- 1Department of Forest Sciences, University of Helsinki, Helsinki, Finland
- 2College of Forestry, Fujian Agriculture and Forestry University, Fuzhou, China
- 3Natural Resources Institute Finland (LUKE), Helsinki, Finland
- 4Department of Environmental and Biological Sciences, University of Eastern Finland, Kuopio, Finland
- 5Natural Resources Institute Finland (LUKE), Suonenjoki, Finland
Root and stem rot caused by Heterobasidion annosum is a severe problem in boreal Scots pine. Dissecting the features of disease resistance is generally an essential step in resistance breeding in plants and forest trees. In this study, we explored inherent resistance factors of Scots pine against H. annosum. A total of 236 families consisting of 85 full-sib (FS), 35 half-sib population mix (HSpm), and 116 half-sib (HS) families of Scots pine seedlings were inoculated with a H. annosum isolate. We sampled needle tissues before inoculation for terpene measurements and RNA sequencing. Based on the lesion area, the extremes of 12 resistant and 12 susceptible families were selected for further analyses. Necrotic lesions resulting from fungal infection were in a weak to moderate relationship with the plant height. Monoterpenes were the principal terpene compounds observed in Scots pine seedlings. Concentrations of 3-carene were significantly higher in pine genotypes inherently resistant compared with susceptible seedlings. By contrast, susceptible genotypes had significantly higher proportions of α-pinene. Gene ontology analysis of differential expressed transcripts (DETs) revealed that response to biotic factors was enriched in resistant seedlings. Functional characterization of individual DETs revealed that higher expression of transcripts involved in response to abiotic stress was common in susceptible genotypes. This observation was supported by the annotation of hub genes in a key module that was significantly correlated with the lesion trait through weighted gene co-expression network analysis (WGCNA) of 16 HS and HSpm samples. These findings contribute to our understanding of constitutive resistance factors of Scots pine against Heterobasidion root and stem rot diseases.
Introduction
The root and stem rot fungi Heterobasidion parviporum and Heterobasidion annosum within a species complex Heterobasidion annosum sensu lato (Fr.) Bref. are necrotrophic pathogens causing severe diseases in forest trees in the boreal region. The preferential host of H. parviporum is Norway spruce [Picea abies (L.) H. Karst.], whereas H. annosum attacks preferentially Scots pine (Pinus sylvestris L.) and also other species, including Norway spruce and common juniper (Juniperus communis L.) (Müller et al., 2018). Scots pine is among the most common conifer species in Finland. It is estimated that Heterobasidion root rot leads to an economic loss of 50 million euros annually in Finland. Abiotic challenges, e.g., climate change is likely to make the situation worse (La Porta et al., 2008). Recently, Heterobasidion root rot has been found in pine trees in central Finland, which was previously solely common in southern Finland (Piri et al., 2021). Stump treatment using urea or Phlebiopsis gigantea is commonly used to prevent Heterobasidion infection (Kärhä et al., 2018). However, the prospect of using resistant genotypes obtained through breeding is a potential and durable approach to manage root and stem rot diseases. Genetic resistance breeding in forest trees is ongoing in some tree species such as Picea sitchensis and Pinus taeda (Sniezko and Koch, 2017; Naidoo et al., 2019).
Plants including forest trees elicit a series of defense reactions when encountering biotic and abiotic stresses. There are elaborate interactions between biotic and abiotic stress responses. The terminology “cross-tolerance” has recently been used to describe a situation where defense responses induced by one type of stress increase tolerance to other types of stresses (Ramegowda et al., 2020). Nevertheless, abiotic stressors may also result in plant susceptibility to pathogens (Nejat and Mantri, 2017). Chemical defense, including phenolics and terpenes, is a strong defense line for forest trees to fight biotic stress. Terpenes play active roles in plant defense to multiple biotic stresses (Keeling and Bohlmann, 2006; Celedon and Bohlmann, 2019; Toffolatti et al., 2021). For example, (+)-3-carene is a monoterpene constitutively produced in many members of Pinaceae for defense against biotic agents (Fäldt et al., 2003; Hall et al., 2011; Roach et al., 2014). The correlation study of preformed terpene contents in trees and the degree of resistance will aid in a better understanding of their roles in tree defense. Candidate genes in the terpenoid pathway in Scots pine are relevant to H. annosum resistance (Mukrimin et al., 2019). Lignin and flavonoid biosynthetic genes together with phenolic and terpene compounds influence root rot resistance (Kovalchuk et al., 2019; Liu et al., 2022). Such findings have shed light on the feasibility and necessity to identify genetic and chemical markers for the prediction of resistant genotypes to improve resistance in conifer trees against root rot diseases.
A transcriptomic analysis offers substantial information on gene expression on a genomic scale and assists researchers in intensely exploring the molecular cues of tree resistance (Kovalchuk et al., 2013; Celedon et al., 2017; Lowe et al., 2017). Thus, conventional methods to test resistant phenotypes, coupled with novel tools such as metabolomics, genomic, and transcriptomic analysis, would deepen our knowledge in tree defense and further accelerate the breeding process. In this study, we sampled needle tissues prior to inoculation and inoculated 236 Scots pine full-siblings and half-siblings with H. annosum. Terpene measurements and differential expression analysis coupled with weighted gene co-expression network analysis (WGCNA) using RNA sequencing (RNA-seq) data were carried out to investigate defense mechanisms underlying inherent resistance. Moreover, we analyzed individual transcripts according to their roles in signaling pathways that are central for crosstalk between biotic and abiotic stress responses. We aimed to explore constitutive variation in different resistant phenotypes.
Materials and Methods
Plant and Fungal Materials, Needle Sampling, Inoculations, and Measurements
In May 2019, Scots pine seedlings (ca 1-year-old, 1,447 seedling plants in total) consisting of 85 full-sib (FS) families, 35 half-sib population mix (HSpm) families, and 116 half-sib (HS) families (Supplementary Table 1) were provided by the Natural Resources Institute Finland (Luke). Each family had six plants, three treated as control (wounds without fungal infection) and three as infected (wounds plus fungal infection). Seedlings were potted using FPM 420 peat substrate (Kekkilä Professional, Finland), and those from different treatments or family types were placed on separate greenhouse tables. Seedlings within the same treatment and family type were randomly arranged. HSpm was originally composed of 50 families from Poland, southern Sweden (Gullabo), southern Finland (Uusikaupunki), central Finland (Kälviä), and northern Finland (Kolari), with 10 families per region. In total, 35 families survived the transfer to the greenhouse, 2, 7, 7, 9, and 10 families from Poland, southern Sweden, southern, central and northern Finland, respectively.
After a 2-month acclimatization in the greenhouse, needle tissues of each plant were sampled before inoculation and stored at −80°C for further use. One H. annosum isolate (homokaryon, 03007) previously shown to induce strong necrotic lesions was cultured at 20°C on malt extract–sawdust agar plates (MEA-S) containing 2% Scots pine sawdust, 2% malt extract, and 1.8% agar for 2 weeks before inoculation. In July 2019, inoculations were conducted on the stem at ca 15 cm above the greenhouse table according to the following steps: surface sterilization of the stem with 70% ethanol, drilling a hole with the puncher of 2 mm in diameter, and filling the punched hole with MEA-S agar plugs pre-colonized by H. annosum. Control treatments received mock inoculation after wounding. Both treatments were sealed with Parafilm M (Heathrow Scientific, USA). At 3 months post-inoculation, the height of each plant was measured from the bottom to the top branches. At 4 months post-inoculation, sapwood lesions of all plants were measured. Lesion size was the sum of vertical and horizontal lesions as described by Keriö et al. (2015). Lesion area was measured using Fiji-ImageJ 1.51 software (Rueden et al., 2017). Lesion area (family mean) of the control and infected plants in each family type were compared by a two-tailed unpaired t-test. Lesion area of HSpm infected plants from three regions in Finland was compared using one-way analysis of variance (ANOVA) followed by Tukey's multiple comparisons at 0.05 confidence level. Pearson correlation coefficients and p values between lesion size, lesion area, and height based on family means by treatments were computed using the corr. test function in the package psych in R 4.1.0 (R Core Team, 2021; Revelle, 2021).
