- 1Crop Health, Faculty of Agricultural and Environmental Sciences, University of Rostock, Rostock, Germany
- 2Department Technology Assessment and Substance Cycles, Leibniz Institute for Agricultural Engineering and Bioeconomy (ATB), Potsdam, Germany
The use of wildflower species as biogas feedstock carries the risk that their seeds survive anaerobic digestion (AD) and cause weed problems if spread with the digestate. Risk factors for seed survival in AD include low temperature, short exposure and hardseededness (HS). However, it is not possible to predict how AD will affect seed viability of previously unstudied species. In laboratory-scale reactors, we exposed seeds of eight species from a mixture of flowering wild plants intended as biogas feedstock and three reference species to AD at two mesophilic temperatures. Half of the species were HS, the other was non-HS (NHS). Viability was determined using a combination of tetrazolium and germination tests. Viability and germinability were modeled as functions of exposure time using a dose-response approach. Responses to AD varied considerably among species, and none of the considered influencing factors (time, temperature, HS) had a consistent effect. Seed lots of a species differed in inactivation times and seed-killing efficacy. The HS species Melilotus officinalis, Melilotus albus, and Malva sylvestris were particularly AD-resistant. They were the only ones that exhibited biphasic viability curves and tended to survive and germinate more at 42°C than at 35°C. Viability of the remaining species declined in a sigmoidal curve. Most NHS species were inactivated within a few days (Cichorium intybus, Daucus carota, Echium vulgare, and Verbascum thapsus), while HS species survived longer (Malva alcea). AD stimulated germination in the HS species A. theophrasti and its AD-resistance overlapped with that of the most resistant NHS species, C. album and tomato. In all seed lots, germinability was lost faster than viability, implying that mainly dormant seeds survived. After the maximum exposure time of 36 days, seeds of HS species and Chenopodium album were still viable. We concluded that viability responses to mesophilic AD were determined by the interplay of AD-conditions and species- and seed-lot-specific traits, of which HS was an important but only one factor. For the use of wildflowers as biogas feedstock, we recommended long retention times and special care with regard to HS species.
Introduction
Anaerobic digestion (AD) of renewable feedstocks in biogas plants is considered one of the most environmentally friendly and energy efficient bioenergy sources. A particular advantage is that the semi-solid leftover of biogas production, the digestate, which is produced in addition to the energy carrier methane, can be used as plant fertilizer, thus tightening nutrient cycles (Weiland, 2010; Guo et al., 2015; Salnikova et al., 2019). AD feedstocks have included energy crops such as maize (Zea mays L.), triticale (× Triticosecale) or beets (Beta vulgaris L.) for decades (e.g., Venendaal et al., 1997; Herrmann et al., 2016; Hofmann et al., 2017). The sustainability of using energy and agrofuel crops, however, has become increasingly controversial (e.g., Altieri, 2009; Eggers et al., 2009; Meyer-Aurich et al., 2012; Gasparatos et al., 2013). In response, there are now calls for biomass production systems to be multifunctional and adapted to local conditions (von Cossel et al., 2019b; Englund et al., 2020b). For this reason, among others, the portfolio of energy crops continues to expand (e.g., Papamatthaiakis et al., 2021). The focus is on perennial species that offer a variety of ecological benefits at low input (Don et al., 2012; Müller-Stöver et al., 2016; Emmerling et al., 2017; Hofmann et al., 2017; Jones, 2017; Englund et al., 2020a). Probably the most diverse option at present is the cultivation of perennial species mixtures (e.g., von Cossel and Lewandowski, 2016; Carlsson et al., 2017; Weißhuhn et al., 2017; Yang et al., 2018).
Since 2008, mixtures of flowering wild plant species were introduced in Germany to supplement silage maize as a biogas feedstock (Vollrath, 2012; Vollrath et al., 2016; von Cossel and Lewandowski, 2016). These flowering mixtures are of interest mainly because they significantly improve ecosystem services such as habitat functioning, soil protection and landscape aesthetics (von Cossel, 2020; Janusch et al., 2021), while their methane yield is rather low (von Cossel et al., 2019a,2021; Lask et al., 2002). However, a sustainability issue rarely considered is that wildflower mixtures and other energy crops can spread undesirably into new habitats. When used as a biogas feedstock, propagules such as seeds that survive the biochemical processes during AD enter the digestate. There is a risk that these establish as weeds on fields fertilized with this digestate. Of course, seed persistence in soil is only one of many criteria for weediness (Baker, 1974), however, according to Harper (1977) it is important for the success of plants in farmed fields. The weed control measures required upon seed survival would compromise sustainability and cause undesirable, additional costs and labor. Non-native or quarantine species not yet widespread are particularly problematic in this context (Raghu et al., 2006; Simberloff, 2008; Westerman and Gerowitt, 2013). In this regard, the biogas wildflower mixtures should be evaluated with care, as they contain various poorly cultivated (wild) species. However, whether seeds of species from biogas wildflower mixtures survive AD has not yet been the subject of investigations. In general, studies on seed susceptibility to AD are scarce and systematic studies on the ability of seeds from different taxonomic and functional groups to survive AD are lacking (Westerman and Gerowitt, 2013). Thus, reliable predictions of seed viability of previously unstudied plant species in AD are not possible.
Most available studies on seed survival in AD dealt with weeds (e.g., Jeyanayagam and Collins, 1984; Šarapatka et al., 1993; Schrade et al., 2003; Eckford et al., 2012; Westerman et al., 2012a; Johansen et al., 2013; Zhou et al., 2020). Of the plants whose biomass is (intended to be) used as biogas feedstock, only 14 species have been studied to date (Heiermann et al., 2010; Strauß et al., 2012; van Meerbeek et al., 2015; Baute et al., 2016; Sölter et al., 2016; Starfinger and Sölter, 2016; Hassani et al., 2021). Based on weeds studied through 2012, Westerman and Gerowitt (2013) identified plant groups whose seeds might have a higher probability of surviving AD than usual. They comprised species that are either hardseeded (HS), i.e., form physically dormant seeds with one or more impermeable layers in the seed or fruit coat (Baskin et al., 2000), and species adapted to dispersal by endozoochory, e.g., by thick seed coats. HS is common in the Fabaceae and occurs in members of the Malvaceae (Baskin et al., 2000), both of which are families of interest for biogas flowering mixtures (Vollrath, 2012). HS as a risk factor for high AD-resistance potential and consequently for seed dispersal with the digestate has been explicitly mentioned by Leonhardt et al. (2010), Westerman et al. (2012a,b), and Hassani et al. (2021). However, not all species resistant to AD are HS, so it is suspected that other seed traits may aid seed survival in AD as well (Westerman and Gerowitt, 2013).
In addition to characteristics of the seeds themselves, temperature and exposure time were found to be the most important factors driving seed inactivation in AD. In general, seed viability decreases exponentially with time, with the seeds remaining unaffected by AD during an initial lag-phase (Westerman and Gerowitt, 2013). In addition, higher temperatures result in a greater decrease in seed viability (reviewed by Westerman and Gerowitt (2013) and confirmed by Johansen et al. (2013); Oechsner et al. (2018), and Zhou et al. (2020). In particular, ADs under thermophilic conditions (approx. 45–55°C) appear to be significantly more effective in killing seeds than mesophilic ones (approx. 30–45°C) (Šarapatka et al., 1993; Lorenz et al., 2001; Schrade et al., 2003; Westerik and Kleizen, 2006; Leonhardt et al., 2010; Johansen et al., 2013; Zhou et al., 2020). This implies that ADs in the mesophilic temperature range pose a higher risk of unintended seed spread – as pointed out by Westerman and Gerowitt (2013); Alsanius et al. (2021), and Hassani et al. (2021). With regard to the use of wildflower species as biogas feedstock, this could be problematic, as they are to be grown mainly in Germany, where 84% of biogas plants are mesophilic (vTI, 2009).
Finally, existing studies differ in which seeds they consider viable. Many studies on the effects of AD on seed viability have based their conclusions solely on germination tests (Engeli et al., 1993; Lorenz et al., 2001; Ryckeboer et al., 2002; Schrade et al., 2003; Marcinisyn et al., 2004; Westerik and Kleizen, 2006; Strauß et al., 2012; Johansen et al., 2013; Milotić and Hoffmann, 2016; Oechsner et al., 2018; Zhou et al., 2020). In doing so, they did not consider that dormant seeds may have survived and could germinate once dormancy is broken. However, HS species and wild plant species in general can exhibit different classes, levels, and types of dormancy (Baskin and Baskin, 1998, 2004). Therefore, to determine the actual risk of spreading viable seed with the digestate, the (total) viability must be determined as the sum of germinable and dormant seeds. This procedure has only been used in some studies on seed survival in AD (Jeyanayagam and Collins, 1984; Eckford et al., 2012; Westerman et al., 2012a,b; Baute et al., 2016).
The objective of this study was to evaluate the effects of mesophilic AD on seed viability of wildflower species intended as biogas feedstocks. In addition, three species that have already been investigated in similar studies were included as references. The focus was on the impact of AD-process control parameters on seed viability of hardseeded (HS) or non-hardseeded (NHS) species. Seed viability was explored as a function of exposure time in AD at two mesophilic temperatures. We hypothesized that in AD, (1) seed viability of species with HS would be reduced less than that of NHS species, (2) seed viability would decrease more at higher incubation temperatures, and (3) seed viability would decrease with increasing exposure time. In addition, for both HS and NHS species, we examined whether seeds that survived AD were germinable or dormant. Finally, we discussed the implications of the results with respect to the use of wildflower species as biogas feedstocks.
Materials and Methods
Plant Species
Species Selection
Seed vitality after mesophilic anaerobic digestion (AD) was studied in eleven different species. Five of the species were hardseeded (HS) the others not (NHS). The majority of species were selected from a wildflower mixture that has been specifically designed for biogas production (‘‘BG70’’ by Saaten Zeller GmbH & Co. KG, Eichenbühl-Guggenberg, Germany).1 From this mixture, Malva alcea L. (rose mallow, Malvaceae), Malva sylvestris L. (common mallow, Malvaceae), Melilotus albus MEDIK. (white sweet clover, Fabaceae) and Melilotus officinalis (L.) PALL. (yellow sweet clover, Fabaceae) were selected to represent HS species. NHS representatives were Cichorium intybus L. (Blue dandelion, Asteraceae), Daucus carota L. (wild carrot, Apiaceae), Echium vulgare L. (viper’s bugloss, Boraginaceae) and Verbascum thapsus L. (great mullein, Scrophulariaceae). In selecting NHS species, emphasis was placed on ensuring that they were from diverse families whose response to mesophilic AD has been poorly investigated.
In addition to the eight flowering species from the biogas mixture, this study included one HS and one NHS weed species that were found to be relatively resistant to mesophilic AD. Abutilon theophrasti MEDIK. (Malvaceae, velvetleaf) is a HS species whose seeds that survived AD with relatively high probability (Katovich et al., 2004; Westerman et al., 2012a,b). The NHS species Chenopodium album L. (Amaranthaceae, common lambsquarters) and tomato (Lycopersicon esculentum Mill., Solanaceae) were among the best surviving NHS species in several AD-treatments (Engeli et al., 1993; Šarapatka et al., 1993; Lorenz et al., 2001; Schrade et al., 2003; Katovich et al., 2004; Westerik and Kleizen, 2006; Leonhardt et al., 2010; Strauß et al., 2012; Westerman et al., 2012a,b; Johansen et al., 2013; Baute et al., 2016; Zhou et al., 2020).
Seed Lots, Seed Acquisition, and Storage
The following species were tested using only one seed lot: D. carota, E. vulgare, and M. sylvestris that were propagated in 2015 and obtained from ‘‘Herbiseed’’ (Twyford, United Kingdom, herbiseed.com). Seeds of C. intybus, M. albus, M. officinalis and V. thapsus were propagated in 2014 by ‘‘Appels Wilde Samen GmbH’’ (Darmstadt, Germany).2 Seeds of C. album were harvested in 2014 from C. album plants grown at the University of Rostock (Germany).
Two seed lots each were examined of M. alcea, A. theophrasti and tomato because one lot ran out during the course of the experiments, so a second lot was needed to obtain results for each species in all AD-treatments. Seeds of ‘‘M. alcea -- 2 years’’ and ‘‘M. alcea -- 1 year’’ were ordered from ‘‘Appels Wilde Samen’’ (see above) and were propagated in 2014 and 2015, respectively. The seed lot ‘‘A. theophrasti -- 7 years’’ was propagated and collected in 2008 in a sunflower field in Vilanova de Bellpuig, Lleida (Spain, collector PW). In 2015, the younger lot ‘‘A. theophrasti -- 1 year’’ was propagated from seeds of ‘‘A. theophrasti -- 7 years’’ in a greenhouse at the University of Rostock (Germany). The seed lots of tomato came from two different varieties: ‘‘paprikaförmige’’ [‘tomato -- PAPRIKA’, propagated in 2014, ‘‘Culinaris -- Saatgut für Lebensmittel’’ (Göttingen, Germany)]3 and ‘‘St. Pierre’’ [‘tomato -- PIERRE,’ propagated in 2015, ‘‘Bingenheimer Saatgut AG’’ (Echzell, Germany)]4.
Until the beginning of and during the experiments in 2015, seeds were stored at room temperature in the dark. The seeds of “A. theophrasti – 7 years” harvested in 2008 had previously been stored at 7°C.
