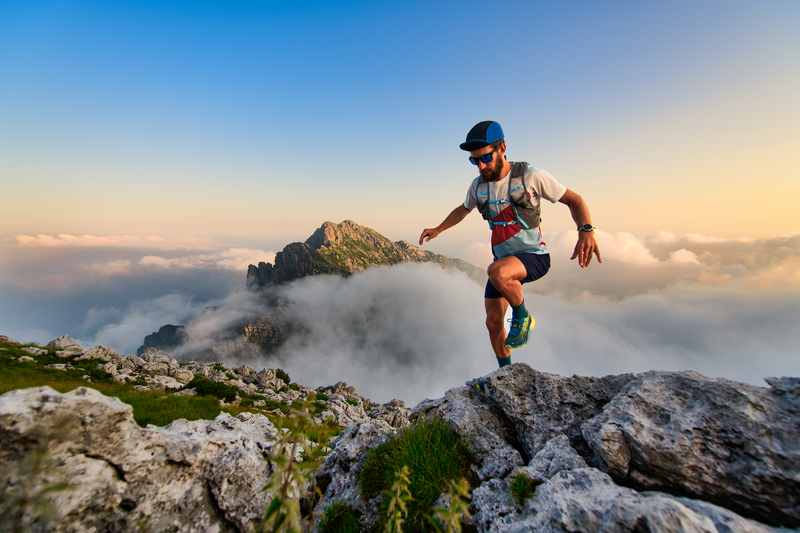
95% of researchers rate our articles as excellent or good
Learn more about the work of our research integrity team to safeguard the quality of each article we publish.
Find out more
ORIGINAL RESEARCH article
Front. Plant Sci. , 25 October 2022
Sec. Plant Abiotic Stress
Volume 13 - 2022 | https://doi.org/10.3389/fpls.2022.947949
This article is part of the Research Topic Nanofertilizers and Abiotic Stress Tolerance in Plants View all 9 articles
The use of calcium carbonate-precipitating bacteria (CCPB) has become a well-established ground-improvement technique. However, the effect of the interaction of CCPB with nanoparticles (NPs) on plant performance is still meager. In this study, we aimed at evaluating the role of CCPB and/or silicon NPs (Si-NPs) on the growth, physio-biochemical traits, and antioxidative defense of wheat (Triticum aestivum L.) under semi-arid environmental conditions. A 2-year pot experiment was carried out to determine the improvement of the sandy soil inoculated with CCPB and the foliar application of Si-NPs on wheat plants. We tested the following treatments: spraying plants with 1.0 or 1.5 mM Si-NPs (control = 0 mM Si-NPs), soil inoculated with Bacillus lichenforms (MA16), Bacillus megaterium (MA27), or Bacillus subtilis (MA34), and the interaction of individual Bacillus species with Si-NPs. Our results showed that soil inoculation with any of the three isolated CCPB and/or foliar application of Si-NPs at the rates of 1.0 or 1.5 mM significantly improved (p ≤ 0.05) the physiological and biochemical attributes as well as the enzymatic antioxidant activities of wheat plants. Therefore, the combined treatments of CCPB + Si-NPs were more effective in enhancing physio-biochemical characteristics and enzymatic antioxidant activities than the individual treatments of CCPB or Si-NPs, thus achieving the best performance in the treatment of MA34 + 1.5 mM Si-NPs. Our results demonstrated that the co-application of CCPB and Si-NPs, particularly MA34 + 1.5 mM Si-NPs, considerably activated the antioxidant defense system to mitigate the adverse effects of oxidative stress, thus increasing tolerance and enhancing the production of wheat plants in sandy soils under semi-arid environmental conditions.
Wheat (Triticum aestivum L.), belonging to the Poaceae family, is the most important cereal crop globally due to its higher content of protein, carbohydrates, vitamins, and calories than other cereal crops (Elrys et al., 2020). It is cultivated in rain-fed and irrigated areas in tropical and subtropical regions. Additionally, it is grown on approximately 200 million hectares globally, yielding about 700 Tg (1012 g) year–1 (FAOSTAT, 2020). Therefore, the global need for wheat is increasing, predominantly in developing nations with limited croplands and resources, including Egypt, which poses challenges in producing the quantities of wheat required to meet this growing demand. For instance, wheat covers around 1.40 million hectares in Egypt, producing 9.0 Tg of grain in 2019, approximately 34.5% of annual consumption (FAOSTAT, 2020).
Consequently, it is imperative to maximize wheat production, especially in soils with poor physicochemical and biological properties, such as sandy soils, which cover about 90% of the Egyptian soils (Merwad and Abdel-Fattah, 2015). Furthermore, under Egypt’s semi-arid climatic conditions, these soils provide significant prospects for agricultural expansion. Promising techniques for increasing productivity in such soils include effective agricultural bio-systems that consider the biochemical diversity of agricultural systems, their ability to reduce the negative influences of low soil fertility, and water-retaining capacity in sandy soils. However, the use of calcium carbonate (CaCO3) precipitating bacteria (CCPB) and silicon (Si) nanoparticles (Si-NPs) are innovative and effective technologies that improve the productivity of crops under semi-arid environmental conditions (Chaparro-Acuña et al., 2018; Desoky et al., 2021).
The precipitation of CaCO3 is a process in which microorganisms, mainly bacteria, provide adequate substrates, creating CaCO3 crystals (Chaparro-Acuña et al., 2018). The Bacillus group is non-pathogenic and tolerant of extreme conditions, with high concentrations of urease enzyme (Achal et al., 2015); therefore, it can potentially be used as CCPB. Urease hydrolyzes urea in soils to carbonate (CO32–) and ammonium (NH4+). The produced NH4+ raises the pH of the solution, causing the reaction to form CaCO3 on the bacterial cell surface whenever there is sufficient calcium (Ca2+) and CO32– ion content in the solution (Chaparro-Acuña et al., 2018). The resulting CaCO3 can coat surfaces and bind various particles together (Seifan et al., 2020). CCPB is a practical approach to enhance soil quality (Chaparro-Acuña et al., 2018) and increase sand stabilization and soil hardness while decreasing soil porosity in sandy soils (Whiffin et al., 2007). Similarly, the induction of CCPB binds sand grains and enhances soil stiffness and strength (DeJong et al., 2010; Mortensen et al., 2011).
Due to its favorable physic-mechanical activities, Si alleviates the adverse effects of water shortage and improves plant performance (Rady et al., 2019; Desoky et al., 2020). Nano-materials have emerged as a promising solution to various technological and environmental problems in several disciplines (Ansari and Husain, 2012). Compared with bulk Si, Si-NPs have a larger surface area with higher surface reactivity and solubility (Qados and Moftah, 2015). Specifically, particle size is a crucial factor influencing particle adhesion, absorption, and transportation in plant cells (Wang et al., 2009). Furthermore, NPs interact with plant cells by aiding the movement of numerous compounds that control plant metabolism and various physiological processes (Giraldo et al., 2014; Desoky et al., 2021).
