- 1Graduate Program in Molecular and Cellular Biology, Biotechnology Center, Federal University of Rio Grande do Sul, Porto Alegre, Brazil
- 2Department of Botany, Institute of Biosciences, Federal University of Rio Grande do Sul, Porto Alegre, Brazil
- 3Center for Plant Aging Research, Institute for Basic Science (IBS), Daegu, South Korea
- 4Department of Agricultural Biotechnology, National Institute of Agricultural Science, Jeonju, South Korea
Iron (Fe) and zinc (Zn) are essential micronutrients needed by virtually all living organisms, including plants and humans, for proper growth and development. Due to its capacity to easily exchange electrons, Fe is important for electron transport in mitochondria and chloroplasts. Fe is also necessary for chlorophyll synthesis. Zn is a cofactor for several proteins, including Zn-finger transcription factors and redox metabolism enzymes such as copper/Zn superoxide dismutases. In humans, Fe participates in oxygen transport, electron transport, and cell division whereas Zn is involved in nucleic acid metabolism, apoptosis, immunity, and reproduction. Rice (Oryza sativa L.) is one of the major staple food crops, feeding over half of the world’s population. However, Fe and Zn concentrations are low in rice grains, especially in the endosperm, which is consumed as white rice. Populations relying heavily on rice and other cereals are prone to Fe and Zn deficiency. One of the most cost-effective solutions to this problem is biofortification, which increases the nutritional value of crops, mainly in their edible organs, without yield reductions. In recent years, several approaches were applied to enhance the accumulation of Fe and Zn in rice seeds, especially in the endosperm. Here, we summarize these attempts involving transgenics and mutant lines, which resulted in Fe and/or Zn biofortification in rice grains. We review rice plant manipulations using ferritin genes, metal transporters, changes in the nicotianamine/phytosiderophore pathway (including biosynthetic genes and transporters), regulators of Fe deficiency responses, and other mutants/overexpressing lines used in gene characterization that resulted in Fe/Zn concentration changes in seeds. This review also discusses research gaps and proposes possible future directions that could be important to increase the concentration and bioavailability of Fe and Zn in rice seeds without the accumulation of deleterious elements. We also emphasize the need for a better understanding of metal homeostasis in rice, the importance of evaluating yield components of plants containing transgenes/mutations under field conditions, and the potential of identifying genes that can be manipulated by gene editing and other nontransgenic approaches.
Introduction
Iron (Fe) and zinc (Zn) are essential micronutrients for plants and humans. Due to its ability to accept and donate electrons, Fe plays a central role in electron transfer reactions in mitochondria and chloroplasts. Fe is also important for chlorophyll biosynthesis, heme biogenesis, and as enzyme cofactor (Briat et al., 2015). Zn is a necessary cofactor for the activities of enzymes such as Cu/Zn superoxide dismutases, carbonic anhydrase, and alkaline phosphatase (Colvin et al., 2010). Transcription factors, such as Zn-finger domain-containing proteins, also need Zn atoms in their functional structures (Maret, 2009). It is estimated that close to 10% of all Arabidopsis thaliana proteins bind Zn (Andreini et al., 2006).
Both elements play roles as cofactors in human proteins (Welch and Graham, 2004). Fe is essential for oxygen transport, because it is part of the functional core of heme group in hemoglobin and myoglobin (Singh et al., 2016). As in plants, Fe is important for electron transport in mitochondria as part of Fe-Sulfur clusters and in DNA replication (Muckenthaler et al., 2017). Zn is important for more than 300 enzymes and 2,000 transcription factors, both structurally and functionally, in humans (Ozyildirim and Baltaci, 2022). Zn is also a signaling molecule in the immune system and in reproduction (Duncan et al., 2016; Singh et al., 2016; Lee et al., 2020; Kim and Lee, 2021).
Rice (Oryza sativa L.) is a staple food for more than half of the world’s population, as 19% of all calories, consumed by humans, are derived from rice grain (Elert, 2014). However, rice grain is low in Fe and Zn. In fact, other grains such as wheat and maize are also poor sources of micronutrients, and populations that rely on these grains as staple food suffer from what is known as “hidden hunger” (Alina et al., 2019; Ali, 2021; Stangoulis and Knez, 2022). It is estimated that over 33% of the world’s population are suffering from Fe deficiency anemia (IDA) and up to 20% are Zn deficient (Garcia-Oliveira et al., 2018). Under this scenario, hidden hunger has become a global challenge, with Fe and Zn being two highly prevalent nutritional deficiencies in humans (Rawat et al., 2013; United Nations Environment Programme, 2021). Biofortification, which aims at increasing nutrient concentration in grains and other edible tissues of plants, is a cost-effective approach that can involve conventional plant breeding, genetic engineering, agronomic practices, or combinations of these (Bouis and Saltzman, 2017).
Rice is mostly consumed as white rice, which is dehusked and polished, avoiding that the oil-rich bran layer (rich in oryzanols, tocopherols, tocotrienols, phytosterols, oil and protein, carbohydrate, and dietary fibers) turns rancid at room temperature when stored for a short period of time (Nagendra Prasad et al., 2011). In rice seeds, Fe is accumulated in the dorsal vascular bundle, the aleurone layer scutellum, and the vascular bundle of the embryo scutellum. Zn can penetrate more into the endosperm, but still has uneven distribution, being more concentrated in the outer layers (Takahashi et al., 2009; Johnson et al., 2011; Kappara et al., 2018). Polishing removes both embryo and aleurone, maintaining the starch-rich but Fe- and Zn-poor endosperm (Sperotto et al., 2012a). On average, polished rice of modern high-yielding varieties has concentrations of approximately 2 µg g-1 Fe and 16 µg g-1 Zn (Trijatmiko et al., 2016). These values are lower than the target values by biofortification programs, 10–15 µg g-1 Fe and 28 µg g-1 Zn. The addition of Fe and Zn up to these values would provide around 30 and 40% above baseline of the estimated average requirement (EAR) for human diet, as has been used as a reference in the field (Bouis et al., 2011; Mahender et al., 2016). Furthermore, a wide genetic variation for Fe and Zn has been reported for brown rice (dehusked grain without polishing), with concentrations varying from 0.4 to 147 µg g-1 and 15.1 to 124 µg g-1 for Fe and Zn, respectively (Zeng et al., 2010; Swamy et al., 2021). However, polishing eliminates up to 85% of all Fe, and the observed variability for Fe concentration in polished rice has been found to be limited (Sperotto et al., 2012a; Suman et al., 2021). For Zn, around 25–30% is lost by polishing and a wider genetic variability for Zn concentrations has been observed (Rao et al., 2020). Although eating unpolished/brown rice could at least partially overcome hidden hunger, because it is richer in vitamins and minerals, polished rice is preferred by consumers and has advantages such as longer storage and faster cooking (Liu et al., 2013; Hashimoto et al., 2022). Given that (1) current breeding has not yet achieved the target concentrations for biofortification, (2) genetic engineering strategies showed potential to increase Fe and Zn concentrations, (3) the phenotypes of biofortified rice need to be stable in different environments, and (4) successful biofortification strategies will need to be introduced in local, high-yield genetic backgrounds, it becomes clear that effective biofortification will need a combination of genetic engineering, breeding, and agronomic practices. Therefore, biotechnology has a great potential to improve the concentration of essential nutrients and tackle hidden hunger through biofortification of cereals and other crop plants (Stangoulis and Knez, 2022).
Genetic engineering aiming to increase Fe content in rice endosperm started to be explored more than two decades ago. Common strategies include (1) overexpressing genes involved in Fe uptake from the soil, (2) increasing Fe translocation from roots to shoot and from shoot to grain by manipulating genes involved in synthesis of metal-chelating molecules such as nicotianamine (NA) and mugineic acid (MA), (3) changing expression of genes involved in Fe storage such as ferritin, and (4) manipulating genes that can lower phytic acid content, an anti-nutrient that decreases micronutrient bioavailability (Das et al., 2020). The bottleneck has been the identification of major genes and/or genomic regions that control grain concentrations of Fe, Zn, or both, and their deployment for developing biofortified crops (Gupta et al., 2021), without increasing concentration of harmful elements that can be co-transported with Fe and Zn (Punshon et al., 2018; Ricachenevsky et al., 2018). Although research has advanced substantially in the past decade, the need to deliver biofortified rice grains that can impact human nutrition remains. Moreover, Zn biofortification is largely not addressed, despite the potential for Zn-rich food to positively impact human nutrition worldwide.
The bioavailability of Fe and Zn in rice grain is associated with the concentration of phytic acid (InsP6), which is considered the major source of phosphorus and inositol phosphates in cereal grains (White and Broadley, 2009) but is also an antinutrient that reduces Fe2+ and Zn2+ uptake in the human gut. Multiple studies have aimed to reduce phytate levels in rice grain (Ali et al., 2013; Yamaji et al., 2017; Bhattacharya et al., 2019), a topic that has been thoroughly reviewed elsewhere and falls out of our scope (Perera et al., 2018; Pramitha et al., 2021).
Here, we review the genetic engineering approaches for rice biofortification, providing a detailed summary of transgenic/mutant lines generated and the increase in Fe and/or Zn seed concentration in each (Table 1). This will allow proper comparisons between approaches and will help researchers decide which ones work best when making new combinations or exploring similar pathways. A graphic representation of these approaches and candidate genes is shown in Figure 1. We direct readers to other reviews (Kawakami and Bhullar, 2021), which provide comparative tables in which yield components are evaluated, as well as to reviews where broader aspects of biofortification are discussed (Jha and Warkwntin, 2020; Kamaral et al., 2022; Stangoulis and Knez, 2022; Stanton et al., 2022; Wani et al., 2022).
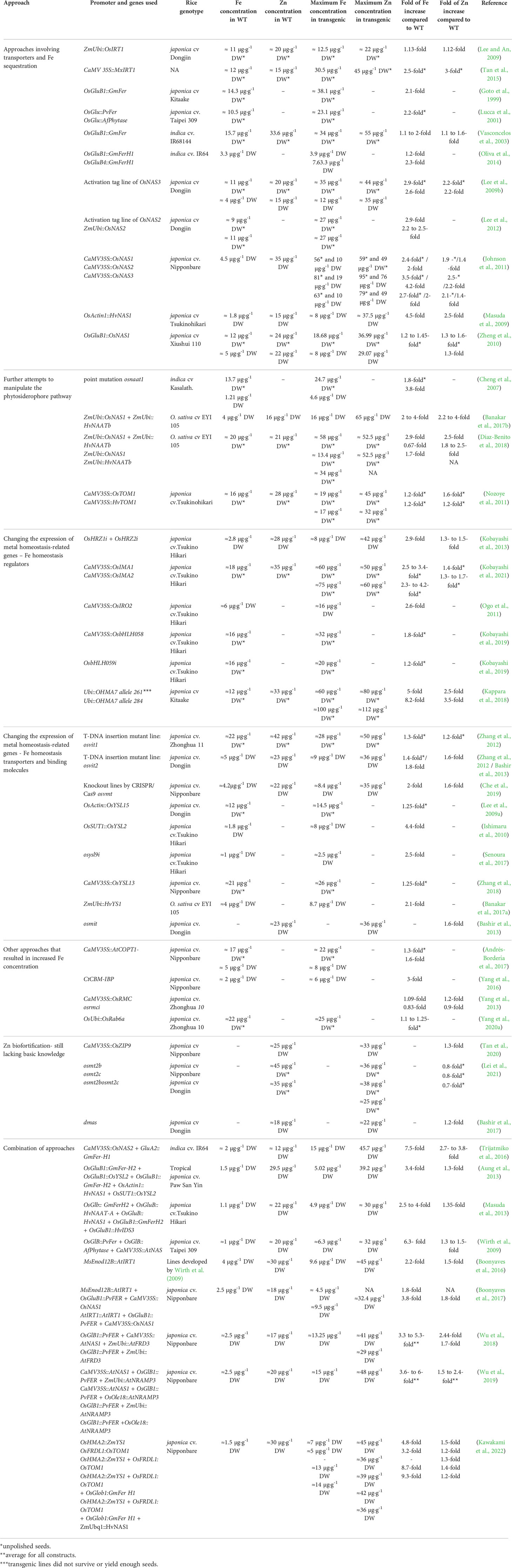
Table 1 List of transgenic/mutant rice approaches to increase rice grain Fe and Zn concentration in seeds.
