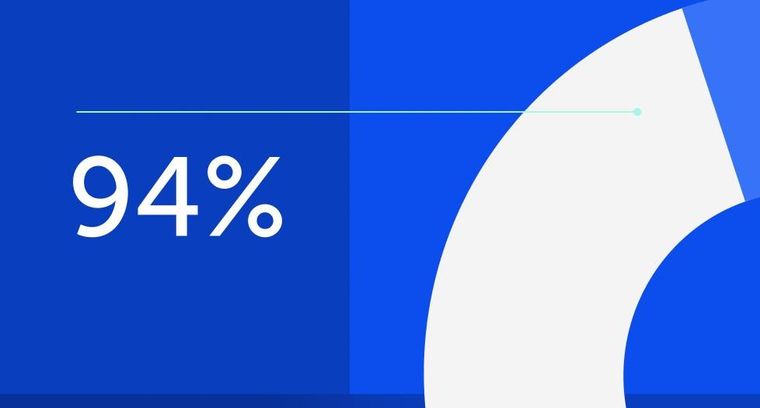
94% of researchers rate our articles as excellent or good
Learn more about the work of our research integrity team to safeguard the quality of each article we publish.
Find out more
REVIEW article
Front. Plant Sci., 06 September 2022
Sec. Plant Abiotic Stress
Volume 13 - 2022 | https://doi.org/10.3389/fpls.2022.936747
This article is part of the Research TopicEnvironmental Extremes Threatening Food CropsView all 37 articles
A pervasive melatonin (N-acetyl-5-methoxytryptamine) reveals a crucial role in stress tolerance and plant development. Melatonin (MT) is a unique molecule with multiple phenotypic expressions and numerous actions within the plants. It has been extensively studied in crop plants under different abiotic stresses such as drought, salinity, heat, cold, and heavy metals. Mainly, MT role is appraised as an antioxidant molecule that deals with oxidative stress by scavenging reactive oxygen species (ROS) and modulating stress related genes. It improves the contents of different antioxidant enzyme activities and thus, regulates the redox hemostasis in crop plants. In this comprehensive review, regulatory effects of melatonin in plants as melatonin biosynthesis, signaling pathway, modulation of stress related genes and physiological role of melatonin under different heavy metal stress have been reviewed in detail. Further, this review has discussed how MT regulates different genes/enzymes to mediate defense responses and overviewed the context of transcriptomics and phenomics followed by the metabolomics pathways in crop plants.
Heavy metals (HMs) stress has emerged as a major problem in a variety of terrestrial habitats around the globe. Due to heavy metals, widespread industrialization negatively influences the crop and soil productivity (Shahid et al., 2015). Metals presence in the soil disturbed the texture of soil, pH, reduces plant growth directly and/or indirectly by interfering with various molecular and physiological activities in crop plants (Hassan et al., 2017; Wang et al., 2019). Metals like Fe, Mn, Cu, Ni, Co, Cd, Zn, Hg, and As have been accumulating in soils for a long time as a result of anthropogenic activities like the waste of different industries, application of fertilizer, sewage disposal and smelting (Zhang and Wang, 2020). Metals are leached into groundwater or accumulated on the soil surface because of these activities (Dağhan and Ozturk, 2015; Hakeem et al., 2015; Basheer, 2018). Toxic metals cannot be removed from the atmosphere by natural processes; so, these metals are non-biodegradable. Some are static, unable to leave the area where they have gathered, while others are mobile and easily absorbed by plant roots (Ali et al., 2015b, 2018; Dehghani et al., 2016; Burakova et al., 2018).
Bioactive metals are classified into two classes based on their physicochemical properties: redox metals such as Mn, Cu, Fe, and Cr, and non-redox metals such as Zn, Cd, Al, Ni, and Hg (Jozefczak et al., 2012). By undergoing Haber-Weiss and Fenton reactions, redox metals can directly cause oxidative injury in plants, breakage of DNA strands, destruction of cell homeostasis, defragmentation of protein, photosynthetic pigment damage and, damage of cell membrane which can lead to death of cell (Flora, 2009). Non-redox active metals, on the other hand, trigger oxidative stress indirectly through a variety of mechanisms, including glutathione depletion, protein sulfhydryl group binding (Jozefczak et al., 2012), antioxidative enzymes inhibition, and induction of ROS-producing enzymes such as NADPH oxidases (Bielen et al., 2013). Plants use a variety of mechanisms to protect themselves from different sources of metals by developing tolerance. The cutting edge “omic tools” are an excellent model for understanding plants molecular mechanism of tolerance/susceptibility under the impact of various stresses, especially under HMs (Wang et al., 2020). Omic approaches used for HMs stress mainly include transcriptomics, proteomics, metabolomics and ionomics (Liu et al., 2020). Another mechanism is exclusive, meaning plants are only enabled when a certain level of metal toxicity is present. All responses fall into two categories: avoidance or tolerance (Krzesłowska, 2011; Shahid et al., 2015). Metabolomics, proteomics, and transcriptomics are common omics approaches used to interpret regulatory networks involved in responding to HMs tolerance in plants (Singh et al., 2016). The above-mentioned omics approaches, when combined with various functional genomic approaches, help to produce such verities which have the ability to tolerate against various abiotic stress conditions (Mosa et al., 2017). Plant growth regulators are chemical substances that control all aspects of plant production and growth (Ali et al., 2013a, 2013b). Several different plant growth-promoting rhizobacteria (PGPRs) have been used in the past to try to achieve efficient plant growth under abiotic stress (Ali et al., 2013a, 2013c).
Plant hormones (phytohormones) work as a messenger which can be either natural or synthetic chemicals, that control growth and development in response to environmental cues and are much effective even at very low absorptions (Rubio et al., 2009). Phytohormones work in various forms and activate various processes, including cell division (cytokinins) and cell development (auxins). Interestingly, plants have evolved several signaling pathways to overcome various stresses. One of them is mitogen-activated protein kinase (MAPK) synthesis (Arnao and Hernández-Ruiz, 2015, 2020). MAPK production regulates several critical stress-related hormones, genes, and chemicals that ultimately defend plants to survive against abiotic stresses (Arnao and Hernández-Ruiz, 2015). Plant hormones have been shown to promote the growth of non-plant microorganisms such as bacteria and fungi in many studies (Chatterjee et al., 2008; Levy et al., 2018; Kunkel and Johnson, 2021). They significantly affect on different physiological processes such as plant growth, development, and movement. Despite extensive research on the topic, no major breakthroughs have been made until now. The most well-known and essential phytohormones are melatonin, indole-3-acetic acid, gibberellic acid, kinetin, 1-triacontanol and abscisic acid.
Melatonin (MT, N-acetyl-5-methoxytryptamine) is a multi-regulatory chemical involved in seed germination, root growth, fruit ripening, senescence, yield, circadian rhythm, and stress response. Practically, it is found in all plant species (Hernández et al., 2015; Sun et al., 2015; Hardeland, 2016) and it is known as growth promoter and rooting agent (Chen et al., 2009a; Sarrou et al., 2014; Zhang et al., 2021). It plays a significant role in plant stress defense in addition to its role in plant growth. Plants can be exposed to stressful environmental conditions regularly. Plant species high in MT have been shown to have a higher stress tolerance ability. Exogenous therapy or ectopic overexpression of MT biosynthesis genes may also improve tolerance to a range of stressors, such as high temperatures, dehydration, salinity, radiation, and chemical challenges, all of which can produce the reactive oxygen species (Zhang et al., 2015). In this review, we have tried to compile all the knowledge about melatonin regulatory effects in plants and physiological functions of MT under different heavy metal stresses. Keeping in mind the importance of MT and its role in alleviating heavy metal stress in plants, recent knowledge about how MT regulates different genes/enzymes in response to abiotic stress, as well as the transcriptomics and phenomics background of MT in crop plants under stressful conditions, is presented in a trendy manner.
Melatonin is a low molecular weight indole molecule found in all kingdoms of life, from prokaryotes to eukaryotes, from animals to plants (Arnao and Hernández-Ruiz, 2014). It was first discovered as an essential animal hormone involved in antioxidant acts, reproduction, circadian cycles, and innate immunity, among other biological processes (Shi et al., 2015). Since, the discovery of MT in Japanese morning glory (Pharbitis nil) in 1993, much progress has been made in understanding the function of melatonin in plants (Sun et al., 2021). Different studies have indicated that melatonin has unique physiological properties in plants, such as growth promotion and rhizogenesis induction, functioning similarly to indolyl-3-acetic acid (IAA) and auxin (Arnao and Hernández-Ruiz, 2014). Melatonin protects plants from abiotic stimuli such as HMs, UV radiation, salt, drought, and ambient temperature and contributes to the aging of leaves (Reiter et al., 2015). Melatonin is thought to have a primary role in plants as it operates as the first line of defense against internal and external oxidative stress by scavenging the ROS (Park et al., 2013; Arnao and Hernández-Ruiz, 2015; Altaf et al., 2021a). The nitrogen in the phytomelatonin molecule’s carbonyl group triggers the development of a new five-membered ring after it interacts with ROS (Kaur et al., 2015). Further, it enhances plant resilience to environmental challenges such drought, salt, cold, and oxidative stress, as well as delaying leaf senescence, whether exogenously administered or endogenously created (Arnao and Hernández-Ruiz, 2014; Zhang et al., 2014). It has also been considered as a broad-spectrum antioxidant and an endogenous free radical scavenger. It eliminates a variety of free radicals and ROS, such as nitric oxide (NO), peroxynitrite anion (NO3−), hydroxyl radical (OH−), and singlet oxygen (O2−). One of the most attractive features of this molecule, which sets it apart from most antioxidants, is its metabolites can also scavenge ROS and nitrogen species (Kwon et al., 2021).
Exogenously applied MT has various effects, from substantial improvement to ineffectiveness or toxicity. The disagreement among researchers is due to the concentration used. At low and high doses, MT can have diverse roles in controlling plant growth and development in the same species. A low dose of MT (0.1 mM) promotes the root growth in wild leaf mustard (Brassica juncea), whereas a high dose (100 mM) inhibits its growth (Chen et al., 2009b). At low quantities, it promotes roots in cherry tissue culture, but at higher doses, it inhibits the root development (Sarropoulou et al., 2012). The high concentrations employed, specifically, 100 mM, could never be achieved in any plant because plant MT levels range from picograms to micrograms per gram of tissue. These values are several orders of magnitude higher than those in the human body. The toxic effects can occur when concentrations are too high. Melatonin levels were found to have a varied effect at low and high levels. However, high MT did not control any of the genes regulated by low MT (Weeda et al., 2014). This implies that MT can have various effects at low and high concentrations. At high concentrations, melatonin can drastically suppress ROS in cells, affecting ROS-dependent signaling and inhibiting cell development (Afreen et al., 2006). Figure 1 shows the graphical presentation of how MT alleviates abiotic stress in plants.