However, knowledge of the effect and interaction of CCPB and Si-NPs on the performance of wheat plants cultivated in sandy soils under semi-arid environmental conditions is limited. Therefore, this study investigated the mechanism of inoculating sandy soil with CCPB and foliar application of Si-NPs to plants in influencing the physio-biochemical characteristics, performance, and antioxidative defenses of wheat grown under semi-arid environmental conditions. We hypothesized that inoculating soil with CCPB or foliar spraying with Si-NPs would improve wheat performance and defense against erosion in sandy soils under semi-arid environmental conditions. However, the co-addition of CCPB and Si-NPs would be more effective than single additions.
Calcium carbonate-precipitating bacterial isolates were isolated from the calcareous soil of the Mariout sector, Alexandria, Egypt. The soil sample was suspended in a sterilized saline solution (0.85% NaCl), and serial dilutions were carried out up to 10–6. Each dilution was plated on a medium containing 2.12 g NaHCO3, 3.0 g nutrient broth (Lab M Limited, Lancashire, UK), 20.0 g urea, 10.0 g NH4Cl, 30.0 mM CaCl2, 20.0 g agar L–1, and pH 8.5. The plates were then incubated at 28°C for 7 d. After isolation, all colonies were individually plated on CaCO3 precipitation medium supplemented with five concentrations of CaCO3 (10.0%, 15.0%, 20.0%, 25.0%, and 30.0%). Individual colonies that are found to be positive were selected based on their crystal formation visibility and purification by streaking on CaCO3 precipitation media without CaCl2.
The selected colonies were assessed under a stereomicroscope and primarily identified using Bergey’s manual of systematic bacteriology morphological and biochemical tests (Vos et al., 2009; Guinebretière et al., 2013). Further identification was performed by matrix-assisted laser desorption/ionization-time of flight (MALDI-TOF) mass spectrometry (MS) (Bruker Daltonics, Bremen, Germany) according to Schumaker et al. (2012) and Sauget et al. (2017). The manufacturers suggested score values of 2.30–3.00, 2.00–2.30, and 1.70–2.00 as highly probable species identification, secure genus identification and probable species identification, and probable genus identification, respectively.
The chosen isolates were inoculated into 10 ml nutrient broth tubes with different pH levels ranging from 1 to 14. The pH was adjusted using 1 N NaOH and 1 N HCl. The turbidity of each isolate was adjusted to the 0.5% McFarland standard, and the tubes were incubated for 24 h at 37°C. The growth was next assessed using a spectrophotometer (UV-2101/3101 PC; Shimadzu Corporation, Analytical Instruments Division, Kyoto, Japan) at an optical density of (OD)600 nm, and the results were compared with a bacterial blank suspension. Results were determined after 30 min, 1 h, 2 h, 4 h, 8 h, 24 h, and 32 h of inoculation.
The selected bacterial isolates were briefly inoculated into 10 ml nutrient broth tubes, and the turbidity of each isolate was adjusted to the 0.5% McFarland standard. The tubes were incubated at 0, 10, 20, 30, 40, 50, and 60°C for 24 h. The growth was next assessed using a spectrophotometer (Shimadzu Corporation) at OD600 nm, and the results were compared with a bacterial blank suspension.
In assaying the urease activity, urea agar media (UAM) containing 15.0 g, 20.0 g, 1.0 g, 1.0 g, 5.0 g, 2.0 g, and 0.012 g of agar, urea, dextrose, pancreatic digest gelatin, sodium chloride, monosodium phosphate, and phenol red, respectively, were used. The medium pH was adjusted to a pH of 6.8 (Hammes et al., 2003; Chahal et al., 2011). Each candidate strain’s cell suspension (106 cells mL–1) was inoculated on UAM. The plates were incubated for 24–48 h at 28°C, and the color change from yellow to pink was determined. Urease activity was measured as the concentration of the produced ammonium ions (NH4 +) as described by Tavares et al. (2021).
All isolates were cultivated aerobically in 500 ml Erlenmeyer flasks with 100 ml of liquid CaCO3 precipitation medium. Flaks were incubated at 28°C for 3 d for CaCO3 precipitation and collection. The uninoculated liquid CaCO3 precipitation medium served as the control. After incubation, the entire culture was centrifuged for 1 min at 10,000 × g. The pellet was resuspended in a 50 ml TE buffer, which contained CaCO3 and bacterial cells (10 mM Tris, 1 mM EDTA at pH 8.5).
To digest the bacterial cell wall, lysozyme was added to the cell suspension at a final rate of 1 mg mL–1, and the tubes were incubated at 37°C for 1 h. Notably, centrifugation was used to remove the cell debris, and sterile distilled water (pH 8.5) was used to wash the pellet before being air-dried at 37°C for 24 h. The pellet was weighed to calculate the number of carbonate crystals precipitated by the various isolates.
A 2-year pot trial was performed in 2019/2020 and 2020/2021 using an open greenhouse at the Botany Department, Faculty of Agriculture, Zagazig University, Zagazig, Egypt. The average daily temperature was 17.7°C ± 2.0°C (15.3°C to 20.1°C), and the average daily relative humidity was 48.2 ± 4.3% (45.4%–51.0%). Wheat (Triticum aestivum L., cv. Misr 2) grains were obtained from the Agronomy Research Institute of the Agriculture Research Centre, Giza, Egypt. Before sowing, the grains were surface-sterilized for 5 min with 1% (v/v) sodium hypochlorite, washed several times with distilled water, and finally, air-dried for 1 h. Additionally, 10 kg of sandy soil was filled into plastic pots with inner diameters of 35 cm and depths of 30 cm. The physicochemical attributes of the tested soil, were measured (Page et al., 1982; Klute and Dirksen, 1986), and are illustrated in Supplementary Table 1.
A total of 240 pots were used in this study with the following investigated treatments: control, spraying plants with 1.00 or 1.50 mM Si-NPs; soil inoculated with Bacillus lichenforms (MA16), Bacillus megaterium (MA27), Bacillus subtilis (MA34), MA16 + 1.00 or 1.50 mM Si-NPs, MA27 + 1.00 or 1.50 mM Si-NPs, and MA34 + 1.00 or 1.50 mM Si-NPs. The recommended dose of inorganic nitrogen (N) as ammonium sulfate (205 g N kg–1 fertilizer) was added to all pots in three equal splits at a rate of 100 mg N kg–1 soil. The first split was added before the first irrigation, while the second and third doses were added 40 and 70 days after the first split.
Before sowing, phosphorus (P) and potassium (K) were applied to all experimental treatments at the recommended rates. Phosphorus was added as ordinary superphosphate at 15 mg P kg–1 soil, and K was applied as potassium sulfate at 40 mg K kg–1 soil. All pots were rotated (moved from one location to another) every 2 d to ensure equal light distribution and sunlight intensity to all plants. Notably, ten homogeneous grains were sown in each pot, leaving only five uniform seedlings in each pot after germination.
