- 1Hainan Jianfengling Forest Ecosystem National Field Science Observation and Research Station, Research Institute of Tropical Forestry, Chinese Academy of Forestry, Guangzhou, China
- 2Key Laboratory of Vegetation Restoration and Management of Degraded Ecosystems, South China Botanical Garden, Chinese Academy of Sciences, Guangzhou, China
Elucidating the effects of atmospheric nitrogen (N) deposition on the photosynthetic capacity of plants is critical to understand forest growth and conservation under global change. However, studies on this topic generally consider only understory N addition, which ignores the effect of canopy interception. In this study, we conducted a field experiment in a subtropical forest to compare the effects of canopy vs. understory N addition on the photosynthetic rate of canopy and understory species. We found that canopy N addition enhanced the photosynthetic rate of canopy species by increasing leaf hydraulic conductivity and shortening the distance of CO2 transportation. In contrast, understory N addition had non-significant effects on the photosynthetic rate of canopy species. Moreover, the photosynthetic rate of understory species was not affected by canopy or understory N addition. Interestingly, changes in hydraulic conductivity contributed more to accelerating the photosynthetic rate than changes in CO2 transport distance. Our results provide important insights into the dissimilar effects of canopy and understory N addition on the photosynthetic rates of species in subtropical forests. Based on our findings, we highlighted the urgent need to consider canopy processes in future studies on N deposition.
Introduction
Nitrogen (N) deposition has increased rapidly in recent decades (Vitousek et al., 1997; Galloway et al., 2004, 2008), and this has greatly impacted plant growth and forest community structures (Swaine, 1996; Zalamea et al., 2016). The physiological features of plants can reflect fundamental processes and the ecological strategies of plants in response to global change (Mao et al., 2017; Wu et al., 2018). Therefore, exploring the physiological mechanisms of how plants adapt to N deposition is fundamental for promoting species growth and conservation (Mo et al., 2008; Simkin et al., 2016).
Photosynthesis is the process by which chloroplasts use CO2 and H2O to synthesize starch. The photosynthetic capacity of plants is collectively governed by suites of traits (Liang et al., 2020). Among these, leaf hydraulic conductivity, stomatal traits, and leaf thickness are critical regulators of photosynthetic rate. Leaf water conductivity represents the capacity of leaves to transport H2O for the photosynthetic process and is associated with carbon accumulation in leaves (Brodribb et al., 2007). Stomatal traits and leaf thickness regulate the distance that CO2 has to travel to reach the chloroplast and may affect CO2 transportation to the site of photosynthesis (Noblin et al., 2008; Drake et al., 2019). A larger stomatal area, higher stomatal density, and lower leaf thickness can shorten the CO2 transport distance and accelerate the photosynthetic rate (Noblin et al., 2008; de Boer et al., 2012; Drake et al., 2019). Previous studies have generally explored the effects of N deposition on these traits individually (Liu N. et al., 2020; Zhang et al., 2021). To the best of our knowledge, no studies have explored the contributions of simultaneous changes in H2O conductivity and CO2 transportation on the photosynthetic rate of plants under N deposition.
Although most previous studies have focused on understory N addition, canopy N addition has received research attention in recent years (Zhang et al., 2015). Unlike understory N addition, canopy N addition takes into consideration the canopy interception process, which is closely associated with natural N deposition. Previous studies have reported that the canopy can intercept a large percentage of N in various types of forests, leading to contrasting nematode communities and differences in fine root biomass between forests with canopy vs. understory N addition (Gaige et al., 2007; Sievering et al., 2007; Liu T. et al., 2020; Li et al., 2021; Wang et al., 2021). If the intercepted N was absorbed by leaves (as suggested by previous studies), it would subsequently affect photosynthesis. However, few studies have explored the underlying mechanism of photosynthesis in species under different N addition treatments (Liu N. et al., 2020; Wang et al., 2021).
In this study, we selected four co-occurring dominant species, including two canopy trees (Castanopsis chinensis and Schima superba) and two understory species (Ardisia quinquegona and Blastus cochinchinensis). Photosynthetic rate, leaf hydraulic conductivity, leaf thickness, and traits related to stomatal anatomy (including stomatal area and stomatal density) were measured in the four species. We addressed the following questions: (1) how does canopy vs. understory N addition affect the leaf hydraulic conductivity, leaf thickness, and stomatal anatomy of canopy trees and understory species? and (2) how do these changes affect plant photosynthetic capacity under N addition?
Materials and methods
Study site
The study site was located in a subtropical natural forest in the Shimentai National Nature Reserve (24°22′–24°31′ N, 113°05′–113°31′ E) of Guangdong Province, Southern China. The mean annual temperature of this region is 20.8°C, with the lowest in January (10.9°C) and the highest in July (28.9°C). The mean annual precipitation is c. 2,300 mm, with 80% of the precipitation occurring during the wet season (June–October). The atmospheric wet N deposition rate in Guangdong province is higher (33.1 kg N/ha/year) than the global average (c. 5.2 kg N/ha/year; Peñuelas et al., 2013), and the soil type is latosolic red clay loam. The age of the broadleaved evergreen forest in Shimentai National Nature Reserve is more than 50 years (thinned in 1965), and the stand density is approximately 818 trees/ha.
Experimental design and treatments
Both canopy (CN) and understory (UN) N addition treatments were designed with a rate of 50 kg N/ha/year. We also set a blank treatment (CK) with an N addition rate of 0 kg N/ha/year. There were four experimental blocks in this study, with each block including three randomly distributed plots (CK, CN, and UN; Figure 1A). The plots were 17 m in diameter and were separated by buffer zones at least 20 m wide. Polyvinyl-chloride boards were inserted to separate the buffer zones. Each plot occupied a 907 m2 area; of this, 400 m2 was the core sampling area, and the remaining area was designated as the buffer zone. The canopy N-addition treatments were achieved via a forest canopy spraying system (height, 35 m) built at the center of each plot. The understory N-addition system consisted of five sprinklers that were evenly distributed in each plot (height, 1.5 m). The sprinklers rotated 360° automatically at a constant speed to ensure uniform spraying (Figures 1B,C). Appropriate amounts of N were mixed to the approximate concentration of NH4NO3 in surface water obtained from an upper-position pond. Starting in 2013, the target N solution was sprinkled (rate, 3 mm precipitation per application) during April–October (seven times a year). The total amount of water sprayed was equivalent to 21 mm precipitation, accounting for less than 1% of the total rainfall of a year in the reserve. Therefore, the effects of water addition were negligible (Zhang et al., 2015; Lu et al., 2021). After 4 years of continuous N addition, we conducted our experiments during August and September of 2017.