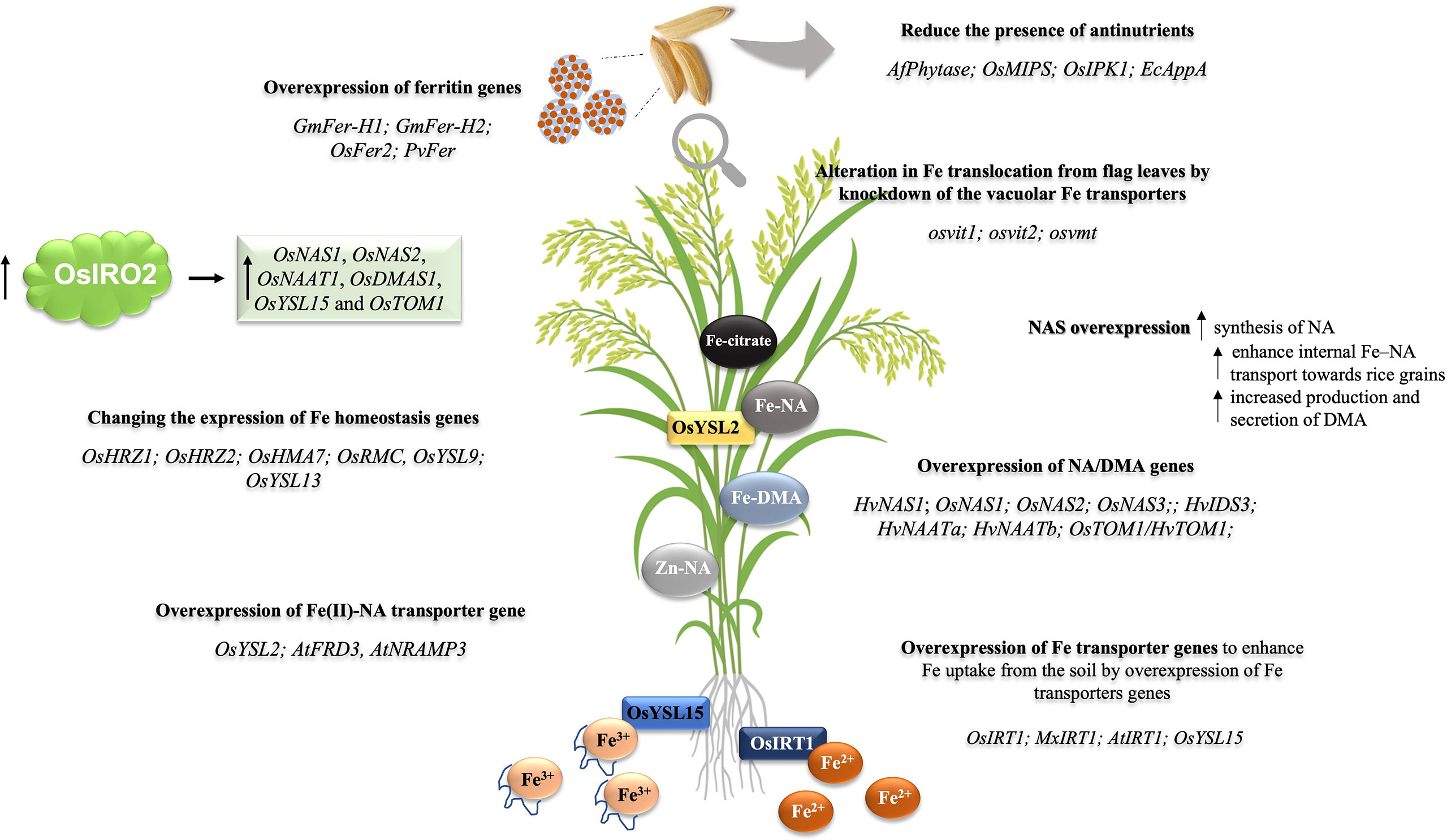
Figure 1 Transgenic approaches to Fe/Zn biofortification in rice plants. The figure presents the main strategies employed for the development of events aiming at higher concentrations of Fe or Fe and Zn in rice grains. (I) The overexpression of ferritin genes, aiming to enhance Fe accumulation in seeds by introducing Fe storage proteins; ferritin genes used: GmFer-H1; GmFer-H2; OsFer2; PvFer. (II) Changing the expression of transcriptional regulators of Fe deficiency responses from the bHLH family, OsIRO2, aiming at enhanced Fe uptake and translocation. (III) Changing the expression of Fe homeostasis-related genes. (IV) Enhancing Fe uptake and translocation by the overexpression of Fe transporters, for example, enhance Fe uptake from soil by the overexpression of Fe transporter gene OsIRT1 or OsYSL15. (V) Reduce the presence of antinutrients aiming to enhance the bioavailability of Fe. (VI) Enhancing the translocation from flag leaves to seeds by knockout/knockdown of the vacuolar Fe transporter genes OsVIT1, OsVIT2, and OsVMT; (VII) Enhancing Fe transport within the plant body by the overexpression of NAS and DMA genes.
Manipulations of Fe transporters and Fe storage
Surprisingly, attempts at biofortification involving Fe and Zn transporter manipulation, either changing expression patterns or substrate specificity, are scarce, which may derive from the lack of literature on how plants deliver Fe and Zn to seeds, especially in grasses. One of the first examples was the overexpression of rice Iron-Regulated Transporter 1 (OsIRT1) under the control of ZmUbi (Lee and An, 2009). Overexpression of OsIRT1 slightly increased Fe and Zn concentrations in brown seeds compared with WT plants (1.13- and 1.12-fold, respectively). However, transgenic plants were shorter and had fewer tillers, probably as a consequence of disturbed metal homeostasis (Lee and An, 2009). Following a similar approach, MxIRT1 (from Malus xiaojinensis) was overexpressed in rice, leading to a threefold increase of Fe and Zn in brown seeds but without significant changes in plant size. However, authors have not reported whether tillering or seed set was altered (Tan et al., 2015).
Conversely, the manipulation of Fe storage or sequestration by changing Ferritin expression is one of first ideas attempted for Fe biofortification. Ferritin is an Fe-storage protein found in plants, animals, and bacteria. Plant ferritin is estimated to accumulate up to 4,500 atoms of Fe in a bioavailable form (Briat et al., 2010). Approaches to induce Fe accumulation in rice seeds by tissue-specific expression of ferritin genes were successful to some extent. Early work using soybean (Glycine max) ferritin gene (GmFerH1) driven by the endosperm-specific OsGluB1 (Glutelin B1, LOC_Os02g15169 / Os02g0249900; Masuda et al., 2013) promoter in the rice Kitaake background (Goto et al., 1999) resulted in threefold higher Fe levels in brown rice of transgenic lines, ranging from 13.3 to 38.1 µg g-1, whereas in WT varied from 8.6 to 14.3 µg g-1. In polished rice, Fe concentrations averaged 3.4 µg g-1 in grains from the transgenic lines, whereas those in WT averaged 1.6 µg g-1 (Goto et al., 1999).
Similar approaches were used in distinct genetic backgrounds, with similar results. GmFer driven by the endosperm-specific OsGluB1 promoter was expressed in the indica elite rice line IR68144 (Gregorio et al., 2000). Fe concentration in polished rice seeds ranged from 17 to 34 µg g-1 compared with 15 µg g-1 in WT seeds. Zn levels also increased, ranging from 36 to 55 µg g-1 compared with 33 µg g-1 in WT (Vasconcelos et al., 2003). Similarly, the expression of ferritin from lima bean (Phaseolus limensis) under the control of GluB1 promoter in the Chinese high-yield variety Wuxiangjin 9 led to 64% increase in Fe concentration in polished rice compared with WT (Liu et al., 2004). The expression of a ferritin gene from Phaseolus vulgaris driven by an endosperm-specific promoter in Taipei 309 background resulted in increased Fe concentration in brown rice, ranged from 11.5 to 22 µg g-1, a twofold increase compared with WT (9.9–10.65 µg g-1 Fe) (Lucca et al., 2001).
Therefore, the endosperm-specific expression of ferritin resulted in increased Fe in polished rice grain. However, despite the increase in ferritin protein in the endosperm, Fe loading seems to be limited, because transgenic lines with six- to sevenfold increase in ferritin protein expression were shown to achieve only approximately threefold increase in grain Fe concentration (Oliva et al., 2014). This suggests that ferritin accumulation does not lead to a proportional increase in Fe concentration. Therefore, other bottlenecks may exist and need to be overcome to further increase Fe loading in the endosperm. Still, these works show that ferritin expression in endosperm is useful for biofortification and that the use of other genes to manipulate Fe allocation to the endosperm might be a promising alternative.
Strategic manipulation of nicotianamine synthase, phytosiderophore synthesis and transport genes in iron and zinc biofortification
Rice is a plant that uses the “Combined Strategy” for Fe uptake, which means that it has a full chelation strategy and also uses the Fe(II) transporter OsIRT1 for Fe uptake (Ishimaru et al., 2006; Wairich et al., 2019; Pradhan et al., 2020; Bashir and Ishimaru, 2021). The chelation strategy is based on phytosiderophore (PS) release to the rhizosphere. In rice, the PS 2′-deoxymugineic acid (DMA) is secreted into the rhizosphere through OsTOM1 (Transporter of Mugineic acid family)/OsZIFL4 (Zinc-Induced Facilitator-Like) transporter (Nozoye et al., 2011; Ricachenevsky et al., 2011). In the rhizosphere, DMA chelates Fe(III) and the complex Fe(III)-DMA is transported into root cells by the transmembrane protein Yellow Stripe-like 15 (OsYSL15) (Inoue et al., 2009; Lee et al 2009a). Rice plants can also directly uptake Fe(II) by OsIRT1/OsIRT2 transporters (Ishimaru et al., 2006), a trait that is shared with Oryza species from the Oryza sativa complex (AA genome; Wairich et al., 2019). This could be an adaptation to habitat changes, such as from upland to deep water (Menguer et al., 2017), where Fe(II) is more available, similar to flooded soils in which domesticated rice is cultivated nowadays.
Interestingly, DMA and its precursor, NA are also involved in long-distance metal transport within the plant. NA is biosynthesized by trimerization of S-adenosylmethionine by NA synthase (NAS) enzymes, which is then converted into a 3′′-keto intermediate by NA amino transferase (NAAT) (Takahashi et al., 1999), and next converted into DMA by the reduction of 3′′-carbon of the keto intermediate by deoxymugineic acid synthase (DMAS) (Bashir et al., 2006). NA chelates Fe(II) and Zn(II) and is involved in transport of both metals in the phloem (Nozoye, 2018). Given that phloem translocation is involved in delivering Fe and Zn to developing seeds (Sperotto et al., 2012b), manipulation of NAS expression has been explored for biofortification.
The rice genome encodes three NAS genes, OsNAS1, OsNAS2, and OsNAS3, which are differentially regulated by Fe (Inoue et al., 2003). In a study with OsNAS3 activation-tagged mutant, Lee et al. (2009b) showed that unpolished grain from mutants had higher concentrations of Fe (2.9-fold), Zn (2.2-fold), and Cu (1.7-fold) than WT plants. Similar increases in Fe (2.6-fold) and Zn (2.2-fold) concentrations were observed in polished rice, whereas NA and DMA concentration in seeds were increased 9.6- and 4.0-fold, respectively (Lee et al., 2009b). Similarly, OsNAS2 activation-tagged mutants and OE-OsNAS2 transgenic plants driven by the maize ubiquitin (ZmUbi) promoter increased Fe concentration in brown rice by 2.3- to 3.0-fold, whereas Fe concentrations in polished rice were 2.2 to 2.9-fold higher, comparing transgenic lines to WT (Lee et al., 2012). Importantly, mice fed with seeds from both activation-tagged mutant lines recovered more rapidly from dietary Fe and Zn deficiency than mice fed with WT seeds, suggesting both nutrients become more bioavailable by increased NA concentration (Lee et al., 2009b; Lee et al., 2011; Lee et al., 2012; Table 1 and Figure 1).
In an independent work, the overexpression of OsNAS1, OsNAS2, or OsNAS3 under the control of the constitutive promoter CaMV35S in rice plants also resulted in increased metal concentrations (Johnson et al., 2011). Brown rice Fe concentrations were 2.4-, 3.5-, and 2.7-fold higher in OE-OsNAS1, OE-OsNAS2, and OE-OsNAS3, respectively, compared with WT, whereas Zn concentrations were 1.9-, 2.5-, and 2.1-fold higher, respectively. Fe and Zn concentrations were also increased in polished seeds, especially in OsNAS2-overexpressing lines, which achieved up to 4.2-fold for Fe and 2.2-fold for Zn. These increases were positively correlated with NA concentration and are well within the target range for biofortification (Johnson et al., 2011; Moreno-Moyano et al., 2016). In a work using heterologous expression, rice transgenic lines expressing barley (Hordeum vulgare) HvNAS1 under the control of OsActin1 promoter were shown to have increased Fe and Zn concentrations in polished seeds by 4.5- and 2.5-fold, respectively, compared with non-transformed plants (Masuda et al., 2009). Besides the variation in fold-change numbers, these studies demonstrated that NAS overexpression in rice results in increased Fe and Zn concentration in seeds.
In a different approach using NAS genes, an elite rice genotype from China expressing OsNAS1 under the control of OsGluB1 promoter reached significant increase in NA concentration in grain, leading to increased concentration of Fe and Zn in brown rice. However, the Fe concentrations in polished grain were similar to WT (Zheng et al., 2010). Still, in vitro Caco-2 cell digestion model showed that Fe from transgenic polished grain was more bioavailable (2- to 2.3-fold), suggesting that NA has a role in increasing Fe bioavailability independently from its role in increasing metal concentration. Zn concentration, on the other hand, increased significantly in these lines (~1.5-fold) in both unpolished and polished grain (Zheng et al., 2010). Taken together, these studies established that manipulation of NAS gene expression enhances internal Fe– and Zn–NA translocation to rice grain and showed that manipulation of NA levels in rice plants is a feasible strategy for biofortification.