Nano-Si dioxide was employed at 99.5% purity, 20–30 nm, and a surface area of 180–600 m2 g–1. A pressurized spray bottle was used to apply foliar sprays of 1 and 1.5 mM Si-NPs. In total, 0.1% of tween 20 was used as a surfactant (Desoky et al., 2021).
Wheat plants were harvested during each growing season to measure the growth attributes, physiology and biochemistry, and antioxidant defense system components after 65 days of planting. The leaf area (cm2) and plant height (cm) were determined. In measuring the dry weight (DW), samples were dried at 70°C until a constant weight was reached. During harvesting, the 1000 grain weight (g), the number of grains spike−1, and DW of grains plant−1 (g) were determined.
The acetone extraction method was used to determine the contents of photosynthetic pigments—carotenoids and total chlorophylls (Arnon, 1949). Absorbance readings at 663 nm, 645 nm, and 480 nm were taken using a spectrophotometer (Shimadzu Corporation) to compute pigment content in mg g–1 leaf fresh weight. In upper fully expanded leaf tissue (second fully expanded leaf), chlorophyll fluorescence parameters using a PAM chlorophyll fluorimeter, the conductance of stomata (gs), net photosynthesis rate (Pn), and transpiration (Tr) rate were measured (Li et al., 2007). The formulas of Maxwell and Johnson (2000) were used to compute the maximum PS II Fv/Fm quantum yield as follows:
Fv/Fm = (Fm–F0)/Fm. Where, Fv; variable fluorescence, Fm; maximum light-adaptive fluorescence, and F0; minimum-adaptive fluorescence.
Photochemical quenching (qP) and non-photochemical quenching (NPQ) were determined as described by Han et al. (2022) and Ruban and Wilson (2021), respectively. Barrs and Weatherley’s (1962) method was used to measure the relative water content (RWC). We also determined the membrane stability index (MSI) based on the method reported by Rady (2011). The total inorganic ions that leached from the leaves (electrolyte leakage, EL) and malondialdehyde (MDA) contents were estimated according to the methods used previously (Heath and Packer, 1968; Sullivan and Ross, 1979). The leaf contents of superoxide oxide radical (O2⋅–; at A580 g–1 FW) and hydrogen peroxide (H2O2; μmol g–1 FW) were assessed following the procedures of Mukherjee and Choudhuri (1983) and Kubiś (2008), respectively. Proline (Pro) accumulation in leaves and total soluble sugar (TSS) content were also determined (Bates et al., 1973; Irigoyen et al., 1992). Additionally, glycine betaine (GB; Grieve and Grattan, 1983), and α-tocopherol (α-TOC; Ching and Mohamed, 2001) were calculated.
The contents (mol g–1 fresh weight) of ascorbate (AsA) and reduced glutathione (GSH) were assessed according to the methods of Griffith (1980) and Kampfenkel et al. (1995), respectively. Enzyme concentrations were extracted according to Vitória et al. (2001). The catalase (CAT) enzyme concentration was measured spectrophotochemically according to Chance and Maehly (1955), and peroxidase (POX) activity was estimated according to Thomas et al. (1982).
Ascorbate peroxidase (APX) was also determined spectrophotochemically (Fielding and Hall, 1978). Additionally, superoxide dismutase (SOD) activity was measured using the method of Sairam et al. (2002) by recording the drop in the absorbance of the superoxide-nitro blue tetrazolium complex by the enzyme. The glutathione reductase activity (GR; A564 min–1 mg–1 protein) was estimated according to Rao et al. (1996) after monitoring NADPH oxidation for three absorbances obtained at 340 nm.
Data are shown as means ± SE. The experiments were designed using a completely randomized block design. We analyzed the data statistically using analysis of variance (ANOVA) and Tukey’s HSD test using SPSS 14.0 (SPSS Chicago, IL, USA) to analyze the significant differences between treatments (p ≤ 0.05).
In total, 140 isolates were successfully isolated from calcareous soil on CCPB medium. Additionally, 34 isolates coded MA1–MA34 were grown on the CCPB plates supplemented with 10% of CaCO3, 26 isolates were grown on the CCPB plates supplemented with 15% of CaCO3, and 11 isolates survived at 20% of CaCO3. On the other hand, only four isolates grew on CCPB medium supplemented with 25% CaCO3. Only three isolates (MA16, MA27, and MA34) precipitated 30% CaCO3 (Table 1), and only these three isolates were selected for the experiments described below. Furthermore, the screened isolates were all gram-negative, aerobic, motile, and non-spore-forming bacilli. According to Bergey’s manual of systematic bacteriology morphological and biochemical tests, the obtained results showed that MA16, MA27, and MA34 isolates were similar to the Bacillus species.
They were identified as B. lichenforms MA16, B. megaterium MA27, and B. subtilis MA34. These three Bacillus spp. were further identified by MALDI–TOF mass spectrometry, as recommended by Biswas and Rolain (2013). Our results showed that they were 99% similar to the numerous Bacillus spp. According to the MALDI–TOF, score values were 2.332, 2.361, and 2.318. The local bacterial isolates B. lichenforms MA16, B. megaterium MA27, and B. subtilis MA34 were similar to B. lichenforms DSM30243T, B. megaterium DSM76T, and B. subtilis ssp. subtilis DSM10T, respectively (Supplementary Table 2). Bacillus subtilis MA34 grew better when treated with a higher concentration of CaCO3, thus inducing the best growth (Table 1).
Furthermore, the selected CCPB (MA16, MA27, and MA34) showed rapid growth and the highest turbidity at pH 8, indicating that these isolates are moderate alkaliphiles (Figure 1A). MA34 showed more growth at optimum pH with a turbidity of 1.5 × 106 with a relative increase of 20% and 40% over MA27 and MA16, respectively. Conversely, the optimum temperature for the best growth of CCPB isolates was in the range of 30–40°C, with a preference for 37°C (Figure 1B). Similarly, the MA34 isolate had the best performance at 37°C.
Figure 1. Optimization of calcium carbonate precipitating bacteria (CCPB). Effect of (A) pH and (B) temperature on Bacillus lichenforms (MA16), Bacillus megaterium (MA27), and Bacillus subtilis (MA34) growth.
According to the precipitation mass per cell, B. subtilis MA34 was the most efficient strain in inducing CaCO3 precipitation. B. subtilis MA34 had the highest growth rate of 7.8 × 106. They precipitated the highest value of CaCO3, i.e., 990 ppm, with a relative increase of 25% and 57% for B. megaterium MA27 and B. lichenforms MA16, respectively (Figure 2).
Figure 2. Calcium carbonate precipitation (CCP) and production of urease by the selected calcium carbonate precipitating bacteria, Bacillus lichenforms (MA16), Bacillus megaterium (MA27), and Bacillus subtilis (MA34). Urease activity was measured as the concentration of the produced ammonium ions (NH4+).