Heritability Estimation
Heritability of lesion area was estimated at an individual (, ) and family-mean basis (, ) separately in the HS and FS data sets using the following equations:
where is the variance of the general combining ability (GCA), is the variance due to the specific combining ability (SCA), is the error (residual) variance, and nH is the harmonic mean of the ramets per family (in this case, nH = 6.11 and nH = 5.99 for HS and FS, respectively). The variance components were derived from two mixed models where Treatment was a fixed factor and female (HS) or female and male parents (FS) were the random factors. The GCA referred either to the effect of the female parent (HS) or to the average effect of female and male parents (FS). The SCA was an interaction of a specific female–male combination (only estimable from the full-sib data). The analysis was performed using the ASReml software (Gilmour et al., 2015).
Terpene Analysis
Terpene compounds of needles sampled before inoculation from 12 resistant and 12 susceptible families were quantified as described earlier (Liu et al., 2022). Approximately 100 mg fresh weight of needle tissues was ground in liquid nitrogen and was then extracted in 2 mL of n-hexane at room temperature for 2 h and washed twice with 2 mL n-hexane. The compound 1-chloro-octane (70.1 μg) was used as an internal standard. The extracts were analyzed using a 6890A gas chromatograph (Agilent Technologies, USA) equipped with a HP-5MS UI column (30 m × 0.25 mm × 0.25 μm, Agilent J&W Scientific, USA) and connected to a 5,973 inert mass selective detector (Agilent Technologies, USA). Helium was used as the carrier gas, and linear velocity was about 40 cm/s. The pulsed splitless sampling technique was used with the pulse pressure 25 psi and duration of 0.5 min, and 1 μl was injected. The initial oven setpoint temperature of 50°C was held for 1 min and the column temperature was increased from 50 to 115°C at 5°C/min, then to 280°C at 15°C/min and held for 10 min. Mass numbers from m/z 33 to 350 were recorded. Authentic standards, ChemStation software, as well as Wiley275 and Nist17 libraries were used to identify and quantify monoterpenes and sesquiterpenes. Compounds for which authentic standards were not available were quantified using a compound with a similar chemical structure. For each terpene compound, concentrations μg/mg fresh weight of needle tissues were shown as the mean of 36 resistant or 36 susceptible seedlings (12 resistant and 12 susceptible families, 3 seedlings per family), and the comparison between the two groups was assessed by two-tailed unpaired t-test. Pearson correlation coefficients with statistical significances between lesion area and terpene concentrations were computed using the same method as described above.
Transcriptome Analysis
Total RNA was extracted from ca 100 mg of ground needle tissues sampled prior to the inoculation using the method previously described by Chang et al. (1993). The ground sample was transferred to a sterile 2 ml Eppendorf tube, and 900 μl extraction buffer (preheated at 65°C) and 9 μl DTT (1 mol/L) were added. The extraction buffer contained 2% CTAB, 4% PVP (K30), 100 mM Tris-HCl, 25 mM EDTA, and 2 M NaCl. The mixture was vortexed and incubated at 65°C for 15 min. Equal volumes of chloroform: isoamyl alcohol (24:1) were added, followed by mixing well, and centrifugation at 10,000 g at room temperature for 10 min. The upper phase was transferred to a new 2 ml Eppendorf tube and then equal volumes of chloroform: isoamyl alcohol (24:1) were added and the above step was repeated. To precipitate RNA, 1/4 volume of 10M LiCl (42.4 g/mol) was added, mixed well, and kept at 4°C overnight. The sample was centrifuged at 10,000 g at 4°C for 30 min. The supernatant was pipetted out and the pellet was washed by adding 100 μl cold 70% ethanol. The centrifugation was repeated for 5 min. The supernatant was pipetted out and the pellet was dried in the sterile hood. The pellet was resuspended in 10–20 μl nuclease-free water. Extracted RNA was quantified by NanoDrop 2000c (Thermo Fisher Scientific, USA) and quality checked with Agilent 2100 bioanalyzer (Agilent Technologies, Germany). Equal amounts (1 μg) of total RNA from three seedlings per family were pooled together as one sample. Samples of 12 resistant and 12 susceptible families (36 resistant and 36 susceptible seedlings) for terpene detection were also used for RNA-seq. RNA-seq was performed with paired-end (150 bp) sequencing using an Illumina NovaSeq 6000 platform at Novogene (UK). RNA-seq reads for 24 samples are available in the NCBI GEO database with the accession number GSE200311. SortMeRNA v4.2.0 was used to remove ribosomal RNAs from raw reads (Kopylova et al., 2012), followed by the trimming of low-quality reads at Q < 20 in 5-base sliding windows and adaptor sequences by Trimmomatic v0.39 with a minimum length of 50 bp (Bolger et al., 2014). Trimmed reads were qualified by FastQC v0.11.8 and MultiQC v1.8. De novo transcriptome assembly was constructed with trinity 2.11.0 (Grabherr et al., 2011), with setting normalize max read cov 50 and min kmer cov 2. A trinity assembly with a size of 288 Mb was produced with GC content 42.08% and E90N50 value 1,824 bp. Sample-specific alignment was conducted with bowtie2 (Langmead and Salzberg, 2012). Alignment-based quantification was performed with RNA-Seq expression estimation by Expectation-Maximization (RSEM) (Li and Dewey, 2011).
Differential expression analysis for each family type was carried out using the package DESeq2 in R (Love et al., 2014). Transcripts that were expressed over one count in at least four libraries were kept. Transformed data by variance stabilizing transformation (VST) were input to perform principal component analysis (PCA). To produce a list of differentially expressed transcripts (DETs), cut-offs were set as Benjamini-Hochberg adjusted p value (FDR) < 0.05 and fold change (FC) > 2. Gene ontology (GO) enrichment of DETs was analyzed using the R package clusterProfiler (Wu et al., 2021). GO terms of all Trinity assembly transcripts were annotated through Blast2GO (Conesa et al., 2005). The description file of all GO terms (go-basic.obo) was downloaded from the Gene Ontology database. Significantly enriched terms (FDR < 0.05) were eliminated due to gene overlaps using the function reduce_overlap in the package GOplot in R (Walter et al., 2015). The R package WGCNA was used for co-expression network analysis of HS and HSpm samples (Langfelder and Horvath, 2008). Lowly expressed transcripts with count <1 in all libraries and count <100 in at least eight libraries were removed followed by VST transformation. Gene modules were detected using 1-step network construction function blockwiseModules with proper soft-thresholding power, minimum module size of 100, and merging threshold function of 0.25. Hub genes in the key module were identified using cut-offs of eigengene-based connectivity (KME) ≥ 0.8 and geneTraitSignificance ≥ 0.2.
Results
Necrotic Lesions and Correlations With Plant Height
Necrotic lesions observed in the stem sapwood caused by H. annosum infection were larger than those of the control samples (Figure 1A and Supplementary Table 2). The average lesion area of control and infected seedlings was 3.2 mm2 (SE 0.07 mm2) and 6.3 mm2 (SE 0.09 mm2), respectively, with significant differences at p < 0.001 for the comparison between the two treatments in each family type. Variations in lesion size, lesion area, and plant height were detected among families and data of the above traits were normally distributed (Figure 2). Lesion area of infected samples ranged from 3.8 to 10.8, 4.0 to 9.3, and 2.3 to 7.9 mm2 for FS, HS, and HSpm, respectively (Figure 1C and Table 1). Lesion size was strongly correlated with lesion area. Plant height showed a weak to moderate relationship with lesion area, of which coefficients were 0.18 and 0.37 for control and infected samples, respectively (Figure 2). Individual heritability estimates of lesion area of FS and HS were similar, around 0.35, whereas FS represented a larger value on the family-mean basis at 0.53 than HS at 0.38 (Table 2).