Anaerobic Digestion of Seeds
Lab-Scale Reactors
From March 2015 until September 2016 seeds were exposed to mesophilic AD in eight lab-scale continuously stirred biogas reactors at the ATB in Potsdam (Germany) (Figure 1). The reactors were run according to German Standard Procedure VDI 4630 (VDI-Fachbereich Energietechnik, 2006). They had a working volume of 8 l each and were operated at a constant organic loading rate of 3 gVS l–1 d–1 fed with maize silage and cattle slurry. Half of the reactors were run at 35°C, while the other half was run at 42°C, representing the lower and upper mesophilic temperature range of agricultural biogas plants (Weiland, 2010). In order to check the stability of the anaerobic digestion process the biogas produced was continuously measured with a milligascounter type TGC1/5 (RITTER, Bochum, Germany). Biogas volume measured was normalized to standard conditions: dry gas, t0 = 273 K, p0 = 1,013 hPa. The biogas composition was determined online using a gas analyzer SSM 6000 (PRONOVA, Berlin, Germany). To evaluate the stability of the biological process, samples of process liquid of each reactor were taken once a week and analyzed for total solids, volatile solids, ammonium nitrogen, total nitrogen, volatile fatty acids and pH (Terboven et al., 2017; Supplementary Data Sheet 1).
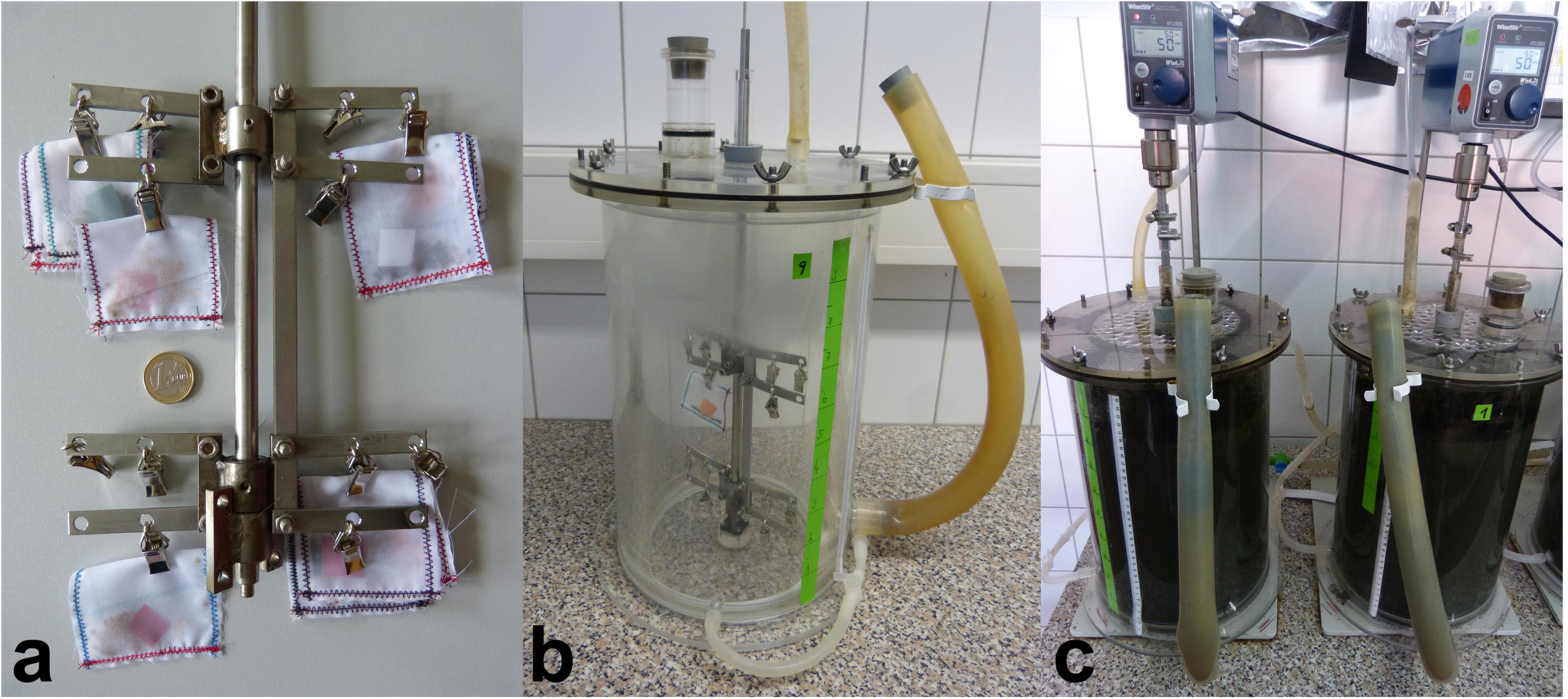
Figure 1. Experimental setup for anaerobic digestion of seeds in lab-scale reactors. (a) Central paddle stirrer with attached fine mesh polyester bags enclosing seeds and identification markers. (b) Lab-scale reactor consisting of a double-walled cylinder for temperature control via a water jacket, a gas-tight lid with tubes for feeding, gas collection, and attachment of the stirrer, and a separate outlet for the digestate at the bottom. (c) Running reactors connected to thermostat, stirrer drive, gas meter and gas analyzer.
Continuous biogas production was monitored at both temperatures during seed exposure experiments. The average biogas production in the twelve reactors was 13.1 ± 2.61 lN d–1 and the methane content was 57 ± 2% (data not shown). The actively proceeding biomethanation process was not disturbed by insertion and removal of the seeds (Supplementary Data Sheet 1).
Exposure of Seeds to Anaerobic Digestion
Seeds were placed in fine-mesh polyester bags attached to the reactors’ stirrer for exposure to AD and subsequent removal (Figure 1). Seeds were sampled after four different exposure times, and the duration of exposure differed between HS and NHS species. The seeds of NHS species were exposed to AD for 1, 3, 6, and 9 days at both 35 and 42°C. The species C. album and tomato were additionally exposed to 35°C for 18 and 36 days. Seeds of the HS species were exposed to AD at both temperatures for 3, 9, 18, and 36 days (Supplementary Table 2). However, since the seeds of A. theophrasti – 1 year and M. alcea – 1 year were harvested after the experiments with exposure times up to 36 days at 35°C had already started, they were exposed to AD at 35°C for only 1, 3, 6, and 9 days (Supplementary Table 2).
For most species, four replicates were taken at each combination of exposure time and temperature. As seed survival is known to decrease with increasing exposure time (Westerman and Gerowitt, 2013), the number of seeds was increased with exposure time in order to optimize the discriminative power of the assay: Depending on the exposure time, 100, 200, or 300 seeds of a species were anaerobically digested per replicate (Supplementary Table 2).
Determination of Seed Viability
After AD, seeds were rinsed with water, transported to the laboratory in Rostock and processed within 5 h after removal from the reactor. Seed viability was determined by testing the germination and metabolic activity of all seeds that did not germinate within 21 days.
In detail, surface sterilized seeds were incubated on plates with “diaspore agar” (agar 13.0 g l–1, KNO3 2.0 g l–1, gibberellic acid 0.5 g l–1, ampicillin 0.1 g l–1, streptomycin 0.1 g l–1, benzimidazole 0.02 g l–1) at 20/4°C day/night temperatures with a 16 h photoperiod. The number of germinated seeds on the plates was recorded at regular intervals during 21 days. A seed was considered germinated if the radical protruded at least 2 mm from the seed. The viability of all remaining non-germinated seeds was tested using 2,3,5-triphenyltetrazolium chloride (TTC) that indirectly determines the metabolic activity and thus viability of seed tissue cells (França-Neto and Krzyzanowski, 2019). To enable the TTC molecules to enter the seed, seed coats were carefully punctured with a needle or scalpel without inflicting injuries to the embryo (Elias et al., 2012). The punctured seeds were placed between two filter papers, soaked with 3 ml of 1.0% TTC solution and incubated in the dark at 35°C for 20–22 h. Based on the guidelines in the Tetrazolium Testing Handbook (Association of Official Seed Analysts, 2010) seeds were judged fully viable but dormant if the embryo - and endosperm, if relevant - was stained red. Embryos that were not stained (white), poorly stained (light pink), or lacked staining in areas critical for normal seedling development were classified as non-viable. Likewise, seeds whose embryo had rotted or which had already been degraded in the reactor and therefore could not be recovered (lost) also fell into the non-viable category. Ultimately, this test procedure provided the number of seeds that (1) germinated during the 21-day germination test after AD-treatment, (2) remained dormant but metabolically active, and (3) were not viable.
The same procedure was used to determine the germination and viability of control seeds that had not been exposed to AD (minimum of three replicates of 300 seeds per species, Supplementary Table 2). In preparation for the germination tests, the previously dry stored control seeds were exposed to a water-saturated atmosphere for 2 days in the dark.
Statistical Analyses
Seed Viability Models
Both germinated and dormant seeds were viable after the different exposure times in mesophilic AD. For each replicate, the proportion of viable seeds, V, was calculated by dividing the cumulative number of germinated seeds after 21 in the germination test plus the number of dormant seeds by the total number of evaluated seeds (eq. 1).
With V, proportion of viable seeds observed in a replicate after a certain exposure time to AD; Σgerminated seeds, cumulative number of germinated seeds from the 21-day germination test; Σdormant seeds, number of seeds viable in tetrazolium testing after the germination test; Σtotal numberofevaluatedseed, total number of evaluated seeds in the replicate.
All statistical analyses were carried out using the software environment R (version 4.1.2) (R Core Team, 2021). Seed viability as a function of exposure time, V(t), was modeled with a dose-response approach using the R-package “drc” (version 3.0.1, Ritz et al., 2015). The response variables, V or V(t), mean the “observed V” of the samples or its values modeled over time. Log-logistic models with a lower limit of zero were fitted to the observed proportions of viable seeds (eq. 2, Supplementary Figure 2A). Models were fitted species-wise for both temperatures simultaneously by setting temperature as a grouping variable. The 35°C- and 42°C-models shared the upper horizontal asymptote, i.e., the maximum proportion of viable seeds, Vmax. The data type was “binomial” and the total number of evaluated seeds was set as weights. The model fit was evaluated both by a Chi2-test and visually (Supplementary Table 3). In case all or almost all seeds had lost viability even after the shortest exposure time to AD (1 day or 3 days), no model was fitted.
With V(t), proportion of viable seeds as a function of the time of exposure in AD (t); Vmax, maximum proportion of viable seeds (upper asymptote); SLP, parameter proportional to the slope of V(t) in the inflection point; MIT (median inactivation time), the time after which V(t) reaches 50% of Vmax.
Due to viability increases in AD, the log-logistic models did not provide a good fit for M. sylvestris, M. albus, and M. officinalis. To improve their fit, one parameter was added to the log-logistic models using the Brain-Cousens modification (Ritz and Streibig, 2016, eq. 3). In these models, the lag-phase at the beginning of exposure is replaced by a sigmoid curve describing hormesis, and thus, turning a monotonically decreasing dose-response relationship into a biphasic one (Cedergreen et al., 2005; Kendig et al., 2010; Supplementary Figure 2B).
With V(t), proportion of viable seeds as a function of the time of exposure in AD (t); Vmax, maximum proportion of viable seeds (upper asymptote); H, size of the hormesis effect, i.e., stimulation of viability at t close to zero; SLP, parameter changing the slope of the model curve; E, parameter shifting and stretching the model curve.
From the viability models the median inactivation times (MITs) and decimal reduction times (DRTs) were estimated, i.e., the times required to reduce viability to 50 or 10% of the initial viability, respectively (Supplementary Figure 2). If models could be fitted to the data obtained for both 35 and 42°C, parameter estimates, MIT and DRT were compared between the two temperatures species-wise using the “drc”-built-in functions compParm and EDcomp (Ritz and Streibig, 2016). The level of significance, α, was set to 0.05.
Seed-Killing Efficacy
To assess how much AD reduced seed viability, the viability models were used to estimate the mean V and standard error after 36 days for AD at both 35 and 42°C. Based on these data and the approach of Hahn et al. (2021), the seed-killing efficacies of 36 days in AD were calculated as follows (eq. 4):
Cumulative Germination and Dormant Seeds
In addition to total viability, its components, i.e., the proportions of germinated and dormant seeds, were analyzed individually. Cumulative germination after the 21-day germination test, cG, was modeled as a function of exposure time, t, similar to V(t). Due to the skewness of the data, however, an asymmetric, three-parameter Weibull type 1 function with a lower limit of zero was chosen for most seed lots (eq. 5). The only exception to this was A. theophrasti – 7 years for which a log-logistic model including hormesis was used (compare eq. 3). The model fit was evaluated by a Chi2-test and visually (Supplementary Table 4).
From the germination models decimal reduction times for cG (DRT(cG)) were estimated and compared to DRT(V) for 35°C and 42°C. In addition, the proportion of dormant seeds, D, was calculated from the difference between the models for V(t) and cG(t). Finally, the percentages of germinated, dormant, and inactive seeds were calculated in the untreated controls and after 36 days in AD at 35°C and 42°C, respectively.
With cG(t), proportion of cumulative germination at the end of the 21 days germination test, cG, as a function of the time of exposure in AD (t); cGmax, maximum proportion of cG (upper asymptote); SLP, parameter proportional to the slope of cG(t) in the inflection point; IFT (inflection time): the time after which the curve of cG(t) changes its flection.
