- 1Rhizobiology Group, Department of Agronomic and Applied Molecular Sciences, Faculty of Agriculture and Veterinary Medicine, University of Buea, Buea, Cameroon
- 2Research Group on Beneficial Microorganisms and Plant Interactions, Leibniz Institute of Vegetable and Ornamental Crops, Großbeeren, Germany
- 3Institute of Agricultural Research for Development (IRAD), Yaoundé, Cameroon
Soybean [Glycine max (L.) Merrill] cultivation is important for its dual role as rich source of dietary protein and soil fertility enhancer, but production is constrained by soil nutrient deficiencies. This is often resolved using chemical fertilizers that exert deleterious effects on the environment when applied in excess. This field study was conducted at Nkolbisson-Yaoundé in the agro-ecological zone V of Cameroon to assess the performance of soybean when inoculated with plant growth-promoting bacteria (PGPB) and arbuscular mycorrhiza fungi (AMF), with or without NPK fertilizer addition. Ten treatments (Control, PGPB, AMF, PGPB+AMF, PGPB+N, PGPB+PK, PGPB+N+PK, PGPB+AMF+N, PGPB+AMF+PK, and PGPB+AMF+N+PK) were established in a randomized complete block design with three replicates. Mycorrhizal colonization was only observed in AMF-inoculated soybean roots. In comparison to control, sole inoculation of PGPB and AMF increased the number of root nodules by 67.2% and 57%, respectively. Co-application of PGPB and AMF increased the number of root nodules by 68.4%, while the addition of NPK fertilizers significantly increased the number of root nodules by 66.9–68.6% compared to control. Acid phosphatase activity in soybean rhizosphere ranged from 46.1 to 85.1 mg h–1 kg–1 and differed significantly across treatments (p < 0.001). When compared to control, PGPB or AMF or their co-inoculation, and the addition of NPK fertilizers increased the acid phosphatase activity by 45.8%, 27%, 37.6%, and 26.2–37.2%, respectively. Sole inoculation of PGPB or AMF and their integration with NPK fertilizer increased soybean yield and grain contents (e.g., carbohydrate, protein, zinc, and iron) compared to the control (p < 0.001). Soil phosphorus correlated significantly (p < 0.05) with soybean grain protein (r = 0.46) and carbohydrate (r = 0.41) contents. The effective root nodules correlated significantly (p < 0.001) with acid phosphatase (r = 0.67) and soybean yield (r = 0.66). Acid phosphatase correlated significantly (p < 0.001) with soybean grain yield (r = 0.63) and carbohydrate (r = 0.61) content. Effective root nodules correlated significantly with carbohydrate (r = 0.87, p < 0.001), protein (r = 0.46, p < 0.01), zinc (r = 0.59, p < 0.001), and iron (r = 0.77, p < 0.01) contents in soybean grains. Overall, these findings indicate strong relationships between farm management practices, microbial activities in the rhizosphere, and soybean performance.
Introduction
Soybean [Glycine max (L.) Merrill] plays crucial roles in food and nutrition security due to its high nutrient contents, while its ability to biologically fix atmospheric nitrogen in symbiosis with Rhizobia enhances the productivity of agricultural systems. Soybean production in Cameroon has been increasing since 2010, and it is the second most cultivated legume after peanuts, with the rapid development of cultivated areas from 6,705 ha in 2008 to 15,020 ha in 2018 (WWF, 2014; Nyahnone, 2017; Nzossié and Bring, 2020). Macroeconomic data show that Cameroon imports an average of 20,000 tons of soybeans worth approximately CFAF 10 billion a year (WWF, 2014), and GMO soybean meal worth CFAF 14 billion (Nyahnone, 2017). Hence, there is a challenge to increase domestic supply to meet agro-industrial demand, which is indicative of the enthusiasm of farmers for soybean production. Wendt and Atemkeng (2004) reported soybean yield ranging between 448 and 709 kg/ha across the first and second planting seasons, with a significant effect of soil nutrients (especially magnesium content) on soybean yield.