Furthermore, the urease activity was recorded in all strains and expressed as the amount of NH4+. B. subtilis MA34 produced 775 ppm of NH4+ compared to 510 ppm and 325 ppm in B. megaterium MA27 and B. lichenforms MA16, respectively. Therefore, our findings showed that the mass of CaCO3 precipitation was directly and positively correlated with urease activity (Figure 2).
The soil inoculated with the three isolated CCPB (MA16, MA27, and MA34) and/or foliar application of wheat plants with Si-NPs at 1.0 and 1.5 mM significantly increased the plant height, leaf area, shoot DW, the 1000 grain weight, grain yield, and the number of grains spike–1 compared to control (Table 2). In addition, the combined treatments (CCPB + Si-NPs) were more effective than the individual applications (CCPB or Si-NPs) in improving the above attributes. Notably, MA34 + 1.5 mM Si-NPs was the best treatment, increasing plant height by 38.1% and 35.6%, shoot DW by 79% and 78.6%, leaf area by 50.5% and 42.7%, number of grains spike–1 by 110% and 116%, the 1000 grain weight by 43.4% and 49.1%, and plant grain yield by 173% and 186% in both seasons, respectively (Table 2).
Table 2. Effect of soil application with calcium carbonate precipitating bacteria (CCPB), Bacillus lichenforms (MA16), Bacillus megaterium (MA27), and Bacillus subtilis (MA34), and foliar application with silicon nanoparticles (Si-NPs) on growth and yield components of wheat plants (cv. Misr 2).
Compared with the control, the soil inoculated with the three isolated CCPB (MA16, MA27, and MA34) and/or foliar application of wheat plants with Si-NPs at 1 and 1.5 mM impacted significant increases in the chlorophylls, carotenoids, Pn, Tr, and gs and photosynthetic efficiency (quantum yield of PSII; FPSII, qP and efficiency of PSII; Fv/Fm) except for the NPQ, which was significantly reduced (Tables 3, 4). The combined addition of CCPB and Si-NPs was more effective than individual applications in improving these parameters. Additionally, MA34 + 1.5 mM Si-NPs showed the best treatment by increasing the total chlorophylls (61.2% and 63.5%), total carotenoids (18.3% and 23.3%), Pn (60.1% and 61.4%), Tr (55.6% and 52.8%), gs (69.9% and 62.1%), FPSII (118% and 124%), qP (49.5% and 50.4%), and Fv/Fm (64.1% and 57%); however, decreased NPQ by 48.9% and 49.7% in both seasons, respectively (Tables 3, 4).
Table 3. Effect of soil application with calcium carbonate precipitating bacteria (CCPB), Bacillus lichenforms (MA16), Bacillus megaterium (MA27), and Bacillus subtilis (MA34), and foliar application with silicon nanoparticles (Si-NPs) on photosynthetic pigments and gas exchange of wheat plants (cv. Misr 2).
Table 4. Effect of soil application with calcium carbonate precipitating bacteria (CCPB), Bacillus lichenforms (MA16), Bacillus megaterium (MA27), and Bacillus subtilis (MA34), and foliar application with silicon nanoparticles (Si-NPs) on chlorophyll fluorescence parameters, RWC and MSI of wheat plants (cv. Misr 2).
Applying three isolated bacteria and/or Si-NPs at different rates considerably increased the RWC and MSI but reduced EL, MDA, O2⋅–, and H2O2 in wheat plants compared with the control (Tables 4, 5). Furthermore, the co-addition of CCPB and Si-NPs was more effective than the individual application. Notably, MA34 + 1.5 mM Si-NPs were more effective than other treatments, increasing RWC by 27.8% and 28.1% and MSI by 37.8% and 38.1%, and decreasing El by 42.7% and 45.2%, MDA by 67.6% and 73.5%, O2⋅– by 65% and 72.5%, and H2O2 by 71.3% and 77% in both seasons, respectively (Tables 4, 5).
Table 5. Effect of soil application with calcium carbonate precipitating bacteria (CCPB), Bacillus lichenforms (MA16), Bacillus megaterium (MA27), and Bacillus subtilis (MA34), and foliar application with silicon nanoparticles (Si-NPs) on oxidative stress of wheat plants (cv. Misr 2).
Compared with the control, the addition of CCPB and/or Si-NPs significantly increased osmoprotectants (Pro, TSS, and GB), α-TOC, AsA, and GSH (Table 6). The treatments of CCPB + Si-NPs were more effective than individual applications (CCPB or Si-NPs). MA34 + 1.5 mM Si-NPs was more effective than other treatments as it increased Pro (39.3% and 38.6%), TSS (114% and 115%), GB (48.5% and 48.9%), α-TOC (76.9% and 80.5%), AsA (94.2% and 93.5%), and GSH (157% and 147%) during the two seasons, respectively (Table 6).
Table 6. Effect of soil application with calcium carbonate precipitating bacteria (CCPB), Bacillus lichenforms (MA16), Bacillus megaterium (MA27), and Bacillus subtilis (MA34), and foliar application with silicon nanoparticles (Si-NPs) on osmoprotectants contents (free Pro and TSS), α-TOC, AsA, GSH and glycine betaine of wheat plants (cv. Misr 2).
Compared with the control, the soil inoculated with CCPB and/or plant spraying Si-NPs showed a significant increase in the activities of POX, CAT, APX, SOD, and GR in wheat plants (Table 7). Compared with individual treatments, the co-addition of CCPB and Si-NPs was demonstrated to be more effective. MA34 + 1.5 mM Si-NPs was the best treatment, showing an increase in the activities of CAT (17.9% and 17.2%), POX (185% and 193%), APX (18.7% and 18.8%), SOD (123% and 115%), and GR (57% and 56.7%) in both seasons, respectively (Table 7).
Table 7. Effect of soil application with calcium carbonate precipitating bacteria (CCPB), Bacillus lichenforms (MA16), Bacillus megaterium (MA27), and Bacillus subtilis (MA34), and foliar application with silicon nanoparticles (Si-NPs) on antioxidant enzymes of wheat plants (cv. Misr 2).
Calcium carbonate precipitation is a type of bio-mineralization that frequently occurs in bacteria (Boquet et al., 1973). It can be accomplished through biologically controlled or induced mineralization mechanisms (Mann, 1995). However, CCPB consists mainly of induced mineralization (Zamarreño et al., 2009). Globally, several bacterial species participate in mineral carbonate precipitation in various conditions such as soils, oceans, saline lakes, and freshwaters. Mineralization induced by microbial metabolic activities raises the alkalinity of the medium, thus facilitating CaCO3 precipitation (Castanier et al., 1999). The most common metabolic activity is urea hydrolysis, predominantly found in many microorganisms, majorly catalyzed by urease enzymes (Mobley and Hausinger, 1989). Urea hydrolysis using the microbial urease enzyme produces CO32– and ammonia (NH3), which raises soil pH and CO32– content which reacts with Ca2+ and precipitates it as CaCO3 (De Muynck et al., 2010).
