organic pollutants (POPs) are one of the major environmental obstacles, which have been gradually intensified during the period of massive industrialization (Ahmad et al., 2021). Now, they are not only harming the environment, but their health hazards have crossed alarming levels. Besides the contaminating nature they are also characterized as quick and passively transmitting pollutants in the environment even in the absence of any anthropogenic activity. POPs possess the tendency to halt in the air, resist environmental degradation, and accumulate in the fat-rich tissues (of plants and/or humans) by passing through the phospholipid membranes (Ibrahim et al., 2016; Berntssen et al., 2017). Considering the health hazards of POPs highlights the disturbed reproductive system, disordered metabolism, and carcinogenicity (Wang et al., 2008). Among POPs, hexachlorobenzene (HCB) is highlighted as a fairly volatile compound with long-range air transportation and lipophilic properties. Although, measures have been taken to control the emission of HCB (e.g., banned in the agriculture sector), some industrial and environmental factors are continuously contributing tonnes of HCB into the air annually. Enlisting the key sources of HCB emission ranks smelting of metals, bleaching of paper pulp, production of cement, impregnation of wood, incineration of solid waste and sewage sludge, etc. Besides, HCB is released as a byproduct of all the chemical industries dealing with or producing chlorinated chemicals/liquids. Vinyl chloride, pentachloronitrobenzene, trichloroethylene, pentachlorophenol, tetrachloroisophthalonitrile, picloram, propazine, mirex, and hundreds of other chemicals add significant HCB quantities into the environment during their synthesis or incineration. HCB is readily absorbed in aerial plant parts, translocated between different tissues, and finally preserved in the lipid-rich areas. High HCB contents have been recorded in plants (e.g., pumpkins) from where they entered into the food chain and traveled in the whole food chain, including fish flesh and fish oil (Berntssen et al., 2017). HCB highly tends to be absorbed in the human body with direct damage to the liver and possesses both acute and subchronic toxicity. It can disrupt the function of glycolysis enzymes and slow down the metabolism (Mazzetti et al., 2004). Whereas, genotoxic effects have also been proven in case of prolonged or frequent exposure to HCB (Ezendam et al., 2004). Most importantly, the pollutant is classified among the carcinogens of group 2B, making its complete avoidance necessarily important, which seems impossible if the pollutant is a part of our daily diet. Limiting HCB contents in agri-food products can be of great assistance in this task. Therefore, some strategies must be developed to control aero-concentration and bioaccumulation of HCB in agricultural crops, especially leafy vegetables, e.g., Brassica alboglabra) which are directly consumed by humans.
Gibberellic Acid (GA3) is the parent molecule of hundreds of gibberellins (GAs) classified as plant growth regulators. Studies have reported multi-directional regulation of plant responses under the influence of GAs. GA3 is a tetracyclic diterpenoid molecule bearing a carboxy group, two hydroxyl groups, and a lactone ring. Researchers have utilized GA3 and its derivatives to control seed germination, plant growth (vigor and height), flowering time, leaf senescence, etc. Considering its diverse physiological effects in plants, GA3 was selected to assess its ability to reduce HCB absorption from the surrounding air. This is the first time the molecule has been tested to limit the absorption and translocation of a POP. However, kinetics models show that the half-life of GA3 is gradually lowered at a temperature higher than 20°C. The half-life of GA3 is only 2 h at 50°C, which is occasionally achieved during the summers of hot regions (Palmer, 1974). However, providing continuous and minute delivery of GA3 to plant cells is a necessary task, rather than spraying larger currents of the growth regulator.
Ever-increasing environmental temperature due to global warming makes the situation more challenging for GA3 delivery. High-temperature fluctuations not only dissipate GA3, but also abnormalize plant development. Temperature is one of the most important phenological stimulators, and induces diverse physiological responses in the plants depending upon their developmental stages (Bahuguna and Jagadish, 2015; Ahmad et al., 2018). Small fluctuations in the temperature cause serious disturbances in the phenological cycle of the crops, which could be a food security threat if the plants are already under stress conditions (Khan et al., 2018; Zaheer et al., 2018). The temperature has been well-documented for its role in regulating almost all the physiological and developmental processes, including stomatal conductivity, water contents, photosynthesis, plant growth, fruit senescence, fruit ripening, etc. (Zandalinas et al., 2018; Farooq et al., 2019). Increased temperature impacts the enzymes’ activities and drives the plants under stress by disturbing evapotranspiration rates (Lamaoui et al., 2018). The modulated enzyme activities become a leading cause of altered protein concentrations, leaf water contents, sugar contents, osmoprotectants, and oxidative enzymes, e.g., superoxide dismutase (SOD), ascorbate peroxidase (APX), and peroxidase (POD) (Sattar et al., 2020). Not only the high temperatures but the low temperature also abnormalizes the plant’s growth, antioxidants activity, chlorophyll contents, reactive oxygen species (ROS), rate of photosynthesis, and several other physiological factors.
Brassica is an oil seed-producing plant that is famous for its consumption as a leafy vegetable and animal feed. Growing such an important crop in an HCB polluted environment causes the pollutant to be absorbed, translocated, and preserved in the lipid-rich plant parts, e.g., seeds. It is very important to restrict the entry of the pollutant into the plant foliage to keep the plant free of HCB contents. On the other side, research on foliar uptake of organic POPs and the way to control their accumulations is dearth studied.
To make plants withstand temperature fluctuations and to release stable and slow release of GA3 on the plant surface, we selected BioClay as a delivery agent. BioClay is a sheet-like clay nanoparticle with functional layered double hydroxide clay nanosheets that precipitates naturally in saline water bodies or through the weathering of basalts. BioClay nanosheets are a family of inorganic layered materials (Ram Reddy et al., 2006, 2008; Xu et al., 2006), which can be synthesized using a cost-effective protocol (Xu et al., 2006). The main features of BioClay are to keep acidic molecules stable and release slowly on the plant surfaces. The BioClay can be topically applied to plants for increased plant growth and defense parameters (Mitter et al., 2017). Therefore, the present research was designed to investigate the role of GA3-coated BioClay (BioClayGA) in alleviating multiple stress (HCB and temperature) conditions in Kale plants and their joint effort to control the bioaccumulation of the toxic organic contaminant. A number of positive physiological impacts have already been documented against the BioClay application (Fletcher et al., 2020). Henceforth, it was hypothesized that the combined application of BioClay with GA3 might have beneficial effects on plant stress alleviation and growth improvement. According to our information, no investigation has been executed to elucidate the role of GA3 or/and BioClay in alleviating the effects of multiple environmental stressors. Therefore, during the current study, the potential of GA3 impregnated BioClay was first time evaluated to alleviate HCB and temperature stress in B. alboglabra plants. This study elucidates the role of BioClayGA on growth, antioxidative system, and gaseous exchange attributes of B. alboglabra plants under HCB and temperature stress.
Materials and methods
Plant material and growth conditions
Brassica seeds were submerged in potassium permanganate (0.1%) for 15 min to ensure their surface sterilization and then washed three times with sterilized deionized water to completely remove any traces of the sterilizing agent. Bedding two Whatman filter papers prepared glass jars, and standard Davtyan (10 mL, 0.75 N) nutrient solution was flooded to maintain the moisture. Then, 10 sterilized seeds were placed into each jar under very low light and placed (Ahmad and Ashraf, 2016). All the jars were exposed to dark and 2°C temperatures for five days in a plant growth chamber. Five-day-old seedlings were transferred to soil pots at the rate of two seedlings per pot, while the pots contained one kilogram of UQ23 pot soil.
BioClayGA synthesis
The method of Mitter et al. (2017) was strictly followed to prepare BioClay nanosheets. The process included non-aqueous precipitation, heat treatment, purification step, and dispersion in water. The complete procedure yielded an average particle size of 45 nm, which was analyzed by a Nanosizer, Nano ZS instrument (Malvern Instruments) to obtain the Z average size and PdI. The chemical composition and crystal structure were verified by powder XRD with five BioClay samples (Rigaku Miniflex X-Ray diffractometer), Fourier transforms infrared spectroscopy (Nicolet 6700 FT-IR; Thermo Electron Corporation) with attenuated total reflection mode (Xu et al., 2006) and imaged by JEOL transmission Electron-microscope, JSM-2010.
Loading of GA3
To define optimal and complete loading of respective GA3 into BioClay nanosheets, the ratio of GA3 to BioClay was adjusted to 1:10. A mixture of GA3 10 mL and BioClay 100 mL was prepared and incubated at room temperature for 30 min with continuous agitation of 120 rpm. Quantifying the residual GA3 was done using Sun et al.’s standard method (Sun et al., 2020). HPLC system equipped with ZORBAX-Eclipse XDB-C18 column (4.6 × 250 mm, 5 μm, Agilent) separated the free GA3 molecules in an aqueous mobile phase of 40% methanol flowing at the rate of 0.4 mL min–1. Samples of 10 μL were separately processed at 40°C, and absorbance readings were recorded at 210 nm. The stable release of GA3 was also ensured at three different incubation temperatures 0, 25, and 50°C for a period of sixty by using the method described above.
Treatment application
GA3 (C19H22O6) was purchased from RPI Research Products International, CAS # 77-06-5. The growth regulator was initially dissolved in ethanol to prepare a stock concentration of (0.5 mM L–1), and then diluted with distilled sterilized water to attain a concentration of 5 μM L–1 as a working concentration recommended by Miao et al. (2017). Similarly, the BioClayGA solution was prepared to get the final working concentration of 0.5 mM L–1 for GA3. However, the concentration of BioClay was 1 g L–1. The third treatment of BioClay nanoparticles was also prepared (1 g L–1). Each seedling received 0.5 mL of the respective spray treatment.