Poor soil fertility is a major constraint for crop production in Cameroon with nitrogen (N) and phosphorus (P) as the main limiting elements (Tening et al., 2013; Ngosong et al., 2019; Nanganoa et al., 2020). Soil nutrient deficiencies are commonly resolved using chemical NPK fertilizers that are deleterious to the environment and humans when applied in excess, which has necessitated alternative management practices that can foster crop productivity without jeopardizing sustainability (Ntambo et al., 2017; Mahmud et al., 2020; Mndzebele et al., 2020). A promising alternative to increase crop performance is the use of beneficial microbes to enhance soil fertility, plant nutrition, and protection (Korir et al., 2017; Tchakounté et al., 2020). Despite the widely demonstrated importance of soil beneficial microbes in fostering biotic interactions in the rhizosphere and improving crop performance, biofertilizers have not been fully incorporated in farming systems relative to chemical fertilizers. Although proficient microbes can sustainably improve plant nutrition and protection (Bender et al., 2016; Venneman et al., 2017; Bello et al., 2018), microbial products are still largely untapped in Africa. Hence, there is a need to develop local microbial biofertilizers that can be harnessed to enhance the grain yield and nutrient contents of soybeans (Sogut, 2016; Marro et al., 2020; Zhang et al., 2020).
Besides the high nutrient contents and income generation potential of soybean cultivation, they additionally improve soil nitrogen via symbiotic biological N2 fixation (Alam et al., 2015; Kalayu, 2019; Soumare et al., 2020). Indigenous or inoculated plant growth-promoting bacteria (PGPB) can improve crop yield via biological N2 fixation, solubilization of inorganic phosphate, or production of phytohormones (Backer et al., 2018; Rosenblueth et al., 2018; Tchakounté et al., 2018; Bechtaoui et al., 2020). Some microbes mediate crop growth via secretion of metabolites, drought tolerance, and protection against pests and diseases (Radhakrishnan et al., 2017). Phosphate-solubilizing bacteria can convert inorganic or organically bound phosphate into bioavailable hydrogen-phosphate ions (H2PO4– or HPO42–) through solubilization and mineralization processes (Behera et al., 2017). These microbes facilitate the conversion of complex forms of N and P to simple available forms for root uptake to enhance crop growth and yield (Kang et al., 2014, 2015; Kuan et al., 2016). Some Bacillus spp. release ammonia from nitrogenous organic matter in the soil or have nifH gene that produces nitrogenase for N2 fixation to supply plants and enhance yield (Ding et al., 2005; Hayat et al., 2010; Kuan et al., 2016). Iron chelation by Bacillus spp. via siderophore production facilitates solubilization of iron from minerals and organic compounds in the rhizosphere by binding Fe3+ in complex substances and reducing them to Fe2+ for plant uptake (Walker and Connolly, 2008; Nadeem et al., 2012).
The bacteria biofertilizer used in this study comprised a consortium of symbiotic Rhizobium for N2 fixation via root nodules to directly support the plants, and non-symbiotic PGPB to freely fix N2 in the rhizosphere for uptake by soybean roots. In addition, inoculation of arbuscular mycorrhiza fungi (AMF) was intended to boost soybean performance by indirectly modulating soil enzyme activities associated with the processes of N2 fixation, P solubilization, and mineralization, or by directly supplying the plants with N and P via its hyphal transport network. Arbuscular mycorrhiza fungi (AMF) exert significant positive effects on N2 fixation via direct or indirect interactions with PGPB through nutrient transport and crop protection (Daniel et al., 2020; Novais et al., 2020). Moreover, microbial activities in the rhizosphere and plant performance can be enhanced by incorporating appropriate amounts of chemical NPK fertilizers in combination with microbial inoculants (Islam et al., 2017; Ntambo et al., 2017; Herliana et al., 2019). Chemical NPK fertilizers were applied in combination with PGPB and/or AMF to assess the possibility of boosting the potential of biofertilizers to enhance soybean performance within the nexus of integrated soil fertility management (Vanlauwe et al., 2010; Kanomanyanga et al., 2021). Hence, this study aims at evaluating microbial dynamics in the soybean rhizosphere, and soybean productivity as influenced by the application of locally produced biofertilizer, with or without the addition of chemical NPK fertilizers. It was hypothesized that inoculating PGPB and AMF will enhance microbial activities in the rhizosphere, including root nodulation and acid phosphatase, and increase the soybean grain yield and nutrient contents.
Materials and methods
Experimental site and setup
The experiment was conducted from April to July 2021 at the Institute of Agricultural Research for Development (IRAD) Nkolbisson, Yaoundé, Cameroon. The site is located in agro-ecological zone V of Cameroon, which is a humid forest zone situated between Latitude 03° 8’ 71.2” N and Longitude 11° 45’ 38.0” E. The area has an equatorial climate with a mean annual temperature of 23.5°C (ranging between 16°C and 31°C), and 1,600 mm rainfall that occurs in a bimodal configuration such that the first and second cropping seasons are separated by a 4-month dry season, which lasts from mid-March to early July and from late August to mid-November, respectively (Ambassa-Kiki and Nill, 1999). However, the duration of each season presently varies and the second season rains are erratic due to global climate change dynamics.