Calcium carbonate-precipitating bacterial inoculation in sandy soil is one of the significant determinants of soil fertility, resulting in improved plant growth and productivity. It boosts the productivity of sandy soil by enhancing its biological activity, available nutrient content, and soil quality (Chaparro-Acuña et al., 2018; Elrys et al., 2018). These benefits of CCPB contributed to the high growth and yield of wheat when combined with Si-NPs foliar application (Table 2). This high performance was attributed to the improved chlorophyll fluorescence parameters and photosynthetic pigments of a wheat leaf affected by Si-NPs or CCPB treatments, principally the integrative CCPB + Si-NPs treatment. In addition, photosynthetic leaf pigments such as chlorophylls and carotenoids exhibited crucial roles in plant photosynthesis by capturing solar energy to fix carbon dioxide (CO2) (Table 3). The chlorophyll fluorescence proportion depends on the amount of solar energy absorbed by the chlorophyll molecules and the photosynthetic apparatus efficiency. As a result, photosynthetic pigments and chlorophyll fluorescence are essential components of photosynthesis (Netto et al., 2005; Singh et al., 2017).
During the light reaction photosynthesis phase, most PSII excitation energy is converted into ATP and NADP(H), consumed in CO2 fixation, and photorespiration coupled in the dark reaction phase. When PSII is not excited, a small fraction is lost as fluorescence, whereas the excess energy is dissipated as heat (Maxwell and Johnson, 2000). The chlorophyll fluorescence yield is detectable and regularly linked with photochemistry changes related to total photochemical efficiency (Singh et al., 2017). Hence, the concurrent increase in photosynthetic pigments and chlorophyll fluorescence, including gas exchange parameters by the co-addition of CCPB and Si-NPs, offers insight into the PSII function (Tables 3, 4).
The measurements of the photosynthetic pigments and chlorophyll fluorescence in this study serve as indicators of plant health. Therefore, supplying plants with CCPB + Si-NPs preserves the leaf pigments and chlorophyll fluorescence content, which is positively correlated with wheat yields. Additionally, maintaining the efficiency of the antioxidant system components and PSII function (Tables 6, 7) contributed to wheat performance, coupled with the beneficial effects of CCPB and/or Si-NPs. Furthermore, preserving the antioxidant system components in CCPB and/or Si-NPs treatments aided cell membrane stabilization in terms of low EL and MDA in plants (Table 5).
Moreover, CCPB and/or Si-NPs (especially the integrative CCPB + Si-NPs treatment) supplied to plants led to better yield and growth, which is attributed to the enhanced translocation of the photosynthetic assimilates from leaf to spike. This result is linked to the ability of plants to improve the antioxidant defense system components (Tables 6, 7). Therefore, this enhanced plant’s performance in cell expansions and meristem activities due to the retention of a sufficient quantity of water under sandy soil conditions causing increased contents of osmoprotectants such as TSS, Pro, and GB (Table 6).
In addition, the application of CCPB and/or Si-NPs induced a rise in wheat growth and production (Table 2), correlated with increased photosynthetic efficiency, chlorophyll biosynthesis, and gas exchange (Tables 3, 4). There was also a decrease in H2O2 and O2⋅–accumulations. Furthermore, after treating the plants with CCPB and/or Si-NPs, the efficiency of photosynthesis is boosted by increasing the ameliorative influences on chlorophyll fluorescence attributes (e.g., exc, PSII, qP, and Fv/Fm) while reducing the NPQ. Therefore, we revealed that the photosynthetic efficiency elevation in this study largely depends on the protected functioning of the photosynthetic light reaction, which functionally concurred with the enzymes PSII and PSI.
Silicon and/or CCPB application reduced H2O2 and O2⋅– accumulation, MDA (a lipid peroxidation marker), and EL in the plants grown in sandy soil (Table 5). In addition, plants provided with CCPB and/or Si-NPs significantly reduced the membrane MDA and EL, improving membrane integrity attributed to the positive influence of the treatments on antioxidant system component maintenance (Table 7) and low peroxidation rates (Table 5). Plants treated with CCPB and/or Si-NPs significantly increased antioxidant enzyme activity with the AsA and GSH contents, shielding them from high H2O2 and O2⋅–. Furthermore, SOD eliminated O2⋅– radicals through ROS dismutation together with APX and CAT. Si-stimulated SOD upregulation influences the substrates H2O2 and O2⋅– (Gong et al., 2003; Desoky et al., 2019). Therefore, this mechanism decreases toxic hydroxyl radical (OH–) formation (Singh and Prasad, 2014).
The accumulation of AsA and GSH initiated by CCPB and/or Si-NPs protects wheat plants from ROS-stimulated injuries. Additionally, CCPB and/or Si-NPs-induced upregulation of the ROS scavenging pathway components such as AsA, GR, APX, and GSH which improves plant tolerance mechanisms against oxidative damage. For example, wheat plants treated with CCPB and/or Si-NPs decreased ROS accumulation (H2O2 and O2⋅–) (Table 5) and increased protection of the photosynthetic pathways (Tables 3, 4), contributing to improved growth and yield productivity (Table 2). H2O2, a byproduct of O2⋅– elimination by the activity of SOD, degrades in the ascorbate–glutathione cycle and cytoplasm by APX and CAT, respectively (Feierabend, 2005). Furthermore, the APX enzyme is critical in scavenging H2O2 in the chloroplasts and cytosol, averting H2O2 diffusion into other organelles that could potentially cause damage. Furthermore, the optimal operation of the AsA-GSH cycle pathway when plants were provided with CCPB and/or Si-NPs (Tables 6, 7) effectively preserved GSH and AsA components, reducing the oxidative stress (H2O2 and O2⋅–; Table 5). Therefore, the increased non-enzymatic and enzymatic antioxidant activity is linked to improved plant health (Elrys et al., 2020; El-Saadony et al., 2021).
Our study treated wheat plants with CCPB and/or Si-NPs, causing the accumulated osmoprotectants (e.g., TSS, GB, Pro, and α-TOC) to raise the RWC, MSI, and water content of plants in sandy soil (Table 4). We also reported limited Pro accumulation, as pro-synthesizing enzymes were upregulated, whereas the catabolizing enzymes were downregulated (Table 6). This result was due to increased antioxidant system components, GB, and TSS. Additionally, Pro was incorporated into proteins (Ahmad, 2010). Si-NPs increased TSS and GB accumulation, maintaining balanced plant water (Ahanger et al., 2014).
Conclusively, wheat plants treated with CCPB and/or Si-NPs developed some potential mechanisms in sandy soils. These include increased accumulation of osmoprotectant compounds that provide a mechanism for water loss reduction in leaves and boost their water content to maintain healthy metabolic processes and membrane stability under sandy soil conditions. Additionally, increased antioxidant activity (enzymatic and non-enzymatic) provides a potential mechanism for strengthening the antioxidant defense system and increasing plant resistance. These mechanisms, combined with others, resulted in plant leaves remaining green, delayed senescence, and enhanced photosynthesis efficiency and chlorophyll content to maintain healthy plants. Therefore, these improvements in antioxidant defense components help limit oxidative damage.