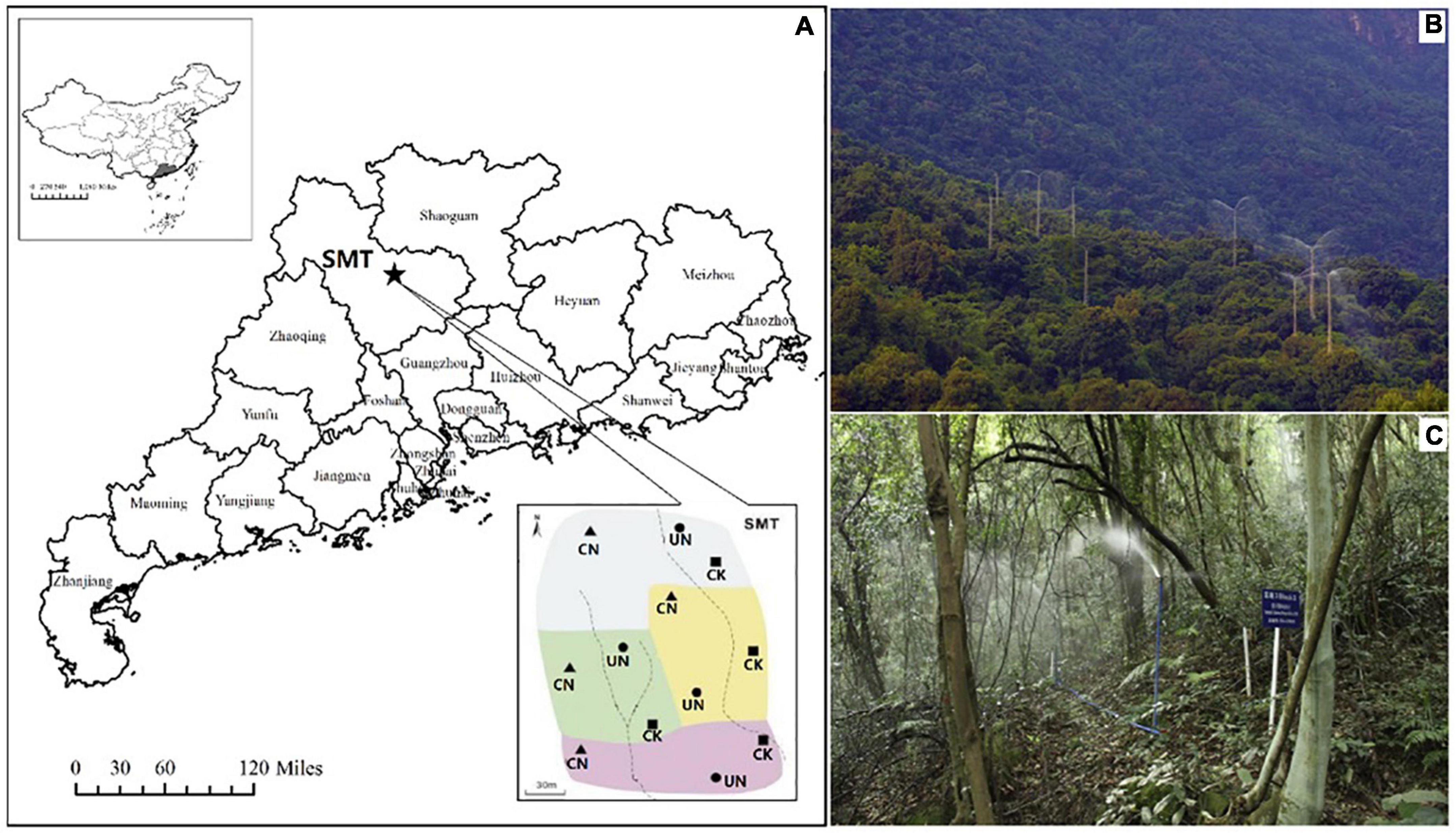
Figure 1. Geographical location and plot layout of the experiment (A) and the setups for canopy (B) and understory (C) N addition. CK, CN, and UN represent control conditions, canopy N addition, and understory N addition, respectively. Pictures of (B,C) were taken by Liu N. et al. (2020).
Four dominant species, including two canopy tree species (C. chinensis and S. superba) and two understory species (A. quinquegona and B. cochinchinensis), were selected in our study. Three replicates were installed in each plot, and a total of 12 samples were collected from each treatment group.
Measurement of gas exchange
Leaf gas exchange was measured on clear days in August and September of 2017 at 9:00–11:00 am using a portable photosynthesis system equipped with a CO2 injector (Li6800, Li-Cor, Lincoln, NE, United States). Each species was measured three times, and the photosynthetic rate was determined for five sun-exposed mature leaves from each individual plant. For understory species (A. quinquegona and B. cochinchinensis), we measured the gas exchange in plants without cutting the branches. For the canopy tree species (C. chinensis and S. superba), we cut the sun-exposed branches, placed the stem immediately in water, and then quickly measured the gas exchange. Based on preliminary trials, the photosynthetic photon flux density was set at 1,500 mol/m2/s to ensure that light-saturated photosynthetic rates were measured for all species. Ambient CO2 was maintained at 400 mol/mol, and leaf temperature was maintained at 26°C for all measurements. To avoid the impact of vapor pressure deficit on gas exchange, we also controlled the relative humidity in the chamber at 70–90% during the measurements. Before the data were recorded, the leaves were exposed to the above conditions for approximately 5–10 min to allow the photosynthetic parameters to stabilize.
Measurement of leaf hydraulic conductivity
Leaf hydraulic conductance (Kleaf) was determined using the method described by Brodribb and Holbrook (2003). Leaf-bearing canopy branches were collected at predawn and placed in a plastic black bag with moist towels for approximately 1 h to ensure that all attached leaves were at a similar water potential. The initial leaf water potential (ψleaf) of equilibrated branches was determined using a pressure chamber (PMS Instruments, Corvallis, OR, United States), and the neighboring leaves were cut under water and allowed to rehydrate for 10 s. The water potential of the rehydrated leaves was measured immediately Zhang et al. (2021). Leaf hydraulic conductance was calculated as follows:
where C is the leaf capacitance, ψ0 is the leaf water potential before rehydration, ψf is the leaf water potential after rehydration for t seconds, and t is 10 s in our study. C-values pre- and post-turgor loss were calculated from the slopes of leaf pressure–volume relationships and were expressed in absolute terms normalized by leaf area:
where RWC is the relative water content, DM is the leaf dry mass (g), LA is the leaf area (m2), WM is the mass of leaf water at 100% RWC (g), and M is the molar mass of water (g/mol).
