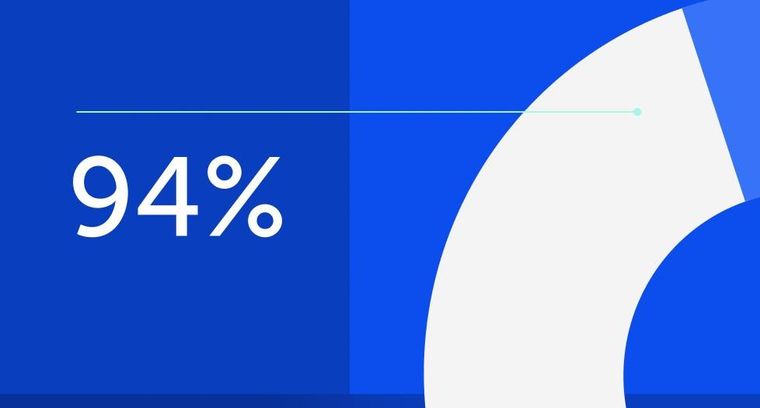
94% of researchers rate our articles as excellent or good
Learn more about the work of our research integrity team to safeguard the quality of each article we publish.
Find out more
REVIEW article
Front. Plant Sci., 07 September 2022
Sec. Plant Abiotic Stress
Volume 13 - 2022 | https://doi.org/10.3389/fpls.2022.946922
Salt stress severely limits the productivity of crop plants worldwide and its detrimental effects are aggravated by climate change. Due to a significant world population growth, agriculture has expanded to marginal and salinized regions, which usually render low crop yield. In this context, finding methods and strategies to improve plant tolerance against salt stress is of utmost importance to fulfill food security challenges under the scenario of the ever-increasing human population. Plant priming, at different stages of plant development, such as seed or seedling, has gained significant attention for its marked implication in crop salt-stress management. It is a promising field relying on the applications of specific chemical agents which could effectively improve plant salt-stress tolerance. Currently, a variety of chemicals, both inorganic and organic, which can efficiently promote plant growth and crop yield are available in the market. This review summarizes our current knowledge of the promising roles of diverse molecules/compounds, such as hydrogen sulfide (H2S), molecular hydrogen, nitric oxide (NO), hydrogen peroxide (H2O2), melatonin, chitosan, silicon, ascorbic acid (AsA), tocopherols, and trehalose (Tre) as potential primers that enhance the salinity tolerance of crop plants.
The improvement of salt tolerance of agricultural crops is indispensable to achieving future food security challenges in different regions around the world (Chourasia et al., 2022; Mangal et al., 2022). Several strategies for improving crop salt tolerance are currently in practice worldwide, including classical breeding and selection, wide crossing, transgenics, and genome editing.
Engineering crop salt tolerance has gained considerable attention during the last few decades (Farhat et al., 2019; Wani et al., 2020), but limited success has been achieved through this approach due to complex interactions and cost-intensive methodologies used (Munns and Tester, 2008; Kotula et al., 2020). Meanwhile, various alternative approaches have been employed for enhancing crop tolerance to salt stress (Zulfiqar, 2021). One of them is seeds/seedling priming. Priming, known as hardening or sensitization, could also be induced naturally or by exposure to a specific abiotic stress (Savvides et al., 2016; Liu et al., 2022a). Such priming acts as a signal, specifying an enhanced possibility of enduring a particular stress response (Filippou et al., 2013; Ibrahim, 2016; Liu et al., 2022a). After the identification of this particular stress, plants prepare themselves to respond quicker and better to their exposure to environmental stress(es) – a phenomenon called the primed state (Johnson and Puthur, 2021). The priming technique triggers the primed state in plants, which is similar to the natural priming occurring after exposure of a seed or a seedling to a specific priming agent that may be a synthetic chemical or a natural compound. As priming is believed to improve the performance of various crops under salt stress (El-Beltagi et al., 2022; Xie et al., 2022), this strategy can be a vital alternative when limited success is expected through genetic engineering.
The use of molecules with signaling properties, such as H2S, NO, and H2O2 or natural compounds, such as chitosan, melatonin, ascorbic acid (AsA), alpha-tocopheol, trehalose (Tre), and polyamines, and plant extracts as priming agents has been demonstrated to enhance salt tolerance in different crop plants.
This review aimed to briefly summarize key characteristics of salt stress in crop plants, introduce chemical priming agents commonly used in inducing a primed state, and present likely physiological and biochemical mechanisms involved in the primer–plant interactions. The practical use of priming agents for improving salt tolerance of different crop plants and future perspectives for exploring their potential for enhancing crop salt tolerance were also discussed.
Soil salinization is considered one of the most important environmental and agricultural issues. Globally, almost 831 million hectares of land were affected by salinization (Butcher et al., 2016). Excessive salt, mainly sodium (Na), can deteriorate soil quality and adversely impact crop productivity worldwide (Yu et al., 2020). Salt stress on crops can be further aggravated by industrial pollution and poor irrigation practices combined with the increasing human population (Hayat et al., 2020). On the other hand, the agricultural sector is under significant pressure to produce more food from saline soils. Exploiting saline–alkali lands for agriculture could provide an opportunity to fulfill the food security challenges (Yang and Guo, 2018).
Salt stress affects crop yields to varying degrees, depending on salt concentrations, crop species, stage of crop development at which the stress occurs, and stress duration (Munns et al., 2020). Saline-alkali land has high Na+ levels, resulting in hypertonic and hyperosmotic conditions that impede water and nutrient uptake by plants. Crops under a saline environment accumulate excessive ions in vacuole to avoid accumulation in the cytoplasm that can impair enzymatic reactions and block vital physiological and biochemical processes. However, excessive amounts of Na+ are lethal for plant growth. Osmotic stress is the first response of plants when salinity levels disturb the water balance. The next phase, ionic stress, increases Na+ ion uptake, disturbing K+ uptake and nutrition imbalance. Salt stress disturbs leaf water balance and ion compartmentalization (Arif et al., 2020). Osmotic and ionic stress conditions lead to oxidative stress through uncontrolled generation of reactive oxygen species (ROS), including singlet oxygen (1O2), hydroxyl radical (⋅OH), H2O2, and superoxide ion (O2⋅–) (Hasanuzzaman et al., 2020). Under the normal growing environment, ROS are the byproduct of several metabolic pathways that involve subcellular compartments, including chloroplasts, cytosol, mitochondria, plasma membrane, and peroxisomes (Corpas et al., 2015, 2020; Hu et al., 2020). The abundant production of ROS triggers oxidative stress, damaging crucial macromolecules (proteins, lipids, DNA, and carbohydrates), plant cellular membranes, cellular redox status, and antioxidant systems, ultimately causing cell death (Gill and Tuteja, 2010). Crops try to protect the cell from oxidative stress by activating scavenger enzymatic and non-enzymatic systems. The excessive production of ROS also disturbs the electron transport system, photosystem I (PSI), PSII system reaction centers in the thylakoid membrane, and membrane structure (Li et al., 2017). Membrane lipid oxidation has deleterious effects by inducing the formation of free radicals by “chain reaction” (Demidchik, 2015). An increase in markers related to oxidative stress, such as protein oxidation, lipid peroxidation, and 8-oxoguanine, reflects excess ROS formation that disturbs the normal functioning of cellular structures under salt stress (Møller et al., 2007; Manai et al., 2014a; Shan and Liu, 2017). Increased ROS due to salt stress also causes various metabolic alterations in protein development, membrane fluidity, blockage of electron transfers, and PSII (Biswal et al., 2011; Silva et al., 2011; Jajoo, 2013). Lipid peroxidation (LP) involves the initiation, propagation, and termination of lipids (Farmer and Mueller, 2013). During the lipid peroxidation initiation stage, one hydrogen (H) atom is excluded from the lipid molecule, resulting in a lipid radical that interacts with O2 to form a lipid peroxyl radical (Demidchik, 2015; Soares et al., 2019). During the propagation stage, the lipid peroxyl radical disturbs adjacent fatty acids, transferring H+ to form a lipid radical and lipid hydroperoxide (LOOH) (Yadav and Ramana, 2013) that damage the membranes and cause organelle degeneration and dysfunction. Malondialdehyde (MDA) is the terminal product of LP under stress (Demidchik, 2015), leading to the oxidation of histidine and lysine (Demidchik, 2015). Hence, an increased MDA in plants reflects oxidative stress (Soares et al., 2019). For instance, ElSayed et al. (2022b) reported increased MDA in zucchini in response to excess salt treatment. Numerous studies have shown that salt stress triggers LP (Shan and Liu, 2017; Shams et al., 2019).
Plant priming is a state of plant sensitization to tolerate upcoming stress conditions (Borges et al., 2014). In a primed state, plants become ready and induced to endure the prevailing stress condition (Molassiotis et al., 2016; Marthandan et al., 2020). Various research reports have demonstrated the positive impacts and the increases in salt-stress tolerance of primed plants (Taïbi et al., 2021; El-Beltagi et al., 2022; Xie et al., 2022). Different chemicals (synthetic and natural) caused a priming state in plants, which then showed enhanced growth and increased yield under saline stress (Gohari et al., 2020a,b; Liu et al., 2022b). Figure 1 displays a simple model of plant priming agents induced plant salt tolerance. Commonly used priming agents include (1) phytohormones including brassinosteroids (BRs), ethylene, melatonin, methyl jasmonate (MeJA), salicylic acid (SA), nitric oxide (NO), and strigolactones (SLs); (2) reactive species, such as hydrogen peroxide (H2O2), hydrogen sulfide (H2S), and molecular hydrogen (H2); (3) osmoprotectants including glycine betaine, polyamines, proline (Pro), and Tre; (4) vitamins, such as alpha-tocopherol, AsA, and thiamine; and (5) mineral elements including silicon and various nanoparticles (NPs), and chitosan polymer. Biochemical, physiological, and cellular responses as well as growth and productivity improvement of different crops induced by the priming agents are presented in Table 1.
Figure 1. Priming induced cellular mechanisms for mediating salt-stress tolerance and yield improvement. Chemical priming (on right) can remediate salt-induced negative impacts on plants by altering metabolic processes (middle) and thus enhancing salt tolerance and yield (on left).
Table 1. Representative examples of beneficial effects of different compounds used for priming to improve salt tolerance in different plant species.
BRs, a group of plant steroids and polyhydroxylated hormones play a vital role in the growth and developmental activities of plants (Gruszka, 2013; Kong et al., 2021). Accumulating evidence shows that the pretreatment with BRs triggered salinity stress resistance in different plant species. For example, Su et al. (2020) reported that the BR application on Malus hupehensis under salt stress eliminated ROS production and improved superoxide dismutase (SOD, E.C. 1.15.1.1) and catalase (CAT, E.C. 1.11.1.6), Pro, soluble sugars, and K+ contents. Further, the Na+ uptake decreased via regulating MhNHXs [Na+ (K+)/H+ antiporter genes]. Yue et al. (2020) compared the seed priming and seedling priming with 24-epibrassinolide on black locusts (Robinia pseudoacacia L.) under salt stress. The authors observed that primed seedlings performed comparatively better on mediating salt tolerance by improving chlorophyll content, leaf gas exchange, and membrane stability while decreasing leaf Na+ level and oxidative stress markers, such as H2O2 and MDA.
The current understanding of BRs mediated stress responses is largely based on the Arabidopsis BR signaling model. The BRI1 (BRASSINOSTEROID INSENSITIVE 1) and BAK1 (BRI1-ASSOCIATED RECEPTOR KINASE 1) as the BRI-BAK1 receptor complex located on the plasma membrane perceives BRs, and the signaling is sequentially transduced by BSK1 (BR-SIGNALING KINASE 1), BSU (BR-SUPPRESOR 1) phosphatase, and BIN2 (BRASSINOSTEROID-INSENSITIVE 2 kinase), which result in the activation of BZR1 and BES1 (BRASSINAZOLE-RESISTANT 1 and BRI1-ETHYLMETHYLSULFONE-SUPPRESSOR 1), two key transcriptional factors of BR signaling (Kim and Wang, 2010). Each of these components is a family of protein members and participates in the regulation of stress tolerance (Ahammed et al., 2020). For example, BAK1 belongs to SERK (SOMATIC EMBRYOGENESIS RECEIPTOR-LIKE kinase) family proteins. The SERK2 can interact with OsBRI1 in rice, and overexpression of SERK2 substantially increased salt tolerance and grain size (Dong et al., 2020). Additionally, exogenous supplementation of BR can induce the expression of RESPIRATORY BURST OXIDASE HOMOLOG 1 (RBOH1) encoding NADPH oxidase which causes ROS generation in the apoplast. Further BR-induced ROS signals moderate redox homeostasis, triggering activation of transcription factors (TFs) that manage transcription of BR-regulated and stress-responsive genes. It ultimately leads to improved resistance to abiotic stresses via an increase in protective protein controls.
Ethylene is a gaseous plant hormone that has also a crucial role in plants’ adaptation under stress conditions. It particularly regulates the photosynthesis process under salinity stress (Fatma et al., 2021; Rasheed et al., 2021). Borbély et al. (2020) evaluated the response of exogenous ethylene on salt-stressed tomato plants, observed a reduction in salt-induced osmotic effects, and regulated carbon assimilation and photosynthetic traits and non-photochemical quenching (NPQ) through regulating starch metabolism and PSI cyclic electron flow in tomatoes. Jahan et al. (2021) evaluated the combined application of ethylene together with sulfur and nitrogen on salt-stressed mustard (Brassica juncea) and observed an improved salt-stress tolerance through the regulation of pro-metabolism and antioxidants. Fatma et al. (2021) inspected the combined effect of sulfur and ethylene on salt-stressed mustard, observed the application improved ABA and the development of chloroplast thylakoids and photosynthetic performance, reduced oxidative stress via non-enzymatic and enzymatic components of ascorbate–glutathione (AsA–GSH) cycle, and decreased H2O2 and Na ions uptake.
Melatonin is a biological indoleamine molecule regulating numerous vital processes in plants (Altaf et al., 2021, 2022; Arnao and Hernández-Ruiz, 2021; Zhao et al., 2022). Different studies have showed that the pretreatment with melatonin positively affects plants under salinity. ElSayed et al. (2020) described that pretreatment of peanut (Arachis hypogaea) with melatonin improved salt stress via reduction of ROS. Further, pretreatment to salt-stressed A. hypogaea upregulated melatonin biosynthesis-associated genes, such as ASMT1, ASMT2, ASMT3, and TDC. Furthermore, tryptamine 5-hydroxylase (T5H) and redox homeostasis were also influenced by the ability of melatonin to induce alterations in antioxidant systems, such as increases in the expressions of APX, CAT, SOD, GR, and DHAR encoding genes. The application of melatonin on roots of watermelon seedlings grown under salinity stress, improved growth, increased photosynthesis, and decreased oxidative stress by improving the redox state coupled with enhanced antioxidant activities (Li et al., 2017). Gao et al. (2019) reported that the improved growth following melatonin application was linked with oxidative stress reduction and upregulation of genes encoding chlorophyll synthase (ChlG), lipid peroxidase, lipoxygenase(LOX), and peroxygenase. Altaf et al. (2021) reported that melatonin application on salt-stressed tomato (Solanum lycopersicum L.) plants ameliorated the negative impact by changing root system architecture and by increasing photosynthetic pigments and assimilations. The authors further explained that this was caused by the obstruction of Na uptake from soils and transport to the aerial parts of the plant and the reduction of the oxidative stress justified by the decrease in O2⋅–, H2O2, electrolytes leakage, and MDA. Moreover, melatonin is also associated with boosting antioxidant enzymes and compounds, such as ascorbate peroxidase (APX E.C. 1.11.1.11), SOD, CAT, AsA, glutathione reductase (GR E.C. 1.8.1.10), and GSH, improving cellular membrane stability. While working with salt-stressed strawberry (Fragaria × ananassa Duch., cv. “Camarosa”) Zahedi et al. (2020) observed that melatonin application not only revived growth but also improved fruit yield and quality. Liu et al. (2022b) examined the impact of melatonin on salt-stressed sugar beet (Beta vulgaris L.) and observed that the application of melatonin improved salt tolerance by activation CAT, SOD, peroxidase (POD, E.C. 1.11.1.7) and reduction of H2O2 and MDA production. In purple-leaved basil (Ocimum basilicum L.), seed priming with melatonin was able to reduce the negative effect of salinity as demonstrated by the metabolites and antioxidant compounds changes (Bahcesular et al., 2020).