Results
Seed Viability
Responses During Anaerobic Digestion
Viability responses to mesophilic AD varied among species and among seed lots of a species (Figure 2). The species most resistant to mesophilic AD were M. officinalis, M. albus, and M. sylvestris (Figures 2a–c and Table 1). The species whose seeds were inactivated most rapidly was V. thapsus (Figure 2n and Table 1).
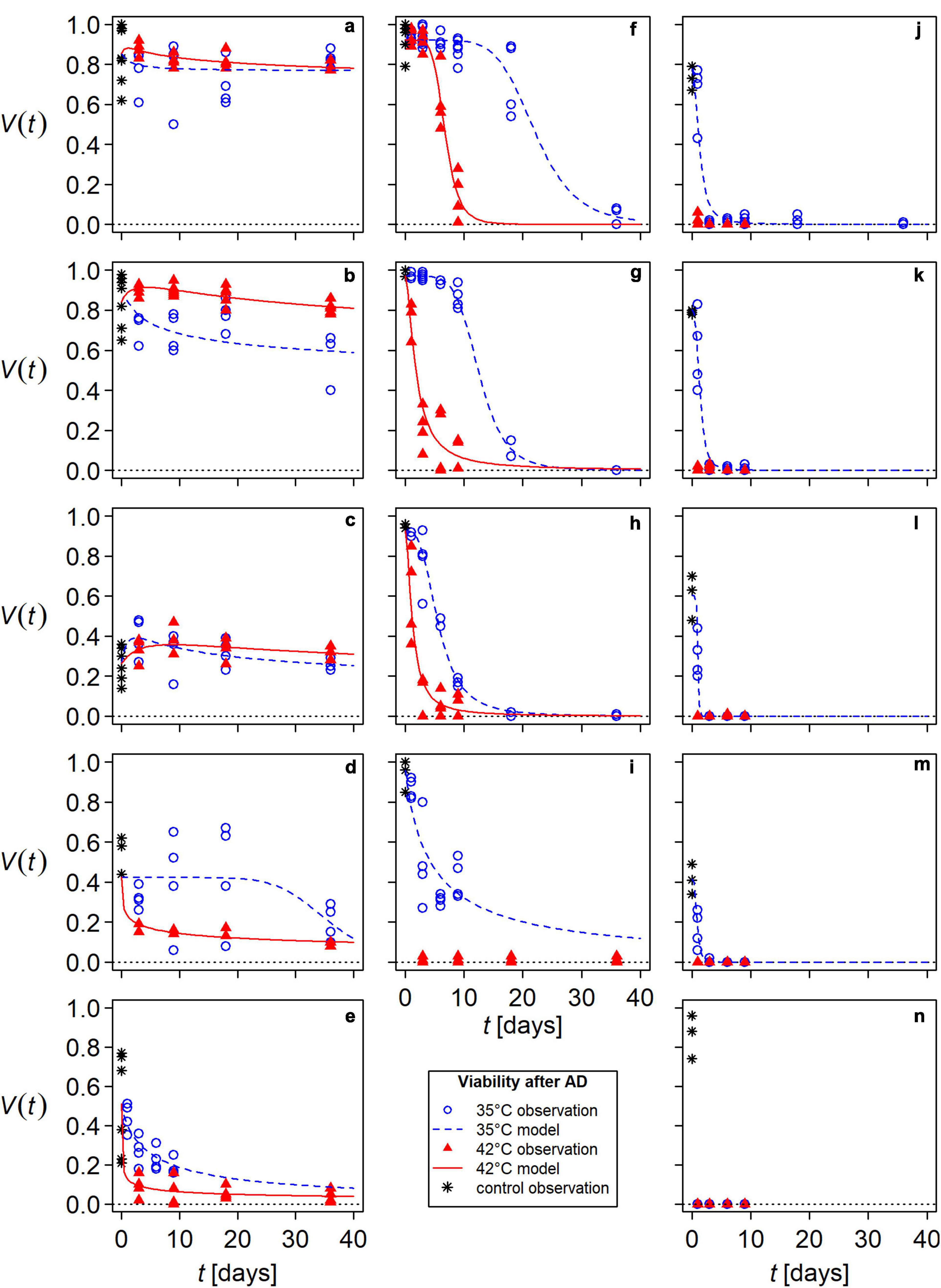
Figure 2. Modeled seed viability, V, of flowering wild plant species and tomato during anaerobic digestion (AD) in lab-scale reactors at 35°C (blue, dashed lines) and 42°C (red, solid lines). Symbols present observations, each containing a minimum of 100 seeds: asterisks for untreated controls, blue open circles for AD at 35°C and red filled triangles for AD at 42°C. (a) Melilotus officinalis, (b) Melilotus albus, (c) Malva sylvestris, (d) Malva alcea – 2 years, (e) Malva alcea – 1 year, (f) Chenopodium album, (g) tomato – PIERRE, (h) tomato – PAPRIKA, (i) Abutilon theophrasti – 1 year, (j) Abutilon theophrasti – 7 years, (k) Daucus carota, (l) Cichorium intybus, (m) Echium vulgare, (n) Verbascum thapsus. For better comparability, panels were arranged according to the species’ viability after 36 days of exposure to AD.
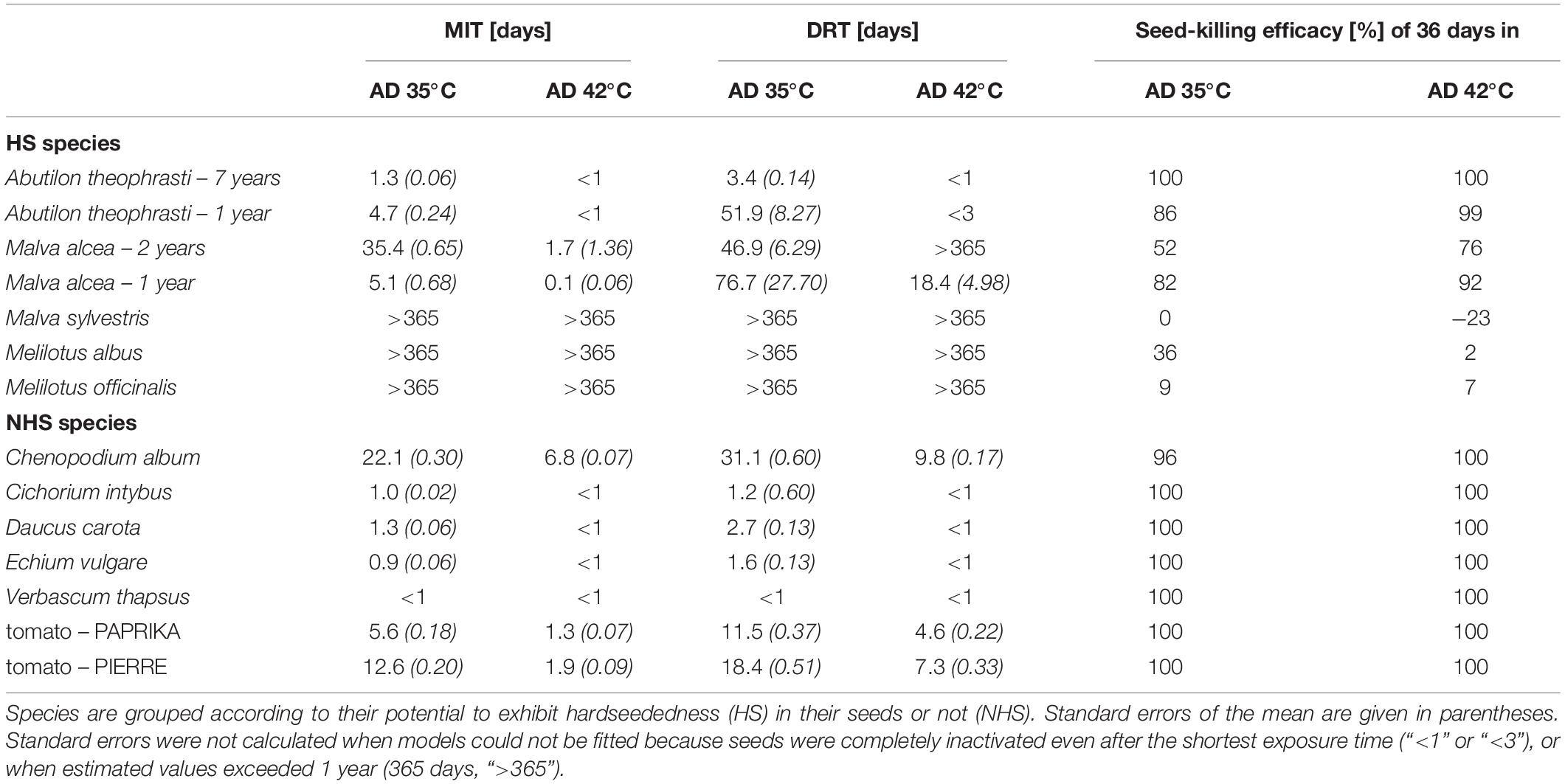
Table 1. Estimated median inactivation times (MITs) and decimal reduction times (DRTs) of seeds of flowering wild plant species and tomato after anaerobic digestion (AD) at 35 or 42°C in lab-scale reactors, and corresponding seed-killing efficacy of AD.
For all species except M. sylvestris, M. albus, and M. officinalis, seed viability continuously decreased with increasing exposure time in the lab-scale reactors. A more or less pronounced lag-phase was followed by an exponential decrease in viability, which led either to complete seed inactivation or a plateau. If a plateau was reached, it was lower at 42°C than at 35°C. In addition, the viability curves dropped more steeply in AD at 42°C than at 35°C (Figures 2d–n).
The viability curves of the particularly AD-resistant HS species M. officinalis, M. albus, and M. sylvestris were biphasic: modeled viability initially increased during the first days of AD (Figures 2a–c). This means that the observed viability of samples treated with AD was higher than that of the untreated controls. This observed increase was most pronounced in M. sylvestris, with some individual values exceeding the maximum of the controls. Modeled viability of M. sylvestris reached its maximum of 149 and 136% of untreated controls, respectively, in AD at 35°C after 2.5 days and in AD at 42°C after 8.9 days. Subsequently, viability decreased, but after 36 days in AD at 35°C, it was as high as in the untreated controls and even 23% higher at 42°C (Figure 2c). In the two Melilotus species, the increase in viability was lower than in M. sylvestris, remaining below the maximum value of the untreated controls. Furthermore, the increase occurred after a shorter time and was stronger in AD at 42°C than in AD at 35°C. Maximum viability values at 42°C were 108% for M. albus (4.3 days) and 103% for M. officinalis (0.9 days) (Figures 2a,b). In addition, M. albus tended to lose viability more rapidly in AD at 35°C than at 42°C (Figure 2b). In tendency, this was also observed in M. officinalis and M. sylvestris (Figures 2a,c).
Seed-Killing Efficacies
Averaged over all species and both temperatures, the mean seed-killing efficacy (SKE) of 36 days in mesophilic AD was 76 ± 40% (n = 28). However, the values for NHS species, particularly AD-resistant HS species, and the remaining HS species (A. theophrasti and M. alcea) differed greatly (Table 1). According to the viability models, NHS species were completely inactivated after 36 days. The only exception was C. album, of which 4 ± 1% of the seeds remained viable after 36 days in AD at 35°C (Table 1). Of the NHS species, only Chenopodium album and tomato were able to survive AD beyond 9 days of exposure at both temperatures (Figures 2f–h). Daucus carota seeds were only 1% viable after 9 days when anaerobically digested at 35°C (Figure 2k). Survival of all other NHS species was poorer: C. intybus, E. vulgare, and V. thapsus, survived less than 3 days at 35°C and less than 1 day at 42°C (Figures 2l–n). The mean SKE on the HS species A. theophrasti and M. alcea was 86 ± 16% (n = 8). The lowest mean SKE was determined for the particularly AD-resistant HS species. It was only 5 ± 19% (n = 6) with a range of −23 to 36% (Table 1).
Inactivation Times
Inactivation times showed a wide range, with the longest determined for M. officinalis, M. albus, and M. sylvestris. To inactivate 50% (MIT) or 90% (DRT) of their originally viable seeds, it was estimated that more than 1 year would have been required. The DRTs for the NHS representatives from the wildflower biogas mixture ranged from only a few hours to 2.7 ± 0.13 days (D. carota). The inactivation times for the other species fell between these values. With 31.1 ± 0.60 days and 9.8 ± 0.17 days at 35°C and 42°C, respectively, DRT values for C. album were in a similar range to those of the HS species, A. theophrasti (Table 1).
For most species, inactivation times were shorter in AD at 42°C than in AD at 35°C. Exceptions were three particularly AD-resistant species and M. alcea – 2 years (Table 1). In addition, inactivation times differed between the seed lots of a species. The tomato variety PAPRIKA was inactivated faster than the variety PIERRE (Figures 2h,g and Table 1). The batch A. theophrasti – 1 year had a 15-times longer DRT at 35°C than the 7-year old batch and even a few viable seeds remaining after 36 days in AD at 42°C (Figures 2i,j and Table 1). The difference between the two seed lots of M. alcea was most apparent when comparing their response to AD at 35°C: inactivation in M. alcea – 2 years was preceded by a 21-day lag phase, whereas it started immediately in M. alcea – 1 year (Figures 2d,e). As a result, it took considerably longer to reduce the viability of the older M. alcea by 50% than the younger one (MIT: 35 days instead of 5 days), but it took less time to reduce it by 90% (DRT: 47 days instead of 77 days) (Table 1).