Measurement of stomatal traits and leaf thickness
On sunny days between 9:00 and 11:00 a.m., mature and sun-exposed leaves were collected in a transparent plastic bag with a moist towel and immediately transported to the laboratory. Stomatal traits were measured using the method described by Carins Murphy et al. (2012). In brief, the cuticles were stained with diluted (c. 0.1%) aqueous toluidine blue, rinsed, mounted on microscope slides, covered with a cover slip, gently pressured with fine-point tweezers, and then immediately observed under a light microscope (YS100, Nikon, Tokyo, Japan). Stomatal density (SD) and the width (W) and length (L) of guard cells were measured using image analysis software (OPTPro 2012 4.0, Optec XTS20, Chongqing Optec Instrument Co. Ltd., China). Stomatal area (SA) was defined as guard length (L) multiplied by the total width (2W) of the two guard cells (Franks et al., 2009).
The base, mid, and tip of each leaf were cut to obtain cross-sections. The sections were photographed with an Optec upright microscope (Chongqing Optec Instrument Co. Ltd.) equipped with a digital camera. The thickness of each section was determined using a computerized image analysis system (OPTPro 2012 version 4.0; Optec Software).
Statistical analysis
Trait values were compared among the CK and N-addition treatments using nested ANOVA. To determine the mechanism of changes in photosynthetic rate under CN and UN conditions, we calculated the increments in physiological variables in N addition treatments (CN or UN) minus those in blank treatments (CK) for each species. We first analyzed the relationship between increments in photosynthetic rate (ΔAn) and the other four variables (i.e., ΔKleaf, ΔLT, ΔSA, and ΔSD) under N deposition using linear regression analysis. We then used the “varpart” function in the R package vegan v.2.5-6 (Oksanen et al., 2019) to estimate the unique contributions of ΔKleaf, ΔLT, and ΔSS (including ΔSA and ΔSD) to ΔAn. All analyses were conducted, and figures were prepared using RStudio version 4.0.3 (R Studio-Team, 2016).
Results
Response of leaf functional traits to canopy vs. understory nitrogen addition
In the two canopy tree species (C. chinensis and S. superba), the photosynthetic rate (An), leaf hydraulic conductivity (Kleaf), and stomatal area (SA) were significantly higher in CN (canopy N addition) than in CK (control). In contrast, leaf thickness (LT) was significantly lower under CN than under CK. The stomatal density (SD) of S. superba was significantly lower in CN than in CK, but the difference was non-significant in C. chinensis. In the understory species (A. quinquegona and B. cochinchinensis), there were no significant differences in An, Kleaf, SA, LT, SA, and SD between the CN and CK treatments. However, An, Kleaf, and SA tended to increase (less than 10%) under the CN treatment.
The leaf functional traits (An, Kleaf, SA, LT, SA, and SD) did not differ significantly between UN and CK in any of the four species (Figures 2, 3).
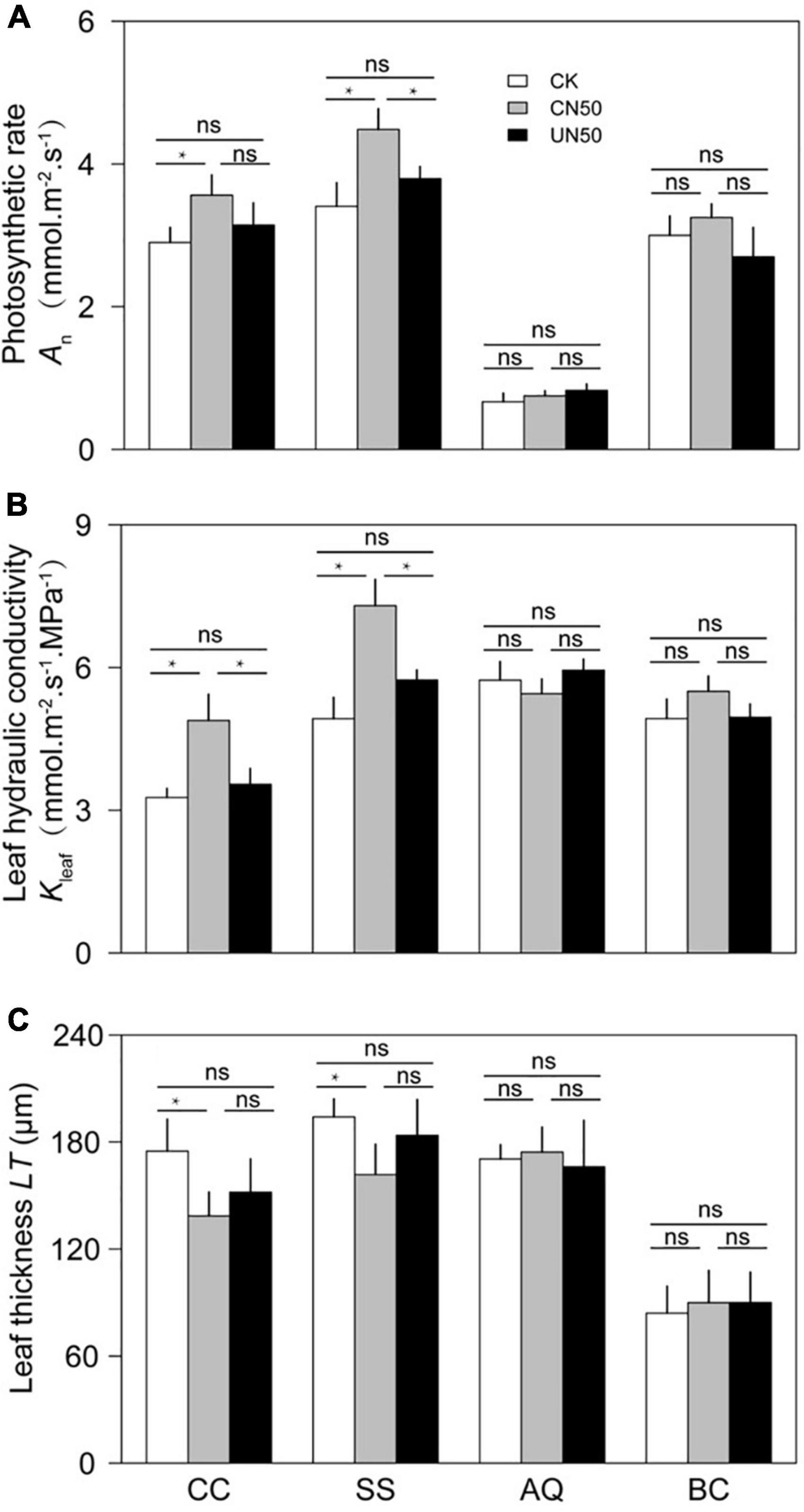
Figure 2. Photosynthetic rate (A), leaf hydraulic conductivity (B), and leaf thickness (C) of two canopy tree species (CC and SS) and two understory species (AQ and BC) under control conditions (CK), canopy N addition (CN), and understory N addition (UN). CC, SS, AQ, and BC are Castanopsis chinensis, Schima superba, Ardisia quinquegona, and Blastus cochinchinensis, respectively. ns: no significant difference; *Significant difference at P < 0.05 (nested ANOVA).