Since, MT is soluble in both water and lipids and rapidly flows throughout the body to any watery area, it is largely employed as an antioxidant (Tan et al., 2013). However, many scientists have suggested that MT promotes plant growth in general (Arnao and Hernandez, 2019a). It has been found to increase the coleoptile length in canary grass, barley, and wheat (Hernández-Ruiz et al., 2005). Previously, maize seeds treated with MT showed greater seed vigor and quality and increased seed storage proteins (Kołodziejczyk et al., 2016). Another study found that coating soybean seeds with MT substantially boosted leaf development, plant height, number of pod plants−1, and number of seeds pod−1 (Wei et al., 2014). Melatonin has been demonstrated to play a comparable role in the promotion of vegetative growth and the regeneration of lateral and adventitious roots in etiolated Lupinus albus (Hernandez-Ruiz et al., 2004; Arnao and Hernández-Ruiz, 2007). While, treatment with MT resulted in increased seedling development, enhanced nutrient uptake efficiency, and improved nitrogen metabolism in cucumber plants, particularly under salt stress conditions (Zhang et al., 2017). According to recent investigations, MT administration also boosted photosynthetic activity, redox homeostasis, root growth and development, and seminal root elongation in barley, wheat, sweet cherry, and rice (Li et al., 2016; Liang et al., 2017; Zuo et al., 2017). Another potential element of MT application is its positive influence on photosynthesis and other growth-related parameters among different crops under various abiotic stress conditions (Meng et al., 2014; Wang et al., 2016a). Auxin, ethylene, cytokinin, gibberellins, IAA (indole 3-acetic acid), and brassinosteroids are all plant hormones that play an important role in maintaining growth and development of crop plants (Denancé et al., 2015). However, exogenous MT application can be used to modulate the effects of these plant hormones (Arnao and Hernández-Ruiz, 2017). Under salt conditions, exogenous MT dramatically increased the levels of endogenous abscisic acid (ABA) and gibberellic acid (GA) in cucumber seedlings, resulting in better salinity resistance (Zhang et al., 2014). The amount of cytokinin is steadily degraded in plants subjected to heat stress. However, once the plants treated with exogenous MT, an increase in the level of cytokinin biosynthesis can be detected, and therefore the increased cytokinin level in the melatonin-treated plants results in enhanced resilience to heat stress (Zhang et al., 2017). In a nutshell, the mechanistic principal of melatonin is to boost the antioxidant defense system; while, also increasing photosynthetic activity. Furthermore, several experts have claimed that MT has a major impact on total plant growth in the face of abiotic challenges with the least amount of impact on the environment (Tiryaki and Keles, 2012; Fan et al., 2015; Zhang et al., 2017; Ali et al., 2021).
Indole acetic acid is comparable in structure and function to the others (Hernández-Ruiz et al., 2005; Pelagio-Flores et al., 2012). In line with this, exogenous MT therapy boosts IAA synthesis (Wang et al., 2016b). On the other hand, both MT and IAA have been shown to enhance root development in a combined and similar manner (Yang et al., 2021). Exogenously administered MT increased IAA levels in Brassica juncea plants, resulting in improved root activity (Chen et al., 2009a). In Mimosa pudica, it showed a favorable effect on root organogenesis (Sun et al., 2021). Hence, MT is thought to play a significant role in plant’s physiological and biological processes. To summarize, MT can be considered a biological plant growth regulator that boosts a plant’s production capability. The concise plant growth alterations by MT in response to HMs stress are given in Table 1.
Table 1. Ameliorative effects of MT supplementation on growth, physiological, and biochemical attributes of plants grown under heavy metals toxicity.
Ionomics is the study of accumulating metalloids, non-metals and metals in living organisms, regarding the accumulated minerals, could be essentials and nonessential (Ayub et al., 2021). Plant ionomics has applied for various kinds of research, such as physiological, evolutionary, and ecological. Furthermore, the elemental analogs measuring can improve data quality with ionomic approaches (Watanabe et al., 2007; Chen et al., 2009b; Campos et al., 2021).
The macro and micro elements present in the plants which efficaciously promoted plants growth and many others boosted plant’s activities at metabolic and physiological levels. Various biotic and abiotic stress conditions can disturb plant behaviors physiologically and genetically. Therefore, these macro and micro elements should be affected by these changes. Thus, ionomics study has provide a reliable presumption regarding the ion concentration changes and various other pathways which control the elements in plant’s body. This accomplished that ionomics can be categorized as a superior tool for distinguishing amendments in the plants physiology with interrelation of environment (Kanwar et al., 2018). Besides that, plant ionomics can be associated to the difference of phylogenetic relationships (Watanabe et al., 2007). The ionomics application (use of mutants) has focused on isolation and gene characterization which were responsible for homeostasis and transmission of different plant elements (Chao et al., 2011; Duan et al., 2017). However, the most important benefit of ionomics implementation in plants has to enhance acomprehensive perception of elemental dynamics.
To reduce the toxicity of HMs, ionome (mineral nutrients) plays a major role, including N, P, K, Ca, S and Mg; along with some trace metals such as Fe, Cu, Mn, Mo, Co, and Zn. Nitrogen (N) is the main essential nutrient, composed of hormones, nucleic acids, proteins, and vitamins. It can alleviate the toxicity of heavy metals by promoting the photosynthetic competency by increasing the chlorophyll synthesis, boosting N-comprised metabolites such as GSH, proline and by increasing of antioxidant enzymatic activities (Lin et al., 2011). The other essential nutrient is phosphorus (P), comprised of nucleic acids and cell membrane, and especially imperative for reaction of phosphorylation. Phosphorus (P) can alleviate metal toxicity by diluting and decreasing metal mobility through metal phosphate complex (Sarwar et al., 2010). Additionally, P can also contribute in the prevention of membrane damage and increase the level of GSH content, thus conferring plant tolerance against metal stress (Wang et al., 2009a). On the other hand, calcium (Ca) has been involved in regulating metabolic activities as well as the intracellular and channel-based Ca-binding sites of Cd2+ and Ca2+ (Lauer Júnior et al., 2008). Further, Ca can take the place of the Cd that is present in the outside medium. Hence, plant development has been influenced, although Ca has shown a reduction in heavy metal-induced deficiencies (Farzadfar et al., 2013). In another study, Khan et al. (2009) reported that AsA–GSH cycle enhanced by 40 mg. S. Kg−1, thereby in mustard plant Cd toxicity reduction was obtained by Anjum et al. (2008). The accumulator plant species were more competitive against the stressed caused by Na, Ni and As based on the different adaptation strategies for excessive stress of these elements, which might form an enlarge variations of their concentration between various kinds of species (Pollard et al., 2014; White et al., 2017). Researchers have revealed that endogenous/exogenous application of MT could contribute in various plant species under salinity environment to enhance K+/Na+ homeostasis (Li et al., 2012). Most of scholars have investigated the strong correlation among salt tolerance and cellular K+ retention of the different kinds of plants, namely sweet potato (Yu et al., 2016), wheat (Cuin et al., 2008), halophytes (Bose et al., 2015), Brassica (Chakraborty et al., 2016), and poplar (Sun et al., 2009b).
The influence of MT on ionomics has been reported in a recent study (Yu et al., 2018a). It was determined that depletive salt effect on the contents of Mg2+, K+, and Ca2+ in the different tissues reversed as MT exogenous application (root/leaf: 0.5/100 mM). Interestingly, MT distinctly reduced the Na+ significantly in the leaf tissues of Xu-32 under the salinized conditions but in the stem and root tissues Na+ content was increased and, MT in the absence of NaCl stress did not show any modifications of elemental levels (Yu et al., 2018b). Additionally, in sweet potato, MT maintained the K+/Na+ homeostasis, contributed to PM HC–ATPase activity and enhanced the energy state.
The effect of water deficits was evaluated on enzymes activities and genes transcriptional abundance included in N metabolism (Meng et al., 2016; Huang et al., 2018a,b). However, MT effect on the regulation of transcriptional genes in terms of N metabolism, uptake, and reduction under the drought stress thoroughly has not been studied. Previously, Dijkstra et al. (2015) investigated the absorption and transformation of N by implementing the 15N tracers. Therefore, Liang et al. (2018) applied some tracers under drought conditions to estimate MT impact on the nutrients uptake in plants. The exogenous MT has a modulating effect on the elements of plants and stress mitigation with the help of regulating those elements. For example, MT improved the reductions in Zn, Mg, Fe, Cu, Mn, K, P, and S concentrations. Meanwhile, it further increased the concentration of Ca and B in maize seedlings at low temperature conditions (Turk and Erdal, 2015). Thus, MT could be the most important hormone which can be helpful for plants to improve the drought tolerance by uptake process of mineral elements. This review proposes that the positive effect of MT prefers more opportunities related to agriculture, plants can be developed with higher drought tolerance and promotes an adaptation capacity for future environmental problems.
Plant functional genetics provides new horizons for studying the molecular mechanisms behind the production of critical regulatory molecules (Thao and Tran, 2016; Abdelrahman et al., 2018). Transcriptomic approaches involve the study of the “transcriptome” of an organism. The transcriptome is a set of entire RNA molecules expressed under a particular circumstance spatially and temporally (Milward et al., 2016). Understanding the plant functional genetics by post-translational studies is getting routine research after the high-throughput sequencing technologies became approachable and much cheaper than before. These studies have a great promise to provide critical information about the molecular mechanism of synthesis, regulation, and metabolic pathways of action of products, by-products, and various critical regulatory molecules in plants (Pang et al., 2021). Transcriptomic approaches are one of the main pillars of molecular genetics, by the wealth of which scientists can determine real-time changes happening after the translation of genetic information imprinted in the plant cells (McGowan and Fitzpatrick, 2020).
Transcriptomic studies cover all aspects of RNA transcripts, their synthesis, expression, and their regulation. These studies also include their trafficking, structures, splicing patterns (mediated by spliceosome), and modifications at the post-transcriptional level (Liang, 2013). There are several types of transcripts; out of them, messenger RNA (mRNA), small RNA (sRNA), long noncoding RNA (lncRNA), and micro-RNA (miRNA) are remarkable (Milward et al., 2016). With the advent of cheap sequencing technologies, we can use these high-throughput methods for the expression analysis of transcripts under the particular conditions (Wang et al., 2009b). This knowledge is prime for linking phenotype to genotype. Several studies are available that reports transcriptional changes in plants under the specific physiological or pathological circumstance.
Plants are consistently encountered by various biotic and abiotic stresses (Małkowski et al., 2019). Some of these stresses are natural, while some result from anthropogenic activities. Heavy metals (HMs), the prime source of toxicity in plants, are being prolonged accumulated in soils through various activities (Chai et al., 2019). These activities include improper disposal of industrial waste, extensive fertilizers, non-treated sewage disposal to agricultural soil (Chai et al., 2019; Lominchar et al., 2019). These activities result in immobile, non-biodegradable, and toxic levels of HMs on the soil surface and ground water (Ahmad et al., 2016). Plants can take heavy metals and lead to toxicity in them (Mustafa and Komatsu, 2016). Plants can uptake HMs by different biological processes by their roots, using either diffusion, endocytosis, or metal transporters for this task (Zhao et al., 2021). Plants are also appreciated as bio-accumulators of HMs (Chaffai and koyama, 2011; Nawaz et al., 2016). Nevertheless, some metals are essential for plants, but their accumulation, when exceeding a specific limit, causes toxicity (Zhao et al., 2019). If animals eat these plants, toxicity can also be carried to their bodies, which can be poisonous in several ways. Effects and symptoms of HMs toxicity in plants include chlorosis, reduced growth, root death (browning), and plant wilting (Varma, 2021).
Ever since discovering melatonin in plants, research related to its role in plant homeostasis and regulation of stress-related signals is going on in many laboratories of the world (Kanwar et al., 2018). Its exogenous application is also remedial against infection from various pathogens. There are direct and indirect references that MT involvement in disease resistance in plants. However, its synthesis and regulation at the transcriptomic level is rarely summarized (Hoang et al., 2017; Abou-Elwafa et al., 2019; Liu et al., 2019). Here, this article will present a comprehensive overview of transcriptomic approaches used to understand the molecular genetics of phytomelatonin production, its regulation and involvement in plant regulatory mechanisms.