Zhang et al. (2020) examined the effect of melatonin on the salt-stress tolerance mechanisms of cucumber. The authors observed that melatonin application improved photosynthesis, cell viability, and antioxidant enzyme activity and reduced MDA content, relative conductivity, and active oxygen explosion in cucumber seedlings. Gene expression analysis in the same study showed that melatonin application in salt-stressed cucumber enhanced the expression of nicotinamide adenine dinucleotide phosphate (NADPH) oxidase genes, antioxidant enzyme gene, salt overly sensitive (SOS) genes, and mitogen-activated protein kinase (MAPK) genes. SOS are genes that regulate the Na+ transporters through the membrane and can regulate the Na+ concentration in the cytoplasm. The activation of SOS genes is mediated by intracellular calcium concentration.
All these reports show that melatonin has a positive outcome for plant priming by regulating the accumulation of antioxidants and endogenous melatonin during salt stress. The molecular mechanism of melatonin-induced alleviation of salt stress still needs to be explored, and the genes related to antioxidants and ions transporters regulated by melatonin need to be determined in more crops.
MeJA is a gas volatile organic compound and plant growth regulator and plays an integral role in the regulation of tolerance mechanisms against environmental stresses. The application of MeJA has been observed to enhance salt-stress resistance in different crop plants. For instance, Ahmadi et al. (2018) evaluated the effect of exogenous MeJA on B. Napus and reported mitigation of salt stress via increasing water content, photosynthesis rate, and soluble sugar content. In salt-stressed sea fennel (Crithmum maritimum L.), Labiad et al. (2021) observed that MeJA improved salt-stress responses by maintaining antioxidant and flavonoid production. While working with salt-stressed sweet basil, Talebi et al. (2018) reported an increase in oil quality and antioxidants that lead to relief from salt stress in sweet basil. In response to MeJA treatment, Gao et al. (2021) observed an improvement in salt-stress tolerance in Nitraria tangutorum Bobrov by increasing the accumulation of osmolytes, antioxidant activity, and antioxidant content and lowering Na+/K+ ratios in shoots and higher Na+ efflux rates in roots of plants. Furthermore, MeJA augmented endogenous ABA and JA, and the transcript levels of their biosynthesis and responsiveness genes (Gao et al., 2021). In salt-stressed Citrus sinensis (L.) Osbeck, the application of MeJA promoted growth, photosynthetic pigment, APX1, CAT, CSD, GSTs, POD, PAL, Na+ co-transporters, and aquaporin proteins (Mahmoud et al., 2021). Lang et al. (2020) reported that MeJA mediates salt-stress tolerance in Glycyrrhiza uralensis Fisch. Ex DC. via the regulation of antioxidative defense, and carbon and nitrogen metabolism. Moreover, MeJA is also reported to provide salt-stress tolerance in strawberry via altering the expression of stress-associated genes (Moradi et al., 2022).
NO is known for its vital role in plant cellular signaling (Sun et al., 2021). It participates in various processes of plant growth under both normal and unfavorable conditions (Corpas and Barroso, 2015; Asgher et al., 2017; Begara-Morales et al., 2018; Kolbert et al., 2019). Various studies have demonstrated the central mediating effect of NO signaling in inducing salinity tolerance (Molassiotis et al., 2010; Manai et al., 2014b). For instance, Gao et al. (2022) studied the impact of NO in mitigating the ill effects of salinity stress on N. tangutorum. They observed that NO relieved salt stress via decreasing leaf senescence and root damage while enhancing endogenous NO, activating the ascorbate–glutathione (AsA–GSH) cycle, and increasing antioxidant enzymes’ activities and the expression of their associated genes, ultimately relieving salt-stress-induced oxidative damage. Moreover, the exogenous NO promoted ion transporter and Na+ efflux gene expression and reduced the Na+/K+ ratio (Gao et al., 2022). Treatment of spinach (Spinacia oleracea L.) and tomato (S. lycopersicum L.) with NO triggered salt-stress tolerance via increasing the biosynthesis of total phenolic content, ascorbate, GSH, flavonoids, and Pro (Hayat et al., 2012; Du et al., 2015). A proteomic study performed by Shen et al. (2018) revealed that under salt stress, NO regulates the accumulation of photosynthesis-associated proteins. They observed that in salt-stressed mangrove plant Avicennia marina (Forssk.) Vierh., the abundance of rubisco large subunit (RBCL), ribulose-phosphate 3-epimerase, quinine oxidoreductase-like protein isoform 1 (QOR1) and rubisco activase A decreased, while the abundance of other proteins, such as RBCL and QOR1 increased by NO application (Shen et al., 2018). Additionally, exogenous NO treatment enhanced salt tolerance via enhancing the accumulation of protein related to primary metabolism, RNA transcription, energy metabolism, and stress response proteins (Shen et al., 2018).
In pepper (Capsicum annuum L. cv. Alova), NO application ameliorated salt stress via the regulation of leaf gas exchange traits, mineral uptake, and osmotic potential. Furthermore, the application of NO decreased MDA and H2O2 resulting in better growth and reduced salt-induced oxidative stress (Shams et al., 2019). Ahanger et al. (2020) demonstrated that exogenously applied NO and salicylic acid (SA) prompted salt-stress tolerance in Vigna angularis (Willd.) via increasing Pro, glycine betaine, and sugars, while upregulated enzymatic and non-antioxidant production, and content of N, P, and Ca. With respect to respiratory terminal oxidase which is responsible for salt-stress tolerance via catalyzing cyanide-resistant respiration, Jian et al. (2016) revealed that exogenous NO boosts the expression of alternative oxidase (AOX) genes and the cyanide-resistant respiration rate, which are induced by salinity stress, to relieve the salt-stress-induced photosynthetic and oxidative damage in plants. Huang et al. (2020) showed that exogenous NO treatment controls the expression of NH4+ transporters in order to mediate NH4+ transport, which may imitate N uptake and sugar transport rate and its subsequent utilization for mitigation of salt-induced oxidative stress. It is considered that programmed cell death (PCD) under salt-stress maintains cellular homeostasis and Sami et al. (2021) observed a decrease in PCD in salt-stressed mustard plants following NO supply. In addition, NO signaling with small active substances, such as polyamines, calcium ions, sulfur, and hormones is associated with increased salt-stress resistance in plants. For instance, Tailor et al. (2019) observed that NO upregulates polyamines biosynthetic enzymes, including S-adenosylmethionine decarboxylase and arginine decarboxylase, while it lowered the activity of polyamine oxidase to prevent its degradation, hence improving salt-stress tolerance in sunflower. Regarding NO and melatonin signaling, Zhao et al. (2021) showed that the NO and melatonin combined application promotes salt-stress tolerance via ion homeostasis, re-established redox and mitigation of ROS as well as modulating the salt overly sensitive 2 (SOS2), antioxidant defense genes, and sodium hydrogen exchanger (NHX1) transcripts. Sulfur (S) and NO are observed to work synergistically against salt stress. For instance, in salt-stressed mustard applications of NO and S decreased the Na+ and Cl– uptake via regulating Na+ transporter and H+ pump function in both roots and leaves. Moreover, the application of NO and S to salt-stressed mustard plants encouraged the activity of ATP-sulfurylase (ATPS, EC 2.7.7.4), CAT, APX, and GR, hence reducing salt-stress-induced oxidative stress (Fatma et al., 2016). A link between NO and phytohormones regarding salt-stress tolerance in plants has been shown; for example, while observing the integrative role of auxins and NO in salt-stressed Arabidopsis, NO function was found to be upstream of auxins (Liu et al., 2015). The authors also observed that NO dropped auxin levels via suppressing the expression of the auxin efflux transporter gene PINFORMED (PIN) and decreasing auxin signal transduction through stabilizing the Aux/IAA suppressor protein IAA17, thereby resulting in restricted root meristem growth. Regarding GA and NO, Chen et al. (2022) reported that NO negatively regulates GA signaling via sulfhydryl nitrosylation (S-nitrosylation) of DELLA proteins to synchronize the plant growth under salt stress. Kong et al. (2016) noted that the foliar application of NO to salt-stressed cotton increased the expression of cytokinin biosynthesis-related genes including IPA, ZR, and IPT1, suggesting the vital role of NO in promoting CK biosynthesis, ultimately lowering salt-stress-induced leaf senescence. Ahmad et al. (2018) observed that NO and jasmonic acid upregulated osmolyte synthesis, antioxidant metabolism, and metabolite accumulation in salt-stressed tomato.
The above-mentioned studies implied that plant priming with NO showed better salt tolerance in crop plants by regulating the anti-oxidative defense system, nutrient uptake, photosynthesis and stress-related proteins, and osmolyte accumulation. However, there is still a need to evaluate NO induced salt tolerance mechanisms, especially anti-oxidative defense responses at the molecular level.
SA is a well-known phenol-based plant hormone regulating various vital endogenous processes and signals in plants. SA has been shown to induce different levels of resistance against biotic and abiotic stresses (Ding and Ding, 2020; Kaya et al., 2020; Sharma et al., 2020). The recent pieces of evidence have revealed that plant priming with SA improved plant salt-stress tolerance. According to Bukhat et al. (2020), salt stress was significantly mitigated in radish (Raphanus sativus L.) after the SA application. The SA-treated radish showed improved photochemistry and antioxidant activity while lowering membrane damage and ROS generation. The foliar application of 0.5-mM SA improved the growth, essential oil, and chlorophyll contents of Salvia officinalis L. Meanwhile, SA decreased Na content and increased Ca, K, and P contents in the leaves and roots of S. officinalis (Es-sbihi et al., 2021). In cucumber seedlings, the application of SA improved the relative growth rate, root growth traits, and leaf photosynthetic traits under salt-stress conditions. Furthermore, SA application downregulated GL2 and RHD2 and upregulated the RHD2, NAC1, NAC2, GL2, and EXP in salt-stressed seedlings (Miao et al., 2020). Ma et al. (2017) tested the influence of SA plant priming and reported enhanced salt-stress tolerance of Dianthus superbus L. through regulating photosynthesis and antioxidant systems. SA-priming is a strategy for increasing major GSH-based H2O2-metabolizing enzymes, such as glutathione-S-transferase (GST E.C., 2.5.1.18) (Khan et al., 2015). SA improved salt tolerance of S. lycopersicum through the expression of GST-gene family members including SlGSTT2, SlGSTT3, and SlGSTF4 (Csiszár et al., 2014). Jayakannan et al. (2015) proposed that the SA receptor, a non-expresser of PR protein 1 (NPR1) could be a leading regulatory protein implicated in SA-based defense responses in plants. NPR1 may enhance root H+-ATPase activity, control Na+ entry into roots and subsequently transport to shoots, prevent K leakage resulting from Na stress, and increase K content in plant shoots. Our understanding of SA-mediated tolerance to salt stress is not conclusive. SA as a signal molecule may induce a large number of gene expressions in salt-stressed plants, including NPR1, K transporters, and genes related to the ROS scavenging pathways (Saleem et al., 2021). Subsequently, the activities of enzymatic antioxidants such as APX and CAT increased; and ROS and MDA are reduced, resulting in the protection of cell membrane, sustaining plant photosynthesis, and improving plant growth.
SLs, being a new class of phytohormones, play vital roles in plant growth and productivity under normal and harsh conditions. Their role in enhancing plant salt-stress tolerance is quite promising. For instance, Zhang et al. (2022a) evaluated the exogenous applications of SLs on salt-stressed cucumber seedlings. The authors observed that the application of SL alleviated salt-stress damage by regulating photosynthesis, enhancing the efficiency of ascorbate–glutathione (AsA–GSH) cycle, and scavenging excessive ROS. Strigolactones boosted antioxidant activity and maintained osmotic and ionic balance in salt-stressed cucumber plants. Strigolactones raised the gene expression levels of nicotinamide adenine dinucleotide phosphate (NADPH) oxidase, antioxidant enzymes, calcium-dependent protein kinases (CDPKs), CBL-interacting protein kinase 2 (CIPK2), SOS1, and calcineurin B-like protein 3 (CBL3) (Zhang et al., 2022b).
Hydrogen peroxide was used to be considered a toxic molecule, but now it has been known as an important signaling molecule with crucial roles in signal transduction pathways (Gohari et al., 2020a,b; Nazir et al., 2020). Several studies have shown that treatment of H2O2 can prompt plant tolerance to salt stress. For example, foliar-fed pistachio seedlings with H2O2 displayed enhanced salt tolerance, which was associated with the production of AsA. The treatment with H2O2 induced glutathione (GSH) and carotenoids and upregulated enzymatic antioxidants, such as CAT and APX (Bagheri et al., 2019). Salt tolerance of Vigna radiata (L.) R. Wilczek (mung bean) was improved by the application of H2O2 to seedlings (Shan and Liu, 2017) (Table 1). The improved salt tolerance of the primed Vigna radiata plants was attributed to attenuated electrolyte leakage (EL) and increased total ascorbate and GSH content, as well as increased APX, dehydroascorbate reductase (DHAR, E.C. E1.6.5.4), GR, and γ-glutamylcysteine synthetase (E.C. 6.3.2.2) activities in leaves. Likewise, Gohari et al. (2020a) tested the individual and combined effects of H2O2 and sodium nitroprusside (SNP, donor of NO) as priming agents on O. basilicum L. plants grown under salt stress. The authors noted that the foliar application of 2.5 mM H2O2 plus 200 μM SNP effectively improved the growth of O. basilicum under salt stress via increasing chlorophyll, carotenoid, and anthocyanin and the activities of both APX and guaiacol peroxidase. Tanou et al. (2009) noted that pre-treatments of citrus root (Citrus aurantium L.) with SNP and H2O2 under salt stress induced a strong antioxidant enzymatic defense response in leaves by increasing SOD, CAT, APX, GR, and related isoform(s) expression. Furthermore, it was found that the salt-induced protein carbonylation (an oxidative damage marker) was reversed and the ascorbate redox status was recovered in the pre-treated citrus plants.
Priming with H2O2 alone or in combination with other potential priming chemicals enabled plants to become tolerant to salt stress particularly by modulating several key components of the oxidative defense system. However, from these reports, it is not evident how far this priming agent triggers physio–biochemical processes other than those of the oxidative defense system, involved in the salt tolerance mechanism.
