- 1Plant Biology and Biofunctional Chemistry Lab, Department of Biochemistry and Molecular Biology, Bangladesh Agricultural University, Mymensingh, Bangladesh
- 2Institute of Oceanography, College of Geography and Oceanography, Minjiang University, Fuzhou, China
- 3Department of Biology, York University, Toronto, ON, Canada
- 4Department of Biotechnology, Bangladesh Agricultural University, Mymensingh, Bangladesh
- 5Breeding Division, Bangladesh Sugarcrop Research Institute, Pabna, Bangladesh
- 6Department of Horticultural Sciences, Faculty of Agriculture and Environment, The Islamia University of Bahawalpur, Bahawalpur, Pakistan
- 7Environmental Horticulture Department and Mid-Florida Research and Education Center, Institute of Food and Agricultural Sciences, University of Florida, Apopka, FL, United States
- 8Department of Agronomy, Faculty of Agriculture and Environment, The Islamia University of Bahawalpur, Bahawalpur, Pakistan
- 9Department of Plant Pathology, Bangladesh Agricultural University, Mymensingh, Bangladesh
- 10Faculty of Animal Husbandry, Bangladesh Agricultural University, Mymensingh, Bangladesh
- 11Department of Plant Production, College of Food and Agriculture, King Saud University, Riyadh, Saudi Arabia
- 12Agronomy Department, Faculty of Agriculture, Al-Azhar University, Cairo, Egypt
- 13Faculty of Agriculture, Department of Agricultural Biotechnology, Siirt University, Siirt, Turkey
- 14Department of Field Crops, Faculty of Agriculture, Siirt University, Siirt, Turkey
- 15Department of Agronomy, Faculty of Agriculture, Kafrelsheikh University, Kafr el-sheikh, Egypt
- 16Graduate School of Environmental and Life Science, Okayama University, Okayama, Japan
Nitric oxide (NO) has received much attention since it can boost plant defense mechanisms, and plenty of studies have shown that exogenous NO improves salinity tolerance in plants. However, because of the wide range of experimental settings, it is difficult to assess the administration of optimal dosages, frequency, timing, and method of application and the overall favorable effects of NO on growth and yield improvements. Therefore, we conducted a meta-analysis to reveal the exact physiological and biochemical mechanisms and to understand the influence of plant-related or method-related factors on NO-mediated salt tolerance. Exogenous application of NO significantly influenced biomass accumulation, growth, and yield irrespective of salinity stress. According to this analysis, seed priming and foliar pre-treatment were the most effective methods of NO application to plants. Moreover, one-time and regular intervals of NO treatment were more beneficial for plant growth. The optimum concentration of NO ranges from 0.1 to 0.2 mM, and it alleviates salinity stress up to 150 mM NaCl. Furthermore, the beneficial effect of NO treatment was more pronounced as salinity stress was prolonged (>21 days). This meta-analysis showed that NO supplementation was significantly applicable at germination and seedling stages. Interestingly, exogenous NO treatment boosted plant growth most efficiently in dicots. This meta-analysis showed that exogenous NO alleviates salt-induced oxidative damage and improves plant growth and yield potential by regulating osmotic balance, mineral homeostasis, photosynthetic machinery, the metabolism of reactive oxygen species, and the antioxidant defense mechanism. Our analysis pointed out several research gaps, such as lipid metabolism regulation, reproductive stage performance, C4 plant responses, field-level yield impact, and economic profitability of farmers in response to exogenous NO, which need to be evaluated in the subsequent investigation.
Introduction
Various biotic and abiotic factors affect the production of crops worldwide. Salinity is one of the most important abiotic factors that significantly limits agronomic field use and declines global crop production (Rahman et al., 2016; Mbarki et al., 2018; El Sabagh et al., 2021a). Soil salinization is increasing due to mismanaged irrigation practices and sea-level rise. Thus, salinity is becoming a significant threat to sustainable and resilient agriculture (Tester and Langridge, 2010; Zörb et al., 2019) because we have to grow 70% more food to feed the 9.3 billion population by 2050 (Shabala, 2013).
The toxic effect of salt impairs plant growth processes by creating physiological drought as excessive accumulations of ions reduce the soil water potential and essential mineral availability. Reduced water and nutrient uptake leads to osmotic stress, ion toxicity, and mineral imbalance in plant cells (Munns and Tester, 2008; Hanin et al., 2016; Rehman et al., 2019; Singhal et al., 2021). Salinity stress disrupts redox homeostasis and causes oxidative damage to cellular biomolecules by excessive production of reactive oxygen species (ROS) in plants (Hernández et al., 2001; Isayenkov, 2012; Monsur et al., 2020; Latef et al., 2021). Plants employ several physiological and biochemical defense mechanisms to alleviate salt-induced injury through mineral homeostasis, salt ion compartmentalization, compatible solute accumulation, antioxidant system upregulation, and phytohormonal regulation (Gupta and Huang, 2014; Fahad et al., 2015; Acosta-Motos et al., 2017; Hernández, 2019; Van Zelm et al., 2020; Ahmed et al., 2022; Hasanuzzaman, 2022). Chemical priming is considered an alternative strategy for improving abiotic stress tolerance in plants (Anwar et al., 2021). Exogenous application of signaling molecules can augment defensive responses and minimize salt-induced damage in plants (Roy et al., 2016; Tahjib-Ul-Arif et al., 2018; Tahjib-Ul-Arif et al., 2019; Dawood et al., 2021; Latef et al., 2021). In the last decade, exogenous nitric oxide (NO) has been extensively used to mitigate the adverse effects of salinity stress in different crops, and most researchers have found positive effects (Fan et al., 2013a; Ahmad et al., 2016; Siddiqui et al., 2017; Shams et al., 2019; Akram et al., 2020; Alnusairi et al., 2021; El Sabagh et al., 2021b).
Nitric oxide is a signaling molecule that positively influences plant growth and development and modulates abiotic stress tolerance in plants (Asgher et al., 2017). Accumulating evidence suggests that exogenous application of NO confers salinity tolerance in plants (Li et al., 2016; Klein et al., 2018; Sharma et al., 2020; Goyal et al., 2021). NO employs a variety of defense mechanisms to protect plants from salinity stress. In particular, it reinforces ion homeostasis and vacuolar compartmentalization, compatible solute accumulation, reactive oxygen species (ROS) metabolism, photosynthesis activity, and the antioxidant defense system in plants (Liu et al., 2013a; Manai et al., 2014; Kaya et al., 2015; Sohag et al., 2020; Alnusairi et al., 2021). Even though many research studies have been undertaken to determine the effect of exogenous NO on salt stress mitigation, it is difficult to provide an overall prescription for farmers based on these studies. Because the administration of correct doses, frequency, timing, and mode of application and overall positive benefits are so divergent, it is difficult to evaluate. Exogenous NO was applied under different growth conditions, such as greenhouses (Shi et al., 2007; Liu et al., 2013a), controlled growth chambers (Dong et al., 2015a; Tian et al., 2015; Adamu et al., 2018), and fields (Habib et al., 2016; Ali et al., 2017), in various methods, such as seed priming (Zheng et al., 2009; Hayat et al., 2012b), foliar pre-treatment (Tian et al., 2015; Adamu et al., 2018), and post-treatments (Liu et al., 2013a; Shen et al., 2018), and root medium (Fan et al., 2013a; Dong et al., 2015a), and at different frequencies, such as regular intervals (Fan et al., 2013a; Dong et al., 2015a), one time (Khan et al., 2012; Ali et al., 2017; Adamu et al., 2018), and continuous (Wu et al., 2011a; Tian et al., 2015) to mitigate salt stress. Although many studies on exogenous NO application have been published, it is unclear what the optimum NO concentrations are and how long or up to what salinity concentration could be alleviated using NO. Moreover, from the published research articles, it is impossible to understand how the plant clades, life forms, and growth stages are involved in NO-mediated salinity tolerance. As a result, a meta-analysis could be a viable alternative in determining the most effective method, concentration, and application duration and identifying potential research needs in this sector.
Meta-analysis is a systematic synthesis process that generates valuable summaries and uncovers new patterns or expands agreement among the findings of several investigations (Hedges et al., 1999; Lehmann and Rillig, 2015). Although meta-analysis has been widely used in medical science to synthesize information for making clinical decisions and policies, it continues to be used in several other disciplines, e.g., plant ecology and evolutionary biology, and has become more prevalent in recent years (Lau et al., 2013; Koricheva and Gurevitch, 2014; Gerstner et al., 2017; Gurevitch et al., 2018). A meta-analysis is more than just a systematic review; it also weighs the impact of an experimental treatment compared to a control group. The inference drawn from a meta‐analysis could be used in developing agricultural management practices that would otherwise be impossible from the individual, typically short-term research projects, most of which are limited to particular climatic conditions (Eagle et al., 2017). We did not come across any reports that used meta-analysis to focus on the effects of exogenous application of NO on salt tolerance. Many reports show that a particular NO level reduces salt stress in some crops. Still, a generalized recommendation for field-level applications cannot be given unless the combined outcome and underlying factors are understood. To this end, we gathered data from 62 relevant research studies found through our literature search. We assessed the effect of NO on diverse agricultural plants in response to morphological, physiological, and biochemical alterations caused by salinity stress. The current study aimed to answer the following key questions:
i. What is the overall strength or magnitude of the effect of NO application in mitigating plant salt stress across various contexts?
ii. How much, or up to what level, can NO alleviate salinity stress, and what concentration of NO is effective for that salinity level?
iii. What are the roles of various factors (plant factors, NO factors, and environmental factors) in No mediated plant salinity tolerance?
iv. How does exogenous NO influence plant physiological and biochemical parameters?
Materials and methods
Literature search and selection criteria
The data for this meta-analysis was gathered according to the general guidelines by Field and Gillett (2010). We used the Web of Science, Scopus, and Google Scholar databases to conduct an extensive literature search until June 2020. Our keywords were “nitric oxide AND salt stress/or salinity” and “NO AND salinity/or salt stress.” Based on the titles and abstracts of all search results, 260 articles were deemed to contain relevant information (Figure 1). For data collection, the articles were selected using the following set of criteria: (i) the experiment had to manipulate at least one concentration of exogenous NO, (ii) the exogenous NO application was used singly, and we avoided mixed or combined exogenous treatments in this analysis, (iii) both NO-treated and non-NO-treated plants were grown under saline and non-saline conditions, (iv) any selected parameter was investigated, and (v) the findings reported sample size, means, standard deviations/errors, or other relevant statistical information such that the outcome could be converted to a standardized measure of effect size.
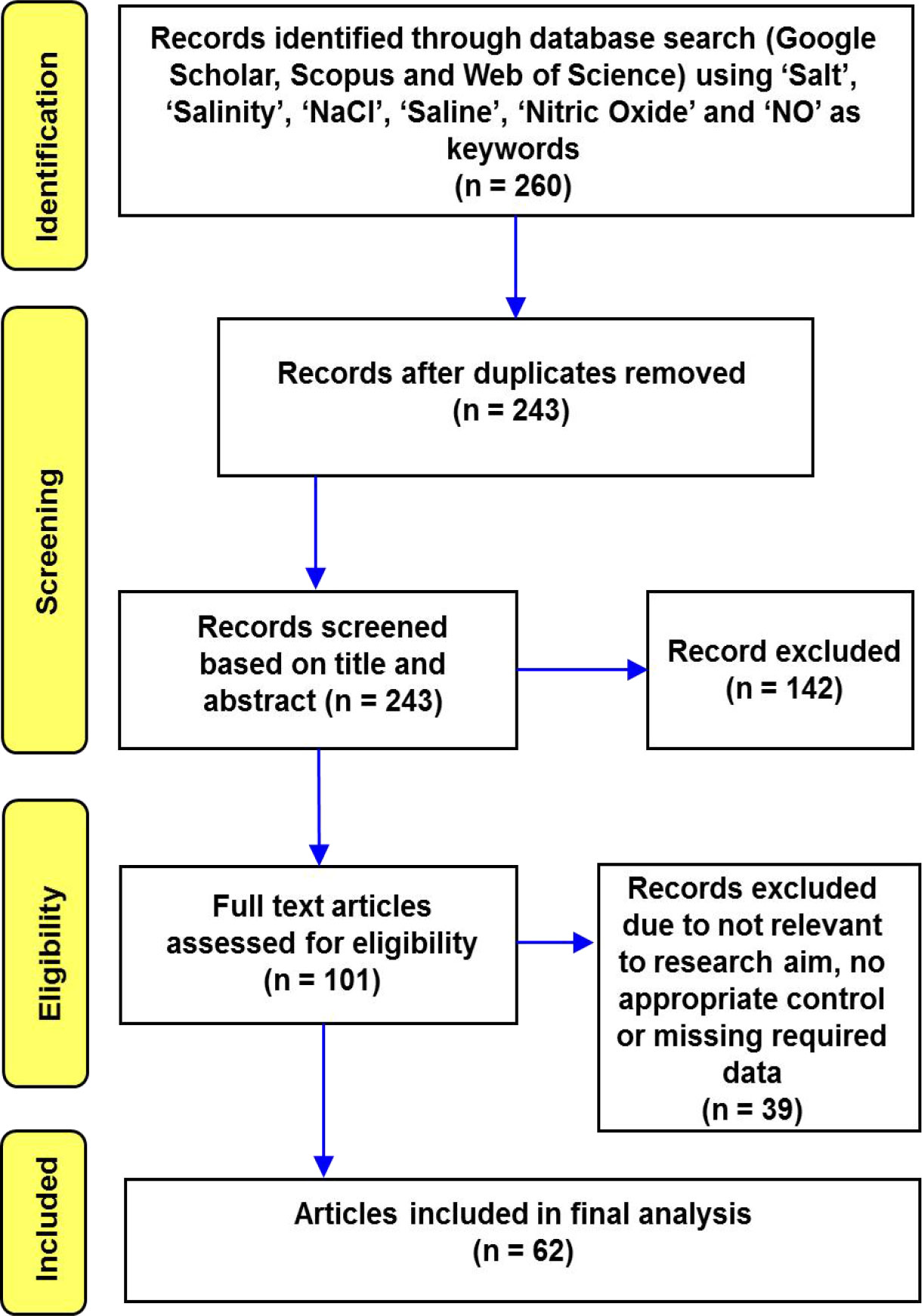
Figure 1 Flow chart depicting the Preferred Reporting Items for Systematic Reviews and Meta-Analysis (PRISMA) search strategy used to find and choose published literature for this analysis.
Based on these criteria, we finally selected 62 studies out of 260 for analysis. The level of fertilizer applied, the growing settings (greenhouse, growth chamber, or field), the duration of time before stress was exposed, and the growth media used in our meta-analysis were all allowed to vary. The papers covered 20 years (2000–2020) and were written in English. The detailed paper selection procedure has been provided in Figure 1 according to PRISMA guidelines.
Data extraction
We retrieved data on dry biomass of plants, NO application, photosynthetic and enzyme parameters, and other relevant data from the studied articles. Mean values, sample sizes (replications), and standard deviations (SDs) were recorded from each investigation. We converted the presented standard errors (SEs) to standard deviations with the equation SD = SE × √ (sample size). The reported 95% CIs (confidence intervals) were translated to SDs where applicable. We employed WebPlotDigitizer V4.2 (https://automeris.io/WebPlotDigitizer/) to digitize the values of figures. Multiple treatments or combinations of plant species/cultivars from the same experiment were treated as different studies and included in the analysis as independent data units. By presuming that the studies are independent, extracting multiple studies from a single experiment may increase the reliability of that study (Gurevitch and Hedges, 1999). We estimated the mean effect size of the dataset using only a single random observation from each research paper (reduced dataset) and compared this with the effect size calculated using the entire dataset (full dataset) to investigate potential publishing biases due to non-independence from numerous observations (He and Dijkstra, 2014). We used Welch’s t-test to assess effect sizes (full dataset vs. reduced dataset) to see if data reduction might significantly impact effect size. We found no significant discrepancies because of data reduction, indicating that overrepresentation was unlikely in this study. Considering multiple observations from the same investigation to be independent is expected to improve meta-analysis statistical power (Lajeunesse and Forbes, 2003). This method has been employed in many biological meta-studies (Veresoglou et al., 2012; Mayerhofer et al., 2013; Mcgrath and Lobell, 2013; Eziz et al., 2017; Dastogeer, 2018; Dastogeer et al., 2020).