The original contributions presented in this study are included in the article/Supplementary material, further inquiries can be directed to the corresponding authors.
E-SD, SA, KE-T, and ME-S conceived and designed the research. MR, SA, KE-T, and ME-S supervised the study. E-SD, MR, MN, NM, and AE performed open greenhouse experiments. E-SD, MR, and MN performed the microscopic experiments. E-SD, SA, KE-T, and ME-S analyzed the data. AE, AM, SA, and KE-T assisted with experiments and/or data evaluation. E-SD, SA, KE-T, and ME-S wrote the manuscript. All authors critically revised the manuscript and approved the final version.
This project was funded by the Khalifa Center for Biotechnology and Genetic Engineering—UAEU (Grant #: 31R286) to SA and the Abu Dhabi Award for Research Excellence—Department of Education and Knowledge (Grant #: 21S105) to KE-T.
KE-T is grateful to the library at Murdoch University, Australia, for the valuable online resources and comprehensive databases.
The authors declare that the research was conducted in the absence of any commercial or financial relationships that could be construed as a potential conflict of interest.
All claims expressed in this article are solely those of the authors and do not necessarily represent those of their affiliated organizations, or those of the publisher, the editors and the reviewers. Any product that may be evaluated in this article, or claim that may be made by its manufacturer, is not guaranteed or endorsed by the publisher.
The Supplementary Material for this article can be found online at: https://www.frontiersin.org/articles/10.3389/fpls.2022.947949/full#supplementary-material
Achal, V., Mukherjee, A., Kumari, D., and Zhang, Q. (2015). Biomineralization for sustainable construction–A review of processes and applications. Earth Sci. Rev. 148, 1–17. doi: 10.1016/j.earscirev.2015.05.008
Ahanger, M. A., Tyagi, S. R., Wani, M. R., and Ahmad, P. (2014). “Drought tolerance: Role of organic osmolytes, growth regulators, and mineral nutrients,” in Physiological mechanisms and adaptation strategies in plants under changing environment, eds P. Ahmad and M. Wani (New York: Springer), 25–55. doi: 10.1007/978-1-4614-8591-9_2
Ahmad, P. (2010). Growth and antioxidant responses in mustard (Brassica juncea L.) plants subjected to combined effect of gibberellic acid and salinity. Arch. Agron. Soil Sci. 56, 575–588. doi: 10.1080/03650340903164231
Ansari, S. A., and Husain, Q. (2012). Potential applications of enzymes immobilized on/in nano materials: A review. Biotechnol. Adv. 30, 512–523. doi: 10.1016/j.biotechadv.2011.09.005
Arnon, D. I. (1949). Copper enzymes in isolated chloroplasts. Polyphenoloxidase in Beta vulgaris. Plant Physiol. 24, 1–15. doi: 10.1104/pp.24.1.1
Barrs, H., and Weatherley, P. (1962). A re-examination of the relative turgidity technique for estimating water deficits in leaves. Aust. J. Biol. Sci. 15, 413–428. doi: 10.1071/bi9620413
Bates, L. S., Waldren, R. P., and Teare, I. (1973). Rapid determination of free proline for water-stress studies. Plant Soil 39, 205–207. doi: 10.1007/bf00018060
Biswas, S., and Rolain, J. M. (2013). Use of MALDI-TOF mass spectrometry for identification of bacteria that are difficult to culture. J. Microbiol. Methods 92, 14–24. doi: 10.1016/j.mimet.2012.10.014
Boquet, E., Boronat, A., and Ramos-Cormenzana, A. (1973). Production of calcite (calcium carbonate) crystals by soil bacteria is a general phenomenon. Nature 246, 527–529. doi: 10.1038/246527a0
Castanier, S., Le Métayer-Levrel, G., and Perthuisot, J.-P. (1999). Ca-carbonates precipitation and limestone genesis—the microbiogeologist point of view. Sediment. Geol. 126, 9–23. doi: 10.1016/S0037-0738(99)00028-7
Chahal, N., Rajor, A., and Siddique, R. (2011). Calcium carbonate precipitation by different bacterial strains. Afr. J. Biotechnol. 10, 8359–8372. doi: 10.5897/ajb11.345
Chance, B., and Maehly, A. C. (1955). Assay of catalase and peroxidase. Methods Enzymol. 2, 764–775. doi: 10.1016/S0076-6879(55)02300-8
Chaparro-Acuña, S. P., Becerra-Jiménez, M. L., Martínez-Zambrano, J. J., and Rojas-Sarmiento, H. A. (2018). Soil bacteria that precipitate calcium carbonate: Mechanism and applications of the process. Acta Agron. 67, 277–288. doi: 10.15446/acag.v67n2.66109
Ching, L. S., and Mohamed, S. (2001). Alpha-tocopherol content in 62 edible tropical plants. J. Agric. Food Chem. 49, 3101–3105. doi: 10.1021/jf000891u
De Muynck, W., De Belie, N., and Verstraete, W. (2010). Microbial carbonate precipitation in construction materials: A review. Ecol. Eng. 36, 118–136. doi: 10.1016/j.ecoleng.2009.02.006
DeJong, J. T., Mortensen, B. M., Martinez, B. C., and Nelson, D. C. (2010). Bio-mediated soil improvement. Ecol. Eng. 36, 197–210. doi: 10.1016/j.ecoleng.2008.12.029
Desoky, E. M., Elsayed, A. I., Merwad, A., and Rady, M. M. (2019). Stimulating antioxidant defenses, antioxidant gene expression, and salt tolerance in Pisum sativum seedling by pretreatment using licorice root extract (LRE) as an organic biostimulant. Plant Physiol. Biochem. 142, 292–302. doi: 10.1016/j.plaphy.2019.07.020
Desoky, E. M., Mansour, E., El-Sobky, E., Abdul-Hamid, I., Taha, T. F., Elakkad, H. A., et al. (2021). Physio-biochemical and agronomic responses of faba beans to exogenously applied nano-silicon under drought stress conditions. Front. Plant Sci. 12:637783. doi: 10.3389/fpls.2021.637783
Desoky, E. M., Mansour, E., Yasin, M. A., El Sobky, E., and Rady, M. M. (2020). Improvement of drought tolerance in five different cultivars of Vicia faba with foliar application of ascorbic acid or silicon. Span. J. Agric. Res. 18:e0802. doi: 10.5424/sjar/2020182-16122
Elrys, A. S., Abdo, A. I., Abdel-Hamed, E. M., and Desoky, E. M. (2020). Integrative application of licorice root extract or lipoic acid with fulvic acid improves wheat production and defenses under salt stress conditions. Ecotoxicol. Environ. Saf. 190:110144. doi: 10.1016/j.ecoenv.2019.110144
Elrys, A. S., Abdo, A. I. E., and Desoky, E. M. (2018). Potato tubers contamination with nitrate under the influence of nitrogen fertilizers and spray with molybdenum and salicylic acid. Environ. Sci. Pollut. Res. 25, 7076–7089. doi: 10.1007/s11356-017-1075-y
El-Saadony, M. T., Desoky, E. M., Saad, A. M., Eid, R. S., Selem, E., and Elrys, A. S. (2021). Biological silicon nanoparticles improve Phaseolus vulgaris L. yield and minimize its contaminant contents on a heavy metals-contaminated saline soil. J. Environ. Sci. 106, 1–14. doi: 10.1016/j.jes.2021.01.012
FAOSTAT (2020). Production-crops. Available online at: http://faostat.Fao.org (accessed on July 3, 2022).