However, it should be noted that changes in NA concentrations in rice also resulted in decreased yield in some studies (Moreno-Moyano et al., 2016; Trijatmiko et al., 2016; for a review, see Kawakami and Bhullar, 2021), suggesting that there are trade-offs in NA overaccumulation. Therefore, future work should focus on fine tuning of NAS expression. For example, NA accumulation in phloem cells is important for biofortification and should be further explored using specific promoters. On the other hand, NA overaccumulation in specific organelles, such as chloroplast and mitochondria, could be detrimental, and should also be addressed (Lee et al., 2009b; Lee et al., 2012). Moreover, despite the known relationship of NAS genes with Fe homeostasis, there is not a clear understanding of the role of each NAS in rice. Functional characterization of NAS genes using single and higher order mutants, as done in A. thaliana (Klatte et al., 2009; Schuler et al., 2012), may shed light on the physiological nature of these trade-offs, which could explain the yield effects observed (Kawakami and Bhullar, 2021) and provide knowledge to use NAS as a tool for biofortification.
A knockout mutation in the gene encoding the PS synthesis enzyme OsNAAT1 led to disrupted DMA biosynthesis. When these lines were cultivated in field conditions under continuous flooding, they grew normally and accumulated more Fe in seeds than WT plants. The interpretation was that, although osnaat1 loss of function precluded DMA secretion and Fe(III) uptake through OsYSL15, Fe(II) uptake was still functional, allowing growth under flooded conditions (Cheng et al., 2007). It also raised the question whether plants relying only on Fe(II) uptake achieve higher Fe concentrations in seeds. Fe concentrations in unpolished and polished grain were 1.8- and 3.8-fold higher than their WT, respectively (Cheng et al., 2007). Banakar et al. (2017b) produced transgenic lines overexpressing rice OsNAS1 and barley HvNAATb under the control of ZmUbi. Endosperm Fe levels in these lines were 1.4–3.7 times higher than in WT, and Zn levels were also boosted by 1.2- to 4.2-fold, while limiting the accumulation of cadmium (Cd), a harmful element that can often co-accumulate in rice endosperm, demonstrating that manipulation of the same pathway beyond NAS genes might be beneficial (Banakar et al., 2017b). Importantly, the authors suggest that there is an upper limit for Fe (~22.5 µg g-1) and Zn (~84 µg g-1) accumulation in the endosperm, regardless of the external Fe concentration in soil solution. This upper limit occurs possibly as a homeostatic mechanism to prevent Fe overloading during seed development, and these values never exceeded 4.6-fold more than the value observed in WT (Banakar et al., 2017b), which may represent a trade-off to biofortification. Additionally, these values are consistent with the limited natural genetic diversity of Fe levels in rice, as was observed ranging from 2 to 5 µg g-1 DW (Sperotto et al., 2012b). A threshold of 6 µg Fe g−1 DW in plants expressing AtNAS1 was observed by Wirth et al. (2009), and a threshold of 14 µg Fe g−1 DW in plants expressing OsNAS2 was observed by Johnson et al. (2011). The results described above highlight how little we know about the mechanisms orchestrating Fe and Zn accumulation in rice seeds.
Rice plants overexpressing OsNAS1 and/or HvNAATb were generated to evaluate whether contrasting levels of NA and DMA would play a role in Fe and Zn distribution in embryo and endosperm of rice seeds. The endosperm Fe concentrations in OsNAS1 and OsNAS1 + HvNAATb co-transformed lines were 1.7- to 2.9-fold higher than in WT plants. On the other hand, the Fe concentrations were 33% lower in the endosperm of HvNAATb-only and co-transformed lines. Additionally, endosperm Zn concentrations in OsNAS1 and OsNAS1 + HvNAATb co-transformed lines increased by 1.8- to 2.5-fold and 2.5-fold, respectively (Diaz-Benito et al., 2018). Taken together, these results demonstrate that changing NA/DMA concentrations by fine tuning the expression of biosynthetic genes is a promising avenue to deliver biofortified rice. However, we still need to clearly understand the physiological roles of each gene involved in the PS synthesis pathway and the precise roles of NA/DMA in rice plants.
An alternative approach to achieve Fe biofortification by manipulating PSs was the overexpression of the OsTOM1/OsZIFL4 transporter, which is directly involved in the efflux of DMA from the roots to the rhizosphere (Nozoye et al., 2011). OsTOM1 is part of the ZIFL gene family that has expanded in grasses probably as a result of larger use of PSs (Ricachenevsky et al., 2011). Rice plants overexpressing either OsTOM1 or HvTOM1 driven by CaMV35S promoter have increased DMA secretion from roots and improved tolerance to Fe deficiency, as well as a 1.2-fold increase in brown seed Fe and Zn concentrations compared with WT plants (Nozoye et al., 2011). It is not clear, however, how the increased expression of this transporter leads to changes in seed concentration, and more studies are necessary to uncover other possible roles of OsTOM1/OsZIFL4 in metal accumulation in seeds.
Changing the expression of metal homeostasis-related genes
Work focused on understanding the biological role of Fe and Zn homeostasis often produce plants with increased concentrations of Fe or Zn. Although these studies were not attempted to generate biofortified plants per se, they inform the community about good targets and mechanisms that can be explored for biofortification. Here, we review these studies, which can be useful for devising new single and multi-gene biofortification strategies. These data are also summarized in Table 1 and Figure 1.
Hemerythrin motif-containing Really interesting new gene- and Zinc-finger proteins 1 and 2 (OsHRZ1 and OsHRZ2) are homologous to the E3 ubiquitin ligases BRUTUS/BRUTUS-Like from A. thaliana (Selote et al., 2015; Hindt et al., 2017; Rodríguez-Celma et al., 2019). These proteins act as negative regulators of the Fe deficiency response, degrading key components involved in increasing Fe uptake by roots. OsHRZ1 and OsHRZ2 play a similar role as negative regulators of Fe deficiency-inducible genes for Fe uptake and translocation in rice roots and are also proposed to function as Fe sensors (Kobayashi et al., 2013). Rice plants knocked down for OsHRZ1 and OsHRZ2 grew healthily and without visible phenotype under normal conditions and accumulated up to 2.9-fold more Fe in polished seeds and up to 1.3- to 1.5-fold Zn compared with WT (Kobayashi et al., 2013). OsHRZs were later shown to be involved in a reciprocal regulatory module linking Fe and phosphorus homeostasis (Guo et al., 2021), suggesting that manipulation of these genes for biofortification, although possible, can have pleiotropic effects. Therefore, aiming at regulators such as HRZ might have deleterious effects and understanding of its functional in integrating multiple signals should be a focus in the field.
IRON MAN (IMA) proteins were first described in A. thaliana as positive regulators of Fe-deficiency-inducible genes for Fe uptake (Grillet et al., 2018). In rice, two IMA genes (OsIMA1 and OsIMA2) were shown to be involved in modulating the Fe deficiency response (Kobayashi et al., 2021). Transgenic rice lines overexpressing OsIMA1 and OsIMA2 under the control of CaMV35S promoter increased Fe concentration in brown rice from 2.5- to 3.4-fold in OE-OsIMA1 and from 2.3- to 4.2-fold in OE-OsIMA2. Likewise, the Zn concentration also increases 1.4-fold in OE-OsIMA1 and from 1.3- to 1.7-fold in OE-OsIMA2. Additionally, these lines were more tolerant to Fe deficiency than WT plants (Kobayashi et al., 2021). As overexpression of OsIMA genes and knockdown of HRZs confer similar phenotypes (Kobayashi et al., 2013; Kobayashi et al., 2021), a promising strategy to enhance Fe and Zn concentrations in rice grain might rely on simultaneously altering the expression of members of both gene families.
bHLH (basic Helix–Loop–Helix) proteins play a role as transcriptional regulators of Fe deficiency responses in plants (reviewed by Gao and Dubos, 2021; Riaz et al., 2021). OsIRO2, an Fe-deficiency inducible bHLH transcription factor, positively regulates genes involved in Fe deficiency response, such as OsNAS1, OsNAS2, OsNAAT1, OsDMAS1, OsYSL15, and OsTOM1 (Ogo et al., 2006). Plants overexpressing OsIRO2 displayed increased tolerance to low-Fe availability in long-term cultivation and grew better than WT plants when grown on calcareous soil. Additionally, OE-OsIRO2 lines accumulated twofold higher Fe in grain than WT when cultivated in calcareous soil (Ogo et al., 2011). The bHLH transcription factors subgroup IVc OsbHLH058, and OsbHLH059 are also associated to Fe deficiency responses and are downregulated under Fe deficiency (Kobayashi et al., 2019). Transgenic lines overexpressing or knockdown for OsbHLH058 and OsbHLH059 were developed. OE-OsbHLH058 lines had improved tolerance to low Fe when compared with WT while also having significantly higher Fe concentration in brown seeds (~1.8-fold). On the other hand, osbHLH058-i and osbHLH059-i knockdown lines were more susceptible to low Fe availability, and brown seeds of osbHLH058-i had approximately 20% less Fe compared with WT lines. Counterintuitively, brown seeds from osbHLH059-i lines had 1.2-fold increase in Fe concentrations (Kobayashi et al., 2019). These results show that manipulation of regulatory proteins that control multiple genes, such as transcription factors protein, stability regulators, and others, should be taken cautiously when aiming at biofortification, because they may lead to undesirable pleiotropic effects.
OsHMA7 is a transporter from the Heavy Metal Associated family, and its function was uncharacterized until recently. Two OsHMA7 alleles from contrasting recombinant introgression lines (261 and 284 with high- and low-grain Fe and Zn concentrations, respectively) were identified (Kappara et al., 2018). The overexpression of the 261 (OE-261) allele resulted in increased grain concentrations of Fe (up to 60 µg g-1) and Zn (up to 80 µg g-1) in the T0 generation. However, the transgenic lines did not survive or yield enough seeds. On the other hand, the OE-284 line resulted in increases of Fe (up to 99.07 µg g-1) and Zn (up to 112.5 µg g-1) concentrations. These values are significantly higher than those observed in non-transformed Kitaake plants (12–13 µg g-1 for Fe and 32–33 µg g-1 for Zn, respectively). In addition, the OE lines acquired tolerance to Fe- and Zn-deficient soils. The transcript level of OsHMA7 was fivefold higher in OE-284 lines than in Kitaake, a change that altered transcript levels of Fe- and Zn-responsive genes (Kappara et al., 2018). These results suggest that OsHMA7 may play a role in regulating Fe and Zn concentrations in rice grain.
Transporters associated with regulation of Fe traffic between cytosol and vacuole have been identified in A. thaliana and rice (Kim et al., 2006; Zhang et al., 2012). Arabidopsis Vacuolar Iron Transporter 1 (AtVIT1) mediates vacuolar sequestration of Fe2+ and Mn2+ and is highly expressed in developing seeds. Interestingly, seeds from atvit1 plants have similar Fe concentrations compared with WT, whereas spatial distribution is severely altered, resulting in lower germination in alkaline soil (Kim et al., 2006). Rice homologous genes OsVIT1 and OsVIT2 were characterized: Both are highly expressed in flag leaves, whereas the encoded proteins transport Fe and Zn into vacuoles. Disruption of OsVIT1 and OsVIT2 leads to increased accumulation of Fe and Zn in brown rice seeds (1.3- to 1.4-fold for Fe and 1.2- to 1.6-fold for Zn), whereas flag leaf concentrations are decreased (Zhang et al., 2012). In polished osvit2 seeds, the concentrations of Fe and Zn are significantly higher (Bashir et al., 2013). Authors suggested that the loss of function of either OsVIT1 or OsVIT2 leads to higher availability of Fe and Zn in flag leaf cells cytoplasm, which would be more readily translocated to seeds. Recently, analyses of a CRISPR-Cas9–generated OsVIT2 mutant showed that the OsVIT2 protein plays a role in Fe accumulation in the aleurone layer cells and that the loss of function leads to increased Fe accumulation in the endosperm (Zhang et al., 2012; Che et al., 2021). Polished grains from osvit2 mutant plants cultivated in soil accumulated 1.4–1.8 times more Fe than WT grains without yield reduction (Che et al., 2021), demonstrating that this gene is a target for biofortification.
OsVMT/OsZIFL12 (vacuolar mugineic acid transporter/zinc-induced facilitator-like 12; Ricachenevsky et al., 2011; Che et al., 2019) is a tonoplast-localized transporter of DMA with high expression in upper nodes, peduncle, and rachis at the flowering stage. osvmt knockout mutants accumulated around twofold higher Fe and 1.6-fold higher Zn than WT lines, in addition to increased DMA concentration, without yield reduction (Che et al., 2019). These results showed the importance of OsVMT to homeostasis of Fe and Zn and suggest the possibility to combine OsVMT knockout with other promising candidate genes to increase in Fe and Zn concentrations in endosperm. Together, OsVIT1/2 and OsVMT are yet underexplored promising candidates for biofortification.