Statistical procedure for meta-analysis
The meta-analyses were conducted in R version 4.2.1 using the “meta” (Balduzzi et al., 2019). The standardized mean difference (SMD) was measured using Hedge’s g statistic to determine the effect size for the difference between means using the “metacont” function. Hedge’s g is a meta-analysis statistic that reflects the difference in means in units of the pooled standard deviation and is favored over other measures like the log-response ratio because it has a lower Type I error rate (Lajeunesse and Forbes, 2003; Van Kleunen et al., 2010; Xie et al., 2018). In the case of our study, the SMD is recommended for meta-analyses, including studies reporting continuous outcomes (Faraone, 2008). A SMDs = 0 indicates that the two treatments (NO-treated or non-treated) have a similar effect, whereas a SMDs >0 reflects how the NO-exposed samples surpassed the non-exposed samples and vice versa. In particular, SMD values of 0.3, 0.5, and 0.8 imply low, moderate, and high impact sizes, respectively (Cohen, 1988). The overall effect was calculated using a random-effects model. A random-effects model was used because diverse types of experiments were included in the model. Due to the diverse locations, conditions, experimental settings, and methodologies utilized in the individual investigations, it is unlikely that all of them would predict a similar effect size (Borenstein et al., 2009). We computed 95% confidence intervals (CIs) and interpreted them in such a way that when the 95% CIs exclude zero, the effect size (SMD) is assumed to be significant. The Sidik–Jonkman estimator (Sidik and Jonkman, 2005) was used with the Hartung–Knapp adjustment (HKSJ) to estimate the random effects variance. When the combined studies are of varying sizes and demonstrate between-study heterogeneity, HKSJ creates inflated error rates, but it outperforms the widely used DerSimonian and Laird technique approach (Sidik and Jonkman, 2007; IntHout et al., 2014). Higgin’s I2 and Cochran’s Q statistics were employed to measure and test for statistical heterogeneity. I2 is the ratio of actual heterogeneity to overall heterogeneity across reported effect sizes, whereas Q is the weighted deviation from the summary effect size attributable to heterogeneity other than due to the sampling error (Higgins and Thompson, 2002; Higgins et al., 2003; Huedo-Medina et al., 2006). In general, I2 values vary from 0% to 100%, and the values of <25, 25–75, and >75% indicate small, medium, and high heterogeneity, respectively (Higgins et al., 2003).
Finding publication biases and adjustment
We used several methods to test the publication bias for each dataset. We visually examined asymmetry in funnel plots, employed “trim-and-fill” analysis, and ran Begg and Mazumdar rank correlation tests based on Kendall’s tau, the Egger regression test, and p-curve analysis (Begg and Mazumdar, 1994; Egger et al., 1997; Simonsohn et al., 2014). If these tests revealed significant bias, we used the trim-and-fill procedure to correct the biases and calculate the effect sizes (SMD), CIs, and heterogeneity statistics (Schmidt and Hunter, 2015) (Duval and Tweedie, 2000). We constructed subgroups from the studies based on the moderator subgroups. We applied trim-and-fill to the subgroups if any subgroups had biases identified by the above tests (Schmidt and Hunter, 2015).
Selected subgroup analyses
We performed subgroup analyses on the data to investigate the influence of factors, such as plant identity, NO treatment settings, plant life cycle, and salinity duration on the shoot and root dry biomass parameters. Using the “dmetar” package in R, we conducted a mixed-effects model with the subgroups as the fixed-effects element (Harrer et al., 2019). The overall impact size for each subgroup was determined using a random-effects model, and then we used a fixed-effects model to test between-subgroup differences (Borenstein and Higgins, 2013).
This approach is appropriate when the subgroup levels under examination are expected to be exhaustive for the characteristics and are not picked at random. Because most of the subgroups in our analysis were fixed, such as plant life cycle (annual and perennial) and plant clade (monocot or dicot), we hypothesized that a mixed-effects model would be a good fit. A factor needs to be reported in at least five studies across two separate papers to be included in the analysis as a subgroup variable. Initially, we considered different salinity levels (low, moderate, and high) but found no significant effects of salinity levels on the shoot and root biomass production (Table S2). Thus, in this meta-analysis, we consider only saline and non-saline conditions.
Growth condition: Plant growth conditions were categorized into three groups: greenhouse, growth chamber, and field.
Method of NO application: the methods of NO were divided into four categories: seed priming, root medium, foliar pre-treatment, and foliar post-treatment.
Duration of NO application: The period of NO supplementation was divided into three categories: regular interval, one time, and continuous.
Duration of salinity: The period of salinity treatment was divided into three categories: short (<8 days), moderate (8–21 days), and long (>21 days).
Plant growth stages were divided into two groups: seedling and germination
Plant clades were classified into two groups: monocots and dicots
Plant life forms were classified into three classes: vines, graminoids, and forbes
Results
In this study, data were collected from 2000–2020, and after 2005, more papers were published on exogenous NO-mediated salinity stress mitigation (Figure 2A). We investigated the effects of exogenous application of NO on 32 plant response parameters at various levels of salt stress. In this study, the summary effect sizes for non-stressed plants were also examined for comparison. Plants were represented by 30 species from 16 families across the 62 articles (Figures 2B, C). Triticum aestivum (6), Zea mays (5), Oryza sativa (4), Helianthus annuus (4), Gossypium hirsutum (4), Glycine max (4), and Cucumis sativus (4) were the most commonly studied plant species (Figure 2C).
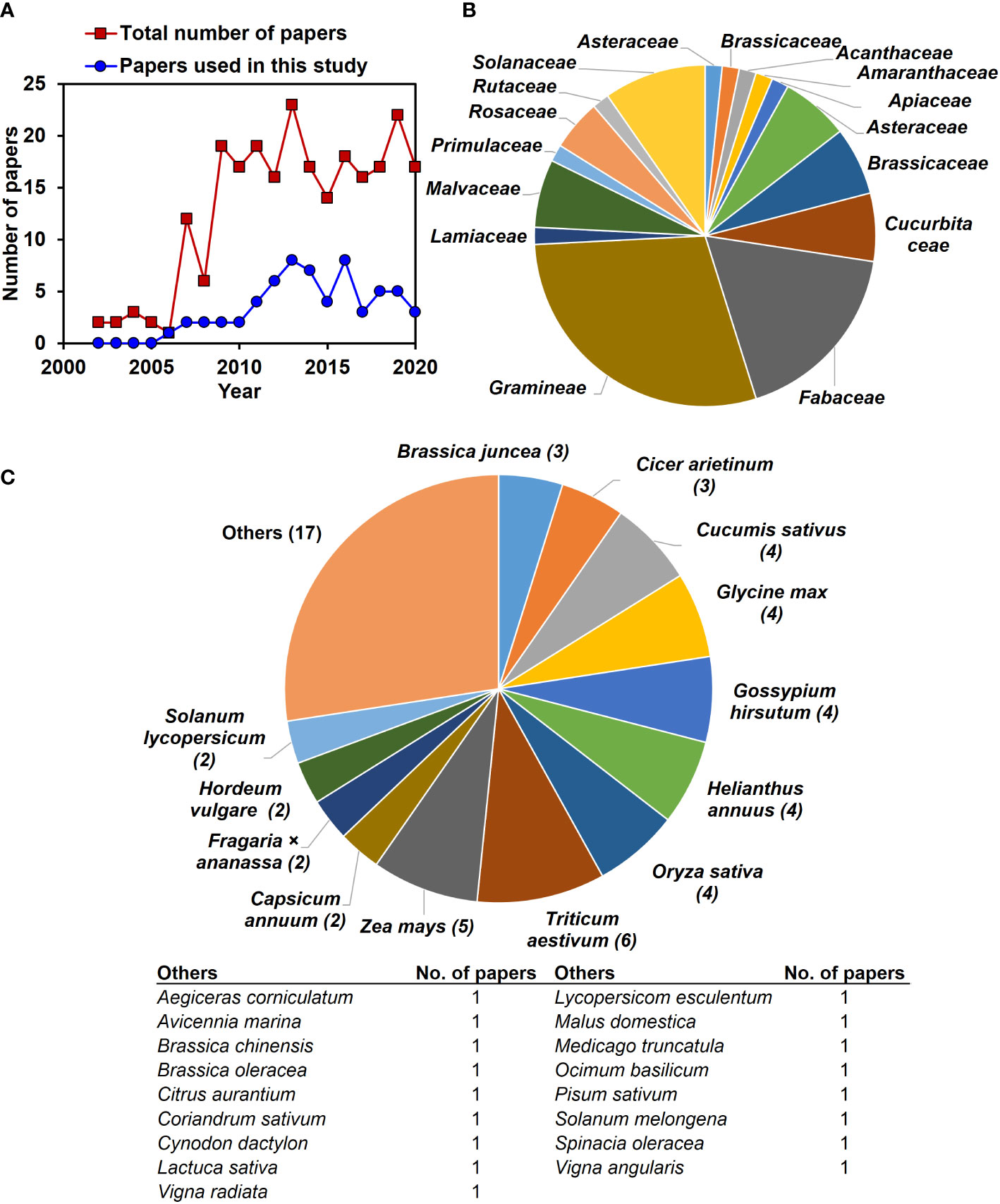
Figure 2 The total number of published articles, plant families and species about exogenous NO effects on plant stress physiology for salt tolerance available in the ‘Web of Science’ and ‘Scopus’ databases between 2000 and 2020, (A) the inlets in the main plots display the total number of articles found in the search, while the main plots exhibit the number of publications by year. (B) The pie chart represents the sixteen diverse families of the studied plants. (C) The pie chart shows the 30 diverse plant species from 16 families across the used articles in this study.
Effects of exogenous NO on plant growth parameters and yield under salinity
Exogenous NO application significantly increased SDW, RDW, and SL both under saline (p <0.001) and non-saline (p <0.001) conditions (Figure 3). The subgroup analysis indicated that there is a significant difference in the effect size of SDW between saline and non-saline conditions. However, the effect sizes of RDW and SL between saline and non-saline conditions were statistically similar because of the overlapping confidence interval values (Figure 3). Moreover, exogenous application of NO significantly increased RL (p = 0.036) and yield (p <0.001) under saline conditions, although no significant effects were observed under non-saline conditions (Figure 3). There are also significant differences in the effect sizes of RL (p = 0.045) and yield (p = 0.029) between salt-stressed and non-stressed conditions (Figure 3; Supplementary Table 3).
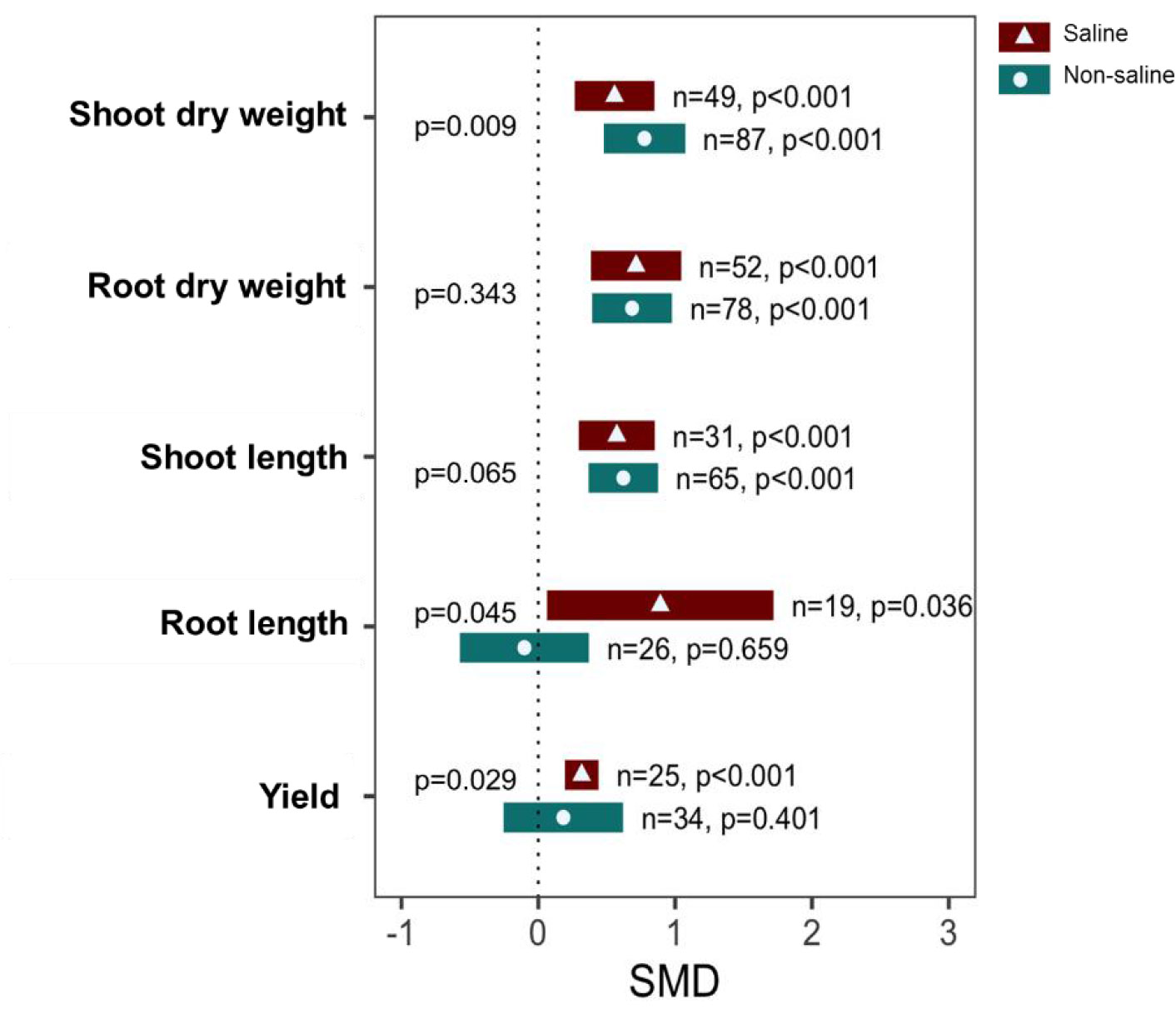
Figure 3 Growth responses and yield of exogenous NO-treated plants compared with those of non-NO-treated plants under non-saline and saline conditions. Error bars are effect size (SMD) means ±95% CIs. Where the CIs do not overlap the vertical dashed lines, the effect size for a parameter is significant, i.e., the growth responses of NO-treated plants were significantly different from those of non-NO-treated plants. n, the number of studies included in the meta-analysis; p, the significance level of SMD.
Categorical analysis of the effects of exogenous NO on SDW
Categorical variables considered in the analysis showed that multiple factors influence the impact of exogenous NO on SDW, e.g., plant growth condition, method, time, and duration of NO application, plant factors, and salinity stress factors. For example, experiments that were performed under growth chamber (p = 0.002) and field conditions (p <0.001) showed significant effects on SDW under saline conditions (Figure 4A). Among the methods of exogenous NO application, “seed priming” (p <0.001) and “foliar application” (p <0.001) before exposure to salinity had a significant effect on the SDW production under salinity stress. On the other hand, “root medium” and “foliar post-treatment” showed a non-significant effect on SDW production both under saline and non-saline conditions (Figure 4B). The effect sizes (SMDs) of “root medium” with NO application were based on only a few studies (n = 8, saline and n = 7, non-saline), and the CIs interval is very long. Moreover, the CIs interval in the case of “foliar post-treatment” NO application is very long (Figure 4B). Thus, further studies should be performed focusing on “root medium” and “foliar post-treatment” with NO application. Applications of NO for “one time” (p <0.001) and “regular interval” (p = 0.006) significantly improved the SDW under saline conditions, but “continuous” application was not effective both under saline and non-saline conditions (Figure 4C). However, the CIs interval for ‘continuous’ NO treatment was very long, which suggests variable results among different studies (Figure 4C). Exogenous NO application most effectively improved SDW under ‘long (>21 days)’ duration salinity but did not improve under ‘short (<8 days)’ and ‘moderate (8-21 days)’ duration salinity stress (Figure 4D). Surprisingly, the effect of NO on SDW became stronger as the duration of salt treatment increased (Figure 4D). The application of NO significantly improved SDW production under saline conditions at both the seedling (p = 0.010) and germination (p <0.001) stages, where NO-mediated SDW improvement was more pronounced at the germination stage (Figure 4E). In both dicot and monocot plants, application of NO increased SDW more than non-NO treatment under salinity and non-salinity conditions (Figure 4F). As is evident in the figure (Figure 4G), application of NO on forbes and graminoid plants tended to increase SDW production under both saline and non-saline conditions.