Germinable and Dormant Seeds
Before Anaerobic Digestion
Untreated controls of HS and NHS species differed in the contribution of germinable and dormant seeds to viability (Figure 3, left). In NHS species, an average of 98 ± 3% (n = 7) of all viable seeds germinated. Dormant seeds occurred only in D. carota, E. vulgare, and tomato – PIERRE (Figure 3, left, bottom). In contrast, in the untreated samples of HS species, only 29 ± 20% (n = 7) of the viable seeds had germinated, while the rest remained dormant (Figure 3, left, top).
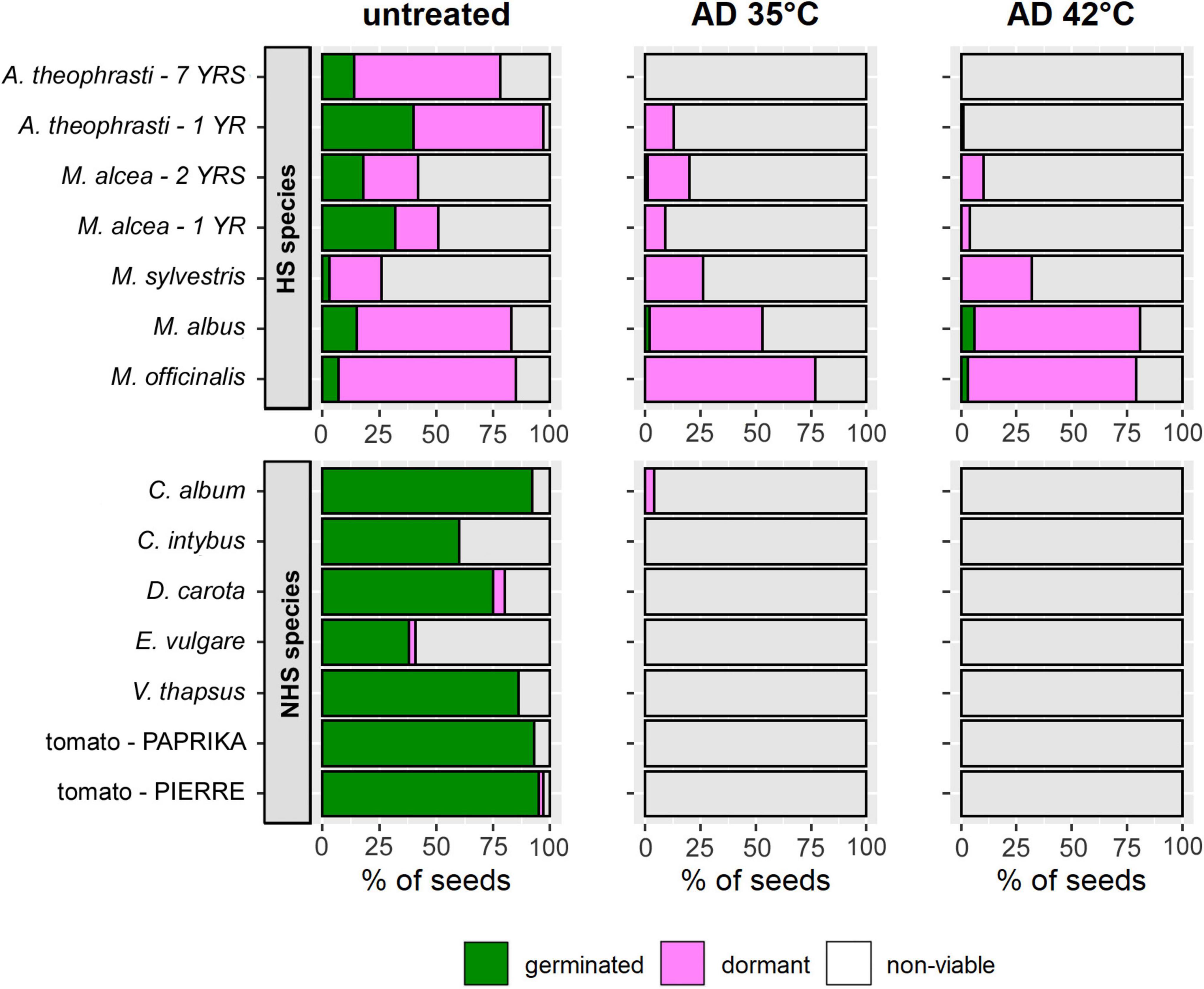
Figure 3. Mean percentages of germinated (green), dormant (pink), and non-viable seeds (gray) in batches of flowering wild plant species and tomato that were either untreated (left) or exposed to AD at 35°C (center) or 42°C (right) for 36 days. The top row shows the values for species with hardseededness (HS), the bottom row those for non-hardseeded (NHS) species. The percentage of germinated seeds equals the cumulative germination (cG) after completion of the 21-day germination test after AD-treatment. Values were predicted from models for V(t) and cG(t).
During Anaerobic Digestion
The contribution of germinable (cumulative germination, cG) and dormant seeds, D, to (total) viability, V, differed between HS and NHS species during the different exposure periods in AD (Figure 4). In NHS species, the majority of viable seeds germinated, and the loss of V corresponded to an approximately equal loss of cG (e.g., C. album in Figures 4a,b). This nearly simultaneous loss of cG and V was reflected in the fact that DRTs of cG were at most 5 days shorter than those of V (Supplementary Table 5). In all HS species except A. theophrasti, most viable seeds remained dormant during exposure to mesophilic AD. The viability curve paralleled that of the proportion of dormant seeds (e.g., M. albus in Figures 4c,d, M. sylvestris in Figures 4e,f). The time interval between 90% loss of cG and V was not days as in NHS species, but weeks or months (Supplementary Table 5).
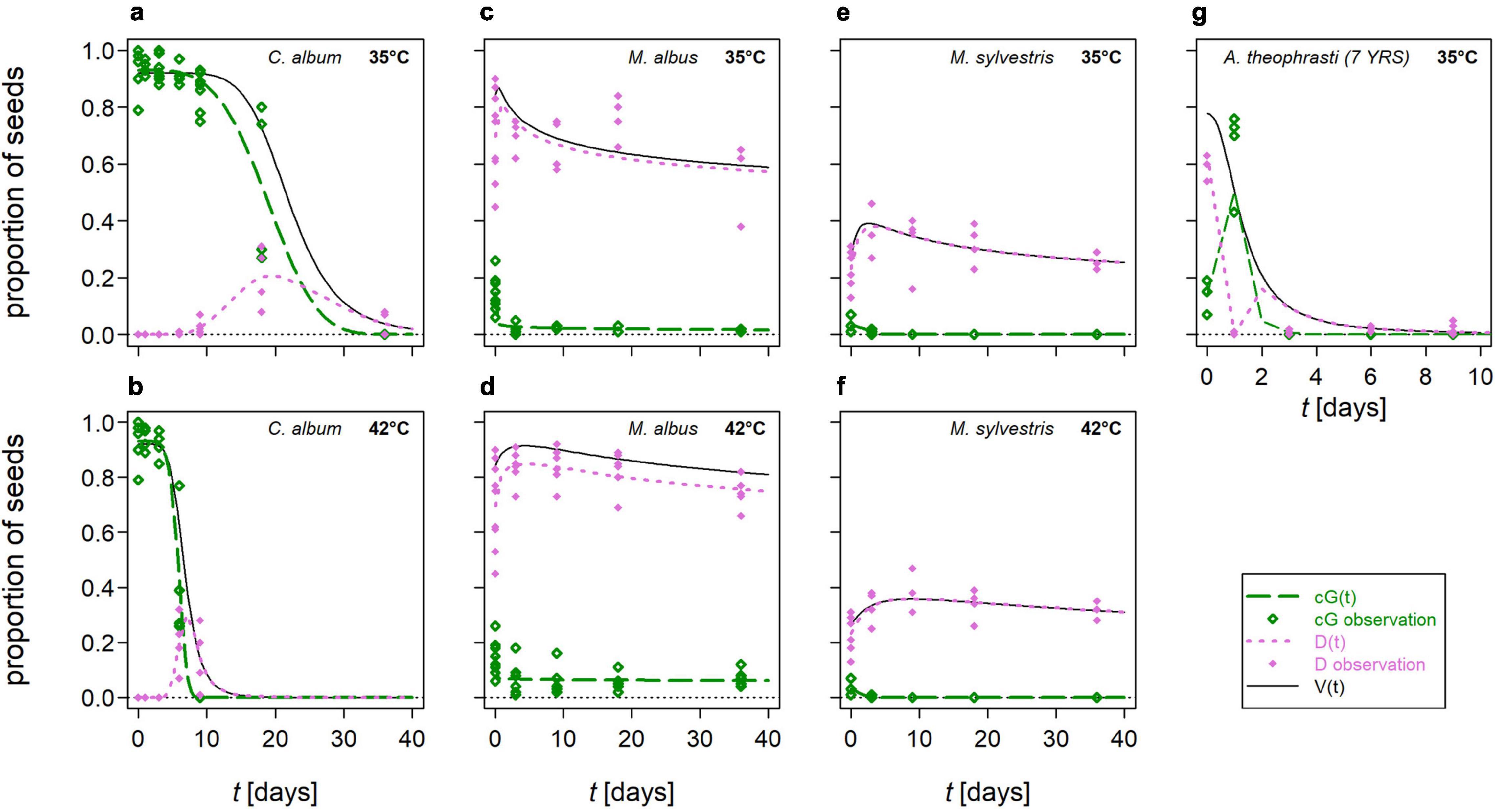
Figure 4. Modeled proportions of germinated (cumulative germination, cG, green long-dashed lines) and dormant seeds (D, pink dotted lines) out of all viable seeds (V, black solid lines, compare Figure 3) of selected flowering wild plant species after mesophilic, anaerobic digestion. D(t) was calculated from the difference between the models for V(t) and cG(t). Symbols represent observations, each based on a minimum of 100 seeds: green open diamonds for cG and pink closed diamonds for D. (a,b) Chenopodium album at 35 and 42°C, respectively; (c,d) Melilotus albus at 35 and 42°C, respectively; (e,f) Malva sylvestris at 35 and 42°C, respectively; (g) Abutilon theophrasti – 7 years at 35°C. Please note the different scaling of the x-axis in (g).
Only in A. theophrasti cG peaked during the first 3 days of exposure and not in the untreated controls as in the other species. For instance, in the A. theophrasti – 7 years seed lot, after 1 day in AD at 35°C, cG was five-times higher than in the untreated control. This peak of germinable seeds was paralleled by a local minimum of dormant seeds. However, D increased again as soon as the cG-peak exceeded its maximum (Figure 4g and Supplementary Figure 3J).
In four species, namely A. theophrasti, C. album, D. carota and tomato, a peak of dormant seeds was observed after cG and V began to decline. In the most extreme case, C. album, these D-peaks could account for up to one-third of V and last up to 30 days (Figure 4a). After longer exposure times, the curves of D and V finally coincided. Thus, the proportion of D, accounted for the sum of all seeds that were still viable after AD.
After 36 Days in Anaerobic Digestion
Seeds that were still viable after 36 days in mesophilic AD were almost exclusively dormant. This was also the case for the only surviving NHS species, C. album (Figure 3, middle + right). Small proportions of germinated seeds were only found in M. alcea, M. albus and M. officinalis (Figure 3, middle + right, top). The highest percentages of germinated seeds were observed for M. albus and M. officinalis: 0.06 and 0.03, respectively, after 36 days of AD at 42°C (Figure 3, right, top). Furthermore, only in the Melilotus species did more seeds germinate after AD at 42°C than at 35°C. Finally, only in Melilotus species were bare, i.e., seed coat-less, embryos frequently found in the sample bags in addition to seeds that were recognizable as viable or non-viable. When some of the bare embryos were subjected to a germination test, it became apparent that they were able to develop and grow.
Discussion
Mesophilic AD affected seed viability of the species studied to varying degrees, and none of the potentially modulating factors had a consistent effect. We rejected the hypotheses: mesophilic AD, reduced seed viability of one of the HS species more than that of two of the NHS species (hypothesis 1). Seed viability of three species did not decrease markedly more at the higher incubation temperature than at the lower one (hypothesis 2). The observed seed viability of three species increased when exposure time increased up to a certain point (hypothesis 3). Instead, we report a more complex response of the wildflower seeds studied: more factors than HS affected AD-resistance (see section “Anaerobic Digestion-Resistance of Hardseeded and Non-hardseeded Species”). Nevertheless, some of the HS species were particularly AD-resistant (see section “Particularly Anaerobic Digestion-Resistant Hardseeded Species”). Furthermore, responses were dependent on both germinable and dormant seeds (see section “Diversity of Viability Responses”). These findings have implications for the use of flowering wild plants as biogas feedstocks (see section “Flowering Wild Plants as Biogas Feedstocks”).