To determine the HCB uptake pathway in the Brassica plants, specially designed incubation chambers (ICs) were introduced in the experimental design. The ICs consisted of glass materials, and the design and manufacturing pattern was adopted from a previous study (Zhu et al., 2016). The design had two separate air inlets, among which one was used for HCB and the second was for air. Both inlets had electrically controlled air pumps wired and connected with polytetrafluoroethylene hoses. The ICs had their own temperature sensing and regulatory system, while an air sampling window was also installed in every IC to collect a sample without significantly disturbing the experimental setup. Fine silica sand with a particle size of 150-380 μm was used to cover the potting soil to avoid direct contact between the HCB-contaminated air and the potting soil (Zhu et al., 2020). The HCB concentration in the air was adjusted to 1,000 mg m–3 as derived from its minimum toxic concentration of 582.4 mg m–3 and kept constant throughout the incubation period (Jarrell et al., 2002). In order to compensate for the time course losses of particulate on the plant surface, the POP dust was sprayed twice a week. Afterward, the growth conditions in ICs were maintained as relative humidity (70-76%), photoperiod (16 h), and light (500-550 mmol m–2 s–1). Five biological replications were used for each treatment.
Temperature treatment
Brassica seedlings were treated with a temperature shock of 0, 5, 15, 25 (experimental temperature), 35, 45, and 50°C for 2 h during the light period (Jiang et al., 2018). By following this design, the experiment contained a total of 124 treatments, including one control treatment (Supplementary Table 1). The entire experiment was incubated until seed production. Plant tissues (roots, shoots, and leaves) were randomly sampled at the age of 8 weeks.
Breakdown of BioClay and release of GA3
Breakdown of BioClay and release of GA3 were necessary to expose plants to GA3 treatment. To detect the degradation, 100 μL of BioClay suspension was dispensed in 20 droplets on detached Brassica alboglabra leaves (four replicates) with a similar surface area. Petri-plates were bedded with moistened filter papers, and the collected leaf samples were placed on them. The leaf petioles were immersed in Murashige-Skoog (MS) basal media (SigmaAldrich) in small glass vials taped to the dish. The Petri-plates were incubated in an incubation chamber with relative humidity (95%), CO2 (5%), and temperature (27°C). Leaf samples were collected after the interval of 1, 3, 5, and 7 days post-application of BioClay. The collected leaf samples were rinsed with a 10 mL aqueous solution of HNO3 (2%) and ethanol (3%). The rinse solutions were collected and analyzed through inductively coupled plasma-optical emission spectrometry (ICP-OES) to determine relative magnesium and aluminium ion concentrations. Four identical samples were processed separately. One sample was maintained outside the chamber at room temperature under normal atmospheric conditions (0.045% CO2). After 7 days of incubation, the tubes were centrifuged at 12,000 rpm for 15 min. The supernatant was pas processed at HPLC to detect the amount of free GA3 contents (Sun et al., 2020). HPLC system equipped with ZORBAX-Eclipse XDB-C18 column (4.6 × 250 mm, 5 μm, Agilent) separated the free GA3 molecules in an aqueous mobile phase of 40% methanol flowing at the rate of 0.4 mL min–1. Samples of 10 μL were separately processed at 40°C, and absorbance was read at 210 nm.
Stability of GA3 bound to BioClay
To check the stability of GA3, the release of the compound was tested at varying temperatures (0-50°C) using the similar HPLC method. Meanwhile, an aqueous GA3 solution of identical concentration was taken as a control treatment under similar temperature conditions. The samples were sprayed on Brassica leaves, and the method of GA3 detection was followed again as described earlier. After an incubation period of 1 month and 2 months, the samples were chromatographically analyzed for GA3 contents (Sun et al., 2020).
Determination of growth, antioxidant, and physiological parameters
After 8 weeks of incubation, all the plants were randomly sampled, and the collected samples were separated into the leaves, roots, and shoots. Each tissue was washed for one minute with the ddH2O and dried on blotting paper prior to its storage at −80°C for further analytical use. The plant samples were estimated for their growth parameters (shoot length, root length, shoot fresh mass, root fresh mass, shoot dry mass, root dry mass, SPAD chlorophyll, and leaf area), soluble sugars, net photosynthetic rate (Pn), photosynthetic pigments (Chl a, Chl b), photosystem quenching (Fv/Fm), gaseous exchange parameters, H2O2 contents, leaf osmotic potential, water potential, electrolyte leakage (EL), membrane stability index (MSI), proline content, lutein, lycopene, β-carotenoids, malondialdehyde (MDA), oxidative and antioxidant enzymatic activities (Carbonic anhydrase (CBH), nitrate reductase (NR), peroxidase (POD), catalase (CAT), superoxide dismutase (SOD), monodehydroascorbate reductase (MDHAR), dehydroascorbate reductase (DHAR), ascorbate peroxidase (APX), glutathione-S-transferase (GST), glutathione reductase (GR), and glutathione peroxidases (GPX)) by strictly following the standard optimized protocols (Li et al., 2021).
Determination of hexachlorobenzene in plants
The method of Liu et al. (2013) was adopted to estimate HCB contents in the plants. An accelerated solvent extraction system (ASE 200, Dionex, Sunnyvale, CA) was used to extract and collect chlorobenzenes (hexachlorobenzene and its metabolites) from Brassica plants. Each sample (seeds, shoots, and roots) was weighed (5 g) and homogenized with 5 g of diatomaceous earth. Whereas the physical conditions were kept at the temperature (90°C) and pressure (10 MPa). An extraction solvent was prepared by mixing hexane/acetone with the ratio of (3:1, vol/vol) and used to extract the chlorobenzenes. The extract was collected in the glass flask and evaporated under a vacuum at 45°C using a rotary evaporator (Yarong, Shanghai, China) to achieve the final volume of 2 mL of concentrated extracts. A step of solid-phase extraction was employed to clean the extracts by passing through the extraction cartridges containing Na2SO4 (2 g), and silica gel (1 g). A solvent system of hexane/dichloromethane (15 mL) was used at the ratio of 9:1 (vol/vol), to elute the extracts.
A pre-optimized gas chromatograph (Agilent 6890, Santa Clara, CA) was used to measure the concentrations of HCB and its metabolites. The gas chromatograph was equipped with a DB-5 capillary column (J&W Scientific, Folsom, CA) with the dimension 30-m length × 0.32-mm inside diameter × 0.25-μm film thickness. An HP 7683 auto-sampler (Hewlett Packard, Mississauga, ON) was used to load the sample on the chromatograph, and a 63Ni electron capture detector was used to plot the chromatograms. The carrier gas in the experimental operation was inert nitrogen. The temperature of the injector was 240°C, while the temperature of the detector was 290°C. One microliter of each sample was injected into the chromatograph in a splitless mode. The recovery for HCB in seeds, shoots and roots was 98.9, 97.4, and 93.7%, respectively. The sensitivity of the gas chromatograph to detect trace amounts of HCB was 0.5 pg μL–1.
Calculation of mobility, avoidance efficiency and pollutant tolerance index
The translocation factor (TF), and avoidance efficiency (AE) were used to evaluate the ability of the plants to absorb, translocate, and/or avoid pollutants, according to the following equations (Xiao et al., 2019; Zeng et al., 2019).
(1) TF = Csubstance shoot/Csubstance leaf
(2) AE = (Csubstance shoot × Mshoot × Csubstanceleaf × Mleaf)/(Csubstance air × Mair)
where the Csubstance shoot, Csubstance leaf, and Csubstance air are the contents of HCB (mg⋅kg–1) in shoots, roots, and air, respectively. However, the mass of one cubic meter of air was standardized as 1.29 kg during the experimentation.
Pollutant tolerance index (PTI) from plant biomass was calculated by employing the following formula of Deng et al. (2007):
Statistical analysis
Data were presented in the form of mean ± SE of the five biological replicates. Analysis of variance (ANOVA) was performed by the MS-Excel add-in statistical package DSAASTAT (Onofri, Italy). The significant differences among treatments were determined by Duncan’s Multiple Range Test (DMRT) at p ≥ 0.5.
Results
Structural estimation of BioClayGA
The structural analysis of BioClayGA revealed a crystalline nature of the nanosheets with 1.7-3.2 μm width and 80-150 nm thickness. An electron diffraction angle of 45°, and an interfacial angle of 43.4° were recorded. The interplanar lattice spacing, atomic plan spacing, and fringe spacing were measured at 0.0272, 0.35, and 0.384 nm, respectively (Supplementary Table 2). Assays clearly showed the GA3 molecules impregnated with BioClay nono sheets. Transmission electron micrograph, FTIR spectrum and XRD analysis collectively revealed a stable adhesion of GA3 with BioClay. The time-course release of GA3 was observed to be greater at a higher temperature (50°C). However, the rate of GA3 release possessed non-significant fluctuation over a period of 60 days (Figure 1).