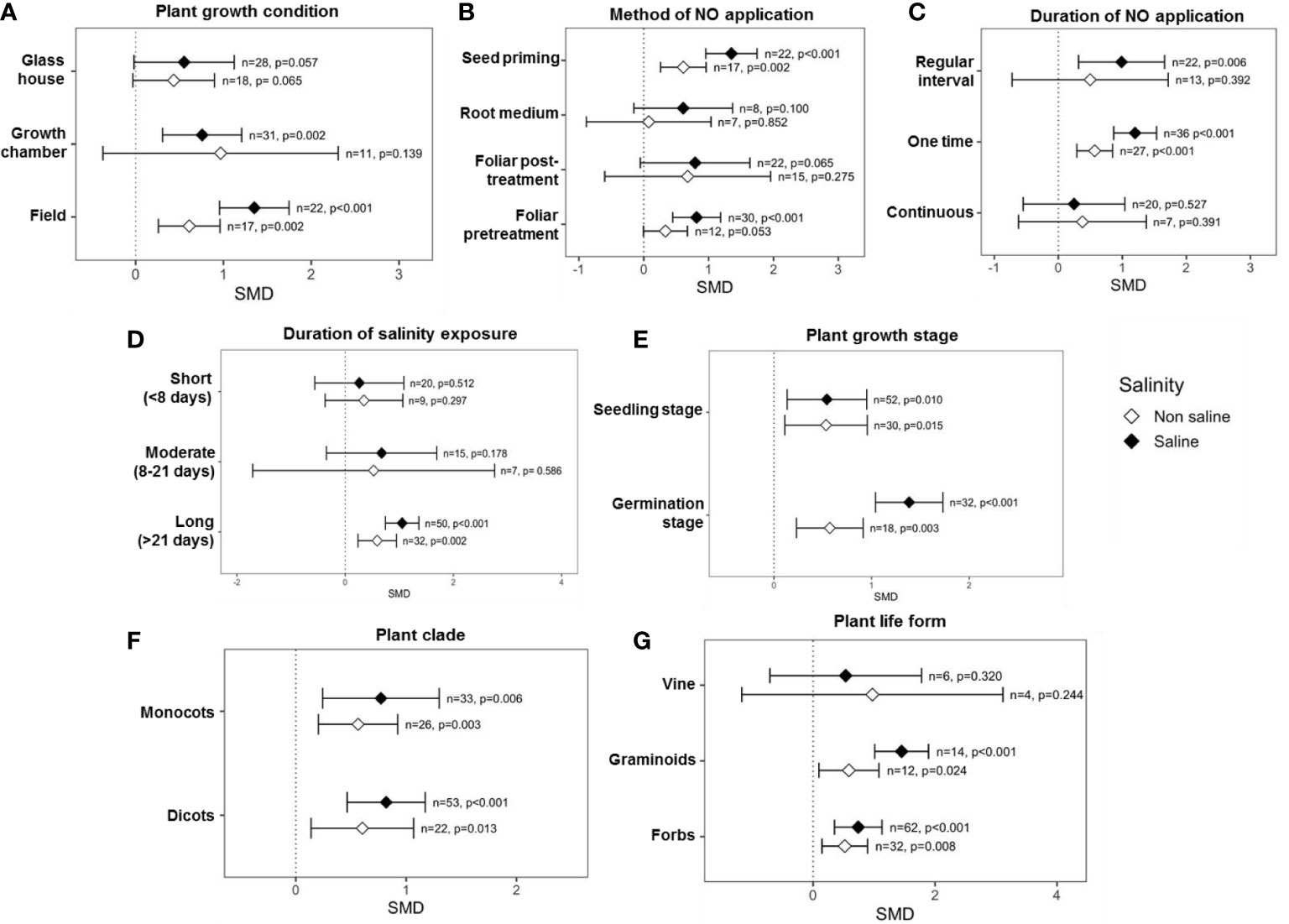
Figure 4 Effects of exogenous NO on SDW under saline and non-saline conditions for various categorical variables such as (A) growth conditions, (B) method of NO application, (C) duration of NO application, (D) duration of salinity, (E) plant growth stages, (F) plant clade, and (G) plant life form. The error bars are the effect size means ±95% CIs. Where the CIs do not overlap the vertical dashed lines, the effect size for a parameter is significant, i.e., the growth responses of NO-treated plants were significantly different from those of non-NO-treated plants. n, the number of studies included in the meta-analysis; p, the significance level of SMD.
Categorical analysis of the effects of exogenous NO on RDW
According to categorical variables evaluated in the analysis, the effect of exogenous NO on RDW is influenced by numerous parameters, including plant growth settings, method, time, and duration of NO administration, plant factors, and salt stress factors. For example, experiments that were undertaken at field conditions (p = 0.002) displayed significant impacts on RDW under saline conditions (Figure 5A). Among the methods of exogenous NO application, “seed priming” (p = 0.001) and “foliar post-treatment” (p = 0.003) had a significant effect on the RDW production under the absence and presence of salinity. On the contrary, “root medium” and “foliar application” exhibited no significant effect on RDW production in the case of both salinity and non-salinity conditions (Figure 5B). The effect sizes (SMDs) of the “root medium” NO application were based on only a few studies (n = 5, saline and n = 5, non-saline), and the CIs interval is very long under saline conditions (Figure 5B).
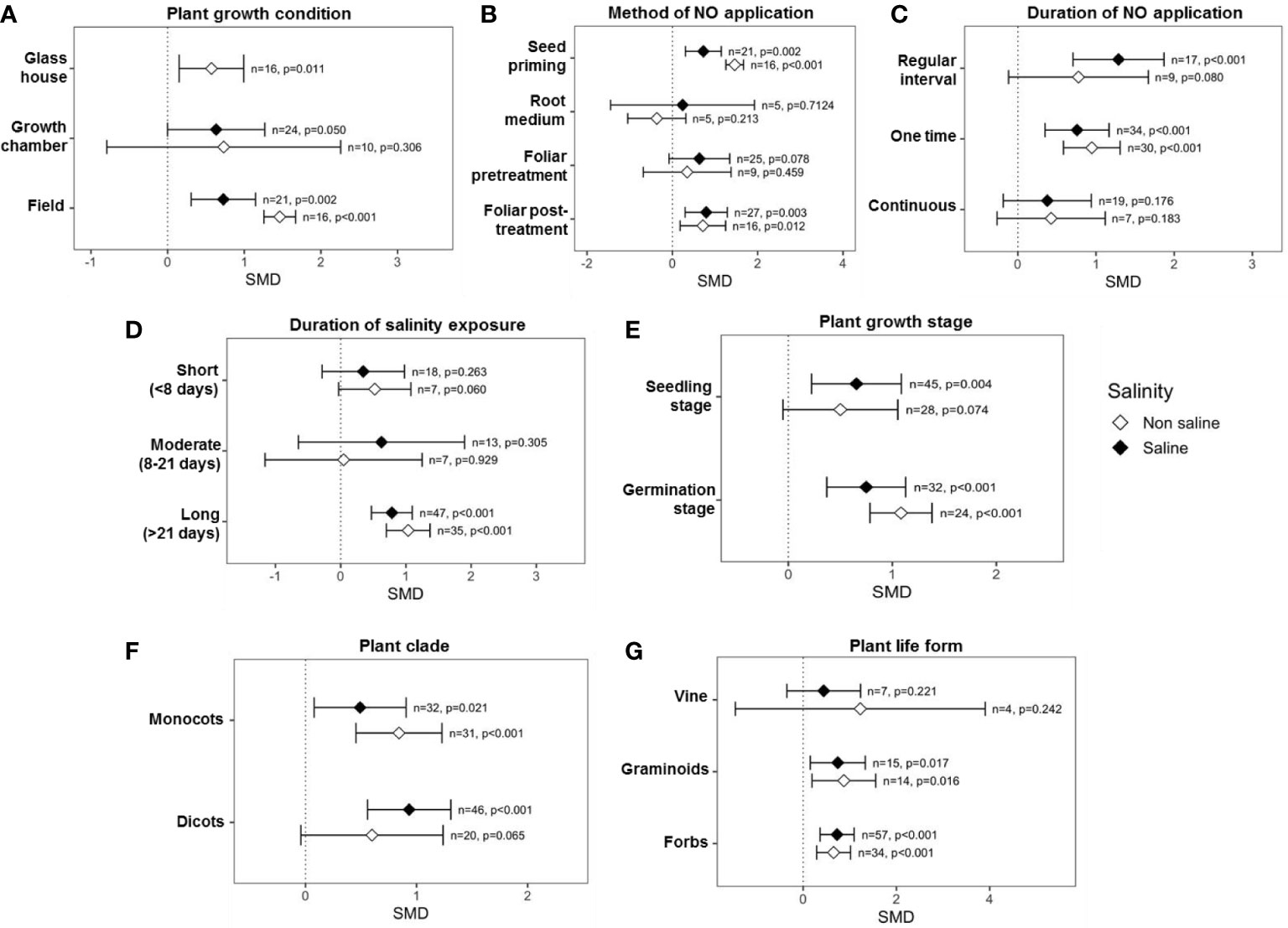
Figure 5 Effects of exogenous NO on RDW under saline and non-saline conditions for various categorical variables such as (A) growth conditions, (B) method of NO application, (C) duration of NO application, (D) duration of salinity, (E) plant growth stages, (F) plant clade, and (G) plant life form. The error bars are the effect size means ± 95% CIs. Where the CIs do not overlap the vertical dashed lines, the effect size for a parameter is significant, i.e., the growth responses of NO-treated plants were significantly different from those of non-NO-treated plants. n, the number of studies included in the meta-analysis; p, the significance level of SMD.
NO application for “one time” (p <0.001) and “regular interval” (p <0.001) considerably enhanced RDW in a saline environment, while “continuous” application was ineffective in both saline and non-saline conditions (Figure 5C). However, the CIs for “continuous” NO treatment were extremely wide, implying that findings differed greatly between investigations (Figure 5C). Exogenous NO treatment enhanced RDW the most under “long (>21 days)” (p <0.001) duration salinity, while no significant improvement was found under “short (0–8 days)” and “moderate (9–21 days)” duration salt stress (Figure 5D). Interestingly, as the duration of salt treatment increased, the effect of NO on RDW grew stronger (Figure 5D). Plant growth stage had no effect on RDW generation; plants acquired considerably greater shoot biomass in response to exogenous NO treatment than non-NO treatment at both seedling (p <0.001) and germination stages (p <0.001) (Figure 5E). Under saline and non-saline circumstances, the application of NO raised SDW in both dicot and monocot plants more than non-NO treatment (Figure 5F). As is evident in the figure (Figure 5G), NO treatment on forb and graminoid plants is supposed to enhance RDW generation under salinity and non-salinity conditions.
Effects of exogenous NO on plant photosynthetic attributes
Most of the photosynthetic parameters, such as chlorophyll a (Chla), chlorophyll b (Chlb), total Chl, rate of photosynthesis (Pn), stomatal conductance (Gs), transpiration (E), and subcellular CO2 concentration (Ci), were significantly influenced by exogenous NO application under saline and non-saline conditions (Figure 6). The subgroup analyses revealed that the favorable effects of exogenous NO treatment on particular plant photosynthetic indices, including Chla, Chlb, Pn, Gs, E, and Ci, were higher when plants were exposed to salt stress than in non-stressed plants (Figure 6). For example, the Chla content was significantly influenced by exogenous NO application under saline (p <0.001) and non-saline (p = 0.03) conditions (Figure 6). Exogenous NO administration significantly impacted Chlb content in saline (p <0.001) but not in non-saline (p = 0.164) conditions (Figure 6). There is no effect of exogenous NO application on leaf area (LA) both under stressed and non-stressed conditions (Figure 6). The Pn, Gs, E, and Ci were significantly improved by exogenous NO application under saline conditions (p <0.001, Pn, Gs; p = 0.017, E; p = 0.004, Ci) but not under non-saline conditions (Figure 6). However, all of the measures studied tended to be more variable under non-stress conditions compared to saline-stress environments, as evidenced by their higher confidence interval values (Figure 6).
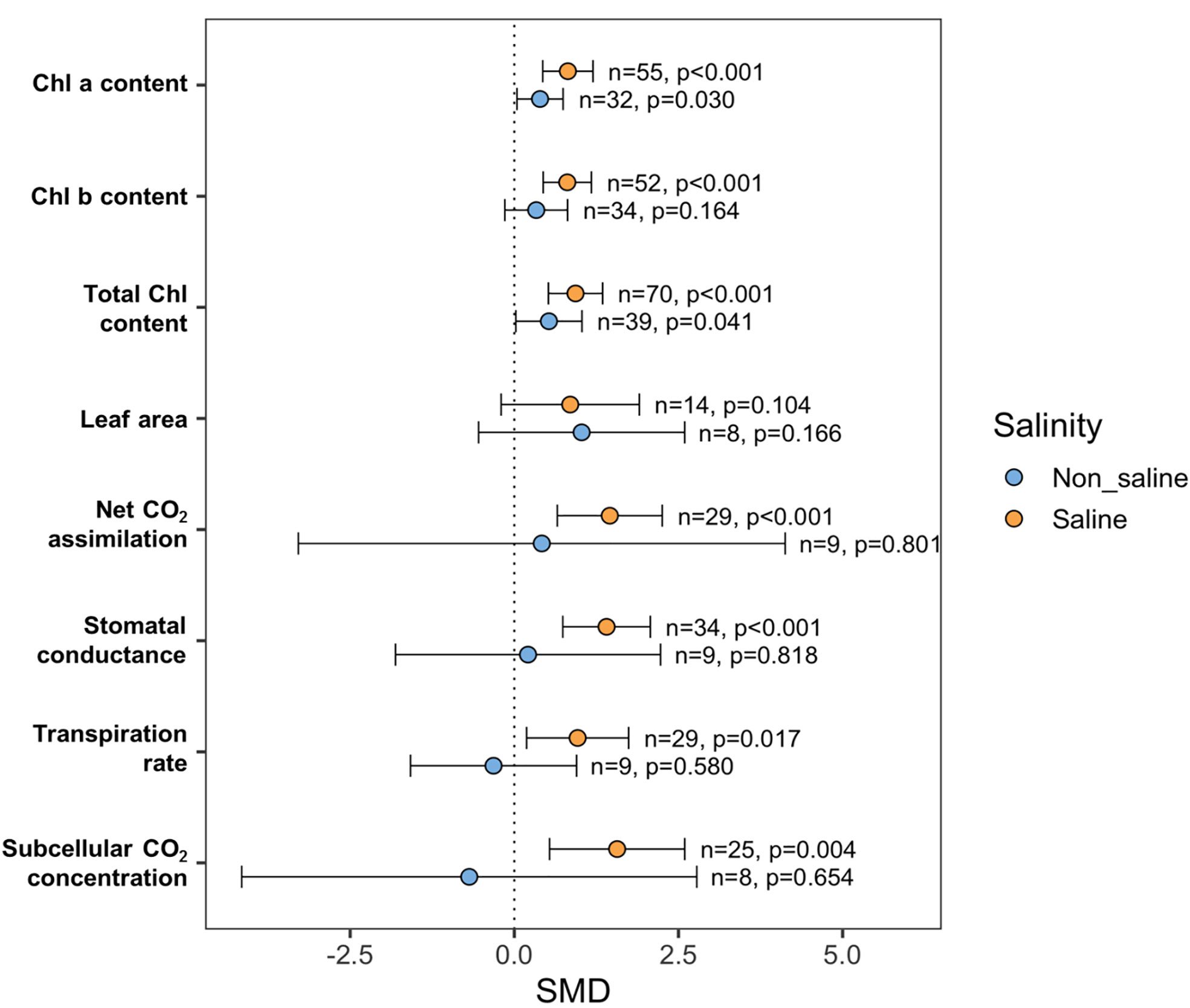
Figure 6 Effects of exogenous NO on photosynthesis-related parameters such as chlorophyll-a (Chla), chlorophyll b (Chlb), total chlorophyll (total Chl) content, leaf area (LA), net CO2 assimilation (Pn), stomatal conductance (Gs), transpiration (E) and sub-cellular CO2 concentration (Ci) under saline and non-saline conditions. The error bars are the effect size means ±95% CIs. Where the CIs do not overlap the vertical dashed lines, the effect size for a parameter is significant, i.e., the growth responses of NO-treated plants were significantly different from those of non-NO-treated plants. n, the number of studies included in the meta-analysis; p, the significance level of SMD.
Effects of exogenous NO on plant water relations and nutrient homeostasis
Exogenous NO application did not increase SS and SP content significantly both under stressed (p = 0.057, SS; p = 0.093, SP) and non-stressed (p = 0.158, SS; p = 0.087, SP) conditions (Figure 7). However, exogenous application of NO improved Pro content significantly both under saline (p = 0.004) and non-saline (p = 0.004) conditions (Figure 7). Electrolyte leakage (EL) decreased in response to exogenous NO application under salt stress conditions (p = 0.135), but this change is non-significant because of the higher CI interval (Figure 7).