Feierabend, J. (2005). “Catalases in plants: Molecular and functional properties and role in stress defence,” in Antioxidants and rreactive oxygen species in plants, ed. N. Smirnoff (UK: Blackwell Publishing), 101–140. doi: 10.1002/9780470988565.ch5
Fielding, J. L., and Hall, J. (1978). A biochemical and cytochemical study of peroxidase activity in roots of Pisum sativum: II. distribution of enzymes in relation to root development. J. Exp. Bot. 29, 983–991. doi: 10.1093/jxb/29.4.983
Giraldo, J. P., Landry, M. P., Faltermeier, S. M., Mcnicholas, T. P., Iverson, N. M., Boghossian, A. A., et al. (2014). Plant nanobionics approach to augment photosynthesis and biochemical sensing. Nat. Mater. 13, 400–408. doi: 10.1038/nmat3890
Gong, H., Chen, K., Chen, G., Wang, S., and Zhang, C. (2003). Effects of silicon on growth of wheat under drought. J. Plant Nutr. 26, 1055–1063. doi: 10.1081/pln-120020075
Grieve, C., and Grattan, S. (1983). Rapid assay for determination of water soluble quaternary ammonium compounds. Plant Soil 70, 303–307. doi: 10.1007/bf02374789
Griffith, O. W. (1980). Determination of glutathione and glutathione disulfide using glutathione reductase and 2-vinylpyridine. Anal. Biochem. 106, 207–212. doi: 10.1016/0003-2697(80)90139-6
Guinebretière, M. H., Auger, S., Galleron, N., Contzen, M., De Sarrau, B., De Buyser, M. L., et al. (2013). Bacillus cytotoxicus sp. nov. is a novel thermotolerant species of the Bacillus cereus group occasionally associated with food poisoning. Int. J. Syst. Evol. Microbiol. 63, 31–40. doi: 10.1099/ijs.0.030627-0
Han, J., Gu, L., Warren, J. M., Guha, A., Mclennan, D. A., Zhang, W., et al. (2022). The roles of photochemical and non-photochemical quenching in regulating photosynthesis depend on the phases of fluctuating light conditions. Tree Physiol. 42, 848–861. doi: 10.1093/treephys/tpab133
Hammes, F., Boon, N., De Villiers, J., Verstraete, W., and Siciliano, S. D. (2003). Strain-specific ureolytic microbial calcium carbonate precipitation. Appl. Environ. Microbiol. 69, 4901–4909. doi: 10.1128/aem.69.8.4901-4909.2003
Heath, R. L., and Packer, L. (1968). Photoperoxidation in isolated chloroplasts: I. Kinetics and stoichiometry of fatty acid peroxidation. Arch. Biochem. Biophys. 125, 189–198. doi: 10.1016/0003-9861(68)90654-1
Irigoyen, J., Einerich, D., and Sánchez-Díaz, M. (1992). Water stress induced changes in concentrations of proline and total soluble sugars in nodulated alfalfa (Medicago sativa) plants. Physiol. Plant 84, 55–60. doi: 10.1111/j.1399-3054.1992.tb08764.x
Kampfenkel, K., Vanmontagu, M., and Inzé, D. (1995). Extraction and determination of ascorbate and dehydroascorbate from plant tissue. Anal. Biochem. 225, 165–167. doi: 10.1006/abio.1995.1127
Klute, A., and Dirksen, C. (1986). “Hydraulic conductivity and diffusivity: Laboratory methods,” in Methods of soil analysis: Part 1 physical and mineralogical methods, ed. A. Klute (USA, Madison: American Society of Agronomy, Inc), 687–734. doi: 10.2136/sssabookser5.1.2ed.c28
Kubiś, J. (2008). Exogenous spermidine differentially alters activities of some scavenging system enzymes, H2O2 and superoxide radical levels in water-stressed cucumber leaves. J. Plant Physiol. 165, 397–406. doi: 10.1016/j.jplph.2007.02.005
Li, P. M., Cai, R. G., Gao, H. Y., Peng, T., and Wang, Z. L. (2007). Partitioning of excitation energy in two wheat cultivars with different grain protein contents grown under three nitrogen applications in the field. Physiol. Plant 129, 822–829. doi: 10.1111/j.1399-3054.2007.00880.x
Mann, S. (1995). Biomineralization and biomimetic materials chemistry. J. Mater. Chem. 5, 935–946. doi: 10.1039/JM9950500935
Maxwell, K., and Johnson, G. N. (2000). Chlorophyll fluorescence—a practical guide. J. Exp. Bot. 51, 659–668. doi: 10.1093/jxb/51.345.659
Merwad, A.-R. M., and Abdel-Fattah, M. K. (2015). Effect of some soil amendments and foliar spray of salicylic and ascorbic acids on sorghum under saline calcareous soil conditions. Int. J. Soil Sci. 10, 28–36. doi: 10.3923/ijss.2015.28.36
Mobley, H., and Hausinger, R. (1989). Microbial ureases: Significance, regulation, and molecular characterization. Microbiol. Rev. 53, 85–108. doi: 10.1128/mr.53.1.85-108.1989
Mortensen, B., Haber, M., Dejong, J., Caslake, L., and Nelson, D. (2011). Effects of environmental factors on microbial induced calcium carbonate precipitation. J. Appl. Microbiol. 111, 338–349. doi: 10.1111/j.1365-2672.2011.05065.x
Mukherjee, S., and Choudhuri, M. (1983). Implications of water stress-induced changes in the levels of endogenous ascorbic acid and hydrogen peroxide in Vigna seedlings. Physiol. Plant 58, 166–170. doi: 10.1111/j.1399-3054.1983.tb04162.x
Netto, A. T., Campostrini, E., De Oliveira, J. G., and Bressan-Smith, R. E. (2005). Photosynthetic pigments, nitrogen, chlorophyll a fluorescence and SPAD-502 readings in coffee leaves. Sci. Hortic. 104, 199–209. doi: 10.1016/j.scienta.2004.08.013
Page, A., Miller, R., and Keeney, D. (1982). Methods of soil analysis. Part 2: Chemical and biological properties. Madison, USA: American Society of Agronomy Inc., 1143.