The cutting edge “omic tools” are an excellent model for understanding plants molecular mechanism of tolerance/susceptibility under the impact of various stresses, especially under HMs (Wang et al., 2020). Omic approaches used for HMs stress mainly include transcriptomics, proteomics, metabolomics and ionomics (Liu et al., 2020). The transcriptome is an entire set of transcripts, transcripted primarily in the form of mRNA, form the genome of an organism under certain conditions. Proteomics is the study of expressed proteins, their modification after translation, their localization, mode of action and their docking, translated from the transcriptome of an organism under a defined environmental condition (Abou-Elwafa et al., 2019). Metabolomic studies include identification, characterization, and quantification of metabolites (produced by cellular regulation, under the effect of external stimuli) and all metabolomic activities, and due to translated proteins, in the effect of conditions provided to plants, likewise economics is the quantitative measurement of production, accumulation of ions in an organism under the effect of external stimuli (Mustafa and Komatsu, 2016). Several biochemical pathways are directly and indirectly activated/suppressed after signal transduction of HMs in plants (Mustafa and Komatsu, 2016; Yu et al., 2021b).
Plants produce differential expression of a set of the transcriptome under the abiotic stress of HMs, which is of significant interest for determining the genes involved in HMs tolerance and susceptibility (Duhan, 2021). RNA sequencing (RNA-seq) is an essential investigation to elucidate the regulation of gene expression at the molecular level. RNA-seq provides data about differential expression of genes and exposes critical biological processes involved in tolerance mediated by plants for HMs (Shahid et al., 2014; Lee and Back, 2017a). Several studies reported that the large set of genes were involved directly or indirectly in tolerance of HMs. These genes are either directly involved in signal transduction to produce metabolites that result in tolerance or indirectly regulate the production of other transcripts (Lee and Back, 2017b; Duhan, 2021). In broad terms, HMs stress-induced genes (transcripts) can be classified based on their functionality and two distinct groups can be made; one includes the functional genes, and the other is regulatory genes. The functional group of the transcript as a single or whole (without inducing the effect of another gene) encodes important compounds which play a critical role in the tolerance against HMs (Nawaz et al., 2016; Wilson et al., 2019). The functional group includes transcripts that mainly encode sugars, alcohols, and several amines. The second class of transcripts includes the stress-related gene involved in the regulatory network of different transcription factors (TFs) which work in groups or individually and involved in the regulation that led to HMs stress tolerance (Table 2; Wilson et al., 2019). Hence it comprises of gene network and has much more importance in understanding the broader picture. These TFs are often found in clusters in the plant genome and belongs to the multi-gene family (Table 2). These genetic regulators have unique expressional control of a single to several genes by binding at a specific site in promotor, acting as a cis-acting binding element to induce transcription. Otherwise, the gene is not expressed anyway (Cheng et al., 2018). This DNA binding class has a unique protein domain that acts as a cis-regulatory element via protein-to-protein interaction, which ultimately results in oligomerization of these transcriptional factors with several other regulatory genes (Wilson et al., 2019). This transcriptional complex-conjugate system is often referred as “regulon.”
Table 2. TFs in response to heavy metal stresses (Li et al., 2022).
A strong connection exists between raised MT levels and HMs stress tolerance (Zhang et al., 2022). MT greatly scavenges the reactive nitrogen species (RNS) along with reactive oxygen species (ROS; Gao et al., 2020), that produced excessively during routine photosynthesis process and respiration, as a prime functionality of plants. Plants critically utilize raised melatonin levels for scavenging elevated levels of RNS and ROS, hence providing tolerance to HMs stress otherwise which causes severe damage (Aslam et al., 2017; Lominchar et al., 2019; Zhang et al., 2020). The production of MT is also consistent with the upregulation of genes that produce stress-tolerant enzymes. These enzymes include serotonin N-acetyltransferase (SNAT), tryptophan hydroxylase (TPH), N-acetylserotonin O-methyltransferase (ASMT) and tryptophan decarboxylase (TDC), that are constantly produced in HMs stress tolerant plants. Fungal specie, i.e., Exophiala pisciphila, highly tolerant to HMs stress was isolated from smelting site of old mine in China. Yu et al. (2015) also reported that this specie’s transcriptomic analysis proved MTs involvement in the Salinity stress. ASMT, SANT and TDC are the enzymes regulating the biosynthesis of MT, were significantly upregulated under the stress of HMs like Cd (Chandramouli and Qian, 2009), indicating direct evidence of the connection of melatonin in HMs stress tolerance. It strongly suggests that melatonin has positive involvement with the enhanced HMs stress in plants. Figure 2 shows the MTs structure and signal transduction of HMs for its production using SNAT, TPH, ASMT, and TDC enzymes.
Figure 2. Overview of signaling pathways of melatonin and its metabolites (Back, 2021). MT activates MAPK cascade through OXI1/MAPKKK3–MAPKK4/5/7/9–MAPK3/6. MAPK activation induces translocation of the SA receptor NPR1 into the nucleus to interact with several transcription factors, resulting in abiotic stress tolerance. Exogenously applied MT attenuates endoplasmic reticulum stress damage by increasing the expression of BIP2, BIP3 and CNX1 genes through the bZIP60 transcription factor. Kinase (RLK) works as a MT receptor responsible for further activation of the MAPK cascade. ROS burst occurs from RBOH under stress conditions. Thus, ROS are powerful inducers of de novo melatonin biosynthesis. MT further metabolizes into AFMK, AMK, 5-MT, 2-OHM, 3-OHM, which are potent antioxidants. Melatonin and its metabolites efficiently scavenge a range of ROS/RNS to maintain cellular ROS balance. Unlike ROS-mediated MAPK activation upon stress (Jalmi and Sinha, 2015), melatonin-mediated MAPK activation is independent of ROS, indicating that melatonin functions downstream of the ROS burst (Lee and Back, 2017a). In this figure, solid arrows indicate confirmed functions; dashed arrows indicate steps not yet demonstrated.
Several transcriptional families (TF) families are characterized in response to abiotic stresses in plants like HMs stress (Table 2). Here we provide a critical link between the production of MT and the contribution of these TFs families in response to HMs stress. Several TFs families are involved in MT production as a stress reliever in the plants under HMs stress (Liu et al., 2020). The transcriptional families that are greatly influenced by HMs stress are AREB/ABF, MYB, AP2/EREBP, WRKY, bHLH, bZIP, MYC, HSF, DREB1/CBF, NAC, HB, ARID, EMF1, CCAAT-HAP2, CCAATDR1, CCAAT-HAP3, CCAAT-HAP5, C2H2, C3H, C2C2-Dof, C2C2-YABBY, C2C2-CO-like, C2C2-Gata, E2F-DP, ABI3VP1, ARF, AtSR, CPP, E2F-DP, SBP, MADS, and TUB (Liu et al., 2020). These TFs are also famous for other abiotic stress inductive, and some of them also play a significant role in biotic stress tolerance. These TFs are regulon of various transcriptional activities in the broader picture after HMs stress induction in plants. In addition, these transcriptional activities lead to stress tolerance or stress resistance in plants. Table 2 represents the summary of TFs involved in the regulation of HMs stress in plants.
Comparative genomic approaches have provided critical inputs for crop improvement, especially by identifying biotic and abiotic stress related genes, quantitative trait loci (QTLs) and figuring out metabolic pathways (Frukh et al., 2020). Melatonin synthesis is directly related to heavy metals stress tolerance and critical for plant survival. Severe loss to crop yield was observed due to HMs stress and, more importantly, cause serious health issues when HMs accumulated plants were eaten up by animals or humans. Genomic approaches use various tools to identify key regulatory genes that are more or less associated with plant melatonin synthesis under the devastating stress of HMs, hence play an important role in plant physiology (Rajasundaram and Selbig, 2016). These approaches are also helpful in understanding the underlying molecular and physiological mechanisms of HMs tolerance. Genomics involve genome-scale studies of genetically diverse plants, using several genome wide transcriptomic approaches, gene expression analysis at the whole genome level, and discovery of novel genes related to various stresses like HMs.
Melatonin synthesis at genome level is controlled by a complex network of enzymes (Singh et al., 2016). Tryptophan, which is an essential amino acid, is responsible for melatonin production using six enzymes. These critical enzymes are tryptophan decarboxylase (TDC), TPH, tryptamine 5-hydroxylase (T5H), serotonin N-acetyltransferase (SNAT), acetylserotonin-Omethyltransferase (ASMT), and caffeic acid O-methyltransferase (Tripathi and Poluri, 2021). The genes responsible to produce these enzymes have been characterized and cloned for several plants. Very first SNAT gene was mapped in rice, which is also a model plant besides Arabidopsis. Several studies reported that SNAT was the penultimate enzyme and involved in final steps of melatonin production (Zhan et al., 2019). As many plants are whole genome sequenced, and researchers have benefited from the availability of high-quality genomic data to find homologs of these enzymes. SNAT homolog was also reported in other species, including alga laver, cyanobacteria, apple, Arabidopsis, grapevine (Tripathi et al., 2021). Furthermore, genome-wide studies have revealed that presence of these enzymes is diversified, with varied in frequency of existence in different plants.
Serotonin N-acetyltransferase is a regulatory enzyme for MT production as it maintains the production level of MT in response to stress (Reiter et al., 2015). Comparative genomic approaches revealed that different species have varied modular activities of SNAT, and it has distinct thermophilic properties. SNAT is primarily regarded as heat resistant enzyme having different temperatures for its catalytic activity in different plant species. Thus, it is anticipated that its part in heat stress tolerance, providing evidence of the role of melatonin in abiotic stress tolerance. Furthermore, ectopic overexpression of MzSNAT5 in Arabidopsis reported increased melatonin concentration and improve drought tolerance. Moreover, when the SNAT production in rice was suppressed, the adverse effects were observed on plant growth, and low melatonin levels (Tripathi et al., 2021). It was certain that low melatonin levels have penalty of increased susceptibility to abiotic stresses and ultimately low yield levels. Melatonin, not only involved in abiotic stress tolerance but also correlated with biotic stresses. Bio-synthetic inhibition of GhSANT1 and other melatonin related genes have compromised the resistance conferred by cotton against phytopathogenic bacteria. Thus, SNAT is an essential compound controlling melatonin synthesis in plants and has a significant role in abiotic stress and biotic stresses (Jayarajan and Sharma, 2021).
Proteomics emerged as cutting-edge tool for understanding the synthesis, functionality, and expressional characterization of proteins at whole genome level (Yu et al., 2018a; Wang et al., 2018). Proteomic studies elucidate differential expression of proteins under various stresses like HMs and characterize them at cell, organ and tissue level as well as provide insights into network modulation of related proteins. Hence, emulating structural models identify HMs stress-tolerant material in other species (Pardo-hernández et al., 2021). Using proteomic approach is one of the prime studies for understanding the fate of a compound and its molecular mechanism of action, interaction at post translational level (Pardo-hernández et al., 2021). This involves whole protein level research related to our molecule of interest. Although genomic analyses significantly contributed to our understanding of basic gene functionality and how genes are translated to proteins, many puzzles for protein fate remained a challenge until we started to study whole proteins at genome level. This is because although the gene is transcribed and translated into protein, but the protein stability, folding, interaction with other proteins, and localization are critical for its functionality. Hence, in depth proteomic studies elucidate the target proteins (Rajasundaram and Selbig, 2016; Tripathi and Poluri, 2021) that directly or indirectly take part in melatonin production, provide interaction pathways, and provide better understanding on HMs stress tolerance.