Rice was previously cultivated on the field site from 2016 to 2019 and fallowed for 1 year in 2020 before this study. The field experiment was laid out as a randomized complete block design with ten treatments and three replicates each, giving a total of thirty experimental units. The treatments include T1 – control (no input), T2 – plant growth-promoting bacteria (PGPB), T3 – Arbuscular mycorrhiza fungi (AMF), T4 – PGPB+AMF, T5 – PGPB+N, T6 – PGPB+PK, T7 – PGPB+N+PK, T8 – PGPB+AMF+N, T9 – PGPB+AMF+PK, and T10 – PGPB+AMF+N+PK. Each experimental plot measured 3.2 m × 4 m (12.8 m2) with a 1 m buffer zone between plots and a 1.5 m buffer between the replicate blocks.
The experimental site was cleared and weed regrowth was allowed for 2 weeks, and the emerging weeds were sprayed with a systemic herbicide (Roundup 360SL, Belgium; comprising active components glyphosate) at a rate of 129.6 kg in 360 L of water ha–1. Three days after the application of herbicide, all plots were tilled manually to produce raised beds of approximately 30 cm high (Figure 1), and soybean was planted 1 week after herbicide spray. Three soybean seeds (Panorama 357 variety) were planted per hole at approximately 4 cm depth and 10 cm intra-row and 40 cm inter-row spacing, making 7 rows per plot. Thinning was done after germination to two vigorous plants per hole, giving a total of 382,813 plants ha–1. Synthetic insecticide K-Optimal (SCPA SIVEX International France; comprising active components Lambda—cyhalothrine 15 g L–1 + Acetamipride 20 g L–1) and fungicide Monchamp 72 WP (Mancozeb 60% + Metalaxyl 12%) were applied at 3 weeks after sowing to control insect pests and fungal infections. Each was applied at the rate of 150 ml in 15 L of knapsack sprayer, which is equivalent to 2 L ha–1 each. The field was regularly monitored and weeding was done manually.
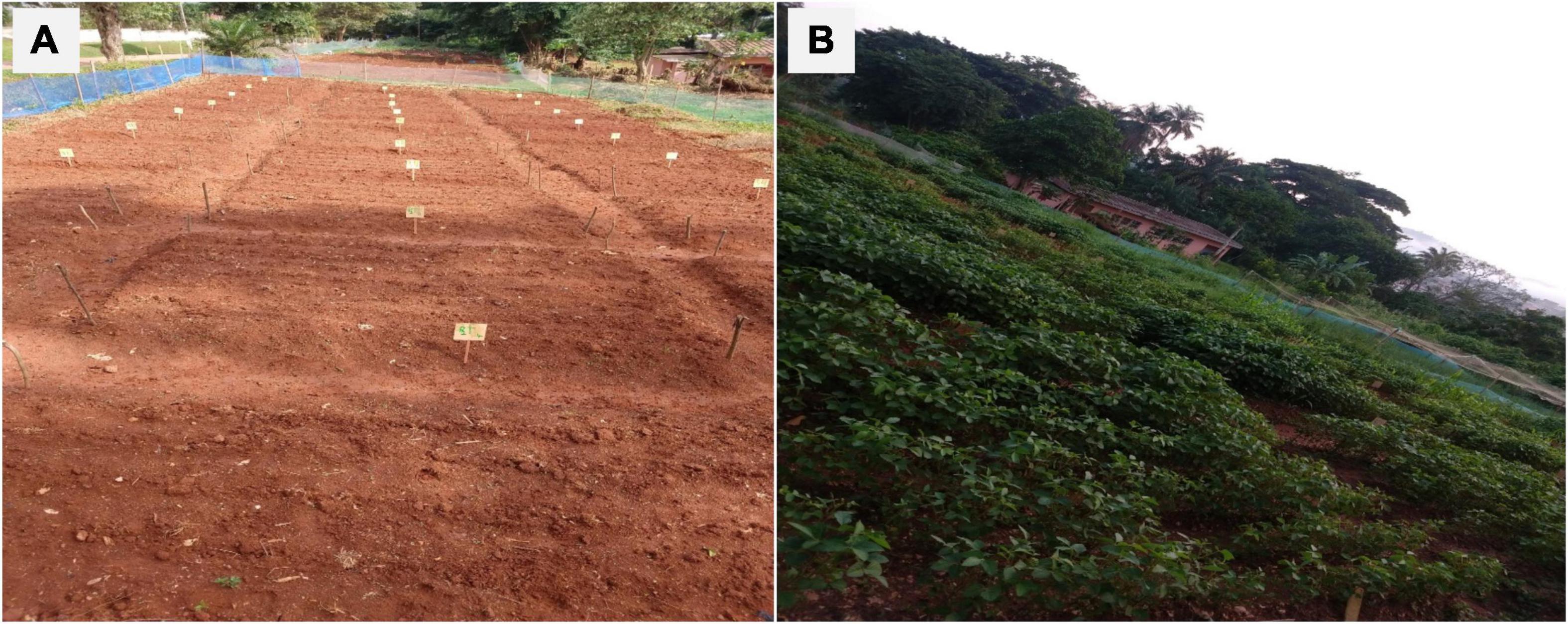
Figure 1. Experimental site with tilled soil beds before sowing (A) and soybean plants at full podding (B).