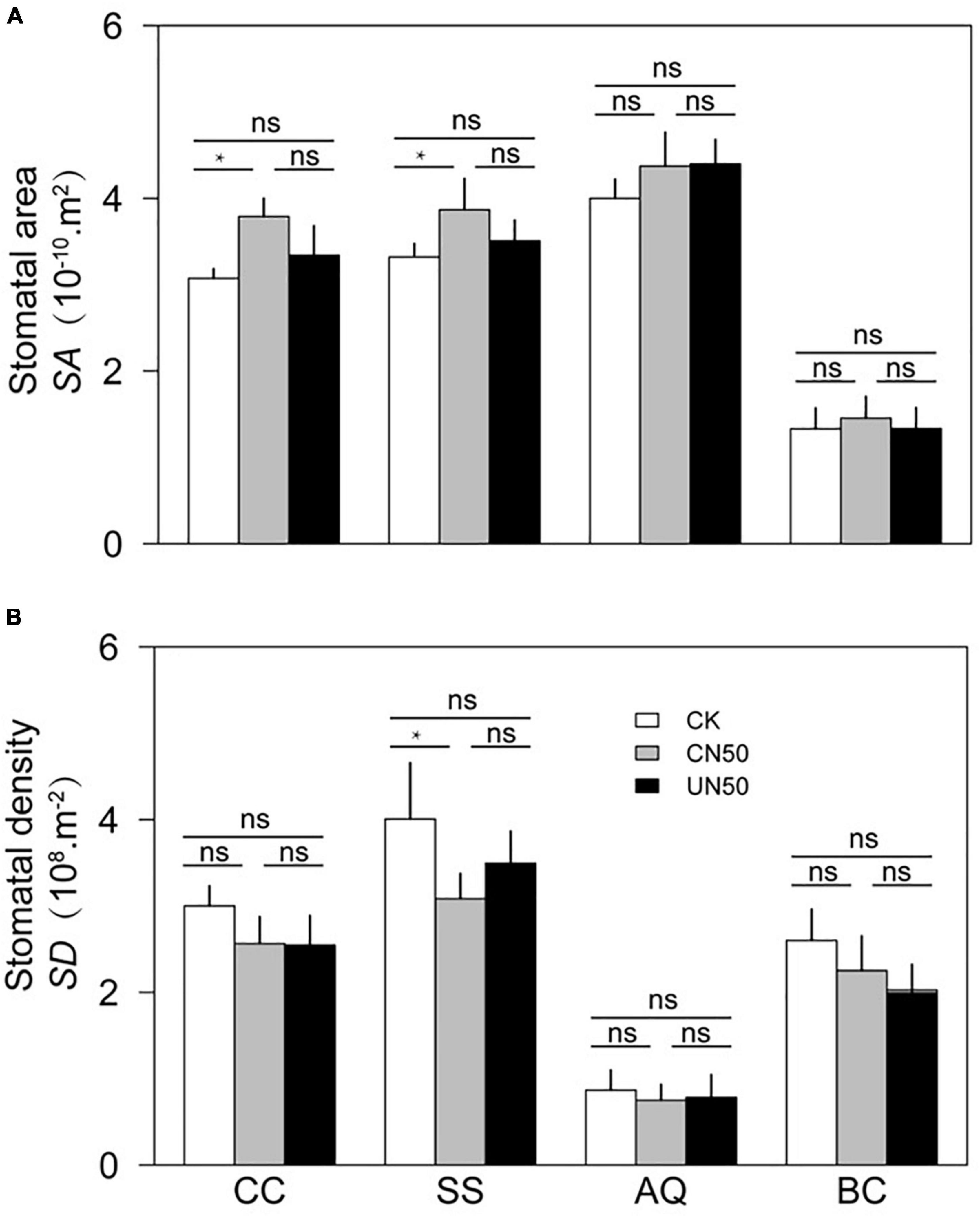
Figure 3. Stomatal area (A) and stomatal density (B) of two canopy tree species (CC and SS) and two understory species (AQ and BC) under control conditions (CK), canopy N addition (CN), and understory N addition (UN). CC, SS, AQ, and BC are Castanopsis chinensis, Schima superba, Ardisia quinquegona, and Blastus cochinchinensis, respectively. ns: no significant difference; *Significant difference at P < 0.05 (nested ANOVA).
Comparison of leaf functional traits in canopy vs. understory nitrogen addition
In canopy species, the values of leaf functional traits in UN were generally intermediate between those in CK and CN. The An of C. chinensis differed between CN and UN, but the difference was not statistically significant. However, the An of S. superba was significantly higher under CN than under UN. The Kleaf values of C. chinensis and S. superba differed significantly between CN and UN. In contrast, LT, SA, and SD were not significantly different between CN and UN for either species (Figures 2, 3). The An, Kleaf, SA, LT, SA, and SD values of the two understory species did not differ significantly between CN and UN (Figures 2, 3).
Associations between functional traits
Increments in An (ΔAn) were significantly correlated with increments in Kleaf (ΔKleaf, Figure 4A) and SA (ΔSA, Figure 4C) and decrements in LT (ΔLT, Figure 4B). However, ΔAn was not significantly associated with ΔSD (Figure 4D).