Hydrogen sulfide (H2S) is a highly soluble, colorless, and flammable gas (Calvert et al., 2010). In plants, H2S is a gas transmitter similar to NO, playing a crucial role in plant vital processes and in the alleviation of various biotic and abiotic stresses (Hancock and Whiteman, 2016; Corpas et al., 2019; Zulfiqar and Hancock, 2020; Cheng et al., 2022). Furthermore, H2S is considered a reactive molecule for cell signaling events in plants (Corpas et al., 2019; Hancock, 2019). It has been reported to mediate salinity tolerance via the regulation of various physiological and biochemical responses (Shan et al., 2014; Ma et al., 2019). For example, Christou et al. (2013) tested H2S as a potent priming agent for strawberry (Fragaria × ananassa cv. Camarosa) plants grown under salt stress. They noted that pretreatment of roots with sodium hydrosulfide (NaHS) (a H2S donor) increased stomatal conductance and leaf relative water content and decreased salt-induced nitrosative and oxidative damage in plants. Furthermore, gene expression analysis revealed that H2S induced various transcriptional changes related to antioxidants and the salt overly sensitive (SOS) pathway. Jiang et al. (2019) reported that H2S priming induced salt tolerance in cucumber (Cucumis sativus L.) cultivar ‘Chunxiaqiuwan’ plants was related to the improved stomatal traits, photosynthetic pigments, and enhanced endogenous H2S levels. Hydrogen sulfide priming induced β-cyanoalanine synthase (E.C. 4.4.1.9) and D/L-cysteine desulfhydrase (E.C. 4.4.1.15) activities and reduced O-acetyl-L-serine (thiol)lyase. Furthermore, under higher NaCl levels, H2S maintained homeostasis of the ions Na+ and K+ by regulating PM H+-ATPase, SKOR, and SOS1 at the transcriptional level (Jiang et al., 2019).
Overall, the current understanding of H2S-mediated plant salt tolerance is largely linked with the regulation of physiological traits, ion balance, and enhanced antioxidant activities that may prevent oxidative damage associated with salinity stress. Further studies are compulsory to enhance the understanding of the salt-stress mitigation phenomenon by H2S plant priming especially focusing on the molecular aspects under field experiments.
The application of hydrogen (H2) gas to plants in the form of hydrogen-rich water (HRW) or H2-saturated water is a new method for boosting the ability of plants against the ill effects of abiotic stresses (Zulfiqar et al., 2021). Currently, H2 has been tested on different crop plants for its ability to impart salt-stress tolerance. For instance, Yu et al. (2021) tested the impact of HRW on salt-stressed cucumber plants. The authors observed a marked improvement in the salt-stress tolerance in cucumber plants following the applications of HRW. In another study with B. napus, Zhao et al. (2021) examined the role of HRW under different stresses including salt stress. The authors observed that the applications of HRW on salt-stressed brassica could ameliorate the negative impact of salt stress via regulating ion and redox balance through the involvement of NO. The hydrogen-rich water applications decreased the Na/K ratio in rapeseed seedlings grown under salt stress (Zhao et al., 2021).
Studies are limited related to the HRW applications on crop plants, and hence further research particularly focusing on the molecular aspect is necessary to better understand the HRW-mediated salt-stress tolerance in plants.
Glycinebetaine (GB) is considered a vital osmoprotectant in plants. Glycinebetaine is involved in the mitigation of adverse effects associated with abiotic stresses including salt stress in plants. Exogenous supplementation of GB is reported to enhance the resistance to salt stress in plants. Yildirim et al. (2015) evaluated the role of exogenous GB on lettuce and reported that the GB applications reduced membrane permeability, MDA, H2O2, and Na accumulation while raising the concentrations of indole acetic acid (IAA), SA, and GA; hence, imparting salt-stress tolerance to lettuce plants. In salt-stressed common beans, the application of GB reduced Na uptake and boosted antioxidant activities resulting in an improved salt-stress tolerance (Sofy et al., 2020). Similarly, in onions grown under salt stress, the application of GB resumed the restricted growth, water use efficiency, photosynthetic pigments, leaf gas exchange, and membrane stability index (Rady et al., 2018).
Polyamines (Pas) play a vital role in lessening the ill effects of abiotic stresses including salt stress. An increase of polyamines in plants on exposure to stress conditions is perceived to be beneficial (Antoniou et al., 2021). Priming using Pas is one of the desirable techniques to improve salt tolerance. Seeds of salt-sensitive and non-sensitive rice cultivars were primed with spermine (SPM) and spermidine (SPD) and seedling growth and transcriptome profiling were analyzed by Paul and Roychoudhury (2017). The results showed that seedlings germinated from SPM and SPD primed seeds showed increased expression of antioxidant and osmolyte biosynthetic genes as well as ABA biosynthesis gene and ABA-inducible transcription factor in roots and shoots compared to non-primed seedlings. Additionally, photosynthetic efficiency was also increased due to the priming with SPM and SPD. Taïbi et al. (2021) reported an altered content of polyamines in Phaseolus vulgaris L. under salt stress, and the tolerant cultivar accumulated a higher content of spermidine while both cultivars showed different gene expression levels encoding enzymes associated with spermine biosynthesis. Antoniou et al. (2021) also noted that salt stress resulted in an increase in spermine in a salt-tolerant cultivar of Medicago truncatulato Gaertn. compared the sensitive cultivars. An altered gene expression related to biosynthesis and catabolism of polyamines was noted in M. truncatula cultivated under salt stress. This depicts that under salt-stress conditions, the exogenous application with polyamines could be a strategic action to improve the negative impact of salt stress. Xiong et al. (2018) reported that the application of putrescine (Put) to salt-stressed tea (Camellia sinensis L.) plants improved their photosynthetic activity and decreased ROS production. This was depicted by a decreasing trend in SOD, POD, and CAT activities. The treatment with Put to salt-stressed cucumber plants improved photosynthetic capacity by regulating photochemical efficiency of PSII, hence mediated the injurious effects of salinity stress (Zhang et al., 2009). In salt-stressed lemon plant, the Put application decreased salt-stress-induced rise in MDA, proposing the involvement of Put in the protection of the plasma membrane (Khorshidi and Hamedi, 2014). In guava seedlings, the Put applications decreased CAT and POX activities and improved plant growth compared to the un-stressed, control plants (Ghalati et al., 2020). The exogenous spermidine application increased salt tolerance in Kentucky bluegrass, which was related to the increased the activity of antioxidant enzymes and decrease in MDA levels in salt-treated plants (Puyang et al., 2015). Exogenously applied spermidine to salt-stressed cucumber and ginseng plants improved the salinity tolerance by improving antioxidant enzymes and osmoprotectants (Duan et al., 2008; Parvin et al., 2014). The application of spermidine to salt-stressed chrysanthemum lowered the uptake of Na+ and enhanced the photosynthetic capacity, enzymatic ROS scavenging capacity, the osmotic and ionic balance, and cells’ membrane balance (Zhang N. et al., 2016). In salt-alkali stressed tomato plants, the exogenous spermidine application decreased MDA content and O2⋅– generation rate and improved the components associated with the ascorbate-glutathione cycle (Zhang Z. et al., 2016). Sang et al. (2016) saw a boost in photosynthesis in salt-stressed cucumber following the applications of spermidine than that of non-treated salt-stressed plants. Qian et al. (2021) studied the impact of exogenous spermidine on the Gladiolus gandavensis grown under salt stress. The authors noted an improvement in photosynthetic pigments, gas exchange, antioxidant enzyme activity, and expression of antioxidants and osmoprotectants-related genes.
Proline is a natural osmoprotectant in plants having a vital role in the regulation of abiotic stresses. The application of Pro to salt-treated crop plants has been observed to benefit the plants. Wani et al. (2019) inspected the combined role of Pro and/or 24-epibrassinolide (EBL) on salt-stressed mustard plant and observed improved plant growth through increased activity of antioxidants along with the regulation of photosynthesis under salt stress. In eggplant, Shahbaz et al. (2013) evaluated the impact of the Pro application under salt stress. The authors observed an improved growth and water use efficiency in salt-stressed eggplant. In B. juncea L., the foliar application of Pro mitigated salt stress via increasing antioxidant capacity. While working with salt-stressed tomato, Kahlaoui et al. (2018) observed that the application of Pro at flowering stage improved salt-stress tolerance via enhancing biosynthesis of Pro, increasing soluble protein contents, activating glutamine synthetase, and decreasing proline oxidase. The combined application of Pro and SA on salt-stressed cucumber improved the salt tolerance by improving endogenous Pro and decreasing Na+ uptake in plants than untreated salt-stressed plants (Mugwanya et al., 2022).
Sugars, such as non-reducing disaccharide trehalose (Tre) are present in minute quantities in plants and possess vital importance in plant growth and productivity under common and stress conditions (Sarkar and Sadhukhan, 2022). It acts as an osmoprotectant under stress conditions including salt stress. Plants vary in their ability to accumulate Tre under salt-stress conditions. Hence, plant priming with Tre shows improved salt-stress tolerance. For instance, Samadi et al. (2019) tested the Tre application on strawberry. Upon treatment with Tre, the salt-stressed plants were able to maintain plant biomass, photosynthesis, and chlorophyll fluorescence traits, and reduced shoot Na+ accumulation, MDA and O2⋅–. Moreover, the Tre application triggered a boost in trehalase enzyme activity and endogenous Pro; phenolics and CAT, SOD, and GPX activities depicting the mitigating role of Tre in salt-stress persuaded oxidative stress. Feng et al. (2019) evaluated the Tre application on salt-stressed tomato plants and found that an improved salt-stress tolerance was associated with decreasing starch content and increasing soluble sugar content and abscisic acid (ABA), and genes related to their metabolism were induced, resulting in increased plant growth and biomass compared to non-treated salt-stress plants. Xie et al. (2022) observed that the application of Tre to tomato improved salt-stress tolerance by enhancing enzymatic activity related to Tre metabolic pathway. Furthermore, the Tre application counteracts the salt-stress induced oxidative stress in tomato plants via boosting antioxidant activities and related gene expression in salt-stressed tomato (Xie et al., 2022).
Tocopherols belonging to the vitamin E group are biosynthesized by plants and regulate plant abiotic stress tolerance as an antioxidant and signaling molecule (Ali et al., 2022). Naqve et al. (2021) evaluated the impact alpha-tocopherol on salt-stressed okra [Abelmoschus esculentus (L.) Moench] and observed an improvement in growth and yield via increasing the activity of antioxidants and via accumulation of Pro and GB. Moreover, the authors observed that alpha-tocopherol eased the hostile effects of salt stress by decreasing Na+ uptake, MDA, and H2O2 levels while boosting the uptake of K+ and Ca2+. El-Bassiouny and Sadak (2015) evaluated the impact of salt stress on flax (Linum usitatissimum L.) cultivars and observed a reduction in LP and activities of polyphenol oxidase, peroxidase and improved the SOD and CAT activities. In salt-stressed P. vulgaris, the exogenous application of alpha-tocopherol improved plant growth, physio–biochemical characteristics, mineral nutrients, soluble sugars and Pro, AsA, glutathione endogenous tocopherol, SOD, CAT, and guaiacol peroxidase (GPOX, E.C. 1.11.1.7) antioxidants than untreated controls (Hemida et al., 2017). In onion grown under salt stress, the application of alpha-tocopherol improved salt tolerance via upregulation of antioxidant activity (Semida et al., 2016). In faba bean, alpha-tocopherol applications improved salt-stress induced stunted growth via improved chlorophyll fluorescence, N, P, K, and Ca, yield, and leaf and stem anatomy (Semida et al., 2014).
Seed priming with AsA has been shown to increase salt-stress tolerance in plants. Alves et al. (2021) observed that tomato seeds priming with AsA exhibited improved tolerance to salt stress, and such tolerance was associated with the modification of the antioxidant defense system and ROS scavenging enzymes. In salt-stressed sweet peppers, El-Beltagi et al. (2022) examined the impact of foliar applied AsA and observed an improved growth and fruit yield via enhancing the photosynthetic pigments, polyphenoloxidase (PPO, E.C. 1.10.3.1) activity, and APX activity. Azizi et al. (2021) evaluated the impact of AsA on Calendula officinalis L. and observed that AsA can ameliorate salt stress and increase the carotenoids, phenolic, and flavonoid concentrations in the flowers. Wang et al. (2019) studied the salt-stressed okra seedlings under the influence of the foliar application of AsA and noted that it improved growth indicators, chlorophyll, carotenoids, and activities of antioxidant enzymes while decreasing EL, H2O2, and LP. In a recent study, Chen et al. (2021) evaluated the AsA-induced photosynthetic ability of tomato seedlings and observed that application of AsA lessened the photoinhibition and reduced the damaging effects on photosynthesis by promoting chlorophyll synthesis and decreasing oxidative stress to the chloroplast by controlling redox state in chloroplast and disintegrating excitation energy in the PSII antennae.
Thiamine, or vitamin B1, is recognized as an enzymatic co-factor that is known to be involved in vital metabolic pathways responsible for cell energy supply in plants. More recently, the pivotal role of thiamine in mitigating abiotic stress has been unraveled through various studies. Moreover, seed priming or the exogenous application of thiamine to plants under salt stress resulted in enhanced intracellular thiamine contents as well as an improved tolerance (Kaya et al., 2015). Thiamine application on maize plants grown under salt-stress enhanced photosynthetic pigments, reduced membrane permeability, MDA and H2O2 levels, and changed activities of some key antioxidant enzymes under a saline regime (Kaya et al., 2015). Rapala-Kozik et al. (2012) reported that upregulation of genes connected with the biosynthesis of thiamine and thiamine diphosphate (TDP)-dependent enzymes occurred in response to osmotic and salt stress in Arabidopsis. Furthermore, the TDP, along with TDP-dependent enzymes (α-ketoglutarate dehydrogenase, pyruvate dehydrogenase, and transketolase) were upregulated simultaneously. All these enzymes are part of the main metabolic pathways responsible for the extreme environmental conditions in plants. Moreover, Rahman et al. (2017) demonstrated that the thiamine biosynthesis increased as a result of its biosynthesis genes upregulation under salinity stress. In another study, Wouyou et al. (2017) reported an improvement in the accumulation of thiamine and other vitamins in Amaranthus leaves under salt stress. Tuna et al. (2013) examined that thiamine-treated maize plants showed alleviation of salinity effects by enhancing the antioxidant activities. Furthermore, thiamine application minimized salt stress induced changes in protein metabolism of plants (El-Shintinawy and El-Shourbagy, 2001).
Spraying thiamine exogenously can enhance salt-stress tolerance in maize and sunflower (Hamada and Al-Hakimi, 2009). El-Metwally and Sadak (2019) reported an improved salt tolerance in faba bean plants (Vicia faba L.) which was found to be linked with enhanced Pro, free amino acids, and total carbohydrates. Furthermore, the authors observed an enhanced leaf area index, improved yield and its attributes, and the chemical composition of the yielded seeds (El-Metwally and Sadak, 2019). The foliar application of thiamine on maize cultivars induced tolerance against the oxidative stress condition induced by salinity (Kaya et al., 2015).