OsYSL15 was characterized as the main Fe(III)-DMA transporter from the soil into root cells (Inoue et al., 2009; Lee et al., 2009a) and, therefore, a key protein in strategy II Fe uptake for rice plants. Fe concentrations in seeds in OE-OsYSL15 plants were higher than in WT, whereas the seed Fe concentration in osysl15 lines was ~85% lower than in WT. Zn concentrations were not changed by overexpression or knockout of OsYSL15 (Lee et al., 2009a). Another functionally characterized metal transporter of the same gene family is OsYSL2, which localizes to the plasma membrane and transports Fe(II)-NA and manganese Mn(II)-NA into the phloem, making it important for long-distance transport of metals, including transport into the grain (Koike et al., 2004). The Fe concentration in seeds of osysl2 knockout lines was 37% lower than in WT plants. Strikingly, when OsYSL2 was overexpressed under the control of OsSUT1 promoter, a transporter of sucrose from the phloem to seeds, Fe concentration increased up to 4.4-fold higher than that in WT polished seeds (Ishimaru et al., 2010).
OsYSL9 was identified as a plasma membrane Fe(II)-NA and Fe(III)-DMA transporter, which is responsible for Fe transport from endosperm to embryo in developing seed (Senoura et al., 2017). Fe concentration was slightly higher in brown seeds of OsYSL9-RNAi plants than in WT seeds, whereas Fe concentration in OsYSL9-RNAi embryos was reduced when compared with WT. However, the Fe concentration was more than twofold higher in polished seeds of OsYSL9-RNAi than of WT (Senoura et al., 2017). OsYSL13 is plasma membrane localized, is induced in roots by Fe deficiency, and has higher expression in the leaf blades and sheaths of plants during vegetative, flowering, and grain filling stage (Zhang et al., 2018). OE-OsYSL13 and osysl13 knockout lines were evaluated for Fe concentration in brown rice and seeds. The Fe concentration in osysl13 mutant plants was lower than in WT whereas higher in OE-OsYSL13 lines (Zhang et al., 2018).
Barley HvYS1 is an Fe(III)-PS transporter, performing a similar role compared with OsYSL15 (Murata et al., 2006). Based on the assumption that constitutive HvYS1 expression might improve Fe uptake, Banakar et al. (2017a) expressed HvYS1 driven by ZmUbi promoter in rice plants. Fe concentration in polished seeds from plants expressing HvYS1 (8.7 µg g-1) were 2.1-fold higher than in WT plants (average 4.0 µg g-1). In addition, transgenic lines also accumulated significantly higher levels of DMA in all tissues evaluated (Banakar et al., 2017a). Additionally, the selective loading of Fe into the endosperm by HvYS1 make this transporter an interesting target for rice and barley Fe biofortification.
OsMIT, a mitochondrial Fe transporter, is essential for rice growth and development (Bashir et al., 2011). Fe concentration was significantly lower in unpolished and polished seeds from osmit knockdown lines than in WT plants. However, polished seeds from osmit plants accumulated higher Zn concentrations when compared with WT (Bashir et al., 2013). These data show that changing transporter expression and/or tissue-specific patterns is a promising avenue for biofortification.
Iron accumulation in rice seeds as a result of genetic modification for gene functional characterization
Studies with other aims, including gene functional characterization, often result in changes in metal concentration, which could then be harnessed for generating biofortified rice plants (Table 1 and Figure 1). A study in rice seedlings based in global gene expression showed that copper (Cu) had an impact on Fe homeostasis (Andrés-Bordería et al., 2017). To investigate this cross talk, the authors developed transgenic lines constitutively expressing AtCOPT1, a high-affinity Cu transport protein from A. thaliana. The Fe concentrations in the unpolished and polished rice grain were higher than in the WT by 30 and 60%, respectively. Additionally, young leaves from mutant lines had higher Fe concentrations than old leaves in mutant lines, confirming that ectopic expression of AtCOPT1 in rice plants affects Fe homeostasis in sink tissues (Andrés-Bordería et al., 2017).
Aiming to increase in planta Fe accumulation, as Fe acts as a catalyst to enhance the degradation of lignocellulosic biomass for biofuel production, a fusion polypeptide CBM-IBP, (combining carbohydrate-binding module family 11 [CBM11] and an iron-binding peptide [IBP]), was engineered for secretion into rice cell walls. There was an increase of threefold in Fe concentration in polished seeds from engineered lines compared with WT (Yang et al., 2016).
OsRMC is a receptor-like protein associated to the positive regulation of Fe acquisition in rice plants. When transgenic OE-OsRMC and knockdown osrmc lines were evaluated, Fe concentration in seeds from OE-OsRMC plants were approximately 9% higher than in WT. However, in knockdown lines the Fe concentrations were 17% lower than in WT seeds. Additionally, Zn concentrations were also significantly higher in OE-OsRMC plants and lower in knockdown lines. These results show that overexpression of OsRMC led to enhanced accumulation of Fe and Zn in mature seeds (Yang et al., 2013).
Recently studies demonstrate that high rates of elevated atmospheric CO2 concentrations have a significant impact on the growth, development, and yield of rice plants (Kanno et al., 2017). Studies have shown that the concentrations of essential macro- and micronutrients as Ca, Mn Mg, Fe, and Zn are decreased by elevated atmospheric CO2 concentrations in rice polished grains (Guo et al., 2015; Al-Hadeethi et al., 2019). A small rice GTPase OsRab6a was identified as playing a role on regulation of Fe homeostasis (Yang and Zhang, 2016). Aiming to understand the role of OsRab6a, overexpressing OsRab6a plants were developed. Transgenic lines produced higher biomass and increased grain yield when cultivated under elevated CO2 rates. The expression of genes associated with Fe acquisition was increased in OE-OsRab6a plants under elevated CO2 rates. Overexpression of OsRab6a had an impact on grain Fe concentration, with higher levels of Fe under elevated CO2 conditions than in WT plants under the same treatment. These results suggest that OsRab6a can potentially mitigate the reduction on nutritional quality and to improve the yield of rice plants under elevated CO2 rates (Yang et al., 2020a).
Zn homeostasis and biofortification: Still lagging behind
Rice grain Zn concentration is also a quantitative trait and is dependent on several processes such as availability of mineral in soil solution, uptake from soil, assimilation within the plant body, and remobilization into the grain (Sperotto et al., 2013; Garcia-Oliveira et al., 2018; Suman et al., 2021). Recently, some significant progress has been made in developing and releasing high Zn rice varieties, with new varieties being released for commercial cultivation in Indonesia, India, Bangladesh, and the Philippines (Inabangan-Asilo et al., 2019; Anusha et al., 2021). However, the capacity to apply basic knowledge of Zn homeostasis to biofortification is still lagging behind compared with Fe. This is highlighted by the fact that most Zn biofortified lines generated were obtained with Fe as a priority. It also demonstrates that our understanding of basic Zn homeostasis is less explored than Fe homeostasis.
Fe and Zn can both be transported as divalent cations by some transporters and seem to share phloem translocation mechanisms to developing seeds (Zhang et al., 2012). However, few studies specifically attempted to increase Zn concentration in rice seeds (Table 1 and Figure 1). Moreover, besides recent advances, functional characterization of genes that resulted in increased Zn concentration are also less common than those related to Fe homeostasis in rice.
The ZIP gene family is the most commonly associated with Zn homeostasis in plants. There are 16 members in the rice ZIP family. Two of those genes, OsZIP5 and OsZIP9, are duplicated in tandem in the rice genome. The proteins encoded by these genes were clearly shown to be the primary Zn transporters from the soil into rice roots (Huang et al., 2020; Tan et al., 2020; Yang et al., 2020b). The knockout of OsZIP5 and/or OsZIP9 resulted in lower grain Zn concentration and in significant yield loss under field condition. Likewise, the overexpression of OsZIP5 resulted in reduced Zn concentration in brown rice and also in yield loss (Lee et al., 2010). On the other hand, the overexpression of OsZIP9 increased grain Zn concentration in brown rice by approximately 1.3-fold, when compared with WT plants, without yield penalty (Tan et al., 2020). If increased OsZIP9 expression can result in high Zn concentration in polished grain as well, this gene could have a high potential for rice Zn biofortification.
Two genes encoding metallothioneins (MTs) highly expressed in rice nodes were identified, OsMT2b and OsMT2c. Both genes play a role on scavenging reactive oxygen species and are associated with stress tolerance (Wong et al., 2004; Steffens and Sauter, 2009; Liu et al., 2015). Knockout lines of osmt2b, osmt2c, and osmt2bosmt2c were evaluated for Zn seed concentration. In brown rice grains, Zn concentration was lower in single and double mutants of OsMT2b and OsMT2c than in WT, with the double mutant showing a more severe reduction in Zn concentration. However, osmt2b and osmt2c accumulated more Zn in node I. In addition, decreased grain yield was observed (Lei et al., 2021). These results indicate that the knockout of OsMT2b and OsMT2c consistently decreased the distribution of Zn to the panicle but increased in the upper nodes and make these genes the targets for Zn biofortified rice using overexpression.
DMAS is a member of the aldo-keto reductase super family, encoding the protein that performs the last step in DMA synthesis. Brown and polished seeds from soil-grown dmas knockdown plants accumulated significantly higher Zn levels (1.2-fold) than WT seeds (Bashir et al., 2017). However, no differences in Fe concentration between brown and polished rice was observed. It would be interesting to further explore how the manipulation of the PS biosynthesis pathway and DMA transport could be combined in order to further increase Fe and/or Zn concentrations in polished grains.
The potential of approaches combining multiple Fe and Zn homeostasis genes
Approaches simultaneously employing multiple Fe and Zn homeostasis genes have the potential to combine the best features of each and be more effective. OsNAS2 was expressed under the control of CaMV35S promoter and soybean Ferritin gene (GmFer-H1) under the control of GlutelinA2 (GluA2) promoter as a single cassette in variety IR64. Fe concentration in polished seeds was 15 µg g-1, an increase of 7.5-fold compared with WT in field conditions in two different sites. Additionally, Zn concentration increased by 2.7- to 3.8-fold WT, achieving up to 53.8 µg g-1 (Trijatmiko et al., 2016).
Transgenic lines simultaneously expressing two soybean Ferritin (GmFer-H2) genes under the control of two endosperm-specific promoters, the OsGlB1 and OsGluB1; barley HvNAS1 gene under the control of OsActin1 promoter; and rice OsYSL2, an Fe(II)-NA transporter (Koike et al., 2004), under the control of a sucrose transporter promoter (OsSUT1) and OsGlB1 promoter; showed 4.4-fold Fe and 1.6-fold Zn concentration increase when cultivated in the field (Masuda et al., 2012). The same strategy was employed by Aung et al. (2013) using variety Paw San Yin, which naturally has high Fe concentration in polished seeds. The transgenic lines accumulated 3.4- and 1.3-fold higher Fe and Zn concentrations, respectively, in polished seeds compared with WT (Aung et al., 2013).
Another strategy was developed by Masuda et al. (2013). Tsukino Hikari rice cultivar was transformed with constructs containing GmFerH2, one driven by OsGluB1 and another by OsGlB endosperm-specific promoters; with barley HvNAS1; with two NAAT barley genes, HvNAAT-A, HvNAAT-B; and an MA synthase gene (HvIDS3). Authors aimed to combine the results from ferritin expression in endosperm while increasing MA concentration in rice plants. The average Fe concentrations in polished seeds ranged from 2.5- to 4-fold higher than WT lines. Additionally, Zn concentrations in polished seeds was, on average, 1.35-fold higher than in the WT (Masuda et al., 2013). This work suggested that ferritin combined with enhanced accumulation of PSs can lead to synergistic effects on biofortification.
Based on the same constructs previously used by Lucca et al. (2001) (and described above), Wirth et al. (2009) introduced in japonica cultivar Taipei 309 the Ferritin gene from P. vulgaris (PvFer) and a phytase gene from Aspergillus fumigatus (AfPhytase - to reduce the phytate content and improve Fe bioavailability in rice seeds) both under the control of OsGlB promoter. Authors also introduced A. thaliana NAS gene (AtNAS1) under the control of a constitutive promoter. Fe concentration in polished seeds was 6.3-fold higher than in WT, whereas Zn was 1.3- to1.5-fold (Wirth et al., 2009). Employing the lines developed by Wirth et al. (2009); Boonyaves et al. (2016) further expressed AtIRT1 driven by Medicago sativa Early Nodulin 12B (MsEnod12B) promoter, which drives expression in rice vascular tissue and root epidermal cells (Boonyaves et al., 2016). The introduction of AtIRT1 increased Fe concentration from 4 µg g-1 (for plants grown in soil) to 9.6 µg g-1 in polished grains of plants grown in soil, a 2.2-fold increase when compared with original lines. In addition, Zn concentrations increased up to 1.5-fold in polished grains when compared with the WT plants (Boonyaves et al., 2016). These results point to the fact that the simultaneous expression of AtIRT1, AtNAS1, and PvFer is an effective strategy to biofortify Fe and Zn in rice. It also highlights the importance of accessing previously generated biofortified lines, allowing for comparison of different combination of transgenes without the need to generate events de novo.