Anaerobic Digestion-Resistance of Hardseeded and Non-hardseeded Species
In this study, seed-killing efficacies tended to be lower and inactivation times longer in HS species than in NHS species. However, inter- and intraspecific variation was high. Considering together viability curves, seed-killing efficacies, and inactivation times, it appears that the HS and the NHS species studied overlapped in AD-resistance potential, with NHS species tending to be at the “low” end of the scale and HS species at the “high” end. The HS species A. theophrasti and the NHS species C. album and tomato mainly caused this overlap. The most AD-resistant NHS species in this study was C. album, and its maximum survival times were in the upper ranges of values from comparable studies (Schrade et al., 2003; Katovich et al., 2004; Leonhardt et al., 2010; Westerman et al., 2012a,b; Johansen et al., 2013; Oechsner et al., 2018; Zhou et al., 2020). The same, although less pronounced, was true for tomato (Engeli et al., 1993; Lorenz et al., 2001; Schrade et al., 2003; Marcinisyn et al., 2004; Westerik and Kleizen, 2006; Strauß et al., 2012; Westerman et al., 2012a,b; Baute et al., 2016). Whether this was due to seed lot characteristics or different AD-conditions cannot be determined. However, several other NHS species survived mesophilic AD treatments for more than 1 week: Amaranthus retroflexus L. (common tumbleweed, Šarapatka et al., 1993; Katovich et al., 2004), Digitaria sanguinalis (L.) SCOP. (purple crabgrass (Engeli et al., 1993), Echinochloa crus-galli (L.) P. BEAUV (common barnyard grass, Šarapatka et al., 1993), Panicum dichotomiflorum MICHX. (autumn millet, Jeyanayagam and Collins, 1984), Rumex obtusifolius L. (broadleaf dock, Engeli et al., 1993; Šarapatka et al., 1993) and Sorghum halepense (L.) PERS. (Jeyanayagam and Collins, 1984). The survival of these NHS species supports the suggestion that HS is not the only mechanism by which seeds can gain resistance to AD (Westerman and Gerowitt, 2013). In the case of C. album and tomato a physically hard (but not HS) or thick seed coat has been discussed as the basis of their AD resistance (Blackshaw and Rode, 1991; Strauß et al., 2012; Aper et al., 2014). The protection provided by this hard seed coat would be lost during AD by microbial degradation processes; first slowly (lag-phase) and then increasingly rapidly. However, it is unclear whether this mechanism alone is sufficient to explain the remarkably high AD-resistance of C. album and tomato in this study.
Resistance differences within HS species were likely related to the fact that both the degree and depth of HS varies among species and seed lots (Baskin and Baskin, 2004). A good example of this is the 7-year-old seeds of A. theophrasti used in this study, which have already been tested in two other studies. Two years after harvest, about half of A. theophrasti seeds survived 30 days of AD in lab-scale batch reactors at 37°C (Westerman et al., 2012b) and all seeds were inactivated after 9 days of AD in commercial fermenters at 41°C (Westerman et al., 2012a). Now 7 years old, this seed lot was completely inactivated after 36 days in AD at both 35 and 42°C, and DRT was shorter at 42°C (<1 day) than at 41°C (2 days, Westerman et al., 2012a). Since other differences can be excluded here, AD-resistance must have decreased due to seed aging during storage (Sano et al., 2016). Aging and loss of AD-resistance appeared to be associated with a reduction in the depth of HS, as the degree of HS was higher at older ages (82% of “hard” seeds in the seed lot) than at younger ages (about 65%, Westerman et al., 2012b). A lower HS depth would also be consistent with the observation that the older seed lot germinated more and was less AD-resistant than the 1-year-old A. theophrasti lot used in this study, which had an even lower degree of HS (59%). However, unlike the first comparison, these two seed lots did not differ only in storage duration. This implies that differences in their AD-resistance could also be due to other factors leading to variations in HS, such as genetic differences, weather and site conditions, seed maturity, endogenous dormancy rhythms, and conditions of storage (Rolston, 1978; Baskin and Baskin, 1998; Hilhorst, 1998; Hay and Probert, 2013; Jaganathan et al., 2016). In this context, Westerman et al. (2012b) noted that genetic factors or environmental conditions during seed filling and maturation could be responsible for the difference in survival probability of mesophilic AD between populations of A. theophrasti harvested in different years and/or locations. Similarly, the higher AD-resistance of the older M. alcea batch in this study can be explained by the fact that its seeds were more mature and therefore had greater depth (Goldberg et al., 1994) and higher degree of HS (58% versus 38%) than the younger batch.
The influence of different seed lots on AD-resistance is not limited to HS species. Different seed lots of NHS species responded differently to AD as well (Traveset, 1998; Westerman et al., 2012b; Zhou et al., 2020). Consequently, AD-resistance seems determined by both species-specific traits and characteristics of the respective seed lots. This raises the question of (1) what factors affecting seed lot quality lead to differences in AD-resistance and (2) how large the differences between seed lots are compared to the differences between species. In addition, the relative importance of seed traits and AD-conditions on seed-killing efficacy would need to be determined.
Particularly Anaerobic Digestion-Resistant Hardseeded Species
Compared to the other species examined in this study, the HS species M. officinalis, M. albus, and M. sylvestris were particularly resistant to mesophilic AD, tended to lose viability more slowly and to germinate more after AD at 42°C than at 35°C, and showed an initial increase in observed viability.
The seed-killing efficacy of AD on M. officinalis, M. albus and M. sylvestris was very low compared to other members of the Fabaceae and Malvaceae. The four other Fabaceae species studied to date, namely Glycine max (L.) MERR. (soybean), Lupinus polyphyllus LINDLEY (garden lupin), Trifolium pratense L. (red clover) and Vicia tetrasperma (L.) Schreb. (smooth vetch) had lost substantially more than 9% – 36% viability when exposed to similar AD-conditions (35–38°C, 7–30 days) (Leonhardt et al., 2010; Strauß et al., 2012; Westerman et al., 2012b; Hassani et al., 2021). Among the three members of the genus Malva studied, seed-killing efficacy was higher in M. alcea (this study) and Malva neglecta Wallr. (dwarf mallow) (Westerman et al., 2012a,b) than in M. sylvestris. It is particularly noteworthy that after 36 days of AD, the measured values for seed viabilities of M. albus and M. officinalis were still in the range of the values of the untreated controls. Values of M. sylvestris were even higher than in the controls, resulting in negative SKEs. The high AD-resistance distinguishes M. officinalis, M. albus and M. sylvestris from all other species.
The second unique characteristic of the particularly AD-resistant HS species was their tendency to lose viability faster and more severely at 35°C than at 42°C. Additionally, the range of responses was wider at 35°C. This was in contrast to all other species studied to date. However, it must be put into perspective that (a) this response was prominent only in M. albus and (b) other Fabaceae and Malvaceae were each exposed to only one temperature in previous studies (Katovich et al., 2004; Leonhardt et al., 2010; Strauß et al., 2012; Westerman et al., 2012a,b; Hassani et al., 2021). Some of our observations in Melilotus sp. suggest that fatal germination has played a role in the unexpected and rather counterintuitive response to temperature increase. First, we found bare but germinable embryos of M. albus and M. officinalis in the sample bags, indicating that AD triggered germination in these species. As a result, seeds would die due to thermosensitivity (Westerman and Gerowitt, 2013) and degrade, unless germination was triggered just before samples were removed from the reactor. Second, fewer Melilotus seeds germinated after AD at 35°C than after AD at 42°C, which may indicate that AD at 35°C caused more seeds to germinate already in the reactor and then die. In this way, viability would decline more at 35°C than at 42°C. It also cannot be ruled out, however, that the tendency of higher survival probability at the higher temperature was related to HS or the increase in observed viability.
Exclusively in M. officinalis, M. albus, and M. sylvestris, there was no lag-phase at the beginning of the AD-treatment, but an increase in observed seed viability. The resulting biphasic viability curves extend the spectrum of known responses to AD because, previously, such increases in seed viability in AD have only been reported for NHS species (Schrade et al., 2003; Westerik and Kleizen, 2006; Leonhardt et al., 2010; Baute et al., 2016; Zhou et al., 2020). In NHS species, the initial viability increase in AD was associated with the breaking of dormancy and initiation of germination (Westerik and Kleizen, 2006; Leonhardt et al., 2010; Zhou et al., 2020). However, in these three studies, germinability was equated with seed viability, which unfortunately does not allow conclusions to be drawn about total viability, i.e., germinating plus dormant seeds. Combining germination and TTC tests, we found that the increase in observed viability of the highly AD-resistant HS species was not due to an increase in germinating seeds, but to that of dormant seeds. This means that the total observed viability of the seed lot increased compared to the untreated control. The only study we know of that attempts to explain a similar result examined Heracleum mantegazzianum SOMMIER & LEVIER (giant hogweed) in a water bath at 35°C (Tanke et al., 2019). The authors attributed the increase in seed viability after 12 h to insufficient hydration of previously dry-stored control seeds. Hence, the TTC assay failed to capture the full respiratory potential of the seeds under these conditions (Elias et al., 2012; Miller, 2014). In other words, the observed viability increase was assumed to be an artifact. However, the controls in our study were well hydrated due to the pretreatment and germination test before the TTC test. Moreover, unlike the NHS species H. mantegazzianum, the HS species M. officinalis, M. albus and M. sylvestris in this study were not inactivated shortly after the viability peak, but their observed viability remained at a high level. Therefore, we hypothesize that the increase in observed viability was due to AD-induced metabolic stimulation of seeds whose metabolic activity was not detectable by TTC staining before AD. If this was the case, the increase in seed viability would be a form of hormesis, i.e., a dose-response phenomenon in which low doses of a stressor - here, brief exposure to AD - have a stimulatory effect, whereas high doses cause inhibition (e.g., Calabrese and Baldwin, 2002; Mattson, 2008; Kendig et al., 2010). To date, hormesis has been demonstrated in over 15,000 experimental studies (Kozumbo and Calabrese, 2019). And biogas reactor conditions, e.g., heat, enzymes, amino and organic acids, alcohols, hydrogen sulfide, ammonia, and cyanides (Westerman and Gerowitt, 2013), resemble stressors known to induce hormesis (e.g., Calabrese et al., 2007). The assumed stimulation by AD would have to activate processes that can repair (oxidative) damage to DNA, membranes, proteins, etc., that occurred before the AD treatment, i.e., during maturation and storage of the seed. Examples of such processes include activation of DNA repair mechanisms and antioxidant (enzyme) systems, expression of growth factors, anti-apoptotic proteins and heat shock proteins, and de-novo synthesis of cellular components. These processes occur in established, conserved hormetic pathways (Calabrese et al., 2007; Mattson, 2008) and enhance seed vigor during pre-germinative metabolic events utilized in seed priming (Paparella et al., 2015; Lutts et al., 2016). However, the observed viability increases in M. officinalis, M. albus and M. sylvestris may also be due to limitations of TTC testing that were detectable only in these three species. It is conceivable that the metabolic activity of (AD-)microorganisms attached to the seeds resulted in an apparent increase in seed viability. With the same result, AD may have facilitated TTC uptake in these HS species. Furthermore, TTC tests are invasive, so the viability of the AD-treated samples in the untreated state may have varied more than that of our controls, even though all samples were from the same seed lot. Then, there is the question of the extent to which the timing and mechanisms of an increase in viability differ between HS and NHS species. The HS species in this study took between 6 hours and 9 days longer to reach the viability maximum than NHS species in other studies (Schrade et al., 2003; Westerik and Kleizen, 2006; Leonhardt et al., 2010; Baute et al., 2016; Zhou et al., 2020). Thus, the shortest sampling interval of 24 h could have been too long to detect viability increases in all species studied here. Finally, given the particular AD-resistance of these HS species, it is of particular interest to determine whether or not the hypothesized metabolic stimulation is due to processes that require imbibition. This aspect is important because repair processes are normally associated with (partial) rehydration of seeds (Weitbrecht et al., 2011; Powell and Matthews, 2012; Paparella et al., 2015; Sano et al., 2016), but the protective effect of HS against AD is irreversibly lost once the water impermeability of the seed coat is broken and seeds absorb water (Westerman and Gerowitt, 2013). However, it is also possible that metabolic stimulation was triggered by factors other than rehydration, because unimbibed, dry seeds can have low-level metabolic activity (e.g., Weitbrecht et al., 2011; Sano et al., 2016) and even priming effects are not necessarily related to seed imbibition (Lutts et al., 2016). In summary, to clarify whether hormesis can be triggered by AD in seeds, future studies should include measurements with a higher resolution in the range of the increase in observed viability and be complemented by molecular analyses.
Diversity of Viability Responses
Observed responses during mesophilic AD ranged from complete inactivation to viability increases. Furthermore, responses differed between germinable and dormant seeds. Germinability was lost faster than viability in all species, indicating that mainly dormant seeds survived AD. In contrast to the NHS species, the HS species lost their germinability very quickly compared to their viability. And at the end of the measurement period, almost all of their surviving seeds were active but not germinating, thus, most probably physically dormant. These observations confirm that HS makes survival in AD more likely (Leonhardt et al., 2010; Westerman et al., 2012b; Jaganathan et al., 2016; Hassani et al., 2021). It remains unclear to what extent, however, as the HS species had lost so little viability at the end of the measurements. It appeared as if the remaining hard seeds could survive AD for even longer periods (viability plateau). The exceptionally long estimated inactivation times for the particularly AD-resistant HS species reflected this. However, they are unlikely to survive longer than a year in mesophilic AD, as the most resistant species studied to date survived only about 30 days (e.g., Jeyanayagam and Collins, 1984; Westerman et al., 2012b), and up to 155 days in extreme cases (Hassani et al., 2021). Moreover, seeds can be inactivated in AD despite intact physical dormancy (Westerman et al., 2012b) and viability plateaus can end quite abruptly, as observed in M. alcea – 2 years (Figure 2d). For these reasons, a realistic assessment of AD-resistance in HS species requires that measurements continue until both the dormant and non-dormant seeds are fully inactivated.