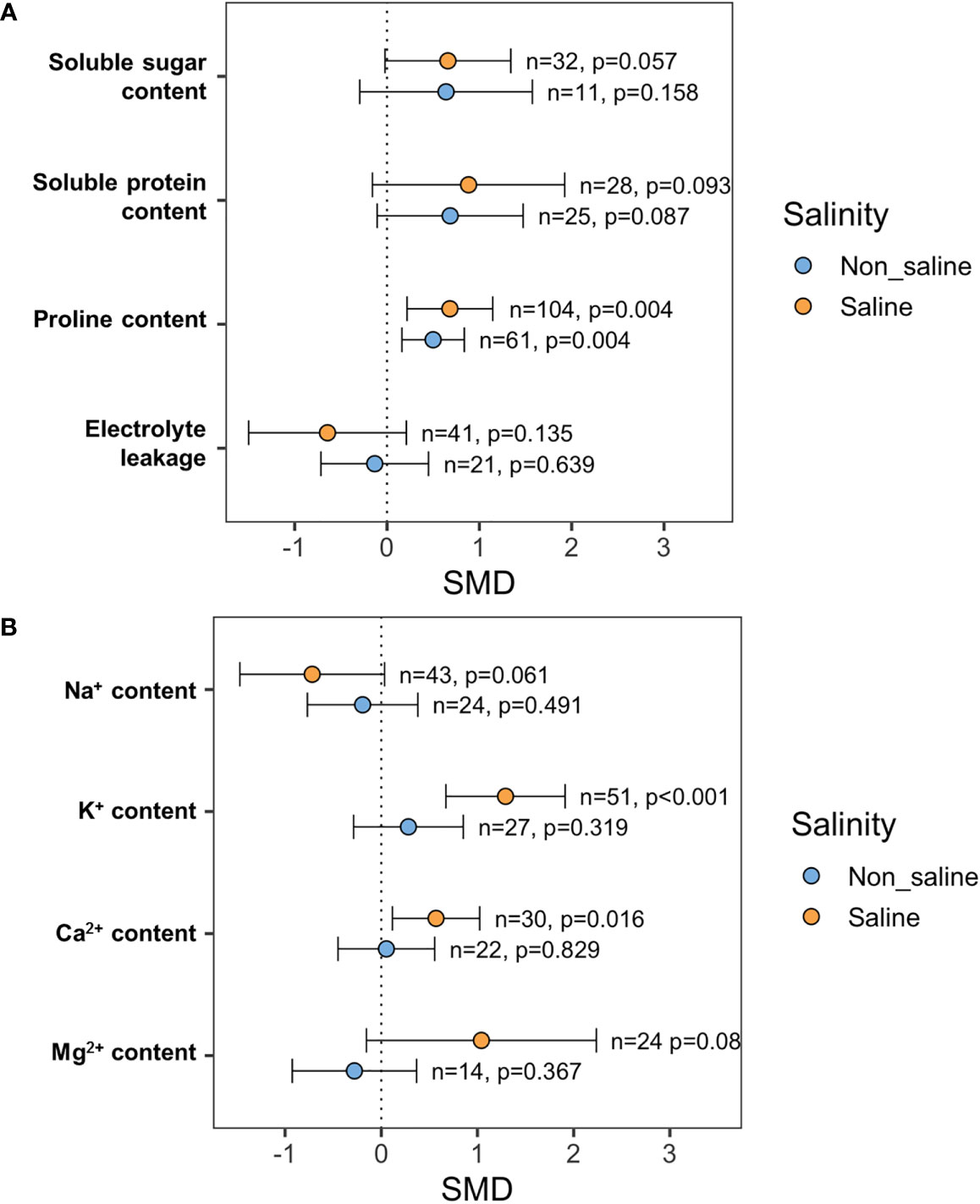
Figure 7 Effects of exogenous NO on (A) soluble sugars (SS), soluble proteins (SP), proline content, and electrolyte leakage (EL) and (B) Na+, K+, Ca2+, and Mg2+ contents under saline and non-saline conditions. The error bars are the effect size means ±95% CIs. Where the CIs do not overlap the vertical dashed lines, the effect size for a parameter is significant, i.e., the growth responses of NO-treated plants were significantly different from those of non-NO-treated plants. n, the number of studies included in the meta-analysis; p, the significance level of SMD.
The leaf K+ content (p <0.001) and Ca2+ content (p = 0.016) in NO-treated plants were consistently higher than those in non-NO-treated plants under salinity conditions (Figure 7). Under saline conditions, leaf Na+ content was dropped in response to exogenous NO delivery, but this shift was non-significant (p = 0.061) due to higher CI intervals (Figure 7). Moreover, leaf Mg2+ content in the salt-stressed plants was increased in reaction to the administration of exogenous NO. However, this change is non-significant (p = 0.08) because of greater CI intervals (Figure 7).
Effects of exogenous NO on plant oxidative damage and antioxidant systems
In non-stressed conditions, there was no change in H2O2 and MDA buildup between NO-treated and non-NO-treated plants (Figure 8). However, exogenous NO-treated plants had significantly lower MDA levels (p <0.001) and H2O2 content (p <0.001) than non-NO-treated plants under saline conditions (Figure 8). The effect of exogenous NO on superoxide content reduction was not substantial both under saline (p = 0.114) and non-saline (p = 0.560) conditions, though the NO treatment reduced the superoxide content under saline conditions (Figure 8). Both under saline and non-saline conditions, NO-treatment increased SOD (p = 0.004 and p <0.001, respectively), CAT (p <0.001 and p <0.001, respectively), POX (p = 0.001 and p = 0.001, respectively), APX (p = 0.002 and p = 0.031, respectively), GR (p = 0.002 and p = 0.012, respectively), and DHAR (p = 0.034 and p = 0.003, respectively) activity than non-NO-treatment (Figure 8). However, in both saline and non-saline environments, there was no difference in GPX activity between NO-treated and non-NO-treated plants (Figure 8). Among the non-enzymatic antioxidants, GSH content was significantly increased only under saline (p = 0.012) conditions, but ASC content was increased both under saline and non-saline conditions in response foliar NO application than non-NO application (Figure 8). The endogenous NO content did not change due to the application of exogenous NO in comparison to non-NO treatment both under saline and non-saline conditions (Figure 8).
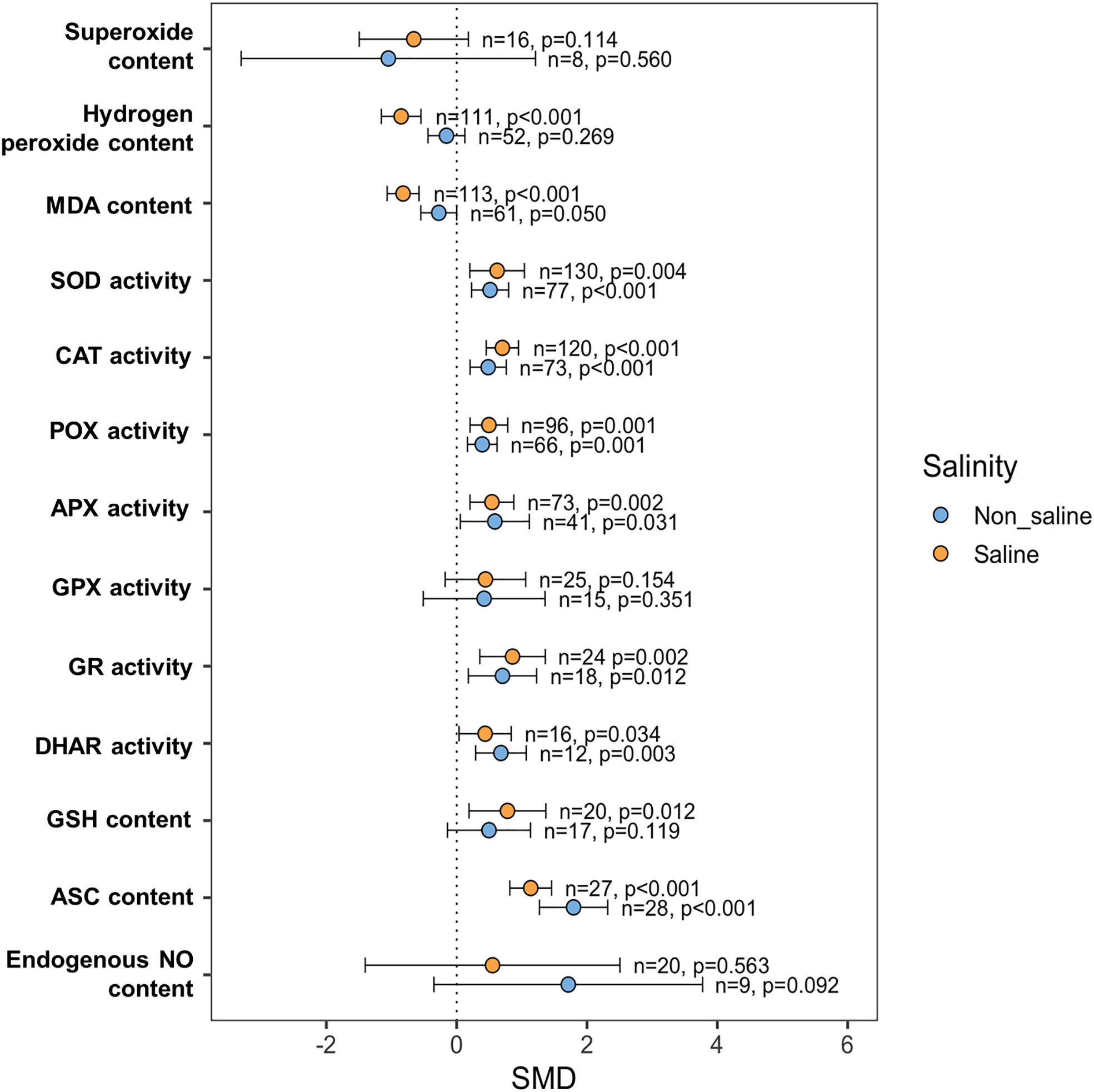
Figure 8 Exogenous NO effects on ROS scavenging and antioxidant capacity such as superoxide, hydrogen peroxide (H2O2), malondialdehyde (MDA), superoxide dismutase (SOD), catalase (CAT), peroxidase (POX), ascorbate peroxidase (APX), glutathione peroxidase (GPX), glutathione reductase (GR), dehydroascorbate reductase (DHAR), glutathione (GSH), ascorbate (ASC) and endogenous NO content in salt-induced plants. The error bars are the effect size means ±95% CIs. Where the CIs do not overlap the vertical dashed lines, the effect size for a parameter is significant, i.e., the growth responses of NO-treated plants were significantly different from those of non-NO-treated plants. n, the number of studies included in the meta-analysis; p, the significance level of SMD.
Discussion
It is urgent to find an economical and effective technique to reduce the damage caused by salinity stress in crops because it causes significant yield loss. Exogenous administration of some signaling molecules has a vast potential for reducing the negative consequences of abiotic stressors. In this regard, exogenous NO has been intensively studied in the last 20 years and can alleviate the harmful effects of salt stress in various plant species. In general, most research studies found that exogenous application of NO enhances salinity stress tolerance in plants, implying that it improves growth or yield under salinity stress compared to the salinity stress condition alone. However, several questions remain: at what concentration, which method, or how long did NO application show the best growth performance or yield? Does exogenous NO enhance the yield of crops? Do plant species or growth stages and conditions affect the NO-mediated effects on plants? Which physiological or biochemical processes are most modulated by exogenous NO application? A meta-analysis was performed to find the answers to those questions.
Does exogenous NO improve plant phenotypic traits and yield during salinity stress?
Salt stress negatively impacts growth parameters (e.g., shoot–root length and root–shoot dry weight) and yield production due to increased toxicity, osmotic effect, and oxidative stress (Yildirim et al., 2009; Shams et al., 2016; Ahmad et al., 2018). The meta-analysis revealed that exogenous NO substantially affects some physiological and biochemical attributes, particularly plant growth and development, photosynthesis, and defense mechanisms to repair oxidative injury under salt stress. Exogenous NO application in different conditions displays divergence from the corresponding diagram. The effects of different salinity regimes on plant growth regulation, such as shoot and root dry weight, shoot–root growth, and comparable output, were observed in several crops, such as wheat (Triticum aestivum) (Perveen et al., 2011; Perveen et al., 2012), rice (Oryza sativa) (Iqbal et al., 2015), sorghum (Sorghum bicolor) (Bashir et al., 2011), and pearl millet (Pennisetum glaucum) (Hussain et al., 2008). According to our findings, NO treatment facilitates growth and yield under salinity-induced circumstances, where NO, as a signaling agent, works as a stress protectant (Parankusam et al., 2017). Several prior studies in maize (Zea mays), rice, wheat, tomato (Lycopersicon esculentum), and marigold (Calendula officinalis) showed an increase in shoot–root length and shoot–root dry weight (Farooq et al., 2009; Molassiotis et al., 2010; Bai et al., 2011; Wu et al., 2011a; Jabbarzadeh et al., 2018; Sehar et al., 2019). Our meta-analysis showed that exogenous NO confers plant salinity tolerance by reestablishing mineral uptake, osmolyte accumulation, and antioxidant enzyme activity, ensuring incredible plant growth and yield (Figures 7, 8).
Salinity induces water scarcity in the root zone and immediately affects plant water status in a short period. On the other hand, the plant recovers over several hours and returns to a modest, steady growth rate. The second phase, which increases over time, is driven by the toxicity of excess Na+ and Cl− ions that concentrate in the cytoplasm. Furthermore, plants under salinity stress require extra energy to counteract the harmful effects of Na+ ions, and they are also vulnerable to nutritional deficiency. These processes harm plant growth and biomass production (Munns and Tester, 2008; Isayenkov and Maathuis, 2019; Tabassum et al., 2021). Our analysis confirmed that exogenous NO supplementation significantly improved the SDW and RDW in both salt-stressed and non-stressed plants (Figure 3). Exogenous NO might improve shoot and root biomass production by enhancing photosynthetic pigment, nutrient uptake, and antioxidant enzymatic activity and mitigating oxidative damage in salt-stressed plants, including rice (Mostofa et al., 2015), maize (Kaya et al., 2015), wheat (Kausar et al., 2013), chickpea (Cicer arietinum) (Ahmad et al., 2016), tomato (Wu et al., 2011a), and pepper (Capsicum annum) (Shams et al., 2019).
How do different sub-categories affect NO-mediated salinity stress tolerance in plants?
This meta-analysis showed that the effects of exogenous NO on plant biomass yield were context-dependent, with various elements playing key roles. For example, exogenous NO significantly increased SDW in field and growth chamber studies, whereas RDW was significantly improved only in field environments under salt stress (Figures 4A, 5A). Plants were typically grown in plots in greenhouses or growth chambers (Egbichi et al., 2014; Jamali et al., 2015; Siddiqui et al., 2017; Khoshbakht et al., 2018; Shams et al., 2019; Jabeen et al., 2021), and many researchers failed to observe changes in root biomass in response to NO under salinity conditions, possibly because optimal root growth is reduced under these growth conditions, as supported by our meta-analysis (Figures 4A, 5A). Thus, studies in the field are required to understand root phenomics in response to NO under salt stress. It is worth mentioning that all the field-level experiments were conducted at the germination stage (Habib et al., 2016; Ali et al., 2017).
This meta-analysis showed that seed priming and foliar pre-treatment were the most effective methods for NO application to plants (Figure 4B). The exogenous NO applications before exposure to salt stress might boost the metabolic activity, energy production, and stress tolerance mechanisms of the plant, improving plant growth responses in response to salinity. On the contrary, root medium showed no noticeable impact on shoot and root biomass accumulation under saline conditions (Figures 4B, 5B). However, this proposition was based on only a few investigations; therefore, further studies should be performed on these NO application methods. NO application as seed priming and foliar post-treatment markedly increased root dry biomass under salt stress (Figure 5B). As plant roots are directly in contact with Na+ ions during salinity stress, foliar post-treatment might activate the NO-based defense machinery at first in roots to trigger a salinity tolerance mechanism. NO triggers root tip elongation and lateral adventitious root development (Correa-Aragunde et al., 2004) and integrates the ABA–IAA signaling network of root system responses in tomatoes under salt stress (Santos et al., 2020). As seed priming with NO showed the maximum effect on root growth improvement (Figure 5B), this method could be used for successful early seedling establishment in salinity soils. Interestingly, foliar pre-treatment and “root medium” showed no considerable effect on root dry mass production under salinity conditions (Figure 5B). However, a few papers on root medium-based studies have been found and examined, and more research into these NO-based approaches is needed.
Both one time and regular interval treatments of exogenous NO were efficient for the significant shoot and root dry biomass production in the presence of salinity (Figures 4C, 5C). These findings are in agreement with the results on maize (Kaya et al., 2015), chickpea (Ahmad et al., 2016), broccoli (Brassica oleracea) (Akram et al., 2020), and chicory (Cichorium intybus) (Abedi et al., 2021) in the case of the regular interval, while in maize (Keyster et al., 2012), cotton (Liu et al., 2013a), and mustard (Brassica Juncea) (Khator and Shekhawat, 2020) for the one-time treatment. However, “continuous” NO treatment limited shoot and root growth under salinity (Figures 4C, 5C), suggesting that a continuous supply of exogenous NO could be toxic to plants instead of providing cellular antioxidant protection. The interaction of NO with ROS accounts for direct sources of both toxicity and protection (Beligni and Lamattina, 1999). ROS acts as a signal for the activation of defense responses when NO is present in small concentrations (Dangl et al., 1996). Higher levels of NO created by unregulated ROS formation, on the other hand, inflict serious damage to plants (Beligni and Lamattina, 1999).