Silicon (Si) is one of the most abundant elements found in the universe. It is part of the group of metalloids which include arsenic, antimony, boron, germanium, and tellurium. Various studies have depicted the impact of Si in improving plants’ ability to better withstand abiotic stresses (Sahoo et al., 2019; Zhu et al., 2019; Hoffmann et al., 2020; Tripathi et al., 2020; Dhiman et al., 2021). For instance, Alves et al. (2020) found that the application of Si on lettuce seeds improved germination rate, CAT, GR, and SOD activities, and decreased lipid peroxidation (LP) and H2O2 content under salt stress. Guo et al. (2020) suggested Si application to mitigate the salt-induced reduction in seed germination and to lessen the salt-induced oxidative injury to seedlings of cucumber. In sweet basil plant, the application of Si improved salt tolerance via restoring osmotic balance and improving photosynthesis, redox homeostasis, osmolyte assimilation, and adjusting the expression of salt-stress-inducible proteins (Farouk et al., 2020). Zhu et al. (2020) studied the role of exogenous Si application in salt-treated cucumber. They reported an improved tolerance against salinity stress after Si treatments. Si application reduced oxidative stress via the regulation of Pro at different stress phases through δ 1-pyrroline-5-carboxylate synthetase (P5CS) and proline dehydrogenase (ProDH) activities and P5CS gene expression and interaction with cytokinin. Furthermore, Si application induced the expression of several cytokinin dehydrogenases (Csa1G589070 and Csa4G647490) and isopentenyl transferase (Csa3G150100 and Csa7G392940) genes which could be important for the regulation of cytokinin under salt stress (Zhu et al., 2020). Abdelaal et al. (2020) demonstrated that Si application improved plant water status, photosynthesis, and production of antioxidants that not only reduced oxidative stress but also improved fruit yield of Capsicum annuum. In salt-stressed tuberose (Polianthes tuberosa) plants, supplementation with Si enhanced growth and yield and induced the antioxidant defense system, while increasing postharvest vase life (Shahzad et al., 2021). In general, seeds priming with Si activates the antioxidant machinery, improves water status through accumulation of osmoprotectants, maintains the balance of nutrient elements, and enhances photosynthetic pigments and plant growth under salt stress (Abdel Latef and Tran, 2016).
From all these studies, seed priming with Si is a practical strategy for improving plant salt tolerance. As the second most abundant element on the earth, its application would not cause environmental problems. However, molecular aspects need to be explored for an improved understanding of mechanisms related to Si-mediated salt tolerance.
The field of nanotechnology has shown great potential for handling salt stress in crop plants. Khan et al. (2020) reported the mediating effect of priming with silver nanoparticles (AgNPs; 20 mM) in salt-stressed pearl millet. The application of carbon NPs to lettuce promoted seedling tolerance to 150-mM NaCl stress (Baz et al., 2020). Primed plants showed higher production of antioxidants, Pro, Na+/K+ and relative water content and minimum oxidative damage. Pérez-Labrada et al. (2019) tested copper (Cu) NPs based priming on tomato plants grown under salinity and reported improved performance via lowering Na+/K+ ratio, increased phenol, vitamin C, and GSH production, and increased phenylalanine ammonia lyase (PAL), APX, GPX, SOD, and CAT activities. Hojjat and Kamyab (2017) evaluated the potential of Ag NPs toward seed germination of fenugreek under salt stress. They observed that seeds treated with Ag NPs showed enhanced germination under salt-stressed conditions. In ajowan [Trachyspermum ammi (L.) Sprague ex Turrill], the foliar applications of nano-Fe2O3 (3 mM) and SA (1 mM) ameliorated the negative impacts of salinity by improving K+/Na+ ratio, endogenous SA production, and Fe content and by increasing SOD, CAT, POX, and osmolytes (Abdoli et al., 2020). Moradbeygi et al. (2020) observed that Dracocephalum moldavica L. plant supplemented with iron oxide (Fe2O3) based NPs showed an improved salt-stress status via improving enzymatic and non-enzymatic activities in roots and shoots and by decreasing ROS accumulation. Gohari et al. (2020a) reported salt-stress tolerance in D. moldavica L: seedlings after titanium dioxide NPs. The combined application of Pro and halloysite nanotube (Hal) mitigated the oxidative stress in sweet basil plants and improved their agronomic performance via enhancing their physiological potential and activities of antioxidants such as APX, SOD, and GP (Masoudniaragh et al., 2021). Cotton seeds primed with poly(acrylic acid)-coated cerium oxide nanoparticles (PNC) produced seedlings with decreased ROS accumulation and increased root length and fresh weight under salt stress (An et al., 2020). Transcriptome analysis showed that both ROS and Ca2+ mediated signaling could be implicated in nanoparticle-mediated salt tolerance as ROS enzymatic pathways (POD, GST, and PRC) as well as calcium transporter CAX1, and calcium binding EF-hand family genes were regulated. Additionally, Mg content in roots of cotton seedlings increased, terpene synthase genes were upregulated.
Based on the current knowledge, plant priming with NPs can be used as potential agents for salt-stress management in crop plants. However, from the perspective of sustainability, it is better to adopt the green-based NPS as plant priming agents and evaluate their role in mediating salt-stress tolerance.
Chitosan is a poly-(D)-glucosamine that is found in crustacean shells, insects, and fungi. Behind its use in biomedical areas, chitosan has been used in agriculture with beneficial effects. Application of chitosan has been reported to boost tolerance to salt stress. Jabeen and Ahmad (2013) evaluated the impact of safflower (Carthamus tinctorius L.) and sunflower (Helianthus annuus L.) seed treatment with chitosan under salt stress and reported enhanced tolerance via reduction of antioxidant activity at lower oxidative stress conditions in both plant species. In Silybum marianum, priming with chitosan NPs improved photosynthesis pigment, antioxidant enzymes, and osmoprotectant Pro under salt stress, hence imparting salt-stress tolerance (Mosavikia et al., 2020). Sheikhalipour et al. (2021) demonstrated that foliar treatment of Chitosan-Selenium NPs at 20 mg L–1 reduced the salt-induced alterations in Bitter Melon via boosting antioxidants, osmoprotectants, K+ uptake, and relative water content, and decreasing Na+, H2O2, and MDA. In salt-stressed tomato, Attia et al. (2021) evaluated the influence of chitosan mixed with different organic acids including AsA, acetic acid, citric acid, and malic acid. They observed increases in carotenoids, antioxidants, and osmoprotectants including soluble sugars, proteins and Pro, total phenols, and AsA and decreases in H2O2, MDA and Na+, especially in response to AsA or citric acid-based chitosan application. Geng et al. (2020) evaluated the effects of chitosan on salt-stressed creeping bentgrass (Agrostis stolonifera L.). They reported improvement in relative water content, water use efficiency, photosynthesis, and photochemical efficiency. Furthermore, the decrease in salt-induced oxidative damage was mitigated by increased polyamines, antioxidants (SOD, POD, and CAT), and by altering sucrose accumulation and metabolism, total amino acids, γ-aminobutyric acid (GABA), and glutamic acid accumulation (Xu et al., 2021). Moreover, Na+ accumulation was decreased in leaves. Furthermore, chitosan application upregulated the expression of genes encoding Na+/H+ exchangers and boosted salt overly sensitive (SOS) pathways under salinity stress (Geng et al., 2020). In sweet pepper, Alkahtani et al. (2020) observed an improvement in the salt-stress induced a decline in chlorophyll a fluorescence (Fv/Fm ratio), chlorophyll concentration, relative water content, enzyme activity, Pro, and fruit yield following the application of chitosan. Further application of chitosan and rhizobacteria decreased oxidative stress as indicated by MDA, EL, O2⋅–, and H2O2. Zhang et al. (2021) evaluated the impact of chitosan applications on salt-stressed cucumber and observed an improvement in growth via an increase in K+, photosynthetic pigments, Pro, and soluble sugar contents, enhanced peroxidase and CAT activities, and alleviated membrane LP, in comparison with salt-stressed untreated plants.
All these reports show the benefits of chitosan in salt-stress tolerance of plants. The available studies depict that the phenomenon may be based on the regulation of ions intake, antioxidant defense, and salt overly sensitive pathway. However, molecular aspects need to be explored to better understand the positive phenomenon of chitosan-mediated salt-stress tolerance in crop plants.
This review highlights a total of 20 chemical priming agents enabling plant tolerance to salt stress. These agents have been categorized into groups: Plant growth regulators, reactive agents, osmoprotectants, vitamins, mineral elements, and polymers. Due to such a diversity of chemicals and also limited availability of molecular information on the action of some agents, it is difficult to speculate if there is a common molecular mechanism underlying different priming agent-mediated salt tolerance in plants. However, as discussed above, priming by different agents results in some common physiological and biochemical responses in plants and also induced a large number of gene expressions. The majority of the genes are defense-related ones, including enzymatic antioxidants, osmoprotectants, and polyamines. Such similarity may indicate that there may be a cross-talk network among the priming agent induced primed state, which requires further investigation.
It is generally agreed that salt-stress induced oxidative stress, i.e., an increase in the cellular levels of ROS which not only can adversely affect plant growth and crop yield but also cause programmed cell death. Elevated ROS can also trigger plant defense responses by upregulation of stress-protective genes and accumulation of metabolites and proteins to counteract the increased ROS and control the ROS at certain levels to sustain cell functions. The priming agents, such as plant hormones, reactive agents, and selected nanoparticles can act as a signal that modulates stress response and enhance plant ability to withstand salt stress. On the other hand, the roles of osmoprotectants include the induction of relevant gene expression to produce more osmoprotectants for reducing shoot Na+ accumulation and activation of enzymes to produce endogenous Pro, phenolics and CAT, glutathione peroxidase (GPX E.C. 1.11.1.9), and SOD to reduce salt-induced oxidative stress. As to the vitamins, they are either enzymatic co-factors or have antioxidant activities, thus, reducing salt-induced oxidative stress. Silicon improved salt tolerance by restoring osmotic balance and redox homeostasis and improving photosynthesis. Similarly, chitosan can mitigate salt stress by increasing antioxidant enzymes (SOD, POD, and CAT) and polyamines.
Seeds or plant priming is to use the priming agents to induce plants to enter the primed state before salt stress. As a result, primed plants are able to rapid activation of defense responses when challenged by salt stress, exhibiting increased tolerance to the stress. The primed state is reached molecularly by the activation of relevant TFs, epigenetic regulation, such as DNA methylation, chromatin remodeling, histone modification, and activation of transposable elements as well as post-translation regulation (Nair et al., 2022), which are outside of the scope of this review.
Salt stress generally causes a reduction in plant growth and hence it is considered a barrier to achieving food security. Genetic approaches are powerful tools that can increase the tolerance of plants to salinity; thus, new techniques for genome-editing, such as the clustered regularly interspaced short palindromic repeat (CRISPR)-associated system (Cas), are very promising (Zhang et al., 2019; Yue et al., 2020). However, this implies large investments in research time and increased costs. Furthermore, genetically modified organisms (GMOs), including plants for human consumption, are not yet socially acknowledged in many countries. Likewise, the classical genetic strategies to find cultivars of species that are more resistant to salinity are also plausible approaches. However, this also takes a long time for analysis at the field level. Therefore, the use of priming could be a complementary and useful strategy at the agronomic level. The plant priming practice is demonstrating tremendous potential to mediate abiotic stresses in a wide range of crop plants. Plant priming with different molecules as discussed above has been shown to mediate salt stress tolerance. Moreover, the application of priming techniques is quick, efficient, low cost, and time-saving compared to the conventional strategies for managing salt stress (Figure 2). Most of the priming agents are environmentally friendly to a wide range of plant species, although some of them, such as NPs need to be critically evaluated in terms of their environmental safeness on a long-term basis. The priming agents not only can be used for seed treatment but also can be used only at some critical time before stress challenges. On the whole, adopting plant priming can fulfill the desire for reducing agrochemicals and assist researchers to refine the solutions for sustainable agriculture. Research on plant priming using novel compounds must expand toward the molecular level for a better understanding of the involved mechanisms induced under salt stress. Although this review presents the main molecules used for priming, other molecules appear as prospects in priming technology. Therefore, more investigations in plant–substrate systems are needed to decipher the role of plant priming at the molecular level and guide its application for improving crop tolerance to salt stress.
FZ conceptualized and wrote the original draft. All authors reviewed and revised the manuscript.
The authors declare that the research was conducted in the absence of any commercial or financial relationships that could be construed as a potential conflict of interest.
All claims expressed in this article are solely those of the authors and do not necessarily represent those of their affiliated organizations, or those of the publisher, the editors and the reviewers. Any product that may be evaluated in this article, or claim that may be made by its manufacturer, is not guaranteed or endorsed by the publisher.