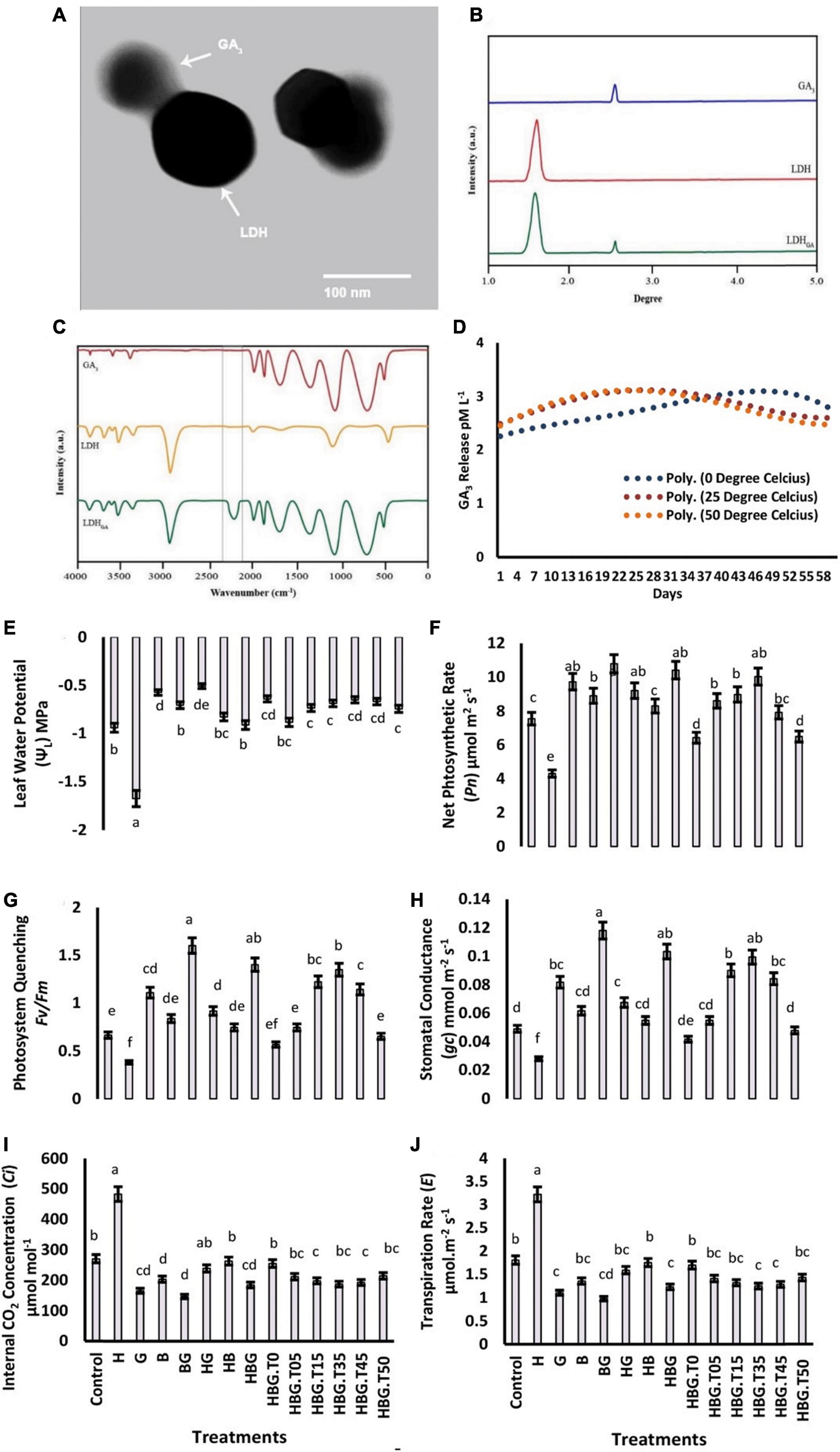
Figure 1. Electron microscopic image of bioclay nanoparticle with impregnated GA3 (A). X-ray diffraction analysis-XRD of gibberellic acid-GA3, bioclay-LDH, and gibberellic acid impregnated bioclay-LDHGA (B). Fourier-transform infrared spectroscopic-FTIR analysis of GA3, LDH, and LDHGA (C). Time course relesse of GA3 molecules by bioclay nanoparticles at three different temperatures, i.e., (0, 25, and 50 degree celcius (D). Effect of GA3 on photosynthesis-related parameters of Brassica alboglabra under HCB toxicity, and temperature stress. Leaf relative water content (E), Net photosynthesis rate (F), Maximum quality yield of PS-II (G), Stomatal conductance (H), Internal CO2 concentration (I), Transpiration rate (J). Values demonstrate means ± SD (n = 5). Different letters indicate a significant difference among the treatments (P ≤ 0.05). H = HCB; G = GA3, B = Bioclay, T0 = 0°C, T05 = 05°C, T15 = 15°C, T35 = 35°C, T45 = 45°C, T50 = 50°C. All treatments without temperature tags got a continuous incubation temperature of 25°C.
Determination of growth attributes
The results of the current study revealed that GA3 alone had the potential to improve the growth parameters of the plants, i.e., root fresh weight, shoot fresh weight, root dry weight, and shoot dry weight under multiple stress conditions of HCB toxicity, and temperature stress. All these growth credentials were enhanced 1.7 to 1.9 times after GA3 treatment. However, the most interesting observation was the positive support of BioClay toward plant growth. Plant growth parameters were elevated by 1.1 to 1.3 times due to BioClay application. The growth regulator GA3 enabled Brassica plants to overcome HCB toxicity by introducing pollutant tolerance in them. Besides, it also elevated the plant growth parameters up to 150%. However, BioClay could enhance the plant growth up to 120% primed with HCB. The maximum growth enhancement (241%) was recorded in the plants exposed to BioClayGA, and it could be reduced up to 211% under HCB toxicity. On the other side, the toxic effects of HCB caused a 50-57% reduction in growth attributes and leaf area of the plants as compared to the control treatment. GA3, bioclay, and BioClayGA proved effective in augmenting chlorophyll contents in Brassica plants. However, enhancement in chlorophyll contents was documented at 129% by GA3 application, 118% by BioClay treatment, and 143% by BioClayGA. HCB stresses plants also showed a significant increase of 138% in chlorophyll contents when primed with BioClayGA. Extreme temperature fluctuations from 25°C have deleterious effects on Brassica plants, retarding 15% of growth attributes, 18% of leaf area, and 14% of chlorophyll contents (Supplementary Figure 1).
Effect leaf relative water content
The results depicted the transposed effects of extreme temperatures and HCB on LRWC (43.55%, and 15.6%, respectively) as compared to control Brassica plants. Considering the temperature gradient, the maximum LRWC was recorded at 25 and 35°C. However, the GA3 treatment increased the value of LRWC (40.08%) as compared to the control. BioClay treatment also enhanced 32.41% LRWC when applied alone, while a 46.76% increment in LRWC was observed under BioClayGA treatment. The HCB treatment reduced Pn up to 47.62%. However, elevation in Pn due to GA3 and BioClay was 22.75%, and 18.96%, respectively. The BioClayGA application recorded the maximum Pn increase of 34.26%. The individual effect of BioClay and GA3 was found in favor of photosystem quenching Fv/Fm significantly higher than the effect of BioClay (20.62% increment). The most promising treatment providing the maximum increase in stomatal conductance, Ci, and transpiration rate were BioClayGA. However, the stress caused by extreme temperatures and HCB toxicity negatively impacted all these physiological parameters. Both the growth regulator and BioClay positively influenced the cell physiology of B. alboglabra (Figure 1).
Determination of photosynthetic pigments and soluble sugars
Hexachlorobenzene toxicity significantly reduced the photosynthetic pigments up to 42.95% in comparison to the control treatment. An increase of 1.29 times was observed by GA3 and 1.18 times by BioClay treatment. BioClayGA treatment provided more support toward total chlorophyll contents, augmenting them upto 240.94%. Low-temperature stress (0°C) caused a 14.77% chlorophyll reduction in Brassica plants, while 3.02% of chlorophyll contents were reduced due to high-temperature treatment (50°C). HCB signposted a significant decrease in the soluble sugar content of 3.15 mg g–1 as compared to 5.52 mg g–1 of control treatment. GA3 (6.73 mg g–1) and BioClay treatment (6.07 mg g–1) recorded significant mitigation of the sugar content. Whereas, BioClayGA enhanced soluble sugar contents up to (7.62 mg g–1) in the presence of HCB, while the contents were raised upto 7.89 mg g–1 in the absence of toxic HCB. Both low and high-temperature stress exerted deleterious effects on Brassica plants in terms of soluble sugars. However, BioClayGA mg g–1 were recorded at 0°C treatment (Table 1 and Figure 4).
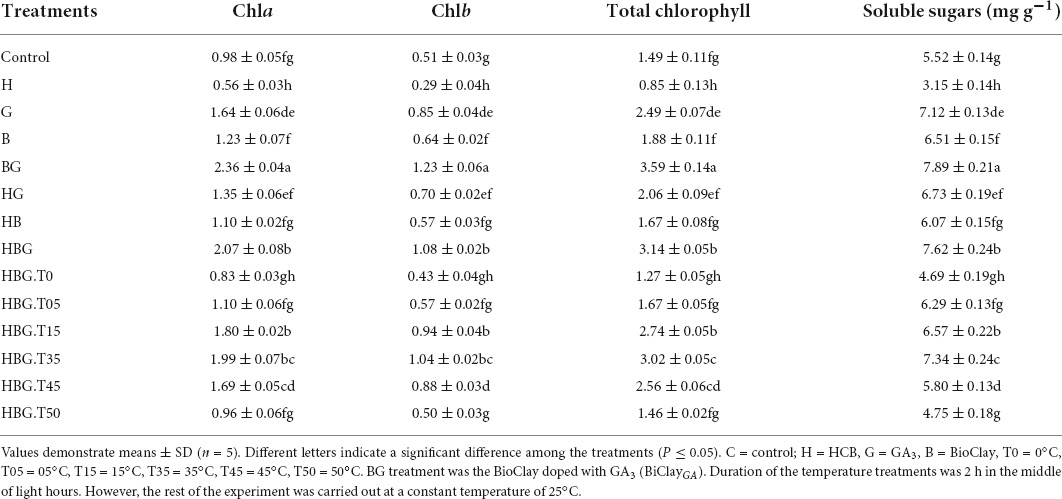
Table 1. Effect of GA3 on chlorophyll a, chlorophyll b, total chlorophyll, and soluble sugars of Brassica alboglabra under temperature stress, and hexachlorobenzene (HCB) toxicity.