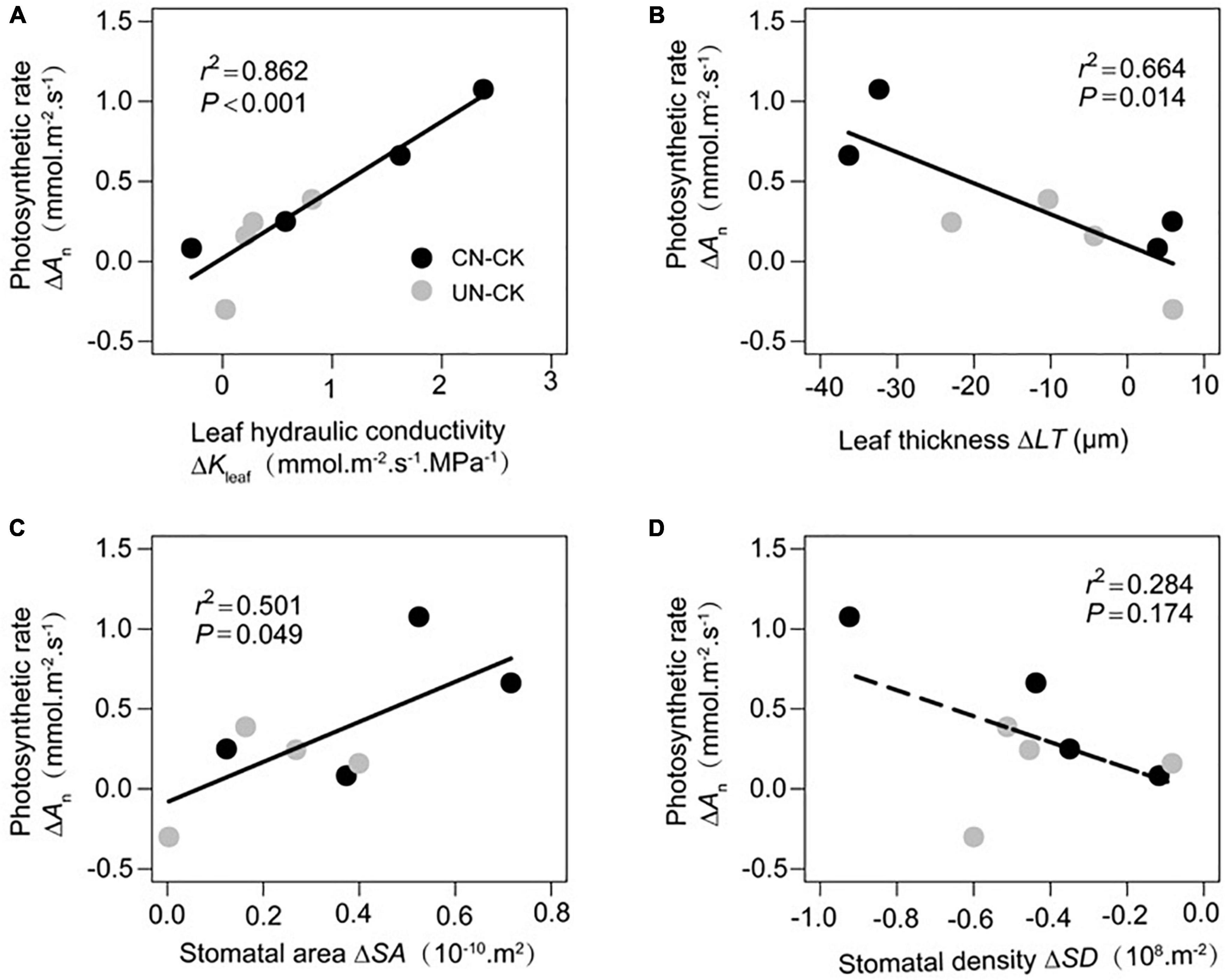
Figure 4. Relationship between increments in photosynthetic rate (ΔAn) and increments in leaf hydraulic conductivity (ΔKleaf, A), leaf thickness (ΔLT, B), stomatal area (ΔSA, C), and stomatal density (ΔSD, D). Gray and black circles represent CN minus CK and UN minus CK, respectively.
To identify which leaf functional traits contributed highly to increments in photosynthetic capacity, we estimated the individual effects of ΔKleaf, ΔLT, and ΔSS (ΔSA and ΔSD) on ΔAn. The unique effect of ΔKleaf on ΔAn was 19%, whereas those of ΔLT and ΔSS were almost negligible (<5%; Figure 5).
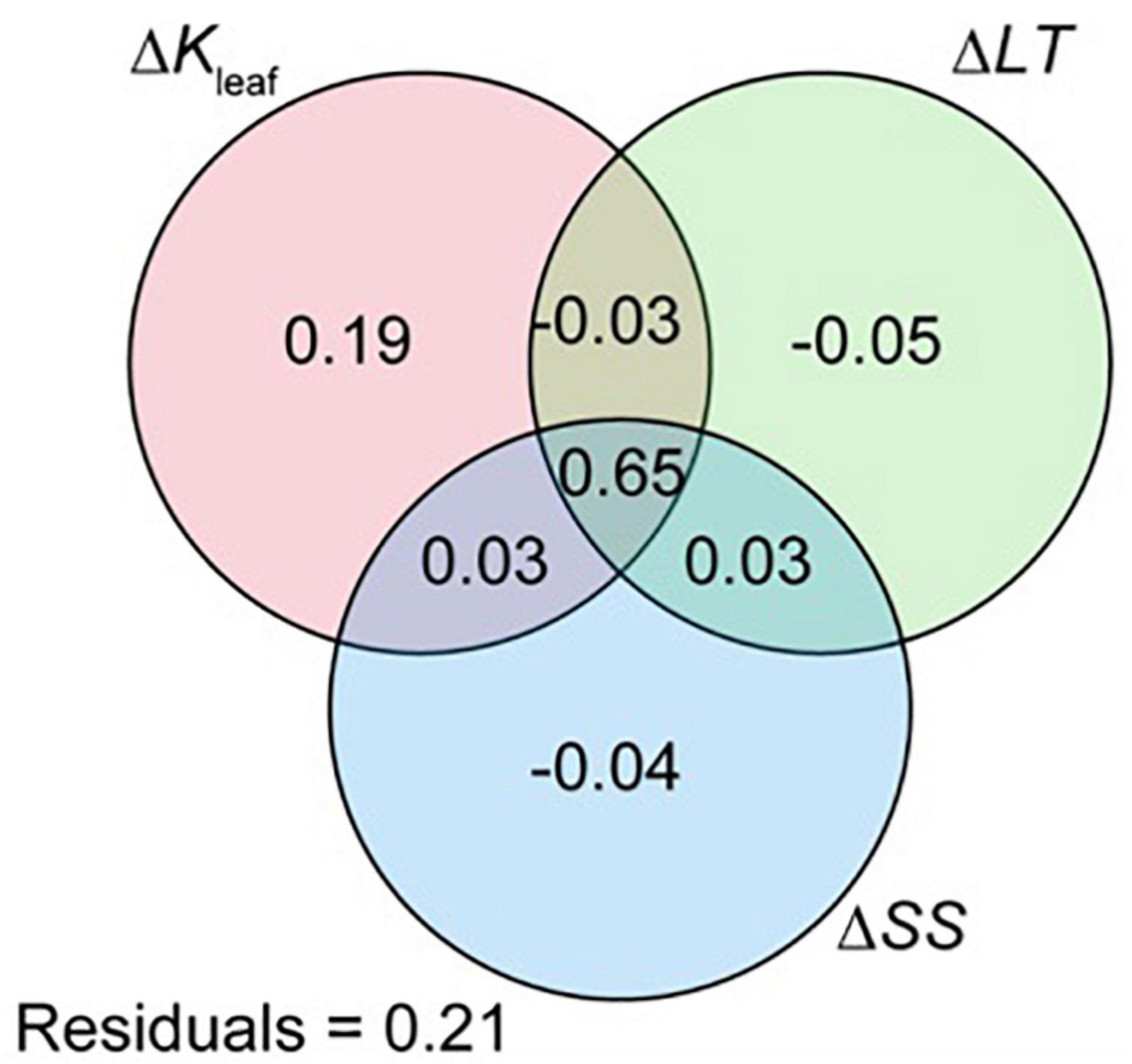
Figure 5. Venn diagram showing the results of variance partitioning on a full model of ΔAn with ΔKleaf, ΔLT, and ΔSS (including ΔSA and ΔSD) as explanatory variables. Results are shown as a percentage of explained variance. An, Kleaf, LT, SA, and SD indicate photosynthetic rate, leaf hydraulic conductivity, leaf thickness, stomatal area, and stomatal density, respectively.
