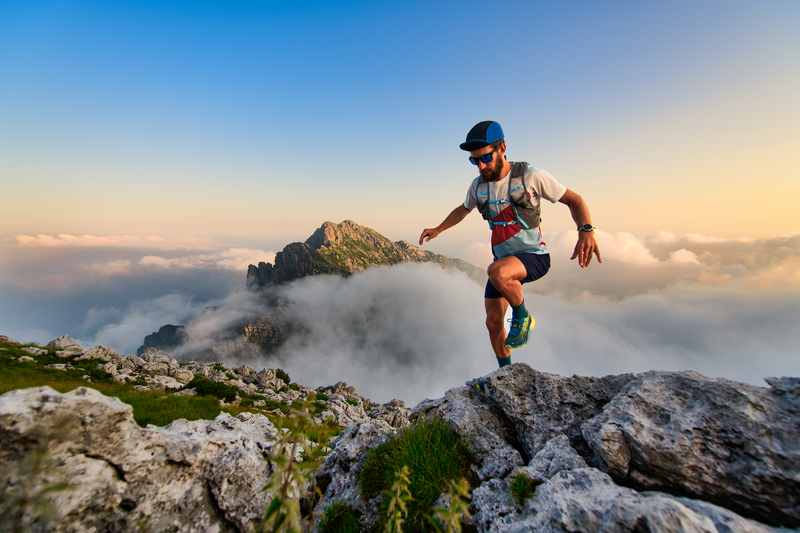
95% of researchers rate our articles as excellent or good
Learn more about the work of our research integrity team to safeguard the quality of each article we publish.
Find out more
REVIEW article
Front. Plant Sci. , 24 August 2022
Sec. Plant Development and EvoDevo
Volume 13 - 2022 | https://doi.org/10.3389/fpls.2022.951844
This article is part of the Research Topic Women in Plant Development and EvoDevo: 2022 View all 10 articles
Chestnuts are multipurpose trees significant for the economy and wildlife. These trees are currently found around the globe, demonstrating their genetic adaptation to different environmental conditions. Several biotic and abiotic stresses have challenged these species, contributing to the decline of European chestnut production and the functional extinction of the American chestnut. Several efforts started over the last century to understand the cellular, molecular, and genetic interactions behind all chestnut biotic and abiotic interactions. Most efforts have been toward breeding for the primary diseases, chestnut blight and ink disease caused by the pathogens, Cryphonectria parasitica and Phytophthora cinnamomi, respectively. In Europe and North America, researchers have been using the Asian chestnut species, which co-evolved with the pathogens, to introgress resistance genes into the susceptible species. Breeding woody trees has several limitations which can be mostly related to the long life cycles of these species and the big genome landscapes. Consequently, it takes decades to improve traits of interest, such as resistance to pathogens. Currently, the availability of genome sequences and next-generation sequencing techniques may provide new tools to help overcome most of the problems tree breeding is still facing. This review summarizes European and American chestnut’s main biotic stresses and discusses breeding and biotechnological efforts developed over the last decades, having ink disease and chestnut blight as the main focus. Climate change is a rising concern, and in this context, the adaptation of chestnuts to adverse environmental conditions is of extreme importance for chestnut production. Therefore, we also discuss the abiotic challenges on European chestnuts, where the response to abiotic stress at the genetic and molecular level has been explored.
The genus Castanea belongs to the Fagaceae family, and it is constituted of three sections: Eucastanon (chestnuts), Balanocastanon (chinquapins), and Hypocastanon (the Henry chestnut). The most representative species and of greater economic importance are included in Eucastanon: European chestnut (Castanea sativa Mill.), the American chestnut [Castanea dentata (Marshall) Borkh.], the Chinese chestnut (Castanea mollissima Blume), and the Japanese chestnut (Castanea crenata Sieb. and Zucc.) (Vieitez and Merkle, 2005; Mellano et al., 2012). Recently, researchers may have discovered a new chestnut species. Castanea alabamensis, was considered a hybrid between Castanea dentata and Castanea pumila Mill. (Allegheny chinquapin), but it was identified as a distinct genetic and morphological group in North America (Perkins et al., 2021).
Chestnuts originated in eastern Asia (Japan and China), from where they dispersed and diverge through Europe and North America (Lang et al., 2007). Nowadays, they are found across the northern hemisphere. Castanea sativa is distributed in temperate and Mediterranean regions of Europe and Western Asia; Castanea dentata’s natural range is across the Appalachian Mountain region; Castanea mollissima is native to China; and Castanea crenata is distributed in the Korean Peninsula, Japan, and the temperate region of East Asia (Pereira-Lorenzo et al., 2012).
In Asia and Europe, chestnuts have been cultivated for decades and have great economical value for their nutritious nuts and quality timber. In North America, they were treasured for being a multipurpose key-stone forest tree very important for populations and wildlife in its natural range (Anagnostakis, 1987). In 2020, the total chestnut plantation area in the world was approximately 582,545 ha, and more than half of this area belongs to China. This translated into an annual world production of approximately 2,300,000 tons, where China is the leading producer with almost 1,750,000 tons, followed by Spain with 189,000 tons (Food and Agriculture Organization of the United Nations, 2022).
The American and European chestnuts are the most susceptible species to several stresses, mainly biotic. The once dominant American chestnut was decimated in the 20th century (Anagnostakis, 1987), while in Europe C. sativa was chastised and nut production declined 251,549 tons from 1961 to 2015 (Food and Agriculture Organization of the United Nations, 2022). Biotic and abiotic stresses in forest systems have not been as well studied at a genomic level as it has for herbaceous crops. The recent technological advances in molecular biology and next-generation sequencing (NGS) technologies (Grabherr et al., 2011) helped overcome several difficulties inherent to studying woody species such as chestnuts. The transcriptome is modifiable under different conditions, making it a great tool to explore stress response. Association mapping and genome-wide association studies (GWASs) allow the association between molecular markers and phenotype in complex populations (Badenes et al., 2016), which can help understand the genetic architecture of stresses. The genomic resources gathered in the last decades have increased our knowledge about Castanea spp. genetic diversity, phenology, adaptation, and interaction with biotic and abiotic stresses. Transcriptomes during stress response (Barakat et al., 2009, 2012; Santamaría et al., 2011; Serrazina et al., 2015), development of molecular markers (Martin et al., 2010; Pereira-lorenzo et al., 2010, Pereira-Lorenzo et al., 2011; Nishio et al., 2011; Kubisiak et al., 2013; Santos et al., 2015b), mapping and identification of Quantitative Trait Loci (QTL) (Kubisiak et al., 1997; Casasoli et al., 2004, 2006; Zhebentyayeva et al., 2014, 2019; Santos et al., 2017b) have set us a step closer to using genomic selection in breeding programs. Biotechnologies implemented in chestnuts also include large-scale micropropagation of improved genotypes and genetic transformation. Several in vitro culture techniques such as axillary shoot propagation, organogenesis, and somatic embryogenesis have been reported over the last decades (reviewed in Corredoira et al., 2017) and recent advances are still being published (Fernandes et al., 2020b; Liu et al., 2022). Genetic transformation can be a powerful tool to study the function of a gene or for crop improvement and has been an important milestone in chestnut research (Powell et al., 2019; Pavese et al., 2021a).
The research and breeding efforts made in the last decades seem to be having a positive impact since, in Europe, chestnut production has been increasing since 2015 for the first time in many decades (Food and Agriculture Organization of the United Nations, 2022). The purpose of this review is to provide a synopsis of the understanding gathered so far about chestnuts’ main biotic and abiotic challenges. The section on biotic stresses will have ink disease and chestnut blight as the main focus, while the abiotic section will mainly focus on Southern Europe, where there is a majority of reports. The development and application of biotechnologies are also discussed as they relate to the efforts of fighting European chestnut’s decline and revive the American species. Figure 1 briefly summarizes the main topics discussed in this review.
Figure 1. Schematic overview of the main topics discussed in this review. Information includes disease/stress, causal agent, and efforts for control/mitigation, in process or in perspective.
Castanea species are challenged by several biotic stresses but the most destructive are Cryphonectria parasitica (Murr.) Barr. (CP) and Phytophthora cinnamomi Rands (PC), the pathogens causing chestnut blight and ink disease (also known as chestnut canker and root rot, respectively). Leaf spot (Marssonina ochroleuca), twig canker (Cryptodiaporthe castanea), and chestnut mosaic virus (ChMV) also affect chestnuts, however, the damage they cause is not as severe as the previously mentioned diseases (Serdar et al., 2019).
CP is a necrotrophic bark-inhabiting fungus whose primary hosts are C. dentata, C. sativa, C. crenata, and C. mollissima, although it also infects oaks (Quercus spp.), maples (Acer spp.), European hornbeam (Carpinus betulus) and chinquapins (C. pumila and Castanea ozarkensis). It is native to Eastern Asia, and it spread to North America and Europe due to imported infected chestnuts (Rigling and Prospero, 2018). CP was first described in 1904 in American chestnut in New York (Anagnostakis, 1987; Rigling and Prospero, 2018). In 50 years, it caused one of the most enormous economic and ecological devastations, leaving the American chestnut functionally extinct by killing an estimated 4 billion trees (Anagnostakis, 1987). This species now survives as stump sprouts (due to repeated blight infections) which are reservoirs of germplasm (Kubisiak and Roberds, 2006). CP was detected in Europe for the first time in 1938 in Italy, from where it rapidly spread to the rest of the continent to countries such as France, Switzerland, Portugal, Spain, and Turkey (Rigling and Prospero, 2018). It threatened the European chestnut stands, affecting production. Still, C. sativa recovered from the disease. This recovery was mainly related to the natural occurrence of mycoviruses in Europe that can infect this fungus and attenuate its virulence (hypovirulence, a viral disease that makes the pathogen less aggressive) (Robin and Heiniger, 2001; Milgroom and Cortesi, 2004). Also, C. sativa has lower susceptibility to the pathogen when compared to C. dentata (Waldboth and Oberhuber, 2009). Nowadays, CP is spread across Europe, North America, Africa (Tunisia), Asia and Australia (Eppo Global Database, 2022).
PC is a devastating hemibiotrophic pathogen with an extensive host range of close to 5,000 plant species (Hardham and Blackman, 2018). It has significant environmental and economic impacts (Weste and Marks, 1987; Hardham, 2005; Kamoun et al., 2015) by infecting plants important for forestry, and agriculture, such as chestnut, avocado, macadamia, oak, peach and pineapple [reviewed in Hardham and Blackman (2018)], causing annual damages of billions of dollars. PC is considered one of the Top 10 Oomycete plant pathogens (Kamoun et al., 2015). Its origin remains uncertain, but evidence indicates it originated near Papua New Guinea and South-East Asian regions (Ko et al., 1978; Zentmyer, 1988; Hardham, 2005). Ink disease was observed in American chestnuts and chinquapins in the southern United States since about 1850 [Milburn and Gravatt, 1932 as cited in Anagnostakis (2012)] and in Portugal since 1853 [Prunet, 1904 as cited in Anagnostakis (2012)]. But the first reports on this disease being caused by PC were a few years later. In European chestnuts, it was in 1860 [Grent, 1961 as cited in Burgess et al. (2017)], and in American chestnuts in 1986 [Corsa, 1986 as cited in Anagnostakis (2001)]. PC was introduced in all continents by plant-moving, except for Antarctica, becoming invasive worldwide (Eppo Global Database, 2022).
Castanea species have different susceptibility levels to these pathogens. The Asian species are the most resistant, probably due to their co-evolution with the pathogens (Crandall et al., 1945; Huang et al., 1996). In the specific case of chestnut blight, varying levels of quantitative resistance have been reported for Asian species, however, Chinese chestnut is considered more blight-resistant than Japanese chestnut (Huang et al., 1996).
Breeding for pathogen resistance in Europe and North America has different goals. The first focus on development and preservation of cultivars and ink disease-resistant rootstocks to reduce mortality, improve orchard production, and avoid further decline of the species; the latter seeks to restore the American chestnut as a forest species. What is common in these efforts is the use of the Asian species resistance and the high interspecies crossability, to introgress resistance genes into the susceptible European and American species (Burnham et al., 1986; Fernández-López et al., 2001; Pereira-lorenzo et al., 2010; Costa et al., 2011; Steiner et al., 2017). In Europe, blight infection has been under control due to hypovirulence and consequently, most research is focused on understanding the interaction with PC, mainly in C. sativa and C. crenata. Contrary to North America, where dedication goes to C. dentata and C. mollissima responses to CP. Nevertheless, in recent years North Americans realized that PC will be a problem to the cultivation of the American chestnut with improved blight resistance, mainly in the south where temperatures allow ink disease establishment (Jeffers et al., 2009).
During the last decades, chestnut breeding programs have been looking into Castanea spp. cellular and molecular responses to CP and PC, which may be comparable since Oomycetes and Fungi share similar infection mechanisms (Latijnhouwers et al., 2003). Several hypotheses have been proposed so far and are discussed in the next sections.
Biotic stresses also include several pests such as the gall wasp [Dryocosmus kuriphilus (Yasumatsu)], chestnut tortrix moths (Cydia splendana, Cydia fagiglandana, and Pammene fasciana), the chestnut weevil (Curculio elephas) and ambrosia beetles [Anisandrus (Xyleborus) dispar]. Less damaging pests currently affecting chestnuts are the potato leafhopper (Empoasca fabae), Japanese beetle (Popillia japonica), Rose chafer (Macrodactylus subspinosus) and spider mites. Also, Peach moth (Dichocrocis punctiferalis) and goat moth (Cossus cossus) were reported as important pests in Japan and Turkey, respectively [reviewed in Serdar et al. (2019)]. The gall wasp is the most globally significant pest. It attacks European, American, and Asian chestnut species, and their hybrids, reducing the quality and quantity of timber (Kato and Hijii, 1997) and nuts (Battisti et al., 2014). The gall wasp does not kill the trees and for that, it has been given less attention than the previously referred pathogens. However, its spread may erase all the breeding efforts toward European and American chestnut sustainability due to the reduced nut production. Also, galls can be an entry point for CP increasing branch mortality (Meyer et al., 2015). The next sections provide an overview of our current knowledge of the chestnut’s interaction with these three biotic stresses, with a focus on the pathogens.
The histopathology of CP infection progress is described in American and Chinese chestnuts (Hebard et al., 1984). After spore germination, the fungus develops mycelial fans, which, by physical pressure, colonize the host cells progressing intercellularly through the bark and cambium. The extent and frequency of mycelial fan formation are essential for the enlargement of cankers. The host responses against the infection are lignification of cell walls succeeded by wound periderm formation. Lignification only blocks individual or small aggregates of hyphae, and only fully formed wound periderm can stop mycelial fans (Hebard et al., 1984). The advance of the mycelial fans kills the host cells by releasing toxins and cell wall-degrading enzymes (Roane et al., 1986) and when the host does not develop deep wound periderms allows the pathogen to keep obtaining nutrients from dying and dead chestnut cells (necrotrophy) (Hebard et al., 1984). Oxalate (oxalic acid) was considered linked with CP virulence when researchers suggested it had a toxic effect on host cells and enhanced cell wall degradation (Havir and Anagnostakis, 1983). This was later confirmed in knockout mutants of the pathogen (Chen et al., 2010).
CP only infects above-ground tree parts, producing orange/reddish-brown cankers (necrotic lesions) on the bark (Figure 2) and killing smaller branches. An early symptom of infection is the wilted and hanging leaves on infected dead branches. Trees react by producing numerous epicormic shoots below the cankers (Rigling and Prospero, 2018). Blight-resistant chestnuts typically survive infection with minimal internal damage, developing superficial lesions or cankers on the trunk. In blight-susceptible species, disease symptoms usually progress rapidly, resulting in host mortality. Also, symptoms in susceptible hosts may vary depending on the virulence of the fungus, tree size, longevity, health, and environmental factors (Roane et al., 1986; Clark et al., 2019). CP can survive and sporulate on the bark of dead or recently dead chestnut branches or stems for more than 1 year (Prospero et al., 2006).
Figure 2. Castanea dentata infected with Cryphonectria parasitica presenting an orange canker on the main trunk. This picture was taken at the Lafayette Experimental Road Station – SUNY-ESF (Syracuse, NY, United States).
Quarantine regulations were implemented worldwide to control the movement and trade of plant material infected with blight. Unfortunately, these measures were ineffective due to asymptomatic infected plants (Rigling and Prospero, 2018). In natural ecosystems, attempts to eradicate the pathogen or apply fungicides are not feasible. Cutting and burning infected trees is a management alternative, but only viable in orchards. The use of chemicals is restricted in forests because it can cause phytotoxicity, and may induce resistance.
Efforts to develop control methods for CP are summarized in Table 1. In Europe, the disease is successfully managed due to the sizeable natural occurrence of hypovirulence, a viral disease in CP population caused by double-stranded RNA viruses which reduces virulence and sporulation of strains. Contrary to North America where hypovirulence was only found outside the American chestnut range and there is limited success in viral transfer between different vegetatively incompatible fungus strains (Milgroom and Cortesi, 2004). Recent reports described a modification using genetically engineered “super donor” fungal strains that may help overcome these difficulties (Stauder et al., 2019).
Due to the hypovirulence success in Europe most efforts to manage blight are focused on the study of hypovirulent strains of CP (Robin and Heiniger, 2001; Bryner et al., 2012; Murolo et al., 2018). North America is focused in developing a blight-resistant chestnut through traditional backcross breeding and genetic engineering. For this reason, the following sections on CP will be mainly focused on North American reports.