Microbial inoculation
Plant growth-promoting bacteria
The bacterial inoculant used in this study consisted of a consortium of symbiotic and non-symbiotic plant growth-promoting bacteria—PGPB (Table 1). The non-symbiotic PGPB included the following organisms: (03) Arthobacter sp., (03) Bacillus sp., (01) Lysinibacillus sp., (03) Paenibacillus sp., and (01) Sinomonas sp., which were isolated from the rhizosphere of maize plants in Cameroon (Tchakounté et al., 2018); and (01) Kosakosania radicincitans isolated from the phyllosphere of winter wheat in Germany and deposited in NCBI as DSM 16656T GenBank: CP018016.1, CP018017.1, CP018018.1 (Ruppel and Merbach, 1995; Becker et al., 2018). The symbiotic bacterium (Bradyrhizobium japonicum) was obtained from the Soil Microbiology Laboratory of the Biotechnology Center of the University of Yaoundé I, Cameroon.
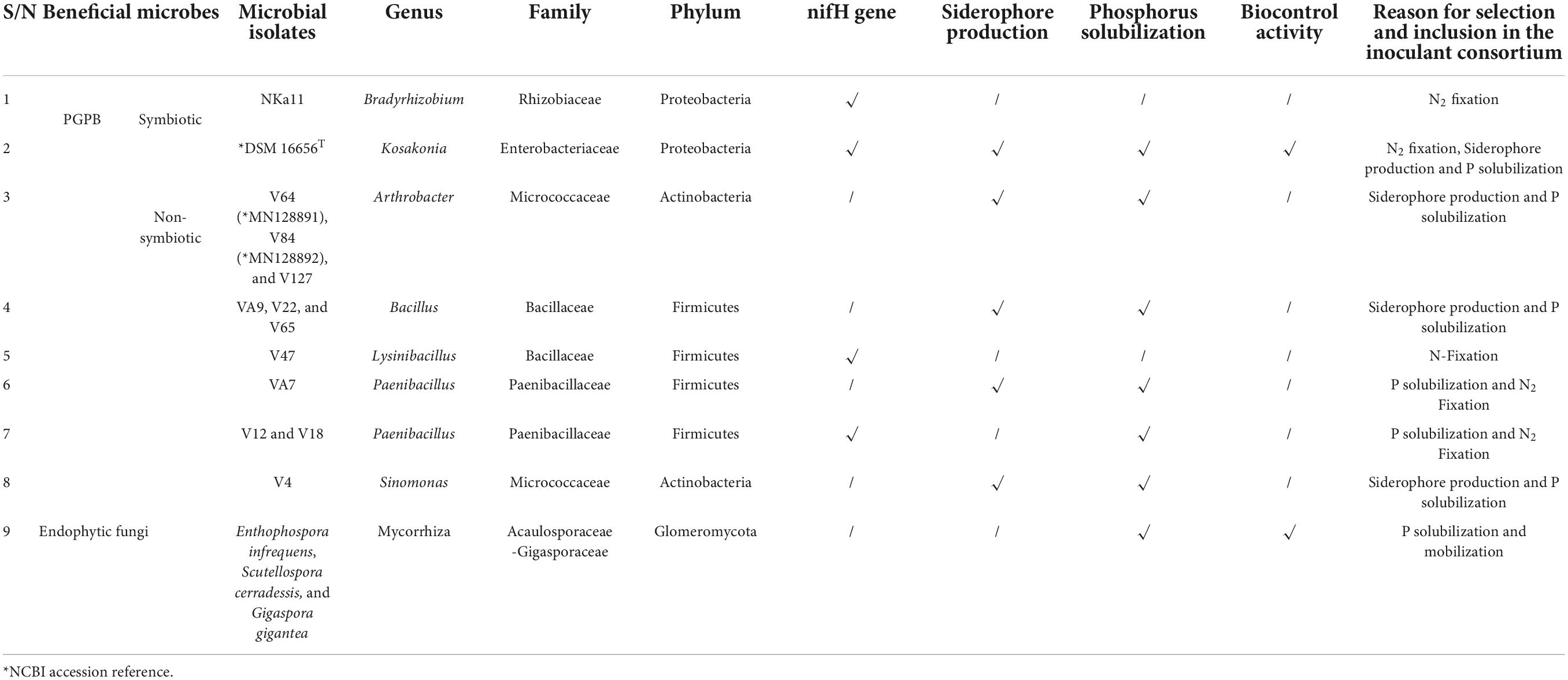
Table 1. Plant growth-promoting bacteria (PGPB) and arbuscular mycorrhiza fungi, their functional traits, and potential roles in the consortium of biofertilizers to enhance soil fertility and performance of soybean plants.
For the production of microbial inoculant consortium, a colony of symbiotic B. japonicum was collected from an inoculum stock and transferred into a 500-ml flask containing 100 ml sterilized yeast mannitol broth (YMB), and incubated in a shaker at 28°C at 200 rpm for 48 h. From a stock culture of each of the non-symbiotic plant growth-promoting bacteria, a pure colony was collected and transferred into a 500-ml flask containing 100 ml sterilized nutrient broth (Standard nutrient broth I, Carl Roth, Germany), and incubated at 28°C for 24–48 h. The individually cultured symbiotic and non-symbiotic plant growth-promoting bacteria were assembled into a microbial consortium in a 5 L container and sugar was added (1:1) to serve as an adjuvant because the B. japonicum used in this study is not sticky as compared to the non-symbiotic microbes. The soybean seeds were immersed in the microbial inoculant consortium (e.g., 1 kg of soybean seeds per 100 ml of biofertilizer inoculum) and thoroughly mixed. The seeds were removed from the inoculum and allowed to air-dry for 1 h before planting (Atieno et al., 2012).