Discussion
Effect of nitrogen addition approaches on plant photosynthetic rate
Nitrogen is an important nutrient for plant growth. Most previous studies have explored the adaptive strategies of plants using understory N addition, which does not account for the canopy interception process. In this study, we compared photosynthetic rates and leaf hydraulic traits between canopy and understory N addition treatments. As expected, our results revealed contrasting patterns of change in photosynthetic rate among canopy species under different N addition treatments. These findings suggest that the effects of atmospheric N deposition on growth in forest ecosystems may be incorrectly estimated using the traditional UN method. A recent meta-analysis by Liang et al. (2020) indicated that changes in photosynthetic rate in response to N deposition varied among species and depended on the N deposition rate, duration of N deposition, species type, and climatic factors. Our study fills the knowledge gap regarding the effects of different N addition approaches on the photosynthetic rates of different species.
Consistent with previous studies (Zhang et al., 2018; Liu et al., 2021; Song et al., 2022), we found that the photosynthetic rate of canopy species was enhanced under canopy N addition. This can be explained by two reasons. First, increased leaf hydraulic conductivity accelerates the photosynthetic rate because CO2 accumulation is associated with water transportation efficiency (Brodribb et al., 2007; Zhu et al., 2013). Second, a larger stomatal area and lower leaf thickness under conditions of canopy N addition can shorten the CO2 transport distance (Noblin et al., 2008; Drake et al., 2019), thereby enhancing the photosynthetic rate. Unlike canopy N addition, understory N addition did not have large effects on the leaf functional traits (including photosynthesis rate, leaf thickness, and stomatal traits) of canopy species. These discrepant responses to understory and canopy N addition may be because canopy leaves absorbed N directly, which immediately accelerated their photosynthetic rate (Chiwa et al., 2012; Wang et al., 2021). In contrast, understory N addition may have only affected the nutrient concentration in the soil. Since subtropical forests in southern China are generally N-rich (Tian et al., 2018), N addition in soil may not affect photosynthesis in canopy leaves. Furthermore, southern China experiences high precipitation, especially in summer (mean annual precipitation, 1,900 mm; 80% in summer). As such, dissolved inorganic N leaching after frequent precipitation would dilute the additional N in the soil. An alternative explanation is that soil N takes longer to be transported to the leaves, which may cause a lag between soil N addition and responses in leaf metabolism. For example, Wang et al. (2021) reported that the photosynthetic rate of aspen saplings increased after 15 days following canopy N addition and after 20 days following understory N addition. Therefore, future studies should conduct long-term and continuous monitoring of photosynthetic rates in plants under understory and canopy N addition treatments.
Somewhat unexpectedly, the photosynthetic rate of understory species did not change significantly under either canopy or understory N addition, even though both approaches can add N to the leaves of understory species. One possible explanation is that subtropical forests are usually complex and dense, and several other factors may be affecting the photosynthetic rate of understory species. For example, the light intensity may play a critical role in regulating the photosynthetic capacity of understory species. Previous studies have indicated that N addition can increase the total leaf area (Liang et al., 2020) or the ratio of distal leaf area to sapwood area (Fang et al., 2018). These changes in the leaves of canopy species would increase the level of shade on the forest floor, which would negatively affect the photosynthetic rate of understory species. This may offset the positive effects of N addition on the leaves of understory species. However, understory N addition has been shown to significantly enhance the photosynthetic capacity of an understory species (Psychotria rubra) in a subtropical forest Liu N. et al., 2020), which is inconsistent with our results. It is possible that adaptation strategies and sensitivity to N addition differ among plant species, as suggested by Liang et al. (2020). Therefore, more species should be included in future studies.
Effect of changes in H2O and CO2 transport capacity on photosynthetic rate
In all species under canopy and understory N addition treatments, increments in photosynthetic rate were significantly correlated with variations in leaf water conductivity and CO2 transport distance (ΔLT and ΔSS). This indicates that the simultaneous adjustment of H2O and CO2 transport capacity due to N addition can enhance leaf photosynthetic capacity. Most previous studies have explored the relationship between leaf hydraulic traits and photosynthetic rate (Brodribb et al., 2007; Zhang and Cao, 2009; Zhu et al., 2013) or have compared leaf hydraulic structures and anatomic traits under different environments (Wu et al., 2018; Zhang et al., 2021). Although the results suggested that hydraulic traits and C accumulation are coupled, few studies have explored the patterns of variation in these traits under changing environments. This study improves our understanding of this association by showing that variations in these traits are also coupled under nitrogen addition.
Interestingly, we found that compared with increments in CO2 transport ability, increments in leaf hydraulic conductivity had a much higher unique contribution to the acceleration of photosynthetic rate under N addition treatment in a subtropical forest. Schneider et al. (2017) reported that in Ochnaceae species, shortening the CO2 transport distance by improving stomatal anatomic traits was more economical than adjusting the water transport structure (e.g., by increasing the density of minor veins). One possible explanation for our results is that adjusting water transport-related traits is more “expensive” for plants than changing CO2 transport-related traits, leading to insufficient adjustment of leaf hydraulic conductivity. As a result, the leaf water transport capacity is not optimized under N addition, and becomes a bottleneck to increments in photosynthetic rate. We also found that the 21% change in photosynthetic rate could not be explained by changes in leaf functional traits in our study. It is possible that other traits (such as chlorophyll content) have additional effects on photosynthetic rate, as indicated in previous studies (Barroco et al., 2006; Lehmeier et al., 2017; Zeng et al., 2022). Therefore, more plant traits should be included as variables in future studies.
Conclusion
Our results indicate that canopy and understory N addition have contrasting effects on the photosynthetic rate of canopy trees in a subtropical forest in southern China. Canopy interception of N enhanced the photosynthetic rate of canopy tree species by increasing leaf hydraulic conductivity and reducing the CO2 transport distance. In contrast, the photosynthetic rates of understory species were not affected under canopy or understory N addition. We also found that compared with changes in CO2 transport distance, changes in hydraulic conductivity contributed more to enhancing the photosynthetic rate. Our results provide important insights into the contrasting effects of canopy vs. understory N addition and highlight the urgent need to consider canopy processes in future studies on N deposition.
Data availability statement
The original contributions presented in the study are included in the article/Supplementary material, further inquiries can be directed to the corresponding author.
Author contributions
DC and GW conceived the idea. GW performed the analyses and drafted the manuscript. GW, DC, ZZ, QY, and JW contributed substantially to the revisions. All authors have read and agreed to the published version of the manuscript.