American chestnut breeding for blight resistance has been ongoing for over 100 years. Inter-species crosses with Asian chestnut species (mainly Chinese) were started by the United States Department of Agriculture (USDA) and the Connecticut Agricultural Experiment Station (CAES) [reviewed in Jacobs et al. (2013)]. However, these programs failed to produce a blight-resistant tree that retained American chestnuts’ growth and quality timber. Besides the difficulties in finding the ideal candidate tree, nowadays we know there are other problems inherent to hybrid breeding that can turn restoration even more difficult such as male sterility (Sisco et al., 2014), internal kernel breakdown (IKB) (Fulbright et al., 2014) and intermediate traits (Cipollini et al., 2017).
In 1989 The American Chestnut Foundation (TACF) backcross breeding started at the Meadowview station by using two backcross hybrids (BC) [BC1 (C. dentata × C. mollissima) × C. dentata] from the USDA and CAES programs as two different sources of blight resistance: the ‘Clapper’ and ‘Graves’ (from C. mollissima variety ‘Nanking’ and ‘Mahogany,’ respectively). To achieve a population with Chinese chestnut resistance and the American chestnut phenotype, the original breeding plan (Burnham et al., 1986) proposed successive hybrid backcrossing with several pure American chestnut lines (to ensure genetic diversity), selecting for blight resistance and American phenotype in every progeny. This plan was based on the assumption that the alleles for resistance were partially dominant and only two genes were involved. Nowadays, we know that chestnut blight resistance is quantitative, involving three main resistance loci (of up to seven in total) (Kubisiak et al., 1997, 2013), which changed the backcross breeding stages to include three backcross generations. The third backcross would be intercrossed to produce a BC3F2 population in which a fraction of the trees was predicted to be homozygous for the Asian resistance alleles. The selected resistant individuals would be planted in seed orchards producing a BC3F3 generation that would be essentially American chestnut morphologically and blight-resistant enough to start restoration [reviewed in Jacobs et al. (2013)]. Indeed, the American phenotype was recovered in 96% of the BC3 generation, which resembled 24 measured traits (Diskin et al., 2006). Approximately 64,000 BC3F2 from both ‘Clapper’ and ‘Graves’ selections were planted between 2002 and 2018, and 7,600 trees remained as of 2018 (Westbrook et al., 2020b). Orchard trials of open-pollinated BC3F3 were made to estimate the genetic resistance of the selected BC3F2 trees, but after inoculations, the highest blight tolerance was more like American chestnut than Chinese chestnut. These findings suggested that blight resistance segregates at more loci than initially predicted and phenotypic selection has not been accurate enough (Steiner et al., 2017). This differs from 8-year-old BC3F3 forest reintroduction trials made in three locations in the southeastern United States, where the resistance of the trees was more like Chinese chestnut (Clark et al., 2019). TACF has ongoing field trials in 35 locations in the eastern United States but are still too young to assess blight resistance (Westbrook et al., 2020b). Nowadays, additional C. mollissima genotypes are being used as resistance sources (Steiner et al., 2017) at the Meadowview breeding station. This program has also been reproduced at 16 other locations by the different chapters of the foundation. After decades of breeding, the current goal is to select 1% of the 7,600 BC3F2 that are most blight-resistant, intercross the selected trees and increase BC3F3 blight resistance (Westbrook et al., 2020b). Also, selected blight-resistant hybrids are being evaluated for resistance to PC (Jeffers et al., 2009).
Traditional screening for blight resistance can be determined by field inoculation of the stems/trunks (Griffin, 1983; Anagnostakis, 1992). These inoculations usually allow the evaluation of parameters such as mean canker length and width (canker expansion rate), stromata production, canker superficiality and swelling, and canker severity (Kubisiak et al., 1997; Steiner et al., 2017; Westbrook et al., 2020b). These methods are accurate but can only be performed in trees with at least 3 years of growth and cankers can take several months to develop. Alternative techniques can test younger plants (Powell et al., 2007; Newhouse et al., 2014). CP lesion progression can be accessed by small stem inoculations in trees with approximately 1-year-old and results can be collected in 3–4 weeks (Powell et al., 2007). However, this may harm the tree even if it has moderate levels of resistance. Alternatively, excised leaves from a few month-old seedlings can be inoculated (Newhouse et al., 2014). Leaves are not CP’s primary organ of infection. Nevertheless, results can be obtained in less than a week and correlate to stem inoculations (Newhouse et al., 2014), representing an expedited way to predict levels of blight resistance in big populations.
Kubisiak et al. (1997) developed a genetic linkage map with F2 hybrids from the backcross breeding program, mapping 184 molecular markers. In three different linkage groups (LG) seven QTLs related to blight resistance were reported. Three major QTLs explained about 40% of the phenotypic variation in canker size. This map was later expanded by Sisco et al. (2005) and Kubisiak et al. (2013) and the three major QTLs identified were sequenced (Table 1; Staton et al., 2015). Of 782 annotated genes, 15 were related to defense response, giving further insight about the candidate resistance genes (Staton et al., 2015). Barakat et al. (2009; 2012) also identified candidate genes for blight resistance by comparing American and Chinese chestnuts transcriptomes after CP challenge. The candidate genes were associated with response to biotic stimuli belonging to several pathways (Table 1; Barakat et al., 2012).
Westbrook et al. (2020b) recently suggested that blight resistance is a polygenic inherited trait. The population under study was the BC3F2 generation (mentioned in Section “Improving characterization of Cryphonectria parasitica resistance”). Genotyping-by-sequencing (GBS) and evaluation of different blight phenotypes in the BC3F2 population, along with canker severity assessment of their progeny (BC3F3), allowed the development of a genomic prediction model for blight resistance breeding (Table 1). They also performed GBS on C. dentata and C. mollissima to estimate hybrid indices.
The PC can saprophytically grow in the soil, and when conditions are favorable (high soil moisture and temperatures above 10°C) to sporulate it produces biflagellate motile zoospores (asexual spores) which seek out roots by chemotactically attracting to suitable infection sites (Carlile, 1983). The early stages of the infection process during PC infection have been characterized at the cellular level for C. sativa and C. crenata (Fernandes et al., 2021b). The zoospores shed their flagella, encyst, and grow a germ tube on the root surface until the development of an appressorium-like swelling that allows the rhizodermis penetration, initiating the infection process. The zoospores can identify and infect susceptible and resistant Castanea roots as quickly as 3.5 h after root inoculation. Hyphae develop until they reach the vascular tissues of the susceptible European chestnut on the third day of infection. At this stage, PC switches from biotrophy to necrotrophy, characterized by cellular collapse and it starts producing resistance structures (chlamydospores) in C. sativa (Fernandes et al., 2021b). Chlamydospores allow PC to persist in plant material and the soil for up to 6 years (Zentmyer and Mircetich, 1966). In the resistant chestnut, C. crenata, the infection progress is slower because the host can induce early defense responses, such as callose deposition, hypersensitive response-like, and production of phenolic-like compounds. Nevertheless, PC can still reach the vascular tissues (Fernandes et al., 2021b). After reaching the vascular tissues of susceptible chestnuts, the pathogen continues colonizing the roots until it obstructs xylem vessels (Gomes-Laranjo et al., 2004), preventing root growth and interfering with water and nutrient uptake to the shoot. The roots and root collar start to rot, resulting in a progressive decline of the tree (Hardham, 2005). The first above-ground symptom is the chlorosis and wilting of leaves at the top followed by the dieback of branches, defoliation, and gradual decline until the host dies (Figure 3; Gomes-Laranjo et al., 2004). In resistant chestnuts the progression of the lesion caused by PC seems to stop at the roots or root collar level (Santos et al., 2015a), preventing further decline of the tree.
Figure 3. Castanea sativa shows symptoms of ink disease such as discoloration of leaves and dieback of branches. This picture was taken in Bragança, Trás-os-Montes region, Portugal.
Control measures to prevent/restrain the pathogen have not been successful so far, mainly due to the easy development and migration of zoospores in humid conditions (especially during rainfalls) and to the resistance structures difficult to eradicate. Phosphite and metalaxyl have been the most efficient chemicals against PC [reviewed in Hardham (2005) and Hardham and Blackman (2018)]. However, the continuous use of these two chemicals has led to resistance development by the pathogen (Hardham and Blackman, 2018).
Until this date, there is no known effective biological control. Still, promising results were reported with soil inoculated with the bacteria Byssochlamys nivea or Scopulariopsis brumptii, which appears to decrease mortality in chestnuts inoculated with PC (Bosso et al., 2016). More common control approaches are the correct management of nurseries/orchards, the use of resistant rootstocks for propagation, or planting of resistant hybrids for production (Hardham, 2005).
In Europe several first-generation Euro-Asian hybrids have been produced by conventional breeding. Large backcross breeding programs have not been carried out to obtain a nearly wild-type disease-resistant European chestnut, and this may have cost a loss in specific characteristics of the European chestnut. However, many of the obtained hybrids have been successfully used as resistant rootstocks for European varieties, or as nut producing trees for having both resistance to the pathogen and sweet-tasting nuts. The most known example of this is the Euro-Japanese hybrid, CA04 or ‘Bouche de Bétizac’ (BB) developed by INRA (France) in 1962 (Table 2; Breisch et al., 1995). This hybrid became popular for having very large, sweet-tasting nuts and fast production. Cultivar selection has also been extensive in Italy, Spain, and Portugal, and regional favorites are developed mainly from local wild populations with large-caliber nuts.
In 1925 the first crosses started in France (Camus, 1929) and in 1926 started in Spain by Gallastegui, initiating the hybridization program between C. crenata and C. sativa (Pereira-lorenzo et al., 2010). After the 1940s, several breeding programs settled across Europe (Schad et al., 1952; Urquijo-Landaluze, 1957; Viéitez, 1960; Molina and Viéitez, 1967; Taveira-Fernándes, 1972; Salesses et al., 1993). Some of these programs obtained hybrid genotypes with low tolerance to cold (Breisch et al., 1995) and poor adaptability to the European environmental conditions in general, mainly because these were F1 hybrids (C. crenata × C. sativa) with 50% of their genetic information from Japanese chestnuts, a species with low tolerance to cold and drought (Fei et al., 2012). Several clones (111-1, 7521, 2671, and 1483) from Spanish breeding programs developed in the mid-20th century are still widely used as rootstocks for their high tolerance to ink disease and very high rootstock compatibility with fruit varieties (Serdar et al., 2019).
In Portugal, the first interspecific crosses were in 1947 by Bernardino Gomes, who used C. crenata (Tamba variety) as pollen donor (Vieira Natividade, 1947; Gomes Guerreiro, 1948, 1957). Later, in the 1990s, Professor Lopes Gomes started a breeding program at the University Trás-os-Montes e Alto Douro developing 53 genotypes resistant to ink disease (Table 2; Gomes Laranjo et al., 2007; Martins et al., 2009). More recently, in 2006, a breeding program was initiated (Costa et al., 2011) from which four F1 hybrids were selected for large-scale propagation due to their ability to multiply and root in vitro, field development and PC resistance levels (Table 2; Santos et al., 2015a; Fernandes et al., 2020a,2021a).
As a consequence of breeding programs, the introgression of Asian alleles has been reported in a natural C. sativa forest (Alcaide et al., 2022). Adult and juvenile (offspring) trees were genotyped and PC resistance was detected. Back in the 1940s, C. crenata and C. mollissima were planted in the studied forest, which justifies the presence of only 70.6 and 28.6% of adults and juveniles, respectively, classified as pure C. sativa in this area. Alcaide et al. (2022) also reported more than 40% of juveniles as C. sativa × C. crenata hybrids and about 10% C. sativa × C. mollissima hybrids. Ten private alleles to Asian species were found in offspring, eight were exclusive to C. crenata, and two were found in C. crenata and C. mollissima species (Alcaide et al., 2022). The studied forest may benefit from the transfer of alleles involved in ink-disease resistance, and this advantage may be present in other European forests and orchards. However, more forest assessments should be performed to ensure that the European genotypes are not lost over generations.
With the increasing demand to support and accelerate breeding, phenotyping chestnut genotypes has been performed using different techniques. Symptom severity scales and measurements for subsequent accurate molecular marker: trait associations were reported turning screening for Phytophthora spp. resistance more efficient. Either by root inoculation of intact seedlings (Vettraino et al., 2001; Santini et al., 2003; Robin et al., 2006; Jeffers et al., 2009), root inoculation of rooted cuttings (Miranda-Fontaíña et al., 2007) or rooted shoots from in vitro culture (Cuenca et al., 2009; Santos et al., 2015a). Also, excised, or intact shoot from seedlings or clones were directly inoculated on its top (Ramos Guedes-Lafargue and Salesses, 1999; Fernández-López et al., 2001; Vettraino et al., 2001; Robin et al., 2006; Miranda-Fontaíña et al., 2007; Cuenca et al., 2009; Santos et al., 2015a). Phenotyping assays in chestnuts are usually performed at leaf-falling time (autumn) and after budburst (spring) (Santos et al., 2015a). According to Santos et al. (2015a), the root inoculation test was the best-resulted method to mimic the infection process in nature. However, this evaluation is expensive, laborious, and sometimes it is not feasible at the population level.
Studies performing the evaluation of responses after root inoculations reported a decrease in the severity of symptoms from the root to shoots. Also, different root-lesion phenotypes were observed depending on genotype susceptibility to the pathogen. The most resistant genotypes can confine or stop the spreading of the pathogen in the roots and from roots to root-collar, unlike the more susceptible ones that usually present their root system majorly affected, and consequently wilting of leaves (Miranda-Fontaíña et al., 2007; Santos et al., 2015a). Furthermore, control plants grow more than those subjected to inoculation (Robin et al., 2006; Miranda-Fontaíña et al., 2007; Santos et al., 2015a), which is expected as one of the consequences of the disease is the reduction of water and nutrient uptake, that consequently affects photosynthetic yield and plant growth (Santos et al., 2015a).
Depending on the phenotyping method (root or excised shoot), different metrics can be used to score the disease damage, and there are dissimilar opinions about which variable should be considered the main discriminator of resistance to PC. Previous studies specified the root or collar rot level should be considered the primary discriminator of resistance to Phytophthora sp. in chestnut (Robin et al., 2006; Miranda-Fontaíña et al., 2007; Cuenca et al., 2009). Other studies consider plant survival the primary indicator of resistance (Vettraino et al., 2001; Santos et al., 2015a). Santos et al. (2015a) stated the variable ‘days of survival’ was an excellent parameter to define resistance because the difference in the response of the genotypes is accentuated, and the heritability values are high (0.9 ± 0.04). In this work, shoot internal lesion-symptom was evaluated for the first time and it was considered crucial because it showed the advance of the disease lesion from roots and collar to aerial plant organs through the vascular system (Santos et al., 2015a).
After realizing the importance of breeding for ink disease, North American TACF researchers started analyzing their trees from the backcross breeding program for PC resistance. In 2010, a preliminary study reported in a single major effect QTL in LG_E that explained more than 30% of the variation in a backcross population (Table 2; Kubisiak, 2010). These findings were later supported by Zhebentyayeva et al. (2014) who identified a major effect QTL for ink disease resistance in the same LG by studying several populations segregating for ink disease resistance (Table 2). In 2015, in Europe, Santos et al. (2015b) developed Simple-Sequence Repeats from Expressed Sequenced Tags (EST-SSR). These, together with Single Nucleotide Polymorphism (SNP) markers, were later used to construct an interspecific linkage map where two QTLs for PC resistance were identified (Santos et al., 2017b). The markers associated with QTL in LG_E and LG_K may enclose candidate genes to PC resistance, and genes putatively involved with the regulation of gene expression, respectively (Table 2; Santos et al., 2017b).
The arrival of next-generation sequencing revolutionized several research areas, including the detection and validation of genetic markers in wild and hybrid populations. Using GBS, Zhebentyayeva et al. (2019) increased the number of available markers for linkage analysis, mapping 7,715 sequence-based SNPs on eight parental genetic maps. Seventeen QTLs were associated with ink disease resistance on LG_A, LG_C, LG_E, and LG_K (Table 2). The most consistent QTLs were detected on LG_E and LG_K, which overlapped with QTLs previously reported by Santos et al. (2017b). The authors suggest the genetic architecture of PC’s resistance in Chinese chestnut × American chestnut hybrid progeny is due to some dominant QTLs together with quantitatively inherited partial resistance conferred by multiple small-effect QTLs (Zhebentyayeva et al., 2019).
In Europe, root transcriptomes of C. crenata and C. sativa inoculated and non-inoculated with PC were compared, resulting in the discovery of 283 differentially expressed genes as candidates for PC resistance (Serrazina et al., 2015). In 2017, eight of those genes were selected for further study and by using digital PCR their expression was evaluated in chestnut roots before and during infection (Table 2; Santos et al., 2017a). The authors suggest that European and Japanese chestnuts have the same defense mechanisms to PC but with different timings. The upregulation of a set of candidate genes after infection, such as Cast_Gnk2-like (anti-fungal function) and Cast_C2CD (pathogen recognition protein), suggests that C. crenata triggers HR-like cell death. The high expression of these genes in non-inoculated C. crenata compared to non-inoculated C. sativa, suggests improved constitutive defense mechanisms by the Japanese chestnut (Santos et al., 2017a). Indeed, these hypotheses were confirmed by Fernandes et al. (2021b) at the cellular level, who reported shared host responses in these two species following pathogen challenge (e.g., callose deposition and phenolic-like compounds accumulation). Cast_Gnk2-like, was identified as the best discriminator between susceptible and resistant genotypes to ink disease (Santos et al., 2017a), and efforts for the validation of its function in chestnuts are ongoing (Table 2; McGuigan et al., 2020). The gene Allene oxide synthase (CcAOS), an ortholog of a key enzyme of the JA pathway, was also selected from 2015 transcriptomes (Serrazina et al., 2015). The importance of this gene in plant defense responses against PC was demonstrated after being overexpressed in a susceptible Arabidopsis ecotype (Ler-0), resulting in a delay of infection progression and an increase in tolerance (Table 2; Serrazina et al., 2021).