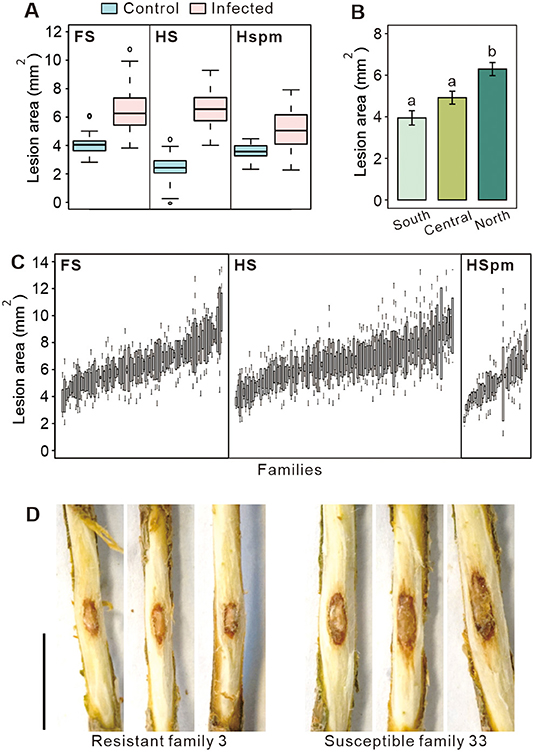
Figure 1. Lesion measurements of Scots pine (Pinus sylvestris L.) seedlings treated as control and Heterobasidion annosum-infected. (A) Boxplots of lesion area of control and infected samples (FS, full-sib, n = 85; HS, half-sib, n = 116; HSpm, half-sib population mix, n = 35). (B) Means of lesion area of infected HSpm families from the south (family 293-302, n = 7), central (family 283-292, n = 9), and north (family 303-312, n = 10) of Finland. Error bars represented the standard error of the mean. Statistical significances were marked with distinct letters above the error bars using one-way ANOVA followed by Turkey's multiple comparisons at the 0.05 confidence level. (C) Boxplots of family-mean lesion area of infected FS, HS, and HSpm seedlings. (D) Necrotic lesions were observed in the sapwood in a resistant family (FS family 3) and a susceptible family (FS family 33). Black lines were the scale bars of 10 mm.
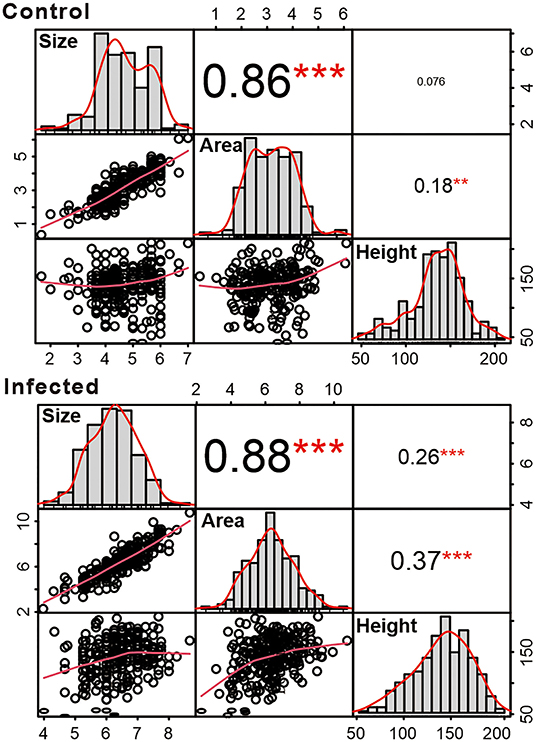
Figure 2. Correlation matrix plot between lesion size, lesion area, and height of Scots pine (Pinus sylvestris L.) families treated as control and H. annosum-infected (n = 236). Bivariate scatterplots with a fitted smooth line were under the diagonal. Pearson correlation coefficients with significance levels were above the diagonal and asterisks indicated the significant differences (*p < 0.05, **p < 0.01, ***p < 0.001).
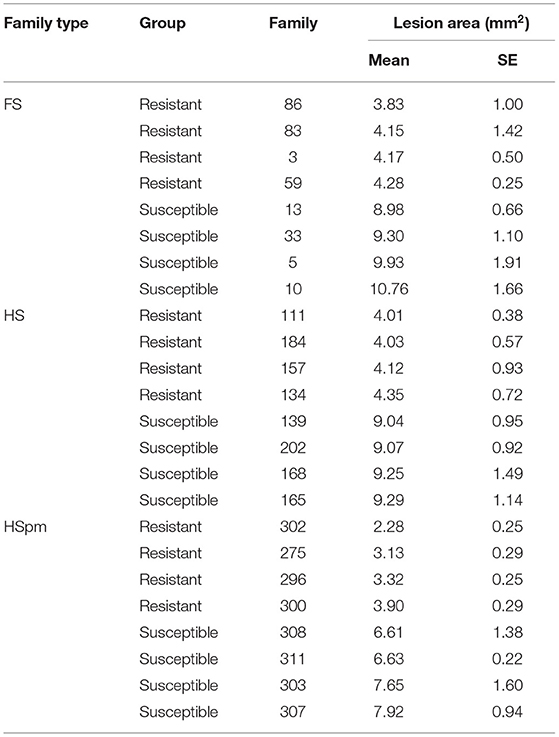
Table 1. Lesion area (mean and standard error, SE) of resistant and susceptible Scots pine (Pinus sylvestris L.) families after being infected by H. annosum (n = 3).

Table 2. Variances of the general and specific combining effects (GCA, SCA) and heritability of lesion area in the HS (half-sib) and FS (full-sib) data of Scots pine (Pinus sylvestris L.) seedlings treated as control and H. annosum-infected.
Seedlings were considered to be resistant if they had lesion areas of 2.3–4.4 mm2 while susceptible seedlings had a lesion area of 6.6–9.9 mm2. Based on the family-mean lesion area, 12 resistant and 12 susceptible families of FS, HS, and HSpm were selected for further terpene and RNA-seq analyses (Table 1). Lesions displayed obvious differences between resistant and susceptible seedlings (Figure 1D). Compared with FS and HS, HSpm seedlings showed a smaller lesion area. Families 302, 296, and 300 in HSpm from southern Finland showed enhanced resistance, whereas 307, 303, 311, and 308 from the north were mostly susceptible. Means of lesion area of infected samples increased from the south (3.9 mm2) to central (4.9 mm2) and north (6.3 mm2) parts of Finland (Figure 1B and Supplementary Table 3).
Differences in Monoterpene Contents Between Resistant and Susceptible Seedlings
From needle tissues of 36 resistant and 36 susceptible seedlings which were sampled prior to inoculation, 17 monoterpene and 12 sesquiterpene compounds were quantified (Supplementary Table 4). Monoterpenes were the predominant terpene compounds, of which total concentration was ca 20-fold higher than sesquiterpenes. α-Pinene and β-caryophyllene showed the highest concentrations among monoterpenes and sesquiterpenes, respectively. A few monoterpenes were significantly different between resistant and susceptible seedlings (Table 3). 3-Carene was highly abundant in resistant genotypes compared to susceptible ones. By contrast, the susceptible group had highly concentrated tricyclene, β-pinene, camphene, limonene+β-phellandrene, p-cymene, and α-pinene. Contents of these compounds were positively highly correlated with each other but negatively correlated with 3-carene (Supplementary Table 5). Correspondingly, lesion area was negatively correlated with 3-carene content with a coefficient at −0.34, but positively with tricyclene and β-pinene at 0.28 and 0.26, respectively.