Direct involvement of proteins in HMs stress tolerance/susceptibility is well known, as tolerance involves proteomic changes in plants. Therefore, with the use of proteomic approaches in plants, we exploited proteins involved in regulating HMs stress (Sage and Kubien, 2007). Furthermore, these studies helped a lot for deciphering the proteomic signals for perception of stress and initiation of signaling cascade that ultimately, with help of network changes at transcriptional and metabolomic level, provides tolerance against HMs-induced phytotoxicity (Mateos-Naranjo et al., 2008; Zhan et al., 2019). It has been categorized as indoleamine compound, synthesized as derivate of tryptophan (Yu et al., 2018a; Kanwar et al., 2018). Tryptophan is dominantly found in almost all higher plants. Besides providing tolerance against HM stress, melatonin also provides tolerance against various other abiotic stresses, like salinity, chilling, and osmatic stresses (Borges et al., 2019; Peharec Štefanić et al., 2019). Many researchers are working on for elucidating the molecular biochemistry of melatonin at proteomic level for its mitigation potential against HMs stress. Melatonin has been reported to improve antioxidant levels in diverse plants like wheat, tomato, and apple, hence reducing the ROS damage, providing tolerance to various stresses (Yu et al., 2018). This review offers insights how melatonin provides tolerance against HMs stress at proteomic level.
Reactive oxygen species (ROS)-scavenging proteins are critical for inducing tolerance against HMs stress. It was observed that under HMs stress, tolerant plants have an abundance of ROS scavenging proteins that performs series of chain reactions to MT homeostasis (Borges et al., 2019). Several metabolic pathways are significantly modulated under HMs stress like rate of respiration, metabolism of sulfur and nitrogen, and rate of photosynthesis. Under HMs stress, the plant power producing apparatus boosts the production of reducing agents like FADH2, NADPH and NADH, which lead to higher production of ATP (energy molecule of plants) to provide HMs stress tolerance. ROS scavenging is also correlated with higher production of reducing agents and increased melatonin production. For example, exogenous application of MT to HMs stressed plants have improved osmoregulation and increment in photosynthetic rate, antioxidants, and carotenoids compounds (Yu et al., 2018b). Heavy metals stress tolerance has evolved many key regulatory processes due to the elevation of MT in planta. These compounds like 1, 2 oxygen enhancer protein (OEP), large subunit binding proteins of RUBISCO, NADPH oxidoreductase and I and II-photosystem proteins were found with a significantly different expression during the HMs toxicity. Interestingly, these proteins are also regulatory to produce MT (Yu et al., 2018,b; Pardo-hernández et al., 2021).
Phytomelatonin has been found to be present in all plants, which has an important role in plants as a biostimulator that improves plant tolerance to both biotic and abiotic stress. The first layer of tolerance in HMs stress tolerance is the signal reception of HMs toxicity then transduction to other cells for HMs stress response. This fundamental communication in response to HMs stress is mediated by protein cipher, which plays a prime role in intracellular signal transduction, and activating signaling cascade. Proteomic analyses of various plants under HMs stress deciphered several molecular mechanisms underlying this cascade in the form of variation in protein level that enable or unable plant species for stress tolerance This work is of critical importance for developing stress-tolerant plants. However, a detailed study involving antioxidant mechanism, metals-regulated differential gene expression, and mineral transporters is needed to understand complex plant responses to metal toxicity.
SA, MS, MK, BA, RG, and WZ designed the manuscript. SA, SAh, NZ, MR, and WZ wrote the manuscript. MN, RF, BA, RG, MS, and WZ revised the manuscript. All authors contributed to the article and approved the submitted version.
This work was supported by the Science and Technology Department of Zhejiang Province (2022C02034), Collaborative Innovation Center for Modern Crop Production co-sponsored by Province and Ministry (CIC-MCP), and the Agriculture and Rural Affairs Department of Zhejiang Province (2022SNJF010).
We thank the Ministry of Agriculture and Rural Affairs Key Laboratory of Spectroscopy Sensing, Rui Sun and Weizhen Hu from Agricultural Experiment Station of Zhejiang University for their assistance.
The authors declare that the research was conducted in the absence of any commercial or financial relationships that could be construed as a potential conflict of interest.
All claims expressed in this article are solely those of the authors and do not necessarily represent those of their affiliated organizations, or those of the publisher, the editors and the reviewers. Any product that may be evaluated in this article, or claim that may be made by its manufacturer, is not guaranteed or endorsed by the publisher.
Abdelrahman, M., Burritt, D. J., and Tran, L. S. P. (2018). The use of metabolomic quantitative trait locus mapping and osmotic adjustment traits for the improvement of crop yields under environmental stresses. Semin. Cell Dev. Biol. 83, 86–94. doi: 10.1016/j.semcdb.2017.06.020
Abou-Elwafa, S. F., Amin, A. E. E. A. Z., and Shehzad, T. (2019). Genetic mapping and transcriptional profiling of phytoremediation and heavy metals responsive genes in sorghum. Ecotox. Environ. Saf. 173, 366–372. doi: 10.1016/j.ecoenv.2019.02.022
Afreen, F., Zobayed, S., and Kozai, T. (2006). Melatonin in Glycyrrhiza uralensis: response of plant roots to spectral quality of light and UV-B radiation. J. Pin. Res. 41, 108–115. doi: 10.1111/j.1600-079X.2006.00337.x
Agarwal, P., Mitra, M., Banerjee, S., and Roy, S. (2020). MYB4 transcription factor, a member of R2R3-subfamily of MYB domain protein, regulates cadmium tolerance via enhanced protection against oxidative damage and increases expression of PCS1 and MT1C in Arabidopsis. Plant Sci. 297:110501. doi: 10.1016/j.plantsci.2020.110501
Ahammed, G. J., Wu, M., Wang, Y., Yan, Y., Mao, Q., Ren, J., et al. (2020). Melatonin alleviates iron stress by improving iron homeostasis, antioxidant defense and secondary metabolism in cucumber. Sci. Hortic. 30, 265–109205.
Ahmad, R., Tehsin, Z., Malik, S. T., Asad, S. A., Shahzad, M., Bilal, M., et al. (2016). Phytoremediation potential of hemp (Cannabis sativa L.): identification and characterization of heavy metals responsive genes. CLEAN–Soil, Air Water 44, 195–201. doi: 10.1002/clen.201500117
Al-Huqail, A. A., Khan, M. N., Ali, H. M., Siddiqui, M. H., Al-Huqail, A. A., AlZuaibr, F. M., et al. (2020). Exogenous melatonin mitigates boron toxicity in wheat. Ecotox. Environ. Saf. 201:110822. doi: 10.1016/j.ecoenv.2020.110822
Ali, I., Alharbi, O. M., Alothman, Z. A., Badjah, A. Y., and Alwarthan, A. (2018). Artifcial neural network modelling of amido black dye sorption on iron composite nano material: kinetics and thermodynamics studies. J. Mol. Liquids 250, 1–8. doi: 10.1016/j.molliq.2017.11.163
Ali, B., Gill, R. A., Yang, S., Gill, M. B., Farooq, M. A., Liu, D., et al. (2015b). Regulation of cadmium-induced proteomic and metabolic changes by 5-aminolevulinic acid in leaves of Brassica napus L. PLoS One 10:e0123328. doi: 10.1371/journal.pone.0123328
Ali, B., Huang, C. R., Qi, Z. Y., Daud, M. K., Geng, X. X., Liu, H. B., et al. (2013c). 5-Aminolevulinic acid ameliorates cadmium-induced morphological, biochemical and ultrastructural changes in seedlings of oilseed rape. Environ. Sci. Pollut. Res. 20, 7256–7267. doi: 10.1007/s11356-013-1735-5
Ali, M., Kamran, M., Abbasi, G. H., Saleem, M. H., Ahmad, S., Parveen, A., et al. (2021). Melatonin-induced salinity tolerance by ameliorating osmotic and oxidative stress in the seedlings of two tomato (Solanum lycopersicum L.) cultivars. J. Plant Growth Regul. 40, 2236–2248. doi: 10.1007/s00344-020-10273-3
Ali, B., Tao, Q. J., Zhou, Y. F., Mwamba, T. M., Rafiq, M. T., Xu, L., et al. (2013b). 5-Aminolevolinic acid mitigates the cadmium-induced changes in Brassica napus as revealed by the biochemical and ultra-structural evaluation of roots. Ecotox. Environ. Saf. 92, 271–280. doi: 10.1016/j.ecoenv.2013.02.006
Ali, B., Wang, B., Ghani, M. A., Hayat, M. T., Yang, C., Xu, L., et al. (2013a). 5-Aminolevulinic acid ameliorates the growth, photosynthetic gas exchange capacity and ultrastructural changes under cadmium stress in Brassica napus L. J. Plant Growth Regul. 32, 604–614. doi: 10.1007/s00344-013-9328-6
Altaf, M. A., Shahid, R., Ren, M. X., Altaf, M. M., Jahan, M. S., and Khan, L. U. (2021a). Melatonin mitigates nickel toxicity by improving nutrient uptake fluxes, root architecture system, photosynthesis, and antioxidant potential in tomato seedling. J. Soil Sci. Plant Nutr. 21, 1842–1855. doi: 10.1007/s42729-021-00484-2
Altaf, M. A., Shahid, R., Ren, M. X., Khan, L. U., Altaf, M. M., Jahan, M. S., et al. (2021b). Protective mechanisms of melatonin against vanadium phytotoxicity in tomato seedlings: insights into nutritional status, photosynthesis, root architecture system, and antioxidant machinery. J. Plant Growth Regul. 12, 1–7. doi: 10.1007/s00344-021-10513-0
Altaf, M. A., Shahid, R., Ren, M. X., Mora-Poblete, F., Arnao, M. B., Naz, S., et al. (2021c). Phytomelatonin: an overview of the importance and mediating functions of melatonin against environmental stresses. Physiol. Plant. 172, 820–846. doi: 10.1111/ppl.13262
Anjum, N. A., Umar, S., Ahmad, A., Iqbal, M., and Khan, N. A. (2008). Sulphur protects mustard (Brassica campestris L.) from cadmium toxicity by improving leaf ascorbate and glutathione. Plant Growth Regul. 54, 271–279. doi: 10.1007/s10725-007-9251-6
Arnao, M. B., and Hernández-Ruiz, J. (2007). Melatonin promotes adventitious-and lateral root regeneration in etiolated hypocotyls of Lupinus albus L. J. Pin. Res. 42, 147–152. doi: 10.1111/j.1600-079X.2006.00396.x
Arnao, M., and Hernández-Ruiz, J. (2014). Melatonin: plant growth regulator and/or biostimulator during stress? Trends Plant Sci. 19, 789–797. doi: 10.1016/j.tplants.2014.07.006
Arnao, M. B., and Hernández-Ruiz, J. (2015). Functions of melatonin in plants: a review. J. Pin. Res. 59, 133–150. doi: 10.1111/jpi.12253
Arnao, M. B., and Hernández-Ruiz, J. (2017). Melatonin and its relationship to plant hormones. Ann. Bot. 121, 195–207. doi: 10.1093/aob/mcx114
Arnao, B. M., and Hernández-Ruiz, J. (2019a). Role of melatonin to enhance phytoremediation capacity. Applied Sci. 9:5293. doi: 10.3390/app9245293
Arnao, M. B., and Hernández-Ruiz, J. (2020). Melatonin in Flowering, Fruit Set and Fruit Ripening. Plant Reprod. 33, 77–87. doi: 10.1007/s00497-020-00388-8
Aslam, B., Basit, M., Nisar, M. A., Khurshid, M., and Rasool, M. H. (2017). Proteomics: technologies and their applications. J. Chromatogr. Sci. 55, 182–196. doi: 10.1093/chromsci/bmw167
Ayub, M. A., Ahmad, Z., Umar, W., Waris, A. A., Fatima, H., Nadeem, M., et al. (2021). Accumulation, partitioning, and bioavailability of micronutrients in plants and their crosstalk with phytohormones. In Plant Growth Regulators. eds. T. Aftab and K. R. Hakeem Cham: Springer.