Reports on ink disease molecular analysis discussed so far have been focused on root inoculations because it mimics what happens in nature. Saiz-Fernández et al. (2020) presented an alternative by inoculating European chestnut stems and collecting tissues bordering the infection site and away from it. Proteomic, metabolomic, and targeted hormone analysis showed that PC led to an accumulation of SA and JA and a massive reprogramming of the chestnut’s proteome. Twenty-five proteins were identified as oppositely regulated in the areas next to and away from the infection site (Saiz-Fernández et al., 2020).
When studying plant-pathogen interactions, the first and most common approach is to unveil the host resistance mechanisms. To date, plant susceptibility (S) genes were only studied in a few woody species, as discussed by Pavese et al. (2021b). S-genes allow the compatibility of the pathogen to the host, facilitating infection. A mutated or lost S-gene may limit the pathogen’s ability to induce host disease. The authors identified and characterized S-genes in C. sativa based on sequence homology, functional domain detection, and phylogenetic relationships. Transcript levels of S-genes after pathogen infection (both PC and CP) were generally higher in C. sativa when compared to C. crenata. Two genes were selected for future studies on their putative role as S-genes in chestnut-pathogen interactions: Powdery mildew resistance 4 (pmr4) and Downy Mildew Resistance 6 (dmr6) which are suggested to act as negative regulators of SA pathway, consequently leading to susceptibility (Pavese et al., 2021b).
The emerging progress of genetic transformation systems for chestnuts has been an extremely encouraging story and nowadays genes can be tested for their ability to confer pathogen resistance. The progress of embryogenic regeneration systems for chestnut species [reviewed in Corredoira et al. (2017)] has provided fitting target material for transformation experiments. Carraway et al. (1994) did the first attempt on Castanea spp. genetic transformation, however, only obtained transgenic calli by using microprojectile bombardment in cultures of American chestnut. Since then, chestnut researchers have been dedicated to improving genetic transformation of this recalcitrant species. The first report of successful Agrobacterium-mediated transformation in Castanea spp. showed transgenic European chestnut shoots regenerated from hypocotyls but, transformation efficiencies were very low, and the number of chimeras was high (Seabra and Pais, 1998). This was followed by the transformation of European chestnut somatic embryos that were regenerated into whole plants and micropropagated (Corredoira et al., 2004). The authors recorded a maximum of 25% transformation efficiency after somatic embryo co-culture with Agrobacterium liquid suspension for 4 days (Corredoira et al., 2004). The first successful genetic transformed American chestnut was reported in 2006 by co-culturing somatic embryos with Agrobacterium liquid suspension (for 1 h) followed by a 2-day desiccation method (Polin et al., 2006). Rothrock et al. (2007) also transformed American chestnut by flooding the embryos with Agrobacterium liquid culture while still in semi-solid multiplication media. After these protocols, several works were published on the transformation of chestnut with pathogen resistance genes or to validate gene function. Genetic transformation studies for pathogen control are summarized in Table 3.
Table 3. Genetic transformation studies performed in European and American chestnuts with the goal of developing pathogen control strategies.
The rise of genetic transformation had a big impact, but public perception of the use of transgenes is not unanimous. Nowadays researchers are looking more into the use of cisgenes (transgenes from related species) (Corredoira et al., 2012, 2016; Steiner et al., 2017), trying to answer public concerns. Corredoira et al. (2012, 2016) obtained cisgene overexpressing lines with a (1) thaumatin-like protein (CsTL1) gene (Table 3; Corredoira et al., 2012); and (2) an endochitinase gene (CsCh3) (Table 3; Corredoira et al., 2016). More recently, McGuigan et al. (2020) transformed American chestnut with Cast_Gnk2-like (Table 3), a candidate resistance gene for PC (Santos et al., 2017a). In McGuigan et al. (2020) the authors also report two alternative methods for transformant selection by using liquid selection medium instead of semi-solid medium like the previously mentioned protocols. After the Agro-kill step, embryos were transferred to temporary immersion bioreactor systems (RITA® bioreactors, Sigma-Aldrich, St. Louis, MO, United States) or We Vitro containers cultivated by Magenta® (We Vitro Inc., Guelph, ON, Canada) where they were intermittently flooded and rocked, respectively. Although the treatments were not significantly different, the liquid medium protocols had more selection efficiency (McGuigan et al., 2020). As the genetic transformation techniques improve, targeted promoters that can replace the most common constitutive promotors also arise. An example of this is the win3.12 inducible promotor from poplar (Populus deltoides), which has positively driven the gene oxalate oxidase (OxO) in American chestnut, showing a low level of baseline expression and being only induced by wounding and pathogen infection (Table 3; Carlson et al., 2021).
North American researchers have allied their breeding efforts to genetic transformation as this can be a quicker way to restoration when compared to just the traditional backcross breeding. In 1990, the TACF New York Chapter and the State University of New York-College of Environmental Science and Forestry (SUNY-ESF) started working on this alternative approach for restoration. A blight-resistant American chestnut tree (Darling 58, named after Herb Darling) was developed by genetic transformation (Polin et al., 2006; Rothrock et al., 2007; McGuigan et al., 2020) by adding to the genome a gene from wheat that encodes for a detoxifying enzyme, oxalate oxidase (OxO), to counter the major virulence factor of the pathogen (Powell et al., 2019). Oxalate oxidase degrades oxalic acid, a toxin produced by CP during infection (Rigling and Prospero, 2018), limiting the pathogen’s damage without killing it. Currently, these trees are in regulated field plots awaiting deregulation for restoration purposes (Table 1; Newhouse et al., 2020). Crosses of transgenic chestnuts with wild-type American chestnuts are being performed in these plots (Westbrook et al., 2020a). The progeny is 100% American chestnut and approximately 50% of the offspring inherits OxO (which is rapidly detected by PCR or enzymatic assays; Zhang et al., 2013a). To increase genetic diversity, up to 4 transgenic chestnuts will be crossed with 1500 wild-type trees over up to 5 generations (Westbrook et al., 2020a). Transgenic pollen can be produced in less than a year (Baier et al., 2012; Pilkey, 2021), which helps expedite the process. Deregulation of the Darling American chestnut represents an important step toward restoring the species. However, public perception of introgressing a transgenic tree into the forest is not unanimous [discussed in Newhouse and Powell (2021)].
Another exciting news for chestnut genome editing is the first proof of concept of CRISPR/Cas9, where the authors obtained albino plants by inducing a point mutation in phytoene desaturase (pds), a gene that disrupts chlorophyll biosynthesis (Pavese et al., 2021a). This new approach opens a new path for the functional characterization of genes involved in plant-pathogen interaction. The same research team characterized and selected two S-genes in C. sativa after PC and CP infection (pmr4 and dmr6 referred to in Section “Improving characterization of Phytophthora cinnamomi resistance”; Pavese et al., 2021a) which are potential candidates for functional characterization via CRISPR/Cas9 knockdown. This approach and the study of S-genes might help us understand if PC is adapted to the susceptible chestnuts and how it is interfering with their immunity and possibly inducing Effector Triggered Susceptibility.
The chestnut gall wasp Dryocosmus kuriphilus Yasumatsu, accidentally introduced into Italy and first reported in 2002 (Brussino et al., 2002), represents a limiting pest for the European chestnut, due to the severe yield losses it creates, as C. sativa has a low tolerance. Figure 4 shows a chestnut infected by the gall wasp. It quickly spread to all Italian regions and later into the surrounding countries, causing a remarkable decrease in production (−60% in 2014 in Italy). Studies on biological control aimed at introducing the parasitoid wasp Torymus sinensis Kamijus, and also the genetic improvement for resistance to the cynipid were promptly started to solve the problem (Marinoni et al., 2020). The susceptibility to the chestnut gall wasp was evaluated in C. sativa and hybrid cultivars. Out of 62 cultivars, two C. sativa, one C. crenata, and four hybrids (C. sativa × C. crenata) showed total resistance (Sartor et al., 2015).
Figure 4. Castanea spp. presenting a gall on the leaf (arrow) after Dryocosmus kuriphilus infection.
Resistance to the gall wasp was found in the hybrid cultivar Bouche de Bétizac (BB; C. sativa × C. crenata) (Dini et al., 2012) and studied by developing genetic linkage maps using a population derived from a cross between BB and the susceptible cultivar ‘Madonna’ (M; C. sativa) (Marinoni et al., 2020). The high-density genetic maps were constructed using double-digest restriction site-associated DNA-seq and simple sequence repeat markers. The map of BB consisted of 1,459 loci and spanned 809.6 cM; the map of ‘Madonna’ consisted of 1,089 loci and spanned 753.3 cM. In both maps, 12 linkage groups were identified. A single major QTL (Rdk1) was identified on the BB map, explaining up to 67–69% of the phenotypic variance of the resistance trait. The Rdk1 QTL region includes eleven scaffolds and two candidate genes putatively involved in the resistance (Marinoni et al., 2020). Acquadro et al. (2020) presented de novo assembly of the chestnut transcriptome of the resistant Euro-Japanese hybrid BB and the susceptible cultivar M, collecting RNA from buds, at different stages of budburst to investigate the plant response and understand which factors can lead the plant to develop or not the gall, to reconstruct the transcriptome of C. sativa buds under biotic stress (i.e., in the presence/absence of the chestnut gall wasp). The two transcriptomes were assembled into 34,081 (BB) and 30,605 (M) unigenes, respectively. The transcriptomes of both cultivars were properly assembled, and while the BB unigenes set was selected for the functional characterization, the M was just used for RNA-seq data analysis, highlighting the presence of 1,444 putative resistance gene analogs (RGAs) and about 1,135 unigenes, as putative miRNA targets. A global quantitative transcriptome profiling revealed some Gene Ontology enrichments as “response to stimulus” and “developmental processes” (e.g., post-embryonic development). Many up-regulated genes appeared to be transcription factors (e.g., RAV1, AP2/ERF, and WRKY33) or protein regulators (e.g., RAPTOR1B) and storage proteins (e.g., LEA D29) involved in “post-embryonic development.” Dini et al. (2012) highlighted the occurrence of HR in BB as a response to the cynipid infestation, resulting in cell and larvae death. This fact was confirmed by Acquadro et al. (2020), since more than 100 genes appeared to be associated with “death” and “apoptosis” processes, including genes for HR response. The analysis was able to provide 7k simple sequence repeat SSR and 335k SNP/INDEL markers and generated the first reference unigene catalog for the European chestnut.
Gall wasp, apart from representing a severe constraint factor for the production of chestnut orchards, can also impact negatively the favorable effect of hypovirulence in Cryphonectria parasitica-C. sativa pathosystem, by the progressive weakening of the trees, caused by intensive attacks of Dryocosmus kuriphilus. Blight damage recurrences were observed in different Italian chestnut areas (in Piemonte, Trentino, and Toscana regions which were highly infested by the Chinese wasp; Turchetti et al., 2010). The use of effective biological control of the parasite with parasitoids like T. sinensis, is essential for the management of chestnut orchards to allow the survival of the trees and their productivity.
The preponderance of reports on abiotic stresses focuses on the European chestnut. Since the beginning of the millennium and the awareness of climate change scenarios, studies on its impact on the species flourished (e.g., Casasoli et al., 2004; Lauteri et al., 2004). C. sativa is susceptible to climate change (Camisón et al., 2020; Castellana et al., 2021), raising the probability of abiotic stress events. Prolonged water deprivation or waterlogging and chill hour reduction, combined with rainfall deficit and extreme summer heat in Europe, may cause and prejudice nut and timber productivity and quality (Vázquez et al., 2016; Castellana et al., 2021). These extreme climate scenarios are predicted to be most frequent in Southern Europe (European Commission, 2022), where chestnut orchards have a great representation (Pérez-Girón et al., 2020). The review in this section will mainly focus on this European area.
Climate change is also predicted to significantly impact chestnut pests and pathogens (Burgess et al., 2017; Bonsignore et al., 2020), leading to differences in disease expressions since new strains and infection mechanisms are more likely to arise.
Three flexible domestication levels of C. sativa are considered: fruit orchards, coppice, and natural forests (Eriksson et al., 2005; Gomes-Laranjo et al., 2012). The flexibility among levels is related to the exchange of genetic material between the three types of populations, even though genetic variability is higher in natural forests (excluding the American species) and lower in orchards, which originated from domestication. Genetic variability encloses the capacity to adapt to adverse environmental conditions, maintaining homeostasis and reproduction, and the potential to evolve. Changes in morphology and physiology in response to abiotic stresses involve complex molecular processes under genetic and epigenetic control. Besides knowledge at the physiological level, knowledge of the response to the stress at the genetic and molecular level in detail is essential to drive efficient tolerance to threatened species.
The genetic resources of C. sativa natural populations represent the existing variation in adaptive traits (Eriksson et al., 2005). They may be the starting material for breeding programs to address tolerance to abiotic stress in elite varieties. Many studies characterize C. sativa ecotypes from the Iberian Peninsula, Italy, Greece, and Turkey (e.g., Villani et al., 1991; Eriksson et al., 2005; Fernandez-Lopez et al., 2005; Pereira-Lorenzo et al., 2011; Míguez-Soto et al., 2019; Castellana et al., 2021).
The environment influences adaptative traits such as annual biomass production, juvenile phenology growth, water use efficiency (WUE), and carbon isotype discrimination (Δ). European chestnut is a temperate-climate tree that requires relatively cool winters for dormancy and then warmer temperatures in spring, allowing physiological and phenological development as bud break, flowering, fruit set, and maturation (Gomes-Laranjo et al., 2012). Phenology depends on temperature, nutritional state, photoperiod, hormones, phytochromes, and others and can represent seasonal and environmental adaptation (Santamaría et al., 2009). WUE is the ratio of plant carbon (C) gain to water loss and is inversely related with Δ, which is affected by CO2 assimilation and stomatal conductance (gs) (Lauteri et al., 2004). From research mainly on non-cultivated populations, several European chestnut ecotypes are adapted to different climates, corresponding to different evolutionary pressures in the genome. In the Iberian Peninsula, Italy, Greece and Turkey, and based on morphological, physiological and/or molecular markers’ studies (SSRs, EST-SSRs), hotter Mediterranean regions with lower water availability or drought drove populations to xeric ecotypes (early phenology, slow growth, high root development, high Δ, low WUE and longer juvenile periods). In comparison, populations in colder Atlantic regions with more water availability are mesophytic or mesic (later phenology, higher growth, low Δ) (Lauteri et al., 2004; Fernandez-Lopez et al., 2005; Gomes-Laranjo et al., 2012; Míguez-Soto et al., 2019; Camisón et al., 2021). Lauteri et al. (2004) suggest that mesic ecotypes respond strongly to favorable climatic conditions by increasing growth, while xeric ecotypes respond slowly to reduce the risk of damage after drought (e.g., xylem embolism, C starvation). This is in accordance with Pérez-Girón et al. (2020). They compared essential physiological parameters in orchards from two regions of the Iberian Peninsula: northern Spain and Portugal, and southern and central Spain. The authors found the highest annual photosynthesis rate and net primary production (net C stored after respiration and transformed into biomass) in the northern ecosystems. Water availability and temperature were the climatic variables that most influenced the two parameters.
Different European chestnut ecotypes may have origin in an overall high genetic diversity (Villani et al., 1991; Pereira-lorenzo et al., 2010; Cuestas et al., 2017; Poljak et al., 2017; Alessandri et al., 2020; Bouffartigue et al., 2020). Dinis et al. (2011a) and Pereira-Lorenzo et al. (2011) found, with the use of SSR markers, that the diversity in chestnut orchards was greatly due to hybridization and discretely due to mutations. C. sativa genetic diversity, with many alleles and a high level of polymorphism and heterozygosity (Casasoli et al., 2006), provides gene pools useful for establishing future conservation strategies. The ecotypes’ traits correspond to adaptations that have the potential to be introgressed in threatened chestnut stands, potentially providing adaptation to climate change.
Most of the reports about C. sativa diseases related to abiotic stress describe the response to drought. C. sativa is strongly represented in the European Mediterranean area, which has been experiencing long and dry summers (high temperatures and low precipitation levels) with increasing drought conditions, causing a negative impact on C. sativa survival, productivity, and biodiversity (Ciordia et al., 2012; Alcaide et al., 2019; Castellana et al., 2021). C. sativa tree growth may be severely affected when the drought period is higher than two consecutive months (Menéndez-Miguélez et al., 2015), with most probable negative consequences on the development of leaves and fruits (Dinis et al., 2011a). Drought response is complex to analyze, as it may be influenced by population history, frequency of drought events, and phenotypic plasticity (Casasoli et al., 2006; Alcaide et al., 2019; Müller and Gailing, 2019).
Martínez-Sancho et al. (2017) consider C. sativa a relatively anisohydric species in the physiological responses to high temperature and drought, meaning that stomata closing is not readily achieved after water deprivation unless under severe drought conditions. Low water potentials (Ψ) in seedlings resulted in an overall native loss of hydraulic conductivity and probable vessel embolism, accompanied by height and stem diameter decrease compared to controls. The authors suggest that the hydraulic conductivity can be potentially recovered in the next growing season with new earlywood vessels and xylem renovation.