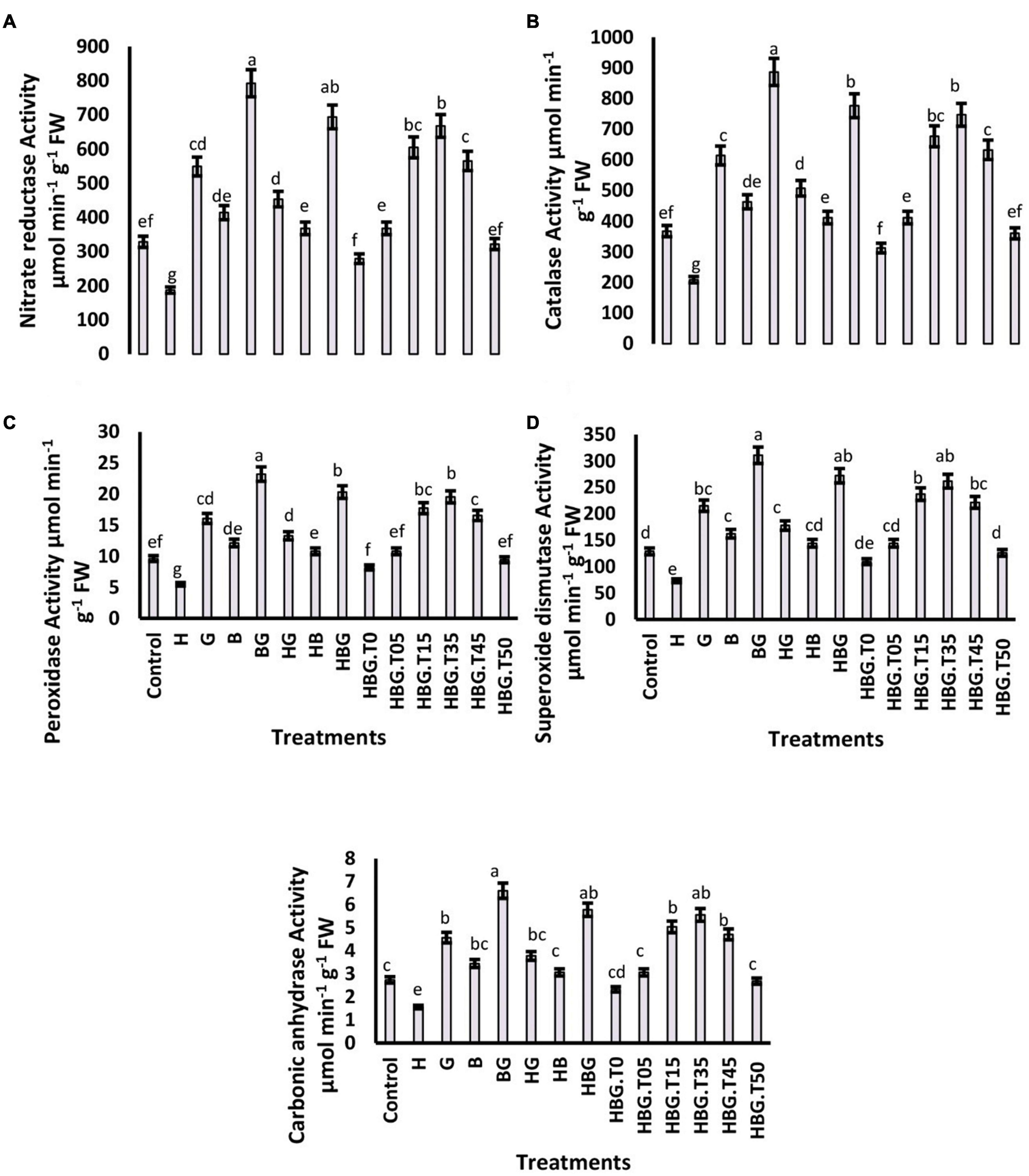
Figure 2. Effect of GA3 on antioxidant defense parameters of Brassica alboglabra under HCB toxicity, and temperature stress. Nitrate Reductase Activity (A), Catalase Activity (B), Peroxidase Activity (C), and Superoxide Dismutase Activity (D). Values demonstrate means ± SD (n = 5). Different letters indicate a significant difference among the treatments (P ≤ 0.05). H = HCB; G = GA3, B = Bioclay, T0 = 0°C, T05 = 05°C, T15 = 15°C, T35 = 35°C, T45 = 45°C, T50 = 50°C. All treatments without temperature tags got a continuous incubation temperature of 25°C.
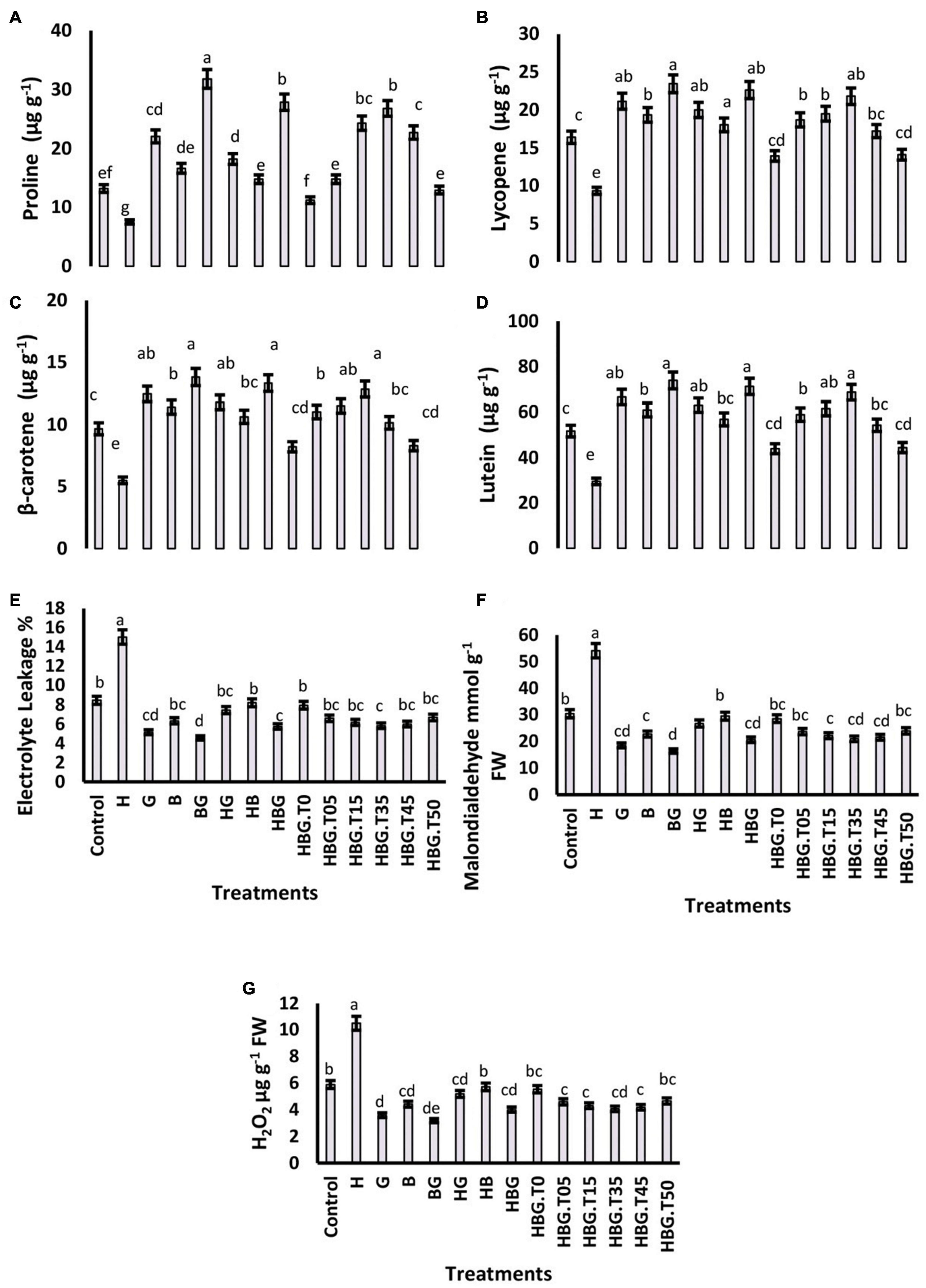
Figure 3. Effect of GA3 on physiological parameters of Brassica alboglabra under HCB toxicity, and temperature stress. Proline content (A), Lycopene content (B), β-carotene content (C), and Lutein content (D), Electrolyte leakage (E), Malondialdehyde content (F), and H2O2 content (G). Values demonstrate means ± SD (n = 5). Different letters indicate a significant difference among the treatments (P ≤ 0.05). H = HCB; G = GA3, B = Bioclay, T0 = 0°C, T05 = 05°C, T15 = 15°C, T35 = 35°C, T45 = 45°C, T50 = 50°C. All treatments without temperature tags got a continuous incubation temperature of 25°C.