Following a similar strategy, different constructs were introduced in rice with two distinct promoters controlling AtIRT1 expression: MsEnod12B or native AtIRT1 promoter. Each construct was expressed together with PvFer driven by OsGlB1 and AtNAS1 driven by CaMV35S promoters as a single locus gene cassette (Boonyaves et al., 2017). In plants carrying AtIRT1 under the control of MsEnod12B promoter, polished grains had 5.0 µg g-1 Fe concentration, whereas the average values observed in control lines were 2.73 µg g-1 (a 1.8-fold increase). When AtIRT1 was expressed under the control of its native promoter, values reached 10.46 µg g-1 Fe, which represents a 3.8-fold increase. Furthermore, these values reached 4.7-fold higher than the Fe concentration observed in WT line in T3 polished grains, demonstrating that the phenotype might be stable after multiple generations. Zn concentrations were also significantly increased in polished grain (1.8-fold) from the line expressing AtIRT1::AtIRT1 (Boonyaves et al., 2017).
The manipulation of citrate efflux into the xylem, which binds Fe and allows its transport with the transpiration stream, was also evaluated for biofortification (Durrett et al., 2007; Roschzttardtz et al., 2011). Transgenic rice plants were generated constitutively expressing AtFRD3 (Ferric Reductase Defective 3), which encodes a transporter that loads citrate into the xylem (Durrett et al., 2007) together with PvFer controlled by the OsGlB1 promoter and expressing AtNAS1 controlled by a constitutive promoter. The rationale was to facilitate long-distance Fe transport as well as efficient Fe uptake and storage in the rice endosperm. Citrate levels in the xylem sap were 2.0-fold higher in transgenic lines than in WT. Overexpressing lines also had increased Fe levels in polished rice grain (3.3- to 5.3-fold), when compared with WT. Zn concentrations in polished grains were also significantly higher (up to 2.44-fold). Furthermore, lines were tolerant to low Fe and to aluminium toxicity (Wu et al., 2018). These results show that manipulating citrate levels through expression of AtFRD3 or other similar transporters has potential for biofortification.
Similarly, Wu et al. (2019) developed transgenic lines expressing AtNRAMP3 (Natural Resistance Associated Macrophage Protein 3) together with AtNAS1 and PvFeR. AtNRAMP3 is localized to the vacuolar membrane (Thomine et al., 2003) and was expressed under the control of ZmUbi or rice embryo/aleurone-specific 18 KDa oleosin (OsOle18) promoters. Fe levels in polished rice grain from all four constructs (CaMV35S::AtNAS1 + OsGlB1::PvFer + ZmUbi::AtNRAMP3; CaMV35S::AtNAS1 + OsGlB1::PvFer + OsOle18::AtNRAMP3; OsGlB1::PvFer + ZmUbi::AtNRAMP3 and OsGlB1::PvFer + OsOle18::AtNRAMP3) were 3.6–6.0 times higher than in WT. Zn levels were also increased (1.5- to 2.4-fold) (Wu et al., 2019). Additionally, the authors introduced AtNRAMP3, AtNAS1, and PvFer cassette in an elite variety, IR64, resulting in higher Fe and Zn levels in polished grain of transgenic IR64 lines, achieving more than 90% of the recommended iron increase (and 170% of the Zn recommended target increase) in rice polished grain (Wu et al., 2019).
Recently, novel rice Fe biofortification strategies have been reported (Kawakami et al., 2022). The first strategy involved expression of maize YS1 controlled by the OsHMA2 promoter (OsHMA2::ZmYS1), leading to 4.8- and 1.5-fold increases in Fe and Zn concentrations in polished grains, respectively. The second strategy involved OsTOM1 expressed under the control of OsFRDL1 promoter (OsFRDL1::OsTOM1), leading to 3.2- and 1.2-fold increases in Fe and Zn concentrations in polished grain, respectively. However, when both strategies were combined, no synergistic effects were observed. Authors further introduced a third construct containing the GmFER gene under control of endosperm-specific promoter (OsGlob1:GmFER H-1). The three-gene strategy resulted in Fe and Zn concentrations increased up to 8.7- and 1.4-fold, respectively, compared with the WT. When the three constructs were combined with constitutive expression of HvNAS1 (ZmUbq1::HvNAS1), Fe and Zn concentrations increased up to 9.3- and 1.2-fold. This last strategy showed that constitutive HvNAS1 expression does not have a strong additive effect on Fe and Zn concentration in polished grain, when compared with the third strategy. Interestingly, the single, two-, and four-gene strategies showed comparable total grain weight to WT, whereas most lines in the three-gene strategy showed decreased total grain weight (Kawakami et al., 2022). This work highlights the variable impact that transgenic approaches can have on yield and suggest that advanced lines should be carefully selected, because some effects might be derived from expression level and cassette insertion position, which could lead to variability between events carrying the same constructs and not only as consequences of transgene expression on physiology.
Concluding remarks and future directions
Although the field of biofortification has made progress in testing multiple strategies and achieved relative success in increasing Fe and Zn in rice grain and endosperm, we still do not have consistent, reliable, and productive elite genotypes with target concentrations being cultivated for human consumption. To deliver that to the public, research in the field might need to focus on issues not addressed yet.
There is a clear need to improve our basic understanding of metal transport to seeds in rice and other model species. Work in the model species A. thaliana showed that Zn loading in developing seeds from the mother plant is performed by AtHMA2/AtHMA4 transporters (Olsen et al., 2016), raising the question whether rice OsHMA2, which was already shown to function similarly to the A. thaliana orthologs in roots (Takahashi et al., 2012), can have a similar function. However, it is important to consider the differences in seed development and structure in eudicots and Poaceae monocots, especially the mature seed endosperm (Galland et al., 2017). Recent work has characterized OsVIT2, which is involved in Fe loading in rice grain (Che et al., 2021), and OsZIP4, which is important for Zn transport to developing reproductive tissues (Mu et al., 2021). OsVIT2 is from the same gene family as AtVIT1, but their functions are not the same regarding how they determine Fe localization in seeds, given the distinct Fe distribution in each species. Moreover, the nodes have been shown to be crucial in controlling metal transport to reproductive tissues in rice (Yamaji and Ma, 2017), highlighting some limitations of using A. thaliana as a model. In that regard, attempts to carry out biofortification in other closely related plant species, such as wheat (Sheraz et al., 2021; Kong et al., 2022), might benefit the rice biofortification field, informing new strategies that could work in rice as well. Still, rice is the model species for cereals, and research on basic Fe and Zn homeostasis in rice is likely to provide the best strategies for biofortification in cereals in general.
Another important problem that should be addressed is the adequate identification of biofortification approaches that do not compromise yield. Kawakami and Bhullar (2021) have summarized which lines maintain and which have decreased yield-related phenotypes. However, this information is not always present in biofortification studies. Moving forward, as different approaches and combinations are attempted, successful events are introduced in local genetic backgrounds, and trials in specific growth conditions are performed, it will be important to compare different studies. One possibility is the adoption of metadata standards, such as those proposed for RNA sequencing (Kumar et al., 2015), microbiome in agriculture (Dundore-Arias et al., 2020), or field trials (Grosse et al., 2021; Walters et al., 2021). At a minimum, reporting on growth, yield and providing open access raw data would improve our ability to evaluate and compare the most successful constructs, events, and combinations of transgenes/mutants.
Thus far, the biofortification field has relied much on transgene insertion for changing nutrient concentration in seeds. However, transgenics are still likely to suffer major resistance from the public, regardless of adequate safety testing. Therefore, a promising strategy could be the identification of mutations, which increase Fe and Zn concentration in seeds and that could be generated by genome editing technologies. For example, CRISPR-Cas9–based loss of function of OsVMT (Che et al., 2019) or OsVIT2 (Che et al., 2021) increased Fe (and Zn in osvmt mutants) concentration in rice endosperm without yield penalties. Identifying other loss-of-function mutations as well as regulatory regions that can be changed by CRISPR-based gene editing and that result in target phenotypes could lead to biofortified rice without transgenes. Pyramiding combinations of such mutations would be feasible to deliver non-transgenic, Fe and Zn-enriched grains to the consumer. Moreover, such initiatives could benefit from better data and materials (such as seeds) sharing among those interested in the field, allowing for statistical and experimental comparisons between lines generated by different groups in variable experimental settings. These practices could accelerate the delivery of biofortified rice to the public.
Author contributions
AW, FR, and SL conceived and initiated the review and were involved in drafting and critically revising the manuscript. All authors contributed to the article and approved the submitted version.
Funding
SL was supported by the Institute for Basic Science (IBS-R013-D1); FR was funded by CNPq (Conselho Nacional de Desenvolvimento Científico e Tecnológico, Brazil) and FAPERGS (Fundação de Amparo à Pesquisa do Estado do Rio Grande do Sul, Brazil). AW funding is currently being provided by the Alexander von Humboldt Foundation (Germany).
Acknowledgments
We would like to thank Dr. Janette P. Fett for proofreading and commenting on the manuscript.
Conflict of interest
The authors declare that the research was conducted in the absence of any commercial or financial relationships that could be construed as a potential conflict of interest.
Publisher’s note
All claims expressed in this article are solely those of the authors and do not necessarily represent those of their affiliated organizations, or those of the publisher, the editors and the reviewers. Any product that may be evaluated in this article, or claim that may be made by its manufacturer, is not guaranteed or endorsed by the publisher.