Ayyaz, A., Farooq, M. A., Dawood, M., Majid, A., Javed, M., Athar, H. U., et al. (2021). Exogenous melatonin regulates chromium stress-induced feedback inhibition of photosynthesis and antioxidative protection in Brassica napus cultivars. Plant Cell Rep. 40, 2063–2080. doi: 10.1007/s00299-021-02769-3
Back, K. (2021). Melatonin metabolism, signaling and possible roles in plants. Plant J. 105, 376–391. doi: 10.1111/tpj.14915
Basheer, A. A. (2018). Chemical chiral pollution: impact on the society and science and need of the regulations in the 21st century. Chirality 30, 402–406. doi: 10.1002/chir.22808
Bielen, A., Remans, T., Vangronsveld, J., and Cuypers, A. (2013). The influence of metal stress on the availability and redox state of ascorbate, and possible interference with its cellular functions. Int. J. Mol. Sci. 14, 6382–6413. doi: 10.3390/ijms14036382
Borges, K. L. R., Salvato, F., Loziuk, P. L., Muddiman, D. C., and Azevedo, R. A. (2019). Quantitative proteomic analysis of tomato genotypes with differential cadmium tolerance. Environ. Sci. Pollut. Res. 26, 26039–26051. doi: 10.1007/s11356-019-05766-y
Bose, J., Rodrigo-Moreno, A., Lai, D., Xie, Y., Shen, W., and Shabala, S. (2015). Rapid regulation of the plasma membrane HC-ATPase activity is essential to salinity tolerance in two halophyte species, Atriplexlentiformis and Chenopodium quinoa. Ann. Bot. 115, 481–494. doi: 10.1093/aob/mcu219
Burakova, E. A., Dyachkova, T. P., Rukhov, A. V., Tugolukov, E. N., Galunin, E. V., Tkachev, A. G., et al. (2018). Novel and economic method of carbon nanotubes synthesis on a nickel magnesium oxide catalyst using microwave radiation. J. Mol. Liq. 253, 340–346. doi: 10.1016/j.molliq.2018.01.062
Campos, A. C. A., van Dijk, W. F., Ramakrishna, P., Giles, T., Korte, P., Douglas, A., et al. (2021). 1,135 ionomes reveal the global pattern of leaf and seed mineral nutrient and trace element diversity in Arabidopsis thaliana. Plant J. 106, 536–554. doi: 10.1111/tpj.15177
Cao, Y. Y., Qi, C. D., Li, S., Wang, Z., Wang, X., Wang, J., et al. (2019). Melatonin alleviates copper toxicity via improving copper sequestration and ROS scavenging in cucumber. Plant Cell Physiol. 60, 562–574. doi: 10.1093/pcp/pcy226
Castrillo, G., Sanchez-Bermejo, E., de Lorenzo, L., Crevillen, P., Fraile-Escanciano, A., Tc, M., et al. (2013). WRKY6 transcription factor restricts arsenate uptake and transposon activation in Arabidopsis. Plant Cell 25, 2944–2957. doi: 10.1105/tpc.113.114009
Chaffai, R., and Koyama, H. (2011). Heavy metal tolerance in Arabidopsis thaliana. Adv. Bot. Res. 60, 1–49. doi: 10.1016/B978-0-12-385851-1.00001-9
Chai, M., Li, R., Tam, N. F. Y., and Zan, Q. (2019). Effects of mangrove plant species on accumulation of heavy metals in sediment in a heavily polluted mangrove swamp in Pearl River estuary, China. Environ. Geochem. Health 41, 175–189. doi: 10.1007/s10653-018-0107-y
Chakraborty, K., Bose, J., Shabala, L., and Shabala, S. (2016). Difference in root K+ retention ability and reduced sensitivity of KC-permeable channels to reactive oxygen species confer differential salt tolerance in three brassica species. J. Exp. Bot. 67, 4611–4625. doi: 10.1093/jxb/erw236
Chamanara, M., Rashidian, A., Mehr, S. E., Dehpour, A. R., Shirkohi, R., Akbarian, R., et al. (2019). Melatonin ameliorates TNBS-induced colitis in rats through the melatonin receptors: involvement of TLR4/MyD88/NF-κB signalling pathway. Inflammopharmacol 27, 361–371.
Chandramouli, K., and Qian, P. Y. (2009). Proteomics: challenges, techniques and possibilities to overcome biological sample complexity. Hum Genomics Proteomics. 1. doi: 10.4061/2009/239204
Chao, D. Y., Gable, K., Chen, M., Baxter, I., Dietrich, C. R., Cahoon, E. B., et al. (2011). Sphingolipids in the root play an important role in regulating the leaf Ionome in Arabidopsis thaliana. Plant Cell 23, 1061–1081. doi: 10.1105/tpc.110.079095
Chatterjee, S., Chatterjee, S., Chatterjee, B. P., and Guha, A. K. (2008). Enhancement of growth and chitosan production by Rhizopusoryzae in whey medium by plant growth hormones. Int. J. Biol. Macromol. 42, 120–126. doi: 10.1016/j.ijbiomac.2007.10.006
Chen, Q., Qi, W. B., Reiter, R. J., Wei, W., and Wang, B. M. (2009a). Exogenously applied melatonin stimulates root growth and raises endogenous indoleacetic acid in roots of etiolated seedlings of Brassica juncea. J. Plant Physiol. 166, 324–328. doi: 10.1016/j.jplph.2008.06.002
Chen, Z., Shinano, T., Ezawa, T., Wasaki, J., Kimura, K., Osaki, M., et al. (2009b). Elemental interconnections in Lotus japonicus: a systematic study of the effects of elements additions on different natural variants. Soil Sci. Plant Nutr. 55, 91–101. doi: 10.1111/j.1747-0765.2008.00311.x
Chen, Y. H., Wu, X. M., Ling, H. Q., and Yang, W. C. (2006). Transgenic expression of DwMYB2 impairs iron transport from root to shoot in Arabidopsis thaliana. Cell Res. 16, 830–840. doi: 10.1038/sj.cr.7310099
Chen, J., Yang, L. B., Yan, X. X., Liu, Y. L., Wang, R., Fan, T. T., et al. (2016). Zinc-finger transcription factor ZAT6 positively regulates cadmium tolerance through the glutathione-dependent pathway in Arabidopsis. Plant Physiol. 171, 707–719. doi: 10.1104/pp.15.01882
Cheng, D., Tan, M., Yu, H., Li, L., Zhu, D., Chen, Y., et al. (2018). Comparative analysis of cd-responsive maize and rice transcriptomes highlights cd co-modulated Orthologs. BMC Genomics 19:709. doi: 10.1186/s12864-018-5109-8
Cuin, T. A., Betts, S. A., Chalmandrier, R., and Shabala, S. (2008). Root’s ability to retain K+correlates with salt tolerance in wheat. J. Exp. Bot. 59, 2697–2706. doi: 10.1093/jxb/ern128
Dağhan, H., and Ozturk, M. (2015). “Soil pollution in Turkey and remediation methods,” in Soil Remediation and Plants: Prospects and Challenges. eds. K. Hakeem, M. Sabir, M. Ozturk, and A. Mermut (New York, NY: Elsevier), 287–312.
Dehghani, M. H., Sanaei, D., Ali, I., and Bhatnagar, A. (2016). Removal of chromium (VI) from aqueous solution using treated waste newspaper as a low-cost adsorbent: kinetic modeling and isotherm studies. J. Mol. Liq. 215, 671–679. doi: 10.1016/j.molliq.2015.12.057
Denancé, N., Sánchez-Vallet, A., Goffner, D., and Molina, A. (2015). Disease resistance or growth: the role of plant hormones in balancing immune responses and fitness costs. Front. Plant Sci. 4:155.
Dijkstra, F. A., He, M. Z., Johansen, M. P., Harrison, J. J., and Keitel, C. (2015). Plant and microbial uptake of nitrogen and phosphorus affected by drought using 15N and 32P tracers. Soil Biol. Biochem. 82, 135–142. doi: 10.1016/j.soilbio.2014.12.021
Duan, G., Hakoyama, T., Kamiya, T., Miwa, H., Lombardo, F., Sato, S., et al. (2017). LjMOT1, a high-affinity molybdate transporter from Lotus japonicus, is essential for molybdate uptake, but not for the delivery to nodules. Plant J. 90, 1108–1119. doi: 10.1111/tpj.13532
Duhan, S. (2021). “Role of reactive nitrogen species in enhancing metal/metalloid tolerance in plants: a basis of phytoremediation” in Handbook of Bioremediation (Cambridge, MA: Elsevier, Academic Press), 195–203.
Fan, J., Hu, Z., Xie, Y., Chan, Z., Chen, K., Amombo, E., et al. (2015). Alleviation of cold damage to photosystem II and metabolisms by melatonin in Bermuda grass. Front. Plant Sci. 6:925. doi: 10.3389/fpls.2015.00925
Fan, L. X., Xu, L., Wang, Y., Tang, M. J., and Liu, L. W. (2019). Genome-and transcriptome-wide characterization of bZIP gene family identifies potential members involved in abiotic stress response and anthocyanin biosynthesis in radish (Raphanus sativus L.). Int. J. Mol. Sci. 20:6334. doi: 10.3390/ijms20246334
Farzadfar, S., Zarinkamar, F., Modarres-Sanavy, S. A. M., and Hojati, M. (2013). Exogenously applied calcium alleviates cadmium toxicity in Matricariachamomilla L. plants. Environ. Sci. Pollut. Res. 20, 1413–1422. doi: 10.1007/s11356-012-1181-9
Flora, S. J. S. (2009). Structural, chemical and biological aspects of antioxidants for strategies against metal and metalloid exposure. Oxidative Med. Cell. Longev. 2, 191–206. doi: 10.4161/oxim.2.4.9112
Frukh, A., Siddiqi, T. O., Khan, M. I. R., and Ahmad, A. (2020). Modulation in growth, biochemical attributes and proteome profile of Rice cultivars under Salt stress. Plant Physiol. Biochem. 146, 55–70. doi: 10.1016/j.plaphy.2019.11.011
Gao, Y., Wang, Y., Qian, J., Si, W., Tan, Q., Xu, J., et al. (2020). Melatonin enhances the cadmium tolerance of mushrooms through antioxidant-related metabolites and enzymes. Food Chem. 330:127263. doi: 10.1016/j.foodchem.2020.127263
Hakeem, K. R., Sabir, M., Ozturk, M., and Mermut, A. (2015). Soil Remediation and Plants: Prospects and Challenges. Elsevier, London p 724.