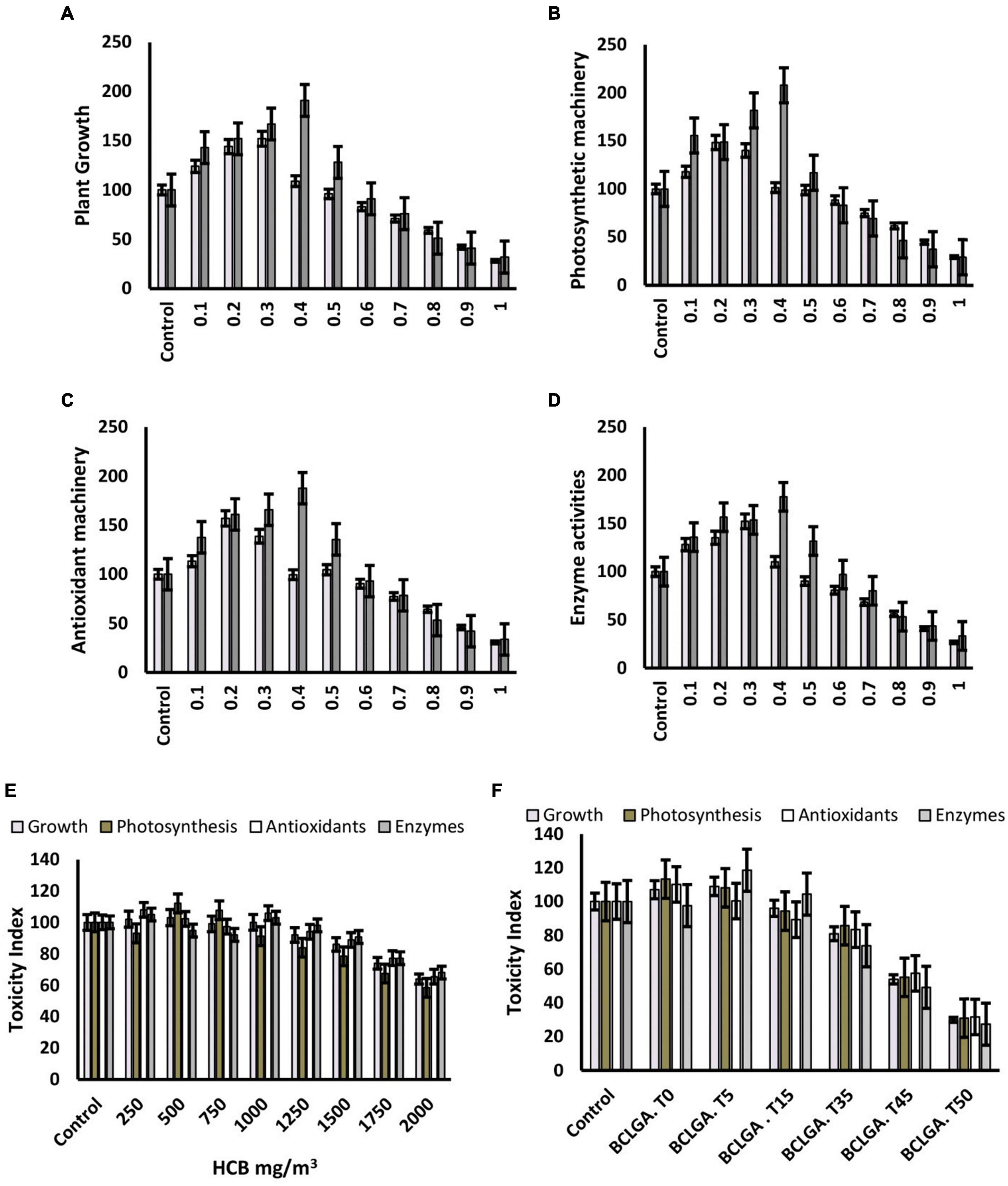
Figure 4. Nanotoxicity assessment of bioclay nanoparticles alone and in combination with gibberellic acid on Brassica alboglabra plant growth (A), photosynthesis (B), antioxidant machinery (C), and enzyme activities (D). The relation of nanotoxicity of bioclay under ambined application with gibberellic acid with HCB (E), and temperature (F). Values demonstrate means ± SD (n = 5). Different letters indicate a significant difference among the treatments (P ≤ 0.05). T0 = 0°C, T05 = 05°C, T15 = 15°C, T35 = 35°C, T45 = 45°C, T50 = 50°C. All treatments without temperature tags got a continuous incubation temperature of 25°C.
Antioxidant defenses
The activity of nitrate reductase was significantly reduced by toxic HCB contents, and elevated by the application of GA3 and bioclay. The most effective mitigation was resulted by BioClayGA, which elevated the enzyme activity by 238.4%. The extreme temperatures adversely affected the enzyme activity, and BioClayGA significantly mitigated temperature stress and HCB toxicity resulting in a 209.92% increase in nitrate reductase activity. Similar trends were observed in the case of other enzymes studied catalase, peroxidase, superoxide dismutase, and carbonic anhydrase. The activities of the enzymes were negatively affected by HCB toxicity and temperature fluctuations. Still, BioClayGA successfully alleviated the hazardous effects and elevated the enzyme activities up to 216%, strengthening the antioxidant defense of the plant (Figures 2, 4).
Enzymatic analysis
A significant increasing effect of GA3 was recorded in the enzymatic contents of monodehydroascorbate reductase (MDHAR), and dehydroascorbate reductase (DHAR), glutathione-s-transferase (GST), glutathione peroxidase (GPX), glutathione reductase (GR), and ascorbate peroxidase (APX) in B. alboglabra. BioClay treatment alone also enhanced the enzyme activities, but the extent of upregulation was significantly lower than GA3. The quantities of all these enzymes shared a similar trend of being elevated after BioClay and GA3 exposure and being reduced under toxic and stress conditions of fluctuated temperatures and the toxic environment of HCB. However, the mitigation role of BioClayGA was dominant and elevated all the tested stress factors (Table 2 and Figure 4).
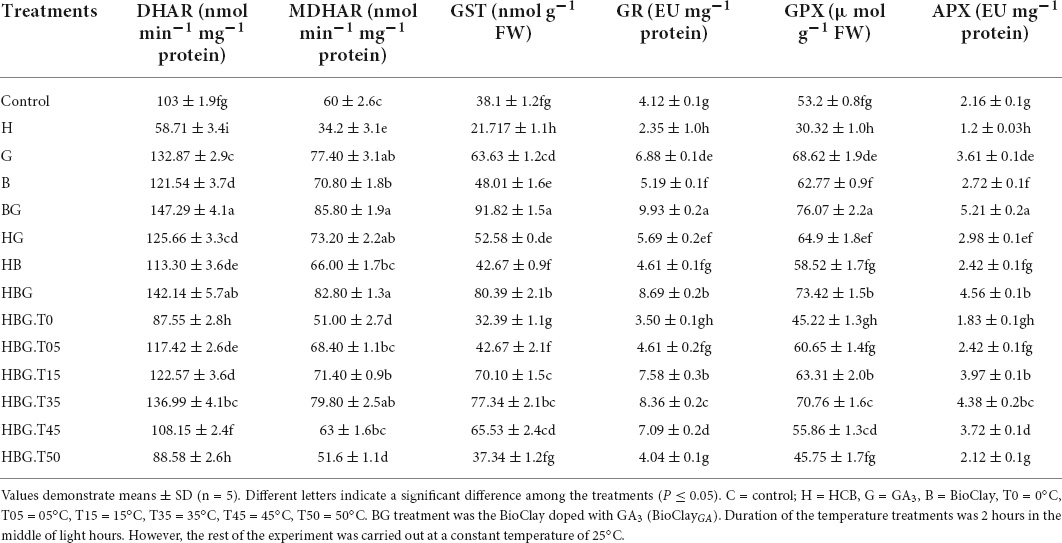
Table 2. Effect of GA3 on dehydroascorbate reductase (DHAR), monodehydroascorbate reductase (MDHAR), glutathione-s-transferase (GST), glutathione reductase (GR), glutathione peroxidase (GPX), and ascorbate peroxidase (APX), in Brassica alboglabra under temperature stress, and hexachlorobenzebe (HCB) toxicity.
Proline, lycopene, β-carotene and lutein contents
Results showed that proline contents were significantly retarded (35.21%) in the plants exposed to HCB. On the other side, GA3 increased proline contents 1.39 times as compared to the control treatment. BioClay treatment also exhibited positive effects on proline contents comparable with GA3. Lycopene, β-carotene, and lutein were also negatively regulated by the temperature extremes (low and high temperatures). However, the three treatments, i.e., GA3, BioClay, and BioClayGA, significantly enhanced all these biochemicals (Figure 3).
Electrolyte leakage MDA and H2O2
The EL was increased by HCB treatment and extreme temperatures, either low or high temperatures. An increase of 210.36% was recorded in EL due to HCB. However, the increased EL due to extreme temperatures was non-significant as compared to the control treatment. MDA was also increased by two times under the toxic influence of HCB. A similar trend was followed by H2O2 contents, which were elevated by toxic HCB and extreme temperatures (215.37 and 10.23%, respectively). Plant growth regulator GA3 and BioClay treatments successfully downregulated H2O2 contents reducing the risk of oxidative damage to plant cells (Figure 3).
Water potential, leaf osmotic potential, and membrane stability index
Exogenously applied GA3 caused a 128.57% enhancement in the leaf water potential of B. alboglabra. However, plants grown in HCB toxic environment contained 57.14% less leaf water potential. Similarly, extreme temperatures also adversely affected leaf water potential. Whereas, significant enhancement in leaf water potential and mitigation of HCB toxicity were observed by BioClay and BioClayGA treatments. A similar trend was found in leaf osmotic potential and membrane stability index (MSI). BioClayGA successfully mitigated the stress effects of extreme temperatures and HCB toxicity (Table 3).