References
Al-Hadeethi, I., Li, Y., Odhafa, A. K., Al-Hadeethi, H., Seneweera, S., Lam, S. K. (2019). Assessment of grain quality in terms of functional group response to elevated [CO2], water, and nitrogen using a meta-analysis: grain protein, zinc, and iron under future climate. Ecol. Evol. 9, 7425–7437. doi: 10.1002/ece3.5210
Ali, J., Anumalla, M., Murugaiyan, V., Li, Z (2021). “Green Super Rice (GSR) traits: breeding and genetics for multiple biotic and abiotic stress tolerance in rice,” in. Rice Improvement: Physiological, Molecular Breeding and Genetic Perspectives eds.Ali, J., Wani, S. H. (Cham: Springer International Publishing), 59–97 doi: 10.1007/978-3-030-66530-2_3
Alina, V. R., Crina Carmen, M., Sevastita, M., Andruţa, M., Vlad, M., Ramona, S., et al. (2019). “Food fortification through innovative technologies,” in Food engineering. Ed. Coldea, T. E. (London: Intertech), 1–25. doi: 10.5772/intechopen.82249
Ali, N., Paul, S., Gayen, D., Sarkar, S. N., Datta, K., Datta, S. K. (2013). Development of low phytate rice by RNAi mediated seed-specific silencing of inositol 1, 3, 4, 5, 6-pentakisphosphate 2-kinase gene (IPK1). PloS One 8, 1–12. doi: 10.1371/journal.pone.0068161
Andreini, C., Banci, L., Bertini, I., Rosato, A. (2006). Zinc through the three domains of life. J. Proteome Res. 5, 3173–3178. doi: 10.1021/pr0603699
Andrés-Bordería, A., Andrés, F., Garcia-Molina, A., Perea-García, A., Domingo, C., Puig, S., et al. (2017). Copper and ectopic expression of the arabidopsis transport protein COPT1 alter iron homeostasis in rice (Oryza sativa l.). Plant Mol. Biol. 95, 17–32. doi: 10.1007/s11103-017-0622-8
Anusha, G., Rao, D. S., Jaldhani, V., Beulah, P., Neeraja, C. N., Gireesh, C., et al. (2021). Grain fe and zn content, heterosis, combining ability and its association with grain yield in irrigated and aerobic rice. Sci. Rep. 11, 1–13. doi: 10.1038/s41598-021-90038-4
Aung, M. S., Masuda, H., Kobayashi, T., Nakanishi, H., Yamakawa, T., Nishizawa, N.-K. (2013). Iron biofortification of Myanmar rice. Front. Plant Sci. 4. doi: 10.3389/fpls.2013.00158
Banakar, R., Fern, A. A., Abad, J., Capell, T., Christou, P. (2017a). The expression of heterologous Fe(III) phytosiderophore transporter HvYS1 in rice increases fe uptake , translocation and seed loading and excludes heavy metals by selective fe transport. Plant Biotechnol. J. 15, 423–432. doi: 10.1111/pbi.12637
Banakar, R., Fernandez, A. A., Díaz-Benito, P., Abadia, J., Capell, T., Christou, P. (2017b). Phytosiderophores determine thresholds for iron and zinc accumulation in biofortified rice endosperm while inhibiting the accumulation of cadmium. J. Exp. Bot. 68, 4983–4995. doi: 10.1093/jxb/erx304
Bashir, K., Inoue, H., Nagasaka, S., Takahashi, M., Nakanishi, H., Mori, S., et al. (2006). Cloning and characterization of deoxymugineic acid synthase genes from graminaceous plants. J. Biol. Chem. 281, 32395–32402. doi: 10.1074/jbc.M604133200
Bashir, K., Ishimaru, Y. (2021). Challenges and opportunities to regulate mineral transport in rice. Biosci. Biotechnol. Biochem. 86, 12–22. doi: 10.1093/bbb/zbab180
Bashir, K., Ishimaru, Y., Shimo, H., Nagasaka, S., Fujimoto, M., Takanashi, H., et al. (2011). The rice mitochondrial iron transporter is essential for plant growth. Nat. Commun. 2, 1–7. doi: 10.1038/ncomms1326
Bashir, K., Nozoye, T., Nagasaka, S., Rasheed, S., Miyauchi, N., Seki, M., et al. (2017). Paralogs and mutants show that one DMA synthase functions in iron homeostasis in rice. J. Exp. Bot. 68, 1785–1795. doi: 10.1093/jxb/erx065
Bashir, K., Takahashi, R., Akhtar, S., Ishimaru, Y., Nakanishi, H., Nishizawa, N. K. (2013). The knockdown of OsVIT2 and MIT affects iron localization in rice seed. Rice 6, 1–6. doi: 10.1186/1939-8433-6-31
Bhattacharya, S., Sengupta, S., Karmakar, A., Sarkar, S. N., Gangopadhyay, G., Datta, K., et al. (2019). Genetically engineered rice with appA gene enhanced phosphorus and minerals. J. Plant Biochem. Biotechnol. 28, 470–482. doi: 10.1007/s13562-019-00505-3
Boonyaves, K., Gruissem, W., Bhullar, N. K. (2016). NOD promoter-controlled AtIRT1 expression functions synergistically with NAS and FERRITIN genes to increase iron in rice grains. Plant Mol. Biol. 90, 207–215. doi: 10.1007/s11103-015-0404-0
Boonyaves, K., Wu, T. Y., Gruissem, W., Bhullar, N. K. (2017). Enhanced grain iron levels in rice expressinig an IRON-REGULATED NICOTIANAMINE SYNTHASE, and FERRITIN gene cassette. Front. Plant Sci. 8, 1–11. doi: 10.3389/fpls.2017.00130
Bouis, H. E., Hotz, C., Mcclafferty, B., Meenakshi, J. V., Pfeiffer, W. H. (2011). Biofortification: a new tool to reduce micronutrient malnutrition. Food Nutr. Bull. 32, 31–40. doi: 10.1177/15648265110321S105
Bouis, H. E., Saltzman, A. (2017). Improving nutrition through biofortification: a review of evidence from HarvestPlus, 2003 through 2016. Glob. Food Sec. 12, 49–58. doi: 10.1016/j.gfs.2017.01.009
Briat, J. F., Dubos, C., Gaymard, F. (2015). Iron nutrition, biomass production, and plant product quality. Trends Plant Sci. 20, 33–40. doi: 10.1016/j.tplants.2014.07.005
Briat, J. F., Duc, C., Ravet, K., Gaymard, F. (2010). Ferritins and iron storage in plants. Biochim. Biophys. Acta 1800, 806–814. doi: 10.1016/j.bbagen.2009.12.003
Cheng, L., Wang, F., Shou, H., Huang, F., Zheng, L., He, F., et al. (2007). Mutation in nicotianamine aminotransferase stimulated the Fe(II) acquisition system and led to iron accumulation in rice. Plant Physiol. 145, 1647–1657. doi: 10.1104/pp.107.107912
Che, J., Yamaji, N., Ma, J. F. (2021). Role of a vacuolar iron transporter OsVIT2 in the distribution of iron to rice grains. New Phytol. 230, 1049–1062. doi: 10.1111/nph.17219
Che, J., Yokosho, K., Yamaji, N., Ma, J. F. (2019). A vacuolar phytosiderophore transporter alters iron and zinc accumulation in polished rice grains. Plant Physiol. 181, 276–288. doi: 10.1104/pp.19.00598
Colvin, R. A., Holmes, W. R., Fontaine, C. P., Maret, W. (2010). Cytosolic zinc buffering and muffling: Their role in intracellular zinc homeostasis. Metallomics 2, 306–317. doi: 10.1039/b926662c
Das, P., Adak, S., Majumder, A. L. (2020). Genetic manipulation for improved nutritional quality in rice. Front. Genet. 11. doi: 10.3389/fgene.2020.00776
Diaz-Benito, P., Banakar, R., Rodriguez-Menédez, S., Capelli, T., Pereoro, R., Christou, P., et al. (2018). Iron and zinc in the embryo and endosperm of rice (Oryza sativa l.) seeds in contrasting 2’-deoximugineic acid/nicotianamine scenarios. Front. Plant Sci. 9, 1–17. doi: 10.3389/fpls.2018.01190
Duncan, F. E., Que, E. L., Zhang, N., Feinberg, E. C., Halloran, T. V. O., Woodruff, T. K. (2016). The zinc spark is an inorganic signature of human egg activation. Sci. Rep. 6, 1–8. doi: 10.1038/srep24737
Dundore-Arias, J. P., Eloe-Fadrosh, E. A., Schrimi, E. A., Beattie, G. A., Brennan, F. P., Busby, P. E., et al. (2020). Community-driven metadata standards for agricultural microbiome research. Phytobiomes J. 4, 115–121. doi: 10.1094/PBIOMES-09-19-0051-P
Durrett, T. P., Gassmann, W., Rogers, E. E. (2007). The FRD3-mediated efflux of citrate into the root vasculature is necessary for efficient iron translocation. Plant Physiol. 144, 197–205. doi: 10.1104/pp.107.097162
Galland, M., He, D., Lounifi, I., Arc, E., Clément, G., Balzergue, S., et al. (2017). An integrated “multi-omics” comparison of embryo and endosperm tissue-specific features and their impact on rice seed quality. Front. Plant Sci. 8. doi: 10.3389/fpls.2017.01984
Gao, F., Dubos, C. (2021). Transcriptional integration of plant responses to iron availability. J. Exp. Bot. 72, 2056–2070. doi: 10.1093/jxb/eraa556
Garcia-Oliveira, A. L., Chander, S., Ortiz, R., Menkir, A., Gedil, M. (2018). Genetic basis and breeding perspectives of grain iron and zinc enrichment in cereals. Front. Plant Sci. 9. doi: 10.3389/fpls.2018.00937
Goto, F., Yoshihara, T., Shigemoto, N., Toki, S., Takaiwa, F. (1999). Iron fortification of rice seed by the soybean ferritin gene. Nat. Biotechnol. 17, 282–286. doi: 10.1038/7029
Gregorio, G. B., Senadhira, D., Htut, H., Graham, R. D. (2000). Breeding for trace mineral density in rice. Food Nutr. Bull. 21, 382–386. doi: 10.1177/156482650002100407
Grillet, L., Lan, P., Li, W., Mokkapati, G., Schmidt, W. (2018). IRON MAN, a ubiquitous family of peptides that control iron transport in plants. Nat. Plants 4, 953–963. doi: 10.1038/s41477-018-0266-y
Grosse, M., Ahlborn, M. C., Hierold, W. (2021). Metadata of agricultural long-term experiments in Europe exclusive of Germany. Data Br. 38, 107322. doi: 10.1016/j.dib.2021.107322
Guo, M., Ruan, W., Zhang, Y., Zhang, Y., Wang, X., Guo, Z., et al. (2021). A reciprocal inhibitory module for Pi and iron signaling. Mol. Plant 15, 138–150. doi: 10.1016/j.molp.2021.09.011
Guo, J., Zhang, M., Wang, X., Zhang, W. (2015). A possible mechanism of mineral responses to elevated atmospheric CO2 in rice grains. J. Integr. Agric. 14, 50–57. doi: 10.1016/S2095-3119(14)60846-7
Gupta, P. K., Balyan, H. S., Sharma, S., Kumar, R. (2021). Biofortification and bioavailability of Zn, Fe and Se in wheat: present status and future prospects. Theor. Appl. Genet. 134, 1–35. doi: 10.1007/s00122-020-03709-7
Hashimoto, M., Hossain, S., Matsuzaki, K., Shido, O., Yoshino, K. (2022). The journey from white rice to ultra-high hydrostatic pressurized brown rice: an excellent endeavor for ideal nutrition from staple food. Crit. Rev. Food Sci. Nutr. 62, 1502–1520. doi: 10.1080/10408398.2020.1844138
Hindt, M. N., Akmakjian, G. Z., Pivarski, K. L., Punshon, T., Baxter, I., Salt, D. E., et al. (2017). BRUTUS and its paralogs, BTS LIKE1 and BTS LIKE2, encode important negative regulators of the iron deficiency response in Arabidopsis thaliana. Metallomics 9, 876–890. doi: 10.1039/C7MT00152E
Huang, S., Sasaki, A., Yamaji, N., Okada, H., Mitani-Ueno, N., Ma, J. F. (2020). The ZIP transporter family member OsZIP9 contributes to root zinc uptake in rice under zinc-limited conditions. Plant Physiol. 183, 1224–1234. doi: 10.1104/pp.20.00125
Inabangan-Asilo, M. A., Mallikarjuna Swamy, B. P., Amparado, A. F., Descalsota-Empleo, G. I. L., Arocena, E. C., Reinke, R. (2019). Stability and G × E analysis of zinc-biofortified rice genotypes evaluated in diverse environments. Euphytica 215, 1–17. doi: 10.1007/s10681-019-2384-7
Inoue, H., Higuchi, K., Takahashi, M., Nakanishi, H., Mori, S., Nishizawa, N. K. (2003). Three rice nicotianamine synthase genes, OsNAS1, OsNAS2, and OsNAS3 are expressed in cells involved in long-distance transport of iron and differentially regulated by iron. Plant J. 36, 366–381. doi: 10.1046/j.1365-313X.2003.01878.x
Inoue, H., Kobayashi, T., Nozoye, T., Takahashi, M., Kakei, Y., Suzuki, K., et al. (2009). Rice OsYSL15 is an iron-regulated iron(III)-deoxymugineic acid transporter expressed in the roots and is essential for iron uptake in early growth of the seedlings. J. Biol. Chem. 284, 3470–3479. doi: 10.1074/jbc.M806042200
Ishimaru, Y., Masuda, H., Bashir, K., Inoue, H., Tsukamoto, T., Takahashi, M., et al. (2010). Rice metal-nicotianamine transporter, OsYSL2, is required for the long-distance transport of iron and manganese. Plant J. 62, 379–390. doi: 10.1111/j.1365-313X.2010.04158.x
Ishimaru, Y., Suzuki, M., Tsukamoto, T., Suzuki, K., Nakazono, M., Kobayashi, T., et al. (2006). Rice plants take up iron as an Fe3+ -phytosiderophore and as Fe2+. Plant J. 45, 335–346. doi: 10.1111/j.1365-313X.2005.02624.x
Jha, A. B., Warkwntin, T. D. (2020). Biofortification of pulse crops: status and future perspectives. Plants 9, 1–29.