Arbuscular mycorrhiza fungi
The composite inoculant product used for this experiment was obtained from the Regional Biocontrol and Applied Microbiology Laboratory of IRAD, Nkolbisson, Cameroon. It comprised the three most dominant arbuscular mycorrhiza strains (Enthophospora infrequens, Scutellospora cerradessis, and Gigaspora gigantea) identified in the soybean rhizosphere of six high-intensity soybean-producing areas across the five different agro-ecological zones of Cameroon. In total, 300 g of mycorrhiza inoculum stock was used to produce a bulk inoculum using a sterilized mixture of fine and coarse sand substrates (1:1), sterilized at 121°C for 1 h in an autoclave (model PTS-B100L). Three polypropylene bags were filled with 20 kg each of sterilized substrates, and highly mycotrophic Sorghum bicolor plants were planted and maintained in the greenhouse for 3 months with regular irrigation, before subjecting the plants to water stress for 1 month to stimulate sporulation by mycorrhiza. The suitability of the mycorrhiza inoculum was determined by the spore density, which was determined from a 100 g sample of the homogenized inoculant product by immersing in 300 ml distilled water in a 1,000-ml beaker, and the mixture was stirred and allowed to stand for 15 s. Four sieves were arranged in decreasing order of mesh size (710, 225, 125, and 45 μm) and used to filter the supernatant of each mixture. The contents of the last three sieves were collected, washed, and the number of observed mycorrhiza spores was counted on a square graduated petri-dish using a stereo microscope (WILD M2B, Germany). The observed spore density of 670 spores 100 g–1 of inoculum was considered suitable for use as AMF inoculum. The AMF inoculum was air-dried and used for field inoculation at 20 g per soybean stand (containing approximately 134 spores). The inoculum was applied by placing the 20 g inoculum at about 80 mm depth in the planting hole before planting soybean seeds.