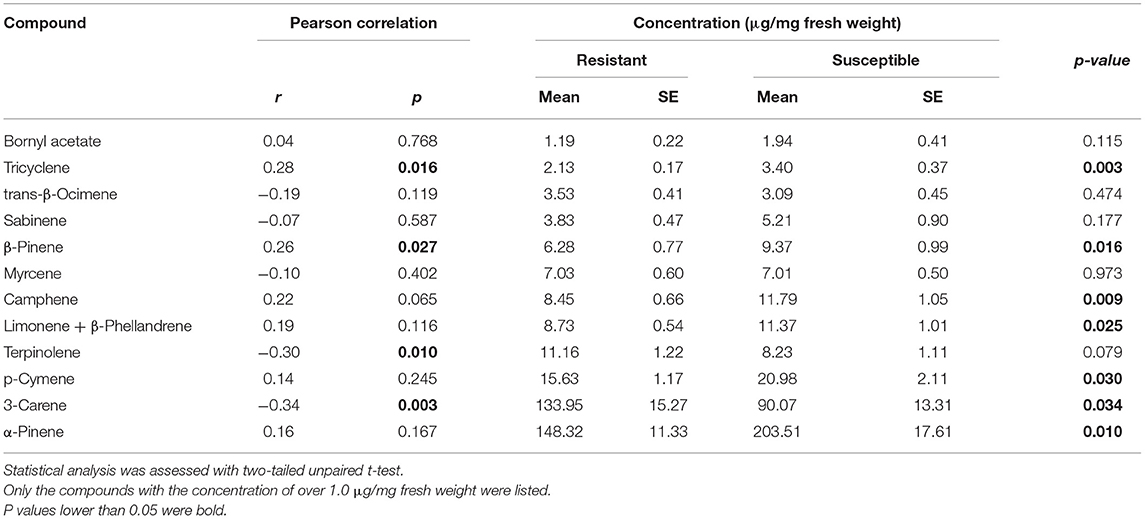
Table 3. Monoterpene concentrations (means and standard error, SE) of resistant and susceptible Scots pine (Pinus sylvestris L.) seedlings and their Pearson correlation coefficients (r) with lesion area (n = 36).
Highly Expressed Transcripts in Resistant or Susceptible Families
Each library obtained 37–54 million paired-end reads, which were de novo-assembled through the Trinity platform with alignment rates of 91.4–98.9%. Although we mapped the reads against an alternative reference genome of Pinus taeda (Pyhäjärvi et al., 2020), the mapping rates were very low for each library. Samples differing in resistance to H. annosum were separated along the y-axis, x-axis, and the diagonal for FS, HS, and HSpm, respectively, in PCA plots (Figure 3).
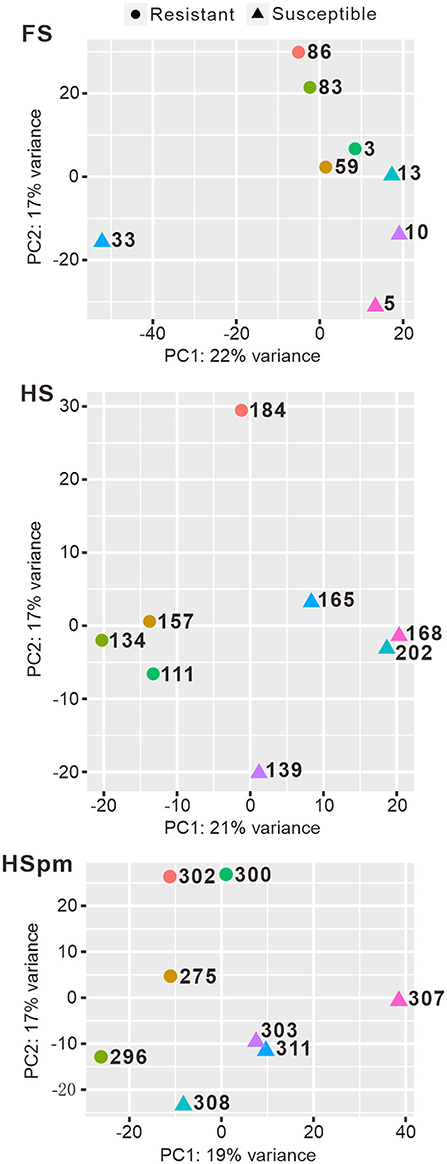
Figure 3. Principal component analysis (PCA) of the expression level of transcripts in resistant and susceptible Scots pine (Pinus sylvestris L.) seedlings.
Differential expression analysis of each family type identified transcripts that were highly expressed either in resistant or susceptible seedlings (Supplementary Table 6). Functional analysis of subsets of DETs showed two significantly enriched GO terms in HSpm and 23 terms in HS (Figure 4). Resistant samples of HSpm were enriched in DETs involved in the flavonol biosynthetic process and response to other organisms. While HS-resistant samples were enriched in auxin metabolic process and linoleate 9S-lipoxygenase activity, other terms such as hormone activity, cellular response to heat, and wax biosynthetic process were mostly prevalent in susceptible genotypes.
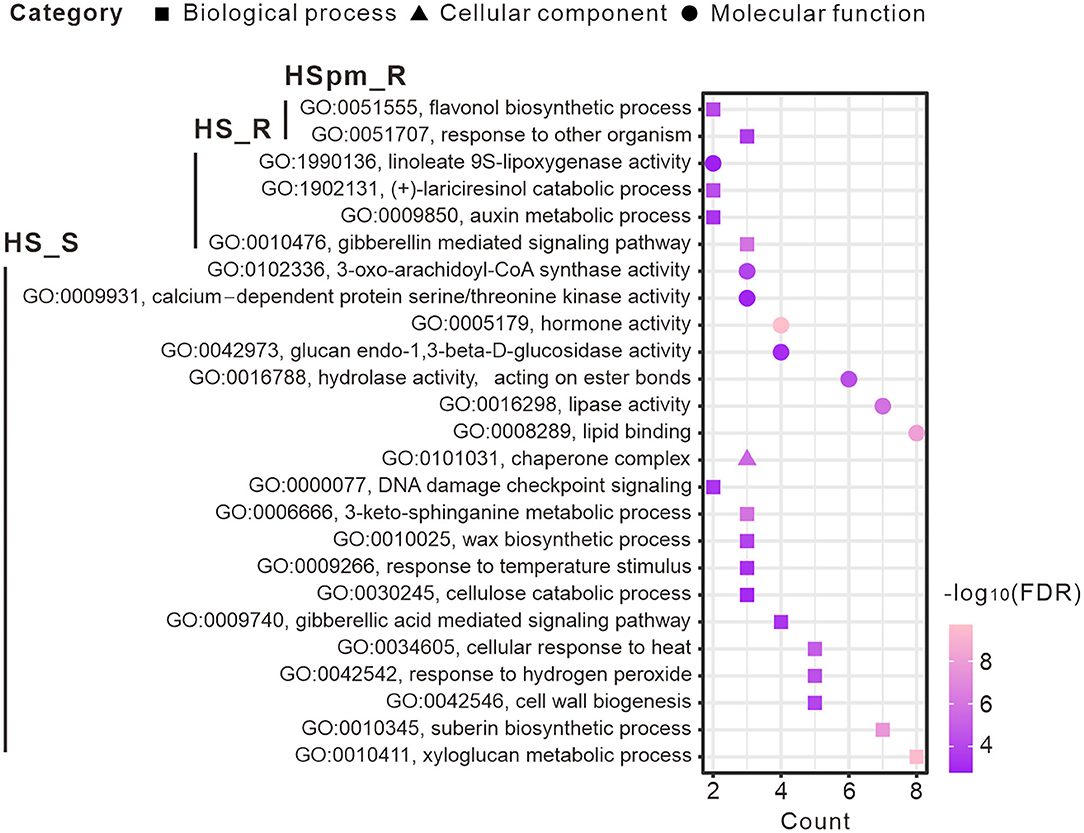
Figure 4. Enriched GO terms of DETs under biological process, cellular component, and molecular function.