A special case with regard to its responses was Abutilon theophrasti. First, it was the only HS species to be completely (7 years old seed lot) or nearly completely (1 year old) inactivated at the end of the measurement period. Possible reasons for this have already been discussed (see section Anaerobic Digestion-Resistance of Hardseededness and Non-hardseeded Species). Second, mesophilic AD stimulated germination in it, which has previously been observed only in NHS species (Schrade et al., 2003; Westerik and Kleizen, 2006; Leonhardt et al., 2010; Baute et al., 2016; Oechsner et al., 2018; Zhou et al., 2020). Third, it was the only HS species in which peaks of metabolically active seeds (D-peaks) occurred when cumulative germination decreased. Otherwise, these D-peaks occurred only in C. album, tomato, and D. carota.
It remains to be clarified how the D-peaks are to be evaluated in terms of seed viability. Based on our investigations, we can either assume that the D-peaks represented the parts of the seed lots no longer protected by the seed coat. These would have been damaged, losing their ability to germinate and retaining only some metabolic activity, which we would have mistakenly interpreted as dormancy. If we follow this line of reasoning, we would agree with Eckford et al. (2012). They assumed that AD-treated seeds of Fallopia convolvulus L. (wild buckwheat) with pink-stained and thus theoretically viable embryos were not capable of normal growth and development, because a TTC test does not measure the capacity for normal cell division, growth speed, or dormancy (Copeland, 1976; Miller, 2014). However, for AD-treatments of perennial biomass species and tomato Baute et al. (2016) found that Petri-dish germination with TTC staining resulted in similar viability estimates as glasshouse germination with cold-moisture stratification. The overestimation by TTC staining was only 5%. Moreover, a TTC test may well determine the number of seeds that would develop normal seedlings in a germination test when all available viability indicators are included in the evaluation (Elias et al., 2012). We did this by classifying only the red-stained, physically intact embryos as viable. Thus, the D-peaks could represent fully viable, in principle germinable but dormant seeds. This would mean that secondary dormancy was induced by AD. Possible triggers such as heat, high moisture and low oxygen levels (Hilhorst, 1998; Bentsink and Koornneef, 2008) occur in biogas reactors. Overud (2002) previously speculated that the low oxygen content was responsible for the induction of secondary dormancy in Rumex crispus L. (curled dock) in ensiling, another anaerobic fermentation process. This reasoning, together with the observation that D-peaks occurred only in those NHS species that were relatively resistant to AD, raises the question of whether dormancy mechanisms other than HS contribute to AD-resistance in seeds. Considering that chemical and biological processes contribute to seed inactivation in addition to temperature (Westerman and Gerowitt, 2013; Zhou et al., 2020), any dormancy-related defenses against microbial or chemical attack (e.g., Fuerst et al., 2011; Chen et al., 2018) could increase the probability of seed survival in AD.
In summary, the way in which species and seed lot traits impact seed viability responses to AD seems multifaceted. Future studies should include physiological and molecular aspects in order to develop a more mechanistic view that allows to better predict seed viability in AD.
Flowering Wild Plants as Biogas Feedstocks
Plant seeds that survive AD in a commercial biogas plant can get particularly widely distributed because the digestate is also traded between farms. In addition, repeated AD of seed-bearing biomass and its fertilization with digestate containing (viable) seeds can lead to the selection for AD-resistant biotypes (Westerman and Gerowitt, 2013). Under these conditions, a few surviving seeds would be sufficient for a new weed flora to emerge. Our finding that a portion of all tested HS species was still viable after 36 days of mesophilic AD suggests that these risks exist when using these species or the flowering mixture as a biogas feedstock. This is especially true for mesophilic biogas plants, which operate with shorter hydraulic retention times (HRTs) than in this study. In Germany, for example, 15% use HRTs between 15 and 35 days (vTI, 2009). In addition, approximately 1–10% of the freshly fed feedstock passes through the reactor after only 6–24 h (Turner et al., 1983; Baier et al., 2010; Eckford et al., 2012). Especially complete mix, one-stage reactors suffer from this short-circuiting (Ward et al., 2008). After such shortened retention times in AD, seeds that are either not yet inactivated or possibly even activated (hormesis, germination stimulation) can enter the digestate. Consequently, it would be safest to exclude HS species in the composition of biogas mixtures - and as a biogas feedstock in principle.
Excluding HS (wild plant) species as biogas feedstock, however, would mean losing their socio-ecological benefits (e.g., Kuhn and Vollrath, 2010; von Cossel, 2020), e.g., the nitrogen fixation of Fabaceae or the attractive flowering offer of Malvaceae. Furthermore, it is still unclear whether the risk of spread found in experiments applies in agricultural practice. In most commercial biogas plants, the proportion of surviving seeds is likely to be lower because the average HRT is longer than that tested, e.g., 91 days in Germany (vTI, 2009). The other steps in the biogas production chain can contribute to seed inactivation as well, namely cultivation, harvest, ensiling, storage, pre-treatment and digestate storage (Fröschle et al., 2015). On farms, biomass is usually ensiled prior to AD. In general, ensiling reduced the viability (e.g., Aper et al., 2014; Simard and Lambert-Beaudet, 2016; Piltz et al., 2017), including that of seeds from the flowering wild plant mixture (Hahn et al., 2021). When the same seed lot was both ensiled and anaerobically digested in biogas reactors (Westerman et al., 2012b) or in the rumen of cattle (Blackshaw and Rode, 1991; Mayer et al., 2000; Stanton et al., 2012; Aper et al., 2014; Piltz et al., 2017), seed-killing efficacy was mostly higher in ensiling than in AD. However, there was considerable variation between and within species. The same picture emerged when comparing the seed-killing efficacies of 36 days in mesophilic AD (Table 1) with those obtained for 8 months of ensiling by Hahn et al. (2021). The same seed lots of C. album, C. intybus, V. thapsus, A. theophrasti – 7 years, M. alcea – 2 years, M. albus and M. officinalis were used in both studies. In NHS species and M. officinalis, a greater proportion of seeds was killed by ensiling than by AD. In contrast, ensiling was less effective on A. theophrasti – 7 years and M. alcea – 2 years, inactivating only 5 and 23%, respectively, while AD killed 100% and about 60%, respectively. Consistent with this, Westerman et al. (2012b) found that their batch of A. theophrasti and the species Malva neglecta were more resistant to ensiling than to AD. Piltz et al. (2017), studying Malva parviflora L. (small-flowered mallow) and referring to Malva pusilla SM. (small mallow) studied by Blackshaw and Rode (1991), even suggested that resistance to ensiling may be characteristic of the Malvaceae genus. Finally, for M. albus, our results showed that seed-killing efficacy did not differ per se between the processes of ensiling and AD, but depended on the respective conditions: AD at 35°C killed 36% of M. albus seeds, ensiling killed 23% and AD at 42°C killed only 2% (Table 1; Hahn et al., 2021). Regarding a combined seed-killing efficacy of ensiling and AD, the few available sources indicate that it is about the same as that of the individual processes, but tends to kill more seeds (Blackshaw and Rode, 1991; Stanton et al., 2012; Westerman et al., 2012b; Piltz et al., 2017).
We conclude that it is not necessary to categorically exclude HS species to avoid unwanted seed spread when mixtures of flowering wild plant species are used as biogas feedstock. Rather, we recommend that conditions during AD be designed to increase the seed-killing efficacy. This can be achieved, for example, by sufficiently long HRTs in the biogas reactor without short-circuiting. Furthermore, HS species whose seeds are easy to inactivate should be identified. Considering that AD is only one step within the biogas process chain, seed-killing efficacy of AD may have been underestimated in our experiments conducted with mature, vigorous, dry-stored seed, because the seed in practice may be immature and damaged by upstream treatments, making it more susceptible to AD (Westerman and Gerowitt, 2013; Zhou et al., 2020). Therefore, the influence of cultivation parameters on seed quality, e.g., harvest timing, and the influence of feedstock storage conditions, pre-treatment and digestate processing technologies (Monfet et al., 2018; Alsanius et al., 2021) on seed viability remain to be explored. And even though our reactors were comparable to a full-scale fermenter in terms of operating parameters (Heiermann and Plogsties, 2018), our laboratory results require validation on the practical scale. Finally, seed establishment studies under field conditions are needed to realistically assess the dispersal risk of seeds that survive AD.
Data Availability Statement
The raw data supporting the conclusions of this article will be made available by the authors, without undue reservation, to any qualified researcher on request.
Author Contributions
JH organized the project, designed the study, analyzed the viability of the seeds, and drafted and edited the manuscript. PW analyzed the viability of the seeds and edited the manuscript in coordination with JH. FM contributed to performance and description of the statistical analyses in coordination with JH. MH administrated the operation of the lab-scale reactors and edited the manuscript in coordination with JH. BG led the project and contributed to the conception and design of the study. All authors contributed to manuscript revision, read, and approved the submitted version.
Funding
This study was funded by the German Federal Ministry of Food and Agriculture through the “Fachagentur Nachwachsende Rohstoffe e.V.” (FNR) under grant numbers 22401114 and 22401513. Open Access publication was funded by the Open Access Publication Fund of the University of Rostock supported by the Deutsche Forschungsgemeinschaft (DFG) (project number 325496636).
Conflict of Interest
The authors declare that the research was conducted in the absence of any commercial or financial relationships that could be construed as a potential conflict of interest.
Publisher’s Note
All claims expressed in this article are solely those of the authors and do not necessarily represent those of their affiliated organizations, or those of the publisher, the editors and the reviewers. Any product that may be evaluated in this article, or claim that may be made by its manufacturer, is not guaranteed or endorsed by the publisher.
Acknowledgments
Our sincere thanks goes to Vincent Plogsties and Tilman Schlief for running the laboratory-scale reactors at the ATB and inserting/removing the plant seeds. Further, we thank Maren Knipping, Rosa Minderlen, and Ophélie Rollin for their tireless assistance during the determination of seed viability. Last but not least, we appreciate the on-time transport of the digested seeds by Markus Weinreich, Julia Schulz, Maria Lipski, and Anne Grauholz.
Supplementary Material
The Supplementary Material for this article can be found online at: https://www.frontiersin.org/articles/10.3389/fpls.2022.942346/full#supplementary-material
Footnotes
References
Alsanius, B., Magnusson, C., Nicolaisen, M., Wright, S. A. I., Wendell, P. H. M., et al. (2021). Assessment of Treatment Methods and Validation Criteria for Composting and Biogas Facilities in Relation to Plant Health Risks and The Risk Of Spreading Alien Organisms: Scientific Opinion of the Panel on Plant Health of the Norwegian Scientific Committee for Food and Environment. VKM Report 2021:19. Oslo: Norwegian Scientific Committee for Food and Environment.
Altieri, M. A. (2009). The ecological impacts of large-scale agrofuel monoculture production systems in the Americas. Bull. Sci. Technol. Soc. 29, 236–244. doi: 10.1177/0270467609333728
Aper, J., de Cauwer, B., de Roo, S., Lourenço, M., Fievez, V., Bulcke, R., et al. (2014). Seed germination and viability of herbicide resistant and susceptible Chenopodium album populations after ensiling, digestion by cattle and manure storage. Weed Res. 54, 169–177. doi: 10.1111/wre.12063
Association of Official Seed Analysts (2010). Tetrazolium Testing Handbook: Contribution No. 29 to the Handbook on Seed Testing. Ithaca, NY: Association of Official Seed Analysts.
Baier, U., Warthmann, R., and Schliess, K. (2010). Vergärungs- und Kompostierungsanlagen als Hygienebarrieren. Winterthur.