According to this meta-analysis, the effect of NO treatment on the shoot and root dry biomass accumulation was significant and more pronounced when the salt stress lasted for a more extended period (>21 days) (Figures 4D, 5D). Several previous studies on maize (Keyster et al., 2012), rice (Habib et al., 2016), soybean (Glycine max) (Egbichi et al., 2014), and tomato (Wu et al., 2011a) demonstrated that exogenous NO confers salinity tolerance and improves shoot and root biomass during long-term salt exposure by lowering salt stress-induced oxidative stress and caspase-like activity through a pathway that restricts ROS formation via activating antioxidant machinery, stress-responsive gene expression, and molecular signaling. However, when plants were exposed to salinity for less than 20 days, NO treatment did not significantly increase plant biomass (Figures 4D, 5D). These findings suggest that salinity tolerance mediated by NO is a slow but long-lasting process. As a result, it may be possible to mitigate salinity stress throughout the crop growing season effectively.
This meta-analysis showed that exogenous application of NO significantly improves shoot and root growth at both germination and seedling growth stages during salinity stress (Figures 4E, 5E), suggesting that exogenous NO can be applied at both growth stages. NO promotes seed germination by breaking seed dormancy and modulating ABA signaling cascades under salinity conditions (Baudouin, 2011; Signorelli and Considine, 2018; Prakash et al., 2019). Moreover, NO alleviates salt-induced growth inhibition in plant seedlings by enhancing physiological and biochemical parameters (Ren et al., 2020), improving plant biomass accumulation. Studying the impacts of categorical factors on plant growth will aid us in identifying some potentially efficient plant–NO interactions that influence plant growth more strongly in the presence of salt. Interestingly, exogenous NO treatment promoted plant growth traits more effectively in dicot plants than in monocots under salinity stress conditions (Figures 4F, 5F), as salt tolerance variation in response to NO is greater in dicots compared to monocot plants (Choudhary et al., 2022). Most of the research focused on NO-mediated salinity stress alleviation in graminoids and forbs type plants, and meta-analysis showed that the application of NO markedly increased shoot and root growth in these plants (Figures 4G, 5G).
In this meta-analysis, most of the cases studied showed that, under salinity stress, the concentration of exogenous NO for improving shoot biomass ranges from 0.1 to 0.2 mM and for root biomass ranges from 0.05 to 0.2 mM (Figures S1A, C). Furthermore, the meta-regression analysis revealed that the SMD for SDW or RDW did not change as NO concentration increased. As a result, we recommend a lower NO concentration (0.1 mM) as the optimal concentration for future experiments or field applications. Moreover, exogenous NO application can mitigate salinity stress up to 150 mM NaCl (Figures S1B, D).
Does exogenous NO enhance photosynthesis in salt-induced plants?
Salinity stress severely affects the photosynthetic activity of plants by hampering chloroplast structure and function, reducing photosynthetic rate and interfering with stomatal conductance (Teixeira and Pereira, 2007; Chaves et al., 2009; Guidi et al., 2017; Sotiras et al., 2019; Landi et al., 2020). In NO-treated salt-stressed plants, all photosynthetic metrics included in this meta-analysis were significantly better than in non-NO-treated salt-stressed plants (Figure 6). In various methods, exogenous NO can assist plants in mitigating or reducing the negative impacts of salt stress on photosynthesis. As shown in our meta-analysis, NO improved plant osmotic adjustment and nutritional balance (Figure 7), allowing them to maintain a greater leaf area, higher chlorophyll content, stomatal conductance, and subcellular CO2 levels, all of which boosted CO2 assimilation rate and photosynthesis efficiency under normal and salt-stress conditions (Huang et al., 2016; Khoshbakht et al., 2018). Moreover, this meta-analysis showed that NO treatment significantly enhanced Ca2+ and Mg2+ in salt-stressed plants (Figure 7). Several studies have revealed that NO-treated plants have a more significant concentration of these cations, which could be linked to increased chlorophyll and carotenoid pigment production in NO-treated perennial ryegrass (Lolium perenne) (Wang et al., 2013), pepper (Shams et al., 2019), and maize (Kaya et al., 2015).
The appropriate presence of NO improves CO2 assimilation, transpiration, photosynthetic rate, and chlorophyll fluorescence characteristics, all of which improve photosynthetic functioning (Procházková et al., 2013; Alnusairi et al., 2021). Our results revealed that exogenous NO enhances transpiration, stomatal opening, and chlorophyll fluorescence in salt-affected plants (Figure 6). These results might be due to maintaining balanced K+ flux and inducing the expression of the plasma membrane H+-ATPase essential for an optimum K+/Na+ ion ratio to generate protection against salt stress by exogenous NO (Zhao et al., 2004); previously, similar findings were reported in tomato and barley (Hordeum vulgare) (Zhang et al., 2006; Wu et al., 2011a). Furthermore, it was demonstrated that NO application improves photosynthesis in salt-stressed mustard by enhancing RuBisCO enzyme activity and stomatal conductance (Fatma and Khan, 2014; Jahan et al., 2020). Nitric oxide alone or combined with sulfur promotes the synthesis of glutathione, assimilation of sulfur, and optimum production of NO and redox state, which represent the basis of NO-triggered defensive mechanisms of mustard plants (Fatma et al., 2016). The authors hypothesized that the application of NO increases GSH content, which plays a role in cellular redox homeostasis and regulation of stomatal movement. It was also demonstrated that GSH interacts with ABA to regulate stomatal movement (Misra et al., 2015).
Does exogenous NO robust plants’ osmotic potential and nutrient balance under salt stress?
Our meta-analysis revealed that exogenous NO-enhanced essential minerals, e.g., K+, Ca2+, and Mg2+ uptake, and decreased toxic Na+ content in the salt-stressed plants (Figure 7B). Under salinity, plants invoke stress tolerance through ion homeostasis by increasing the K+/Na+ ratio, improving Ca2+/Mg2+ uptake, regulating ion uptake from roots, ion transport, and compartmentalization (Lu et al., 2014; Kolbert, 2016; Goyal et al., 2021). The Na+/H+ antiporter enzyme is involved in the elimination of cytosolic Na+ and the increase of Ca2+ and Mg2+ influx during this compartmentalization process (Chen et al., 2013; Goyal et al., 2021). Nitric oxide activates the H+-ATPase and H+-PPase enzymes in the vacuole, forcing the Na+/H+ ion exchange to detoxify the cell. Several studies have found that during Na+ compartmentalization, H+-ATPase activity increases. Under salt stress, NO mediates root K+/Na+ balance in maize (Zhang et al., 2006), sunflower (Helianthus annus) (David et al., 2010), and mangrove plant Kandelia obovata (Chen et al., 2013), via enhancing the expression of the AKT1-type K+ channel and Na+/H+ antiporter. It has also been reported that exogenous combined NO and H2S treatment increases salinity tolerance in barley by boosting H+-ATPase and Na+/H+ antiporter expression and K+ channel activity (Chen et al., 2015).
Osmotic stress caused by salinity can be alleviated by the buildup of osmolytes such as proline, soluble carbohydrates, and soluble proteins (Hayat et al., 2012a; Li et al., 2016). According to this meta-analysis (Figure 7A), the treatment of NO increases proline accumulation in plants but not soluble carbohydrates or soluble proteins under salt stress, suggesting that NO-mediated osmotic balance is mainly conferred by proline, and soluble sugar accumulation might not be stimulated by exogenous NO under salt stress. It has been previously reported that exogenous NO promotes the accumulation of osmotic components (e.g., proline and glycine betaine), which play an essential role in osmoregulation, membrane stability, cell water content maintenance, and stress mitigation in crop plants (Ahmad and Sharma, 2008; Ahanger et al., 2017). Exogenous NO has also been discovered to stimulate the P5CS1 gene, which codes for d1-pyrroline-5-carboxylate synthase, a critical enzyme involved in proline production in Chlamydomonas reinhardtii (Zhang et al., 2008; Rejeb et al., 2014). According to the review by Ahmad et al. (2016), NO-induced cell osmoregulation has been found in a variety of crops, including linseed (Linum usitatissimum), Brassica, chickpea, and mulberry (Morus alba).
Several investigations have demonstrated that exogenous NO treatment increases the level of soluble sugars and soluble proteins along with proline in salt-exposed plants through inducing osmotic homeostasis mechanisms, for example, salt-stress related sugars and proteins synthesis, CO2 assimilation, enzyme activities, and expression of specific genes (Gibson, 2005; Gupta and Kaur, 2005; Doganlar et al., 2010) in tomato (Wu et al., 2011a) and cotton (Dong et al., 2014). Our meta-analysis results showed no significant increase in soluble sugar and protein content (Figure 7A), which contradicted the previously described results. Further investigations are required to determine whether the exogenous NO triggers the possible pathways for increasing total soluble sugar and soluble protein levels during salinity stress.
How does exogenous NO regulate plants’ antioxidant system and ROS scavenging during salt-induced stress?
Salt stress causes plants to accumulate many ROS, resulting in oxidative damage and a loss of membrane lipid and membrane integrity (Ahmad, 2010; Hayat et al., 2012a; AbdElgawad et al., 2016). This meta-analysis showed that NO supplementation detoxifies ROS generation and pacifies oxidative damage by significantly reducing MDA production and markedly boosting the antioxidants SOD, CAT, POX, APX, GR, DHAR, GSH, and ASC in salt-stressed plants (Figure 8). Farooq et al. (2009) and Qiao et al. (2014) previously described the mechanisms by which NO provides salt tolerance in plants by restoring redox equilibrium in salt-stressed plants by enhancing the activity of ROS scavenging enzymes such as CAT, SOD, APX, GR, and GPX. NO helps attenuate oxidative stress by upregulating both enzymatic and non-enzymatic antioxidants (Christou et al., 2014; Kong et al., 2016; da-Silva et al., 2018). The antioxidant enzymes in NO-treated plants were dramatically upregulated, and ROS-producing enzymes were downregulated, resulting in the rapid removal of excess accumulated free radicals and the stability of structural and functional elements of cellular membranes in agricultural plants like Brassica oleracea (Hernandez et al., 2010), maize (Carrasco-Ríos and Pinto, 2014), pepper (Shams et al., 2019), and wheat (Alnusairi et al., 2021).
NO can operate as a signaling molecule or ROS scavenger by modulating or boosting the activities of antioxidant enzymes under sustained stress circumstances (Hao et al., 2008; Xu et al., 2010; Fan and Liu, 2012). The expression of representative SOD, CAT, and APX genes examined was upregulated by exogenous NO treatment in salt-stressed plants like soybean (Egbichi et al., 2014), chickpea (Ahmad et al., 2016), tomato (Aydin et al., 2014), Limonium sinense (Zhang et al., 2014), Lotus japonicus (Rubio Luna et al., 2009) and rice (Shafi et al., 2015). Similarly, this meta-analysis indicates that upregulation of CAT, SOD, APX, GR, GPX, and DHAR genes might enhance the activities of the SOD, CAT, APX, and DHAR enzymes; as a result, the cells are better protected against oxidative damage caused by high salinity (Lu et al., 2007; Yamane et al., 2010; Hu et al., 2012). Therefore, NO stimulates the expression of antioxidant enzyme-related biosynthetic genes, resulting in the buildup of antioxidant enzymes and hence greater stress tolerance in plants. NO suppress oxidative damage and avert cell membrane injury and proton extrusion through upregulated H+-ATPase activity in stressed plants (Kharbech et al., 2020). Reports have suggested that NO-signaling helps to regulate this cycle via S-nitrosylation. Under salt-induced stress, the activities of four vital enzymes of the ASC–GSH cycle, namely, APX, DHAR, and GR, are hampered (Rahman et al., 2016). Furthermore, NO and sulfur combine to increase APX activity, allowing it to efficiently detoxify H2O2 and O2•, resulting in a robust antioxidative defense against salinity (Fatma and Khan, 2014; Fatma et al., 2016).
Recommendations and research gaps
The current systematic review emphasizes the role of NO-mediated plant salinity tolerance and exogenous NO application in alleviating plant salinity stress. This meta-analysis also highlights various aspects that require further investigation to gain a thorough and robust understanding of NO-conferred salinity stress tolerance in plants. Based on this meta-analysis, we enclose the following recommendations or research gaps:
• The majority of research has concentrated on the effects of NO on plant physiology by utilizing seed priming and foliar application under saline conditions. However, there has been less attention dedicated to determining the effects of NO in root medium-based approaches. Before advocating for root medium-based treatments, we need further evidence on the variability and durability of NO-induced salinity tolerance.
• From a mechanistic standpoint, most articles measured osmolyte content in NO-treated and non-NO-treated plants, but the underlying mechanisms of this response at the molecular level need to be investigated further.
• Plant lipid metabolism is altered during salt stress, which is linked to changes in membrane integrity, constitution, and activity (Parihar et al., 2015). Although lipid peroxidation has been examined in salt-stressed NO-treated plants, overall changes in lipid metabolism in response to exogenous NO have gained less priority.
• Very few studies have described the effects of exogenous NO on the nutritional aspects of crops. We have yet to explore whether the contents of bioactive nutritional components are improved or decreased by NO under salt stress.
• Salinity stress limits plant growth and biomass building by disrupting photosynthetic pigments, osmotic equilibrium, enzyme activity, and ionic homeostasis. Exogenously applied NO reliably mitigates the impact of salt stress on plants by regulating their physiological functions and oxidative tolerance. NO-mediated salinity stress tolerance in plants has far-reaching ecological and agro-economic implications. Improved salinity tolerance may ensure higher crop yields in some agronomic fields. Continued research on exogenous NO application could lead to discovering the fundamental processes of plant-NO interactions, which appear to be well-founded.
• Many previous studies and our meta-analysis proved that exogenous NO application significantly improves the growth and yield of plants. However, there was no conclusive data and findings on the economic feasibility of NO-mediated crop cultivation in the saline-pone agronomic fields. A further investigation is recommended to study the impact level of exogenous NO on crop yield performance following the economic profitability and cost-effectiveness of farmers.
• NO treatment effect on C3 plants has been extensively described, whereas only a few investigations on C4 plants have been conducted under salt stress. More studies on exogenous NO-mediated salinity tolerance in C4 plants are needed to get a definitive conclusion.
• Although many studies on exogenous NO-induced salinity tolerance have been reported at germination stage in field levels, a limited study was conducted at the reproductive stage. Therefore, the ameliorative role of NO on plant growth and yields at the reproductive stage in field conditions needs to be investigated further.
• Many of these experiments were undertaken in greenhouses or under controlled growth chamber conditions, with limited investigations conducted at the field level. Further research on the variability and stability of NO-induced salinity tolerance is required before farm-level recommendations.
• Continuous NO application in root did not positively affect but rather had adverse effects. Thus, future studies should avoid continuous NO application for mitigating salinity stress.
• Typically, studies are conducted on a single method. Further research should be conducted in which NO will be used in various methods to provide a comparative conclusion. Furthermore, different methods should be used throughout the plant life cycle in a single study. For example, studies should be conducted in which seed priming with NO will be applied at the seedling stage and foliar pretreatment at the vegetative or reproductive stage.
Conclusion
The present meta-analysis of 62 peer-reviewed articles demonstrated the NO-mediated morpho-physiological and biochemical response and stress tolerance mechanisms in plants under salinity conditions. In particular, we determined the effects of different sub-categories in NO-treated and salt-stressed plants. Exogenous application of NO considerably influences biomass accumulation, growth, and yield in both salt-stressed and non-stressed plants. Our meta-analysis reveals that exogenous NO application is more effective when the salt-stressed plants are grown in growth chambers and at germination and seedling growth stages under prolonged salinity stress. The number of field-level reproductive stage experiments is minimal, and future research should concentrate on this topic. Among the NO application methods, seed priming and foliar pre-treatment are the most efficient when NO is applied for a one-time or regular interval. Furthermore, the optimum NO concentration ranges from 0.1 to 0.2 mM, which alleviates salinity stress up to 150 mM. Interestingly, exogenous NO treatment boosts plant growth most efficiently in dicots. This meta-analysis shows exogenous NO strongly confers plant salinity stress tolerance by maintaining physiological and biochemical processes such as osmotic and nutritional balance, and photosynthetic and antioxidant activity. Further investigations are recommended to study the roles of lipid metabolism, nutritional content changes of grain crops, responses of C4 plants, and field-level crop yield improvements under salinity stress in response to exogenous NO application.
Data availability statement
The original contributions presented in the study are included in the article/Supplementary Materials. Further inquiries can be directed to the corresponding authors.