Maurel et al. (2004) report that gs, transpiration rate (E), hydraulic conductance (K) from soil to leaf, leaf Ψ, and root biomass decreased in C. sativa subjected to drought, whereas abscisic acid (ABA) concentration in xylem increased. The authors also showed that gs was regulated by the root-sourced ABA and by hydraulic signals, namely the relative sap flux from root to leaves. Leaf transpiration is an essential factor in establishing Ψ, or the flow of water from the soil to the roots, stems, leaves (stomata) and atmosphere, with the purpose of mineral uptake and regulation of leaf temperature. During water deprivation, E became seriously compromised, contributing to a significant decrease in overall plant metabolism and productivity (Gomes-Laranjo et al., 2012).
Ciordia et al. (2012) and Gomes-Laranjo et al. (2012) studied progenies of C. sativa cultivars (seedlings) from two areas in the Iberian Peninsula, North (Asturias and Galicia, with mesic or moderately humid environment) and Central/South (Canary Islands and Andalusia, with xeric and drier environment). Merging the results from both studies, restricted water supply reduced the Ψ, K (especially in xeric plantlets), CO2 assimilation rate (A), E, gs and, consequently, photosynthetic efficiency. Gomes-Laranjo et al. (2012) associated the reduced efficiency of Photosystem II (PII, low Fv/Fm) with an internal CO2 concentration increase (Ci) and lower C assimilation, especially in Northern plant leaves. During water deprivation there is a need to reduce light absorption to avoid heat accumulation, resulting in the reduction of PSII efficiency or even photooxidative reduction in extreme conditions. An expected consequence is a reduction of growth (height and dry weight, except stem diameter) (Ciordia et al., 2012). Also, both studies found a reduction of leaf area, number (with no leaf fall) and sprouting, attributed to lower absorption of nutrients. The morphology of the leaves suffered modifications, with an increase in leaf lobation, resulting in a smaller boundary layer and more efficient heat exchange. The root:shoot ratio increased due to biomass distribution changes in response to the low water content in the soil, promoting root biomass that may improve the capacity to absorb water (Ciordia et al., 2012). The same authors consider that the north cultivars are more tolerant to drought than the Central ones, as the first demonstrated a better ability to recover Ψ after re-watering. Both mesic and xeric groups demonstrate phenotypic plasticity that is consistent with the genetic variation found using SSR and EST-SSR (Martin et al., 2010; Pereira-lorenzo et al., 2010), providing such stands the potential to respond to drought stress (Ciordia et al., 2012).
Camisón et al. (2020) (Figure 5) found that in drought-tolerant C. sativa seedlings (of the xeric ecotype from central Spain) the gs was close to zero, associated with a decrease in relative water content (RWC), height and weight loss, increase in stem diameter, leaf wilting with occasional drop and plant dieback. Stomatal closure was associated with the reduction of A and soluble sugar accumulation in leaves, which may impair C supply. The authors suggest that soluble sugar accumulation in leaves and stems may have a role in plant osmoregulation. A decrease in leaf biomass was accompanied by an augment in nitrogen (N) levels in leaves due to N transport from senescent to green leaves. The peak of soluble sugar levels in leaves and stems coincided with the highest reduction in starch levels, probably due to starch mobilization as a source of soluble sugars for cell metabolism, osmotic adjustment, and consequent xylem vessel water refilling after drought-induced embolism. High respiration levels in stressed plants are related to the metabolism of soluble sugars to counteract the stress. The authors did not observe changes in total carbohydrate content or C starvation attributable to drought-tolerant species in non-extreme drought conditions.
Figure 5. Castanea sativa seedlings under regular watering (A), drought (B), and waterlogging (C), from Camisón et al. (2020). Control plants present green and turgid leaves. Leaves of drought plants present wilting and some may fall. Dieback can be observed. Leaves of plants with waterlogging present chlorosis, chlorophyll degradation in the central part of leaves, necrotic borders and senescence.
Economically important chestnut orchards for nut production in Southern Europe are distributed through regions of provenance, many with several decades old, and established recurring to grafting without a conscient concern about the effects of global climate change. Camisón et al. (2021) analyzed if drought tolerance in C. sativa could be improved using drought-tolerant scions and rootstocks from xeric populations (X) from southern Spain, in comparison with drought-sensitive scions and rootstocks from humid populations (H, with mesic ecotype) from northern Spain, based in the research of Alcaide et al. (2019). Grafted and non-grafted 2 years old plants were used in the drought treatments. When X scions or rootstocks were used in these conditions, budbreak occurred earlier, and higher gs and lower plant mortality were observed. C. sativa families of X origin advance budbreak phenology and may be used to induce early flushing in scions of H origin. Benefits could be attributed to the use of X rootstocks to advance budbreak in mesic areas, especially if enhanced growth, flowering, and fruit production are obtained. Grafted plants with X rootstocks (H/X and X/X) showed higher A, gs, Fv/Fm of PII and leaf RWC than plants with H rootstocks (X/H and H/H). Rootstocks from xeric areas increase drought tolerance and survival of the more drought-susceptible material of mesic origin. On other hand, H used as rootstock lead to minimum values of leaf RWC and Fv/Fm levels, confirming its susceptibility to drought. Concerning scion, grafts with X rootstock wilted less than grafts with H rootstocks. Scions of xeric origin may also have the potential to improve drought-susceptible rootstocks. Grafting may be implemented as an adaptative tool to surpass climate change’s effects (Camisón et al., 2021).
Camisón et al. (2021) also analyzed constitutive and drought-induced hormones [ABA, SA, JA, its conjugate (+)-7-iso-jasmonoyl-L-isoleucine (JA-Ile)], and the amino acid proline in leaves and roots of grafted and non-grafted plants of X and H origin. Before drought induction, in watered plants, leaf ABA and proline contents were higher in X than in H plants, in non-grafted and grafted material. The constitutive higher levels of ABA in X material may have contributed to lower gs and the delay in plant dehydration in X rootstocks after drought treatment (Allario et al., 2013). The same rationale may be applied to proline, which may have conferred to X rootstocks a more effective osmotic adjustment when drought was applied (Amudha and Balasubramani, 2011). After drought treatments, ABA and proline levels increased in leaves and roots of all materials. H/H plant presented the highest levels of ABA in roots and proline in leaves, which points to extreme stress. SA levels were higher in H, H/H and X/H plants. SA increase along with ABA has been reported in citrus response to drought (Santana-Vieira et al., 2016; Neves et al., 2017). JA-Ile level was higher in leaves, especially in H/H plants. JA-Ile in leaves under drought stress, allied to ABA, is involved in stomatal closure modulation (de Ollas et al., 2013). JA-Ile and JA levels in roots generally decreased, and the lowest value was found in H and H/H plants. More studies are needed to understand the hormone crosstalk in C. sativa response to drought.
Casasoli et al. (2004) performed a QTL analysis for three adaptative traits (bud burst, growth, and Δ), for 3 years, in an F1 progeny of C. sativa originated from two Turkish populations adapted to drought (female parent) and humid (male parent) environment. Thirty-five distinct QTLs were identified for phenology, 28 for growth, and 17 for Δ. The authors report phenotypic correlations and co-localization among QTLs for the three adaptative traits related to the genetic adaptation of the female parent to drought. Moreover, the adaptative traits seem to be regulated by several genes or gene groups of low and moderate effects, suggesting that the adaptation, and consequently the response to abiotic stress at the genetic level is highly complex in chestnuts.
Santamaría et al. (2011), after analyzing the transcriptome of dormant and non-dormant tree buds in C. sativa trees of Asturias (North of Spain), suggest that bud dormancy is associated with abiotic stress tolerance. There was a high representation of genes involved in low-temperature stress and dehydration protection of cellular structures: Late embryogenesis abundant proteins (LEA), including Dehydrins and Em protein, Heat shock proteins (HSP), and transcription factors that control the expression of HSPs [reviewed in Kalemba and Pukacka (2007)]. Also, galactinol synthase (GOLS) and Raffinose family oligosaccharides (RFOs) are involved in desiccation tolerance through protection to oxidative damage (Vinson et al., 2020).
Alcaide et al. (2019) quantified drought response in populations of C. sativa localized in contrasting environment regions of the North (lower average temperature and higher precipitation level) and South (higher average temperature and lower precipitation level) of Spain. In 1-year-old seedlings from selected trees, they found a direct correlation between leaf wilting and resprout with survival, indicative of drought tolerance. Individuals from populations in the South with xeric ecotype, thriving in severe drought conditions, were selected as a drought-tolerant resource. Allied to the data on phenotypic tolerance to drought, EST-SSR MAS permitted separation of North and South populations. Four markers were classified as significantly involved in the differentiation of C. sativa individuals to drought tolerance (Table 4). FIR080 showed one allele for drought susceptibility and may correspond to a Ricin B-like lectin EULS3, involved in drought stress response through stomatal closure in Arabidopsis thaliana (van Hove et al., 2015). VIT057 corresponds to Ethylene-responsive transcription factor ERF017, may act as a transcriptional activator and may be involved in gene regulation by stress factors1. GOT045, a probable E3 ubiquitin-protein ligase, may be involved in regulating ABA-mediated drought stress through ubiquitination (Lee and Kim, 2011; Seo et al., 2012). FIR059 is putatively linked to the RH7 gene of the DEAD-box-RNA helicase family, which has been implicated in RNA processing and related to abiotic stress responses (Kim et al., 2008). Three alleles of FIR059 were linked to drought-susceptible individuals, while two alleles were linked to drought-tolerant ones. FIR059 is pointed as the best marker to identify putative drought-tolerant unstressed trees.
Castellana et al. (2021) associated C. sativa EST-SSR markers previously related with drought stress (Martin et al., 2010; Alcaide et al., 2019) with xeric or mesophytic natural populations in Spain, Greece and Turkey. Those EST-SSRs differentiated three genetic clusters: group I form areas with low precipitation and high temperatures along the year (Table 4); group II with low temperatures and low precipitations; and group III with moderate-low temperatures and high precipitations. Relations were found between climatic variables and alleles in the locus FIR059 above mentioned: allele 152 was associated with heavy rain, allele 181 with warm and dry areas, and allele 185 with mild temperatures. Moreover, alleles 152 and 176 were associated to drought-tolerant plants, while allele 160 was linked to drought susceptibility.
Global climate change will cause, among others, extreme rainfall events with a higher probability of long-term waterlogging in winter and spring, and short-term flooding events during summer (Christensen and Christensen, 2003; Kundzewicz et al., 2005). As C. sativa naturally grows on well-drained mid-sloped soils, it has a low tolerance to waterlogging (Glenz et al., 2006). Chestnut orchards established in floodplains can be severely affected by soil flooding, and there are still few studies that characterize the species response to this abiotic stress. Camisón et al. (2020), already mentioned before for analyzing drought stress in C. sativa, also analyzed waterlogging effects. The two stresses caused some analogous effects on 1-year-old seedlings with progeny from central Spain, with xeric ecotype: reduced gs, A, and growth. The main negative effect of waterlogging in trees is oxygen deprivation in roots (hypoxia, Kreuzwieser and Rennenberg, 2014), causing a decrease in root hydraulic conductivity, xylem sap flow, and phloem transport. Consequently, the first responses to waterlogging include stomatal closure, followed by a decrease in net CO2 assimilation and transpiration. The decrease in gs was not associated with low water content in soil/roots, and Camisón et al. (2020) point to the involvement of chemical signals that regulate gs in waterlogged plants.
Contrary to C. sativa plants with drought stress, lower N content and C/N ratio in leaves of waterlogged plants were observed (Camisón et al., 2020; Figure 6). This can also be attributed to the disturbances in CO2 processing and N uptake by roots. There was also an initial augment of soluble sugar content in all tissues (glucose and sucrose) in waterlogged plants and later an accumulation of starch in stems and roots. This was attributed to an active allocation of C for reserve formation, given the low A rates, and the inability of C. sativa to use carbohydrates for respiration and growth during waterlogging. Typically, susceptible plants decrease the activities of key enzymes for glycolysis in leaves and roots during the stress, not stimulating fermentative pathways as an alternative to producing energy (ATP) (Kreuzwieser and Rennenberg, 2014). Although and unexpectedly for susceptible plants, chestnuts formed aerenchyma at the root collar, pointing to the ability of the use of soluble sugars as C sources. When compared to drought-stressed plants, reduced respiration rates in waterlogged chestnuts, were attributed to a low use of soluble carbohydrates, as already mentioned. Another effect in C. sativa waterlogged plants was chlorophyll degradation (Camisón et al., 2020). This effect, allied to soluble sugar accumulation in leaves, may have resulted in A reduction. A visible related symptom in waterlogged plants was leaf shedding and chlorosis.
Figure 6. Castanea sativa seedling under heat stress conditions described in Dorado et al. (2022).
Camisón et al. (2020) consider that the susceptibility of C. sativa to waterlogging is related to the residual use of non-structural carbohydrates and the active allocation of C to reserve formation, resulting in an overall dropdown of net primary production and growth. Stress-sensitive trees cannot maintain carbohydrate availability resulting in the decrease of anabolic processes and the dieback of stressed tissues (Kreuzwieser and Rennenberg, 2014).
As drought stress response, the waterlogging stress response, is a highly complex process at the molecular and metabolic levels. The physiological adaptations of trees to waterlogging have a lack of data and data interpretation at the -omics level to advance in the understanding of the tolerance processes that serve as guidance to tree improvement programs.
Soto et al. (1999) describes C. sativa response to heat, cold and salt stress. The study focuses on a small heat-shock protein (sHSP) gene purified from mature C. sativa cotyledons, named CsHSP17.5. sHSP are stress-inducible proteins that can prevent irreversible aggregation reactions between stress-labile proteins, maintaining the cytosolic proteins soluble. They are also denominated ATP-independent molecular chaperones (Santhanagopalan et al., 2015). CsHSP17.5 was overexpressed in Escherichia coli and improved viability under heat stress at 50°C and cold stress at 4°C. On the other hand, C. sativa seedlings under 1 year-old were subjected to heat-stress treatments (32°C or 40°C and 80% of relative humidity for 8 h), cold-treatments (4°C for up to 4 weeks) and salt-stress treatments (watering with 200 mM NaCl for up to 48 h). In vegetative organs of chestnut plantlets, transcripts hybridized with a CsHSP17.5 cDNA probe in heat, cold, but not in salt stress. Despite the complexity and polygenic response of plants to abiotic stresses, CsHSP17.5 is an interesting candidate to consider for biotechnological approaches to chestnut improvement to heat, drought (to which heat is mostly allied) and cold stresses (Table 4).
In a just released report (Dorado et al., 2022) where heat stress was tested, a significant increase in the osmolyte proline was observed in C. sativa leaves from humid forests, less thermophilic-tolerant. No variation was noted in C. sativa of xeric origin. Two EST-SSR markers are suggested to be included in the early selection of tolerant chestnuts to heat stress: locus VIT099 (NAC domain-containing protein 78) and POR016 (Heat shock protein 70 k) (Table 4 and Figure 6).
Natural populations of European chestnut seem to adapt better to dry climates than to waterlogging (Vázquez et al., 2016; Camisón et al., 2020). Waterlogging is especially challenging for chestnuts susceptible to Phytophthora spp. growing in favorable edaphoclimatic conditions, as the pathogen causes high mortality in those scenarios (Vázquez et al., 2016). Drought and waterlogging negatively influence plant growth and induce loss of plant vigor, which causes susceptibility to pests and diseases (Maurel et al., 2001; Gomes-Laranjo et al., 2004, 2012; Dinis et al., 2011b).
C. sativa infected with hypovirulent strains of CP, located in the Central Eastern Alps in Italy, showed increased mortality caused by a synergistic effect of blight infection and drought stress (Waldboth and Oberhuber, 2009). The trees were standing in regions with low precipitation during winter and high temperatures in spring and summer. Gomes-Laranjo et al. (2004) suggest that PC infectious capacity may increase with drought and heat, as plants have superior water uptake by roots and higher E, and the root damage caused by the pathogen action may accelerate plant decay. Moreover, Vázquez et al. (2016) report that if drought-stressed C. sativa seedlings are infected with PC and drought conditions are prolonged or waterlogging is applied, the mortality caused by the pathogen is highly significant.
Camisón et al. (2019) made an interesting study in which they assessed the drought stress tolerance in offspring of PC-infected C. sativa trees (from a forest in Southwest Spain of the xeric ecotype). Despite the increased tolerance to the pathogen in 1 year-old plants, suggesting the response was triggered in the subsequent generation, increased tolerance to water stress was not verified, therefore the infection did not influence dehydration stress memory.
Asian Chestnut species have different edaphoclimatic growth conditions when compared to C. sativa, being adapted to more humid environments. Pereira-lorenzo et al. (2010), Fernandez-Lopez (2011) and Vázquez et al. (2016) reveal that Euro-Asian hybrids bred for PC resistance, are susceptible to the frequently occurring summer water stresses in Southern latitudes of Spain. Deep studies on the impact of abiotic stresses in those hybrid clones cultivated in Europe, compared to C. sativa, need to come to light.
Contrary to the chestnuts in Europe, a different positive scenario occurs for the C. dentata x C. mollissima blight-resistant hybrids of the backcross breeding program previously mentioned. These hybrids, ∼94% C. dentata and 6% C. mollissima, are indistinguishable from the original C. dentata. Bauerle et al. (2006) studied the ecophysiological response of C. dentata seedlings to drought. They found that seedlings can decrease gs and maintain Ψ, permitting a balance between transpiration and photosynthesis rate (A), and consequently increase WUE and C gain. The results point to drought tolerance of C. dentata, and if the trait is introgressed in blight-resistant hybrids, the reintroduction of the species in climate change scenarios predicted for the native C. dentata sites of the eastern United States may be facilitated (Brown et al., 2014) (Table 4).