Among DETs of each family type, 36–57% were annotated (Supplementary Tables 6, 7). Signaling was primarily mediated by plant hormones such as jasmonic acid, abscisic acid, and gibberellins (Figure 5). Calcineurin B-like (CBL) interacting-protein kinases (CIPKs), RALF-like proteins (RALFLs), and calcium-dependent protein kinases (CDPKs) in susceptible seedlings could play a role to regulate calcium-dependent signaling. Despite differences in resistant capability against H. annosum, all samples had highly expressed transcripts involved in defense encoding disease resistance proteins, pathogenesis-related proteins, and key enzymes in the phenylpropanoid and terpenoid biosynthesis. Susceptible samples showed increases in DETs such as MYB transcription factors (MYBs) and triphosphate tunnel metalloenzyme 2 (TTM2), as well as abundant DETs associated with abiotic stress responses (Figure 5). Susceptible samples of HS had increased levels of 22 transcripts encoding heat shock proteins (HSPs), but negligible transcript levels were observed in other samples.
In addition to functional characterization of DETs, we applied WGCNA to shed light on co-expression networks between transcripts according to their common expression trends across all half-sib (HS and HSpm) samples. After filtering, 20,618 transcripts were used to construct the network to find key modules of highly correlated genes. Samples from the same family type, having a similar lesion area clustered well (Figure 6A), where red means a large lesion and white a small lesion. Using the soft-thresholding power of 6, we identified 49 modules (Supplementary Figures 1A,B). Module size ranged from 123 to 2,448 transcripts. There were 957 transcripts in the gray module that failed to cluster to any module. The dark gray, saddle brown, and pale turquoise modules were significantly correlated with lesion area with coefficients at −0.53, 0.59, and 0.63, respectively (Supplementary Figure 1C). We considered the pale turquoise as the key module since it was closely clustered with the lesion area (Figure 6B). A total of 196 transcripts in the module were highly correlated with the lesion area (Figure 6C). Among them, 72 transcripts were hub genes with high connectivity, and 58 of them were annotated with 20 transcripts for HSPs (Supplementary Table 8), mostly enhanced in the susceptible genotypes. Hub genes are transcripts with high intramodular connectivity, which tend to be strongly correlated with the lesion trait.
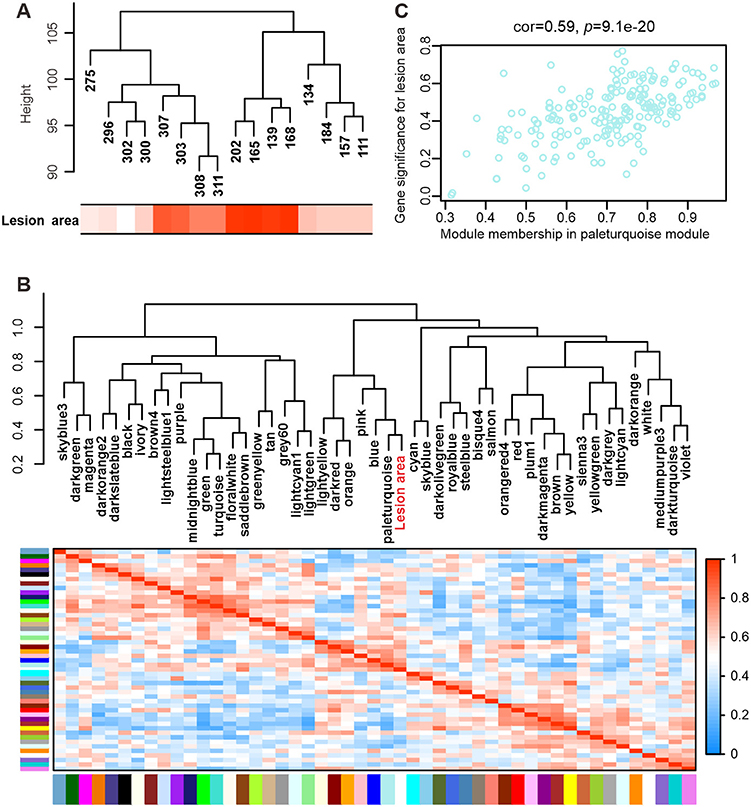
Figure 6. Weighted gene co-expression network analysis (WGCNA) of HS (half-sib) and HSpm (half-sib population mix) samples. (A) Clustering dendrogram of samples based on their Euclidean distance and the heatmap of lesion area. (B) Visualization of the eigengene network representing the relationships among modules and lesion area. Panel (above) shows a hierarchical clustering dendrogram of the eigengenes. Panel (below) displays a heatmap of the eigengene adjacency. (C) Scatterplot gene significance for lesion area vs. module membership in the paleturquoise module.
Discussion
Lesion area, instead of lesion size, was used to evaluate resistant phenotypes, which further highlights the differences in response to fungal infection, especially for small-sized plants (Liu et al., 2022). We reasoned that lesion area could be a more accurate method to evaluate resistance partly based on the dichotomous branching and mycelia nature of fungal morphology during invasive growth. Variation in lesion area among families indicated that susceptibility to H. annosum varied among genotypes. Lesions observed in wounded control samples implied that defense responses could be triggered by mock inoculation (Lim et al., 2021). Moderate estimates of heritability for lesion area in FS and HS suggested that the lesion trait was influenced by host genetics. Similar results regarding disease resistance in Scots pine have been reported. Narrow-sense heritability estimates of 0.38–0.75 for Dothistroma needle blight severity differed between half-siblings and full-siblings (Perry et al., 2016). A narrow-sense heritability of 0.37 for root rot resistance was higher than those for many growth traits (Rieksts-Riekstinš et al., 2020), and the high estimates were documented to be valuable for breeding (Marčiulynas et al., 2020). A positive relationship between height and lesion area indicated that seedlings of larger size were much more susceptible to H. annosum. These results were consistent with a previous study by Mukrimin et al. (2019). They reported a significant correlation of 0.53 between stem diameter and phloem vertical lesion length of Scots pine seedlings at 10–15 years old resulting from H. annosum infection, but a negligible value for the mock control. In Norway spruce (17 years old), growth traits including diameter and height were positively correlated with lesion length in the inner bark and fungal growth (Swedjemark and Karlsson, 2004). Results with respect to phenotyping measurements suggested that resistance to H. annosum in Scots pine seedlings was under genetic control and supported the growth and defense trade-off hypothesis, where bigger seedlings are susceptible to fungal infection with larger lesions.
Monoterpenes, as major constituents of oleoresin, play essential roles in the physical and chemical defense of conifer trees (Kovalchuk et al., 2013). Similar to our observations, 3-carene and α-pinene were the most abundant terpene compounds in needles of Scots pine and whitebark pine trees either under healthy conditions or infected by fungal pathogens (Bullington et al., 2018; Mukrimin et al., 2019). Several highly concentrated monoterpenes were detected in tree genotypes susceptible to H. annosum. Terpenoid biosynthesis was documented to be enriched in Masson pine that was susceptible to pine wood nematode compared to resistant-genotypes (Liu et al., 2017). Mukrimin et al. (2019) found that the expression of α-pinene synthase in Scots pine had a positive relationship with lesion size. By contrast, increased monoterpene contents (α-pinene, tricyclene, and α-longipinene) were observed in asymptomatic (resistant) Norway spruce genotypes infected with Heterobasidion (Kovalchuk et al., 2019; Liu et al., 2022). Two subspecies of Scots pine showed different terpene concentrations and proportions (Achotegui-Castells et al., 2013). Variation in terpene concentrations between the samples of different susceptibility demonstrated constitutive genetic differences in resistance. Other authors have noted that the monoterpene amount increases in resistant trees after the induction of defense responses, but remains unchanged in susceptible trees (Hall et al., 2011). Thus, monoterpene contents merit to be further explored as a chemical marker for resistance or susceptibility. In our study, 3-carene was the only compound showing a negative and moderate correlation with lesion area. Notably, Scots pine trees in the south of Finland had lower α-pinene and higher 3-carene contents, but the opposite composition for the trees from the north (Muona et al., 1986; Manninen et al., 2002). In this study, smaller lesions in seedlings from southern Finland with enhanced resistance were observed. It is possible that the increased resistance could be due to the high 3-carene concentration prevalent in seedlings from southern Finland compared to northern Finland.