Abdel Latef, A. A., and Tran, L.-S. P. (2016). Impacts of priming with silicon on the growth and tolerance of maize plants to alkaline stress. Front. Plant Sci. 7:243. doi: 10.3389/fpls.2016.00243
Abdelaal, K. A., Mazrou, Y. S., and Hafez, Y. M. (2020). Silicon foliar application mitigates salt stress in sweet pepper plants by enhancing water status, photosynthesis, antioxidant enzyme activity and fruit yield. Plants 9:733. doi: 10.3390/plants9060733
Abdoli, S., Ghassemi-Golezani, K., and Alizadeh-Salteh, S. (2020). Responses of ajowan (Trachyspermum ammi L.) to exogenous salicylic acid and iron oxide nanoparticles under salt stress. Environ. Sci. Pollut. Res. 27, 36939–36953. doi: 10.1007/s11356-020-09453-1
Ahammed, G. J., Li, X., Liu, A., and Chen, S. (2020). Brassinosteroids in plant tolerance to abiotic stress. J. Plant Growth Regul. 39, 1451–1464. doi: 10.1007/s00344-020-10098-0
Ahanger, M. A., Aziz, U., Alsahli, A. A., Alyemeni, M. N., and Ahmad, P. (2020). Influence of exogenous salicylic acid and nitric oxide on growth, photosynthesis, and ascorbate-glutathione cycle in salt stressed Vigna angularis. Biomolecules 10:42. doi: 10.3390/biom10010042
Ahmad, P., Abass Ahanger, M., Nasser Alyemeni, M., Wijaya, L., Alam, P., and Ashraf, M. (2018). Mitigation of sodium chloride toxicity in Solanum lycopersicum L. by supplementation of jasmonic acid and nitric oxide. J. Plant Interact. 13, 64–72. doi: 10.1080/17429145.2017.1420830
Ahmadi, F. I., Karimi, K., and Struik, P. C. (2018). Effect of exogenous application of methyl jasmonate on physiological and biochemical characteristics of Brassica napus L. cv. Talaye under salinity stress. South African J. Bot. 115, 5–11. doi: 10.1016/j.sajb.2017.11.018
Al Murad, M., and Muneer, S. (2022). Silicon supplementation modulates physiochemical characteristics to balance and ameliorate salinity stress in Mung Bean. Front. Plant Sci. 13:810991. doi: 10.3389/fpls.2022.810991
Ali, E., Hussain, S., Hussain, N., Kakar, K. U., Shah, J. M., Zaidi, S. H. R., et al. (2022). Tocopherol as plant protector: An overview of Tocopherol biosynthesis enzymes and their role as antioxidant and signaling molecules. Acta Physiol. Plant 44:20. doi: 10.1007/s11738-021-03350-x
Ali, M., Afzal, S., Parveen, A., Kamran, M., Javed, M. R., Abbasi, G. H., et al. (2021). Silicon mediated improvement in the growth and ion homeostasis by decreasing Na+ uptake in maize (Zea mays L.) cultivars exposed to salinity stress. Plant Physiol. Biochem. 158, 208–218. doi: 10.1016/j.plaphy.2020.10.040
Alkahtani, M. D., Attia, K. A., Hafez, Y. M., Khan, N., Eid, A. M., Ali, M. A., et al. (2020). Chlorophyll fluorescence parameters and antioxidant defense system can display salt tolerance of salt acclimated sweet pepper plants treated with chitosan and plant growth promoting rhizobacteria. Agronomy 10:1180. doi: 10.3390/agronomy10081180
Altaf, M. A., Shahid, R., Ren, M. X., Khan, L. U., Altaf, M. M., Jahan, M. S., et al. (2021). Protective mechanisms of melatonin against vanadium phytotoxicity in tomato seedlings: Insights into nutritional status, photosynthesis, root architecture system, and antioxidant machinery. J. Plant Growth Regul. 1–17. doi: 10.1007/s00344-021-10513-0
Altaf, M. A., Shahid, R., Ren, M. X., Naz, S., Altaf, M. M., Khan, L. U., et al. (2022). Melatonin improves drought stress tolerance of tomato by modulating plant growth, root architecture, photosynthesis, and antioxidant defense system. Antioxidants 11:309. doi: 10.3390/antiox11020309
Alves, R. C., Nicolau, M. C. M., Checchio, M. V., Sousa, G. S., de Oliveira, F. A., Prado, R. M., et al. (2020). Salt stress alleviation by seed priming with silicon in lettuce seedlings: An approach based on enhancing antioxidant responses. Bragantia 79, 19–29. doi: 10.1590/1678-4499.20190360
Alves, R. D. C., Rossatto, D. R., da Silva, J. S., Checchio, M. V., de Oliveira, K. R., Oliveira, F. A., et al. (2021). Seed priming with ascorbic acid enhances salt tolerance in micro-tom tomato plants by modifying the antioxidant defense system components. Biocatal. Agric. Biotechnol. 31:101927. doi: 10.1016/j.bcab.2021.101927
An, J., Hu, P., Li, F., Wu, H., Shen, Y., White, J., et al. (2020). Emerging investigator series: Molecular mechanisms of plant salinity stress tolerance improvement by seed priming with cerium oxide nanoparticles. Environ. Sci. 7, 2214–2228. doi: 10.1039/D0EN00387E
Antoniou, C., Zarza, X., Gohari, G., Panahirad, S., Filippou, P., Tiburcio, A. F., et al. (2021). Involvement of polyamine metabolism in the response of Medicago truncatula genotypes to salt stress. Plants 10:269. doi: 10.3390/plants10020269
Arif, Y., Singh, P., Siddiqui, H., Bajguz, A., and Hayat, S. (2020). Salinity induced physiological and biochemical changes in plants: An omic approach towards salt stress tolerance. Plant Physiol. Biochem. 156, 64–77. doi: 10.1016/j.plaphy.2020.08.042
Arnao, M. B., and Hernández-Ruiz, J. (2021). Melatonin as a regulatory hub of plant hormone levels and action in stress situations. Plant Biol. 23, 7–19. doi: 10.1111/plb.13202
Asgher, M., Per, T. S., Masood, A., Fatma, M., Freschi, L., Corpas, F. J., et al. (2017). Nitric oxide signaling and its crosstalk with other plant growth regulators in plant responses to abiotic stress. Environ. Sci. Pollut. Res. Int. 24, 2273–2285. doi: 10.1007/s11356-016-7947-8
Attia, M. S., Osman, M. S., Mohamed, A. S., Mahgoub, H. A., Garada, M. O., Abdelmouty, E. S., et al. (2021). Impact of foliar application of chitosan dissolved in different organic acids on isozymes, protein patterns and physio-biochemical characteristics of tomato grown under salinity stress. Plants 10:388. doi: 10.3390/plants10020388
Azizi, F., Farsaraei, S., and Moghaddam, M. (2021). Application of exogenous ascorbic acid modifies growth and pigment content of Calendula officinalis L. flower heads of plants exposed to NaCl stress. J. Soil Sci. Plant Nutr. 21, 2803–2814. doi: 10.1007/s42729-021-00567-0
Bagheri, M., Gholami, M., and Baninasab, B. (2019). Hydrogen peroxide-induced salt tolerance in relation to antioxidant systems in pistachio seedlings. Sci. Hortic. 243, 207–213. doi: 10.1016/j.scienta.2018.08.026
Bahcesular, B., Yildirim, E. D., Karaçocuk, M., Kulak, M., and Karaman, S. (2020). Seed priming with melatonin effects on growth, essential oil compounds and antioxidant activity of basil (Ocimum basilicum L.) under salinity stress. Ind. Crops Prod. 146:112165. doi: 10.1016/j.indcrop.2020.112165
Baniasadi, F., Saffari, V. R., and Moud, A. A. M. (2018). Physiological and growth responses of Calendula officinalis L. plants to the interaction effects of polyamines and salt stress. Sci. Hort. 234, 312–317. doi: 10.1016/j.scienta.2018.02.069
Baz, H., Creech, M., Chen, J., Gong, H., Bradford, K., and Huo, H. (2020). Water-soluble carbon nanoparticles improve seed germination and post-germination growth of lettuce under salinity stress. Agronomy 10:1192. doi: 10.3390/agronomy10081192
Begara-Morales, J. C., Chaki, M., Valderrama, R., Sánchez-Calvo, B., Mata-Pérez, C., Padilla, M. N., et al. (2018). Nitric oxide buffering and conditional nitric oxide release in stress response. J. Exp. Bot. 69, 3425–3438. doi: 10.1093/jxb/ery072
Biswal, B., Joshi, P. N., Raval, M. K., and Biswal, U. C. (2011). Photosynthesis, a global sensor of environmental stress in green plants: Stress signalling and adaptation. Curr. Sci. 101, 47–56. doi: 10.1371/journal.pone.0032124
Borbély, P., Poór, P., and Tari, I. (2020). Changes in physiological and photosynthetic parameters in tomato of different ethylene status under salt stress: Effects of exogenous 1-aminocyclopropane-1-carboxylic acid treatment and the inhibition of ethylene signalling. Plant Physiol. Biochem. 156, 345–356. doi: 10.1016/j.plaphy.2020.09.019
Borges, A. A., Jiménez-Arias, D., Expósito-Rodríguez, M., Sandalio, L. M., and Pérez, J. A. (2014). Priming crops against biotic and abiotic stresses: MSB as a tool for studying mechanisms. Front. Plant Sci. 5:642. doi: 10.3389/fpls.2014.00642
Bouallègue, A., Souissi, F., Nouairi, I., Souibgui, M., Abbes, Z., and Mhadhbi, H. (2019). Physiological and biochemicals changes modulated by seeds’priming of lentil (Lens culinaris L.) under salt stress at germination stage. Acta Sci. Pol-Hortoru. 18, 27–38. doi: 10.24326/asphc.2019.5.3
Bukhat, S., Manzoor, H., Zafar, Z. U., Azeem, F., and Rasul, S. (2020). Salicylic acid induced photosynthetic adaptability of Raphanus sativus to salt stress is associated with antioxidant capacity. J. Plant Growth Regul. 39, 809–822. doi: 10.1007/s00344-019-10024-z
Butcher, K., Wick, A. F., DeSutter, T., Chatterjee, A., and Harmon, J. (2016). Soil salinity: A threat to global food security. Agron. J. 108, 2189–2200.
Calvert, J. W., Coetzee, W. A., and Lefer, D. J. (2010). Novel insights into hydrogen sulfide-mediated cytoprotection. Antioxid. Redox. Signal 12, 1203–1217. doi: 10.1089/ars.2009.2882
Chen, L., Sun, S., Song, C. P., Zhou, J. M., Li, J., and Zuo, J. (2022). Nitric oxide negatively regulates gibberellin signaling to coordinate growth and salt tolerance in Arabidopsis. J. Genetics Genom. (in press). doi: 10.1016/j.jgg.2022.02.023
Chen, X., Zhou, Y., Cong, Y., Zhu, P., Xing, J., Cui, J., et al. (2021). Ascorbic acid-induced photosynthetic adaptability of processing tomatoes to salt stress probed by fast OJIP fluorescence rise. Front. Plant Sci. 12:594400. doi: 10.3389/fpls.2021.594400
Cheng, P., Zhang, Y., Wang, J., Guan, R., Pu, H., and Shen, W. (2022). Importance of hydrogen sulfide as the molecular basis of heterosis in hybrid Brassica napus: A case study in salinity response. Environ. Exp. Bot. 193:104693. doi: 10.1016/j.envexpbot.2021.104693
Chourasia, K. N., More, S. J., Kumar, A., Kumar, D., Singh, B., Bhardwaj, V., et al. (2022). Salinity responses and tolerance mechanisms in underground vegetable crops: An integrative review. Planta 255:68. doi: 10.1007/s00425-022-03845-y
Christou, A., Manganaris, G. A., Papadopoulos, I., and Fotopoulos, V. (2013). Hydrogen sulfide induces systemic tolerance to salinity and non-ionic osmotic stress in strawberry plants through modification of reactive species biosynthesis and transcrip-tional regulation of multiple defence pathways. J. Exp. Bot. 64, 1953–1966. doi: 10.1093/jxb/ert055
Corpas, F. J., and Barroso, J. B. (2015). Functions of nitric oxide (NO) in roots during development and under adverse stress conditions. Plants 4, 240–252. doi: 10.3390/plants4020240
Corpas, F. J., González-Gordo, S., Cañas, A., and Palma, J. M. (2019). Nitric oxide and hydrogen sulfide in plants: Which comes first? J. Exp. Bot. 70, 4391–4404. doi: 10.1093/jxb/erz031
Corpas, F. J., González-Gordo, S., and Palma, J. M. (2020). Plant peroxisomes: A factory of reactive species. Front. Plant Sci. 11:853. doi: 10.3389/fpls.2020.00853
Corpas, F. J., Gupta, D., and Palma, J. (2015). “Production sites of reactive oxygen species (ROS) in organelles from plant cells,” in Reactive oxygen species and oxidative damage in plants under stress, eds D. K. Gupta, J. M. Palma, and F. J. Corpas (Cham: Springer), 1–22.
Csiszár, J., Horváth, E., Váry, Z., Gallé, Á, Bela, K., Brunner, S., et al. (2014). Glutathione transferase supergene family in tomato: Salt stress-regulated expression of representative genes from distinct GST classes in plants primed with salicylic acid. Plant Physiol. Biochem. 78, 15–26. doi: 10.1016/j.plaphy.2014.02.010
de Freitas, P. A. F., de Souza Miranda, R., Marques, E. C., Prisco, J. T., and Gomes-Filho, E. (2018). Salt tolerance induced by exogenous proline in maize is related to low oxidative damage and favorable ionic homeostasis. J. Plant Growth Regul. 37, 911–924. doi: 10.1007/s00344-018-9787-x
Demidchik, V. (2015). Mechanisms of oxidative stress in plants: From classical chemistry to cell biology. Environ. Exp. Bot. 109, 212–228. doi: 10.1016/j.envexpbot.2014.06.021
Dhiman, P., Rajora, N., Bhardwaj, S., Sudhakaran, S. S., Kumar, A., Raturi, G., et al. (2021). Fascinating role of silicon to combat salinity stress in plants: An updated overview. Plant Physiol. Biochem. 162, 110–123. doi: 10.1016/j.plaphy.2021.02.023
Ding, P., and Ding, Y. (2020). Stories of salicylic acid: A plant defense hormone. Trends Plant Sci. 25, 549–565. doi: 10.1016/j.tplants.2020.01.004
Dong, N., Yin, W., Liu, D., Zhang, X., Yu, Z., Huang, W., et al. (2020). Regulation of brassinosteroid signaling and salt resistance by SERK2 and potential utilization for crop improvement in rice. Front. Plant Sci. 11:621859. 621859 doi: 10.3389/fpls.2020
Du, S. T., Liu, Y., Zhang, P., Liu, H. J., Zhang, X. Q., and Zhang, R. R. (2015). Atmospheric application of trace amounts of nitric oxide enhances tolerance to salt stress and improves nutritional quality in spinach (Spinacia oleracea L.). Food Chem. 173, 905–911. doi: 10.1016/j.foodchem.2014.10.115
Duan, J., Li, J., Guo, S., and Kang, Y. (2008). Exogenous spermidine affects polyamine metabolism in salinity-stressed Cucumis sativus roots and enhances short-term salinity tolerance. J. Plant Physiol. 165, 1620–1635. doi: 10.1016/j.jplph.2007.11.006
El-Bassiouny, H., and Sadak, M. S. (2015). Impact of foliar application of ascorbic acid and α-tocopherol on antioxidant activity and some biochemical aspects of flax cultivars under salinity stress. Acta Biológica. Colombiana. 20, 209–222. doi: 10.15446/abc.v20n2.43868
El-Beltagi, H. S., Ahmad, I., Basit, A., Shehata, W. F., Hassan, U., Shah, S. T., et al. (2022). Ascorbic Acid Enhances Growth and Yield of Sweet Peppers (Capsicum annum) by Mitigating Salinity Stress. Gesunde Pflanzen 74, 423–433. doi: 10.1007/s10343-021-00619-6
El-Metwally, I. M., and Sadak, M. S. (2019). Physiological role of thiamine and weed control treatments on faba bean and associated weeds grown under salt affected soil. Bull. Natl. Res. Centre 43:105. doi: 10.1186/s42269-019-0142-6
ElSayed, A. I., Boulila, M., Rafudeen, M. S., Mohamed, A. H., Sengupta, S., Rady, M., et al. (2020). Melatonin regulatory mechanisms and phylogenetic analyses of melatonin biosynthesis related genes extracted from peanut under salinity stress. Plants 9:854. doi: 10.3390/plants9070854
ElSayed, A. I., Rafudeen, M. S., Ganie, S. A., Hossain, M. S., and Gomaa, A. M. (2022b). Seed priming with cypress leaf ex-tract enhances photosynthesis and antioxidative defense in zucchini seedlings under salt stress. Sci. Hortic. 293:110707. doi: 10.1016/j.scienta.2021.110707
ElSayed, A. I., Mohamed, A. H., Rafudeen, M. S., Omar, A. A., Awad, M. F., and Mansour, E. (2022a). Polyamines mitigate the destructive impacts of salinity stress by enhancing photosynthetic capacity, antioxidant defense system and upregulation of calvin cycle-related genes in rapeseed (Brassica napus L.). Saudi J. Biol. Sci. 29, 3675–3686. doi: 10.1016/j.sjbs.2022.02.053
El-Shintinawy, F., and El-Shourbagy, M. N. (2001). Alleviation of changes in protein metabolism in NaCl-stressed wheat seedlings by thiamine. Biol. Plant. 44, 541–545. doi: 10.1023/A:1013738603020
Es-sbihi, F. Z., Hazzoumi, Z., Aasfar, A., and Joutei, K. A. (2021). Improving salinity tolerance in Salvia officinalis L. by foliar application of salicylic acid. Chem. Biol. Technol. Agric. 8:25. doi: 10.1186/s40538-021-00221-y
Farhat, S., Jain, N., Singh, N., Sreevathsa, R., Dash, P. K., Rai, R., et al. (2019). CRISPR-Cas9 directed genome engineering for enhancing salt stress tolerance in rice. Semin. Cell Dev. Biol. 96, 91–99. doi: 10.1016/j.semcdb.2019.05.003
Farmer, E. E., and Mueller, M. J. (2013). ROS-mediated lipid peroxidation and RES-activated signaling. Annu. Rev. Plant Biol. 64, 429–450. doi: 10.1146/annurev-arplant-050312-120132
Farouk, S., Elhindi, K. M., and Alotaibi, M. A. (2020). Silicon supplementation mitigates salinity stress on Ocimum basilicum L. via improving water balance, ion homeostasis, and antioxidant defense system. Ecotoxicol. Environ. Saf. 206:111396. doi: 10.1016/j.ecoenv.2020.111396
Fatma, M., Iqbal, N., Gautam, H., Sehar, Z., Sofo, A., D’Ippolito, I., et al. (2021). Ethylene and sulfur coordinately modulate the antioxidant system and ABA accumulation in mustard plants under salt stress. Plants 10:180. doi: 10.3390/plants10010180
Fatma, M., Masood, A., Per, T. S., and Khan, N. A. (2016). Nitric oxide alleviates salt stress inhibited photosynthetic performance by interacting with sulfur assimilation in mustard. Front. Plant Sci. 7:521. doi: 10.3389/fpls.2016.00521
Feng, Y., Chen, X., He, Y., Kou, X., and Xue, Z. (2019). Effects of exogenous trehalose on the metabolism of sugar and abscisic acid in tomato seedlings under salt stress. Trans. Tianjin Univ. 25, 451–471. doi: 10.1007/s12209-019-00214-x
Filippou, P., Tanou, G., Molassiotis, A., and Fotopoulos, V. (2013). “Plant acclimation to environmental stress using priming agents,” in Plant acclimation to environmental stress, eds N. Tuteja and G. S. Singh (New York, NY: Springer), 1–27.