Arbuscular mycorrhizal fungi (AMF) are part of the soil microbiome and the symbiotic association with the rooting system of forest species is essential to plant nutrition and growth. AMF also helps recovery of abiotic stresses in plants, such as drought, flooding, extreme temperatures, salinity, heavy metals (Diagne et al., 2020), and biotic stresses caused by soil pathogens such as PC (e.g., in C. sativa, Branzanti et al., 1999). Equally important is that AMF improves soil aggregation and establishes interactions with beneficial soil microorganisms, upgrading the ecosystem (Diagne et al., 2020).
The AMF increases plant tolerance to extreme temperatures (heat and cold) by maintaining water and nutrient uptake, photosynthesis capacity, alleviating oxidative damage, increasing osmolytes’ level, and consequently, improving growth. In plants subjected to high salinity, AMF enhances water and nutrient uptake, the accumulation of osmoregulators such as proline and sugars, and ionic homeostasis, including reducing Na+ and Cl– uptake (Diagne et al., 2020).
C. dentata × C. mollissima seedlings inoculated with ectomycorrhizal fungi (Pisolithus tinctorius and four Rhizopogon species), and subjected to drought, recovered faster than non-inoculated plants after rewetting (Aryal, 2017) (Table 4). The author based the conclusions on chlorophyll fluorescence levels (Fv/Fm).
At the genetic level, C. sativa in symbiosis with the AMF Pisolithus tinctorius may regulate the expression of genes related to abiotic stress (Sebastiana et al., 2009), as Cystatin, coding a cysteine protease inhibitor that may limit stress-induced proteolysis (Kunert et al., 2015), and Lipid transfer/seed storage/trypsin-alpha amylase inhibitor, corresponding to soluble proteins that facilitate the transfer of fatty acids (García-Olmedo et al., 1995). Curiously, Pernas et al. (2000) reported that non-mycorrhized C. sativa plantlets subjected to cold-, saline- and heat-shocks strongly upregulated a cystatin in roots and leaves, pointing to a relevant role of cystatins in abiotic stress mitigation.
Silicon (Si) is being studied as an ally for the resilience of crops to abiotic and biotic stresses, such as drought, high temperature, cold, salinity, heavy metal toxicity [reviewed in Choudhury et al. (2020)] and fungi, bacteria and herbivores [reviewed in Ma (2004)]. Recently it has been found that the described effects of Si in the alleviation of abiotic and biotic stresses are related to the modulation and regulation of genes involved in photosynthesis, transcription, water transport, phenylpropanoid- and ABA-dependent pathway (Manivannan and Ahn, 2017).
Zhang et al. (2013b) evaluated the foliar application of Sili-K solution on leaves after short but intense water deprivation, in potted plants of the genotype Ca90 (C. sativa × C. crenata, PC-resistant). They observed mitigation in heat stress: the improved leaf growth was related to the increase in A. Si may have increased gs and E, allowing more CO2 to enter in the leaf, decreasing leaf temperature and increasing the transport of nutrients to leaves, respectively. The improved A was also associated with higher Fv/Fm of PSII and chlorophyll a and b content, suggested by a higher proportion of stacking in thylakoid membranes with less photo inhibitory damage. Moreover, Si may participate in the thermal stability of phospholipids in membranes. However, the foliar application of Si also increases the susceptibility to drought. Si application in potted plants with restricted space for root growth resulted in a lower concentration of soluble sugars, which was related to a lower osmotic pressure that may have decreased the cellular turgor and lowered leaf sap osmotic pressure, causing the plant to lose water more quickly (Zhang et al., 2013b).
Gomes-Laranjo et al. (2018) noted that chestnut roots have a good ability to absorb and accumulate Si in the plant. Two-month-old C. sativa were subjected to high temperatures and the soil was fertilized with Si. The authors observed the deposition of phytoliths in leaves and conducting vessels, which was associated with the increase of chlorophyll a/b and carotenoid content, and with the protection of the photosynthetic apparatus from oxidative damage. Also, a lower decrease in A and the increase of WUE was reported when compared to non-fertilized plants. The presence of phytoliths was also involved in the regulation of the stomatal movement, increase of cuticle thickness, and decrease in water loss, related to the lower values of E and gs. Moreover, Si fertilization resulted in plants with a higher level of unsaturated lipids, improving membrane stability and integrity under high temperatures (Gomes-Laranjo et al., 2018) (Table 4).
In a later report, (Carneiro-Carvalho et al., 2019) verified the recovery capacity of 2-month-old C. sativa plants after a turnover from a high temperature to an adequate temperature. Si-fertilized plants showed a better recovery rate when compared to non-fertilized plants, reducing the oxidative damage and improving osmoregulation through the increase in the activity of antioxidant enzymes and metabolites such as catalase, ascorbate peroxidase, peroxidase, and phenols. These may have contributed to the reduction of electrolyte leakage, lipid peroxidation, and reactive oxygen species content.
Si fertilization seems to be absent of negative secondary effects for the environment or human health, making it sustainable for agriculture purposes.
Although many biotic and abiotic stresses threaten chestnuts, many efforts are being developed to overcome the challenges and save these important species. The incidence of PC and CP infections might become more severe in the context of climate change with the possible rise of new strains. Increasing the number of genetic and genomic resources combined with the development of high-throughput phenotyping technologies will help us reach marker-assisted selection (MAS). Screening individual trees within a breeding population and identifying the genetic basis of important traits should become faster, possibly reducing the number of BC needed in traditional breeding programs. The de novo transcriptome assembly provided a significant contribution, however, future studies can now rely on genomes of C. mollissima (Xing et al., 2019; Staton et al., 2020), C. crenata (Shirasawa et al., 2021), and C. dentata (Sandercock et al., 2022).
The potential of new approaches such as CRISPR/Cas systems in trees can result in desired changes such as introducing a gene of interest or targeted gene(s) knockouts of undesirable genes in plants (Limera et al., 2017). Added to gene silencing, the activation of genes and overexpression of proteins can be achieved by CRISPR/dCas9 (nuclease-dead Cas9), conferring new possibilities for gene functional analysis and characterization (Moradpour and Abdulah, 2020). The integrated use of these technological developments with biotic and abiotic stresses will help expand our capabilities of response to chestnut challenges.
The next steps for chestnut breeding may include improving resistance to both pathogens and looking for durable resistance. After screening Asian-American hybrid seedlings with improved blight resistance to PC, TACF found out that many of their hybrids were resistant to the oomycete (Zhebentyayeva et al., 2014, 2019). Researchers now believe that traditional breeding could be used to combine resistance (Steiner et al., 2017). It is also suggested that this could be achieved by stacking multiple resistance genes now that chestnut genetic transformation techniques have been optimized (Powell et al., 2019; McGuigan et al., 2020). There is also an increasing interest in cisgenes possibly because the deregulation process would be more straightforward. Moreover, the public perception may not be as fragmented as it is on the use of transgenics. Stacking cisgenes related to different defense mechanisms can help enhance resistance by different mechanisms. However, it is not expected that any of these genes will confer full resistance to the pathogens. For example, OxO is still the gene demonstrating the highest levels of resistance to blight disease (Steiner et al., 2017). Thus, we should just take advantage of all tools and combine transgenes and cisgenes.
The future roadmap for Castanea-pathogen studies may benefit from exploring the pathogen’s virulence/avirulence factors specific to chestnut interactions. The available genomes of PC (Engelbrecht et al., 2021) and CP (Crouch et al., 2020) may bring some insight. Additionally, dual RNA sequencing enables the determination of responses and changes in the cellular networks of both organisms, which has already helped understand ink disease in other plants (Meyer et al., 2016; Evangelisti et al., 2017). Identifying CP molecular weapons might increase the understanding of the vegetative incompatibility system. Consequently, this may improve hypovirulence biological control in North America where there is high vegetative incompatibility among the fungus strains (Milgroom and Cortesi, 2004). Success with hypovirulence may help keep the surviving American chestnuts alive while breeding for blight resistance is still ongoing.
It is also important to start making more efforts toward the understanding of gall wasp. Sequencing the genome of Ozark chinquapin (C. ozarkensis) will be an important tool since this species of chestnuts is resistant to the gall wasp (Anagnostakis, 2001).
The response of plants to abiotic stress is complex, and its complexity rises in tree species with long life cycles as chestnuts. In the case of C. sativa, there is a lack of systematic research on expressed genes and proteins, and involved metabolites and microbiome, allied to morphological and physiological responses to abiotic stresses. More studies in these subjects would permit directional genetic modification strategies for a more expedited species improvement besides breeding strategies (Figure 1), to cope with the rapidly advancing global climate change.
The chestnut species with more reports on abiotic stresses is C. sativa, most of them related to drought. Advances have been made in QTL identification and MAS has been used, however, a small number of genes involved in drought tolerance have been identified. The molecular markers used, reflect a limited part of the genome for such a complex stress. GBS of large sets of individuals was already used in Castanea species. This cost-effective technique could potentially improve the estimation of genetic diversity based on hundreds to thousands of genetic markers (Müller and Gailing, 2019).
Ultimately, global climate change will differently affect the ecosystems in which chestnut stands. Chestnut may adapt via alterations of physical traits, or it will occupy other ecosystems in new favorable geographical locations. The latter may be the most probable hypothesis (Pérez-Girón et al., 2020), which may guide the establishment of new chestnut orchards. The established regions of chestnut provenance are at risk of being seriously affected or even eliminated with climate change (Pérez-Girón et al., 2020). Their resilience and adaptation will depend on the extension of the climatic variations.
The contributions gathered for the past 40 years have given chestnut researchers great tools to support restoration and programs for developing sustainable control measures of biotic and abiotic stresses. This research also represents valuable knowledge that may be applied to other forest species, mainly related members of the Fagaceae family.
PF wrote the introduction. PF and MC compiled and wrote the information regarding the biotic stresses. SS compiled and wrote the information regarding the abiotic stresses. RC compiled the work done on Dryocosmus kuriphilus. All authors reviewed the successive drafts of the manuscript, contributed to the article, and approved the submitted version.
This publication was funded by Fundação para a Ciência e Tecnologia (FCT/MCTES/PIDDAC, Portugal) under the project 414103 FCT-Lx-FEDER-28760, Ph.D. grant SFRH/BD/115424/2016 (awarded to PF) and work contract 2477 of the contract-program 12345/20181 defined by DL nr. 57/2016 (awarded to SS).
The authors would like to thank Alejandro Solla and Javier Dorado [Faculty of Forestry, Institute for Dehesa Research (INDEHESA), Universidad de Extremadura, Spain] for gently providing images for Figures 5, 6.
The authors declare that the research was conducted in the absence of any commercial or financial relationships that could be construed as a potential conflict of interest.
All claims expressed in this article are solely those of the authors and do not necessarily represent those of their affiliated organizations, or those of the publisher, the editors and the reviewers. Any product that may be evaluated in this article, or claim that may be made by its manufacturer, is not guaranteed or endorsed by the publisher.
Acquadro, A., Torello Marinoni, D., Sartor, C., Dini, F., Macchio, M., and Botta, R. (2020). Transcriptome characterization and expression profiling in chestnut cultivars resistant or susceptible to the gall wasp Dryocosmus kuriphilus. Mol. Genet. Genomics 295, 107–120. doi: 10.1007/s00438-019-01607-2
Alcaide, F., Solla, A., Cuenca, B., and Martín, M. Á (2022). Molecular evidence of introgression of Asian germplasm into a natural Castanea sativa forest in Spain. Forestry 95, 95–104. doi: 10.1093/forestry/cpab030
Alcaide, F., Solla, A., Mattioni, C., Castellana, S., and Martín, M. Á (2019). Adaptive diversity and drought tolerance in Castanea sativa assessed through EST-SSR genic markers. Forestry 92, 287–296. doi: 10.1093/FORESTRY/CPZ007
Alessandri, S., Krznar, M., Ajolfi, D., Cabrer, A. M. R., Pereira-Lorenzo, S., and Dondini, L. (2020). Genetic Diversity of Castanea sativa Mill. Accessions from the Tuscan-Emilian Apennines and Emilia Romagna Region (Italy). Agronomy 10:1319. doi: 10.3390/AGRONOMY10091319
Allario, T., Brumos, J., Colmenero-Flores, J. M., Iglesias, D. J., Pina, J. A., Navarro, L., et al. (2013). Tetraploid Rangpur lime rootstock increases drought tolerance via enhanced constitutive root abscisic acid production. Plant Cell Environ. 36, 856–868. doi: 10.1111/PCE.12021
Amudha, J., and Balasubramani, G. (2011). Recent molecular advances to combat abiotic stress tolerance in crop plants. Biotechnol. Mol. Biol. Rev. 6, 31–58. doi: 10.3389/fpls.2017.01768
Anagnostakis, S. L. (1987). Chestnut Blight: The Classical Problem of an Introduced Pathogen. Mycologia 79:23. doi: 10.2307/3807741
Anagnostakis, S. L. (1992). Measuring resistance of chestnut trees to chestnut blight. Can. J. of Forest Res. 22, 568–571. doi: 10.1139/x92-075
Anagnostakis, S. L. (2001). The effect of multiple importations of pests and pathogens on a native tree. Biol. Invasions 3, 245–254. doi: 10.1023/A:1015205005751
Anagnostakis, S. L. (2012). Chestnut Breeding in the United States for Disease and Insect Resistance. Plant Dis. 96, 1392–1403. doi: 10.1094/PDIS-04-12-0350-FE
Aryal, P. (2017). Evaluation of Ecto-Mycorrhizae as a Determinant of Chestnut Growth and Stress Response. Ph.D thesis Charleston, IL: Eastern Illinois University.
Badenes, M. L., Fernández i Martí, A., Ríos, G., and Rubio-Cabetas, M. J. (2016). Application of genomic technologies to the breeding of trees. Front Genetics 7:198. doi: 10.3389/FGENE.2016.00198/BIBTEX
Baier, K., Maynard, C., Powell, W., Barakat, A., Staton, M., Cheng, C.-H., et al. (2012). Early flowering in chestnut species induced under high dose light in growth chambers. J. Am. Chestnut. Foundation 12, 8–10. doi: 10.1186/1471-2229-12-38
Barakat, A., Diloreto, D. S., Zhang, Y., Smith, C., Baier, K., Powell, W. A., et al. (2009). Comparison of the transcriptomes of American chestnut (Castanea dentata) and Chinese chestnut (Castanea mollissima) in response to the chestnut blight infection. BMC Plant Biol 9:51 doi: 10.1186/1471-2229-9-51
Barakat, A., Staton, M., Cheng, C.-H., Park, J., Yassin, N. B. M., Ficklin, S., et al. (2012). Chestnut resistance to the blight disease: Insights from transcriptome analysis. BMC Plant Biol. 12:38. doi: 10.1186/1471-2229-12-38
Battisti, A., Benvegnù, I., Colombari, F., and Haack, R. A. (2014). Invasion by the chestnut gall wasp in Italy causes significant yield loss in Castanea sativa nut production. Agricultural Forest Entomol. 16, 75–79. doi: 10.1111/afe.12036
Bauerle, W. L., Wang, G. G., Bowden, J. D., and Hong, C. M. (2006). An analysis of ecophysiological responses to drought in American Chestnut. Ann. Forest Sci. 63, 833–842. doi: 10.1051/FOREST:2006066
Bonsignore, C. P., Vizzari, G., Vono, G., and Bernardo, U. (2020). Short-Term Cold Stress Affects Parasitism on the Asian Chestnut Gall Wasp Dryocosmus kuriphilus. Insects 11:841. doi: 10.3390/INSECTS11120841
Bosso, L., Scelza, R., Varlese, R., Meca, G., Testa, A., Rao, M. A., et al. (2016). Assessing the effectiveness of Byssochlamys nivea and Scopulariopsis brumptii in pentachlorophenol removal and biological control of two Phytophthora species. Fungal Biol. 120, 645–653. doi: 10.1016/J.FUNBIO.2016.01.004
Bouffartigue, C., Debille, S., Fabreguette, O., Cabrer, A. R., Pereira-Lorenzo, S., Flutre, T., et al. (2020). High admixture between forest an cultivated chestnut (Castanea sativa Mill.) in France. bioRxiv [Preprint]. doi: 10.1101/792259
Branzanti, B., Rocca, E., Pisi, A., Branzanti, M. B., Rocca, E., and Pisi, A. (1999). Effect of ectomycorrhizal fungi on chestnut ink disease. Mycorrhiza 9, 103–109. doi: 10.1007/S005720050007
Breisch, H., Boutitie, A., Reyne, J., Salesses, G., and Vaysse, P. (1995). Châtaignes et marrons. Paris, RA: CTIFL Centre Technique des Fruits et Légumes
Brown, C. E., Mickelbart, M. V., and Jacobs, D. F. (2014). Leaf physiology and biomass allocation of backcross hybrid American chestnut (Castanea dentata) seedlings in response to light and water availability. Tree Physiol. 34, 1362–1375. doi: 10.1093/TREEPHYS/TPU094
Brussino, G., Bosio, G., Baudino, M., Giordano, R., Ramello, F., and Melika, G. (2002). Dangerous exotic insect for the European chestnut. Inf. Agrario 58, 59–61.