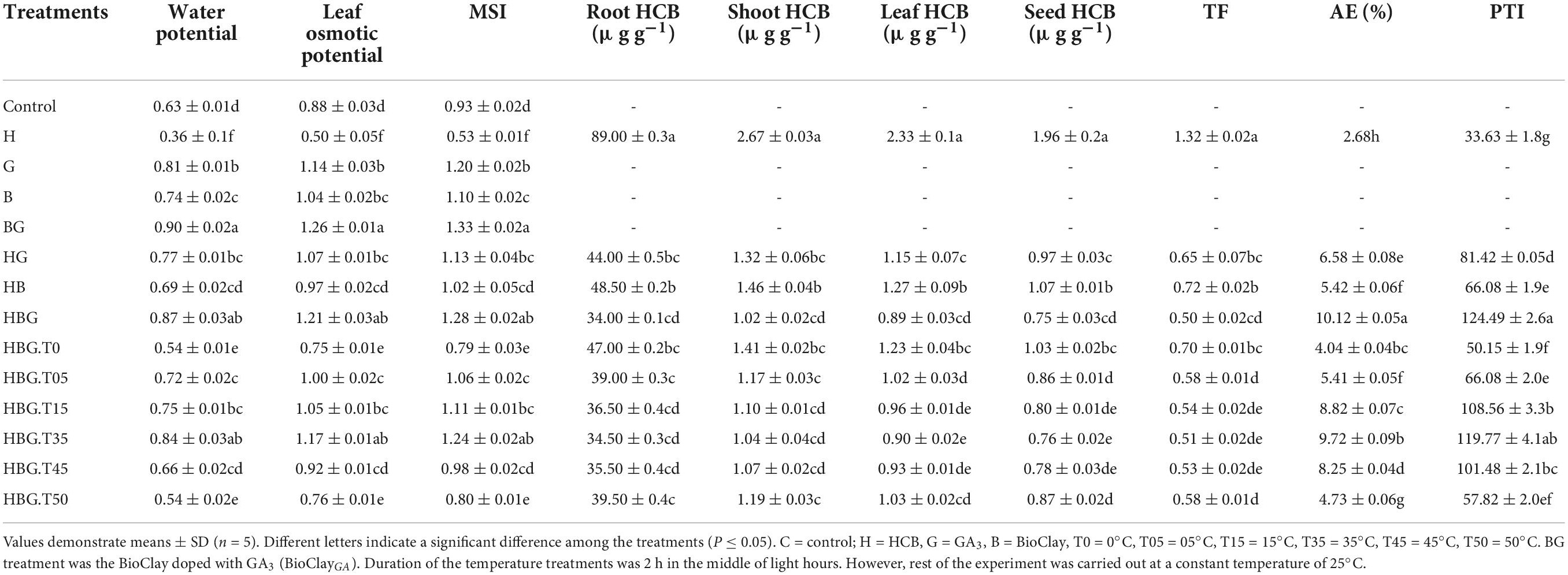
Table 3. Effect of GA3 and BioClay nanosheets on water potential, leaf osmotic potential, membrane stability index (MSI), root, shoot, leaf and seed HCB uptake, translocation factor (TF), avoidance efficacy (AE) and pollutant tolerance index (PTI) of Brassica alboglabra under temperature stress, and hexachlorobenzebe (HCB) toxicity.
Hexachlorobenzene contents, translocation factor and pollutant tolerance index
During the current study, the HCB accumulation was recorded higher in roots (89.0 μg g–1) as compared to shoots (2.67 μg g–1) and leaves (2.33). An interesting observation was of 1.96 μg g–1 HCB contents in the Brassica seeds. In extreme temperatures, especially the low temperature, was HCB accumulations enhancer facilitating HCB aero-concentration in the plant tissues as well as in seeds (0.86 μg g–1). Both the GA3 and BioClay significantly reduced the deleterious effect of HCB, and extreme temperatures lowering down the HCB accumulations in Brassica roots, shoots, leaves, and seeds. The most promising stress alleviation was inherited by BioClayGA treatment resulting in 0.75 μg g–1 HCB concentration in Brassica seeds. Three treatments, GA3, BioClay, and BioClayGA, bear an optimistic activity with respect to the TF of HCB. Brassica plants primed with GA3 showed a TF value of 0.65 in comparison to the 1.32 TF value of the control treatment. The minimum translocation of HCB (0.5) occurred in BioClayGA treated plants. Pollutant tolerance index in BioClayGA treated plants was also recorded at the maximum (124.49), followed by GA3 (81.42) and BioClay (66.08). However, extreme temperatures have detrimental effects on plants’ ability to tolerate the pollutant. Similarly, the plants’ avoidance efficacy (AE) was negatively impacted by the temperature fluctuations, whereas BioClay, GA3, and BioClayGA elevated the avoidance of the plants against HCB (Table 3 and Figure 4).
Discussion
For the first time, the present investigation sets a procedure for sustainable exogenous delivery of GA3 to a plant surface through impregnating with bioclay. GA3 is a plant hormone that is mainly responsible for cell elongation, internodal length, and defining plant height (Montague, 1993). The plant response toward GA3 is truly dose and duration dependent, making the exogenous application of GA3 to attain the desired results (Chandra et al., 2019). In this way, sustained availability of the fixed hormonal portion is essential to involve GA3 in field conditions to get the wanted results. The present research makes it simpler to support the GA3 portion of the plants during longer development periods by utilizing BioClay impregnated with GA3. Besides, GA3 bears the propensity to tune the genetic controls of the plant. The growth hormone is engaged with initializing the expression of starch and sugar-related h genetic cascade, which at last characterizes the plant’s energy economy (Chandra et al., 2019). This property of the phytohormone has been utilized to harmonize the physiological balances during stress conditions.
The stress factors (e.g., HCB) incite physiological imbalances and promote EL, and oxidative stress, leading to adaptive cellular responses in the form of solute accumulation (Shaddad et al., 2013). This establishes a groundwork for the expanded air fixation coefficient of HCB in various tissues of Brassica plants. Furthermore, the study first time decides the movement variable of HCB in B. alboglabra plant tissues, expounding the destiny of the toxin when consumed in the eatable plant. Additionally, the scientific investigation explored the mitigation behavior of BioClay, and GA3 by reharmonization of the stress indicators, e.g., EL, LRWC, gaseous exchange, antioxidant defenses, and enzymes’ activities. Ci, EL, Pn, leaf water potential, etc. are the main tools of environmental toxicants to retard plant growth and disturb metabolism (Khan et al., 2017; Yasin et al., 2018; Li et al., 2021). The study recommends BioClayGA as a resilient stress alleviator that can reharmonize the plant metabolism and rehabilitate plant cell homeostasis. The current study reports the BioClayGA as an effective stress alleviator that elevates the Pn, photosynthetic pigments (Chla, and Chlb), and total soluble sugars. This multitude of variables mutually restored the physiological elements and growth parameters because of work on the photosynthetic action of Brassica. Then again, temperature stress and HCB harmfulness are the significant stressors of the present climate that can shorten plan development and physiological properties. The augmented biosynthesis of chlorophyll contents positively affected the photosynthesis and growth of the plants treated by BioClayGA.
During stress conditions, the minimal objective of a plant is to make due through the shocking time, and the minimal objective of a rancher is to get sufficient respect to meet the development costs. This is achieved through the plant tolerance mechanism against stress factors (Ahmad et al., 2014b; Li et al., 2021). The study calculated the PTI for HCB-affected plants of B. alboglabra. Additionally, the AE of Brassica plants against toxic HCB was a prime finding of the current research work, which was calculated against twice the concentration of the pollutant reported harmful to the plants. Evasion is the best methodology to remediate the environmental pollutants, letting them out of the food chain for normal debasement (Waheed Ullah Khan et al., 2017). Using GA3 impregnated BioClay has been proved a unique technique, hampering the entry of HCB into the food chain, which would surely be detoxified by natural degradation with the passage of time.
Moreover, the alone and combined effect of BioClay with the phytohormone resulted in positive physiochemical alterations in plants. Beforehand a couple of studies involved BioClay for the effective conveyance of RNAi to get the plants safe against microorganisms (Fletcher et al., 2020). In any case, there was no extreme end in regards to the development guideline conduct of the nanosheets. The ongoing concentrate first time featured the steady job of the earth nanosheets toward plant development. It not only supported the growth parameters of B. alboglabra but also augmented antioxidant enzymes and other physiological factors. The study proved that the BioClay was of immense potential to be used as a plant growth enhancer under stress conditions. Having no negative cross-talk with GA3 is an advantageous feature of BioClay explored in this study.
LRWC value acts as a biomarker for plant-water relationships. High-temperature stress has negative effects on osmotic potential as well as water. Low temperature also negatively impacted LRWC values of Brassica plants, indicating reduced drought resistance (Wang et al., 2019). During current research, all the three test treatments, i.e., BioClay, GA3, and BioClayGA, significantly improved LRWC proving a good choice for low rainfall areas and areas adversely affected by global warming. Lipid peroxidation is one of the major reasons of membrane injuries, is one of the leading causes of disrupted metabolic patterns in the plant cells, and is considered a biomarker of cellular injuries for a plant under stress (Ayala et al., 2014). A majority of writing accessible fosters an association between expanded biosynthesis of H2O2 with oxidative wounds of the phones and extreme driving toward putrefaction (Ayala et al., 2014). Yet, BioClay and GA3 inhibited the biosynthesis of the oxidative stressor H2O2. Moreover, the utilization of BioClayGA decreased the MDA, the last result of lipid peroxidation, and demonstrated the proficiency of the treatment against natural stressors. The EL caused by damaged cellular membrane reduces plant growth and biomass production. A few examinations uncovered that the up-directed exercises of antioxidative hardware decline the blend of ROS in focused plants. Superoxide is detoxified into a less injurious H2O2 by the activity of SOD, which is subsequently converted into H2O through the activity of POD, CAT, and APX (Chen and Heuer, 2013). The exogenous utilization of BioClayGA upgraded pressure resistance by working on the action of the antioxidative framework in focused plants.Henceforward, the up-regulation in antioxidative machinery besides the decreased level of EL, H2O2, and MDA highlighted the favorable role of BioClayGA in the reduction of oxidative injuries and regulation of redox homeostasis in stressed plants.