Baker, H. G. (1974). The evolution of weeds. Annu. Rev. Ecol. Syst. 5, 1–24. doi: 10.1146/annurev.es.05.110174.000245
Baskin, C. C., and Baskin, J. M. (1998). Seeds: Ecology, Biogeography, and Evolution of Dormancy and Germination. San Diego, CA: Academic Press. doi: 10.1017/CBO9780511525445.004
Baskin, J. M., and Baskin, C. C. (2004). A classification system for seed dormancy. Seed Sci. Res. 14, 1–16. doi: 10.1079/SSR2003150
Baskin, J. M., Baskin, C. C., and Li, X. (2000). Taxonomy, anatomy and evolution of physical dormancy in seeds. Plant Species Biol. 15, 139–152. doi: 10.1046/j.1442-1984.2000.00034.x
Baute, K. A., Robinson, D. E., van Eerd, L. L., Edson, M., Sikkema, P. H., and Gilroyed, B. H. (2016). Survival of seeds from perennial biomass species during commercial-scale anaerobic digestion. Weed Res. 56, 258–266. doi: 10.1111/wre.12202
Bentsink, L., and Koornneef, M. (2008). Seed dormancy and germination. Arabidopsis Book 2008:e0119. doi: 10.1199/tab.0119
Blackshaw, R. E., and Rode, L. M. (1991). Effect of Ensiling and Rumen Digestion by Cattle on Weed Seed Viability. Weed Sci. 39, 104–108. doi: 10.1017/S0043174500057957
Calabrese, E. J., Bachmann, K. A., Bailer, A. J., Bolger, P. M., Borak, J., Cai, L., et al. (2007). Biological stress response terminology: integrating the concepts of adaptive response and preconditioning stress within a hormetic dose-response framework. Toxicol. Appl. Pharmacol. 222, 122–128. doi: 10.1016/j.taap.2007.02.015
Calabrese, E. J., and Baldwin, L. A. (2002). Defining hormesis. Hum. Exp. Toxicol. 21, 91–97. doi: 10.1191/0960327102ht217oa
Carlsson, G., Mårtensson, L.-M., Prade, T., Svensson, S.-E., and Jensen, E. S. (2017). Perennial species mixtures for multifunctional production of biomass on marginal land. GCB Bioenergy 9, 191–201. doi: 10.1111/gcbb.12373
Cedergreen, N., Ritz, C., and Streibig, J. C. (2005). Improved empirical models describing hormesis. Environ. Toxicol. Chem. 24, 3166–3172. doi: 10.1897/05-014R.1
Chen, T., Nan, Z., Zhang, X., Hou, F., Christensen, M., and Baskin, C. C. (2018). Does dormancy protect seeds against attack by the pathogenic fungus Fusarium tricinctum in a semiarid grassland of Northwest China? Plant Soil 422, 155–168. doi: 10.1007/s11104-017-3420-9
Copeland, L. O. (1976). Principles of Seed Science and Technology. Minneapolis, MN: Burgess Publishing Company.
Don, A., Osborne, B., Hastings, A., Skiba, U., Carter, M. S., Drewer, J., et al. (2012). Land-use change to bioenergy production in Europe: implications for the greenhouse gas balance and soil carbon. GCB Bioenergy 4, 372–391. doi: 10.1111/j.1757-1707.2011.01116.x
Eckford, R. E., Newman, J. C., Li, X., and Watson, P. R. (2012). Thermophilic anaerobic digestion of cattle manure reduces seed viability for four weed species. Int. J. Agric. Biol. Eng. 5, 71–75.
Eggers, J., Trölzsch, K., Falcucci, A., Maiorano, L., Verburg, P. H., Framstadt, E., et al. (2009). Is biofuel policy harming biodiversity in Europe? GCB Bioenergy 1, 18–34. doi: 10.1111/j.1757-1707.2009.01002.x
Elias, S. G., Copeland, L. O., McDonald, M. B., and Baalbaki, R. Z. (2012). Seed testing: Principles and Practices. East Lansing, MI: Michigan State University Press.
Emmerling, C., Schmidt, A., Ruf, T., von Francken-Welz, H., and Thielen, S. (2017). Impact of newly introduced perennial bioenergy crops on soil quality parameters at three different locations in W-Germany. J. Plant Nutr. Soil Sci. 180, 759–767. doi: 10.1002/jpln.201700162
Engeli, H., Edelmann, W., Fuchs, J., and Rottermann, K. (1993). Survival of plant pathogens and weed seeds during anaerobic digestion. Water Sci. Technol. 27, 69–76. doi: 10.2166/wst.1993.0079
Englund, O., Börjesson, P., Berndes, G., Scarlat, N., Dallemand, J.-F., Grizzetti, B., et al. (2020a). Beneficial land use change: strategic expansion of new biomass plantations can reduce environmental impacts from EU agriculture. Glob. Environ. Change 60, 1–13. doi: 10.1016/j.gloenvcha.2019.101990
Englund, O., Dimitriou, I., Dale, V. H., Kline, K. L., Mola-Yudego, B., Murphy, F., et al. (2020b). Multifunctional perennial production systems for bioenergy: performance and progress. WIREs Energy Environ. 9, 1–24. doi: 10.1002/wene.375
França-Neto, J. D. B., and Krzyzanowski, F. C. (2019). Tetrazolium: an important test for physiological seed quality evaluation. J. Seed Sci. 41, 359–366. doi: 10.1590/2317-1545v41n3223104
Fröschle, B., Heiermann, M., Lebuhn, M., Messelhäusser, U., and Plöchl, M. (2015). “Hygiene and sanitation in biogas plants,” in Biogas Science and Technology, eds G. Gübitz, A. Bauer, G. Bochmann, A. Gronauer, and S. Weiss (Cham: Springer International Publishing), 63–99. doi: 10.1007/978-3-319-21993-6_3
Fuerst, E. P., Anderson, J. V., Kennedy, A. C., and Gallagher, R. S. (2011). Induction of polyphenol oxidase activity in dormant wild oat (Avena fatua) seeds and caryopses: a defense response to seed decay fungi. Weed Sci. 59, 137–144. doi: 10.1614/WS-D-10-00123.1
Gasparatos, A., Stromberg, P., and Takeuchi, K. (2013). Sustainability impacts of first-generation biofuels. Anim. Front. 3, 12–26. doi: 10.2527/af.2013-0011
Goldberg, R. B., de Paiva, G., and Yadegari, R. (1994). Plant embryogenesis: Zygote to seed. Science 266, 605–614. doi: 10.1126/science.266.5185.605
Guo, M., Song, W., and Buhain, J. (2015). Bioenergy and biofuels: history, status, and perspective. Renew. Sustain. Energy Rev. 42, 712–725. doi: 10.1016/j.rser.2014.10.013
Hahn, J., de Mol, F., and Müller, J. (2021). Ensiling reduces seed viability: implications for weed management. Front. Agron. 3:708851. doi: 10.3389/fagro.2021.708851
Hassani, M., Vallius, E., Rasi, S., and Sormunen, K. (2021). Risk of invasive Lupinus polyphyllus seed survival in biomass treatment processes. Diversity 13:264. doi: 10.3390/d13060264
Hay, F. R., and Probert, R. J. (2013). Advances in seed conservation of wild plant species: a review of recent research. Conserv. Physiol. 1:cot030. doi: 10.1093/conphys/cot030
Heiermann, M., Herrmann, C., Idler, C., and Starfinger, U. (2010). “Can Ambrosia seeds survive the biogas process?” in Biological Invasions in a Changing World: From Science to Management, eds J. Kollmann, T. van Mölken, and H. P. Ravn (Copenhagen: Copenhagen University).
Heiermann, M., and Plogsties, V. (2018). Schlussbericht, Wildpflanzen-Samen in der Biogas-Prozesskette - Eintrags- und Überlebensrisiko unter dem Einfluss von Prozessparametern“: Teilprojekt 2 (FKZ 22401513). Rostock: University of Rostock.
Herrmann, C., Plogsties, V., Willms, M., Hengelhaupt, F., Eberl, V., Eckner, J., et al. (2016). Methane production potential of various crop species grown in energy crop rotations. Landtechnik 71, 194–209.
Hilhorst, H. W. M. (1998). The regulation of secondary dormancy. The membrane hypothesis revisited. Seed Sci. Res. 8, 77–90. doi: 10.1017/S0960258500003974
Hofmann, D., Uhl, J., Lunenberg, T., Fritz, M., and Marzini, K. (2017). Energiepflanzen für die Biogaserzeugung. Available online at: https://www.biogas-forum-bayern.de/De/Fachinformationen/Substrate/nachhaltig-erneuerbar-energie_EnergiepflanzenfurdieBiogasproduktion.html (accessed September 16, 2019).
Jaganathan, G. K., Yule, K., and Liu, B. (2016). On the evolutionary and ecological value of breaking physical dormancy by endozoochory. Perspect. Plant Ecol. Evol. Syst. 22, 11–22. doi: 10.1016/j.ppees.2016.07.001
Janusch, C., Lewin, E. F., Battaglia, M. L., Rezaei-Chiyaneh, E., and von Cossel, M. (2021). Flower-power in the bioenergy sector – A review on second generation biofuel from perennial wild plant mixtures. Renew. Sustain. Energy Rev. 147:111257. doi: 10.1016/j.rser.2021.111257
Jeyanayagam, S. S., and Collins, E. R. Jr. (1984). Weed seed survival in a dairy manure anaerobic digester. Trans. ASAE 27, 1518–1523. doi: 10.13031/2013.32997
Johansen, A., Nielsen, H. B., Hansen, C. M., Andreasen, C., Carlsgart, J., Hauggaard-Nielsen, H., et al. (2013). Survival of weed seeds and animal parasites as affected by anaerobic digestion at meso- and thermophilic conditions. Waste Manage. 33, 807–812. doi: 10.1016/j.wasman.2012.11.001
Jones, M. (2017). Perennial biomass crops for a resource-constrained world. GCB Bioenergy 9, 4–5. doi: 10.1111/gcbb.12406
Katovich, E. J., Becker, R. L., and Doll, J. (2004). Weed Seed Survival in Anaerobic Digesters. Available online at: www.mnproject.org (accessed February 3, 2020).
Kendig, E. L., Le, H. H., and Belcher, S. M. (2010). Defining hormesis: evaluation of a complex concentration response phenomenon. Int. J. Toxicol. 29, 235–246. doi: 10.1177/1091581810363012
Kozumbo, W. J., and Calabrese, E. J. (2019). Two decades (1998-2018) of research Progress on Hormesis: advancing biological understanding and enabling novel applications. J. Cell Commun. Signal. 13, 273–275. doi: 10.1007/s12079-019-00517-7
Kuhn, W., and Vollrath, B. (2010). Neophyten als Energiepflanzen - Chancen und Risiken. Available online at: https://www.lv-wli.de/files/pdf/Fachbereiche/Bienenweide/NeophytenalsEnergiepflanzen.pdf (accessed May 1, 2022).
Lask, J., Martínez Guajardo, A., Weik, J., von Cossel, M., Lewandowski, I., and Wagner, M. (2020). Comparative environmental and economic life cycle assessment of biogas production from perennial wild plant mixtures and maize (Zea mays L.) in southwest Germany. GCB Bioenergy 12, 571–585. doi: 10.1111/gcbb.12715
Leonhardt, C., Weinhappel, M., Gansberger, M., Brandstetter, A., Schally, H., and Pfundtner, E. (2010). Untersuchungen zur Verbreitungsgefahr von samenübertragbaren Krankheiten, Unkräutern und austriebsfähigen Pflanzenteilen mit Fermentationsendprodukten aus Biogasanlagen: Endbericht zum Forschungsprojekt 100296/2. Available online at: http://www.ages.at/uploads/media/100296_Endbericht_biogas_dafne_letztfassung.pdf (accessed November 1, 2011).
Lorenz, H., Hellwald, K.-H., and Buchenauer, H. (2001). “Untersuchung en zur Inaktivierung von Indikatororganismen (Phytohygiene) in anaeroben Kofermentationsanlagen: Teil 1,” in Untersuchungen zur Seuchen-und Phytohygiene in Anaerobanlagen (Halb-bzw. großtechnische Anlagen), eds A. Knie, R. Haumacher, W. Philipp, W. Martens, and R. Böhm (Stuttgart: Forschungsbericht), 1–76.
Lutts, S., Benincasa, P., Wojtyla, Ł, Kubala, S., Pace, R., Lechowska, K., et al. (2016). “Seed priming: new comprehensive approaches for an old empirical technique,” in New Challenges in Seed Biology - Basic and Translational Research Driving Seed Technology, eds S. D. S. Araújo and A. Balestrazzi (Rijeka: InTech), 1–46. doi: 10.5772/64420
Marcinisyn, E., Peitzmeier, M., and Heckmann, J. (2004). Überprüfung der phyto-und seuchenhygienischen Unbedenklichkeit von Vergärungsrückständen aus der anaeroben Behandlung von Bioabfällen: TV 3 - Praxisuntersuchungen. Abschlussbericht, FuE-Vorhaben FKZ 200 33 331. Hohenheim: Universität Hohenheim.
Mayer, F., Albrecht, H., and Pfadenhauer, J. (2000). “The influence of digestion and storage in silage and organic manure on the germinability of six wees species (Papaver argemone, P. dubium, Legousia speculum-veneris; Centaurea cyanus, Spergula arvensis, Trifolium arvense). J. Plant Dis. Prot. 17, 47–54.
Meyer-Aurich, A., Schattauer, A., Hellebrand, H. J., Klauss, H., Plöchl, M., and Berg, W. (2012). Impact of uncertainties on greenhouse gas mitigation potential of biogas production from agricultural resources. Renew. Energy 37, 277–284. doi: 10.1016/j.renene.2011.06.030
Miller, A. (2014). Tetrazolium Testing. Available online at: https://d3n8a8pro7vhmx.cloudfront.net/aosa/pages/42/attachments/original/1408467811/TZintrolecture2014A.pdf?1408467811 (accessed October 27, 2020).
Milotić, T., and Hoffmann, M. (2016). How does gut passage impact endozoochorous seed dispersal success? Evidence from a gut environment simulation experiment. Basic Appl. Ecol. 17, 165–176. doi: 10.1016/j.baae.2015.09.007
Monfet, E., Aubry, G., and Ramirez, A. A. (2018). Nutrient removal and recovery from digestate: a review of the technology. Biofuels 9, 247–262. doi: 10.1080/17597269.2017.1336348
Müller-Stöver, D. S., Sun, G., Kroff, P., Thomsen, S. T., and Hauggaard-Nielsen, H. (2016). Anaerobic co-digestion of perennials: methane potential and digestate nitrogen fertilizer value. J. Plant Nutr. Soil Sci. 179, 696–704. doi: 10.1002/jpln.201500599
Oechsner, H., Knödler, P., and Gerhards, R. (2018). Bedingungen zur Inaktivierung von Unkrautsamen im Biogasprozess. Available online at: http://docplayer.org/75306345-Bedingungen-zur-inaktivierung-von-unkrautsamen-im-biogasprozess.htm (accessed September 03, 2020).