Han, Y. Y., Fan, T. T., Zhu, X. Y., Wu, X., Ouyang, J., Jiang, L., et al. (2019). WRKY12 represses GSH1 expression to negatively regulate cadmium tolerance in Arabidopsis. Plant Mol. Biol. 99, 149–159. doi: 10.1007/s11103-018-0809-7
Han, Y. X., Hou, Z. N., He, Q. L., Zhang, X. M., Yan, K. J., Han, R. L., et al. (2021). Genome-wide characterization and expression analysis of bZIP gene family under abiotic stress in Glycyrrhiza uralensis. Front. Genet. 12:754237. doi: 10.3389/fgene.2021.754237
Hardeland, R. (2016). Melatonin in plants—diversity of levels and multiplicity of functions. Front. Plant Sci. 7:198. doi: 10.3389/fpls.2016.00198
Hasan, M. K., Ahammed, G. J., Sun, S., Li, M., Yin, H., and Zhou, J. (2019). Melatonin inhibits cadmium translocation and enhances plant tolerance by regulating sulfur uptake and assimilation in Solanum lycopersicum L. J. Agric. Food Chem. 67, 10563–10576. doi: 10.1021/acs.jafc.9b02404
Hassan, T. U., Bano, A., and Naz, I. (2017). Alleviation of heavy metals toxicity by the application of plant growth promoting rhizobacteria and effects on wheat grown in saline sodic field. Int. J. Phytoremediation 19, 522–529. doi: 10.1080/15226514.2016.1267696
Hernández, I. G., Gomez, F. J. V., Cerutti, S., Arana, M. V., and Silva, M. F. (2015). Melatonin in Arabidopsis thaliana acts as plant growth regulator at low concentrations and preserves seed viability at high concentrations. Plant Physiol. Biochem. 94, 191–196. doi: 10.1016/j.plaphy.2015.06.011
Hernandez-Ruiz, J., Cano, A., and Arnao, M. B. (2004). Melatonin: A growth-stimulating compound present in lupin tissues. Planta 220, 140–144. doi: 10.1007/s00425-004-1317-3
Hernández-Ruiz, J., Cano, A., and Arnao, M. B. (2005). Melatonin acts as a growth-stimulating compound in some monocot species. J. Pineal Res. 39, 137–142. doi: 10.1111/j.1600-079X.2005.00226.x
Hoang, X. L. T., Nhi, D. N. H., Thu, N. B. A., Thao, N. P., and Tran, L.-S. P. (2017). Transcription factors and their roles in signal transduction in plants under abiotic stresses. Curr. Genomics 18, 483–497. doi: 10.2174/1389202918666170227150057
Hu, S. B., Yu, Y., Chen, Q. H., Mu, G. M., Shen, Z. G., and Zheng, L. Q. (2017). OsMYB45 plays an important role in rice resistance to cadmium stress. Plant Sci. 264, 1–8. doi: 10.1016/j.plantsci.2017.08.002
Huang, L., Li, M., Shao, Y., Sun, T., Li, C., and Ma, F. (2018a). Ammonium uptake increases in response to PEG-induced drought stress in Malushupehensis Rehd. Environ. Exp. Bot. 151, 32–42. doi: 10.1016/j.envexpbot.2018.04.007
Huang, L., Li, M., Zhou, K., Sun, T., Hu, L., Li, C., et al. (2018b). Uptake and metabolism of ammonium and nitrate in response to drought stress in Malusprunifolia. Plant Physiol. Biochem. 127, 185–193. doi: 10.1016/j.plaphy.2018.03.031
Jalmi, S. K., and Sinha, A. K. (2015). ROS mediated MAPK signaling in abioticand biotic stress-striking similarities and differences. Front. Plant Sci. 6:769. doi: 10.3389/fpls.2015.00769
Jayarajan, S., and Sharma, R. R. (2021). Melatonin: A blooming biomolecule for postharvest management of perishable fruits and vegetables. Trends Food Sci. Technol. 116, 318–328. doi: 10.1016/j.tifs.2021.07.034
Jozefczak, M., Remans, T., Vangronsveld, J., and Cuypers, A. (2012). Glutathione is a key player in metal-induced oxidative stress defenses. Int. J. Mol. Sci. 13, 3145–3175. doi: 10.3390/ijms13033145
Kanwar, M. K., Yu, J., and Zhou, J. (2018). Phytomelatonin: recent advances and future prospects. J. Pineal Res. 65:e12526. doi: 10.1111/jpi.12526
Kaur, H., Mukherjee, S., Baluska, F., and Bhatla, S. C. (2015). Regulatory roles of serotonin and melatonin in abiotic stress tolerance in plants. Plant Signal. Behav. 10:e1049788. doi: 10.1080/15592324.2015.1049788
Khan, N. A., Anjum, N. A., Nazar, R., and Iqbal, N. (2009). Increased activity of ATP-sulfurylase and increased contents of cysteine and glutathione reduce high cadmium- induced oxidative stress in mustard cultivar with high photosynthetic potential. Russ. J. Plant Physiol. 56, 670–677. doi: 10.1134/S1021443709050136
Kholodova, V. P., Vasil’ev, S. V., Efimova, M. V., Voronin, P. Y., Rakhmankulova, Z. F., Danilova, E. Y., et al. (2018). Exogenous melatonin protects canola plants from toxicity of excessive copper. Russ. J. Plant Physiol. 65, 882–889. doi: 10.1134/S1021443718060080
Kołodziejczyk, I., Dzitko, K., Szewczyk, R., and Posmyk, M. M. (2016). Exogenous melatonin expediently modifies proteome of maize (Zea mays L.) embryo during seed germination. Acta Physiol. Plant. 38:146. doi: 10.1007/s11738-016-2166-y
Krzesłowska, M. (2011). The cell wall in plant cell response to trace metals: polysaccharide remodeling and its role in defense strategy. Acta Physiol. Plant. 33, 35–51. doi: 10.1007/s11738-010-0581-z
Kunkel, B. N., and Johnson, J. M. (2021). Auxin plays multiple roles during plant–pathogen interactions. Cold Spring Harb. Perspect. Biol. 13:a040022. doi: 10.1101/cshperspect.a040022
Kwon, N., Kim, D., Swamy, K. M. K., and Yoon, J. (2021). Metal-coordinated fluorescent and luminescent probes for reactive oxygen species (ROS) and reactive nitrogen species (RNS). Coord. Chem. Rev. 427:213581. doi: 10.1016/j.ccr.2020.213581
Lauer Júnior, C. M., Bonatto, D., Mielniczki-Pereira, A. A., Schuch, A. Z., Dias, J. F., Yoneama, M. L., et al. (2008). The Pmr1 protein, the major yeast Ca2+− ATPase in the grefecvolgi, regulates intracellular levels of the cadmium ion. FEMS Microbiol. Lett. 285, 79–88. doi: 10.1111/j.1574-6968.2008.01214.x
Lee, H. Y., and Back, K. (2017a). Cadmium disrupts subcellular organelles, including chloroplasts, resulting in melatonin induction in plants. Molecules 22. doi: 10.3390/molecules22101791
Lee, K., and Back, K. (2017b). Overexpression of rice serotonin N-Acetyltransferase 1 in transgenic Rice plants confers resistance to cadmium and senescence and increases grain yield. J. Pineal Res. 62:e12392. doi: 10.1111/jpi.12392
Levy, A., Conway, J. M., Dangl, J. L., and Woyke, T. (2018). Elucidating bacterial gene functions in the plant microbiome. Cell Host Microbe 24, 475–485. doi: 10.1016/j.chom.2018.09.005
Li, X., Ahammed, G. J., Zhang, X. N., Zhang, L., Yan, P., Zhang, L. P., et al. (2021). Melatonin-mediated regulation of anthocyanin biosynthesis and antioxidant defense confer tolerance to arsenic stress in Camellia sinensis L. J. Hazard. Mater. 403:123922. doi: 10.1016/j.jhazmat.2020.123922
Li, S., Han, X., Lu, Z., Qiu, W., Yu, M., Li, H., et al. (2022). MAPK cascades and transcriptional factors: regulation of heavy metal tolerance in plants. Int. J. Mol. Sci. 23:4463. doi: 10.3390/ijms23084463
Li, X., Tan, D. X., Jiang, D., and Liu, F. (2016). Melatonin enhances cold tolerance in drought-primed wild-type and abscisic acid-deficient mutant barley. J. Pineal Res. 61, 328–339. doi: 10.1111/jpi.12350
Li, C., Wang, P., Wei, Z., Liang, D., Liu, C., Yin, L., et al. (2012). The mitigation effects of exogenous melatonin on salinity-induced stress in Malushupehensis. J. Pineal Res. 53, 298–306. doi: 10.1111/j.1600-079X.2012.00999.x
Li, C. X., Yan, J. Y., Ren, J. Y., Sun, L., Xu, C., Li, G. X., et al. (2020). A WRKY transcription factor confers aluminum tolerance via regulation of cell wall modifying genes. J. Integr. Plant Biol. 62, 1176–1192. doi: 10.1111/jipb.12888
Liang, K.-H. (2013). “Transcriptomics” in Bioinformatics for Biomedical Science and Clinical Applications (Amsterdam: Elsevier), 49–82.
Liang, C., Li, A., Yu, H., Li, W., Liang, C., Guo, S., et al. (2017). Melatonin regulates root architecture by modulating auxin response in rice. Front. Plant Sci. 8:134. doi: 10.3389/fpls.2017.00134
Liang, B., Ma, C., Zhang, Z., Wei, Z., Gao, T., Zhao, Q., et al. (2018). Long-term exogenous application of melatonin improves nutrient uptake fluxes in apple plants under moderate drought stress. Environ. Exp. Bot. 155, 650–661. doi: 10.1016/j.envexpbot.2018.08.016
Lilay, G. H., Castro, P. H., Campilho, A., and Assunção, A. G. (2018). The Arabidopsis bZIP19 and bZIP23 activity requires zinc deficiency-insight on regulation from complementation lines. Front. Plant Sci. 9:1955. doi: 10.3389/fpls.2018.01955
Lilay, G. H., Persson, D. P., Castro, P. H., Liao, F., and Assuno, A. (2021). Arabidopsis bZIP19 and bZIP23 act as zinc sensors to control plant zinc status. Nat. Plants 7, 137–143. doi: 10.1038/s41477-021-00856-7
Lin, L., Li, J., Chen, F., Liao, M. A., Tang, Y., Liang, D., et al. (2018). Effects of melatonin on the growth and cadmium characteristics of Cyphom and rabetacea seedlings. Environ. Monit. Assess. 190, 1–8.
Lin, T. T., Yang, W. M., Lu, W., Wang, Y., and Qi, X. T. (2017). Transcription factors PvERF15 and PvMTF-1 form a cadmium stress transcriptional pathway. Plant Physiol. 173, 1565–1573. doi: 10.1104/pp.16.01729
Lin, T., Zhu, X., Zhang, F., and Wan, X. (2011). The detoxification effect of cadmium stress in Populusyunnanensis. Res. J. Bot. 4, 13–19. doi: 10.3923/brj.2011.13.19
Liu, Y., Lu, S., Liu, K., Wang, S., Huang, L., and Guo, L. (2019). Proteomics: a powerful tool to study plant responses to biotic stress. Plant Methods 15, 1–20. doi: 10.1186/s13007-019-0515-8
Liu, A., Zhou, Z., Yi, Y., and Chen, G. (2020). Transcriptome analysis reveals the roles of stem nodes in cadmium transport to Rice grain. BMC Genomics 21. doi: 10.1186/s12864-020-6474-7
Lominchar, M. Á., Sierra, M. J., Jiménez-Moreno, M., Guirado, M., Martín-Doimeadios, R. C. R., and Millán, R. (2019). Mercury species accumulation and distribution in Typha Domingensis under real field conditions (Almadén, Spain). Environ. Sci. Pollut. Res. 26, 3138–3144. doi: 10.1007/s11356-018-1861-1
Lou, H. Q., Fan, W., Jin, J. F., Xu, J. M., Chen, W. W., Yang, J. L., et al. (2020). A NAC-type transcription factor confers aluminium resistance by regulating cell wall-associated receptor kinase 1 and cell wall pectin. Plant Cell Environ. 43, 463–478. doi: 10.1111/pce.13676
Małkowski, E., Sitko, K., Zieleźnik-Rusinowska, P., Gieroń, Ż., and Szopiński, M. (2019). “Heavy metal toxicity: physiological implications of metal toxicity in plants” in Plant Metallomics and Functional Omics: A System-Wide Perspective. ed. G. Sablok (Cham: Springer International Publishing), 253–301.