Author contributions
MTUA developed the concept, performed the database search, partially collected the data, analyzed and interpreted the results, and wrote the draft. IJ, ZHS, AAMS, SHT, and IH extracted the data. KMGD analyzed the data and produced graphs. MH and IJ wrote the draft. XW, FZ, JC, RI, IA, and MM revised the manuscript. MTUA, AES, and YM revised the manuscript and supervised the project. All authors approved the final version of the manuscript.
Conflict of interest
The authors declare that the research was conducted in the absence of any commercial or financial relationships that could be construed as a potential conflict of interest.
Publisher’s note
All claims expressed in this article are solely those of the authors and do not necessarily represent those of their affiliated organizations, or those of the publisher, the editors and the reviewers. Any product that may be evaluated in this article, or claim that may be made by its manufacturer, is not guaranteed or endorsed by the publisher.
Supplementary material
The Supplementary Material for this article can be found online at: https://www.frontiersin.org/articles/10.3389/fpls.2022.957735/full#supplementary-material
Supplementary Figure 1 | Effects of different concentrations of NO and salinity on SDW (A, B) and RDW (C, D) of different plant species.
Supplementary Table 1 | List of published research articles used for data extraction in this meta-analysis.
Supplementary Table 2 | Comparative effects of different salinity levels on shoot and root biomass production in NO-treated plants.
Supplementary Table 3 | Heterogeneity statistics for the growth-related traits summary effect sizes under non-saline and saline stress conditions.
References
AbdElgawad, H., Zinta, G., Hegab, M. M., Pandey, R., Asard, H., Abuelsoud, W. (2016). High salinity induces different oxidative stress and antioxidant responses in maize seedlings organs. Front. Plant Sci. 7, 276. doi: 10.3389/fpls.2016.00276
Abedi, S., Iranbakhsh, A., Oraghi Ardebili, Z., Ebadi, M. (2021). Nitric oxide and selenium nanoparticles confer changes in growth, metabolism, antioxidant machinery, gene expression, and flowering in chicory (Cichorium intybus l.): potential benefits and risk assessment. Environ. Sci. pollut. Res. 28, 3136–3148. doi: 10.1007/s11356-020-10706-2
Acosta-Motos, J. R., Ortuño, M. F., Bernal-Vicente, A., Diaz-Vivancos, P., Sanchez-Blanco, M. J., Hernandez, J. A. (2017). Plant responses to salt stress: adaptive mechanisms. Agronomy 7, 18. doi: 10.3390/agronomy7010018
Adamu, T. A., Mun, B.-G., Lee, S.-U., Hussain, A., Yun, B.-W. (2018). Exogenously applied nitric oxide enhances salt tolerance in rice (Oryza sativa l.) at seedling stage. Agronomy 8, 276. doi: 10.3390/agronomy8120276
Ahanger, M. A., Tomar, N. S., Tittal, M., Argal, S., Agarwal, R. (2017). Plant growth under water/salt stress: ROS production; antioxidants and significance of added potassium under such conditions. Physiol. Mol. Biol. Plants 23, 731–744. doi: 10.1007/s12298-017-0462-7
Ahmad, P. (2010). Growth and antioxidant responses in mustard (Brassica juncea l.) plants subjected to combined effect of gibberellic acid and salinity. Arch. Agron. Soil Sci. 56, 575–588. doi: 10.1080/03650340903164231
Ahmad, P., Abdel Latef, A. A., Hashem, A., Abd_Allah, E. F., Gucel, S., Tran, L.-S. P. (2016). Nitric oxide mitigates salt stress by regulating levels of osmolytes and antioxidant enzymes in chickpea. Front. Plant Sci. 7, 347. doi: 10.3389/fpls.2016.00347
Ahmad, H., Hayat, S., Ali, M., Liu, T., Cheng, Z. (2018). The combination of arbuscular mycorrhizal fungi inoculation (Glomus versiforme) and 28-homobrassinolide spraying intervals improves growth by enhancing photosynthesis, nutrient absorption, and antioxidant system in cucumber (Cucumis sativus l.) under salinity. Ecol. Evol. 8, 5724–5740. doi: 10.1002/ece3.4112
Ahmad, P., Sharma, S. (2008). Salt stress and phyto-biochemical responses of plants. Plant Soil Environ. 54, 89–99. doi: 10.17221/2774-PSE
Ahmed, H., Zeng, Y., Raza, H., Muhammad, D., Iqbal, M., Uzair, M., et al. (2022). Characterization of wheat accessions under salinity stress. Front. Plant Sci. 2583. doi: 10.3389/fpls.2022.953670
Akram, N. A., Hafeez, N., Farid-ul-Haq, M., Ahmad, A., Sadiq, M., Ashraf, M. (2020). Foliage application and seed priming with nitric oxide causes mitigation of salinity-induced metabolic adversaries in broccoli (Brassica oleracea l.) plants. Acta Physiologiae Plantarum 42, 1–9. doi: 10.1007/s11738-020-03140-x
Ali, Q., Daud, M., Haider, M. Z., Ali, S., Rizwan, M., Aslam, N., et al. (2017). Seed priming by sodium nitroprusside improves salt tolerance in wheat (Triticum aestivum l.) by enhancing physiological and biochemical parameters. Plant Physiol. Biochem. 119, 50–58. doi: 10.1016/j.plaphy.2017.08.010
Alnusairi, G. S., Mazrou, Y. S., Qari, S. H., Elkelish, A. A., Soliman, M. H., Eweis, M., et al. (2021). Exogenous nitric oxide reinforces photosynthetic efficiency, osmolyte, mineral uptake, antioxidant, expression of stress-responsive genes and ameliorates the effects of salinity stress in wheat. Plants 10, 1693. doi: 10.3390/plants10081693
Anwar, M. P., Khalid, M. A. I., Islam, A. M., Yeasmin, S., Sharif, A., Hadifa, A., et al. (2021). Potentiality of different seed priming agents to mitigate cold stress of winter rice seedling. Phyton 90 (5), 1491. doi: 10.32604/phyton.2021.015822
Asgher, M., Per, T. S., Masood, A., Fatma, M., Freschi, L., Corpas, F. J., et al. (2017). Nitric oxide signaling and its crosstalk with other plant growth regulators in plant responses to abiotic stress. Environ. Sci. pollut. Res. 24, 2273–2285. doi: 10.1007/s11356-016-7947-8
Aydin, S., BÜyÜk, İ., ARAS, E. S. (2014). Expression of SOD gene and evaluating its role in stress tolerance in NaCl and PEG stressed lycopersicum esculentum. Turkish J. Bot. 38, 89–98. doi: 10.3906/bot-1305-1
Bai, X., Yang, L., Yunqiang, Y., Ahmad, P., Yongping, Y., Hu, X. (2011). Deciphering the protective role of nitric oxide against salt stress at the physiological and proteomic levels in maize. J. Proteome Res. 10, 4349–4364. doi: 10.1021/pr200333f
Balduzzi, S., Rücker, G., Schwarzer, G. (2019). How to perform a meta-analysis with r: a practical tutorial. Evidence-Based Ment. Health 22, 153–160. doi: 10.1136/ebmental-2019-300117
Bashir, F., Ali, M., Hussain, K., Majeed, A., Nawaz, K. (2011). Morphological variations in sorghum (Sorghum bicolor l.) under different levels of Na2SO4 salinity. Bot. Res. Int. 4, 1–3.
Baudouin, E. (2011). The language of nitric oxide signalling. Plant Biol. 13, 233–242. doi: 10.1111/j.1438-8677.2010.00403.x
Begg, C. B., Mazumdar, M. (1994). Operating characteristics of a rank correlation test for publication bias. Biometrics 50, 1088–1101. doi: 10.2307/2533446
Beligni, M., Lamattina, L. (1999). Is nitric oxide toxic or protective? Trends Plant Sci. 4 (50), 299–300. doi: 10.1016/S1360-1385(99)01451-X
Borenstein, M., Hedges, L., Higgins, J., Rothstein, H. (2009). Introduction to meta-analysis (New York, NY: JohnWiley and Sons).
Borenstein, M., Higgins, J. (2013). Meta-analysis and subgroups. Prev. Sci. 14, 134–143. doi: 10.1007/s11121-013-0377-7
Carrasco-Ríos, L., Pinto, M. (2014). Effect of salt stress on antioxidant enzymes and lipid peroxidation in leaves in two contrasting corn,’Lluteno’and’Jubilee’. Chilean J. Agric. Res. 74, 89–95. doi: 10.4067/S0718-58392014000100014
Chaves, M. M., Flexas, J., Pinheiro, C. (2009). Photosynthesis under drought and salt stress: regulation mechanisms from whole plant to cell. Ann. Bot. 103, 551–560. doi: 10.1093/aob/mcn125
Chen, J., Wang, W.-H., Wu, F.-H., He, E.-M., Liu, X., Shangguan, Z.-P., et al. (2015). Hydrogen sulfide enhances salt tolerance through nitric oxide-mediated maintenance of ion homeostasis in barley seedling roots. Sci. Rep. 5, 1–19. doi: 10.1038/srep12516
Chen, J., Xiong, D.-Y., Wang, W.-H., Hu, W.-J., Simon, M., Xiao, Q., et al. (2013). Nitric oxide mediates root K+/Na+ balance in a mangrove plant, kandelia obovata, by enhancing the expression of AKT1-type k+ channel and Na+/H+ antiporter under high salinity. PLos One 8, e71543. doi: 10.1371/journal.pone.0071543
Choudhary, S., Wani, K. I., Naeem, M., Khan, M., Aftab, T. (2022). Cellular responses, osmotic adjustments, and role of osmolytes in providing salt stress resilience in higher plants: Polyamines and nitric oxide crosstalk. J. Plant Growth Regul., 1–15. doi: 10.1007/s00344-022-10584-7
Christou, A., Filippou, P., Manganaris, G. A., Fotopoulos, V. (2014). Sodium hydrosulfide induces systemic thermotolerance to strawberry plants through transcriptional regulation of heat shock proteins and aquaporin. BMC Plant Biol. 14, 1–11. doi: 10.1186/1471-2229-14-42
Cohen, J. (1988). Statistical power analysis for the behavioral sciences (Hillsdale, NJ: Lawrence Erlbaum Associates), 20–26.
Correa-Aragunde, N., Graziano, M., Lamattina, L. (2004). Nitric oxide plays a central role in determining lateral root development in tomato. Planta 218, 900–905. doi: 10.1007/s00425-003-1172-7
Dangl, J. L., Dietrich, R. A., Richberg, M. H. (1996). Death don’t have no mercy: cell death programs in plant-microbe interactions. Plant Cell 8, 1793. doi: 10.1105/tpc.8.10.1793
da-Silva, C. J., Canatto, R. A., Cardoso, A. A., Ribeiro, C., de Oliveira, J. A. (2018). Oxidative stress triggered by arsenic in a tropical macrophyte is alleviated by endogenous and exogenous nitric oxide. Braz. J. Bot. 41, 21–28. doi: 10.1007/s40415-017-0431-y
Dastogeer, K. M. (2018). Influence of fungal endophytes on plant physiology is more pronounced under stress than well-watered conditions: a meta-analysis. Planta 248, 1403–1416. doi: 10.1007/s00425-018-2982-y
Dastogeer, K. M., Zahan, M. I., Tahjib-Ul-Arif, M., Akter, M. A., Okazaki, S. (2020). Plant salinity tolerance conferred by arbuscular mycorrhizal fungi and associated mechanisms: a meta-analysis. Front. Plant Sci. 1927. doi: 10.3389/fpls.2020.588550
David, A., Yadav, S., Bhatla, S. C. (2010). Sodium chloride stress induces nitric oxide accumulation in root tips and oil body surface accompanying slower oleosin degradation in sunflower seedlings. Physiologia Plantarum 140, 342–354. doi: 10.1111/j.1399-3054.2010.01408.x
Dawood, M. F., Sohag, A. A. M., Tahjib-Ul-Arif, M., Latef, A. A. H. A. (2021). Hydrogen sulfide priming can enhance the tolerance of artichoke seedlings to individual and combined saline-alkaline and aniline stresses. Plant Physiol. Biochem. 159, 347–362. doi: 10.1016/j.plaphy.2020.12.034
Doganlar, Z. B., Demir, K., Basak, H., Gul, I. (2010). Effects of salt stress on pigment and total soluble protein contents of three different tomato cultivars. Afr. J. Agric. Res. 5, 2056–2065. doi: 10.5897/AJAR10.258
Dong, Y., Jinc, S., Liu, S., Xu, L., Kong, J. (2014). Effects of exogenous nitric oxide on growth of cotton seedlings under NaCl stress. J. Soil Sci. Plant Nutr. 14, 1–13. doi: 10.4067/S0718-95162014005000001
Dong, Y., Wang, Z., Zhang, J., Liu, S., He, Z., He, M. (2015a). Interaction effects of nitric oxideand salicylic acid in alleviating salt stress of gossypium hirsutum l. J. Soil Sci. Plant Nutr. 15, 561–573. doi: 10.4067/S0718-95162015005000024
Duval, S., Tweedie, R. (2000). Trim and fill: a simple funnel-plot–based method of testing and adjusting for publication bias in meta-analysis. Biometrics 56, 455–463. doi: 10.1111/j.0006-341X.2000.00455.x
Eagle, A. J., Christianson, L. E., Cook, R. L., Harmel, R. D., Miguez, F. E., Qian, S. S., et al. (2017). Meta-analysis constrained by data: Recommendations to improve relevance of nutrient management research. Agron. J. 109, 2441–2449. doi: 10.2134/agronj2017.04.0215
Egbichi, I., Keyster, M., Ludidi, N. (2014). Effect of exogenous application of nitric oxide on salt stress responses of soybean. South Afr. J. Bot. 90, 131–136. doi: 10.1016/j.sajb.2013.11.002
Egger, M., Smith, G. D., Schneider, M., Minder, C. (1997). Bias in meta-analysis detected by a simple, graphical test. Bmj 315, 629–634. doi: 10.1136/bmj.315.7109.629
El Sabagh, A., Islam, M. S., Iqbal, M. A., Hossain, A., Mubeen, M., Jabeen, T., et al. (2021a). “Salinity stress in cotton: Adverse effects, survival mechanisms and management strategies,” in Engineering tolerance in crop plants against abiotic stress (Boca Raton: CRC Press), pp. 59–80.
El Sabagh, A., Mbarki, S., Hossain, A., Iqbal, M.A., Islam, M.S., Raza, A., et al (2021b). Potential role of plant growth regulators in administering crucial processes against abiotic stresses. Front. Agron 3, 648694. doi: 10.3389/fagro.2021.64869
Eziz, A., Yan, Z., Tian, D., Han, W., Tang, Z., Fang, J. (2017). Drought effect on plant biomass allocation: A meta-analysis. Ecol. Evol. 7, 11002–11010. doi: 10.1002/ece3.3630
Fahad, S., Hussain, S., Matloob, A., Khan, F. A., Khaliq, A., Saud, S., et al. (2015). Phytohormones and plant responses to salinity stress: a review. Plant Growth Regul. 75, 391–404. doi: 10.1007/s10725-014-0013-y
Fan, H.-F., Du, C.-X., Ding, L., Xu, Y.-L. (2013a). Effects of nitric oxide on the germination of cucumber seeds and antioxidant enzymes under salinity stress. Acta physiologiae plantarum 35, 2707–2719. doi: 10.1007/s11738-013-1303-0
Fan, Q.-J., Liu, J.-H. (2012). Nitric oxide is involved in dehydration/drought tolerance in poncirus trifoliata seedlings through regulation of antioxidant systems and stomatal response. Plant Cell Rep. 31, 145–154. doi: 10.1007/s00299-011-1148-1
Faraone, S. V. (2008). Interpreting estimates of treatment effects: implications for managed care. Pharm. Ther. 33, 700.
Farooq, M., Wahid, A., Kobayashi, N., Fujita, D., Basra, S. (2009). Plant drought stress: effects, mechanisms and management, in: Sustainable agriculture (Netherlands: Springer), 153–188.