Gao, W., Feng, Z., Bai, Q., He, J., and Wang, Y. (2019). Melatonin-mediated regulation of growth and antioxidant capacity in salt-tolerant naked oat under salt stress. Int. J. Mol. Sci. 20:1176. doi: 10.3390/ijms20051176
Gao, Z., Gao, S., Li, P., Zhang, Y., Ma, B., and Wang, Y. (2021). Exogenous methyl jasmonate promotes salt stress-induced growth inhibition and prioritizes defense response of Nitraria tangutorum Bobr. Physiol. Plant. 172, 162–175. doi: 10.1111/ppl.13314
Gao, Z., Zhang, J., Zhang, J., Zhang, W., Zheng, L., Borjigin, T., et al. (2022). Nitric oxide alleviates salt-induced stress damage by regulating the ascorbate–glutathione cycle and Na+/K+ homeostasis in Nitraria tangutorum Bobr. Plant Physiol. Biochem. 173, 46–58. doi: 10.1016/j.plaphy.2022.01.017
Geng, W., Li, Z., Hassan, M. J., and Peng, Y. (2020). Chitosan regulates metabolic balance, polyamine accumulation, and Na+ transport contributing to salt tolerance in creeping bentgrass. BMC Plant Biol. 20:506. doi: 10.1186/s12870-020-02720-w
Ghalati, R. E., Shamili, M., and Homaei, A. (2020). Effect of putrescine on biochemical and physiological characteristics of guava (Psidium guajava L.) seedlings under salt stress. Sci. Hortic. 261:108961. doi: 10.1016/j.scienta.2019.108961
Gill, S. S., and Tuteja, N. (2010). Reactive oxygen species and antioxidant machinery in abiotic stress tolerance in crop plants. Plant Physiol. Biochem. 48, 909–930. doi: 10.1016/j.plaphy.2010.08.016
Gohari, G., Alavi, Z., Esfandiari, E., Panahirad, S., Hajihoseinlou, S., and Fotopoulos, V. (2020a). Interaction between hydrogen per-oxide and sodium nitroprusside following chemical priming of Ocimum basilicum L. against salt stress. Physiol. Plant. 168, 361–373. doi: 10.1111/ppl.13020
Gohari, G., Mohammadi, A., Akbari, A., Panahirad, S., Dadpour, M. R., Fotopoulos, V., et al. (2020b). Titanium dioxide nanoparticles (TiO2 NPs) promote growth and ameliorate salinity stress effects on essential oil profile and biochemical at-tributes of Dracocephalum moldavica. Sci. Rep. 10:912. doi: 10.1038/s41598-020-57794-1
Gruszka, D. (2013). The brassinosteroid signaling pathway-new key players and interconnections with other signaling networks crucial for plant development and stress tolerance. Intl. J. Mol. Sci. 14, 8740–8774. doi: 10.3390/ijms14058740
Gou, T., Yang, L., Hu, W., Chen, X., Zhu, Y., Guo, J., et al. (2020). Silicon improves the growth of cucumber under excess nitrate stress by enhancing nitrogen assimilation and chlorophyll synthesis. Plant Physiol. Biochem. 152, 53–61. doi: 10.1016/j.plaphy.2020.04.031
Hamada, A., and Al-Hakimi, A. (2009). Exogenous ascorbic acid or thiamine increases the resistance of sunflower and maize plants to salt stress. Acta Agronomica. Hungarica. 57, 335–347. doi: 10.1556/AAgr.57.2009.3.8
Hamani, A. K. M., Li, S., Chen, J., Amin, A. S., Wang, G., Xiaojun, S., et al. (2021). Linking exogenous foliar application of glycine betaine and stomatal characteristics with salinity stress tolerance in cotton (Gossypium hirsutum L.) seedlings. BMC Plant Biol. 21:146. doi: 10.1186/s12870-021-02892-z
Hancock, J. T. (2019). Hydrogen sulfide and environmental stresses. Environ. Exp. Bot. 161, 50–56. doi: 10.1016/j.envexpbot.2018.08.034
Hancock, J. T., and Whiteman, M. (2016). Hydrogen sulfide signaling: Interactions with nitric oxide and reactive oxygen species. Ann. N. Y. Acad. Sci. 1365, 5–14. doi: 10.1111/nyas.12733
Hasanuzzaman, M., Bhuyan, M. H. M., Zulfiqar, F., Raza, A., Mohsin, S. M., Mahmud, J. A., et al. (2020). Reactive oxygen species and antioxidant defense in plants under abiotic stress: Revisiting the crucial role of a universal defense regulator. Antioxidants 9:681. doi: 10.3390/antiox9080681
Hayat, K., Bundschuh, J., Jan, F., Menhas, S., Hayat, S., Haq, F., et al. (2020). Combating soil salinity with combining saline agriculture and phytomanagement with salt-accumulating plants. Critic. Rev. Environ. Sci. Technol. 50, 1085–1115. doi: 10.1080/10643389.2019.1646087
Hayat, S., Yadav, S., Wani, A. S., Irfan, M., Alyemini, M. N., and Ahmad, A. (2012). Impact of sodium nitroprusside on nitrate reduc-tase, proline content, and antioxidant system in tomato under salinity stress. Hortic. Environ. Biotechnol. 53, 362–367. doi: 10.1007/s13580-012-0481-9
Hemida, K. A., Eloufey, A. Z., Seif El-Yazal, M. A., and Rady, M. M. (2017). Integrated effect of potassium humate and α-tocopherol applications on soil characteristics and performance of Phaseolus vulgaris plants grown on a saline soil. Arch. Agron. Soil Sci. 63, 1556–1571. doi: 10.1080/03650340.2017.1292033
Hoffmann, J., Berni, R., Hausman, J. F., and Guerriero, G. (2020). A review on the beneficial role of silicon against salinity in non-accumulator crops: Tomato as a model. Biomolecules 10:1284. doi: 10.3390/biom10091284
Hojjat, S. S., and Kamyab, M. (2017). The effect of silver nanoparticle on Fenugreek seed germination under salinity levels. Russian Agric. Sci. 43, 61–65. doi: 10.3103/S1068367417010189
Hu, C. H., Wang, P. Q., Zhang, P. P., Nie, X. M., Li, B. B., Tai, L., et al. (2020). NADPH oxidases: The vital performers and center hubs during plant growth and signaling. Cells 9:437. doi: 10.3390/cells9020437
Huang, J., Zhu, C., Hussain, S., Huang, J., Liang, Q., Zhu, L., et al. (2020). Effects of nitric oxide on nitrogen metabolism and the salt resistance of rice (Oryza sativa L.) seedlings with different salt tolerances. Plant Physiol. Biochem. 155, 374–383. doi: 10.1016/j.plaphy.2020.06.013
Ibrahim, E. A. (2016). Seed priming to alleviate salinity stress in germinating seeds. J. Plant Physiol. 192, 38–46. doi: 10.1016/j.jplph.2015.12.011
Jabeen, N., and Ahmad, R. (2013). The activity of antioxidant enzymes in response to salt stress in safflower (Carthamus tinctorius L.) and sunflower (Helianthus annuus L.) seedlings raised from seed treated with chitosan. J. Sci. Food Agric. 93, 1699–1705. doi: 10.1002/jsfa.5953
Jahan, B., Iqbal, N., Fatma, M., Sehar, Z., Masood, A., Sofo, A., et al. (2021). Ethylene supplementation combined with split application of nitrogen and sulfur protects salt-inhibited photosynthesis through optimization of proline metabolism and antioxidant system in mustard (Brassica juncea L.). Plants 10:1303. doi: 10.3390/plants10071303
Jajoo, A. (2013). “Changes in photosystem II in response to salt stress,” in Eco-physiology and responses of plants under salt stress, eds P. Ahmad, M. M. Azooz, and M. N. V. Prasad (New York, NY: Springer), 149–168.
Jayakannan, M., Bose, J., Babourina, O., Rengel, Z., and Shabala, S. (2015). Salicylic acid in plant salinity stress signalling and tolerance. Plant Growth Regul. 76, 25–40. doi: 10.1007/s10725-015-0028-z
Jian, W., Zhang, D. W., Zhu, F., Wang, S. X., Pu, X. J., Deng, X. G., et al. (2016). Alternative oxidase pathway is involved in the exogenous SNP-elevated tolerance of Medicago truncatula to salt stress. J. Plant Physiol. 193, 79–87. doi: 10.1016/j.jplph.2016.01.018
Jiang, J. L., Tian, Y., Li, L., Yu, M., Hou, R. P., and Ren, X. M. (2019). H2S alleviates salinity stress in cucumber by maintaining the Na+/K+ balance and regulating H2S metabolism and oxidative stress response. Front. Plant Sci. 10:678. doi: 10.3389/fpls.2019.00678
Johnson, R., and Puthur, J. T. (2021). Seed priming as a cost effective technique for developing plants with cross tolerance to salinity stress. Plant Physiol. Biochem. 162, 247–257. doi: 10.1016/j.plaphy.2021.02.034
Kahlaoui, B., Hachicha, M., Misle, E., Fidalgo, F., and Teixeira, J. (2018). Physiological and biochemical responses to the exogenous application of proline of tomato plants irrigated with saline water. J. Saudi Soc. Agric. Sci. 17, 17–23. doi: 10.1016/j.jssas.2015.12.002
Kaya, C., Ashraf, M., Alyemeni, M. N., Corpas, F. J., and Ahmad, P. (2020). Salicylic acid-induced nitric oxide enhances arsenic toxicity tolerance in maize plants by upregulating the ascorbate-glutathione cycle and glyoxalase system. J. Hazard Mater 399:123020. doi: 10.1016/j.jhazmat.2020.123020
Kaya, C., Ashraf, M., Sonmez, O., Tuna, A. L., Polat, T., and Aydemir, S. (2015). Exogenous application of thiamin promotes growth and antioxidative defense system at initial phases of development in salt-stressed plants of two maize cultivars differing in salinity tolerance. Acta Physiol. Plant 37, 1–12. doi: 10.1007/s11738-014-1741-3
Khan, I., Raza, M. A., Awan, S. A., Shah, G. A., Rizwan, M., Ali, B., et al. (2020). Amelioration of salt induced toxicity in pearl millet by seed priming with silver nanoparticles (AgNPs): The oxidative damage, antioxidant enzymes and ions uptake are major determinants of salt tolerant capacity. Plant Physiol. Biochem. 156, 221–232. doi: 10.1016/j.plaphy.2020.09.018
Khan, M. I., Fatma, M., Per, T. S., Anjum, N. A., and Khan, N. A. (2015). Salicylic acid-induced abiotic stress tolerance and underlying mechanisms in plants. Front. Plant Sci. 6:462. doi: 10.3389/fpls.2015.00462
Khorshidi, M., and Hamedi, F. (2014). Effect of putrescine on lemon balm under salt stress. Int. J. Agric. Crop Sci. 7, 601–609.