Funding
This work was supported by the Central Public-Interest Scientific Institution Basal Research Fund (CAFYBB2020ZE002), the Forestry Science and Technology Innovation Project of Guangdong (2020KJCX006), the National Natural Science Foundation of China (41773071 and 31872701), and the Jianfengling National Key Field Station (2021132018).
Conflict of interest
The authors declare that the research was conducted in the absence of any commercial or financial relationships that could be construed as a potential conflict of interest.
Publisher’s note
All claims expressed in this article are solely those of the authors and do not necessarily represent those of their affiliated organizations, or those of the publisher, the editors and the reviewers. Any product that may be evaluated in this article, or claim that may be made by its manufacturer, is not guaranteed or endorsed by the publisher.
Supplementary material
The Supplementary Material for this article can be found online at: https://www.frontiersin.org/articles/10.3389/fpls.2022.942851/full#supplementary-material
References
Barroco, R. M., Peres, A., Droual, A. M., De Veylder, L., Nguyen le, S. L., De Wolf, J., et al. (2006). The cyclin-dependent kinase inhibitor Orysa; KRP1 plays and import role in seed development of rice. Plant Physiol. 142, 1053–1064. doi: 10.1104/pp.106.087056
Brodribb, T. J., and Holbrook, N. M. (2003). Stomatal closure during leaf dehydration, correlation with other leaf physiological traits. Plant Physiol. 132, 2166–2173. doi: 10.1104/pp.103.023879
Brodribb, T. J., Field, T. S., and Jordan, G. J. (2007). Leaf maximum photosynthetic rate and venation are linked by hydraulics. Plant Physiol. 144, 1890–1898. doi: 10.1104/pp.107.101352
Carins Murphy, M. R., Jordan, G. J., and Brodribb, T. J. (2012). Differential leaf expansion can enable hydraulic acclimation to sun and shade. Plant Cell Environ. 35, 1407–1418. doi: 10.1111/j.1365-3040.2012.02498.x
Chiwa, M., Matsuda, T., Nakatani, N., Kobayashi, T., Kume, A., and Sakugawa, H. (2012). Effects of canopy N uptake on foliar CO2 assimilation rates and biomass production and allocation in Japanese red pine seedlings. Can. J. Forest Res. 42, 1395–1403. doi: 10.1139/x2012-091
de Boer, H. J., Eppinga, M. B., Wassen, M. J., and Dekker, W. S. (2012). A critical transition in leaf evolution facilitated the Cretaceous angiosperm revolution. Nat. Commun. 3:1221. doi: 10.1038/ncomms2217
Drake, P. L., de Boer, H. J., Schymanski, S. J., and Veneklaas, E. J. (2019). Two sides to every leaf: water and CO2 transport in hypostomatous and amphistomatous leaves. New Phytol. 222, 1179–1187. doi: 10.1111/nph.15652
Fang, L. D., Zhao, Q., Liu, Y. Y., and Hao, G. Y. (2018). The influence of a five-year nitrogen fertilization treatment on hydraulic architecture of Pinus sylvestris var. mongolica in a water-limited plantation of NE China. Forest Ecol. Manage. 418, 15–22. doi: 10.1016/j.foreco.2017.11.059
Franks, P. J., Beerling, D. J., and Berner, R. A. (2009). Maximum leaf conductance driven by CO2 effects on stomatal size and density over geologic time. Proc. Natl. Acad. Sci. U.S.A. 106, 10343–10347. doi: 10.1073/pnas.0904209106
Gaige, E., Dail, D. B., Hollinger, D. Y., Davidson, E. A., Fernandez, I. J., Sievering, H., et al. (2007). Changes in canopy processes following whole-forest canopy nitrogen fertilization of a mature spruce-hemlock forest. Ecosystems 10, 1133–1147. doi: 10.1007/s10021-007-9081-4
Galloway, J. N., Dentener, F. J., Capone, D. G., Boyer, E. W., Howarth, R. W., Seitzinger, S. P., et al. (2004). Nitrogen cycles: past, present, and future. Biogeochemistry 70, 153–226. doi: 10.1007/s10533-004-0370-0
Galloway, J. N., Townsend, A. R., Erisman, J. W., Bekunda, M., Cai, Z., Freney, J. R., et al. (2008). Transformation of the nitrogen cycle: recent trends, questions, and potential solutions. Science 320, 889–892. doi: 10.1126/science.1136674
Lehmeier, C., Pajor, R., Lundgren, M. R., Mathers, A., Sloan, J., Bauch, M., et al. (2017). Cell density and airspace patterning in the leaf can be manipulated to increase leaf photosynthetic capacity. Plant J. 92, 981–994. doi: 10.1111/tpj.13727
Li, X., Zhang, C., Zhang, B., Wu, D., Shi, Y., Zhang, W., et al. (2021). Canopy and understory nitrogen addition have different effects on fine root dynamics in a temperate forest: implications for soil carbon storage. New Phytol. 231, 1377–1386. doi: 10.1111/nph.17460
Liang, X., Zhang, T., Lu, X., Ellsworth, D. S., BassiriRad, H., You, C., et al. (2020). Global response patterns of plant photosynthesis to addition: A meta-analysis. Global Change Biol. 26, 3585–3600. doi: 10.1111/gcb.15071
Liu, H., Wang, Z., Qi, F., Zhang, C., Fu, S., Xu, X., et al. (2021). Effects of an eight-year nitrogen and phosphorous addition on leaf photosynthesis and chemistry of mature castanopsis sclerophylla trees in subtropical China. Appl. Ecol. Env. Res. 19, 3737–3751. doi: 10.15666/aeer/1905_37373751
Liu, N., Zhang, S., Huang, Y., Wang, J., and Cai, H. (2020). Understory and canopy additions of nitrogen differentially affect carbon and nitrogen metabolism of Psychotria rubra in a evergreen broad-leaved forest. Sci. Total Environ. 724:138183. doi: 10.1016/j.scitotenv.2020.138183
Liu, T., Mao, P., Shi, L., Eisenhauer, N., Liu, S., Wang, X., et al. (2020). Forest canopy maintains the soil community composition under elevated nitrogen deposition. Soil Biol. Biochem. 143: 107733. doi: 10.1016/j.soilbio.2020.107733
Lu, X. F., Kuang, Y. W., Mou, L. Y., Hou, E. Q., Fu, S. L., and Li, J. L. (2021). Canopy mitigates the effects of nitrogen deposition on soil carbon-related processes in a subtropical forest. Sci. Total Environ. 757:143847. doi: 10.1016/j.scitotenv.2020.143847
Mao, Q., Lu, X., Wang, C., Zhou, K., and Mo, J. (2017). Responses of understory plant physiological traits to a decade of nitrogen addition in a tropical reforested ecosystem. Forest Ecol. Manage. 401, 65–74. doi: 10.1016/j.foreco.2017.06.047
Mo, J., Li, D., and Gundersen, P. (2008). Seedling growth response of two tropical tree species to nitrogen deposition in southern China. Eur. J. Forest Res. 127, 275–283. doi: 10.1007/s10342-008-0203-0
Noblin, X., Mahadevan, L., Coomaraswamy, I. A., Weitz, D. A., Holbrook, N. M., and Zwieniecki, M. A. (2008). Optimal vein density in artificial and real leaves. Proc. Natl Acad. Sci. U.S.A. 105, 9140–9144. doi: 10.1073/pnas.0709194105
Oksanen, J., Blanchet, F. G., Friendly, M., Kindt, R., Legendre, P., McGlinn, D., et al. (2019). vegan: Community ecology package. R package version 2.5-6.