Fatma, M., Khan, N. A. (2014). Nitric oxide protects photosynthetic capacity inhibition by salinity in Indian mustard Funct. Environ. Botany 4:106–116. doi: 10.5958/2231-1750.2014.00009.2
Fatma, M., Masood, A., Per, T. S., Khan, N. A. (2016). Nitric oxide alleviates salt stress inhibited photosynthetic performance by interacting with sulfur assimilation in mustard. Funct. Environ. Botany 4, 106–116. doi: 10.3389/fpls.2016.00521
Field, A. P., Gillett, R. (2010). How to do a meta-analysis. Br. J. Math. Stat. Psychol. 63, 665–694. doi: 10.1348/000711010X502733
Gerstner, K., Moreno-Mateos, D., Gurevitch, J., Beckmann, M., Kambach, S., Jones, H. P., et al. (2017). Will your paper be used in a meta-analysis? Make reach your Res. broader longer lasting. Methods Ecol. Evol. 8, 777–784. doi: 10.1111/2041-210X.12758
Gibson, S. I. (2005). Control of plant development and gene expression by sugar signaling. Curr. Opin. Plant Biol. 8, 93–102. doi: 10.1016/j.pbi.2004.11.003
Goyal, V., Jhanghel, D., Mehrotra, S. (2021). Emerging warriors against salinity in plants: Nitric oxide and hydrogen sulphide. Physiologia Plantarum 171, 896–908. doi: 10.1111/ppl.13380
Guidi, L., Remorini, D., Cotrozzi, L., Giordani, T., Lorenzini, G., Massai, R., et al. (2017). The harsh life of an urban tree: the effect of a single pulse of ozone in salt-stressed quercus ilex saplings. Tree Physiol. 37, 246–260. doi: 10.1093/treephys/tpw103
Gupta, B., Huang, B. (2014). Mechanism of salinity tolerance in plants: physiological, biochemical, and molecular characterization. Int. J. Genomics 2014, 701596. doi: 10.1155/2014/701596
Gupta, A. K., Kaur, N. (2005). Sugar signalling and gene expression in relation to carbohydrate metabolism under abiotic stresses in plants. J. Biosci. 30, 761–776. doi: 10.1007/BF02703574
Gurevitch, J., Hedges, L. V. (1999). Statistical issues in ecological meta-analyses. Ecology 80, 1142–1149. doi: 10.1890/0012-9658(1999)080[1142:SIIEMA]2.0.CO;2
Gurevitch, J., Koricheva, J., Nakagawa, S., Stewart, G. (2018). Meta-analysis and the science of research synthesis. Nature 555, 175–182. doi: 10.1038/nature25753
Habib, N., Akram, M., Javed, M., Azeem, M., Ali, Q., Shaheen, H., et al. (2016). Nitric oxide regulated improvement in growth and yield of rice plants grown under salinity stress: antioxidant defense system. Appl. Ecol. Environ. Res. 14, 91–105. doi: 10.15666/aeer/1405_091105
Hanin, M., Ebel, C., Ngom, M., Laplaze, L., Masmoudi, K. (2016). New insights on plant salt tolerance mechanisms and their potential use for breeding. Front. Plant Sci. 7, 1787. doi: 10.3389/fpls.2016.01787
Hao, G., Xing, Y., Zhang, J. (2008). Role of nitric oxide dependence on nitric oxide synthase-like activity in the water stress signaling of maize seedling. J. Integr. Plant Biol. 50, 435–442. doi: 10.1111/j.1744-7909.2008.00637.x
Harrer, M., Cuijpers, P., Furukawa, T., Ebert, D. D. (2019). “Dmetar: Companion r package for the guide’Doing meta-analysis in r’,” in R package version 0.0, 9000. Available at: http://dmetar.protectlab.org/.
Hasanuzzaman, M. (2022). Salt stress tolerance in rice and wheat: Physiological and molecular mechanism. Plant Defense Mechanisms (IntechOpen). doi: 10.5772/intechopen.101529
Hayat, S., Hayat, Q., Alyemeni, M. N., Wani, A. S., Pichtel, J., Ahmad, A. (2012a). Role of proline under changing environments: a review. Plant Signaling Behav. 7, 1456–1466. doi: 10.4161/psb.21949
Hayat, S., Yadav, S., Wani, A. S., Irfan, M., Alyemini, M. N., Ahmad, A. (2012b). Impact of sodium nitroprusside on nitrate reductase, proline content, and antioxidant system in tomato under salinity stress. Horticult. Environment Biotechnol. 53, 362–367. doi: 10.1007/s13580-012-0481-9
Hedges, L. V., Gurevitch, J., Curtis, P. S. (1999). The meta-analysis of response ratios in experimental ecology. Ecology 80, 1150–1156. doi: 10.1890/0012-9658(1999)080[1150:TMAORR]2.0.CO;2
He, M., Dijkstra, F. A. (2014). Drought effect on plant nitrogen and phosphorus: a meta-analysis. New Phytol. 204, 924–931. doi: 10.1111/nph.12952
Hernández, J. A. (2019). Salinity tolerance in plants: Trends and perspectives. Int. J. Mol. Sci. 20, 2408. doi: 10.3390/ijms20102408
Hernandez, M., Fernandez-Garcia, N., Diaz-Vivancos, P., Olmos, E. (2010). A different role for hydrogen peroxide and the antioxidative system under short and long salt stress in brassica oleracea roots. J. Exp. Bot. 61, 521–535. doi: 10.1093/jxb/erp321
Hernández, J. A., Ferrer, M. A., Jiménez, A., Barceló, A. R., Sevilla, F. (2001). Antioxidant systems and O2.–/H2O2 production in the apoplast of pea leaves. Its relation salt-induced necrotic lesions minor veins. Plant Physiol. 127, 817–831. doi: 10.1104/pp.010188
Higgins, J. P., Thompson, S. G. (2002). Quantifying heterogeneity in a meta-analysis. Stat Med. 21, 1539–1558. doi: 10.1002/sim.1186
Higgins, J., Tompson, S., Deeks, J., Altman, D. (2003). A meta-analysis on the effectiveness of smart-learning. BMJ 327, 557–560. doi: 10.1136/bmj.327.7414.557
Huang, S., Van Aken, O., Schwarzländer, M., Belt, K., Millar, A. H. (2016). The roles of mitochondrial reactive oxygen species in cellular signaling and stress response in plants. Plant Physiol. 171, 1551–1559. doi: 10.1104/pp.16.00166
Huedo-Medina, T. B., Sánchez-Meca, J., Marin-Martinez, F., Botella, J. (2006). Assessing heterogeneity in meta-analysis: Q statistic or I2 index? psychol. Methods 11, 193. doi: 10.1037/1082-989X.11.2.193
Hu, L., Li, H., Pang, H., Fu, J. (2012). Responses of antioxidant gene, protein and enzymes to salinity stress in two genotypes of perennial ryegrass (Lolium perenne) differing in salt tolerance. J. Plant Physiol. 169, 146–156. doi: 10.1016/j.jplph.2011.08.020
Hussain, K., Ashraf, M., Ashraf, M. Y. (2008). Relationship between growth and ion relation in pearl millet (Pennisetum glaucum (L.) r. br.) at different growth stages under salt stress. Afr. J. Plant Sci. 2, 023–027.
IntHout, J., Ioannidis, J., Borm, G. F. (2014). The hartung-Knapp-Sidik-Jonkman method for random effects meta-analysis is straightforward and considerably outperforms the standard DerSimonian-laird method. BMC Med. Res. Method. 14, 1–12. doi: 10.1186/1471-2288-14-25
Iqbal, M. M., Murtaza, G., Saqib, Z. A., Rashid, A. (2015). Growth and physiological responses of two rice varieties to applied lead in normal and salt-affected soils. Int. J. Agric. Biol. 17, 901–910. doi: 10.17957/IJAB/15.0026
Isayenkov, S. (2012). Physiological and molecular aspects of salt stress in plants. Cytology Genet. 46, 302–318. doi: 10.3103/S0095452712050040
Isayenkov, S. V., Maathuis, F. J. (2019). Plant salinity stress: many unanswered questions remain. Front. Plant Sci. 10, 80. doi: 10.3389/fpls.2019.00080
Jabbarzadeh, M., Tehranifar, A., Amiri, J. (2018). The effect of different concentrations of sodium nitroprusside (SNP) on some morphological, physiological and biochemical traits of zinnia elegans under salinity stress. J. Plant Process Funct. 6, 105–116.
Jabeen, Z., Fayyaz, H. A., Irshad, F., Hussain, N., Hassan, M. N., Li, J., et al. (2021). Sodium nitroprusside application improves morphological and physiological attributes of soybean (Glycine max l.) under salinity stress. PLos One 16, e0248207. doi: 10.1371/journal.pone.0248207
Jahan, B., AlAjmi, M. F., Rehman, M. T., Khan, N. A. (2020). Treatment of nitric oxide supplemented with nitrogen and sulfur regulates photosynthetic performance and stomatal behavior in mustard under salt stress. Physiologia plantarum 168, 490–510. doi: 10.1111/ppl.13056
Jamali, B., Eshghi, S., Tafazoli, E. (2015). Mineral composition of ‘Selva’strawberry as affected by time of application of nitric oxide under saline conditions. Horticult. Environment Biotechnol. 56, 273–279. doi: 10.1007/s13580-015-0116-z
Kausar, F., Shahbaz, M., Ashraf, M. (2013). Protective role of foliar-applied nitric oxide in triticum aestivum under saline stress. Turkish J. Bot. 37, 1155–1165. doi: 10.3906/bot-1301-17
Kaya, C., ASHRAF, M., Sönmez, O., Tuna, A. L., Aydemir, S. (2015). Exogenously applied nitric oxide confers tolerance to salinity-induced oxidativestress in two maize (Zea mays l.) cultivars differing in salinity tolerance. Turkish J. Agric. Forestry 39, 909–919. doi: 10.3906/tar-1411-26
Keyster, M., Klein, A., Ludidi, N. (2012). Caspase-like enzymatic activity and the ascorbate-glutathione cycle participate in salt stress tolerance of maize conferred by exogenously applied nitric oxide. Plant Signaling Behav. 7, 349–360. doi: 10.4161/psb.18967
Khan, M. N., Siddiqui, M. H., Mohammad, F., Naeem, M. (2012). Interactive role of nitric oxide and calcium chloride in enhancing tolerance to salt stress. Nitric. Oxide 27, 210–218. doi: 10.1016/j.niox.2012.07.005
Kharbech, O., Sakouhi, L., Massoud, M. B., Mur, L. A. J., Corpas, F. J., Djebali, W., et al. (2020). Nitric oxide and hydrogen sulfide protect plasma membrane integrity and mitigate chromium-induced methylglyoxal toxicity in maize seedlings. Plant Physiol. Biochem. 157, 244–255. doi: 10.1016/j.plaphy.2020.10.017
Khator, K., Shekhawat, G. (2020). Nitric oxide mitigates salt-induced oxidative stress in brassica juncea seedlings by regulating ROS metabolism and antioxidant defense system. 3 Biotech. 10, 1–12. doi: 10.1007/s13205-020-02493-x
Khoshbakht, D., Asghari, M., Haghighi, M. (2018). Effects of foliar applications of nitric oxide and spermidine on chlorophyll fluorescence, photosynthesis and antioxidant enzyme activities of citrus seedlings under salinity stress. Photosynthetica 56, 1313–1325. doi: 10.1007/s11099-018-0839-z
Klein, A., Hüsselmann, L., Keyster, M., Ludidi, N. (2018). Exogenous nitric oxide limits salt-induced oxidative damage in maize by altering superoxide dismutase activity. South Afr. J. Bot. 115, 44–49. doi: 10.1016/j.sajb.2017.12.010
Kolbert, Z. (2016). Implication of nitric oxide (NO) in excess element-induced morphogenic responses of the root system. Plant Physiol. Biochem. 101, 149–161. doi: 10.1016/j.plaphy.2016.02.003
Kong, X., Wang, T., Li, W., Tang, W., Zhang, D., Dong, H. (2016). Exogenous nitric oxide delays salt-induced leaf senescence in cotton (Gossypium hirsutum l.). Acta Physiologiae Plantarum 38, 1–9. doi: 10.1007/s11738-016-2079-9
Koricheva, J., Gurevitch, J. (2014). Uses and misuses of meta-analysis in plant ecology. J. Ecol. 102, 828–844. doi: 10.1111/1365-2745.12224
Lajeunesse, M. J., Forbes, M. R. (2003). Variable reporting and quantitative reviews: a comparison of three meta-analytical techniques. Ecol. Lett. 6, 448–454. doi: 10.1046/j.1461-0248.2003.00448.x
Landi, M., Araniti, F., Flamini, G., Piccolo, E. L., Trivellini, A., Abenavoli, M. R., et al. (2020). “Help is in the air”: volatiles from salt-stressed plants increase the reproductive success of receivers under salinity. Planta 251, 1–15. doi: 10.1007/s00425-020-03344-y
Latef, A. A. A., Hasanuzzaman, M., Tahjib-Ul-Arif, M. (2021). Mitigation of salinity stress by exogenous application of cytokinin in faba bean (Vicia faba l.). Notulae Botanicae Horti Agrobotanici Cluj-Napoca 49, 12192–12192. doi: 10.15835/nbha49112192
Lau, J., Rothstein, H. R., Stewart, G. B. (2013). “History and progress of meta-analysis,” in Handbook of meta-analysis in ecology and evolution (New Jersey: Princeton University Press), 407–419.
Lehmann, A., Rillig, M. C. (2015). Arbuscular mycorrhizal contribution to copper, manganese and iron nutrient concentrations in crops–a meta-analysis. Soil Biol. Biochem. 81, 147–158. doi: 10.1016/j.soilbio.2014.11.013
Li, S., Jin, H., Zhang, Q. (2016). The effect of exogenous spermidine concentration on polyamine metabolism and salt tolerance in zoysiagrass (Zoysia japonica steud) subjected to short-term salinity stress. Front. Plant Sci. 7, 1221. doi: 10.3389/fpls.2016.01221
Liu, S., Dong, Y., Xu, L., Kong, J., Bai, X. (2013a). Roles of exogenous nitric oxide in regulating ionic equilibrium and moderating oxidative stress in cotton seedlings during salt stress. J. Soil Sci. Plant Nutr. 13, 929–941. doi: 10.4067/S0718-95162013005000073
Lu, S., Peng, X., Guo, Z., Zhang, G., Wang, Z., Wang, C., et al. (2007). In vitro selection of salinity tolerant variants from triploid bermudagrass (Cynodon transvaalensis× c. dactylon) and their physiological responses to salt and drought stress. Plant Cell Rep. 26, 1413–1420. doi: 10.1007/s00299-007-0339-2
Lu, S., Zhuo, C., Wang, X., Guo, Z. (2014). Nitrate reductase (NR)-dependent NO production mediates ABA-and H2O2-induced antioxidant enzymes. Plant Physiol. Biochem. 74, 9–15. doi: 10.1016/j.plaphy.2013.10.030
Manai, J., Kalai, T., Gouia, H., Corpas, F. (2014). Exogenous nitric oxide (NO) ameliorates salinity-induced oxidative stress in tomato (Solanum lycopersicum) plants. J. Soil Sci. Plant Nutr. 14, 433–446. doi: 10.4067/S0718-95162014005000034
Mayerhofer, M. S., Kernaghan, G., Harper, K. A. (2013). The effects of fungal root endophytes on plant growth: a meta-analysis. Mycorrhiza 23, 119–128. doi: 10.1007/s00572-012-0456-9
Mbarki, S., Sytar, O., Cerda, A., Zivcak, M., Rastogi, A., He, X., et al. (2018). “Strategies to mitigate the salt stress effects on photosynthetic apparatus and productivity of crop plants,” in Salinity responses and tolerance in plants, vol. Volume 1. (Cham: Springer), 85–136.
Mcgrath, J. M., Lobell, D. B. (2013). Reduction of transpiration and altered nutrient allocation contribute to nutrient decline of crops grown in elevated CO2 concentrations. Plant Cell Environ. 36, 697–705. doi: 10.1111/pce.12007
Misra, B. B., Acharya, B. R., Granot, D., Assmann, S. M., Chen, S. (2015). The guard cell metabolome: functions in stomatal movement and global food security. Front. Plant Sci. 6, 334. doi: 10.3389/fpls.2015.00334
Molassiotis, A., Tanou, G., Diamantidis, G. (2010). NO says more than ‘YES’to salt tolerance: salt priming and systemic nitric oxide signaling in plants. Plant Signaling Behav. 5, 209–212. doi: 10.4161/psb.5.3.10738
Monsur, M. B., Ivy, N. A., Haque, M. M., Hasanuzzaman, M., El Sabagh, A., Rohman, M. M. (2020). Oxidative stress tolerance mechanism in rice under salinity. Phyton 89 (3), 497. doi: 10.32604/phyton.2020.09144
Mostofa, M. G., Fujita, M., Tran, L.-S. P. (2015). Nitric oxide mediates hydrogen peroxide-and salicylic acid-induced salt tolerance in rice (Oryza sativa l.) seedlings. Plant Growth Regul. 77, 265–277. doi: 10.1007/s10725-015-0061-y
Munns, R., Tester, M. (2008). Mechanisms of salinity tolerance. Annu. Rev. Plant Biol. 59, 651–681. doi: 10.1146/annurev.arplant.59.032607.092911
Parankusam, S., Adimulam, S. S., Bhatnagar-Mathur, P., Sharma, K. K. (2017). Nitric oxide (NO) in plant heat stress tolerance: current knowledge and perspectives. Front. Plant Sci. 8, 1582. doi: 10.3389/fpls.2017.01582
Parihar, P., Singh, S., Singh, R., Singh, V. P., Prasad, S. M. (2015). Effect of salinity stress on plants and its tolerance strategies: a review. Environ. Sci. pollut. Res. 22, 4056–4075. doi: 10.1007/s11356-014-3739-1
Perveen, S., Shahbaz, M., Ashraf, M. (2011). Modulation in activities of antioxidant enzymes in salt stressed and non-stressed wheat (Triticum aestivum l.) plants raised from seed treated with triacontanol. Pak. J. Bot. 43, 2463–2468.