Bryner, S. F., Rigling, D., and Brunner, P. C. (2012). Invasion history and demographic pattern of Cryphonectria hypovirus 1 across European populations of the chestnut blight fungus. Ecol. Evol. 2, 3227–3241. doi: 10.1002/ece3.429
Burgess, T. I., Scott, J. K., Mcdougall, K. L., Stukely, M. J. C., Crane, C., Dunstan, W. A., et al. (2017). Current and projected global distribution of Phytophthora cinnamomi, one of the world’s worst plant pathogens. Glob. Change Biol. 23, 1661–1674. doi: 10.1111/gcb.13492
Burnham, C., French, D., and Rutter, P. (1986). Breeding blight-resistant chestnuts. Plant Breed Rev., 4, 347–397
Camisón, Á, Ángela Martín, M., Dorado, F. J., Moreno, G., and Solla, A. (2020). Changes in carbohydrates induced by drought and waterlogging in Castanea sativa. Trees Struct. Funct. 34, 579–591. doi: 10.1007/S00468-019-01939-X/FIGURES/6
Camisón, Á, Martín, M. Á, Flors, V., Sánchez-Bel, P., Pinto, G., Vivas, M., et al. (2021). Exploring the use of scions and rootstocks from xeric areas to improve drought tolerance in Castanea sativa Miller. Environ. Exp. Bot. 187:104467. doi: 10.1016/J.ENVEXPBOT.2021.104467
Camisón, Á, Martín, M. Á, Sánchez-Bel, P., Flors, V., Alcaide, F., Morcuende, D., et al. (2019). Hormone and secondary metabolite profiling in chestnut during susceptible and resistant interactions with Phytophthora cinnamomi. J. Plant Physiol. 241:153030. doi: 10.1016/J.JPLPH.2019.153030
Camus, A. (1929). Les chataigniers. Monographie des genres Castanea et Castanopsis. Paris: Paul Lechevalier.
Carlile, M. J. (1983). “Motility, taxis, and tropism in Phytophthora,” in Phytophthora: its Biology, Ecology, and Pathology. St. Paul, MN, USA: The American Phytopathological Society, eds D. C. Erwin, S. Barticki-Garcia, and P. H. Tsao 95–107. doi: 10.3/JQUERY-UI.JS
Carlson, E., Stewart, K., Baier, K., McGuigan, L., Culpepper, T., and Powell, W. (2021). Pathogen-induced expression of a blight tolerance transgene in American chestnut. Mol. Plant Pathol. 23, 370–382. doi: 10.1111/MPP.13165
Carneiro-Carvalho, A., Anjos, R., Aires, A., Marques, T., Pinto, T., and Gomes-Laranjo, J. (2019). Ecophysiological study of the impact of SiK§fertilization on Castanea sativa Mill. seedling tolerance to high temperature. Photosynthetica 57, 1165–1175. doi: 10.32615/PS.2019.099
Carraway, D., Wilde, H., and Merkle, S. (1994). Somatic embryogenesis and gene transfer in American chestnut. J. Am. Chestnut. Found. 8, 29–33.
Casasoli, M., Derory, J., Morera-Dutrey, C., Brendel, O., Porth, I., Guehl, J.-M., et al. (2006). Comparison of quantitative trait loci for adaptive traits between oak and chestnut based on an expressed sequence tag consensus map. Genetics 172, 533–546. doi: 10.1534/genetics.105.048439
Casasoli, M., Pot, D., Plomion, C., Monteverdi, M. C., Barreneche, T., Lauteri, M., et al. (2004). Identification of QTLs affecting adaptive traits in Castanea sativa Mill. Plant Cell Environ. 27, 1088–1101. doi: 10.1111/j.1365-3040.2004.01214.x
Castellana, S., Martin, M. Á, Solla, A., Alcaide, F., Villani, F., Cherubini, M., et al. (2021). Signatures of local adaptation to climate in natural populations of sweet chestnut (Castanea sativa Mill.) from southern Europe. Ann. Forest Sci. 78:27. doi: 10.1007/S13595-021-01027-6/FIGURES/12
Chen, C., Sun, Q., Narayanan, B., Nuss, D. L., and Herzberg, O. (2010). Structure of Oxalacetate Acetylhydrolase, a Virulence Factor of the Chestnut Blight Fungus. J. Biol. Chem. 285:26685. doi: 10.1074/JBC.M110.117804
Choudhury, M., Brahma, R., and Ahmed, P. (2020). Silicon nutrition for alleviation of abiotic stress in plants: A review. J. Pharmacogn. Phytochem. 9, 1374–1381.
Christensen, J. H., and Christensen, O. B. (2003). Severe summertime flooding in Europe. Nature 421, 805–806. doi: 10.1038/421805a
Ciordia, M., Feito, I., Pereira-Lorenzo, S., Fernández, A., and Majada, J. (2012). Adaptive diversity in Castanea sativa Mill. half-sib progenies in response to drought stress. Environ. Exp. Bot. 78, 56–63. doi: 10.1016/J.ENVEXPBOT.2011.12.018
Cipollini, M., Dingley, N. R., Felch, P., and Maddox, C. (2017). Evaluation of phenotypic traits and blight-resistance in an American chestnut backcross orchard in Georgia. Glob. Ecol. Conserv. 10, 1–8. doi: 10.1016/J.GECCO.2017.01.004
Clark, S. L., Schlarbaum, S. E., Saxton, A. M., and Baird, R. (2019). Eight-year blight (Cryphonectria parasitica) resistance of backcross-generation American chestnuts (Castanea dentata) planted in the southeastern United States. Forest Ecol. Manag. 433, 153–161. doi: 10.1016/J.FORECO.2018.10.060
Corredoira, E., Martínez, M., Cernadas, M., and San José, M. (2017). Application of Biotechnology in the Conservation of the Genus Castanea. Forests 8:394. doi: 10.3390/f8100394
Corredoira, E., Montenegro, D., San-José, M. C., Vieitez, A. M., and Ballester, A. (2004). Agrobacterium-mediated transformation of European chestnut embryogenic cultures. Plant Cell Rep. 23, 311–318. doi: 10.1007/s00299-004-0804-0
Corredoira, E., San José, M. C., Vieitez, A. M., Allona, I., Aragoncillo, C., and Ballester, A. (2016). Agrobacterium-mediated transformation of European chestnut somatic embryos with a Castanea sativa (Mill.) endochitinase gene. New Forests 47, 669–684. doi: 10.1007/s11056-016-9537-5
Corredoira, E., Valladares, S., Allona, I., Aragoncillo, C., Vieitez, A. M., and Ballester, A. (2012). Genetic transformation of European chestnut somatic embryos with a native thaumatin-like protein (CsTL1) gene isolated from Castanea sativa seeds. Tree Physiol. 32, 1389–402. doi: 10.1093/treephys/tps098
Costa, R., Santos, C., Tavares, F., Machado, H., Gomes-Laranjo, J., Kubisiak, T., et al. (2011). Mapping and transcriptomic approches implemented for understanding disease resistance to Phytophthora cinammomi in Castanea sp. BMC Proc. 5:O18 doi: 10.1186/1753-6561-5-S7-O18
Crandall, B. S., Gravatt, G. F., and Ryan, M. M. (1945). Root disease of Castanea species and some coniferous and broadleaf nursery stocks, caused by Phytophthora cinnamomi. Phytopathology 35, 162–180.
Crouch, J. A., Dawe, A., Aerts, A., Barry, K., Churchill, A. C. L., Grimwood, J., et al. (2020). Genome sequence of the chestnut blight fungus Cryphonectria parasitica EP155: A fundamental resource for an archetypical invasive plant pathogen. Phytopathology 110, 1180–1188. doi: 10.1094/PHYTO-12-19-0478-A/ASSET/IMAGES/LARGE/PHYTO-12-19-0478-A_T1.JPEG
Cuenca, B., Fernández, M., and Ocaña, L. (2009). “Testaje de resistencia a Phytophthora cinnamomi de genotipos adultos seleccionados de Castanea sativa Mill,” in Proceedings of the 5th Congreso Forestal Español, Avila, 1–10.
Cuestas, M. I., Mattioni, C., Martín, L. M., Vargas-Osuna, E., Cherubini, M., and Martín, M. A. (2017). Short communication: Functional genetic diversity of chestnut (Castanea sativa Mill.) populations from southern Spain. Forest Syst. 26:eSC06. doi: 10.5424/FS/2017263-11547
de Ollas, C., Hernando, B., Arbona, V., and Gómez-Cadenas, A. (2013). Jasmonic acid transient accumulation is needed for abscisic acid increase in citrus roots under drought stress conditions. Physiol. Plantarum 147, 296–306. doi: 10.1111/J.1399-3054.2012.01659.X
Diagne, N., Ngom, M., Djighaly, P. I., Fall, D., Hocher, V., and Svistoonoff, S. (2020). Roles of Arbuscular Mycorrhizal Fungi on Plant Growth and Performance: Importance in Biotic and Abiotic Stressed Regulation. Diversity 12:370. doi: 10.3390/D12100370
Dini, F., Sartor, C., and Botta, R. (2012). Detection of a hypersensitive reaction in the chestnut hybrid ‘Bouche de Bétizac’ infested by Dryocosmus kuriphilus Yasumatsu. Plant Physiol. Biochem. 60, 67–73. doi: 10.1016/j.plaphy.2012.07.023
Dinis, L. T., Peixoto, F., Pinto, T., Costa, R., Bennett, R. N., and Gomes-Laranjo, J. (2011a). Study of morphological and phenological diversity in chestnut trees (‘Judia’ variety) as a function of temperature sum. Environ. Exp. Bot. 70, 110–120. doi: 10.1016/J.ENVEXPBOT.2010.08.003
Dinis, L. T., Peixoto, F., Zhang, C., Martins, L., Costa, R., and Gomes-Laranjo, J. (2011b). Physiological and biochemical changes in resistant and sensitive chestnut (Castanea) plantlets after inoculation with Phytophthora cinnamomi. Physiol. Mol. Plant Pathol. 75, 146–156. doi: 10.1016/j.pmpp.2011.04.003
Diskin, M., Steiner, K. C., and Hebard, F. V. (2006). Recovery of American chestnut characteristics following hybridization and backcross breeding to restore blight-ravaged Castanea dentata. Forest Ecol. Manag. 223, 439–447. doi: 10.1016/J.FORECO.2005.12.022
Dorado, F. J., Solla, A., Alcaide, F., and Martín, M. A. (2022). Assessing heat stress tolerance in Castanea sativa. Forestry cac021. doi: 10.1093/forestry/cpac021
Engelbrecht, J., Duong, T. A., Prabhu, S. A., Seedat, M., and van den Berg, N. (2021). Genome of the destructive oomycete Phytophthora cinnamomi provides insights into its pathogenicity and adaptive potential. BMC Genomics 22:302. doi: 10.1186/s12864-021-07552-y
Eppo Global Database (2022). Cryphonectria parasitica. Distribution. Available online at: https://gd.eppo.int/taxon/ENDOPA/distribution (accessed on Feb 15, 2022).
Eriksson, G., Pliura, A., Villani, F., Bucci, G., Casasoli, M., Cherubini, M., et al. (2005). Management of genetic resources of the multi-purpose tree species castanea sativa mill. Acta Horticulturae 693, 373–386. doi: 10.17660/ACTAHORTIC.2005.693.47
European Commission (2022). Consequences of climate change. Available online at: https://ec.europa.eu/clima/climate-change/climate-change-consequences_en (accessed on May 21, 2022).
Evangelisti, E., Gogleva, A., Hainaux, T., Doumane, M., Tulin, F., Quan, C., et al. (2017). Time-resolved dual transcriptomics reveal early induced Nicotiana benthamiana root genes and conserved infection-promoting Phytophthora palmivora effectors. BMC Biol. 15:39. doi: 10.1186/s12915-017-0379-1
Fei, S., Liang, L., Paillet, F. L., Steiner, K. C., Fang, J., Shen, Z., et al. (2012). Modelling chestnut biogeography for American chestnut restoration. Divers. Distrib. 18, 754–768. doi: 10.1111/j.1472-4642.2012.00886.x
Fernandes, P., Amaral, A., Colavolpe, B., Balonas, D., Serra, M., Pereira, A., et al. (2020a). Propagation of New Chestnut Rootstocks with Improved Resistance to Phytophthora cinnamomi–New Cast Rootstocks. Silva Lusit. 28, 15–29. doi: 10.1051/silu/20202801015
Fernandes, P., Amaral, A., Colavolpe, B., Pereira, A., and Costa, R. (2021a). Avaliação agronómica de genótipos de castanheiro selecionados do programa de melhoramento genético para a resistência à tinta. Vida Rural 76–82.
Fernandes, P., Machado, H., do Céu Silva, M., and Costa, R. L. (2021b). A histopathological study reveals new insights into responses of chestnut (Castanea spp.) to root infection by Phytophthora cinnamomi. Phytopathology 111, 345–355. doi: 10.1094/PHYTO-04-20-0115-R
Fernandes, P., Tedesco, S., da Silva, I. V., Santos, C., Machado, H., and Costa, R. L. (2020b). A new clonal propagation protocol develops quality root systems in chestnut. Forests 11:826. doi: 10.3390/F11080826
Fernandez-Lopez, J. (2011). Identification of the genealogy of interspecific hybrids between Castanea sativa, Castanea crenata and Castanea mollissima. Forest Syst. 20, 65–80. doi: 10.5424/fs/2011201-9136
Fernández-López, J., Vazquez-Ruiz-De-Ocenda, R. A., Díaz-Vázquez, R., and Pereira-Lorenzo, S. (2001). Evaluation of resistance of Castanea sp. clones to Phytophthora sp. using excised chestnut shoots. For. Snow Landsc. Res. 76, 451–454.
Fernandez-Lopez, J., Zas, R., Diaz, R., Villani, F., Cherubini, M., Aravanopoulos, F. A., et al. (2005). Geographic variability among extreme european wild chestnut populations. Acta Hortic. 693, 181–186. doi: 10.17660/ACTAHORTIC.2005.693.21
Food and Agriculture Organization of the United Nations (2022). Food and agriculture data. Available online at: http://www.fao.org/faostat/en/#data/QC (accessed on Feb 15, 2022)
Fulbright, D. W., Stadt, S., Medina-Mora, C., Mandujano, M., Donis-González, I. R., and Serdar, U. (2014). Kernel breakdown appears when hybrid Castanea cultivars are pollinized by Castanea mollissima. Acta Hortic. 1019, 91–97.
García-Olmedo, F., Molina, A., Segura, A., and Moreno, M. (1995). The defensive role of nonspecific lipid-transfer proteins in plants. Trends Microbiol. 3, 72–74. doi: 10.1016/S0966-842X(00)88879-4
Glenz, C., Schlaepfer, R., Iorgulescu, I., and Kienast, F. (2006). Flooding tolerance of Central European tree and shrub species. Forest Ecol. Manag. 235, 1–13. doi: 10.1016/J.FORECO.2006.05.065
Gomes Guerreiro, M. (1948). Alguns estudos do género Castanea. Relatório. Tomos I e II, 111, Vol. XV. Alcobaça: Publicação da Direcção Geral dos Serviços Florestais e Aquícolas, Tipografia Alcobacence Lda.
Gomes Guerreiro, M. (1957). Castanheiros. Alguns estudos sobre a sua ecologia e o seu melhoramento genético. Dissertation presented for the title of Aggregate Professor to the Instituto Superior de Agronomia. Lisboa.
Gomes Laranjo, J., Ferreira Cardoso, J., Portela, E., and Abreu, C. (2007). Castanheiros. Edição Programa AGRO 499–Contributo para difusão do conhecimento das cultivares portuguesas de castanheiro. 373.
Gomes-Laranjo, J., Araujo-Alves, J., Ferreira-Cardoso, J., Pimentel-Pereira, M., Abreu, C. G., and Torres-Pereira, J. (2004). Effect of Chestnut Ink Disease on Photosynthetic Performance. J. Phytopathol. 152, 138–144. doi: 10.1111/j.1439-0434.2004.00814.x
Gomes-Laranjo, J., Dinis, L. T., Marques, T., Mota, M., Carvalho, A., Pinto, T., et al. (2018). Increasing chestnut resilience to climate change with innovative management practices. Acta Hortic. 1220, 163–176. doi: 10.17660/ACTAHORTIC.2018.1220.24
Gomes-Laranjo, J., Dinis, L., Martins, L., Portela, E., Pinto, T., Ara, M. C., et al. (2012). “Characterization of chestnut behavior with photosynthetic traits,” in Applied Photosynthesis, ed. M. M. Najafpour (London: IntechOpen). doi: 10.5772/26227
Grabherr, M. G., Haas, B. J., Yassour, M., Levin, J. Z., Thompson, D. A., Amit, I., et al. (2011). Full-length transcriptome assembly from RNA-Seq data without a reference genome. Nat. Biotechnol. 29, 644–652. doi: 10.1038/NBT.1883
Griffin, G. J. (1983). Survival of American Chestnut Trees: Evaluation of Blight Resistance and Virulence in Endothia parasitica. Phytopathology 73:1084. doi: 10.1094/PHYTO-73-1084
Hardham, A. R. (2005). Phytophthora cinnamomi. Mol. Plant Pathol. 6, 589–604. doi: 10.1111/j.1364-3703.2005.00308.x
Hardham, A. R., and Blackman, L. M. (2018). Phytophthora cinnamomi. Mol. Plant Pathol. 19, 260–285. doi: 10.1111/mpp.12568
Havir, E. A., and Anagnostakis, S. L. (1983). Oxalate production by virulent but not by hypovirulent strains of Endothia parasitica. Physiol. Plant Pathol. 23, 369–376. doi: 10.1016/0048-4059(83)90021-8
Hebard, F. V., Griffin, G. J., and Elkins, J. R. (1984). Developmental Histopathology of Cankers Incited by Hypovirulent and Virulent Isolates of Endothia parasitica on Susceptible and Resistant Chestnut Trees. Phytopathology 74:140. doi: 10.1094/Phyto-74-140
Huang, H., Carey, W. A., Dane, F., and Norton, J. D. (1996). Evaluation of Chinese chestnut cultivars for resistance to Cryphonectria parasitica. Plant Dis. 80, 45–47. doi: 10.1094/PD-80-0045
Jacobs, D. F., Dalgleish, H. J., and Nelson, C. D. (2013). A conceptual framework for restoration of threatened plants: The effective model of American chestnut (Castanea dentata) reintroduction. New Phytol. 197, 378–393. doi: 10.1111/nph.12020
Jeffers, S. N., James, J. B., and Sisco, P. H. (2009). “Screening for resistance to Phytophthora cinnamomi in hybrid seedlngs of American chestnut,” in in Proceedings of the Fourth Meeting of the International Union of Forest Research Organizations (IUFRO) Working Party S07.02.09, (Monterey), 188–194.