Qados, A. M. A., and Moftah, A. E. (2015). Influence of silicon and nano-silicon on germination, growth and yield of faba bean (Vicia faba L.) under salt stress conditions. Am. J. Exp. Agric. 5, 509–524. doi: 10.9734/ajea/2015/14109
Rady, M. M. (2011). Effect of 24-epibrassinolide on growth, yield, antioxidant system and cadmium content of bean (Phaseolus vulgaris L.) plants under salinity and cadmium stress. Sci. Hortic. 129, 232–237. doi: 10.1016/j.scienta.2011.03.035
Rady, M. M., Elrys, A. S., El-Maati, M. F. A., and Desoky, E. M. (2019). Interplaying roles of silicon and proline effectively improve salt and cadmium stress tolerance in Phaseolus vulgaris plant. Plant Physiol. Biochem. 139, 558–568. doi: 10.1016/j.plaphy.2019.04.025
Rao, M. V., Paliyath, G., and Ormrod, D. P. (1996). Ultraviolet-B-and ozone-induced biochemical changes in antioxidant enzymes of Arabidopsis thaliana. Plant Physiol. 110, 125–136. doi: 10.1104/pp.110.1.125
Ruban, A. V., and Wilson, S. (2021). The mechanism of non-photochemical quenching in plants: Localization and driving forces. Plant Cell Physiol. 62, 1063–1072. doi: 10.1093/pcp/pcaa155
Sairam, R. K., Rao, K. V., and Srivastava, G. (2002). Differential response of wheat genotypes to long term salinity stress in relation to oxidative stress, antioxidant activity and osmolyte concentration. Plant Sci. 163, 1037–1046. doi: 10.1016/S0168-9452(02)00278-9
Sauget, M., Valot, B., Bertrand, X., and Hocquet, D. (2017). Can MALDI-TOF mass spectrometry reasonably type bacteria? Trends Microbiol. 25, 447–455. doi: 10.1016/j.tim.2016.12.006
Schumaker, S., Borror, C. M., and Sandrin, T. R. (2012). Automating data acquisition affects mass spectrum quality and reproducibility during bacterial profiling using an intact cell sample preparation method with matrix-assisted laser desorption/ionization time-of-flight mass spectrometry. Rapid Commun. Mass Spectrom. 26, 243–253. doi: 10.1002/rcm.5309
Seifan, M., Sarabadani, Z., and Berenjian, A. (2020). Microbially induced calcium carbonate precipitation to design a new type of bio self-healing dental composite. Appl. Microbiol. Biotechnol. 104, 2029–2037. doi: 10.1007/s00253-019-10345-9
Singh, S., and Prasad, S. M. (2014). Growth, photosynthesis and oxidative responses of Solanum melongena L. seedlings to cadmium stress: Mechanism of toxicity amelioration by kinetin. Sci. Hortic. 176, 1–10. doi: 10.1016/j.scienta.2014.06.022
Singh, S., Reddy, V., Fleisher, D., and Timlin, D. (2017). Relationship between photosynthetic pigments and chlorophyll fluorescence in soybean under varying phosphorus nutrition at ambient and elevated CO2. Photosynthetica 55, 421–433. doi: 10.1007/s11099-016-0657-0
Sullivan, C. Y., and Ross, W. M. (1979). “Selecting for drought and heat resistance in grain Sorghum,” in Stress physiology in crop plants, eds H. Mussell and R. C. Staples (New York: John Wiley and Sons), 263–281.
Tavares, M. C., Oliveira, K. A., de Fátima, Â., Coltro, W. K. T., and Santos, J. C. C. (2021). Paper-based analytical device with colorimetric detection for urease activity determination in soils and evaluation of potential inhibitors. Talanta 230:122301. doi: 10.1016/j.talanta.2021.122301
Thomas, R. L., Jen, J. J., and Morr, C. V. (1982). Changes in soluble and bound peroxidase—IAA oxidase during tomato fruit development. J. Food Sci. 47, 158–161. doi: 10.1111/j.1365-2621.1982.tb11048.x
Vitória, A. P., Lea, P. J., and Azevedo, R. A. (2001). Antioxidant enzymes responses to cadmium in radish tissues. Phytochemistry 57, 701–710. doi: 10.1016/s0031-9422(01)00130-3
Vos, P., Garrity, G. M., Jones, D., Krieg, N. R., Ludwig, W., Rainey, F. A., et al. (2009). Bergey’s manual of systematic bacteriology. Volume 3: The firmicutes. New York, NY: Springer, 1450. doi: 10.1007/978-0-387-68489-5
Wang, L., Wang, Z., Xu, Y., Joo, S. H., Kim, S. K., Xue, Z., et al. (2009). OsGSR1 is involved in crosstalk between gibberellins and brassinosteroids in rice. Plant J. 57, 498–510. doi: 10.1111/j.1365-313X.2008.03707.x
Whiffin, V. S., Van Paassen, L. A., and Harkes, M. P. (2007). Microbial carbonate precipitation as a soil improvement technique. Geomicrobiol. J. 24, 417–423. doi: 10.1080/01490450701436505
Keywords: antioxidant system, Bacillus, reactive oxygen species, sandy soil, silicon, wheat production
Citation: Desoky E-SM, Rady MM, Nader MM, Mostafa NG, Elrys AS, Mathai A, AbuQamar SF, El-Tarabily KA and El-Saadony MT (2022) Integrated application of bacterial carbonate precipitation and silicon nanoparticles enhances productivity, physiological attributes, and antioxidant defenses of wheat (Triticum aestivum L.) under semi-arid conditions. Front. Plant Sci. 13:947949. doi: 10.3389/fpls.2022.947949
Received: 19 May 2022; Accepted: 22 August 2022;
Published: 25 October 2022.
Edited by:
Heba Mahmoud Mohammad Abdel-Aziz, Mansoura University, EgyptReviewed by:
Suresh Kaushik, Indian Agricultural Research Institute (ICAR), IndiaCopyright © 2022 Desoky, Rady, Nader, Mostafa, Elrys, Mathai, AbuQamar, El-Tarabily and El-Saadony. This is an open-access article distributed under the terms of the Creative Commons Attribution License (CC BY). The use, distribution or reproduction in other forums is permitted, provided the original author(s) and the copyright owner(s) are credited and that the original publication in this journal is cited, in accordance with accepted academic practice. No use, distribution or reproduction is permitted which does not comply with these terms.
*Correspondence: Synan F. AbuQamar, c2FidXFhbWFyQHVhZXUuYWMuYWU=; Khaled A. El-Tarabily, a3RhcmFiaWx5QHVhZXUuYWMuYWU=
Disclaimer: All claims expressed in this article are solely those of the authors and do not necessarily represent those of their affiliated organizations, or those of the publisher, the editors and the reviewers. Any product that may be evaluated in this article or claim that may be made by its manufacturer is not guaranteed or endorsed by the publisher.
Research integrity at Frontiers
Learn more about the work of our research integrity team to safeguard the quality of each article we publish.