Mateos-Naranjo, E., Redondo-Gómez, S., Cambrollé, J., Luque, T., and Figueroa, M. E. (2008). Growth and photosynthetic responses to zinc stress of an invasive Cordgrass, Spartina Densiflora. Plant Biol. 10, 754–762. doi: 10.1111/j.1438-8677.2008.00098.x
McGowan, J., and Fitzpatrick, D. A. (2020). Recent advances in oomycete genomics. Adv. Genet. 105, 175–228. doi: 10.1016/bs.adgen.2020.03.001
Meng, J. F., Xu, T. F., Wang, Z. Z., Fang, Y. L., Xi, Z. M., and Zhang, Z. W. (2014). The ameliorative effects of exogenous melatonin on grape cuttings under water-deficient stress: antioxidant metabolites, leaf anatomy, and chloroplast morphology. J. Pineal Res. 57, 200–212. doi: 10.1111/jpi.12159
Meng, S., Zhang, C. X., Su, L., Li, Y. M., and Zhao, Z. (2016). Nitrogen uptake and metabolism of Populussimoniiin response to PEG-induced drought stress. Environ. Exp. Bot. 123, 78–87. doi: 10.1016/j.envexpbot.2015.11.005
Milward, E. A., Shahandeh, A., Heidari, M., Johnstone, D. M., Daneshi, N., and Hondermarck, H. (2016). Transcriptomics. Encyclopedia of Cell Biology 4, 160–165. doi: 10.1016/B978-0-12-394447-4.40029-5
Mosa, K. A., Ismail, A., and Helmy, M. (2017). Functional genomics combined with other omics approaches for better understanding abiotic stress tolerance in plants. In Plant Stress Tolerance, Springer, Cham: Springer Briefs in Systems Biology. 55–73.
Mustafa, G., and Komatsu, S. (2016). Toxicity of heavy metals and metal-containing nanoparticles on plants. Biochim. Biophys. Acta Proteins Proteom. 1864, 932–944. doi: 10.1016/j.bbapap.2016.02.020
Nawaz, M. A., Huang, Y., Bie, Z., Ahmed, W., Reiter, R. J., Niu, M., et al. (2016). Melatonin: current status and future perspectives in plant science. Front. Plant Sci. 6:1230. doi: 10.3389/fpls.2015.01230
Ni, J., Wang, Q., Shah, F. A., Liu, W., Wang, D., Huang, S., et al. (2018). Exogenous melatonin confers cadmium tolerance by counterbalancing the hydrogen peroxide homeostasis in wheat seedlings. Molecules 23:799. doi: 10.3390/molecules23040799
Pang, Z., Chen, J., Wang, T., Gao, C., Li, Z., Guo, L., et al. (2021). Linking plant secondary metabolites and plant microbiomes: a review. Front. Plant Sci. 12:621276. doi: 10.3389/fpls.2021.621276
Pardo-hernández, M., López-delacalle, M., Martí-guillen, J. M., Mar, S. E., and Rivero, R. M. (2021). ROS and NO Phytomelatonin-induced signaling mechanisms under metal toxicity in plants. Antioxidants 10:775. doi: 10.20944/preprints202104.0637.v1
Park, S., Lee, D. E., Jang, H., Byeon, Y., Kim, Y. S., and Back, K. (2013). Melatonin-rich transgenic rice plants exhibit resistance to herbicide-induced oxidative stress. J. Pineal Res. 54, 258–263. doi: 10.1111/j.1600-079X.2012.01029.x
Peharec Štefanić, P., Jarnević, M., Cvjetko, P., Biba, R., Šikić, S., Tkalec, M., et al. (2019). Comparative proteomic study of phytotoxic effects of silver nanoparticles and silver ions on tobacco plants. Environ. Sci. Pollut. Res. 26, 22529–22550. doi: 10.1007/s11356-019-05552-w
Pelagio-Flores, R., Muñoz-Parra, E., Ortiz-Castro, R., and López-Bucio, J. (2012). Melatonin regulates Arabidopsis root system architecture likely acting independently of auxin signaling. J. Pineal Res. 53, 279–288. doi: 10.1111/j.1600-079X.2012.00996.x
Pollard, A. J., Reeves, R. D., and Baker, A. J. M. (2014). Facultative hyperaccumulation of heavy metals and metalloids. Plant Sci. 217-218, 8–17. doi: 10.1016/j.plantsci.2013.11.011
Rajasundaram, D., and Selbig, J. (2016). More effort - more results: recent advances in integrative “omics” data analysis. Curr. Opin. Plant Biol. 30, 57–61. doi: 10.1016/j.pbi.2015.12.010
Reiter, R. J., Tan, D. X., Zhou, Z., Cruz, M. H. C., Fuentes-Broto, L., and Galano, A. (2015). Phytomelatonin: assisting plants to survive and thrive. Molecules 20, 7396–7437. doi: 10.3390/molecules20047396
Rubio, V., Bustos, R., Irigoyen, M. L., Cardona-Lopez, X., Rojas-Triana, M., and Paz-Ares, J. (2009). Plant hormones and nutrient signaling. Plant Mol. Biol. 69, 361–373. doi: 10.1007/s11103-008-9380-y
Sage, R. F., and Kubien, D. S. (2007). The temperature response of C3 and C4 photosynthesis. Plant Cell Environ. 30, 1086–1106. doi: 10.1111/j.1365-3040.2007.01682.x
Sami, A., Shah, F. A., Abdullah, M., Zhou, X., Yan, Y., Zhu, Z., et al. (2020). Melatonin mitigates cadmium and aluminium toxicity through modulation of antioxidant potential in Brassica napus L. Plant Biol. 22, 679–690. doi: 10.1111/plb.13093
Sapara, K. K., Khedia, J., Agarwal, P., Gangapur, D. R., and Agarwal, P. K. (2019). SbMYB15 transcription factor mitigates cadmium and nickel stress in transgenic tobacco by limiting uptake and modulating antioxidative defence system. Funct. Plant Biol. 46, 702–714. doi: 10.1071/FP18234
Sarropoulou, V. N., Dimassi-Theriou, K., Therios, I., and KoukourikouPetridou, M. (2012). Melatonin enhances root regeneration, photosynthetic pigments, biomass, total carbohydrates and proline content in the cherry rootstock PHL-C (Prunusavium×Prunuscerasus). Plant Physiol. Biochem. 61, 162–168. doi: 10.1016/j.plaphy.2012.10.001
Sarrou, E., Therios, I., and Dimassi-Theriou, K. (2014). Melatonin and other factors that promote rooting and sprouting of shoot cuttings in Punicagranatum cv. Wonderful. Turkish J. Bot. 38, 293–301. doi: 10.3906/bot-1302-55
Sarwar, N., Saifullah,, Malhi, S. S., Zia, M. H., Naeem, A., Bibia, S., et al. (2010). Role of mineral nutrition in minimizing cadmium accumulation by plants. J. Sci. Food Agric. 90, 925–937. doi: 10.1002/jsfa.3916
Shahid, M., Khalid, S., Abbas, G., Shahid, N., Nadeem, M., Sabir, M., et al. (2015). “Heavy metal stress and crop productivity” in Crop Production and Global Environmental Issues. (Cham: Springer), 1–25.
Shahid, M., Pourrut, B., Dumat, C., Nadeem, M., Aslam, M., and Pinelli, E. (2014). Heavy-metal-induced reactive oxygen species: Phytotoxicity and physicochemical changes in plants. Rev. Environ. Contam. Toxicol. 232, 1–44. doi: 10.1007/978-3-319-06746-9_1
Sheng, Y. B., Yan, X. X., Huang, Y. Y., Han, Y., Zhang, C., Ren, Y. B., et al. (2019). The WRKY transcription factor, WRKY13, activates PDR8 expression to positively regulate cadmium tolerance in Arabidopsis. Plant Cell Environ. 42, 891–903. doi: 10.1111/pce.13457
Shi, H., Reiter, R. J., Tan, D. X., and Chan, Z. (2015). INDOLE-3-ACETIC ACID INDUCIBLE 17 positively modulates natural leaf senescence through melatonin-mediated pathway in Arabidopsis. J. Pineal Res. 58, 26–33. doi: 10.1111/jpi.12188
Singh, S., Parihar, P., Singh, R., Singh, V. P., and Prasad, S. M. (2016). Heavy metal tolerance in plants: role of Transcriptomics, proteomics, metabolomics, and Ionomics. Front. Plant Sci. 6, 1–36. doi: 10.3389/fpls.2015.01143
Sun, J., Dai, S., Wang, R., Chen, S., Li, N., Zhou, X., et al. (2009b). Calcium mediates root KC /NaC homeostasis in poplar species differing in salt tolerance. Tree Physiol. 29, 1175–1186. doi: 10.1093/treephys/tpp048
Sun, C., Liu, L., Wang, L., Li, B., Jin, C., and Lin, X. (2021). Melatonin: a master regulator of plant development and stress responses. J. Integr. Plant Biol. 63, 126–145. doi: 10.1111/jipb.12993
Sun, Q., Zhang, N., Wang, J., Zhang, H., Li, D., Shi, J., et al. (2015). Melatonin promotes ripening and improves quality of tomato fruit during postharvest life. J. Exp. Bot. 66, 657–668. doi: 10.1093/jxb/eru332
Tan, D. X., Manchester, L. C., Liu, X., Rosales-Corral, S. A., Acuna-Castroviejo, D., and Reiter, R. J. (2013). Mitochondria and chloroplasts as the original sites of melatonin synthesis: a hypothesis related to melatonin’s primary function and evolution in eukaryotes. J. Pineal Res. 54, 127–138. doi: 10.1111/jpi.12026
Thao, N. P., and Tran, L.-S. P. (2016). Enhancement of plant productivity in the post-genomics era. Curr. Genomics 17, 295–296. doi: 10.2174/138920291704160607182507
Tiryaki, I., and Keles, H. (2012). Reversal of the inhibitory effect of light and high temperature on germination of Phacelia tanacetifolia seeds by melatonin. J. Pineal Res. 52, 332–339. doi: 10.1111/j.1600-079X.2011.00947.x
Tripathi, G. D., Javed, Z., Mishra, M., Fasake, V., and Dashora, K. (2021). Phytomelatonin in stress management in agriculture. Heliyon 7:e06150. doi: 10.1016/j.heliyon.2021.e06150
Tripathi, S., and Poluri, K. M. (2021). Heavy metal detoxification mechanisms by microalgae: insights from Transcriptomics analysis. Environ. Pollut. 285:117443. doi: 10.1016/j.envpol.2021.117443
Turk, H., and Erdal, S. (2015). Melatonin alleviates cold-induced oxidative damage in maize seedlings by up-regulating mineral elements and enhancing antioxidant activity. J. Plant Nutr. Soil Sci. 178, 433–439. doi: 10.1002/jpln.201400476
Ulhassan, Z., Huang, Q., Gill, R. A., Ali, S., Mwamba, T. M., Ali, B., et al. (2019). Protective mechanisms of melatonin against selenium toxicity in Brassica napus: insights into physiological traits, thiol biosynthesis and antioxidant machinery. BMC Plant Biol. 19, 1–6. doi: 10.1186/s12870-019-2110-6
Van de Mortel, J. E., Schat, H., Moerland, P. D., Van Themaat, E. V. L., Van Der Ent, S. J. O. E. R. D., Blankestijn, H., et al. (2010). Expression differences for genes involved in lignin, glutathione and sulphate metabolism in response to cadmium in Arabidopsis thaliana and the related Zn/cd-hyperaccumulator Thlaspi caerulescens. Plant Cell Environ. 31, 301–324. doi: 10.1111/j.1365-3040.2007.01764.x
Varma, S. (2021). Heavy metals stress and defense strategies in plants: an overview. J. Pharmacogn. Phytochem. 10, 608–614.