According to the transcriptome analysis, resistant and susceptible seedlings had comparable amounts of DETs involved in signaling pathways. Susceptible seedlings particularly in the HS family type showed a predominantly larger number of DETs associated with abiotic stress responses, which was in line with our previous findings (Liu et al., 2022). The WGCNA study displayed a large proportion at 34.5% of hub genes for HSPs, which might contribute to enhanced seedling susceptibility to H. annosum. Mechanisms governing abiotic stress tolerance have been shown to have both synergistic and antagonistic effects on disease resistance (Kissoudis et al., 2014). Dissecting the interaction between stress signaling pathways can aid in understanding these different effects.
MYB transcription factors are a group of components in the modulation of stress signal transduction, regulating stress responses such as the biosynthesis of the cell wall and secondary metabolites related to defense. PtMYB1, PtMYB4, and PtMYB8 are involved in the phenylpropanoid pathway in loblolly pine, and PtMYB14 induces the sesquiterpene production in conifer trees (Bedon et al., 2010). According to the review by Ramegowda et al. (2020), OsMYB4, MYB96, and PacMYBA confer tolerance to abiotic stress and resistance against pathogens in Arabidopsis, where ABA-SA signaling networks had influence. Members of various MYB families can positively and negatively regulate cold stress responses in Arabidopsis and Korean pine (Wang et al., 2020).
Heat shock triggers the production of a group of low molecular weight proteins, HSPs in spruce and pine seedlings (Gifford and Taleisnik, 1994). As molecular chaperones, HSPs have been considered as stress proteins, enabling the activation and stabilization of signaling proteins including hormone receptors, transcription factors (TFs), and kinases (Xiang et al., 2018). The main functions of HSPs were summarized by Wang et al. (2004), with roles in protein folding, assembly, translocation, and degradation; the stabilization of proteins and membranes; and protein refolding under stress conditions. Most HSPs detected in this study belonged to the small HSP family with a molecular mass of around 20 kDa. Small HSPs in plants respond to multiple environmental stressors such as heat, cold, drought, salinity stress, and their accumulation is promisingly useful to improve tolerance to stress (Wang et al., 2004; Xiang et al., 2018). Even though roles of HSPs relevant to stress tolerance have not been much studied in conifer trees compared with crop plants, a real-time PCR study of loblolly pine revealed that HSP genes can be induced by drought stress (Vásquez-Robinet et al., 2010), and seasonal variation in the HSP content in needles of Scots pine assists plants to adapt to temperature changes all year round (Korotaeva et al., 2012).
According to GO analysis, resistant seedlings evoke a variety of responses to repel a fungal attack, for instance, lipoxygenase (LOX) together with the ethylene response factor (ERF) and the TF function in response to necrotrophs (Bürger and Chory, 2019). Transcripts associated with Ca2+-mediated signaling, MYBs and HSPs, represented higher abundance in susceptible seedlings, which suggests that the activation of signaling pathways in abiotic stress might enhance susceptibility to root rot. Susceptible seedlings had other DETs encoding defense proteins (Supplementary Table 7). A negative regulator of SA-signaling, TTM2, has a negative effect on the defense response to pathogens (Ung et al., 2014). EDS1 cooperating with PAD4 triggers a hypersensitive response (HR) that cannot control the invasive growth of necrotrophic pathogens, consequently resulting in larger lesions (Feys et al., 2001). Protein EXORDIUM in the brassino-steroid-dependent regulation of growth-defense trade-offs can promote growth but suppress disease resistance (Coll-Garcia et al., 2004; Bürger and Chory, 2019), which is in agreement with positive correlations between height and lesion area in our study. Besides, it appears that seedlings from northern Finland showed enhanced tolerance to environmental challenges during transportation and re-potting, whereas they were more susceptible to infection. The results further lend credence to the fact that seedlings with tolerance to abiotic stress seem to be more susceptible to H. annosum.
In this study, we selected a set of candidate resistant and susceptible full-sib and half-sib families. The resistance ability of their mother trees can be tested further, which will provide promisingly valuable plant materials for genetic breeding programs in Scots pine. We resorted to chemical and transcriptome analysis to understand the inherent resistance to H. annosum in Scots pine. The results revealed that resistant genotypes had higher contents of 3-carene, whereas susceptible genotypes had higher concentrations of α-pinene. Terpene composition, especially for α-pinene and 3-carene, also seems to be influenced by the geographical origin of the Scots pine families. Additionally, transcripts associated with abiotic and biotic response interactions were found to be relevant to increased susceptibility of the seedlings. Compared with our previous work on Norway spruce (Liu et al., 2022), the results revealed some commonality and differences in the metabolic pathway for disease resistance in these two conifer species. Overall, our findings indicate that the approach of sampling needle tissue prior to destructive inoculation may have a potential merit for speeding up the large-scale disease resistance screening work.
Data Availability Statement
The datasets presented in this study can be found in online repositories. The names of the repository/repositories and accession number(s) can be found in the article/Supplementary Material.
Author Contributions
FA and ML designed the study. ML performed greenhouse experiments and extracted RNA. MH estimated the heritability. RG and MK conducted terpene detection. ML and KW analyzed the data and drafted the manuscript. FA conceived the study and helped to draft the manuscript. All authors edited and revised the manuscript.
Funding
The study was supported by the research funding from Academy of Finland (Grant No. 307580) to FA, scholarship from China Scholarship Council (Grant No. 201607960002), and Niemi Foundation (Grant No. 20200006) to ML.
Conflict of Interest
The authors declare that the research was conducted in the absence of any commercial or financial relationships that could be construed as a potential conflict of interest.
Publisher's Note
All claims expressed in this article are solely those of the authors and do not necessarily represent those of their affiliated organizations, or those of the publisher, the editors and the reviewers. Any product that may be evaluated in this article, or claim that may be made by its manufacturer, is not guaranteed or endorsed by the publisher.
Acknowledgments
We thank Florian Pusch and Julia Wenzel from University of Dresden, and Antoine Taupenot from École normale supérieure Paris-Saclay for their help in the greenhouse work. We acknowledge CSC (IT Center for Science, Finland) for computational resources. We are grateful to Genowood consortium for the supply of plant materials.