Perveen, S., Shahbaz, M., Ashraf, M. (2012). Changes in mineral composition, uptake and use efficiency of salt stressed wheat (Triticum aestivum l.) plants raised from seed treated with triacontanol. Pak. J. Bot. 44, 27–35.
Prakash, V., Singh, V. P., Tripathi, D. K., Sharma, S., Corpas, F. J. (2019). Crosstalk between nitric oxide (NO) and abscisic acid (ABA) signalling molecules in higher plants. Environ. Exp. Bot. 161, 41–49. doi: 10.1016/j.envexpbot.2018.10.033
Procházková, D., Haisel, D., Wilhelmová, N., Pavlíková, D., Száková, J. (2013). Effects of exogenous nitric oxide on photosynthesis. Photosynthetica 51, 483–489. doi: 10.1007/s11099-013-0053-y
Qiao, J., Kong, X., Kong, A., Han, M. (2014). Pharmacokinetics and biotransformation of tea polyphenols. Curr. Drug Metab. 15, 30–36. doi: 10.2174/1389200214666131229111336
Rahman, M., Habib, M., Sikdar, M., Md, S., Islam, M. (2016). Evaluation of mungbean genotypes for salt tolerance at seedling stage and alleviation of saline stress by gypsum. Fundam. Appl. Agric. 1, 39–43.
Rehman, S., Abbas, G., Shahid, M., Saqib, M., Farooq, A. B. U., Hussain, M., et al. (2019). Effect of salinity on cadmium tolerance, ionic homeostasis and oxidative stress responses in conocarpus exposed to cadmium stress: Implications for phytoremediation. Ecotoxicology Environ. Saf. 171, 146–153. doi: 10.1016/j.ecoenv.2018.12.077
Rejeb, K. B., Abdelly, C., Savouré, A. (2014). How reactive oxygen species and proline face stress together. Plant Physiol. Biochem. 80, 278–284. doi: 10.1016/j.plaphy.2014.04.007
Ren, Y., Wang, W., He, J., Zhang, L., Wei, Y., Yang, M. (2020). Nitric oxide alleviates salt stress in seed germination and early seedling growth of pakchoi (Brassica chinensis l.) by enhancing physiological and biochemical parameters. Ecotoxicology Environ. Saf. 187, 109785. doi: 10.1016/j.ecoenv.2019.109785
Roy, P. R., Tahjib-Ul-Arif, M., Akter, T., Ray, S. R., Sayed, M. A. (2016). Exogenous ascorbic acid and hydrogen peroxide alleviates salt-induced oxidative stress in rice (Oryza sativa l.) by enhancing antioxidant enzyme activities and proline content. Adv. Environ. Biol. 10, 148–155.
Rubio Luna, M. C., Bustos-Sanmamed, P., Clemente, M. R., Becana Ausejo, M. (2009). Effects of salt stress on the expression of antioxidant genes and proteins in the model legume lotus japonicus. New Phytol. 181, 851–859. doi: 10.1111/j.1469-8137.2008.02718.x
Santos, M. P., Zandonadi, D. B., de Sá, A. F. L., Costa, E. P., de Oliveira, C. J. L., Perez, L. E., et al. (2020). Abscisic acid-nitric oxide and auxin interaction modulates salt stress response in tomato roots. Theor. Exp. Plant Physiol. 32, 301–313. doi: 10.1007/s40626-020-00187-6
Schmidt, F. L., Hunter, J. E. (2015). “Meta-analysis methods for d values,” in Methods of meta-analysis: Correcting error and bias in research findings, 3rd ed (London, England: Sage), 279–342.
Sehar, Z., Masood, A., Khan, N. A. (2019). Nitric oxide reverses glucose-mediated photosynthetic repression in wheat (Triticum aestivum l.) under salt stress. Environ. Exp. Bot. 161, 277–289. doi: 10.1016/j.envexpbot.2019.01.010
Shabala, S. (2013). Learning from halophytes: physiological basis and strategies to improve abiotic stress tolerance in crops. Ann. Bot. 112, 1209–1221. doi: 10.1093/aob/mct205
Shafi, A., Chauhan, R., Gill, T., Swarnkar, M. K., Sreenivasulu, Y., Kumar, S., et al. (2015). Expression of SOD and APX genes positively regulates secondary cell wall biosynthesis and promotes plant growth and yield in arabidopsis under salt stress. Plant Mol. Biol. 87, 615–631. doi: 10.1007/s11103-015-0301-6
Shams, M., Ekinci, M., Ors, S., Turan, M., Agar, G., Kul, R., et al. (2019). Nitric oxide mitigates salt stress effects of pepper seedlings by altering nutrient uptake, enzyme activity and osmolyte accumulation. Physiol. Mol. Biol. Plants 25, 1149–1161. doi: 10.1007/s12298-019-00692-2
Shams, M., Yildirim, E., Ekinci, M., Turan, M., Dursun, A., Parlakova, F., et al. (2016). Exogenously applied glycine betaine regulates some chemical characteristics and antioxidative defence systems in lettuce under salt stress. Horticult. Environment Biotechnol. 57, 225–231. doi: 10.1007/s13580-016-0021-0
Sharma, A., Kapoor, D., Wang, J., Landi, M., Zheng, B., Yan, D., et al. (2020). Nitric oxide mediated mechanisms adopted by plants to cope with salinity. Biol. plantarum 64, 512–518. doi: 10.32615/bp.2020.070
Shen, Z., Chen, J., Ghoto, K., Hu, W., Gao, G., Luo, M., et al. (2018). Proteomic analysis on mangrove plant avicennia marina leaves reveals nitric oxide enhances the salt tolerance by up-regulating photosynthetic and energy metabolic protein expression. Tree Physiol. 38, 1605–1622. doi: 10.1093/treephys/tpy058
Shi, Q., Ding, F., Wang, X., Wei, M. (2007). Exogenous nitric oxide protect cucumber roots against oxidative stress induced by salt stress. Plant Physiol. Biochem. 45, 542–550. doi: 10.1016/j.plaphy.2007.05.005
Siddiqui, M. H., Alamri, S. A., Al-Khaishany, M. Y., Al-Qutami, M. A., Ali, H. M., AL-Rabiah, H., et al. (2017). Exogenous application of nitric oxide and spermidine reduces the negative effects of salt stress on tomato. Horticult. Environment Biotechnol. 58, 537–547. doi: 10.1007/s13580-017-0353-4
Sidik, K., Jonkman, J. N. (2005). Simple heterogeneity variance estimation for meta-analysis. J. R. Stat. Society: Ser. C (Applied Statistics) 54, 367–384. doi: 10.1111/j.1467-9876.2005.00489.x
Sidik, K., Jonkman, J. N. (2007). A comparison of heterogeneity variance estimators in combining results of studies. Stat Med. 26, 1964–1981. doi: 10.1002/sim.2688
Signorelli, S., Considine, M. J. (2018). Nitric oxide enables germination by a four-pronged attack on ABA-induced seed dormancy. Front. Plant Sci. 9, 296. doi: 10.3389/fpls.2018.00296
Simonsohn, U., Nelson, L. D., Simmons, J. P. (2014). P-curve: a key to the file-drawer. J. Exp. psychology: Gen. 143, 534. doi: 10.1037/a0033242
Singhal, R. K., Saha, D., Skalicky, M., Mishra, U. N., Chauhan, J., Behera, L. P., et al. (2021). Crucial cell signaling compounds crosstalk and integrative multi-omics techniques for salinity stress tolerance in plants. Front. Plant Sci. 1227. doi: 10.3389/fpls.2021.670369
Sohag, A. A. M., Tahjib-Ul-Arif, M., Afrin, S., Khan, M. K., Hannan, M. A., Skalicky, M., et al. (2020). Insights into nitric oxide-mediated water balance, antioxidant defence and mineral homeostasis in rice (Oryza sativa l.) under chilling stress. Nitric. Oxide 100, 7–16. doi: 10.1016/j.niox.2020.04.001
Sotiras, M., Papadakis, I., Landi, M., Tsaniklidis, G., Tsiantas, P., Psychoyou, M. (2019). Allocation pattern, photosynthetic performance and sugar metabolism in hydroponically grown seedlings of loquat (Eriobotrya japonica lindl.) subjected to salinity. Photosynthetica 57, 258–267. doi: 10.32615/ps.2019.018
Tabassum, R., Tahjib-Ul-Arif, M., Hasanuzzaman, M., Sohag, A. A. M., Islam, M. S., Shafi, S. S. H., et al. (2021). Screening salt-tolerant rice at the seedling and reproductive stages: an effective and reliable approach. Environ. Exp. Bot. 192, 104629. doi: 10.1016/j.envexpbot.2021.104629
Tahjib-Ul-Arif, M., Afrin, S., Polash, M. A. S., Akter, T., Ray, S. R., Hossain, M., et al. (2019). Role of exogenous signaling molecules in alleviating salt-induced oxidative stress in rice (Oryza sativa l.): A comparative study. Acta Physiologiae Plantarum 41, 1–14. doi: 10.1007/s11738-019-2861-6
Tahjib-Ul-Arif, M., Siddiqui, M. N., Sohag, A. A. M., Sakil, M. A., Rahman, M. M., Polash, M. A. S., et al. (2018). Salicylic acid-mediated enhancement of photosynthesis attributes and antioxidant capacity contributes to yield improvement of maize plants under salt stress. J. Plant Growth Regul. 37, 1318–1330. doi: 10.1007/s00344-018-9867-y
Teixeira, J., Pereira, S. (2007). High salinity and drought act on an organ-dependent manner on potato glutamine synthetase expression and accumulation. Environ. Exp. Bot. 60, 121–126. doi: 10.1016/j.envexpbot.2006.09.003
Tester, M., Langridge, P. (2010). Breeding technologies to increase crop production in a changing world. Science 327, 818–822. doi: 10.1126/science.1183700
Tian, X., He, M., Wang, Z., Zhang, J., Song, Y., He, Z., et al. (2015). Application of nitric oxide and calcium nitrate enhances tolerance of wheat seedlings to salt stress. Plant Growth Regul. 77, 343–356. doi: 10.1007/s10725-015-0069-3
Van Kleunen, M., Dawson, W., Schlaepfer, D., Jeschke, J. M., Fischer, M. (2010). Are invaders different? a conceptual framework of comparative approaches for assessing determinants of invasiveness. Ecol. Lett. 13, 947–958. doi: 10.1111/j.1461-0248.2010.01503.x
Van Zelm, E., Zhang, Y., Testerink, C. (2020). Salt tolerance mechanisms of plants. Annu. Rev. Plant Biol. 71, 403–433. doi: 10.1146/annurev-arplant-050718-100005
Veresoglou, S. D., Menexes, G., Rillig, M. C. (2012). Do arbuscular mycorrhizal fungi affect the allometric partition of host plant biomass to shoots and roots? a meta-analysis of studies from 1990 to 2010. Mycorrhiza 22, 227–235. doi: 10.1007/s00572-011-0398-7
Wang, Q., Liang, X., Dong, Y., Xu, L., Zhang, X., Hou, J., et al. (2013). Effects of exogenous nitric oxide on cadmium toxicity, element contents and antioxidative system in perennial ryegrass. Plant Growth Regul. 69, 11–20. doi: 10.1007/s10725-012-9742-y
Wu, X., Zhu, W., Zhang, H., Ding, H., Zhang, H. J. (2011a). Exogenous nitric oxide protects against salt-induced oxidative stress in the leaves from two genotypes of tomato (Lycopersicom esculentum mill.). Acta physiologiae plantarum 33, 1199–1209. doi: 10.1007/s11738-010-0648-x
Xie, C., Xiong, X., Huang, Z., Sun, L., Ma, J., Cai, S., et al. (2018). Exogenous melatonin improves lead tolerance of bermudagrass through modulation of the antioxidant defense system. Int. J. phytoremediation 20, 1408–1417. doi: 10.1080/15226514.2018.1488813
Xu, Y., Sun, X., Jin, J., Zhou, H. (2010). Protective effect of nitric oxide on light-induced oxidative damage in leaves of tall fescue. J. Plant Physiol. 167, 512–518. doi: 10.1016/j.jplph.2009.10.010
Yamane, K., Mitsuya, S., Taniguchi, M., Miyake, H. (2010). Transcription profiles of genes encoding catalase and ascorbate peroxidase in the rice leaf tissues under salinity. Plant Production Sci. 13, 164–168. doi: 10.1626/pps.13.164
Yildirim, E., Karlidag, H., Turan, M. (2009). Mitigation of salt stress in strawberry by foliar K, Ca and mg nutrient supply. Plant Soil Environ. 55, 213–221. doi: 10.17221/383-PSE
Zhang, L. P., Mehta, S. K., Liu, Z. P., Yang, Z. M. (2008). Copper-induced proline synthesis is associated with nitric oxide generation in chlamydomonas reinhardtii. Plant Cell Physiol. 49, 411–419. doi: 10.1093/pcp/pcn017
Zhang, Y., Wang, L., Liu, Y., Zhang, Q., Wei, Q., Zhang, W. (2006). Nitric oxide enhances salt tolerance in maize seedlings through increasing activities of proton-pump and Na+/H+ antiport in the tonoplast. Planta 224, 545–555. doi: 10.1007/s00425-006-0242-z
Zhang, L., Wang, Y., Zhao, L., Shi, S., Lixin, Z. (2006). Involvement of nitric oxide in light-mediated greening of barley seedlings. J. Plant Physiol. 163, 818–826. doi: 10.1016/j.jplph.2005.07.011
Zhang, X., Yin, H., Chen, S., He, J., Guo, S. (2014). Changes in antioxidant enzyme activity and transcript levels of related genes in limonium sinense kuntze seedlings under NaCl stress. J. Chem. 2014, 749047. doi: 10.1155/2014/749047
Zhao, L., Zhang, F., Guo, J., Yang, Y., Li, B., Zhang, L. (2004). Nitric oxide functions as a signal in salt resistance in the calluses from two ecotypes of reed. Plant Physiol. 134, 849–857. doi: 10.1104/pp.103.030023
Zheng, C., Jiang, D., Liu, F., Dai, T., Liu, W., Jing, Q., et al. (2009). Exogenous nitric oxide improves seed germination in wheat against mitochondrial oxidative damage induced by high salinity. Environ. Exp. Bot. 67, 222–227. doi: 10.1016/j.envexpbot.2009.05.002
Keywords: abiotic stress, antioxidants, NO, photosynthesis, plant growth, oxidative stress, salt stress
Citation: Tahjib-Ul-Arif M, Wei X, Jahan I, Hasanuzzaman M, Sabuj ZH, Zulfiqar F, Chen J, Iqbal R, Dastogeer KMG, Sohag AAM, Tonny SH, Hamid I, Al-Ashkar I, Mirzapour M, El Sabagh A and Murata Y (2022) Exogenous nitric oxide promotes salinity tolerance in plants: A meta-analysis. Front. Plant Sci. 13:957735. doi: 10.3389/fpls.2022.957735
Received: 31 May 2022; Accepted: 23 September 2022;
Published: 07 November 2022.
Edited by:
Loredana F. Ciarmiello, University of Campania Luigi Vanvitelli, ItalyReviewed by:
Mostafa M. Rady, Fayoum University, EgyptZhiyong Zhang, Henan Institute of Science and Technology, China
Copyright © 2022 Tahjib-Ul-Arif, Wei, Jahan, Hasanuzzaman, Sabuj, Zulfiqar, Chen, Iqbal, Dastogeer, Sohag, Tonny, Hamid, Al-Ashkar, Mirzapour, El Sabagh and Murata. This is an open-access article distributed under the terms of the Creative Commons Attribution License (CC BY). The use, distribution or reproduction in other forums is permitted, provided the original author(s) and the copyright owner(s) are credited and that the original publication in this journal is cited, in accordance with accepted academic practice. No use, distribution or reproduction is permitted which does not comply with these terms.
*Correspondence: Md. Tahjib-Ul-Arif, dGFoamliQGJhdS5lZHUuYmQ=; Xiangying Wei, eGlhbmd5aW5nd2VpQG1qdS5lZHUuY24=; Ayman El Sabagh, YXltYW4uZWxzYWJhZ2hAYWdyLmtmcy5lZHUuZWc=
†Present address: Md. Tahjib-Ul-Arif, Graduate School of Environmental and Life Science, Okayama University, Okayama, Japan Khondoker M. G. Dastogeer, Department of Environmental Science, Policy, and Management, University of California Berkeley, Berkeley, CA, United States
‡These authors have contributed equally to this work