Wang, Q., An, B., Wei, Y., Reiter, R. J., Shi, H., Luo, H., et al. (2016b). Melatonin regulates root meristem by repressing auxin synthesis and polar auxin transport in Arabidopsis. Front. Plant Sci. 7:1882. doi: 10.3389/fpls.2016.01882
Wang, Z., Gerstein, M., and Snyder, M. (2009b). RNA-Seq: a revolutionary tool for Transcriptomics. Nat. Rev. Genet. 10, 57–63. doi: 10.1038/nrg2484
Wang, L., Liu, J., Wang, W., and Sun, Y. (2016a). Exogenous melatonin improves growth and photosynthetic capacity of cucumber under salinity-induced stress. Photosynthetica 54, 19–27. doi: 10.1007/s11099-015-0140-3
Wang, Y., Reiter, R. J., and Chan, Z. (2018). Phytomelatonin: A universal abiotic stress regulator. J. Exp. Bot. 69, 963–974. doi: 10.1093/jxb/erx473
Wang, Q., Zeng, X., Song, Q., Sun, Y., Feng, Y., and Lai, Y. (2020). Identification of key genes and modules in response to cadmium stress in different Rice varieties and stem nodes by weighted gene co-expression network analysis. Sci. Rep. 10:9525. doi: 10.1038/s41598-020-66132-4
Wang, H., Zhao, S. C., Liu, R. L., Zhou, W., and Jin, J. Y. (2009a). Changes of photosynthetic activities of maize (Zea mays L.) seedlings in response to cadmium stress. Photosynthetica 47, 277–283. doi: 10.1007/s11099-009-0043-2
Wang, Y., Zhong, B., Shafi, M., Ma, J., Guo, J., Wu, J., et al. (2019). Effects of biochar on growth, and heavy metals accumulation of moso bamboo (Phyllostachy pubescens), soil physical properties, and heavy metals solubility in soil. Chemosphere 219, 510–516. doi: 10.1016/j.chemosphere.2018.11.159
Watanabe, T., Broadley, M. R., Jansen, S., White, P. J., Takada, J., Satake, K., et al. (2007). Evolutionary control of leaf element composition in plants. New Phytol. 174, 516–523. doi: 10.1111/j.1469-8137.2007.02078.x
Weeda, S., Zhang, N., Zhao, X., Ndip, G., Guo, Y., Buck, G. A., et al. (2014). Arabidopsis transcriptome analysis reveals key roles of melatonin in plant defense systems. PLoS One 9:e93462. doi: 10.1371/journal.pone.0093462
Wei, W., Li, Q. T., Chu, Y. N., Reiter, R. J., Yu, X. M., Zhu, D. H., et al. (2014). Melatonin enhances plant growth and abiotic stress tolerance in soybean plants. J. Exp. Bot. 66, 695–707.
White, P. J., Bowen, H. C., Broadley, M. R., El-Serehy, H. A., Neugebauer, K., Taylor, A., et al. (2017). Evolutionary origins of abnormally large shoot sodium accumulation in nonsaline environments within the Caryophyllales. New Phytol. 214, 284–293. doi: 10.1111/nph.14370
Wilson, A., Fox, E. M., Fegan, N., and Ípek Kurtböke, D. (2019). Comparative genomics and phenotypic investigations into antibiotic, heavy metal, and disinfectant susceptibilities of salmonella Enterica strains isolated in Australia. Front. Microbiol. 10:1620. doi: 10.3389/fmicb.2019.01620
Yang, L., You, J., Li, J., Wang, Y., and Chan, Z. (2021). Melatonin promotes Arabidopsis primary root growth in an IAA-dependent manner. J. Exp. Bot. 72, 5599–5611. doi: 10.1093/jxb/erab196
Yu, C., Liu, Y., Ma, T., Liu, K., Xu, S., Zhang, Y., et al. (2015). Small molecules enhance CRISPR genome editing in pluripotent stem cells. Cell Stem Cell. 16, 142–147.
Yu, Y., Lv, Y., Shi, Y., Li, T., Chen, Y., Zhao, D., et al. (2018a). The role of Phyto-melatonin and related metabolites in response to stress. Molecules 23:23. doi: 10.3390/molecules23081887
Yu, Y., Teng, Z., Mou, Z., Lv, Y., Li, T., Chen, S., et al. (2021a). Melatonin confers heavy metal-induced tolerance by alleviating oxidative stress and reducing the heavy metal accumulation in Exophialapisciphila, a dark septate endophyte (DSE). BMC Microbiol. 21:1. doi: 10.1186/s12866-021-02098-1
Yu, Y., Teng, Z., Mou, Z., Lv, Y., Li, T., Chen, S., et al. (2021b). Melatonin confers heavy metal-induced tolerance by alleviating oxidative stress and reducing the heavy metal accumulation in ExophialaPisciphila, a dark Septate Endophyte (DSE). BMC Microbiol. 21:40. doi: 10.1186/s12866-021-02098-1
Yu, Y., Wang, A., Li, X., Kou, M., Wang, W., Chen, X., et al. (2018). Melatonin-stimulated triacylglycerol breakdown and energy turnover under salinity stress contributes to the maintenance of plasma membrane H+–ATPase activity and K+/Na+ homeostasis in sweet potato. Front. Plant Sci. 9:256.
Yu, Y., Wang, A., Li, X., Kou, M., Wang, W., Chen, X., et al. (2018b). Melatonin-stimulated triacylglycerol breakdown and energy turnover under salinity stress contributes to the maintenance of plasma membrane HC–ATPase activity and KC/NaC homeostasis in sweet potato. Front. Plant Sci. 9:256. doi: 10.3389/fpls.2018.00256
Yu, Y., Xu, T., Li, X., Tang, J., Ma, D., Li, Z., et al. (2016). NaCl-induced changes of ion homeostasis and nitrogen metabolism in two sweet potato (Ipomoea batatas L.) cultivars exhibit different salt tolerance at adventitious root stage. Environ. Exp. Bot. 129, 23–36. doi: 10.1016/j.envexpbot.2015.12.006
Zhan, H., Nie, X., Zhang, T., Li, S., Wang, X., Du, X., et al. (2019). Melatonin: a small molecule but important for Salt stress tolerance in plants. Int. J. Mol. Sci. 20:709. doi: 10.3390/ijms20030709
Zhang, J., Shi, Y., Zhang, X., Du, H., Xu, B., and Huang, B. (2017). Melatonin suppression of heat-induced leaf senescence involves changes in abscisic acid and cytokinin biosynthesis and signaling pathways in perennial ryegrass (Loliumperenne L.). Environ. Exp. Bot. 138, 36–45. doi: 10.1016/j.envexpbot.2017.02.012
Zhang, R., Sun, Y., Liu, Z., Jin, W., and Sun, Y. (2021). Effects of melatonin on seedling growth, mineral nutrition, and nitrogen metabolism in cucumber under nitrate stress. J. Pineal Res. 62:E12403. doi: 10.1111/jpi.12403
Zhang, N., Sun, Q. Q., Zhang, H. J., Cao, Y. Y., Weeda, S., Ren, S. X., et al. (2015). Roles of melatonin in abiotic stress resistance in plants. J. Exp. Bot. 66, 647– 659. doi: 10.1093/jxb/eru336
Zhang, X. W., Liu, F. J., Zhai, J., Li, F. D., Bi, H. G., Ai, X. Z., et al. (2020). Auxin acts as a downstream signaling molecule involved in hydrogen sulfide-induced chilling tolerance in cucumber. Planta 251:69.
Zhang, Q., and Wang, C. (2020). Natural and human factors affect the distribution of soil heavy metal pollution: a review. Water Air Soil Pollut. 231, 1–13. doi: 10.1007/s11270-020-04728-2
Zhang, T., Wang, Y., Ma, X., Ouyang, Z., Deng, L., Shen, S., et al. (2022). Melatonin alleviates copper toxicity via improving ROS metabolism and antioxidant defense response in tomato seedlings. Antioxidants 11:758. doi: 10.3390/antiox11040758
Zhang, H. Z., Yang, J. L., Li, W. L., Chen, Y. X., Lu, H., Zhao, S. C., et al. (2019). PuHSFA4a enhances tolerance to excess Zn by regulating ROS production and root development in Populus. Plant Physiol. 180, 2254–2271. doi: 10.1104/pp.18.01495
Zhang, H. J., Zhang, N., Yang, R. C., Wang, L., Sun, Q. Q., Li, D. B., et al. (2014). Melatonin promotes seed germination under high salinity by regulating antioxidant systems, ABA and GA4 interaction in cucumber (Cucumis sativus L.). J. Pineal Res. 57, 269–279.
Zhao, D., Wang, H., Chen, S., Yu, D., and Reiter, R. J. (2021). Phytomelatonin: an emerging regulator of plant biotic stress resistance. Trends Plant Sci. 26, 70–82. doi: 10.1016/j.tplants.2020.08.009
Zhao, D., Yu, Y., Shen, Y., Liu, Q., Zhao, Z., Sharma, R., et al. (2019). Melatonin synthesis and function: evolutionary history in animals and plants. Front. Endocrinol. 10:249. doi: 10.3389/fendo.2019.00249
Keywords: signaling molecule, sRNAs analysis, transcriptomics, genetic modification, ionomics
Citation: Ali S, Gill RA, Shafique MS, Ahmar S, Kamran M, Zhang N, Riaz M, Nawaz M, Fang R, Ali B and Zhou W (2022) Role of phytomelatonin responsive to metal stresses: An omics perspective and future scenario. Front. Plant Sci. 13:936747. doi: 10.3389/fpls.2022.936747
Received: 05 May 2022; Accepted: 08 August 2022;
Published: 06 September 2022.
Edited by:
Mukhtar Ahmed, Pir Mehr Ali Shah Arid Agriculture University, PakistanReviewed by:
Noshin Ilyas, Pir Mehr Ali Shah Arid Agriculture University, PakistanCopyright © 2022 Ali, Gill, Shafique, Ahmar, Kamran, Zhang, Riaz, Nawaz, Fang, Ali and Zhou. This is an open-access article distributed under the terms of the Creative Commons Attribution License (CC BY). The use, distribution or reproduction in other forums is permitted, provided the original author(s) and the copyright owner(s) are credited and that the original publication in this journal is cited, in accordance with accepted academic practice. No use, distribution or reproduction is permitted which does not comply with these terms.
*Correspondence: Weijun Zhou, d2p6aG91QHpqdS5lZHUuY24=; Basharat Ali, ZHIuYmFzaGFyYXRAa2Z1ZWl0LmVkdS5waw==
Disclaimer: All claims expressed in this article are solely those of the authors and do not necessarily represent those of their affiliated organizations, or those of the publisher, the editors and the reviewers. Any product that may be evaluated in this article or claim that may be made by its manufacturer is not guaranteed or endorsed by the publisher.
Research integrity at Frontiers
Learn more about the work of our research integrity team to safeguard the quality of each article we publish.