Superoxide dismutase is ranked among the primary antioxidant defenses of the plant cell, crucial to surviving under stress conditions. Although SOD and CAT are mainly localized in peroxisomes and mitochondria of the plant cells (Zaheer et al., 2018), they still showed a significant response to stress conditions. However, BioClayGA treatment mitigated the stress physiology of B. alboglabra. The ongoing study gives nitty-gritty interrelation between GA3, bioclay, BioClayGA, H2O2, MDA, and cell reinforcements catalyst exercises. By taking into account the by and large physiological reactions of the plant, it tends to be presumed that every one of the three medicines (GA3, bioclay, and BioClayGA) strengthened plant defenses against environmental stresses and HCB toxicity. However, BioClayGA was the most promising formulation in this regard. The scavenging of reactive oxygen species (ROS) is a prime function in a plant cell to thrive under stress conditions (Abbas et al., 2020). H2O2 is the main source of ROS, and ascorbate is one of the most powerful substrates for scavenging H2O2. The higher ROS biosynthesis than it is being scavenged (by the antioxidant system) leads to irreversible oxidative damages (Ahmad et al., 2014a). Ascorbate, glutathione, and carotenoids belong to the non-enzymatic antioxidant defense system. However, DHAR, MDHAR, GST, GR, GPX, APX, SOD, and CAT are key components of the enzymatic antioxidant system (Konieczny et al., 2014). BioClayGA has carried out a double role to reinforce the oxidative guard arrangement of B. alboglabra while lifting the movement of cell reinforcements to build the guarded layers of the plant further.
Researchers have always been interested in increasing edible plants’ proline and carotenoids (lycopene, β-carotene, and lutein) contents. Carotenoids are the pigmented elements of plants which contribute to the photosynthetic machinery and perform a protective function against photo-damage (Singh and Sahota, 2018). Foods rich in carotenoid contents protect humans from age-related diseases. Lycopene is a major carotenoid in Brassica with characteristic red color and special antioxidant properties (Elbadrawy and Sello, 2016). Proline is an excellent part of protein biosynthesis hardware, which characterizes the cell structure, digestion, sustenance profile, wound recuperating, antioxidative responses, and protection reactions (Wu et al., 2011). Particularly proline plays a vital role in the stability of macromolecules structure and turgidity of cellular membrane structure in stressed plants. Proline scavenges ROS and attenuates stress in plants. During the present study, BioClayGA successfully enhanced proline and carotenoid contents in Brassica plants under multiple stress conditions. It hardened the plants and made them tolerant against temperature stress and HCB toxicity.
The increased HCB concentration in roots of plants growing in a POP toxic environment is because vacuole and cell walls of roots may amass and hold higher HCB contents compared to shoot cells (Dong et al., 2017). The exogenous application of BioClayGA decreased HCB contents in plants due to modified homeostasis. Moreover, every one of the advantages of lessening HCB contents in foliage and seeds of B. alboglabra is the principal accomplishment of the study, which lies with the limited section of HCB in the plant. Taking into account the exchange nature, persistance, bioaccumulation, and deadly impacts of HCB, the BioClayGA treatment accomplished a sign of hindering airborne contamination from entering the human food chain. Also, BioClayGA application decreased the ACF of the Brassica shoots, obstructing the HCB move at the initial step from air to food. The study can be expanded to seek the bio-concentration conduct of different POPs in food plants.
Conclusion
This study revealed that the combination of BioClay and GA3 successfully improved the plant growth and physiological performance of B. alboglabra under multiple stresses (hexachlorobenzene and temperature extreme). Moreover, exogenously applied BioClayGA3 ameliorated the combined stress of hexachlorobenzene and temperature extremes through decreased production of ROS, modulated synthesis of osmoregulators, and improved antioxidative system. BioClayGA3 inhibited air absorption of HCB and reduced its transfer coefficient among different plants. Accordingly, we are confident that the stress alleviation behavior of BioClayGA3 offers an innovative approach to sustainable agricultural practice for future food security.
Data availability statement
The original contributions presented in this study are included in the article/Supplementary material, further inquiries can be directed to the corresponding author.
Author contributions
AaA, NY, IS, SS, and WA conceived the idea, planned experiments, and managed resources for the study. SU, TK, AqA, and MY performed the experimentations, collected and anlayzed the data, and wrote the manuscript draft. AaA, NY, WA, IS, SS, SU, TK, AqA, and MY revised and finalized the manuscript. All authors contributed to the article and approved the submitted version.
Funding
Research funding was granted by Chinese Scholarship Council (CSC).
Conflict of interest
The authors declare that the research was conducted in the absence of any commercial or financial relationships that could be construed as a potential conflict of interest.
Publisher’s note
All claims expressed in this article are solely those of the authors and do not necessarily represent those of their affiliated organizations, or those of the publisher, the editors and the reviewers. Any product that may be evaluated in this article, or claim that may be made by its manufacturer, is not guaranteed or endorsed by the publisher.
Supplementary material
The Supplementary Material for this article can be found online at: https://www.frontiersin.org/articles/10.3389/fpls.2022.964041/full#supplementary-material
References
Abbas, H. M. K., Ahmad, A., Dong, W., Xiang, J., Iqbal, J., Ali, S., et al. (2020). Heterologous WRKY and NAC transcription factors triggered resistance in Nicotiana benthamiana. J. King Saud Univ. Sci. 32, 3005–3013. doi: 10.1016/j.jksus.2020.08.005
Ahmad, A., and Ashraf, Y. (2016). In vitro and in Vivo management of alternaria leaf spot of Brassica campestris L. J. plant pathol. microbiol. 7:1000365. doi: 10.4172/2157-7471.1000365
Ahmad, A., Shafique, S., and Shafique, S. (2014a). Molecular basis of antifungal resistance in tomato varieties. Pak. J. Agric. Sci. 51, 683–687.
Ahmad, A., Shafique, S., Shafique, S., and Akram, W. (2014b). Penicillium oxalicum directed systemic resistance in tomato against Alternaria alternata. Acta Physiol. Plant. 36, 1231–1240. doi: 10.1007/s11738-014-1500-5
Ahmad, A., Shahzadi, I., Mubeen, S., Yasin, N. A., Akram, W., Khan, W. U., et al. (2021). Karrikinolide alleviates BDE-28, heat and Cd stressors in Brassica alboglabra by correlating and modulating biochemical attributes, antioxidative machinery and osmoregulators. Ecotoxicol. Environ. Saf. 213:112047. doi: 10.1016/j.ecoenv.2021.112047
Ahmad, A., Yasin, N. A., Ibrahim, A., Shahzadi, I., Gohar, M., Bashir, Z., et al. (2018). Modelling of cotton leaf curl viral infection in Pakistan and its correlation with meteorological factors up to 2015. Clim. Dev. 10, 520–525. doi: 10.1080/17565529.2017.1318738
Ayala, A., Muñoz, M. F., and Argüelles, S. (2014). Lipid peroxidation: Production, metabolism, and signaling mechanisms of malondialdehyde and 4-hydroxy-2-nonenal. Oxid. Med. Cell. Longev. 2014, 1–31. doi: 10.1155/2014/360438
Bahuguna, R. N., and Jagadish, K. S. V. (2015). Temperature regulation of plant phenological development. Environ. Exp. Bot. 111, 83–90. doi: 10.1016/j.envexpbot.2014.10.007
Berntssen, M. H. G., Maage, A., and Lundebye, A. K. (2017). “Chemical contamination of finfish with organic pollutants and metals,” in Chemical contaminants and residues in food, 2nd Edn (Amsterdam: Elsevier Inc), 517–551. doi: 10.1016/B978-0-08-100674-0.00020-5
Chandra, A., Roopendra, K., and Verma, I. (2019). Transcriptome analysis of the effect of GA3 in sugarcane culm. 3 Biotech 9, 1–12. doi: 10.1007/s13205-019-1908-0
Chen, S., and Heuer, B. (2013). Effect of genotype and exogenous application of glycinebetaine on antioxidant enzyme activity in native gels of 7-day-old salt-stressed tomato (Solanum lycopersicum) seedlings. Sci. Hortic. (Amsterdam) 162, 106–116. doi: 10.1016/j.scienta.2013.07.001
Deng, D. M., Shu, W. S., Zhang, J., Zou, H. L., Lin, Z., Ye, Z. H., et al. (2007). Zinc and cadmium accumulation and tolerance in populations of Sedum alfredii. Environ. Pollut. 147, 381–386. doi: 10.1016/j.envpol.2006.05.024
Dong, Q., Xu, P., and Wang, Z. (2017). Differential cadmium distribution and translocation in roots and shoots related to hyper-tolerance between tall fescue and Kentucky bluegrass. Front. Plant Sci. 8:113. doi: 10.3389/fpls.2017.00113
Elbadrawy, E., and Sello, A. (2016). Evaluation of nutritional value and antioxidant activity of tomato peel extracts. Arab. J. Chem. 9, S1010–S1018. doi: 10.1016/j.arabjc.2011.11.011
Ezendam, J., Hassing, I., Bleumink, R., Vos, J. G., and Pieters, R. (2004). Hexachlorobenzene-induced immunopathology in Brown Norway rats is partly mediated by T cells. Toxicol. Sci. 78, 88–95. doi: 10.1093/toxsci/kfh034
Farooq, M., Hussain, M., Ul-Allah, S., and Siddique, K. H. M. (2019). Physiological and agronomic approaches for improving water-use efficiency in crop plants. Agric. Water Manage. 219, 95–108. doi: 10.1016/j.agwat.2019.04.010
Fletcher, S. J., Reeves, P. T., Hoang, B. T., and Mitter, N. (2020). A perspective on RNAi-based biopesticides. Front. Plant Sci. 11:51. doi: 10.3389/fpls.2020.00051
Ibrahim, A., Shahid, A. A., Noreen, S., and Ahmad, A. (2016). Physiological changes against meloidogyne incognita in rhizobacterial treated eggplant under organic conditions. J. Anim. Plant Sci. 26, 805–813.