Supplementary Material
The Supplementary Material for this article can be found online at: https://www.frontiersin.org/articles/10.3389/fpls.2022.947734/full#supplementary-material
References
Achotegui-Castells, A., Llusia, J., Hódar, J. A., and Penuelas, J. (2013). Needle terpene concentrations and emissions of two coexisting subspecies of Scots pine attacked by the pine processionary moth (Thaumetopoea pityocampa). Acta Physiol. Plant. 35, 3047–3058. doi: 10.1007/s11738-013-1337-3
Bedon, F., Bomal, C., Caron, S., Levasseur, C., Boyle, B., Mansfield, S. D., et al. (2010). Subgroup 4 R2R3-MYBs in conifer trees: gene family expansion and contribution to the isoprenoid-and flavonoid-oriented responses. J. Exp. Bot. 61, 3847–3864. doi: 10.1093/jxb/erq196
Bolger, A. M., Lohse, M., and Usadel, B. (2014). Trimmomatic: a flexible trimmer for Illumina sequence data. Bioinformatics 30, 2114–2120. doi: 10.1093/bioinformatics/btu170
Bullington, L. S., Lekberg, Y., Sniezko, R., and Larkin, B. (2018). The influence of genetics, defensive chemistry and the fungal microbiome on disease outcome in whitebark pine trees. Mol. Plant Pathol. 19, 1847–1858. doi: 10.1111/mpp.12663
Bürger, M., and Chory, J. (2019). Stressed out about hormones: how plants orchestrate immunity. Cell Host Microbe 26, 163–172. doi: 10.1016/j.chom.2019.07.006
Celedon, J. M., and Bohlmann, J. (2019). Oleoresin defenses in conifers: chemical diversity, terpene synthases and limitations of oleoresin defense under climate change. N. Phytol. 224, 1444–1463. doi: 10.1111/nph.15984
Celedon, J. M., Yuen, M. M., Chiang, A., Henderson, H., Reid, K. E., and Bohlmann, J. (2017). Cell-type-and tissue-specific transcriptomes of the white spruce (Picea glauca) bark unmask fine-scale spatial patterns of constitutive and induced conifer defense. Plant J. 92, 710–726. doi: 10.1111/tpj.13673
Chang, S., Puryear, J., and Cairney, J. (1993). A simple and efficient method for isolating RNA from pine trees. Plant Mol. Biol. Rep. 11, 113–116. doi: 10.1007/BF02670468
Coll-Garcia, D., Mazuch, J., Altmann, T., and Müssig, C. (2004). EXORDIUM regulates brassinosteroid-responsive genes. FEBS Lett. 563, 82–86. doi: 10.1016/S0014-5793(04)00255-8
Conesa, A., Götz, S., García-Gómez, J. M., Terol, J., Talón, M., and Robles, M. (2005). Blast2GO: a universal tool for annotation, visualization and analysis in functional genomics research. Bioinformatics 21, 3674–3676. doi: 10.1093/bioinformatics/bti610
Fäldt, J., Martin, D., Miller, B., Rawat, S., and Bohlmann, J. (2003). Traumatic resin defense in Norway spruce (Picea abies): methyl jasmonate-induced terpene synthase gene expression, and cDNA cloning and functional characterization of (+)-3-carene synthase. Plant Mol. Biol. 51, 119–133. doi: 10.1023/A:1020714403780
Feys, B. J., Moisan, L. J., Newman, M. A., and Parker, J. E. (2001). Direct interaction between the Arabidopsis disease resistance signaling proteins, EDS1 and PAD4. EMBO J. 20, 5400–5411. doi: 10.1093/emboj/20.19.5400
Gifford, D. J., and Taleisnik, E. (1994). Heat-shock response of Pinus and Picea seedlings. Tree Physiol. 14, 103–110.
Gilmour, A., Gogel, B., Cullis, B., Welham, S., and Thompson, R. (2015). ASReml User Guide Release 4.1 Structural Specification. Hemel hempstead: VSN international ltd.
Grabherr, M. G., Haas, B. J., Yassour, M., Levin, J. Z., Thompson, D. A., Amit, I., et al. (2011). Full-length transcriptome assembly from RNA-Seq data without a reference genome. Nat. Biotechnol. 29, 644–652. doi: 10.1038/nbt.1883
Hall, D. E., Robert, J. A., Keeling, C. I., Domanski, D., Quesada, A. L., Jancsik, S., et al. (2011). An integrated genomic, proteomic and biochemical analysis of (+)-3-carene biosynthesis in Sitka spruce (Picea sitchensis) genotypes that are resistant or susceptible to white pine weevil. Plant J. 65, 936–948. doi: 10.1111/j.1365-313X.2010.04478.x
Kärhä, K., Koivusalo, V., Palander, T., and Ronkanen, M. (2018). Treatment of Picea abies and Pinus sylvestris stumps with urea and Phlebiopsis gigantea for control of Heterobasidion. Forests 9, 139. doi: 10.3390/f9030139
Keeling, C. I., and Bohlmann, J. (2006). Genes, enzymes and chemicals of terpenoid diversity in the constitutive and induced defence of conifers against insects and pathogens. New Phytol. 170, 657–675. doi: 10.1111/j.1469-8137.2006.01716.x
Keriö, S., Niemi, S., Haapanen, M., Daniel, G., and Asiegbu, F. (2015). Infection of Picea abies clones with a homokaryotic isolate of Heterobasidion parviporum under field conditions. Can. J. For. Res. 45, 227–235. doi: 10.1139/cjfr-2014-0247
Kissoudis, C., van de Wiel, C., Visser, R. G. F., and van der Linden, G. (2014). Enhancing crop resilience to combined abiotic and biotic stress through the dissection of physiological and molecular crosstalk. Front. Plant Sci. 5, 207. doi: 10.3389/fpls.2014.00207
Kopylova, E., Noé, L., and Touzet, H. (2012). SortMeRNA: fast and accurate filtering of ribosomal RNAs in metatranscriptomic data. Bioinformatics 28, 3211–3217. doi: 10.1093/bioinformatics/bts611
Korotaeva, N., Oskorbina, M., Kopytova, L., Suvorova, G., Borovskii, G., and Voinikov, V. (2012). Variations in the content of stress proteins in the needles of common pine (Pinus sylvestris L.) within an annual cycle. J. For. Res. 17, 89–97. doi: 10.1007/s10310-011-0260-y
Kovalchuk, A., Kerio, S., Oghenekaro, A. O., Jaber, E., Raffaello, T., and Asiegbu, F. O. (2013). Antimicrobial defenses and resistance in forest trees: challenges and perspectives in a genomic era. Annu. Rev. Phytopathol. 51, 221–244. doi: 10.1146/annurev-phyto-082712-102307
Kovalchuk, A., Zeng, Z., Ghimire, R. P., Kivimäenpää, M., Raffaello, T., Liu, M., et al. (2019). Dual RNA-seq analysis provides new insights into interactions between Norway spruce and necrotrophic pathogen Heterobasidion annosum s.l. BMC Plant Biol. 19, 2. doi: 10.1186/s12870-018-1602-0
La Porta, N., Capretti, P., Thomsen, I. M., Kasanen, R., Hietala, A. M., and Von Weissenberg, K. (2008). Forest pathogens with higher damage potential due to climate change in Europe. Can. J. Plant. Pathol. 30, 177–195. doi: 10.1080/07060661.2008.10540534
Langfelder, P., and Horvath, S. (2008). WGCNA: an R package for weighted correlation network analysis. BMC Bioinformatics. 9, 559. doi: 10.1186/1471-2105-9-559
Langmead, B., and Salzberg, S. L. (2012). Fast gapped-read alignment with Bowtie 2. Nat. Methods 9, 357–359. doi: 10.1038/nmeth.1923
Li, B., and Dewey, C. N. (2011). RSEM: accurate transcript quantification from RNA-Seq data with or without a reference genome. BMC Bioinformatics. 12, 323. doi: 10.1186/1471-2105-12-323
Lim, K. J., Paasela, T., Harju, A., Venäläinen, M., Paulin, L., Auvinen, P., et al. (2021). A transcriptomic view to wounding response in young Scots pine stems. Sci. Rep. 11, 3778. doi: 10.1038/s41598-021-82848-3
Liu, M., Wang, K., Ghimire, R., Haapanen, M., Kivimäenpää, M., and Asiegbu, F. O. (2022). Molecular and chemical screening for inherent disease resistance factors of Norway spruce (Picea abies) clones against conifer stem rot pathogen Heterobasidion parviporum. Phytopathology 112, 872–880. doi: 10.1094/PHYTO-09-21-0379-R
Liu, Q., Wei, Y., Xu, L., Hao, Y., Chen, X., and Zhou, Z. (2017). Transcriptomic profiling reveals differentially expressed genes associated with pine wood nematode resistance in masson pine (Pinus massoniana Lamb.). Sci. Rep. 7, 4693. doi: 10.1038/s41598-017-04944-7