Johnson, A. A. T., Kyriacou, B., Callahan, D. L., Carruthers, L., Stangoulis, J., Lombi, E., et al. (2011). Constitutive overexpression of the OsNAS gene family reveals single-gene strategies for effective iron- and zinc-biofortification of rice endosperm. PloS One 6, 1–11. doi: 10.1371/journal.pone.0024476
Kamaral, C., Neate, S. M., Gunasinghe, N., Milham, P. J., Paterson, D. J., Kopittke, P. M., et al. (2022). Genetic biofortification of wheat with zinc: opportunities to fine-tune zinc uptake, transport and grain loading. Physiol. Plant 174e, 1–18. doi: 10.1111/ppl.13612
Kanno, K., Suzuki, Y., Makino, A. (2017). A small decrease in rubisco content by individual suppression of RBCS genes leads to improvement of photosynthesis and greater biomass production in rice under conditions of elevated CO2. Plant Cell Physiol. 58, 635–642. doi: 10.1093/pcp/pcx018
Kappara, S., Neelamraju, S., Ramanan, R. (2018). Down regulation of a heavy metal transporter gene influences several domestication traits and grain Fe-Zn content in rice. Plant Sci. 276, 208–219. doi: 10.1016/j.plantsci.2018.09.003
Kawakami, Y., Bhullar, N. K. (2021). Delineating the future of iron biofortification studies in rice: Challenges and future perspectives. J. Exp. Bot. 72, 2099–2113. doi: 10.1093/jxb/eraa446
Kawakami, Y., Gruissem, W., Bhullar, N. K. (2022). Novel rice iron biofortification approaches using expression of ZmYS1 and OsTOM1 controlled by tissue-specific promoters. J. Exp. Bot 73, 5440–5459. doi: 10.1093/jxb/erac214
Kim, B., Lee, W. (2021). Regulatory role of zinc in immune cell signaling. Mol. Cells 44, 335–341. doi: 10.14348/molcells.2021.0061
Kim, S. A., Punshon, T., Lanzirotti, A., Li, L., Alonso, J. M., Ecker, J. R., et al. (2006). Localization of iron in arabidopsis seed requires the vacuolar membrane transporter VIT1. Sci. (80-. ). 314, 1295–1298. doi: 10.1126/science.1132563
Klatte, M., Schuler, M., Wirtz, M., Fink-Straube, C., Hell, R., Bauer, P. (2009). The analysis of arabidopsis nicotianamine synthase mutants reveals functions for nicotianamine in seed iron loading and iron deficiency responses. Plant Physiol. 150, 257–271. doi: 10.1104/pp.109.136374
Kobayashi, T., Nagano, A. J., Nishizawa, N. K. (2021). Iron deficiency-inducible peptide-coding genes OsIMA1 and OsIMA2 positively regulate a major pathway of iron uptake and translocation in rice. J. Exp. Bot. 72, 2196–2211. doi: 10.1093/jxb/eraa546
Kobayashi, T., Nagasaka, S., Senoura, T., Itai, R. N., Nakanishi, H., Nishizawa, N. K. (2013). Iron-binding haemerythrin RING ubiquitin ligases regulate plant iron responses and accumulation. Nat. Commun. 4, 1–12. doi: 10.1038/ncomms3792
Kobayashi, T., Ozu, A., Kobayashi, S., An, G., Jeon, J.-S., Nishizawa, N. K. (2019). OsbHLH058 and OsbHLH059 transcription factors positively regulate iron deficiency responses in rice. Plant Mol. Biol. 101, 471–486. doi: 10.1007/s11103-019-00917-8
Koike, S., Inoue, H., Mizuno, D., Takahashi, M., Nakanishi, H., Mori, S., et al. (2004). OsYSL2 is a rice metal-nicotianamine transporter that is regulated by iron and expressed in the phloem. Plant J. 39, 415–424. doi: 10.1111/j.1365-313X.2004.02146.x
Kong, D., Khan, S. A., Wu, H., Liu, Y., Ling, H.-Q. (2022). Biofortification of iron and zinc in rice and wheat. J. Integr. Plant Biol. 64, 1157–1167. doi: 10.1111/jipb.13262
Kumar, P., Halama, A., Hayat, S., Billing, A. M., Gupta, M., Yousri, N. A., et al. (2015). MetaRNA-seq: an interactive tool to browse and annotate metadata from RNA-seq studies. BioMed. Res. Int. 2015, 1–6. doi: 10.1155/2015/318064
Lee, S., An, G. (2009). Over-expression of OsIRT1 leads to increased iron and zinc accumulations in rice. Plant Cell Environ. 32, 408–416. doi: 10.1111/j.1365-3040.2009.01935.x
Lee, S., Chiecko, J. C., Kim, S. A., Walker, E. L., Lee, Y., Guerinot, M., et al. (2009a). Disruption of OsYSL15 leads to iron inefficiency in rice plants. Plant Physiol. 150, 786–800. doi: 10.1104/pp.109.135418
Lee, H. C., Edmonds, M. E., Duncan, F. E., Halloran, T. V. O., Woodruff, T. K. (2020). Zinc exocytosis is sensitive to myosin light chain kinase inhibition in mouse and human eggs. Mol. Hum. Reprod. 26, 228–239. doi: 10.1093/molehr/gaaa017
Lee, S., Jeong, H. J., Kim, S. A., Lee, J., Guerinot, M., Lou, et al. (2010). OsZIP5 is a plasma membrane zinc transporter in rice. Plant Mol. Biol. 73, 507–517. doi: 10.1007/s11103-010-9637-0
Lee, S., Jeon, U. S., Lee, J. S., Kim, Y., Persson, D. P., Husted, S., et al. (2009b). Iron fortification of rice seeds through activation of the nicotianamine synthase gene. PNAS 106, 22014–22019. doi: 10.1073/pnas.0910950106
Lee, S., Kim, Y. S., Jeon, U. S., Kim, Y. K., Schjoerring, J. K., An, G. (2012). Activation of rice nicotianamine synthase 2 (OsNAS2) enhances iron availability for biofortification. Mol. Cells 33, 269–275. doi: 10.1007/s10059-012-2231-3
Lee, S., Persson, D. P., Hansen, T. H., Husted, S., Schjoerring, J. K., Kim, Y.-S., et al. (2011). Bio-available zinc in rice seeds is increased by activation tagging of nicotianamine synthase. Plant Biotechnol. J. 9, 865–873. doi: 10.1111/j.1467-7652.2011.00606.x
Lei, G. J., Yamaji, N., Ma, J. F. (2021). Two metallothionein genes highly expressed in rice nodes are involved in distribution of zn to the grain. New Phytol. 229, 1007–1020. doi: 10.1111/nph.16860
Liu, J., Shi, X., Qian, M., Zheng, L., Lian, C., Xia, Y., et al. (2015). Copper-induced hydrogen peroxide upregulation of a metallothionein gene, OsMT2c, from Oryza sativa L . confers copper tolerance in Arabidopsis thaliana. J. Hazard. Mater. 294, 99–108. doi: 10.1016/j.jhazmat.2015.03.060
Liu, L., Waters, D. L. E., Rose, T. J., Bao, J., King, G. J. (2013). Phospholipids in rice: significance in grain quality and health benefits: a review. Food Chem. 139, 1133–1145. doi: 10.1016/j.foodchem.2012.12.046
Liu, Q. Q., Yao, Q. H., Wang, H. M., Gu, M. H. (2004). Endosperm-specific expression of the ferritin gene in transgenic rice (Oryza sativa L.) results in increased iron content of milling rice. Acta Genet. Sin. 31, 518–524.
Lucca, P., Hurrell, R., Potrykus, I. (2001). Genetic engineering approaches to improve the bioavailability and the level of iron in rice grains. Theor. Appl. Genet. 102, 392–397. doi: 10.1007/s001220051659
Mahender, A., Anandan, A., Pradhan, S. K., Pandit, E. (2016). Rice grain nutritional traits and their enhancement using relevant genes and QTLs through advanced approaches. Springerplus 5, 1–18. doi: 10.1186/s40064-016-3744-6
Maret, W. (2009). Molecular aspects of human cellular zinc homeostasis: redox control of zinc potentials and zinc signals. BioMetals 22, 149–157. doi: 10.1007/s10534-008-9186-z
Masuda, H., Ishimaru, Y., Aung, M. S., Kobayashi, T., Kakei, Y., Takahashi, M., et al. (2012). Iron biofortification in rice by the introduction of multiple genes involved in iron nutrition. Sci. Rep. 2, 1–7. doi: 10.1038/srep00543
Masuda, H., Kobayashi, T., Ishimaru, Y., Takahashi, M., Aung, M. S., Nakanishi, H., et al. (2013). Iron-biofortification in rice by the introduction of three barley genes participated in mugineic acid biosynthesis with soybean ferritin gene. Front. Plant Sci. 4. doi: 10.3389/fpls.2013.00132
Masuda, H., Usuda, K., Kobayashi, T., Ishimaru, Y., Kakei, Y., Takahashi, M., et al. (2009). Overexpression of the barley nicotianamine synthase gene HvNAS1 increases iron and zinc concentrations in rice grains. Rice 2, 155–166. doi: 10.1007/s12284-009-9031-1
Menguer, P. K., Sperotto, R. A., Ricachenevsky, F. K. (2017). A walk on the wild side: Oryza species as source for rice abiotic stress tolerance. Genet. Mol. Biol. 40, 238–252. doi: 10.1590/1678-4685-gmb-2016-0093
Moreno-Moyano, L. T., Bonneau, J. P., Sànchez-Palacios, J. T., Tohme, J., Johnson, A. A. T. (2016). Association of increased grain iron and zinc concentrations with agro-morphological traits of biofortified rice. Front. Plant Sci. 7. doi: 10.3389/fpls.2016.01463
Muckenthaler, M. U., Rivella, S., Hentze, M. W., Galy, B. (2017). A red carpet for iron metabolism. Cell 168, 344–361. doi: 10.1016/j.cell.2016.12.034
Murata, Y., Ma, J. F., Yamaji, N., Ueno, D., Nomoto, K., Iwashita, T. (2006). A specific transporter for iron(III)-phytosiderophore in barley roots. Plant J. 46, 563–572. doi: 10.1111/j.1365-313X.2006.02714.x
Mu, S., Yamaji, N., Sasaki, A., Luo, L., Du, B., Che, J., et al. (2021). A transporter for delivering zinc to the developing tiller bud and panicle in rice. Plant J. 105, 786–799. doi: 10.1111/tpj.15073
Nagendra Prasad, M., Sanjay, K., Shravya Khatokar, M., Vismaya, M., Manjunda Swany, S. (2011). Health benefits of rice bran - a review. Nutr. Food 1, 1–7. doi: 10.4172/2155-9600.1000108
Nozoye, T. (2018). The nicotianamine synthase gene is a useful candidate for improving the nutritional qualities and fe-deficiency tolerance of various crops. Front. Plant Sci. 9, 1–7. doi: 10.3389/fpls.2018.00340
Nozoye, T., Nagasaka, S., Kobayashi, T., Takahashi, M., Sato, Y., Sato, Y., et al. (2011). Phytosiderophore efflux transporters are crucial for iron acquisition in graminaceous plants. J. Biol. Chem. 286, 5446–5454. doi: 10.1074/jbc.M110.180026
Ogo, Y., Itai, R. N., Kobayashi, T., Aung, M. S., Nakanishi, H., Nishizawa, N. K. (2011). OsIRO2 is responsible for iron utilization in rice and improves growth and yield in calcareous soil. Plant Mol. Biol. 75, 593–605. doi: 10.1007/s11103-011-9752-6
Ogo, Y., Itai, R. N., Nakanishi, H., Inoue, H., Kobayashi, T., Suzuki, M., et al. (2006). Isolation and characterization of IRO2, a novel iron-regulated bHLH transcription factor in graminaceous plants. J. Exp. Bot. 57, 2867–2878. doi: 10.1093/jxb/erl054
Oliva, N., Chadha-Mohanty, P., Poletti, S., Abrigo, E., Atienza, G., Torrizo, L., et al. (2014). Large-Scale production and evaluation of marker-free indica rice IR64 expressing phytoferritin genes. Mol. Breed. 33, 23–37. doi: 10.1007/s11032-013-9931-z
Olsen, L. I., Hansen, T. H., Larue, C., Østerberg, J. T., Hoffmann, R. D., Liesche, J., et al. (2016). Mother-plant-mediated pumping of zinc into the developing seed. Nat. Plants 2, 1–6. doi: 10.1038/nplants.2016.36
Ozyildirim, S., Baltaci, S. B. (2022). Cardiovascular diseases and zinc. Biol. Trace Elem. Res. doi: 10.1007/s12011-022-03292-6
Perera, I., Seneweera, S., Hirotsu, N. (2018). Manipulating the phytic acid content of rice grain toward improving micronutrient bioavailability. Rice 11, 1–13. doi: 10.1186/s12284-018-0200-y
Pradhan, S. K., Pandit, E., Pawar, S., Pradhan, A., Behera, L., Das, S. R., et al. (2020). Genetic regulation of homeostasis, uptake, bio-fortification and efficiency enhancement of iron in rice. Environ. Exp. Bot. 177, 104066. doi: 10.1016/j.envexpbot.2020.104066
Pramitha, J. L., Rana, S., Aggarwal, P. R., Ravikesavan, R., Joel, A. J., Muthamilarasan, M. (2021). “Diverse role of phytic acid in plants and approaches to develop low-phytate grains to enhance bioavailability of micronutrients,” in. ed. D. B. T.-A. in Kumar, G. (Academic Press), 89–120. doi: 10.1016/bs.adgen.2020.11.003
Punshon, T., Carey, A., Ricachenevsky, F. K., Meharg, A. A. (2018). Elemental distribution in developing rice grains and the effect of flag-leaf arsenate exposure. Environ. Exp. Bot. 149, 51–58. doi: 10.1016/j.envexpbot.2018.02.007.Elemental
Rao, D. S., Neeraja, C. N., Babu, P. M., Nirmala, B., Suman, K., Rao, L. V. S., et al. (2020). Zinc biofortified rice varieties: challenges, possibilities, and progress in India. Front. Nutr. 7. doi: 10.3389/fnut.2020.00026
Rawat, N., Neelam, K., Tiwari, V. K., Dhaliwal, H. S. (2013). Biofortification of cereals to overcome hidden hunger. Plant Breed. 132, 437–445. doi: 10.1111/pbr.12040
Riaz, N., Guerinot, M., Lou (2021). All together now: regulation of the iron deficiency response. J. Exp. Bot. 72, 2045–2055. doi: 10.1093/jxb/erab003