Jarrell, J. F., Gocmen, A., Akyol, D., and Brant, R. (2002). Hexachlorobenzene exposure and the proportion of male births in Turkey 1935-1990. Reprod. Toxicol. 16, 65–70. doi: 10.1016/S0890-6238(01)00196-4
Jiang, J., Bai, J., Li, S., Li, X., Yang, L., and He, Y. (2018). HTT2 promotes plant thermotolerance in Brassica rapa. BMC Plant Biol. 18:127. doi: 10.1186/s12870-018-1346-x
Khan, T. A., Yusuf, M., and Fariduddin, Q. (2018). Hydrogen peroxide in regulation of plant metabolism: Signalling and its effect under abiotic stress. Photosynthetica 56, 1237–1248. doi: 10.1007/s11099-018-0830-8
Khan, W. U., Ahmad, S. R., Yasin, N. A., Ali, A., Ahmad, A., and Akram, W. (2017). Application of Bacillus megaterium MCR-8 improved phytoextraction and stress alleviation of nickel in Vinca rosea. Int. J. Phytoremediation 19, 813–824. doi: 10.1080/15226514.2017.1290580
Konieczny, R., Banaś, A. K., Surówka, E., Michalec, Ż, Miszalski, Z., and Libik-Konieczny, M. (2014). Pattern of antioxidant enzyme activities and hydrogen peroxide content during developmental stages of rhizogenesis from hypocotyl explants of Mesembryanthemum crystallinum L. Plant Cell Rep. 33, 165–177. doi: 10.1007/s00299-013-1520-4
Lamaoui, M., Jemo, M., Datla, R., and Bekkaoui, F. (2018). Heat and drought stresses in crops and approaches for their mitigation. Front. Chem. 6:26. doi: 10.3389/fchem.2018.00026
Li, G., Shah, A. A., Khan, W. U., Yasin, N. A., Ahmad, A., Abbas, M., et al. (2021). Hydrogen sulfide mitigates cadmium induced toxicity in Brassica rapa by modulating physiochemical attributes, osmolyte metabolism and antioxidative machinery. Chemosphere 263:127999. doi: 10.1016/j.chemosphere.2020.127999
Liu, C. Y., Jiang, X., Fan, J. L., and Ziadi, N. (2013). Hexachlorobenzene accumulation in rice plants as affected by farm manure and urea applications in dissimilar soils. Can. J. Soil Sci. 93, 631–638. doi: 10.4141/CJSS2013-001
Mazzetti, M. B., Taira, M. C., Lelli, S. M., Dascal, E., Basabe, J. C., and de Viale, L. C. S. M. (2004). Hexachlorobenzene impairs glucose metabolism in a rat model of porphyria cutanea tarda: A mechanistic approach. Arch. Toxicol. 78, 25–33. doi: 10.1007/s00204-003-0470-y
Miao, H. Y., Wang, M. Y., Chang, J. Q., Tao, H., Sun, B., and Wang, Q. M. (2017). Effects of glucose and gibberellic acid on glucosinolate content and antioxidant properties of Chinese kale sprouts. J. Zhejiang Univ. Sci. B 18, 1093–1100. doi: 10.1631/jzus.B1700308
Mitter, N., Worrall, E. A., Robinson, K. E., Li, P., Jain, R. G., Taochy, C., et al. (2017). Clay nanosheets for topical delivery of RNAi for sustained protection against plant viruses. Nat. Plants 3:16207. doi: 10.1038/nplants.2016.207
Montague, M. J. (1993). Calcium antagonists inhibit sustained gibberellic acid-induced growth of Avena (oat) stem segments. Plant Physiol. 101, 399–405. doi: 10.1104/pp.101.2.399
Palmer, G. H. (1974). The industrial use of gibberellic acid and its scientific basis-a review. J. Inst. Brew. 80, 13–30. doi: 10.1002/j.2050-0416.1974.tb03578.x
Ram Reddy, M. K., Xu, Z. P., Lu (Max), G. Q., and Diniz da Costa, J. C. (2006). Layered double hydroxides for CO2 capture: Structure evolution and regeneration. Ind. Eng. Chem. Res. 45, 7504–7509. doi: 10.1021/ie060757k
Ram Reddy, M. K., Xu, Z. P., Lu (Max), G. Q., and Diniz da Costa, J. C. (2008). Effect of SOx adsorption on layered double hydroxides for CO2 capture. Ind. Eng. Chem. Res. 47, 7357–7360. doi: 10.1021/ie8004226
Sattar, A., Sher, A., Ijaz, M., Ul-Allah, S., Rizwan, M. S., Hussain, M., et al. (2020). Terminal drought and heat stress alter physiological and biochemical attributes in flag leaf of bread wheat. PLoS One 15:e0232974. doi: 10.1371/journal.pone.0232974
Shaddad, M. A. K., Abd El-Samad, H. M., and Mostafa, D. (2013). Role of gibberellic acid (GA 3) in improving salt stress tolerance of two wheat cultivars. Int. J. Plant Physiol. Biochem. 5, 50–57. doi: 10.5897/IJPPB11.055
Singh, G., and Sahota, H. K. (2018). Impact of benzimidazole and dithiocarbamate fungicides on the photosynthetic machinery, sugar content and various antioxidative enzymes in chickpea. Plant Physiol. Biochem. 132, 166–173. doi: 10.1016/j.plaphy.2018.09.001
Sun, W., Liu, C., Luo, J., Niu, C., Wang, J., Zheng, F., et al. (2020). Residue analysis of gibberellic acid isomer (iso-GA3) in brewing process and its toxicity evaluation in mice. Regul. Toxicol. Pharmacol. 110:104514. doi: 10.1016/j.yrtph.2019.104514
Wang, Q., Zhao, R., Chen, Q., Teixeira Da Silva, J. A., Chen, L., and Yu, X. (2019). Physiological and biochemical responses of two herbaceous peony cultivars to drought stress. Hortscience 54, 492–498. doi: 10.21273/HORTSCI13704-18
Wang, S.-L., Tsai, P.-C., Yang, C.-Y., and Leon Guo, Y. (2008). Increased risk of diabetes and polychlorinated biphenyls and dioxins: A 24-year follow-up study of the Yucheng cohort. Diabetes Care 31, 1574–1579. doi: 10.2337/dc07-2449
Wu, G., Bazer, F. W., Burghardt, R. C., Johnson, G. A., Kim, S. W., Knabe, D. A., et al. (2011). Proline and hydroxyproline metabolism: Implications for animal and human nutrition. Amino Acids 40, 1053–1063. doi: 10.1007/s00726-010-0715-z
Xiao, R., Ali, A., Wang, P., Li, R., Tian, X., and Zhang, Z. (2019). Comparison of the feasibility of different washing solutions for combined soil washing and phytoremediation for the detoxification of cadmium (Cd) and zinc (Zn) in contaminated soil. Chemosphere 230, 510–518. doi: 10.1016/j.chemosphere.2019.05.121
Xu, Z. P., Stevenson, G. S., Lu, C.-Q. Q., Lu (Max), G. Q., Bartlett, P. F., and Gray, P. P. (2006). Stable suspension of layered double hydroxide nanoparticles in aqueous solution. J. Am. Chem. Soc. 128, 36–37. doi: 10.1021/ja056652a
Yasin, N. A., Akram, W., Khan, W. U., Ahmad, S. R., Ahmad, A., and Ali, A. (2018). Halotolerant plant-growth promoting rhizobacteria modulate gene expression and osmolyte production to improve salinity tolerance and growth in Capsicum annum L. Environ. Sci. Pollut. Res. 25, 23236–23250. doi: 10.1007/s11356-018-2381-8
Zaheer, M. M. M. M., Yasin, N. A. N. A., Ahmad, S. R. S. R., Khan, W. U. W. U., Ahmad, A., Ali, A., et al. (2018). Amelioration of cadmium stress in gladiolus (Gladiolus grandiflora L.) by application of potassium and silicon. J. Plant Nutr. 41, 461–476. doi: 10.1080/01904167.2017.1385808
Zandalinas, S. I., Mittler, R., Balfagón, D., Arbona, V., and Gómez-Cadenas, A. (2018). Plant adaptations to the combination of drought and high temperatures. Physiol. Plant. 162, 2–12. doi: 10.1111/ppl.12540
Zeng, H., Chen, L., Zhou, X., and Zeng, Q. (2019). Cadmium accumulation in winter crops and the assessment of paddy soil phytoremediation in southern China. Environ. Sci. Pollut. Res. 26, 17173–17182. doi: 10.1007/s11356-019-05054-9
Zhu, H., Sun, H., Zhang, Y., Xu, J., Li, B., and Zhou, Q. (2016). Uptake pathway, translocation, and isomerization of hexabromocyclododecane diastereoisomers by wheat in closed chambers. Environ. Sci. Technol. 50, 2652–2659. doi: 10.1021/acs.est.5b05118
Keywords: BioClay, Brassica alboglabra, HCB, stress, temperature extremes
Citation: Ahmad A, Khan TA, Shahzad S, Ullah S, Shahzadi I, Ali A, Akram W, Yasin NA and Yusuf M (2022) BioClay nanosheets infused with GA3 ameliorate the combined stress of hexachlorobenzene and temperature extremes in Brassica alboglabra plants. Front. Plant Sci. 13:964041. doi: 10.3389/fpls.2022.964041
Received: 08 June 2022; Accepted: 28 July 2022;
Published: 06 October 2022.
Edited by:
Anket Sharma, Zhejiang Agriculture and Forestry University, ChinaReviewed by:
Muhammad Faheem Adil, Zhejiang University, ChinaMohd Asgher, Baba Ghulam Shah Badshah University, India
Copyright © 2022 Ahmad, Khan, Shahzad, Ullah, Shahzadi, Ali, Akram, Yasin and Yusuf. This is an open-access article distributed under the terms of the Creative Commons Attribution License (CC BY). The use, distribution or reproduction in other forums is permitted, provided the original author(s) and the copyright owner(s) are credited and that the original publication in this journal is cited, in accordance with accepted academic practice. No use, distribution or reproduction is permitted which does not comply with these terms.
*Correspondence: Mohammad Yusuf, bXl1c3VmLmFsaWdAdWFldS5hYy5hZQ==
†These authors have contributed equally to this work