Love, M. I., Huber, W., and Anders, S. (2014). Moderated estimation of fold change and dispersion for RNA-seq data with DESeq2. Genome Biol. 15, 550. doi: 10.1186/s13059-014-0550-8
Lowe, R., Shirley, N., Bleackley, M., Dolan, S., and Shafee, T. (2017). Transcriptomics technologies. PLoS Comp. Biol. 13, e1005457. doi: 10.1371/journal.pcbi.1005457
Manninen, A.-M., Tarhanen, S., Vuorinen, M., and Kainulainen, P. (2002). Comparing the variation of needle and wood terpenoids in Scots pine provenances. J. Chem. Ecol. 28, 211–228. doi: 10.1023/A:1013579222600
Marčiulynas, A., Sirgedaite-ŠeŽiene, V., Žemaitis, P., Jansons, Ā., and Baliuckas, V. (2020). Resistance of Scots pine half-sib families to Heterobasidion annosum in progeny field trials. Silva Fenn. 54, 10276. doi: 10.14214/sf.10276
Mukrimin, M., Kovalchuk, A., Ghimire, R. P., Kivimäenpää, M., Sun, H., Holopainen, J. K., et al. (2019). Evaluation of potential genetic and chemical markers for Scots pine tolerance against Heterobasidion annosum infection. Planta 250, 1881–1895. doi: 10.1007/s00425-019-03270-8
Müller, M. M., Henttonen, H. M., Penttilä, R., Kulju, M., Helo, T., and Kaitera, J. (2018). Distribution of Heterobasidion butt rot in northern Finland. For. Ecol. Manage. 425, 85–91. doi: 10.1016/j.foreco.2018.05.047
Muona, O., Hiltunen, R., Shaw, D., and Morén, E. (1986). Analysis of monoterpene variation in natural stands and plustrees of Pinus sylvestris in Finland. Silva Fenn. 20, 5356. doi: 10.14214/sf.a15435
Naidoo, S., Slippers, B., Plett, J. M., Coles, D., and Oates, C. N. (2019). The road to resistance in forest trees. Front. Plant Sci. 10, 273. doi: 10.3389/fpls.2019.00273
Nejat, N., and Mantri, N. (2017). Plant immune system: crosstalk between responses to biotic and abiotic stresses the missing link in understanding plant defence. Curr. Issues Mol. Biol. 23, 1–16. doi: 10.21775/cimb.023.001
Perry, A., Wachowiak, W., Brown, A., Ennos, R., Cottrell, J., and Cavers, S. (2016). Substantial heritable variation for susceptibility to Dothistroma septosporum within populations of native British Scots pine (Pinus sylvestris). Plant Pathol. 65, 987–996. doi: 10.1111/ppa.12528
Piri, T., Vainio, E. J., Nuorteva, H., and Hantula, J. (2021). High seedling mortality of Scots pine caused by Heterobasidion annosum ss. Forests 12, 1289. doi: 10.3390/f12091289
Pyhäjärvi, T., Kujala, S. T., and Savolainen, O. (2020). 275 years of forestry meets genomics in Pinus sylvestris. Evol. Appl. 13, 11–30. doi: 10.1111/eva.12809
R Core Team. (2021). R: A Language and Environment for Statistical Computing. Vienna: R Foundation for Statistical Computing. Available online at: https://www.R-project.org/
Ramegowda, V., Da Costa, M. V. J., Harihar, S., Karaba, N. N., and Sreeman, S. M. (2020). “Abiotic and biotic stress interactions in plants: a cross-tolerance perspective,” in Priming-Mediated Stress and Cross-Stress Tolerance in Crop Plants, eds M. A. Hossain, F. Liu, D. J. Burritt, M. Fujita, and B. Huang (Cambridge, MA: Academic Press), 267–302.
Revelle, W. (2021). psych: Procedures for Personality and Psychological Research. Northwestern University, Evanston, IL, United States. Available online at: https://CRAN.R-project.org/package=psychVersion=2.1.9
Rieksts-Riekstinš, R., Zeltinš, P., Baliuckas, V., Bruna, L., Zaluma, A., and Kāpostinš, R. (2020). Pinus sylvestris breeding for resistance against natural infection of the fungus Heterobasidion annosum. Forests 11, 23. doi: 10.3390/f11010023
Roach, C. R., Hall, D. E., Zerbe, P., and Bohlmann, J. (2014). Plasticity and evolution of (+)-3-carene synthase and (-)-sabinene synthase functions of a Sitka spruce monoterpene synthase gene family associated with weevil resistance. J. Biol. Chem. 289, 23859–23869. doi: 10.1074/jbc.M114.571703
Rueden, C. T., Schindelin, J., Hiner, M. C., DeZonia, B. E., Walter, A. E., Arena, E. T., et al. (2017). ImageJ2: ImageJ for the next generation of scientific image data. BMC Bioinformatics. 18, 529. doi: 10.1186/s12859-017-1934-z
Sniezko, R. A., and Koch, J. (2017). Breeding trees resistant to insects and diseases: putting theory into application. Biol. Invasions 19, 3377–3400. doi: 10.1007/s10530-017-1482-5
Swedjemark, G., and Karlsson, B. (2004). Genotypic variation in susceptibility following artificial Heterobasidion annosum inoculation of Picea abies clones in a 17-year-old field test. Scand. J. For. Res. 19, 103–111. doi: 10.1080/02827580310018032
Toffolatti, S. L., Maddalena, G., Passera, A., Casati, P., Bianco, P. A., and Quaglino, F. (2021). “Role of terpenes in plant defense to biotic stress,” in Biocontrol Agents and Secondary Metabolites, ed S. Jogaiah (Woodhead publishing), 401–417. doi: 10.1016/B978-0-12-822919-4.00016-8
Ung, H., Moeder, W., and Yoshioka, K. (2014). Arabidopsis triphosphate tunnel metalloenzyme2 is a negative regulator of the salicylic acid-mediated feedback amplification loop for defense responses. Plant Physiol. 166, 1009–1021. doi: 10.1104/pp.114.248757
Vásquez-Robinet, C., Watkinson, J. I., Sioson, A. A., Ramakrishnan, N., Heath, L. S., and Grene, R. (2010). Differential expression of heat shock protein genes in preconditioning for photosynthetic acclimation in water-stressed loblolly pine. Plant Physiol. Biochem. 48, 256–264. doi: 10.1016/j.plaphy.2009.12.005
Walter, W., Sánchez-Cabo, F., and Ricote, M. (2015). GOplot: an R package for visually combining expression data with functional analysis. Bioinformatics 31, 2912–2914. doi: 10.1093/bioinformatics/btv300
Wang, F., Chen, S., Liang, D., Qu, G.-Z., Chen, S., and Zhao, X. (2020). Transcriptomic analyses of Pinus koraiensis under different cold stresses. BMC Genom. 21, 10. doi: 10.1186/s12864-019-6401-y
Wang, W., Vinocur, B., Shoseyov, O., and Altman, A. (2004). Role of plant heat-shock proteins and molecular chaperones in the abiotic stress response. Trends Plant Sci. 9, 244–252. doi: 10.1016/j.tplants.2004.03.006
Wu, T., Hu, E., Xu, S., Chen, M., Guo, P., Dai, Z., et al. (2021). clusterProfiler 4.0: A universal enrichment tool for interpreting omics data. Innovation 2, 100141. doi: 10.1016/j.xinn.2021.100141
Keywords: differential expression, monoterpene, pine, resistance breeding, root rot, WGCNA
Citation: Liu M, Wang K, Haapanen M, Ghimire RP, Kivimäenpää M and Asiegbu FO (2022) Analysis of Transcriptome and Terpene Constituents of Scots Pine Genotypes Inherently Resistant or Susceptible to Heterobasidion annosum. Front. Plant Sci. 13:947734. doi: 10.3389/fpls.2022.947734
Received: 19 May 2022; Accepted: 20 June 2022;
Published: 13 July 2022.
Edited by:
Youlian Pan, National Research Council Canada (NRC-CNRC), CanadaReviewed by:
Ahmed Najar, University of Alberta, CanadaNadir Erbilgin, University of Alberta, Canada
Copyright © 2022 Liu, Wang, Haapanen, Ghimire, Kivimäenpää and Asiegbu. This is an open-access article distributed under the terms of the Creative Commons Attribution License (CC BY). The use, distribution or reproduction in other forums is permitted, provided the original author(s) and the copyright owner(s) are credited and that the original publication in this journal is cited, in accordance with accepted academic practice. No use, distribution or reproduction is permitted which does not comply with these terms.
*Correspondence: Fred O. Asiegbu, ZnJlZC5hc2llZ2J1JiN4MDAwNDA7aGVsc2lua2kuZmk=
†These authors have contributed equally to this work and share first authorship