Kalemba, E. M., and Pukacka, S. (2007). 3 LEA PROTEINS AND sHSPs IN SEED PROTECTION Possible roles of LEA proteins and sHSPs in seed protection: A short review. Biological. Lett. 44, 3–16.
Kamoun, S., Furzer, O., Jones, J. D. G., Judelson, H. S., Ali, G. S., Dalio, R. J. D., et al. (2015). The Top 10 oomycete pathogens in molecular plant pathology. Mol. Plant Pathol. 16, 413–434. doi: 10.1111/mpp.12190
Kato, K., and Hijii, N. (1997). Effects of gall formation by Dryocosmus kuriphilus Yasumatsu (Hym., Cynipidae) on the growth of chestnut trees. J. Appl. Entomol. 121, 9–15. doi: 10.1111/j.1439-0418.1997.tb01363.x
Kim, J. S., Kim, K. A., Oh, T. R., Park, C. M., and Kang, H. (2008). Functional Characterization of DEAD-Box RNA Helicases in Arabidopsis thaliana under Abiotic Stress Conditions. Plant Cell Physiol. 49, 1563–1571. doi: 10.1093/PCP/PCN125
Ko, W. H., Chang, H. S., and Su, H. J. (1978). Isolates of Phytophthora cinnamomi from Taiwan as evidence for an Asian origin of the species. Trans. Br. Mycol. Soc. 71, 496–499. doi: 10.1016/S0007-1536(78)80080-1
Kreuzwieser, J., and Rennenberg, H. (2014). Molecular and physiological responses of trees to waterlogging stress. Plant Cell Environ. 37, 2245–2259. doi: 10.1111/PCE.12310
Kubisiak, T. (2010). “QTL analysis of Phytophthora resistance in a BC1 population,” in NE-1033 Annual Meeting Minutes, (Maggie Valley).
Kubisiak, T. L., and Roberds, J. H. (2006). “Genetic structure of American chestnut populations based on neutral DNA markers. in Restoration of American Chestnut to Forest Lands,”in Proceedings of a Conference and Workshop held at The North Carolina Arboretum, Asheville, North Carolina, U. S. A., May 4–6, 2004. Natural Resources Report NPS/NCR/CUE/NRR–2006/001, 109–122, (Washington, DC: National Park Service).
Kubisiak, T. L., Hebard, F. V., Nelson, C. D., Zhang, J., Bernatzky, R., Huang, H., et al. (1997). Molecular Mapping of Resistance to Blight in an Interspecific Cross in the Genus Castanea. Phytopathology 87, 751–759. doi: 10.1094/PHYTO.1997.87.7.751
Kubisiak, T. L., Nelson, C. D., Staton, M. E., Zhebentyayeva, T., Smith, C., Olukolu, B. A., et al. (2013). A transcriptome-based genetic map of Chinese chestnut (Castanea mollissima) and identification of regions of segmental homology with peach (Prunus persica). Tree Genet. Genomes 1019, 557–571. doi: 10.1007/s11295-012-0579-3
Kundzewicz, Z. W., Ulbrich, U., Brücher, T., Graczyk, D., Krüger, A., Leckebusch, G. C., et al. (2005). Summer Floods in Central Europe–Climate Change Track? Nat. Hazards 36, 165–189. doi: 10.1007/S11069-004-4547-6
Kunert, K. J., van Wyk, S. G., Cullis, C. A., Vorster, B. J., and Foyer, C. H. (2015). Potential use of phytocystatins in crop improvement, with a particular focus on legumes. J. Exp. Bot. 66, 3559–3570. doi: 10.1093/JXB/ERV211
Lang, P., Dane, F., Kubisiak, T. L., and Huang, H. (2007). Molecular evidence for an Asian origin and a unique westward migration of species in the genus Castanea via Europe to North America. Mol. Phylogenet. Evol. 43, 49–59. doi: 10.1016/J.YMPEV.2006.07.022
Latijnhouwers, M., de Wit, P. J. G. M., and Govers, F. (2003). Oomycetes and fungi: Similar weaponry to attack plants. Trends Microbiol. 11, 462–469. doi: 10.1016/J.TIM.2003.08.002
Lauteri, M., Pliura, A., Monteverdi, M. C., Brugnoli, E., Villani, F., and Eriksson, G. (2004). Genetic variation in carbon isotope discrimination in six European populations of Castanea sativa Mill. originating from contrasting localities. J. Evol. Biol. 17, 1286–1296. doi: 10.1111/J.1420-9101.2004.00765.X
Lee, J. H., and Kim, W. T. (2011). Regulation of abiotic stress signal transduction by E3 ubiquitin ligases in Arabidopsis. Mol. Cells 31, 201–208. doi: 10.1007/S10059-011-0031-9
Limera, C., Sabbadini, S., Sweet, J. B., and Mezzetti, B. (2017). New biotechnological tools for the genetic improvement of major woody fruit species. Front. Plant Sci. 8:1418. doi: 10.3389/FPLS.2017.01418/BIBTEX
Liu, Z., Bi, W.-L., Shukla, M. R., and Saxena, P. K. (2022). In Vitro Technologies for American Chestnut (Castanea dentata (Marshall) Borkh) Conservation. Plants 11:464. doi: 10.3390/plants11030464
Ma, J. F. (2004). Role of silicon in enhancing the resistance of plants to biotic and abiotic stresses. Soil Sci. Plant Nutr. 50, 11–18. doi: 10.1080/00380768.2004.10408447
Manivannan, A., and Ahn, Y. K. (2017). Silicon regulates potential genes involved in major physiological processes in plants to combat stress. Front. Plant Sci. 8:1346. doi: 10.3389/FPLS.2017.01346/BIBTEX
Marinoni, D. T., Nishio, S., Valentini, N., Shirasawa, K., Acquadro, A., Portis, E., et al. (2020). Development of High-Density Genetic Linkage Maps and Identification of Loci for Chestnut Gall Wasp Resistance in Castanea spp. Plants 9:1048. doi: 10.3390/PLANTS9081048
Martin, M. A., Mattioni, C., Cherubini, M., Taurchini, D., and Villani, F. (2010). Genetic diversity in European chestnut populations by means of genomic and genic microsatellite markers. Tree Genet. Genomes 6, 735–744. doi: 10.1007/s11295-010-0287-9
Martínez-Sancho, E., Navas, L. K. V., Seidel, H., Dorado-Liñán, I., and Menzel, A. (2017). Responses of Contrasting Tree Functional Types to Air Warming and Drought. Forests 8:450. doi: 10.3390/F8110450
Martins, L., Anjos, R., Costa, R., and Gomes-Laranjo, J. (2009). “Colutad. Um clone de castanheiro resistente à doença da tinta,” in Castanheiros. Técnicas e Práticas, eds J. Gomes-Laranjo, F. Peixoto, and J. Ferreira-Cardoso (Vila Real: Pulido Consulting - Indústria Criativa and Universidade Trás-os-Montes e Alto Douro), 135–142.
Maurel, M., Robin, C., Capdevielle, X., Loustau, D., and Desprez-Loustau, M. L. (2001). Effects of variable root damage caused by Phytophthora cinnamomi on water relations of chestnut saplings. Ann. For. Sci. 58, 639–651. doi: 10.1051/FOREST:2001151
Maurel, M., Robin, C., Simonneau, T., Loustau, D., Dreyer, E., and Desprez-Loustau, M. L. (2004). Stomatal conductance and root-to-shoot signalling in chestnut saplings exposed to Phytophthora cinnamomi or partial soil drying. Funct. Plant Biol. 31, 41–51. doi: 10.1071/FP03133
McGuigan, L., Fernandes, P., Oakes, A., Stewart, K., and Powell, W. (2020). Transformation of american chestnut (Castanea dentata (marsh.) borkh) using RITA® temporary immersion bioreactors and we vitro containers. Forests 11:1196. doi: 10.3390/f11111196
Mellano, M. G., Beccaro, G. L., Donno, D., Marinoni, D. T., Boccacci, P., Canterino, S., et al. (2012). Castanea spp. biodiversity conservation: Collection and characterization of the genetic diversity of an endangered species. Genet. Resour. Crop Evol. 59, 1727–1741. doi: 10.1007/s10722-012-9794-x
Menéndez-Miguélez, M., Álvarez-Álvarez, P., Majada, J., and Canga, E. (2015). Effects of soil nutrients and environmental factors on site productivity in Castanea sativa Mill. coppice stands in NW Spain. New For. 46, 217–233. doi: 10.1007/S11056-014-9456-2/TABLES/2
Meyer, F. E., Shuey, L. S., Naidoo, S., Mamni, T., Berger, D. K., Myburg, A. A., et al. (2016). Dual RNA-sequencing of Eucalyptus nitens during Phytophthora cinnamomi challenge reveals pathogen and host factors influencing compatibility. Front. Plant Sci. 7:191. doi: 10.3389/fpls.2016.00191
Meyer, J. B., Gallien, L., and Prospero, S. (2015). Interaction between two invasive organisms on the European chestnut: Does the chestnut blight fungus benefit from the presence of the gall wasp? FEMS Microbiol. Ecol. 91:fiv122. doi: 10.1093/femsec/fiv122
Míguez-Soto, B., Fernández-Cruz, J., and Fernández-López, J. (2019). Mediterranean and Northern Iberian gene pools of wild Castanea sativa Mill. are two differentiated ecotypes originated under natural divergent selection. PLoS One 14:e0211315. doi: 10.1371/JOURNAL.PONE.0211315
Milburn, M., and Gravatt, G. F. (1932). Preliminary note on a Phytophthora root disease of chestnut. Phytopathology 22, 977–978.
Milgroom, M. G., and Cortesi, P. (2004). Biological control of chestnut blight with hypovirulence: A critical analysis. Annu. Rev. Phytopathol. 42, 311–338. doi: 10.1146/ANNUREV.PHYTO.42.040803.140325
Miranda-Fontaíña, M. E., Fernández-López, J., Vettraino, A. M., and Vannini, A. (2007). Resistance of Castanea clones to Phytophthora cinnamomi: Testing and genetic control. Silvae Genet. 56, 11–21. doi: 10.1515/sg-2007-0002
Molina, F., and Viéitez, E. (1967). Defense du chataignier contre ses maladies en Espagne. Atti Convegno Internazionallesul Castagno. Cuneo: Ottobre.
Moradpour, M., and Abdulah, S. N. A. (2020). CRISPR/dCas9 platforms in plants: Strategies and applications beyond genome editing. Plant Biotechnol. J. 18, 32–44. doi: 10.1111/PBI.13232
Müller, M., and Gailing, O. (2019). Abiotic genetic adaptation in the Fagaceae. Plant Biol. 21, 783–795. doi: 10.1111/PLB.13008
Murolo, S., Angelini, R. M., de, M., Faretra, F., and Romanazzi, G. (2018). Phenotypic and molecular investigations on hypovirulent cryphonectria parasitica in Italy. Plant Dis. 102, 540–545. doi: 10.1094/PDIS-04-17-0517-RE
Neves, D. M., Almeida, L. A. D. H., Santana-Vieira, D. D. S., Freschi, L., Ferreira, C. F., Soares Filho, W. D. S., et al. (2017). Recurrent water deficit causes epigenetic and hormonal changes in citrus plants. Sci. Rep. 7:13684. doi: 10.1038/s41598-017-14161-x
Newhouse, A. E., and Powell, W. A. (2021). Intentional introgression of a blight tolerance transgene to rescue the remnant population of American chestnut. Conserv. Sci. Pract. 3:e348. doi: 10.1111/CSP2.348
Newhouse, A. E., Maynard, C. A., and Powell, W. A. (2014). Chestnut Leaf Inoculation Assay as a Rapid Predictor of Blight Susceptibility. Plant Dis. 98, 4–9. doi: 10.1094/PDIS-01-13-0047-RE
Newhouse, A., Coffey, V., McGuigan, L., Oakes, A., Breda, K., Matthews, D., et al. (2020). Petition for Determination of Nonregulated Status for Blight-Tolerant Darling 58 American Chestnut (Castanea dentata). Available Online at: https://www.regulations.gov/document/APHIS-2020-0030-0002 (accessed on Oct 16, 2021).
Nishio, S., Yamamoto, T., Terakami, S., Sawamura, Y., Takada, N., Nishitani, C., et al. (2011). Novel genomic and EST-derived SSR markers in Japanese chestnuts. Sci. Hortic. 130, 838–846. doi: 10.1016/J.SCIENTA.2011.09.012
Pavese, V., Moglia, A., Corredoira, E., Martínez, M. T., Torello Marinoni, D., and Botta, R. (2021a). First Report of CRISPR/Cas9 Gene Editing in Castanea sativa Mill. Front. Plant Sci. 12:1752. doi: 10.3389/fpls.2021.728516
Pavese, V., Moglia, A., Gonthier, P., Marinoni, D. T., Cavalet-Giorsa, E., and Botta, R. (2021b). Identification of Susceptibility Genes in Castanea sativa and Their Transcription Dynamics following Pathogen Infection. Plants 10:913. doi: 10.3390/PLANTS10050913
Pereira-Lorenzo, S., Ballester, A., Corredoira, E., Vieitez, A. M., Agnanostakis, S., Costa, R., et al. (2012). “Chestnut,” in Handbook of Plant Breeding - Fruit Breeding, eds M. L. Badenes and D. H. Byrne (Berlin: Springer Science), 729–769. doi: 10.1007/978-1-4419-0763-9_19
Pereira-Lorenzo, S., Costa, R. M. L., Ramos-Cabrer, A. M., Ciordia-Ara, M., Ribeiro, C. A. M., Borges, O., et al. (2011). Chestnut cultivar diversification process in the Iberian Peninsula. Canary Islands, and Azores. Genome 54, 301–315. doi: 10.1139/g10-122
Pereira-lorenzo, S., Costa, R., Ramos-Cabrer, A. M., Ribeiro, C., Silva, M., Manzano, G., et al. (2010). Variation in grafted European chestnut and hybrids by microsatellites reveals two main origins in the Iberian Peninsula. Tree Genet. Genomes 6, 701–715. doi: 10.1007/s11295-010-0285-y
Pérez-Girón, J. C., Álvarez-Álvarez, P., Díaz-Varela, E. R., and Mendes Lopes, D. M. (2020). Influence of climate variations on primary production indicators and on the resilience of forest ecosystems in a future scenario of climate change: Application to sweet chestnut agroforestry systems in the Iberian Peninsula. Ecol. Indic. 113:106199. doi: 10.1016/J.ECOLIND.2020.106199
Perkins, M. T., Zhebentyayeva, T., Sisco, P. H., and Craddock, J. H. (2021). Genome-Wide Sequence-Based Genotyping Supports a Nonhybrid Origin of Castanea alabamensis. Syst. Bot. 46, 973–984. doi: 10.1600/036364421X16370109698524
Pernas, M., Sánchez-Monge, R., and Salcedo, G. (2000). Biotic and abiotic stress can induce cystatin expression in chestnut. FEBS Lett. 467, 206–210. doi: 10.1016/S0014-5793(00)01157-1
Pilkey, H. (2021). Developing and Optimizing Conservation Outcrossing Methods to Produce Diversified Blight-Tolerant American Chestnut Trees (Castanea Dentata [Marsh.] Borkh.), Ph.D thesis, New York NY: State University of New York-College of Environmental Science and Forestry. Syracuse.
Polin, L. D., Liang, H., Rothrock, R. E., Nishii, M., Diehl, D. L., Newhouse, A. E., et al. (2006). Agrobacterium-mediated transformation of American chestnut (Castanea dentata (Marsh.) Borkh.) somatic embryos. Plant Cell Tissue Organ Cult. 84, 69–79. doi: 10.1007/s11240-005-9002-1
Poljak, I., Idžojtić, M., Šatović, Z., Ježić, M., Ćurković-Perica, M., Simovski, B., et al. (2017). Genetic diversity of the sweet chestnut (Castanea sativa Mill.) in Central Europe and the western part of the Balkan Peninsula and evidence of marron genotype introgression into wild populations. Tree Genet. Genomes 13:18. doi: 10.1007/S11295-017-1107-2/FIGURES/3
Powell, W. A., Morley, P., King, M., and Maynard, C. A. (2007). Small stem chestnut blight resistance assay. J. Am. Chestnut Foundation 21, 34–38. doi: 10.1007/978-1-4939-1658-0_13
Powell, W. A., Newhouse, A. E., and Coffey, V. (2019). Developing Blight-Tolerant American Chestnut Trees. Cold Spring Harb. Perspect. Biol. 11:a034587. doi: 10.1101/CSHPERSPECT.A034587
Prospero, S., Conedera, M., Heiniger, U., and Rigling, D. (2006). Saprophytic Activity and Sporulation of Cryphonectria parasitica on Dead Chestnut Wood in Forests with Naturally Established Hypovirulence. Phytopathology® 96, 1337–1344. doi: 10.1094/PHYTO-96-1337
Prunet, A. (1904). Le reconstitution des Châtaigneries. Bul. Mensuel de L’Office de Reseignements Agricoles 3, 536–541.