Peñuelas, J., Poulter, B., Sardans, J., Ciais, P., Velde, M., Bopp, L., et al. (2013). Human-induced nitrogen-phosphorus imbalances alter natural and managed ecosystems across the globe. Nat. Commun. 4:2934. doi: 10.1038/ncomms3934
Schneider, J. V., Habersetzer, J., Rabenstein, R., Wesenberg, J., Wesche, K., and Zizka, G. (2017). Water supply and demand remain coordinated during breakdown of the global scaling relationship between leaf size and major vein density. New Phytol. 214, 473–486. doi: 10.1111/nph.14382
Sievering, H., Tomaszewski, T., and Torizzo, J. (2007). Canopy uptake of atmospheric N deposition at a conifer forest: part I- canopy N budget, photosynthetic efficiency and net ecosystem exchange. Tellus 59, 483–492.
Simkin, S. M., Allen, E. B., Bowman, W. D., Clark, C. M., Belnap, J., Brooks, M. L., et al. (2016). Conditional vulnerability of plant diversity to atmospheric nitrogen deposition across the United States. Proc. Natl. Acad. Sci. U.S.A. 113, 4086–4091. doi: 10.1073/pnas.1515241113
Song, W., Loik, M. E., Cui, H., Fan, M., and Sun, W. (2022). Effects of nitrogen addition on leaf photosynthesis and water use efficiency of the dominant species Leymus chinensis (Trin.) tzvelev in a semi-arid meadow steppe. Plant Growth Regul. [Epub ahead of print]. doi: 10.1007/s10725-022-00835-8
Swaine, M. D. (1996). Rainfall and soil fertility as factors limiting forest species distributions in Ghana. J. Ecol. 84, 419–428.
Tian, D. T., Du, E. Z., Jiang, L., Ma, S. H., Zeng, W. J., Zou, A. L., et al. (2018). Response of forest ecosystems to increasing N deposition in China: A critical review. Environ. Pollut. 243, 75–86. doi: 10.1016/j.envpol.2018.08.010
Vitousek, P. M., Aber, J. D., Howarth, R. W., Likens, G. E., Matson, P. A., Schindler, D. W., et al. (1997). Human alteration of the global nitrogen cycle: Sources and consequences. Ecol. Appl. 7, 737–750.
Wang, X., Wang, B., Wang, C. Z., Wang, Z. H., Li, J., Jia, Z., et al. (2021). Canopy processing of N deposition increases short-term leaf N uptake and photosynthesis, but not long-term N retention for aspen seedlings. New Phytol. 229, 2601–2610. doi: 10.1111/nph.17041
Wu, G., Liu, H., Hua, L., Luo, Q., Lin, Y., He, P., et al. (2018). Differential responses of stomata and photosynthesis to elevated temperature in two co-occurring subtropical forest tree species. Front. Plant Sci. 9:467. doi: 10.3389/fpls2018.00467
Zalamea, P., Turner, B. L., Winter, K., Jones, F. A., Sarmiento, C., and Dalling, J. W. (2016). Seedling growth responses to phosphorus reflect adult distribution patterns of tropical trees. New Phytol. 212, 400–408. doi: 10.1111/nph.14045
Zeng, F., Zhu, L., Wang, G., Liang, Y., Ma, D., and Wang, J. (2022). Higher CO2 assimilation in selected rice recombinant inbred lines is driven by higher CO2 diffusion and light use efficiency related to leaf anatomy and mesophyll cell density. Front. Plant Sci. 13:915050. doi: 10.3389/fpls.2022.915.5050
Zhang, H., Li, W., Adams, H. D., Wang, A., Wu, J., Jin, C., et al. (2018). Response of woody plant functional traits to nitrogen addition: A meta-analysis of leaf economics, gas exchange, and hydraulic traits. Front. Plant Sci. 9:683. doi: 10.3389/fpls.2018.00683
Zhang, J. L., and Cao, K. F. (2009). Stem hydraulics mediates leaf water status, carbon gain, nutrient use efficiencies and plant growth rates across dipterocarp species. Funct. Ecol. 23, 658–667. doi: 10.1111/j.1365-2435.2009.01552.x
Zhang, T., Liang, X., Ye, Q., BassiriRad, H., Liu, H., He, P., et al. (2021). Leaf hydraulic acclimation to nitrogen addition of two dominant tree species in a subtropical forest. Sci. Total Environ. 771:145415. doi: 10.1016/j.scitotenv.2021.145415
Zhang, W., Shen, W., Zhu, S., Wan, S., Luo, Y., Yan, J., et al. (2015). CAN canopy addition of nitrogen better illustrate the effect of atmospheric nitrogen deposition on forest ecosystem? Sci. Rep. 5:11245. doi: 10.1038/srep11245
Keywords: canopy N addition, photosynthetic rate, leaf hydraulic conductivity, CO2 transportation distance, understory N addition
Citation: Wu G, Chen D, Zhou Z, Ye Q and Wu J (2022) Canopy nitrogen addition enhance the photosynthetic rate of canopy species by improving leaf hydraulic conductivity in a subtropical forest. Front. Plant Sci. 13:942851. doi: 10.3389/fpls.2022.942851
Received: 13 May 2022; Accepted: 13 July 2022;
Published: 05 August 2022.
Edited by:
Yanbo Hu, Northeast Forestry University, ChinaReviewed by:
Yunting Fang, Institute of Applied Ecology (CAS), ChinaGuoyong Yan, Qufu Normal University, China
Dong Wang, Henan University, China
Copyright © 2022 Wu, Chen, Zhou, Ye and Wu. This is an open-access article distributed under the terms of the Creative Commons Attribution License (CC BY). The use, distribution or reproduction in other forums is permitted, provided the original author(s) and the copyright owner(s) are credited and that the original publication in this journal is cited, in accordance with accepted academic practice. No use, distribution or reproduction is permitted which does not comply with these terms.
*Correspondence: Dexiang Chen, ZGNoZW5AY2FmLmFjLmNu