Application of chemical fertilizers
So far there is no specific fertilizer recommendation for soybean production on the study site. Therefore, the application of nitrogen as urea, phosphorus as triple superphosphate (TSP), and potassium as muriate of potash (MOP) was based on general fertilizer recommendations for soybean. Urea was applied at the rate of 40 kg N ha–1 (Uko et al., 2002) as two split doses of 20 kg N ha–1 each 2 weeks after planting and beginning of seed development at the R5 stage. Phosphorus was applied at 30 kg P2O5 ha–1 (Lamptey et al., 2014) and potassium at 40 kg K2O ha–1 (Islam et al., 2017) 2 weeks after planting. All fertilizers were applied by ringing at approximately 5 cm from plants to avoid burns and minimize nutrient loss through leaching and volatilization.
Data collection
Soil properties
Pre-planting soil was sampled for the entire experimental site after clearing and laying out but before tillage, while post-planting soil was sampled for each plot at harvest. An auger was used to collect three pre-planting soil samples randomly using a Z-pattern at 0–15 cm depth and bulked to form a composite sample. Three post-planting soil samples were also collected randomly from each treatment plot at 0–15 cm depth using an auger, and thoroughly mixed to form a composite sample. All soil samples were air-dried at room temperature and stored in polybags before analysis. The soil samples were crushed and sieved through a 2-mm sieve for the determination of soil’s physical and chemical properties.
The soil particle size distribution was determined using the pipette sampling method with sodium hexametaphosphate as a dispersing agent, and the textural class was assigned according to USDA textural triangle (Van Reeuwijk, 1992). The soil pH was determined potentiometrically in water (H2O) and 1 N potassium chloride (KCl) solutions after 24 h in soil suspension (soil/liquid 1:2.5 w/v) using a glass electrode pH meter. The exchangeable bases (Ca2+, Mg2+, K+, and Na+) were extracted using 1 N ammonium acetate (NH4CH3CO2) solution at pH 7. Calcium (Ca) and magnesium (Mg) were determined by the titration method using Eriochrome Black T (EBT or Erio T) as an indicator while potassium (K) and sodium (Na) were determined using the flame photometer (Rowell, 1994). Exchange acidity was extracted with 1 N KCl and determined by titrating the extract with 0.01 N NaOH, using a phenolphthalein indicator (Van Reeuwijk, 1992). Effective cation exchange capacity (ECEC) was determined by the summation of exchangeable bases and exchange acidity. The total soil nitrogen (N) was determined by the macro Kjeldahl digestion method (Bremner and Mulvaney, 1982). Plant available phosphorus (P) in the soil was determined by the Bray II method (Van Reeuwijk, 1992), and organic carbon was determined by the wet oxidation method (Kalra and Maynard, 1991).
Mycorrhiza colonization
An assessment of AMF root colonization was conducted according to Begoude et al. (2016). Briefly, 1–2 cm segments of root samples were placed in 5% KOH solution for 24 h at room temperature and rinsed three times with water on a fine sieve. Root samples were acidified in 10% HCl (v/v) for 15 min and stained with 0.01% (w/v) fuchsine acid for 24 h at room temperature. Root segments were randomly selected from the stained samples and three replicates of 10 roots per slide were assessed for the occurrence of AMF structures (e.g., vesicles, arbuscules, and hyphae) using an optical microscope (Biological compound microscope with replaceable LED light, OMAX 40X-2500X Trinocular, Germany) (Figure 2). The mycorrhizal frequency (F%) was given as the ratio of colonized root fragments to the total number of observed root fragments.