Ramos Guedes-Lafargue, M., and Salesses, G. (1999). ink disease resistance: Some preliminary elements from the study of different crosses. Acta Hortic. 494, 355–362. doi: 10.17660/ActaHortic.1999.494.54
Rigling, D., and Prospero, S. (2018). Cryphonectria parasitica, the causal agent of chestnut blight: Invasion history, population biology and disease control. Mol. Plant Pathol. 19, 7–20. doi: 10.1111/mpp.12542
Roane, M., Griffin, G., and Elkins, J. (1986). Chestnut blight, other Endothia diseases, and the genus Endothia. Saint Paul, MIN: APS Press.
Robin, C., and Heiniger, U. (2001). Chestnut blight in Europe: Diversity of Cryphonectria parasitica, hypovirulence and biocontrol. For. Snow Landsc. Res. 76, 361–367.
Robin, C., Morel, O., Vettraino, A. M., Perlerou, C., Diamandis, S., and Vannini, A. (2006). Genetic variation in susceptibility to Phytophthora Cambivora in European chestnut (Castanea sativa). For. Ecol. Manag. 226, 199–207. doi: 10.1016/j.foreco.2006.01.035
Rothrock, R. E., Polin-McGuigan, L. D., Newhouse, A. E., Powell, W. A., and Maynard, C. A. (2007). Plate flooding as an alternative Agrobacterium-mediated transformation method for American chestnut somatic embryos. Plant Cell Tissue Organ Cult. 88, 93–99. doi: 10.1007/s11240-006-9170-7
Saiz-Fernández, I., Milenković, I., Berka, M., Černý, M., Tomšovský, M., Brzobohatý, B., et al. (2020). Integrated Proteomic and Metabolomic Profiling of Phytophthora cinnamomi Attack on Sweet Chestnut (Castanea sativa) Reveals Distinct Molecular Reprogramming Proximal to the Infection Site and Away from It. Int. J. Mol. Sci. 21:8525. doi: 10.3390/ijms21228525
Salesses, G., Ronco, L., Chauvin, J., and Chapa, J. (1993). Amélioration génétique du chataignier misse aupoint de tests d’évaluation du comportement vis-á-vis dela maladie de l’encre. L’Arboric. Fruitiére 458, 23–31.
Sandercock, A., Westbrook, J., Zhang, Q., Johnson, H., Saielli, T., Scrivani, J., et al. (2022). Whole-genome resequencing reveals the population structure, genomic diversity, and demographic history of American chestnut (Castanea dentata). bioRxiv [Preprint]. doi: 10.1101/2022.02.11.480151
Santamaría, M. E., Hasbún, R., Valera, M. J., Meijón, M., Valledor, L., Rodríguez, J. L., et al. (2009). Acetylated H4 histone and genomic DNA methylation patterns during bud set and bud burst in Castanea sativa. J. Plant Physiol. 166, 1360–1369. doi: 10.1016/J.JPLPH.2009.02.014
Santamaría, M. E., Rodríguez, R., Cañal, M. J., and Toorop, P. E. (2011). Transcriptome analysis of chestnut (Castanea sativa) tree buds suggests a putative role for epigenetic control of bud dormancy. Ann. Bot. 108, 485–498. doi: 10.1093/aob/mcr185
Santana-Vieira, D. D. S., Freschi, L., da Hora, Almeida, L. A., Moraes, D. H. S., and de, et al. (2016). Survival strategies of citrus rootstocks subjected to drought. Sci. Rep. 6:38775. doi: 10.1038/srep38775
Santhanagopalan, I., Basha, E., Ballard, K. N., Bopp, N. E., and Vierling, E. (2015). “Model Chaperones: Small Heat Shock Proteins from Plants,” in The Big Book on Small Heat Shock Proteins. Heat Shock Proteins, eds R. Tanguay and L. Hightower (Cham: Springer), 119–153. doi: 10.1007/978-3-319-16077-1_5
Santini, A., Barzanti, G. P., and Capretti, P. (2003). Susceptibility of some mesophilic hardwoods to alder phytophthora. J. Phytopathol. 151, 406–410. doi: 10.1046/j.1439-0434.2003.00739.x
Santos, C., Duarte, S., Tedesco, S., Fevereiro, P., and Costa, R. L. (2017a). Expression profiling of Castanea genes during resistant and susceptible interactions with the oomycete pathogen Phytophthora cinnamomi reveal possible mechanisms of immunity. Front. Plant Sci. 8:515. doi: 10.3389/fpls.2017.00515
Santos, C., Machado, H., Correia, I., Gomes, F., Gomes-Laranjo, J., and Costa, R. (2015a). Phenotyping Castanea hybrids for Phytophthora cinnamomi resistance. Plant Pathol. 64, 901–910. doi: 10.1111/ppa.12313
Santos, C., Nelson, C. D., Zhebentyayeva, T., Machado, H., Gomes-Laranjo, J., and Costa, R. L. (2017b). First interspecific genetic linkage map for Castanea sativa x Castanea crenata revealed QTLs for resistance to Phytophthora cinnamomi. PLoS One 12:e0184381. doi: 10.1371/journal.pone.0184381
Santos, C., Zhebentyayeva, T., Serrazina, S., Nelson, C. D., and Costa, R. (2015b). Development and characterization of EST-SSR markers for mapping reaction to Phytophthora cinnamomi in Castanea spp. Sci. Hortic. 194, 181–187. doi: 10.1016/j.scienta.2015.07.043
Sartor, C., Dini, F., Torello Marinoni, D., Mellano, M. G., Beccaro, G. L., Alma, A., et al. (2015). Impact of the Asian wasp Dryocosmus kuriphilus (Yasumatsu) on cultivated chestnut: Yield loss and cultivar susceptibility. Sci Hortic. 197, 454–460. doi: 10.1016/j.scienta.2015.10.004
Schad, C., Solignat, G., Grente, J., and Venot, P. (1952). Recherches sur le chataignier a la station de Brive. Annales de L’amélioration des plantes 3, 369–458.
Seabra, R. C., and Pais, M. S. (1998). Genetic transformation of European chestnut. Plant Cell Rep. 17, 177–182.
Sebastiana, M., Figueiredo, A., Acioli, B., Sousa, L., Pessoa, F., Baldé, A., et al. (2009). Identification of plant genes involved on the initial contact between ectomycorrhizal symbionts (Castanea sativa–European chestnut and Pisolithus tinctorius). Eur. J. Soil Biol. 45, 275–282. doi: 10.1016/J.EJSOBI.2009.02.001
Seo, D. H., Ryu, M. Y., Jammes, F., Hwang, J. H., Turek, M., Kang, B. G., et al. (2012). Roles of Four Arabidopsis U-Box E3 Ubiquitin Ligases in Negative Regulation of Abscisic Acid-Mediated Drought Stress Responses. Plant Physiol. 160, 556–568. doi: 10.1104/PP.112.202143
Serdar, Ü, Saito, T., Cuenca, B., Akyüz, B., Laranjo, J. G., Beccaro, G., et al. (2019). “Advances in cultivation of chestnuts,” in Achieving sustainable cultivation of tree nuts, (Sawston: Burleigh Dodds Science Publishing Limited), 349–388. doi: 10.19103/as.2018.0042.22
Serrazina, S., Machado, H., Costa, R. L., Duque, P., and Malhó, R. (2021). Expression of Castanea crenata Allene Oxide Synthase in Arabidopsis Improves the Defense to Phytophthora cinnamomi. Front. Plant Sci. 12:149. doi: 10.3389/fpls.2021.628697
Serrazina, S., Santos, C., Machado, H., Pesquita, C., Vicentini, R., Pais, M. S., et al. (2015). Castanea root transcriptome in response to Phytophthora cinnamomi challenge. Tree Genet. Genomes 11:6. doi: 10.1007/s11295-014-0829-7
Shirasawa, K., Nishio, S., Terakami, S., Botta, R., Marinoni, D. T., and Isobe, S. (2021). Chromosome-level genome assembly of Japanese chestnut (Castanea crenata Sieb. et Zucc.) reveals conserved chromosomal segments in woody rosids. DNA Res. 28:dsab016. doi: 10.1093/DNARES/DSAB016
Sisco, P. H., Neel, T. C., Hebard, F. V., Craddock, J. H., and Shaw, J. (2014). Cytoplasmic male sterility in interspecific hybrids between american and asian Castanea species is correlated with the american d chloroplast haplotype. Acta Hortic. 1019, 215–222. doi: 10.17660/ACTAHORTIC.2014.1019.32
Sisco, P., Kubisiak, T., and Casasoli, M. (2005). “An improved genetic map for Castanea mollissima/Castanea dentata and its relationship to the genetic map of Castanea sativa,” in Proceedings of the III International Chestnut Congress, eds C. G. Abreu, E. Rosa, and A. A. Monteiro (Leuven: Acta Horticulturae), 693.
Soto, A., Allona, I., Collada, C., Guevara, M. A., Casado, R., Rodriguez-Cerezo, E., et al. (1999). Heterologous Expression of a Plant Small Heat-Shock Protein Enhances Escherichia coli Viability under Heat and Cold Stress. Plant Physiol. 120, 521–528. doi: 10.1104/PP.120.2.521
Staton, M., Addo-Quaye, C., Cannon, N., Yu, J., Zhebentyayeva, T., Huff, M., et al. (2020). A reference genome assembly and adaptive trait analysis of Castanea mollissima ‘Vanuxem,’ a source of resistance to chestnut blight in restoration breeding. Tree Genet. Genomes 16:57. doi: 10.1007/S11295-020-01454-Y/FIGURES/10
Staton, M., Zhebentyayeva, T., Olukolu, B., Fang, G. C., Nelson, D., Carlson, J. E., et al. (2015). Substantial genome synteny preservation among woody angiosperm species: Comparative genomics of Chinese chestnut (Castanea mollissima) and plant reference genomes. BMC Genomics 16:744. doi: 10.1186/s12864-015-1942-1
Stauder, C. M., Nuss, D. L., Zhang, D.-X., Double, M. L., MacDonald, W. L., Metheny, A. M., et al. (2019). Enhanced hypovirus transmission by engineered super donor strains of the chestnut blight fungus, Cryphonectria parasitica, into a natural population of strains exhibiting diverse vegetative compatibility genotypes. Virology 528, 1–6. doi: 10.1016/j.virol.2018.12.007
Steiner, K. C., Westbrook, J. W., Hebard, F. V., Georgi, L. L., Powell, W. A., and Fitzsimmons, S. F. (2017). Rescue of American chestnut with extraspecific genes following its destruction by a naturalized pathogen. New Forests 48, 317–336. doi: 10.1007/S11056-016-9561-5/FIGURES/4
Taveira-Fernándes, C. (1972). Aspects de l’ameliorationdu chataignier pour la résistance a la maladie de l’encre. In In Actas III Congr Un Fitopat. Oeiras: Medit, 313–320.
Turchetti, T., Addario, A., and Maresi, M. (2010). Interazioni tra cinipide galligeno e cancro della corteccia: Una nuova criticità per il castagno. Forest@ J. Silvic. Forest Ecol. 7:252. doi: 10.3832/EFOR0642-007
Urquijo-Landaluze, P. (1957). La regeneración del castaño. Boletín de Patología Vegetal y Entomología Agrícola 22, 217–232.
van Hove, J., de Jaeger, G., de Winne, N., Guisez, Y., and van Damme, E. J. M. (2015). The Arabidopsis lectin EULS3 is involved in stomatal closure. Plant Sci. 238, 312–322. doi: 10.1016/J.PLANTSCI.2015.07.005
Vázquez, A., Martín, L. M., and Solla, A. (2016). Influencia de escenarios cambiantes de estrés hídrico y encharcamiento en la susceptibilidad de Castanea sativa a Phytophthora cinnamomi. Cuadernos de la Sociedad Española de Ciencias Forestales 43, 227–234. doi: 10.31167/CSEF.V0I42.17478
Vettraino, A. M., Natili, G., Anselmi, N., and Vannini, A. (2001). Recovery and pathogenicity of Phytophthora species associated with a resurgence of ink disease in Castanea sativa in Italy. Plant Pathol. 50, 90–96. doi: 10.1046/j.1365-3059.2001.00528.x
Vieira Natividade, J. (1947). Quatro anos na defesa da campanha e reconstituição dos soutos. Junta Nacional das Frutas (Ed.). Sintra: Tipografia Medina, 18.
Viéitez, E. (1960). “Obtención de castaños resistentes a la enfermedad de la tinta,” in investigaciones y experiencias forestales de Lourizán, Pontevedra, Spain, ed. C. R. Estudios (Sucesores de Rivadeneyra S.A: Madrid).
Vieitez, F. J., and Merkle, S. A. (2005). “Fagaceae,” in Biotechnology of Fruit and Nut Crops, ed. R. E. Litz (Wallingford, UK: CAB International), 263–296.
Villani, F., Pigliucci, M., Benedettelli, S., and Cherubini, M. (1991). Genetic differentiation among Turkish chestnut (Castanea sativa Mill.) populations. Heredity 66, 131–136. doi: 10.1038/hdy.1991.16
Vinson, C. C., Mota, A. P. Z., Porto, B. N., Oliveira, T. N., Sampaio, I., Lacerda, A. L., et al. (2020). Characterization of raffinose metabolism genes uncovers a wild Arachis galactinol synthase conferring tolerance to abiotic stresses. Sci. Rep. 10:15258. doi: 10.1038/s41598-020-72191-4
Waldboth, M., and Oberhuber, W. (2009). Synergistic effect of drought and chestnut blight (Cryphonectria parasitica) on growth decline of European chestnut (Castanea sativa). Forest Pathol. 39, 43–55. doi: 10.1111/j.1439-0329.2008.00562.x
Westbrook, J. W., Holliday, J. A., Newhouse, A. E., and Powell, W. A. (2020a). A plan to diversify a transgenic blight-tolerant American chestnut population using citizen science. Plants People Planet 2, 84–95. doi: 10.1002/PPP3.10061
Westbrook, J. W., Zhang, Q., Mandal, M. K., Jenkins, E. V., Barth, L. E., Jenkins, J. W., et al. (2020b). Optimizing genomic selection for blight resistance in American chestnut backcross populations: A trade-off with American chestnut ancestry implies resistance is polygenic. Evol. Appl. 13, 31–47. doi: 10.1111/eva.12886
Weste, G., and Marks, G. C. (1987). The Biology of Phytophthora cinnamomi in Australasian Forests. Annu. Rev. Phytopathol. 25, 207–229. doi: 10.1146/annurev.py.25.090187.001231
Xing, Y., Liu, Y., Zhang, Q., Nie, X., Sun, Y., Zhang, Z., et al. (2019). Hybrid de novo genome assembly of Chinese chestnut (Castanea mollissima). Gigascience 8:giz112. doi: 10.1093/gigascience/giz112
Zentmyer, G. A. (1988). Origin and distribution of four species of Phytophthora. Trans. Br. Mycol. Soc. 91, 367–378. doi: 10.1016/S0007-1536(88)80111-6
Zentmyer, G. A., and Mircetich, S. M. (1966). Saprophytism and persistence in soil by Phytophthora cinnamomi. Phytopathology 56, 710–712.
Zhang, B., Oakes, A. D., Newhouse, A. E., Baier, K. M., Maynard, C. A., and Powell, W. A. (2013a). A threshold level of oxalate oxidase transgene expression reduces Cryphonectria parasitica-induced necrosis in a transgenic American chestnut (Castanea dentata) leaf bioassay. Transgenic. Res. 22, 973–982. doi: 10.1007/s11248-013-9708-5
Zhang, C., Moutinho-Pereira, J. M., Correia, C., Coutinho, J., Gonçalves, A., Guedes, A., et al. (2013b). Foliar application of Sili-K§increases chestnut (Castanea spp.) growth and photosynthesis, simultaneously increasing susceptibility to water deficit. Plant Soil 365, 211–225. doi: 10.1007/S11104-012-1385-2/FIGURES/4
Zhebentyayeva, T. N., Sisco, P. H., Georgi, L. L., Jeffers, S. N., Perkins, M. T., James, J. B., et al. (2019). Dissecting Resistance to Phytophthora cinnamomi in Interspecific Hybrid Chestnut Crosses Using Sequence-Based Genotyping and QTL Mapping. Phytopathology® 109, 1594–1604. doi: 10.1094/PHYTO-11-18-0425-R
Keywords: breeding, Castanea, climate change, chestnut blight, Cryphonectria parasitica, drought, ink disease, Phytophthora cinnamomi
Citation: Fernandes P, Colavolpe MB, Serrazina S and Costa RL (2022) European and American chestnuts: An overview of the main threats and control efforts. Front. Plant Sci. 13:951844. doi: 10.3389/fpls.2022.951844
Received: 24 May 2022; Accepted: 29 July 2022;
Published: 24 August 2022.
Edited by:
Neusa Steiner, Federal University of Santa Catarina, BrazilReviewed by:
Mohammad Rashed Hossain, Bangladesh Agricultural University, BangladeshCopyright © 2022 Fernandes, Colavolpe, Serrazina and Costa. This is an open-access article distributed under the terms of the Creative Commons Attribution License (CC BY). The use, distribution or reproduction in other forums is permitted, provided the original author(s) and the copyright owner(s) are credited and that the original publication in this journal is cited, in accordance with accepted academic practice. No use, distribution or reproduction is permitted which does not comply with these terms.
*Correspondence: Rita Lourenço Costa, cml0YS5sY29zdGFAaW5pYXYucHQ=
†These authors share first authorship
Disclaimer: All claims expressed in this article are solely those of the authors and do not necessarily represent those of their affiliated organizations, or those of the publisher, the editors and the reviewers. Any product that may be evaluated in this article or claim that may be made by its manufacturer is not guaranteed or endorsed by the publisher.
Research integrity at Frontiers
Learn more about the work of our research integrity team to safeguard the quality of each article we publish.