Ricachenevsky, F. K., de Araújo Junior, A. T., Fett, J. P., Sperotto, R. A. (2018). You shall not pass: root vacuoles as a symplastic checkpoint for metal translocation to shoots and possible application to grain nutritional quality. Front. Plant Sci. 9. doi: 10.3389/fpls.2018.00412
Ricachenevsky, F. K., Sperotto, R. A., Menguer, P. K., Sperb, E. R., Lopes, K. L., Fett, J. P. (2011). ZINC-INDUCED FACILITATOR-LIKE family in plants: lineage-specific expansion in monocotyledons and conserved genomic and expression features among rice (Oryza sativa) paralogs. BMC Plant Biol. 11, 1–22. doi: 10.1186/1471-2229-11-20
Rodríguez-Celma, J., Chou, H., Kobayashi, T., Long, T. A., Balk, J. (2019). Hemerythrin E3 ubiquitin ligases as negative regulators of iron homeostasis in plants. Front. Plant Sci. 10. doi: 10.3389/fpls.2019.00098
Roschzttardtz, H., Séguéla-Arnaud, M., Briat, J.-F., Vert, G., Curie, C. (2011). The FRD3 citrate effluxer promotes iron nutrition between symplastically disconnected tissues throughout arabidopsis development. Plant Cell 23, 2725–2737. doi: 10.1105/tpc.111.088088
Schuler, M., Fink-Straube, C., Abadia, J., Bauer, P. (2012). Nicotianamine functions in the phloem-based transport of iron to sink organs, in pollen development and pollen tube growth in arabidopsis. Plant Cell 24, 2380–2400. doi: 10.1105/tpc.112.099077
Selote, D., Samira, R., Matthiadis, A., Gillikin, J. W., Long, T. A. (2015). Iron-binding E3 ligase mediates iron response in plants by targeting basic helix-loop-helix transcription factors. Plant Physiol. 167, 273–286. doi: 10.1104/pp.114.250837
Senoura, T., Sakashita, E., Kobayashi, T., Takahashi, M., Aung, M. S., Masuda, H., et al. (2017). The iron-chelate transporter OsYSL9 plays a role in iron distribution in developing rice grains. Plant Mol. Biol. 95, 375–387. doi: 10.1007/s11103-017-0656-y
Sheraz, S., Wan, Y., Venter, E., Verma, S. K., Xiong, Q., Waites, J., et al. (2021). Subcellular dynamics studies of iron reveal how tissue-specific distribution patterns are established in developing wheat grains. New Phytol. 231, 1644–1657. doi: 10.1111/nph.17440
Singh, U., Praharaj, C. S., Chaturvedi, S. K., Bohra, A. (2016). “Biofortification: introduction, approaches, limitations, and challenges,” in Biofortification of Food Crops (New Delhi), 3–18. doi: 10.1007/978-81-322-2716-8
Sperotto, R. A., Ricachenevsky, F. K., de Waldow, V. A., Müller, A. L. H., Dressler, V. L., Fett, J. P. (2013). Rice grain Fe, Mn and Zn accumulation: how important are flag leaves and seed number? Plant Soil Environ. 59, 262–266. doi: 10.17221/841/2012-PSE
Sperotto, R. A., Ricachenevsky, F. K., Waldow, V., de, A., Fett, J. P. (2012a). Iron biofortification in rice: it’s a long way to the top. Plant Sci. 190, 24–39. doi: 10.1016/j.plantsci.2012.03.004
Sperotto, R. A., Vasconcelos, M. W., Grusak, M. A., Fett, J. P. (2012b). Effects of different fe supplies on mineral partitioning and remobilization during the reproductive development of rice (Oryza sativa L.). Rice 5, 1–11. doi: 10.1186/1939-8433-5-27
Stangoulis, J. C. R., Knez, M. (2022). Biofortification of major crop plants with iron and zinc - achievements and future directions. Plant Soil 474, 57–76. doi: 10.1007/s11104-022-05330-7
Stanton, C., Sanders, D., Krämer, U., Podar, D. (2022). Zinc in plants: integrating homeostasis and biofortification. Mol. Plant 15, 65–85. doi: 10.1016/j.molp.2021.12.008
Steffens, B., Sauter, M. (2009). Epidermal cell death in rice is confined to cells with a distinct molecular identity and is mediated by ethylene and H2O2 through an autoamplified signal pathway. Plant Cell 21, 184–196. doi: 10.1105/tpc.108.061887
Suman, K., Neeraja, C. N., Madhubabu, P., Rathod, S., Bej, S., Jadhav, K. P., et al. (2021). Identification of promising RILs for high grain zinc through genotype × environment analysis and stable grain zinc QTL using SSRs and SNPs in rice (Oryza sativa L .). Front. Plant Sci. 12, 1–24. doi: 10.3389/fpls.2021.587482
Swamy, B. P. M., Marathi, B., Ribeiro-Barros, A. I. F., Calayugan, M. I. C., Ricachnevsky, F. K. (2021). Iron biofortification in rice: an update on quantitative trait loci and candidate genes. Front. Plant Sci. 12. doi: 10.3389/fpls.2021.647341
Takahashi, R., Ishimaru, Y., Shimo, H., Ogo, Y., Senoura, T., Nishizawa, N. K., et al. (2012). The OsHMA2 transporter is involved in root-to-shoot translocation of Zn and Cd in rice. Plant Cell Environ. 35, 1948–1957. doi: 10.1111/j.1365-3040.2012.02527.x
Takahashi, M., Nozoye, T., Kitajima, N., Fukuda, N., Hokura, A., Terada, Y., et al. (2009). In vivo analysis of metal distribution and expression of metal transporters in rice seed during germination process by microarray and X-ray fluorescence imaging of fe, zn, Mn, and Cu. Plant Soil 325, 39–51. doi: 10.1007/s11104-009-0045-7
Takahashi, M., Yamaguchi, H., Nakanishi, H., Shioiri, T., Nishizawa, N.-K., Mori, S. (1999). Cloning two genes for nicotianamine aminotransferase, a critical enzyme in iron acquisition (Strategy II) in graminaceous plants. Plant Physiol. 121, 947–956. doi: 10.1104/pp.121.3.947
Tan, S., Han, R., Li, P., Yang, G., Li, S., Zhang, P., et al. (2015). Over-expression of the MxIRT1 gene increases iron and zinc content in rice seeds. Transgenic Res. 24, 109–122. doi: 10.1007/s11248-014-9822-z
Tan, L., Qu, M., Zhu, Y., Peng, C., Wang, J., Gao, D., et al. (2020). Zinc transporter5 and zinc transporter9 function synergistically in zinc/cadmium uptake. Plant Physiol. 183, 1235–1249. doi: 10.1104/pp.19.01569
Thomine, S., Lelièvre, F., Debarbieux, E., Schroeder, J. I., Barbier-Brygoo, H. (2003). AtNRAMP3, a multispecific vacuolar metal transporter involved in plant responses to iron deficiency. Plant J. 34, 685–695. doi: 10.1046/j.1365-313x.2003.01760.x
Trijatmiko, K. R., Dueñas, C., Tsakirpaloglou, N., Torrizo, L., Arines, F. M., Adeva, C., et al. (2016). Biofortified indica rice attains iron and zinc nutrition dietary targets in the field. Sci. Rep. 6, 1–13. doi: 10.1038/srep19792
United Nations Environment Programme (2021) Food systems hold key to ending world hunger. Available at: https://www.unep.org/news-and-stories/story/food-s.
Vasconcelos, M., Datta, K., Oliva, N., Khalekuzzaman, M., Torrizo, L., Krishnan, S., et al (2003). Enhanced iron and zinc accumulation in transgenic rice with the ferritin gene. Plant Sci. 164, 371–378. doi: 10.1016/S0168-9452(02)00421-1
Wairich, A., de Oliveira, B. H. N., Arend, E. B., Duarte, G. L., Ponte, L. R., Sperotto, R. A., et al. (2019). The combined strategy for iron uptake is not exclusive to domesticated rice (Oryza sativa). Sci. Rep. 9, 1–17. doi: 10.1038/s41598-019-52502-0
Walters, J., Light, K., Robinson, N. (2021). Using agricultural metadata: a novel investigation of trends in sowing date in on-farm research trials using the online farm trials database. F1000 Res. 9, 1–23. doi: 10.12688/f1000research.26903.2
Wani, S. H., Gaikwad, K., Razzaq, A., Samantara, K., Kumar, M., Govidan, V. (2022). Improving zinc and iron biofortification in wheat through genomics approaches. Mol. Biol. Rep., 1–17. doi: 10.1007/s11033-022-07326-z
Welch, R. M., Graham, R. D. (2004). Breeding for micronutrients in staple food crops from a human nutrition perspective. J. Exp. Bot. 55, 353–364. doi: 10.1093/jxb/erh064
White, P. J., Broadley, M. R. (2009). Biofortification of crops with seven mineral elements often lacking in human diets - iron, zinc, copper, calcium, magnesium, selenium and iodine. New Phytol. 182, 49–84. doi: 10.1111/j.1469-8137.2008.02738.x
Wirth, J., Poletti, S., Aeschlimann, B., Yakandawala, N., Drosse, B., Osorio, S., et al. (2009). Rice endosperm iron biofortification by targeted and synergistic action of nicotianamine synthase and ferritin. Plant Biotechnol. J. 7, 631–644. doi: 10.1111/j.1467-7652.2009.00430.x
Wong, H. L., Sakamoto, T., Kawasaki, T., Umemura, K., Shimamoto, K. (2004). Down-regulation of metallothionein, a reactive oxygen scavenger, by the small GTPase OsRac1 in rice. Plant Physiol. 135, 1447–1456. doi: 10.1104/pp.103.036384.invoke
Wu, T.-Y., Gruissem, W., Bhullar, N. K. (2018). Facilitated citrate-dependent iron translocation increases rice endosperm iron and zinc concentrations. Plant Sci. 270, 13–22. doi: 10.1016/j.plantsci.2018.02.002
Wu, T.-Y., Gruissem, W., Bhullar, N. K. (2019). Targeting intracellular transport combined with efficient uptake and storage significantly increases grain iron and zinc levels in rice. Plant Biotechnol. J. 17, 9–20. doi: 10.1111/pbi.12943
Yamaji, N., Ma, J. F. (2017). Node-controlled allocation of mineral elements in Poaceae. Curr. Opin. Plant Biol. 39, 18–24. doi: 10.1016/j.pbi.2017.05.002
Yamaji, N., Takemoto, Y., Miyaji, T., Mitani-Ueno, N., Yoshida, K. T., Ma, J. F. (2017). Reducing phosphorus accumulation in rice grains with an impaired transporter in the node. Nature 541, 92–95. doi: 10.1038/nature20610
Yang, A., Li, Q., Chen, L., Zhang, W. (2020a). A rice small GTPase, Rab6a, is involved in the regulation of grain yield and iron nutrition in response to CO2 enrichment. J. Exp. Bot. 71, 5680–5688. doi: 10.1093/jxb/eraa279
Yang, M., Li, Y., Liu, Z., Tian, J., Liang, L., Qiu, Y., et al. (2020b). A high activity zinc transporter OsZIP9 mediates zinc uptake in rice. Plant J. 103, 1695–1709. doi: 10.1111/tpj.14855
Yang, A., Li, Y., Xu, Y., Zhang, W. H. (2013). A receptor-like protein RMC is involved in regulation of iron acquisition in rice. J. Exp. Bot. 64, 5009–5020. doi: 10.1093/jxb/ert290
Yang, H., Wei, H., Ma, G., Antunes, M. S., Vogt, S., Cox, J., et al. (2016). Cell wall targeted in planta iron accumulation enhances biomass conversion and seed iron concentration in arabidopsis and rice. Plant Biotechnol. J. 14, 1998–2009. doi: 10.1111/pbi.12557
Yang, A., Zhang, W. (2016). A small GTPase, OsRab6a, is involved in the regulation of iron homeostasis in rice. Plant Cell Physiol. 57, 1271–1280. doi: 10.1093/pcp/pcw073
Zeng, Y., Zhang, H., Wang, L., Pu, X., du, J., Yang, S., et al. (2010). Genotypic variation in element concentrations in brown rice from yunnan landraces in China. Environ. Geochem. Health 32, 165–177. doi: 10.1007/s10653-009-9272-3
Zhang, C., Shinwari, K. I., Luo, L., Zheng, L. (2018). OsYSL13 is involved in iron distribution in rice. Int. J. Mol. Sci. 19, 1–14. doi: 10.3390/ijms19113537
Zhang, Y., Xu, Y. H., Yi, H. Y., Gong, J. M. (2012). Vacuolar membrane transporters OsVIT1 and OsVIT2 modulate iron translocation between flag leaves and seeds in rice. Plant J. 72, 400–410. doi: 10.1111/j.1365-313X.2012.05088.x
Keywords: iron, zinc, rice, biofortification, transporter
Citation: Wairich A, Ricachenevsky FK and Lee S (2022) A tale of two metals: Biofortification of rice grains with iron and zinc. Front. Plant Sci. 13:944624. doi: 10.3389/fpls.2022.944624
Received: 15 May 2022; Accepted: 11 October 2022;
Published: 07 November 2022.
Edited by:
Victoria Fernandez, Polytechnic University of Madrid, SpainReviewed by:
Simon Gerben Strobbe, Ghent University, BelgiumFaisal Nadeem, University of The Punjab, Pakistan
Copyright © 2022 Wairich, Ricachenevsky and Lee. This is an open-access article distributed under the terms of the Creative Commons Attribution License (CC BY). The use, distribution or reproduction in other forums is permitted, provided the original author(s) and the copyright owner(s) are credited and that the original publication in this journal is cited, in accordance with accepted academic practice. No use, distribution or reproduction is permitted which does not comply with these terms.
*Correspondence: Felipe K. Ricachenevsky, ZmVsaXBlY3J1emFsdGFAZ21haWwuY29t; Sichul Lee, c2Npcm9ubGVlQGdtYWlsLmNvbQ==
†Present address: Andriele Wairich, Department of Agronomy and Crop Physiology, Institute for Plant Production and Plant Breeding I, Justus-Liebig University Gieβen, Gieβen, Germany