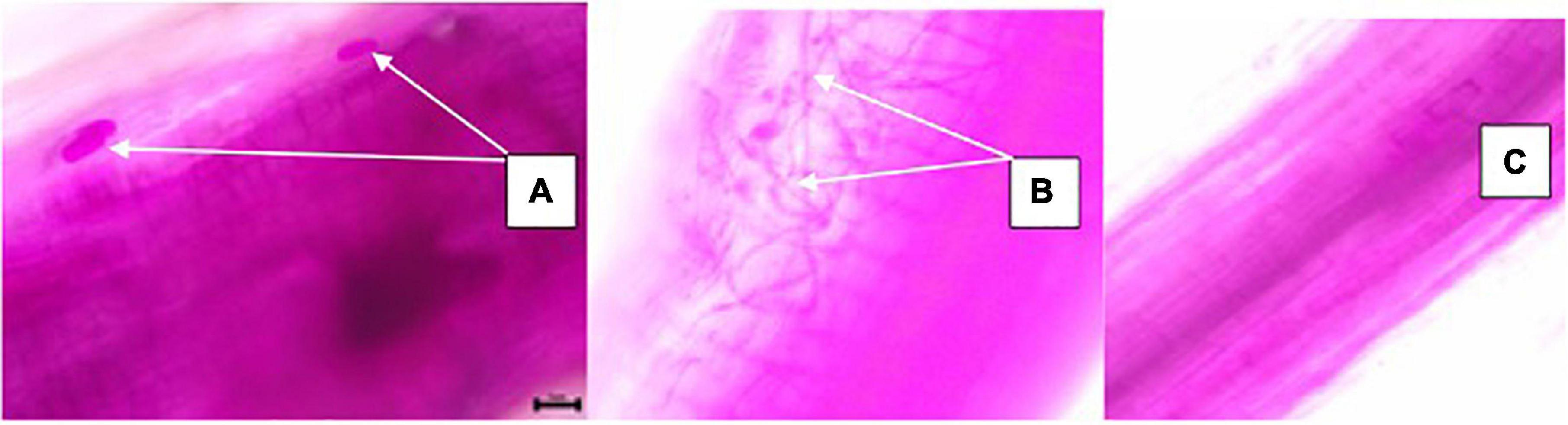
Figure 2. Microscopic identification of mycorrhiza structures in soybean roots; Vesicles (A), Hyphae (B), and un-infected roots (C) as influenced by inoculation of plant growth-promoting bacteria (PGPB) and arbuscular mycorrhiza fungi (AMF), with or without the addition of nitrogen (N), phosphorus (P), and potassium (K) fertilizers.
Quantification of rhizosphere acid phosphatase activity
Five plants were randomly selected from the center of each plot at full podding for assessment of acid phosphatase activity. A spade was used to dig approximately 5 cm around each plant at the depth of 20 cm, and 1 g root adhering soil was collected from each plant and bulked to form a composite sample of 5 g, from which 1 g was taken into a microcentrifuge tube and 0.5 ml of 100 mM phosphate buffer was added. P-nitrophenyl phosphate (p-NPP, 10 mM) in 100 μl solution was used as substrate. The final volume of the reaction mixture was adjusted to 1 ml by adding the required amount of distilled water. The tube was vortexed (2 min) at room temperature and incubated at 37°C for 60 min in shake condition (100 rpm). After the incubation, samples were centrifuged at 10,000 rpm (5 min) and the clear supernatants were transferred into clean test tubes and 2 ml of 1 M NaOH was added. The yellow filtrate was analyzed using a colorimeter (Klett colorimeter, Clinical model, 800-3, 115 VAC) at λ = 430 nm. The concentration of soluble protein in the supernatant was determined using the Bradford reactive procedure, and the specific activity of soil acid phosphatase was estimated using the formula:
Where, 10 = constant, C = concentration of soluble protein, and 17,800 is the molecular extension coefficient of phosphatase. All derived values were then converted to mg h–1 kg–1 soil by multiplying by 100,000.
Root nodulation
Five plants were randomly selected from the second border rows of each plot at full podding and assessed for nodulation parameters. The nodulation rating was assessed according to Tamiru et al. (2012) as follows:
Where the number of plants showing tap root nodulation (a), plants with nodules in secondary roots but close to tap root (b), plants with scattered nodulation (c), and plants without nodulation (d) were evaluated.
The nodule volume was also assessed according to Tamiru et al. (2012), where nodules of the five sampled plants were immersed in a 50 ml plastic cylinder containing 30 ml of water. The amount of water displaced after immersion of the nodules was recorded and the mean was considered as nodule volume per plant. For the effectiveness of root nodules, all the nodules from the five sampled plants per treatment were dissected using a sharp knife, and a hand lens was used to observe the internal nodule color. The presence of pink or reddish coloration was considered as effective and used to distinguish nodules that are actively fixing nitrogen from the inactive nodules (Ngeno et al., 2012).
Soybean grain yield
Soybean plants were harvested at physiological maturity and the weight of 1,000 randomly selected grains from each plot maintained at 10% moisture content was reported as a thousand grain weight. Soybean grain yield was obtained by adjusting the moisture level to 10% according to the following formula and converted to tons ha–1:
Where MC is the moisture content of soybean seeds at the time of measurement, and 10 is the percentage standard moisture content of soybean seeds at harvest.