Kim, T. W., and Wang, Z. Y. (2010). Brassinosteroid Signal Transduction from Receptor Kinases to Transcription Factors. Annu. Rev. Plant Biol. 61, 681–704. doi: 10.1146/annurev.arplant.043008.092057
Kolbert, Z., Barroso, J. B., Brouquisse, R., Corpas, F. J., Gupta, K. J., Lindermayr, C., et al. (2019). A forty year journey: The generation and roles of NO in plants. Nitric Oxide 93, 53–70. doi: 10.1016/j.niox.2019.09.006
Kong, Q., Mostafa, H. H., Yang, W., Wang, J., Nuerawuti, M., Wang, Y., et al. (2021). Comparative transcriptome profiling reveals that brassinosteroid-mediated lignification plays an important role in garlic adaption to salt stress. Plant Physiol. Biochem. 158, 34–42. doi: 10.1016/j.plaphy.2020.11.033
Kong, X., Wang, T., Li, W., Tang, W., Zhang, D., and Dong, H. (2016). Exogenous nitric oxide delays salt-induced leaf senescence in cotton (Gossypium hirsutum L.). Acta Physiol. Plant. 38:61. doi: 10.1007/s11738-016-2079-9
Kotula, L., Garcia Caparros, P., Zörb, C., Colmer, T. D., and Flowers, T. J. (2020). Improving crop salt tolerance using transgenic ap-proaches: An update and physiological analysis. Plant Cell Environ. 43, 2932–2956. doi: 10.1111/pce.13865
Labiad, M. H., Giménez, A., Varol, H., Tüzel, Y., Egea-Gilabert, C., Fernández, J. A., et al. (2021). Effect of exogenously applied methyl jasmonate on yield and quality of salt-stressed hydroponically grown sea fennel (Crithmum maritimum L.). Agronomy 11:1083. doi: 10.3390/agronomy11061083
Lang, D., Yu, X., Jia, X., Li, Z., and Zhang, X. (2020). Methyl jasmonate improves metabolism and growth of NaCl-stressed Glycyrrhiza uralensis seedlings. Scientia. Horticult. 266:109287. doi: 10.1016/j.scienta.2020.109287
Li, H., Chang, J., Chen, H., Wang, Z., Gu, X., Wei, C., et al. (2017). Exogenous melatonin confers salt stress tolerance to watermelon by improving photosynthesis and redox homeostasis. Front. Plant Sci. 8:295. doi: 10.3389/fpls.2017.00295
Ling, F., Su, Q., Jiang, H., Cui, J., He, X., Wu, Z., et al. (2020). Effects of strigolactone on photosynthetic and physiological characteristics in salt-stressed rice seedlings. Sci. Rep. 10:6183. doi: 10.1038/s41598-020-63352-6
Liu, W., Li, R. J., Han, T. T., Cai, W., Fu, Z. W., and Lu, Y. T. (2015). Salt stress reduces root meristem size by nitric oxide-mediated modulation of auxin accumulation and signaling in Arabidopsis. Plant physiol. 168, 343–356. doi: 10.1104/pp.15.00030
Liu, X., Quan, W., and Bartels, D. (2022a). Stress memory responses and seed priming correlate with drought tolerance in plants: An overview. Planta 255:45. doi: 10.1007/s00425-022-03828-z
Liu, Y., Cao, X., Yue, L., Wang, C., Tao, M., Wang, Z., et al. (2022b). Foliar-applied cerium oxide nanomaterials improve maize yield under salinity stress: Reactive oxygen species homeostasis and rhizobacteria regulation. Environ. Pollut. 299:118900. doi: 10.1016/j.envpol.2022.118900
Ma, X., Zheng, J., Zhang, X., Hu, Q., and Qian, R. (2017). Salicylic acid alleviates the adverse effects of salt stress on Dianthus superbus (Caryophyllaceae) by activating photosynthesis, protecting morphological structure, and enhancing the antioxidant system. Front. Plant Sci. 8:600. doi: 10.3389/fpls.2017.00600
Ma, Y., Zhang, W., Niu, J., Ren, Y., and Zhang, F. (2019). Hydrogen sulfide may function downstream of hydrogen peroxide in salt stress-induced stomatal closure in Vicia faba. Funct. Plant Biol. 46, 136–145. doi: 10.1071/FP18096
Mahmoud, L. M., Shalan, A. M., El-Boray, M. S., Vincent, C. I., El-Kady, M. E., Grosser, J. W., et al. (2022). Application of silicon nanoparticles enhances oxidative stress tolerance in salt stressed ‘Valencia’ sweet orange plants. Sci. Hortic. 295:110856. doi: 10.1016/j.scienta.2021.110856
Mahmoud, L. M., Vincent, C. I., Grosser, J. W., and Dutt, M. (2021). The response of salt-stressed Valencia sweet orange (Citrus sinensis) to salicylic acid and methyl jasmonate treatments. Plant Physiol. Rep. 26, 137–151. doi: 10.1007/s40502-020-00563-z
Manai, J., Gouia, H., and Corpas, F. J. (2014a). Redox and nitric oxide homeostasis are affected in tomato (Solanum lycopersicum) roots under salinity-induced oxidative stress. J Plant Physiol. 171, 1028–1035. doi: 10.1016/j.jplph.2014.03.012
Manai, J., Kalai, T., Gouia, H., and Corpas, F. J. (2014b). Exogenous nitric oxide (NO) ameliorates salinity-induced oxidative stress in tomato (Solanum lycopersicum) plants. J. Soil Sci. Plant Nutr. 14, 433–446. doi: 10.4067/S0718-95162014005000034
Mangal, V., Lal, M. K., Tiwari, R. K., Altaf, M. A., Sood, S., Kumar, D., et al. (2022). Molecular insights into the role of reactive oxygen, nitrogen and sulphur species in conferring salinity stress tolerance in plants. J. Plant Growth Regul. 1–21. doi: 10.1007/s00344-022-10591-8
Marthandan, V., Geetha, R., Kumutha, K., Renganathan, V. G., Karthikeyan, A., and Ramalingam, J. (2020). Seed priming: A feasible strategy to enhance drought tolerance in crop plants. Int. J. Mol. Sci. 21:8258. doi: 10.3390/ijms21218258
Masoudniaragh, A., Oraei, M., Gohari, G., Akbari, A., and Faramarzi, A. (2021). Using halloysite nanotubes as carrier for proline to alleviate salt stress effects in sweet basil (Ocimum basilicum L.). Sci. Hortic. 285:110202. doi: 10.1016/j.scienta.2021.110202
Miao, Y., Luo, X., Gao, X., Wang, W., Li, B., and Hou, L. (2020). Exogenous salicylic acid alleviates salt stress by improving leaf photosynthesis and root system architecture in cucumber seedlings. Sci. Hort. 272:109577. doi: 10.1016/j.scienta.2020.109577
Molassiotis, A., Job, D., Ziogas, V., and Tanou, G. (2016). Citrus plants: A model system for unlocking the secrets of NO and ROS-inspired priming against salinity and drought. Front. Plant Sci. 7:229. doi: 10.3389/fpls.2016.00229
Molassiotis, A., Tanou, G., and Diamantidis, G. (2010). NO says more than ‘YES’ to salt tolerance: Salt priming and systemic nitric oxide signaling in plants. Plant Signal. Behav. 5, 209–212. doi: 10.4161/psb.5.3.10738
Møller, I. M., Jensen, P. E., and Hansson, A. (2007). Oxidative modifications to cellular components in plants. Annu. Rev. Plant Biol. 58, 459–481. doi: 10.1146/annurev.arplant.58.032806.103946
Moradbeygi, H., Jamei, R., Heidari, R., and Darvishzadeh, R. (2020). Investigating the enzymatic and non-enzymatic antioxidant defense by applying iron oxide nanoparticles in Dracocephalum moldavica L. plant under salinity stress. Sci. Hort. 272:109537. doi: 10.1016/j.scienta.2020.109537
Moradi, P., Vafaee, Y., Mozafari, A. A., and Tahir, N. A. R. (2022). Silicon nanoparticles and methyl jasmonate improve physiological response and increase expression of stress-related genes in strawberry cv. Paros under salinity stress. Silicon 1–11. doi: 10.1007/s12633-022-01791-8
Mosavikia, A. A., Mosavi, S. G., Seghatoleslami, M., and Baradaran, R. (2020). Chitosan nanoparticle and pyridoxine seed priming improves tolerance to salinity in milk thistle seedling. Notulae Botanicae Horti Agrobotanici Cluj-Napoca 48, 221–233. doi: 10.15835/nbha48111777
Mugwanya, M., Kimera, F., Dawood, M., and Sewilam, H. (2022). Elucidating the effects of combined treatments of salicylic acid and l-proline on greenhouse-grown cucumber under saline drip irrigation. J. Plant Growth Regul. 1–17. doi: 10.1007/s00344-022-10634-0
Munns, R., Day, D. A., Fricke, W., Watt, M., Arsova, B., Barkla, B. J., et al. (2020). Energy costs of salt tolerance in crop plants. New Phytol. 225, 1072–1090. doi: 10.1111/nph.15864
Munns, R., and Tester, M. (2008). Mechanisms of salinity tolerance. Ann. Rev. Plant. Physiol. Plant Mol Biol. 59, 651–681. doi: 10.1146/annurev.arplant.59.032607.092911
Naheed, R., Zahid, M., Aqeel, M., Maqsood, M. F., Kanwal, H., Khalid, N., et al. (2022). Mediation of growth and metabolism of pisum sativum in salt stress potentially be credited to thiamine. J. Soil Sci. Plant Nutr. 1–14. doi: 10.1007/s42729-022-00854-4
Nair, A., Bhukya, D. P. N., Sunkar, R., Chavali, S., and Allu, A. D. (2022). Molecular basis of priming-induced acquired tolerance to multiple abiotic stresses in plants. J. Exp. Bot. 73, 3355–3371. doi: 10.1093/jxb/erac089
Naqve, M., Wang, X., Shahbaz, M., Fiaz, S., Naqvi, W., Naseer, M., et al. (2021). Foliar spray of alpha-tocopherol modulates antioxidant potential of okra fruit under salt stress. Plants 10:1382. doi: 10.3390/plants10071382
Nazir, F., Fariduddin, Q., and Khan, T. A. (2020). Hydrogen peroxide as a signalling molecule in plants and its crosstalk with other plant growth regulators under heavy metal stress. Chemosphere 252:126486. doi: 10.1016/j.chemosphere.2020.126486
Noreen, S., Sultan, M., Akhter, M. S., Shah, K. H., Ummara, U., Manzoor, H., et al. (2021). Foliar fertigation of ascorbic acid and zinc improves growth, antioxidant enzyme activity and harvest index in barley (Hordeum vulgare L.) grown under salt stress. Plant Physiol. Biochem. 158, 244–254. doi: 10.1016/j.plaphy.2020.11.007
Orsini, F., Pennisi, G., Mancarella, S., Al Nayef, M., Sanoubar, R., Nicola, S., et al. (2018). Hydroponic lettuce yields are improved under salt stress by utilizing white plastic film and exogenous applications of proline. Sci. Hortic. 233, 283–293. doi: 10.1016/j.scienta.2018.01.019
Parvin, S., Lee, O. R., Sathiyaraj, G., Khorolragchaa, A., Kim, Y. J., and Yang, D. C. (2014). Spermidine alleviates the growth of saline-stressed ginseng seedlings through antioxidative defense system. Gene 537, 70–78. doi: 10.1016/j.gene.2013.12.021
Paul, S., and Roychoudhury, A. (2017). Seed priming with spermine and spermidine regulates the expression of diverse groups of abiotic stress responsive genes during salinity stress in the seedlings of indica rice varieties. Plant Gene. 11, 124–132. doi: 10.1016/j.plgene.2017.04.004
Pérez-Labrada, F., López-Vargas, E. R., Ortega-Ortiz, H., Cadenas-Pliego, G., Benavides-Mendoza, A., and Juárez-Maldonado, A. (2019). Responses of tomato plants under saline stress to foliar application of copper nanoparticles. Plants 8:151. doi: 10.3390/plants8060151
Puyang, X., An, M., Han, L., and Zhang, X. (2015). Protective effect of spermidine on salt stress induced oxidative damage in two Kentucky bluegrass (Poa pratensis L.) cultivars. Ecotoxicol. Environ. Saf. 117, 96–106. doi: 10.1016/j.ecoenv.2015.03.023
Qian, R., Ma, X., Zhang, X., Hu, Q., Liu, H., and Zheng, J. (2021). Effect of exogenous spermidine on osmotic adjustment, antioxidant enzymes activity, and gene expression of Gladiolus gandavensis seedlings under salt stress. J. Plant Growth Regul. 40, 1353–1367. doi: 10.1007/s00344-020-10198-x
Rady, M. O., Semida, W. M., Abd El-Mageed, T. A., Hemida, K. A., and Rady, M. M. (2018). Up-regulation of antioxidative defense systems by glycine betaine foliar application in onion plants confer tolerance to salinity stress. Sci. Hortic. 240, 614–622. doi: 10.1016/j.scienta.2018.06.069
Rahman, N. S. A., Ghazali, N. S., and Yusof, Z. N. B. (2017). Changes in the expressions of thiamine biosynthesis genes (THIC and THI1/THI4) in oil palm (Elaies guineensis) as response to salinity stress. Res. Crops 18, 634–641. doi: 10.5958/2348-7542.2017.00106.1
Rapala-Kozik, M., Wolak, N., Kujda, M., and Banas, A. K. (2012). The upregulation of thiamine (vitamin B1) biosynthesis in Arabidopsis thaliana seedlings under salt and osmotic stress conditions is mediated by abscisic acid at the early stages of this stress response. BMC Plant Biol. 12:2. doi: 10.1186/1471-2229-12-2
Rasheed, F., Sehar, Z., Fatma, M., Iqbal, N., Masood, A., Anjum, N. A., et al. (2021). Involvement of ethylene in reversal of salt stress by salicylic acid in the presence of sulfur in mustard (Brassica juncea L.). J. Plant Growth Regul. 1–18. doi: 10.1007/s00344-021-10526-9
Ren, Y., Wang, W., He, J., Zhang, L., Wei, Y., and Yang, M. (2020). Nitric oxide alleviates salt stress in seed germination and early seedling growth of pakchoi (Brassica chinensis L.) by enhancing physiological and biochemical parameters. Ecotoxicol. Environ. Saf. 187:109785. doi: 10.1016/j.ecoenv.2019.109785
Sahoo, S., Borgohain, P., Saha, B., Moulick, D., Tanti, B., and Panda, S. K. (2019). “Seed priming and seedling pre-treatment induced tolerance to drought and salt stress: Recent advances,” in Priming and pretreat-ment of seeds and seedlings, eds M. Hasanuzzaman and V. Fotopoulos (Singapore: Springer), 253–263.