Overud, S. (2002). Effects of Ensiling on Seed Germinability and Viability in Rumex crispus L. Master’s thesis. Uppsala: Swedish University of Agricultural Sciences.
Papamatthaiakis, N., Laine, A., Haapala, A., Ikonen, R., Kuittinen, S., Pappinen, A., et al. (2021). New energy crop alternatives for Northern Europe: yield, chemical and physical properties of Giant knotweed (Fallopia sachalinensis var. ‘Igniscum’) and Virginia mallow (Sida hermaphrodita). Fuel 304, 1–8. doi: 10.1016/j.fuel.2021.121349
Paparella, S., Araújo, S. D. S., Rossi, G., Wijayasinghe, M., Carbonera, D., and Balestrazzi, A. (2015). Seed priming: state of the art and new perspectives. Plant Cell Rep. 34, 1281–1293. doi: 10.1007/s00299-015-1784-y
Piltz, J. W., Stanton, R. A., and Wu, H. (2017). Effect of ensiling and in sacco digestion on the viability of seeds of selected weed species. Weed Res. 57, 382–389. doi: 10.1111/wre.12269
Powell, A. A., and Matthews, S. (2012). Seed aging/repair hypothesis leads to new testing methods. J. Seed Technol. 34, 15–25.
R Core Team (2021). R: A Language and Environment for Statistical Computing. Vienna: R Foundation for Statistical Computing.
Raghu, S., Anderson, R. C., Daehler, C. C., Davis, A. S., Wiedenmann, R. N., Simberloff, D., et al. (2006). Ecology. Adding biofuels to the invasive species fire? Science 313:1742. doi: 10.1126/science.1129313
Ritz, C., Baty, F., Streibig, J. C., and Gerhard, D. (2015). Dose-Response Analysis Using R. PLoS One 10:e0146021. doi: 10.1371/journal.pone.0146021
Ritz, C., and Streibig, J. C. (2016). R package “drc”: Analysis of Dose-Response Curves. Available online at: https://cran.r-project.org/web/packages/drc/drc.pdf (accessed May 2, 2022).
Rolston, M. P. (1978). Water impermeable seed dormancy. Bot. Rev. 44, 365–396. doi: 10.1007/BF02957854
Ryckeboer, J., Cops, S., and Coosemans, J. (2002). The fate of plant pathogens and seeds during anaerobic digestion and aerobic composting of source separated household wastes. Compost Sci. Util. 10, 204–216. doi: 10.1080/1065657X.2002.10702082
Salnikova, A. A., Slavjanov, A. S., Khrustalev, E. Y., and Khrustalev, O. E. (2019). Environmental effects evaluation of innovative renewable energy projects. J. Environ. Manage. Tour. 10, 100–108. doi: 10.14505//jemt.v10.1(33).10
Sano, N., Rajjou, L., North, H. M., Debeaujon, I., Marion-Poll, A., and Seo, M. (2016). Staying alive: molecular aspects of seed longevity. Plant Cell Physiol. 57, 660–674. doi: 10.1093/pcp/pcv186
Šarapatka, B., Holub, M., and Lhotská, M. (1993). The effect of farmyard manure anaerobic treatment on weed seed viability. Biol. Agric. Hortic. 10, 1–8. doi: 10.1080/01448765.1993.9754646
Schrade, S., Oechsner, H., Pekrun, C., and Claupein, W. (2003). Einfluss des Biogasprozesses auf die Keimfähigkeit von Samen. Landtechnik 58, 90–91.
Simard, M.-J., and Lambert-Beaudet, C. (2016). Weed seed survival in experimental mini-silos of corn and alfalfa. Can. J. Plant Sci. 96, 448–454. doi: 10.1139/cjps-2015-0261
Simberloff, D. (2008). Invasion biologists and the biofuels boom: cassandras or colleagues. Weed Sci. 56, 867–872. doi: 10.1614/WS-08-046.1
Sölter, U., Starfinger, U., and Verschwele, A. (eds) (2016). HALT Ambrosia - Final Project Report and General Publication of Project Findings. Quedlinburg: Julius-Kühn-Archiv.
Stanton, R. A., Piltz, J. W., Rodham, C., and Wu, H. (2012). “Silage for managing weed seeds,” in Proceedings of the 18th Australasian Weeds Conference 2012: Developing Solutions to Evolving Weed Problems, ed. V. Eldershaw (Melbourne, VIC: Weed Society of Victoria), 219–221.
Starfinger, U., and Sölter, U. (2016). “Recommendations on safety of composting or use as biogas fuel of common ragweed seed contaminated material,” in HALT Ambrosia - Final Project Report and General Publication of Project Findings, eds U. Sölter, U. Starfinger, and A. Verschwele (Quedlinburg: Julius-Kühn-Archiv), 50–57.
Strauß, G., Kaplan, T., and Jacobi, T. (2012). Keimfähigkeit von Samen verschiedener (gentechnisch veränderter) Nutzpflanzen in Abhängigkeit von Prozessparametern und Verweildauer in einer Biogasanlage. J. Verbr. Lebensm. 7, 19–25. doi: 10.1007/s00003-011-0756-6
Tanke, A., Müller, J., and de Mol, F. (2019). Seed Viability of Heracleum mantegazzianum (Apiaceae) is quickly reduced at temperatures prevailing in biogas plants. Agronomy 9:332. doi: 10.3390/agronomy9060332
Terboven, C., Ramm, P., and Herrmann, C. (2017). Demand-driven biogas production from sugar beet silage in a novel fixed bed disc reactor under mesophilic and thermophilic conditions. Bioresour. Technol. 241, 582–592. doi: 10.1016/j.biortech.2017.05.150
Traveset, A. (1998). Effect of seed passage through vertebrate frugivores’ guts on germination: a review. Perspect. Plant Ecol. Evol. Syst. 1, 151–190. doi: 10.1078/1433-8319-00057
Turner, J., Stafford, D. A., Hughes, D. E., and Clarkson, J. (1983). The Reduction of Three Plant Pathogens (Fusarium, Corynebacterium and Globodera) in Anaerobic Digesters. Agric. Wastes 6, 1–11. doi: 10.1016/0141-4607(83)90002-1
van Meerbeek, K., Appels, L., Dewil, R., Calmeyn, A., Lemmens, P., Muys, B., et al. (2015). Biomass of invasive plant species as a potential feedstock for bioenergy production. Biofuels Bioprod. Bioref. 9, 273–282. doi: 10.1002/bbb.1539
VDI-Fachbereich Energietechnik (2006). VDI 4630: Fermentation of Organic Materials - Characterization of the Substrate, Sampling, Collection of Material Data, Fermentation Tests. Düsseldorf: Verlag des Vereins Deutscher Ingenieure.
Venendaal, R., Jørgensen, U., and Foster, C. A. (1997). European energy crops: a synthesis. Biomass Bioenergy 13, 147–185. doi: 10.1016/S0961-9534(97)00029-9
Vollrath, B. (2012). Energetische Verwertung von Kräuterreichen Ansaaten in der Agrarlandschaft und im Siedlungsbereich: eine Ökologische und wirtschaftliche Alternative bei der Biogasproduktion. Schlussbericht zum Forschungsvorhaben Nr. 22005308. Veitshöchheim: Bayerische Landesanstalt für Weinbau und Gartenbau (LWG)
Vollrath, B., Werner, A., Kretzer, D., Marzini, K., Illies, I., and Klemisch, M. (2016). Energetische Verwertung von Kräuterreichen Ansaaten in der Agrarlandschaft - eine Ökologische und Wirtschaftliche Alternative bei der Biogasproduktion (Phase II): Schlussbericht. Veitshöchheim: Bayerische Landesanstalt für Weinbau und Gartenbau (LWG).
von Cossel, M. (2020). Renewable energy from wildflowers—perennial wild plant mixtures as a social-ecologically sustainable biomass supply system. Adv. Sustain. Syst. 4, 1–36. doi: 10.1002/adsu.202000037
von Cossel, M., and Lewandowski, I. (2016). Perennial wild plant mixtures for biomass production: impact of species composition dynamics on yield performance over a five-year cultivation period in southwest Germany. Eur. J. Agron. 79, 74–89. doi: 10.1016/j.eja.2016.05.006
von Cossel, M., Pereira, L. A., and Lewandowski, I. (2021). Deciphering substrate-specific methane yields of perennial herbaceous wild plant species. Agronomy 11:451. doi: 10.3390/agronomy11030451
von Cossel, M., Steberl, K., Hartung, J., Pereira, L. A., Kiesel, A., and Lewandowski, I. (2019a). Methane yield and species diversity dynamics of perennial wild plant mixtures established alone, under cover crop maize (Zea mays L.), and after spring barley (Hordeum vulgare L.). GCB Bioenergy 11, 1376–1391. doi: 10.1111/gcbb.12640
von Cossel, M., Wagner, M., Lask, J., Magenau, E., Bauerle, A., von Cossel, V., et al. (2019b). Prospects of bioenergy cropping systems for A more social-ecologically sound bioeconomy. Agronomy 9, 1–32. doi: 10.3390/agronomy9100605
vTI (2009). Biogasmessprogramm II: 61 Biogasanlagen im Vergleich. Available online at: https://edocs.tib.eu/files/e01fb10/62358767X.pdf (accessed May 1, 2022).
Ward, A. J., Hobbs, P. J., Holliman, P. J., and Jones, D. L. (2008). Optimisation of the anaerobic digestion of agricultural resources. Bioresour. Technol. 99, 7928–7940. doi: 10.1016/j.biortech.2008.02.044
Weiland, P. (2010). Biogas production: current state and perspectives. Appl. Microbiol. Biotechnol. 85, 849–860. doi: 10.1007/s00253-009-2246-7
Weitbrecht, K., Müller, K., and Leubner-Metzger, G. (2011). First off the mark: early seed germination. J. Exp. Bot. 62, 3289–3309. doi: 10.1093/jxb/err030
Weißhuhn, P., Reckling, M., Stachow, U., and Wiggering, H. (2017). Supporting agricultural ecosystem services through the integration of perennial polycultures into crop rotations. Sustainability 9:2267. doi: 10.3390/su9122267
Westerik, M., and Kleizen, R. (2006). Onderzoek Sanitatie Tijdens Anaërobe Vergisting ter Bestrijding van Onkruidzaden en Ziektekiemen. Hengelo: HoSt Bio-energy installations BV.
Westerman, P. R., and Gerowitt, B. (2013). Weed seed survival during anaerobic digestion in biogas plants. Bot. Rev. 79, 281–316. doi: 10.1007/s12229-013-9118-7
Westerman, P. R., Heiermann, M., Pottberg, U., Rodemann, B., and Gerowitt, B. (2012a). Weed seed survival during mesophilic anaerobic digestion in biogas plants. Weed Res. 52, 307–316. doi: 10.1111/j.1365-3180.2012.00927.x
Westerman, P. R., Hildebrandt, F., and Gerowitt, B. (2012b). Weed seed survival following ensiling and mesophilic anaerobic digestion in batch reactors. Weed Res. 52, 286–295. doi: 10.1111/j.1365-3180.2012.00918.x
Yang, Y., Tilman, D., Lehman, C., and Trost, J. J. (2018). Sustainable intensification of high-diversity biomass production for optimal biofuel benefits. Nat. Sustain. 1, 686–692. doi: 10.1038/s41893-018-0166-1
Keywords: dose response models, exposure time, flowering wild plant mixtures, hardseededness, physical dormancy, seed survival, seed viability, temperature
Citation: Hahn J, Westerman PR, de Mol F, Heiermann M and Gerowitt B (2022) Viability of Wildflower Seeds After Mesophilic Anaerobic Digestion in Lab-Scale Biogas Reactors. Front. Plant Sci. 13:942346. doi: 10.3389/fpls.2022.942346
Received: 12 May 2022; Accepted: 24 June 2022;
Published: 14 July 2022.
Edited by:
David Horvath, Edward T. Schafer Agricultural Research Center, Agricultural Research Service (USDA), United StatesReviewed by:
Cathal Connolly, Alltech, IrelandJohn Piltz, New South Wales Department of Primary Industries, Australia
Marie-Josee Simard, Saint-Jean-sur-Richelieu Research and Development Centre, Agriculture and Agri-Food Canada (AAFC), Canada
Copyright © 2022 Hahn, Westerman, de Mol, Heiermann and Gerowitt. This is an open-access article distributed under the terms of the Creative Commons Attribution License (CC BY). The use, distribution or reproduction in other forums is permitted, provided the original author(s) and the copyright owner(s) are credited and that the original publication in this journal is cited, in accordance with accepted academic practice. No use, distribution or reproduction is permitted which does not comply with these terms.
*Correspondence: Juliane Hahn, dG8uanVsaWFuZS5oYWhuQHdlYi5kZQ==; orcid.org/0000-0003-2393-0852