Saleem, M., Fariduddin, Q., Castroverde, C., and Danve, M. (2021). Salicylic acid: a key regulator of redox signalling and plant immunity. Plant Physiol. Biochem. 168, 381–397. doi: 10.1016/j.plaphy.2021.10.011
Samadi, S., Habibi, G., and Vaziri, A. (2019). Exogenous trehalose alleviates the inhibitory effects of salt stress in strawberry plants. Acta Physiol. Plant. 41:112. doi: 10.1007/s11738-019-2905-y
Sami, F., Siddiqui, H., Alam, P., and Hayat, S. (2021). Nitric oxide mitigates the salt-induced oxidative damage in mustard by upregulating the activity of various enzymes. J. Plant Growth Regul. 40, 2409–2432. doi: 10.1007/s00344-021-10331-4
Sang, T., Shan, X., Li, B., Shu, S., Sun, J., and Guo, S. (2016). Comparative proteomic analysis reveals the positive effect of exogenous spermidine on photosynthesis and salinity tolerance in cucumber seedlings. Plant Cell Rep. 35, 1769–1782. doi: 10.1007/s00299-016-1995-x
Sarkar, A. K., and Sadhukhan, S. (2022). Imperative role of trehalose metabolism and trehalose-6-phosphate signaling on salt stress responses in plants. Physiol. Plant. 174:e13647. doi: 10.1111/ppl.13647
Sarwar, Y., and Shahbaz, M. (2020). Modulation in growth, photosynthetic pigments, gas exchange attributes and inorganic ions in sunflower (Helianthus annuus L.) by strigolactones (GR24) achene priming under saline conditions. Pak. J. Bot. 52, 23–31. doi: 10.30848/PJB2020-1(4)
Savvides, A., Ali, S., Tester, M., and Fotopoulos, V. (2016). Chemical priming of plants against multiple abiotic stresses: Mission possible? Trends Plant Sci. 21, 329–340. doi: 10.1016/j.tplants.2015.11.003
Sehar, Z., Iqbal, N., Khan, M. I. R., Masood, A., Rehman, M., Hussain, A., et al. (2021). Ethylene reduces glucose sensitivity and reverses photosynthetic repression through optimization of glutathione production in salt-stressed wheat (Triticum aestivum L.). Sci. Rep. 11:12650. doi: 10.1038/s41598-021-92086-2
Semida, W. M., Abd El-Mageed, T. A., Howladar, S. M., and Rady, M. M. (2016). Foliar-applied alpha-tocopherol enhances salt-tolerance in onion plants by improving antioxidant defence system. Australian J. Crop Sci. 10, 1030–1039. doi: 10.3316/informit.327621977858527
Semida, W. M., Taha, R. S., Abdelhamid, M. T., and Rady, M. M. (2014). Foliar-applied α-tocopherol enhances salt-tolerance in Vicia faba L. plants grown under saline conditions. South African J. Bot. 95, 24–31. doi: 10.1016/j.sajb.2014.08.005
Shahbaz, M., Mushtaq, Z., Andaz, F., and Masood, A. (2013). Does proline application ameliorate adverse effects of salt stress on growth, ions and photosynthetic ability of eggplant (Solanum melongena L.)? Sci. Hortic. 164, 507–511. doi: 10.1016/j.scienta.2013.10.001
Shahzad, S., Ali, S., Ahmad, R., Ercisli, S., and Anjum, M. A. (2021). Foliar application of silicon enhances growth, flower yield, quality and postharvest life of tuberose (Polianthes tuberosa L.) under saline conditions by improving antioxidant defense mechanism. Silicon 1–8. doi: 10.1007/s12633-021-00974-z
Shams, M., Ekinci, M., Ors, S., Turan, M., Agar, G., Kul, R., et al. (2019). Nitric oxide mitigates salt stress effects of pepper seedlings by altering nutrient uptake, enzyme activity and osmolyte accumulation. Physiol. Mol. Biol. Plants. 25, 1149–1161. doi: 10.1007/s12298-019-00692-2
Shan, C., Liu, H., Zhao, L., and Wang, X. (2014). Effects of exogenous hydrogen sulfide on the redox states of ascorbate and glutathione in maize leaves under salt stress. Biologia. Plant. 58, 169–173. doi: 10.1007/s10535-013-0366-5
Shan, C., and Liu, R. (2017). Exogenous hydrogen peroxide up-regulates the contents of ascorbate and glutathione in the leaves of Vigna radiata (Linn.) Wilczek. exposed to salt stress. Brazil. J. Bot. 40, 583–589. doi: 10.1007/s40415-016-0354-z
Sharma, A., Sidhu, G. P. S., Araniti, F., Bali, A. S., Shahzad, B., Tripathi, D. K., et al. (2020). The role of salicylic acid in plants exposed to heavy metals. Molecules 25:540. doi: 10.3390/molecules25030540
Sheikhalipour, M., Esmaielpour, B., Behnamian, M., Gohari, G., Giglou, M. T., Vachova, P., et al. (2021). Chitosan–Selenium nanoparticle (Cs–Se NP) foliar spray alleviates salt stress in bitter melon. Nanomaterials 11:684. doi: 10.3390/nano11030684
Shen, Z. J., Chen, J., Ghoto, K., Hu, W. J., Gao, G. F., Luo, M. R., et al. (2018). Proteomic analysis on mangrove plant Avicennia marina leaves reveals nitric oxide enhances the salt tolerance by up-regulating photosynthetic and energy metabolic protein expression. Tree Physiol. 38, 1605–1622. doi: 10.1093/treephys/tpy058
Silva, E. N., Ribeiro, R. V., Ferreira-Silva, S. L., Viegas, R. A., and Silveira, J. A. G. (2011). Salt stress induced damages on the photosynthesis of physic nut young plants. Sci Agric. 68, 62–68. doi: 10.1590/S0103-90162011000100010
Soares, C., Carvalho, M., Azevedo, R., and Fidalgo, F. (2019). Plants facing oxidative challenges – a little help from the antioxidant networks. Environ. Exp. Bot. 161, 4–25. doi: 10.1016/j.envexpbot.2018.12.009
Sofy, M. R., Elhawat, N., and Alshaal, T. (2020). Glycine betaine counters salinity stress by maintaining high K+/Na+ ratio and antioxidant defense via limiting Na+ uptake in common bean (Phaseolus vulgaris L.). Ecotoxicol. Environ. Saf. 200:110732. doi: 10.1016/j.ecoenv.2020.110732
Su, Q., Zheng, X., Tian, Y., and Wang, C. (2020). Exogenous brassinolide alleviates salt stress in Malus hupehensis Rehd. by regulating the transcription of NHX-Type Na+ (K+)/H+ Antiporters. Front. Plant Sci. 11:38. doi: 10.3389/fpls.2020.00038
Sun, C., Zhang, Y., Liu, L., Liu, X., Li, B., Jin, C., et al. (2021). Molecular functions of nitric oxide and its potential applications in horticultural crops. Hortic. Res. 8:71. doi: 10.1038/s41438-021-00500-7
Taïbi, K., Abderrahim, L. A., Boussaid, M., Bissoli, G., Taïbi, F., Achir, M., et al. (2021). Salt-tolerance of Phaseolus vulgaris L. is a function of the potentiation extent of antioxidant enzymes and the expression profiles of polyamine encoding genes. South African J. Bot. 140, 114–122. doi: 10.1016/j.sajb.2021.03.045
Tailor, A., Tandon, R., and Bhatla, S. C. (2019). Nitric oxide modulates polyamine homeostasis in sunflower seedling cotyledons under salt stress. Plant Signal. Behav. 14:1667730. doi: 10.1080/15592324.2019.1667730
Talaat, N. B., and Shawky, B. T. (2022). Synergistic effects of salicylic acid and melatonin on modulating ion homeostasis in salt-stressed wheat (Triticum aestivum L.) plants by enhancing root H+-pump activity. Plants 11:416. doi: 10.3390/plants11030416
Talebi, M., Moghaddam, M., and Pirbalouti, A. G. (2018). Methyl jasmonate effects on volatile oil compounds and antioxidant activity of leaf extract of two basil cultivars under salinity stress. Acta Physiol. Plant. 40:34. doi: 10.1007/s11738-018-2611-1
Tanou, G., Molassiotis, A., and Diamantidis, G. (2009). Hydrogen peroxide- and nitric oxide-induced systemic antioxidant prime-like activity under NaCl-stress and stress-free conditions in citrus plants. J. Plant Physiol. 166, 1904–1913. doi: 10.1016/j.jplph.2009.06.012
Torun, H., Novák, O., Mikulík, J., Strnad, M., and Ayaz, F. A. (2022). The Effects of exogenous salicylic acid on endogenous phytohormone status in Hordeum vulgare L. under salt stress. Plants 11:618. doi: 10.3390/plants11050618
Tripathi, D. K., Rai, P., Guerriero, G., Sharma, S., Corpas, F. J., and Singh, V. P. (2020). Silicon induces adventitious root formation in rice (Oryza sativa L.) under arsenate stress with the involvement of nitric oxide and indole-3-acetic acid. J. Exp. Bot. 72, 4457–4471. doi: 10.1093/jxb/eraa488
Tuna, A. L., Kaya, C., Altunlu, H., and Ashraf, M. (2013). Mitigation effects of non-enzymatic antioxidants in maize (Zea mays L.) plants under salinity stress. Aust. J. Crop Sci. 7, 1181–1188. doi: 10.3316/informit.409462099413821
Wang, Y. H., Zhang, G., Chen, Y., Gao, J., Sun, Y. R., Sun, M. F., et al. (2019). Exogenous application of gibberellic acid and ascorbic acid improved tolerance of okra seedlings to NaCl stress. Acta Physiol. Plant. 41:93. doi: 10.1007/s11738-019-2869-y
Wani, A. S., Ahmad, A., Hayat, S., and Tahir, I. (2019). Epibrassinolide and proline alleviate the photosynthetic and yield inhibition under salt stress by acting on antioxidant system in mustard. Plant Physiol. Biochem. 135, 385–394. doi: 10.1016/j.plaphy.2019.01.002
Wani, S. H., Kumar, V., Khare, T., Guddimalli, R., Parveda, M., Solymosi, K., et al. (2020). Engineering salinity tolerance in plants: Progress and prospects. Planta 251:76. doi: 10.1007/s00425-020-03366-6
Wouyou, A., Gandonou, C. B., Komlan, F. A., Montcho, D., Zanklan, A. S., Lutts, S., et al. (2017). Salinity resistance of five amaranth (Amaranthus cruentus) cultivars at young plants stage. Int. J. Plant. Soil Sci. 14, 1–11. doi: 10.9734/IJPSS/2017/31611
Xie, J., Yang, Y., and Patience, B. E. (2022). Trehalose alleviated salt stress in tomato by regulating ROS metabolism, photo-synthesis, osmolyte synthesis, and trehalose metabolic pathways. Front. Plant Sci. 13:772948. doi: 10.3389/fpls.2022.772948
Xiong, F., Liao, J., Ma, Y., Wang, Y., Fang, W., and Zhu, X. (2018). The protective effect of exogenous putrescine in the re-sponse of tea plants (Camellia sinensis) to salt stress. HortSci 53, 1640–1646. doi: 10.21273/HORTSCI13283-18
Xu, J., Liu, T., Qu, F., Jin, X., Huang, N., Wang, J., et al. (2021). Nitric oxide mediates γ-aminobutyric acid-enhanced muskmelon tolerance to salinity–alkalinity stress conditions. Sci. Hortic. 286:110229. doi: 10.1016/j.scienta.2021.110229
Yadav, U. C. S., and Ramana, K. V. (2013). Regulation of NF-kappaB-induced inflammatory signaling by lipid peroxidation-derived aldehydes. Oxid. Med. Cell Longev. 2013:690545. doi: 10.1155/2013/690545
Yang, A., Akhtar, S. S., Iqbal, S., Qi, Z., Alandia, G., Saddiq, M. S., et al. (2018). Saponin seed priming improves salt tolerance in quinoa. J. Agron. Crop Sci. 204, 31–39. doi: 10.1111/jac.12229
Yang, Y., and Guo, Y. (2018). Unraveling salt stress signaling in plants. J. Integrat. Plant Biol. 60, 796–804. doi: 10.1111/jipb.12689
Yildirim, E., Ekinci, M., Turan, M., Dursun, A., Kul, R., and Parlakova, F. (2015). Roles of glycine betaine in mitigating deleterious effect of salt stress on lettuce (Lactuca sativa L.). Arch. Agron. Soil Sci. 61, 1673–1689. doi: 10.1080/03650340.2015.1030611
Yu, Y., Zhang, H., Xing, H., Cui, N., Liu, X., Meng, X., et al. (2021). Regulation of growth and salt resistance in cucumber seedlings by hydrogen-rich water. J. Plant Growth Regul. 1–20. doi: 10.1007/s00344-021-10536-7
Yu, Z., Duan, X., Luo, L., Dai, S., Ding, Z., and Xia, G. (2020). How plant hormones mediate salt stress responses. Trends Plant Sci. 25, 1117–1130. doi: 10.1016/j.tplants.2020.06.008
Yue, E., Cao, H., and Liu, B. (2020). OsmiR535, a potential genetic editing target for drought and salinity stress tolerance in Oryza sativa. Plants 9:1337. doi: 10.3390/plants9101337
Zahedi, S. M., Hosseini, M. S., Abadía, J., and Marjani, M. (2020). Melatonin foliar sprays elicit salinity stress tolerance and enhance fruit yield and quality in strawberry (Fragaria × ananassa Duch.). Plant Physiol. Biochem. 149, 313–323. doi: 10.1016/j.plaphy.2020.02.021
Zhang, A., Liu, Y., Wang, F., Li, T., Chen, Z., Kong, D., et al. (2019). Enhanced rice salinity tolerance via CRISPR/Cas9-targeted mutagenesis of the OsRR22 gene. Mol. Breed. 39:47. doi: 10.1007/s11032-019-0954-y
Zhang, G., Wang, Y., Wu, K., Zhang, Q., Feng, Y., Miao, Y., et al. (2021). Exogenous application of chitosan alleviate salinity stress in lettuce (Lactuca sativa L.). Horticulturae 7:342. doi: 10.3390/horticulturae7100342
Zhang, N., Shi, X., Guan, Z., Zhao, S., Zhang, F., Chen, S., et al. (2016). Treatment with spermidine protects chrysanthemum seedlings against salinity stress damage. Plant Physiol. Biochem. 105, 260–270. doi: 10.1016/j.plaphy.2016.05.002
Zhang, R. H., Li, J., Guo, S. R., and Tezuka, T. (2009). Effects of exogenous putrescine on gas-exchange characteristics and chlorophyll fluorescence of NaCl-stressed cucumber seedlings. Photosynth. Res. 100, 155–162. doi: 10.1007/s11120-009-9441-3
Zhang, T., Shi, Z., Zhang, X., Zheng, S., Wang, J., and Mo, J. (2020). Alleviating effects of exogenous melatonin on salt stress in cucumber. Sci. Hortic. 262:109070. doi: 10.1016/j.scienta.2019.109070
Zhang, X. H., Ma, C., Zhang, L., Su, M., Wang, J., Zheng, S., et al. (2022a). GR24-mediated enhancement of salt tolerance and roles of H2O2 and Ca2+ in regulating this enhancement in cucumber. J. Plant Physiol. 270, 153640. doi: 10.1016/j.jplph.2022.153640
Zhang, X., Zhang, L., Ma, C., Su, M., Wang, J., Zheng, S., et al. (2022b). Exogenous strigolactones alleviate the photosynthetic inhibition and oxidative damage of cucumber seedlings under salt stress. Sci. Hortic. 297:110962. doi: 10.1016/j.scienta.2022.110962
Zhang, Z., Chang, X. X., Zhang, L., Li, J. M., and Hu, X. H. (2016). Spermidine application enhances tomato seedling tolerance to salinity-alkalinity stress by modifying chloroplast antioxidant systems. Russ. J. Plant Physiol. 63, 461–468. doi: 10.1134/S102144371604018X
Zhao, C., Nawaz, G., Cao, Q., and Xu, T. (2022). Melatonin is a potential target for improving horticultural crop resistance to abiotic stress. Sci. Hortic. 291:110560. doi: 10.1016/j.scienta.2021.110560
Zhao, G., Cheng, P., Zhang, T., Abdalmegeed, D., Xu, S., and Shen, W. (2021). Hydrogen-rich water prepared by ammonia borane can enhance rapeseed (Brassica napus L.) seedlings tolerance against salinity, drought or cadmium. Ecotoxicol. Environ. Saf. 224:112640. doi: 10.1016/j.ecoenv.2021.112640
Zhu, Y., Jiang, X., Zhang, J., He, Y., Zhu, X., Zhou, X., et al. (2020). Silicon confers cucumber resistance to salinity stress through regulation of proline and cytokinins. Plant Physiol. Biochem. 156, 209–220. doi: 10.1016/j.plaphy.2020.09.014
Zhu, Y. X., Gong, H. J., and Yin, J. L. (2019). Role of silicon in mediating salt tolerance in plants: A review. Plants 8:147. doi: 10.3390/plants8060147
Zulfiqar, F. (2021). Effect of seed priming on horticultural crops. Sci. Hortic. 286:110197. doi: 10.1016/j.scienta.2021.110197
Zulfiqar, F., and Hancock, J. T. (2020). Hydrogen sulfide in horticulture: Emerging roles in the era of climate change. Plant Physiol. Biochem. 155, 667–675. doi: 10.1016/j.plaphy.2020.08.010
Keywords: antioxidants, bioregulator, hydrogen peroxide, hydrogen sulfide, nitric oxide, chitosan, molecular hydrogen, thiamine
Citation: Zulfiqar F, Nafees M, Chen J, Darras A, Ferrante A, Hancock JT, Ashraf M, Zaid A, Latif N, Corpas FJ, Altaf MA and Siddique KHM (2022) Chemical priming enhances plant tolerance to salt stress. Front. Plant Sci. 13:946922. doi: 10.3389/fpls.2022.946922
Received: 18 May 2022; Accepted: 25 July 2022;
Published: 07 September 2022.
Edited by:
Shah Fahad, The University of Haripur, PakistanReviewed by:
Milan Kumar Lal, Central Potato Research Institute (ICAR), IndiaCopyright © 2022 Zulfiqar, Nafees, Chen, Darras, Ferrante, Hancock, Ashraf, Zaid, Latif, Corpas, Altaf and Siddique. This is an open-access article distributed under the terms of the Creative Commons Attribution License (CC BY). The use, distribution or reproduction in other forums is permitted, provided the original author(s) and the copyright owner(s) are credited and that the original publication in this journal is cited, in accordance with accepted academic practice. No use, distribution or reproduction is permitted which does not comply with these terms.
*Correspondence: Faisal Zulfiqar, Y2guZmFpc2FsLnp1bGZpcWFyQGdtYWlsLmNvbQ==; Kadambot H. M. Siddique, a2FkYW1ib3Quc2lkZGlxdWVAdXdhLmVkdS5hdQ==
Disclaimer: All claims expressed in this article are solely those of the authors and do not necessarily represent those of their affiliated organizations, or those of the publisher, the editors and the reviewers. Any product that may be evaluated in this article or claim that may be made by its manufacturer is not guaranteed or endorsed by the publisher.
Research integrity at Frontiers
Learn more about the work of our research integrity team to safeguard the quality of each article we publish.