Nutrient contents of soybean grains
The proximate (e.g., protein and carbohydrate) composition of soybean grains were determined according to the official method described by the Association of Official and Analytical Chemist (AOAC, 2005). Each sample was analyzed in triplicates and values were presented in percentages. The Anthrone standard method (David, 1978) was used to estimate the content of carbohydrates where different volumes of glucose solution from 200 μg mol–1 stock solution were pipetted and made up to 1 ml with distilled water. In total, 15 tubes were used with tube 1 considered as blank, tubes 2–9 were used to construct a standard curve, and tubes 10–15 were used for unknown samples. Anthrone (5 ml) was added to each tube and vortexed to thoroughly mix and allowed to cool. The tubes were covered with marble/caps and incubated at 90°C for 17 min and allowed to cool at room temperature, and optical density was measured at 620 nm against the blank sample. The amount of glucose in the unknown sample was determined by plotting a standard curve of A620 on Y-axis and μg glucose on X-axis.
Crude protein was determined using the Kjeldahl digestion method by measuring the nitrogen content of the soybean grain samples and multiplying it by a factor of 6.25, based on the fact that protein contains approximately 16% nitrogen. Approximately 2 g of crushed soybean grain powder was weighed into a Kjeldahl flask and 25 ml of concentrated sulfuric acid, 0.5 g of copper sulfate, 5 g of sodium sulfate, and a speck of selenium tablet acid were added. Heat was first applied in a fume cupboard slowly to prevent undue frothing. The digestion continued for 45 min until the digestate became clear pale green. The digestate was transferred into a 100 ml volumetric flask and this was made up to the mark with distilled water. The Kjeldahl distillation apparatus used for distillation was steamed up and 10 ml of the digest was added into the apparatus via a funnel and allowed to boil. Sodium hydroxide (10 ml) was added from the measuring cylinder so that ammonia was not lost. It was later distilled into 50 ml of 2% boric acid containing screened methyl red indicator. The contents of the collecting flask (50 ml) were titrated with sulfuric acid standard volumetric solution using a burette and the amount of titrant used was read. When colorimetric end-point detection was applied, the end-point was reached when the color of the solution changed from green to red. The burette reading was estimated to the nearest 0.01 ml. To confirm that the reagents were free from nitrogen, a blank test was conducted (e.g., performing digestion, distillation, and titration) using only reagents without adding soybean material. The nitrogen content of soybean grains was calculated as follows:
where Va = volume of standard HCl solution when titrating sample, Vb = volume of standard HCl solution when titrating blank, CHCl = concentration of HCl (mol L–1), MN = nitrogen molar mass (g mol–1), and mvz = weight of sample (g).
The mineral content of zinc (Zn) and iron (Fe) were determined using atomic absorption spectrometry according to the standard method (AOAC, 2005). Samples were ashed at 550°C and boiled with 10 ml of 20% hydrochloric acid in a beaker and then filtered into a 100 ml standard flask. This was made up to the mark with distilled water. The mineral content of Zn and Fe was determined from the resulting solution using Atomic Absorption Spectrophotometer at 510, 213.86, 766.5, 213.6 nm. Different electrode lamps were used for each mineral and the equipment was run for standard solutions of each mineral before and during determination to ascertain the efficiency. All values were expressed in mg 100 g–1.
Data analysis
All statistical analyses were done using SPSS (Ver. 23), and data sets were analyzed for normality and homogeneity using Kolmogorov–Smirnov and Levene’s tests, respectively. Data on soil chemical properties, root nodulation, acid phosphatase activity, grain yield, and nutrient contents were subjected to analysis of variance (ANOVA, p < 0.05) to test the effects of treatments as categorical predictors. Significantly different means were separated using Tukey’s HSD test (Tukey’s HSD, p < 0.05). Where applicable, correlation (p < 0.05) was performed to determine the degree of association between the dependent and independent variables.
Results
Soil chemical properties
The baseline soil analysis before establishing the experiment indicates an acidic soil with very low soil nutrient contents (e.g., N, P, Ca, Mg, K, Na) and cation exchange capacity (Table 2). Only the organic C content (1.4–2.4%) is suitable, which results in a quite wide pre-experiment C/N ratio of approximately 20 units. The post-planting soil Ca and Mg contents differed significantly across the experimental treatments. When compared to pre-planting soil, treatments with or without microbial and chemical fertilizer application increased the soil calcium content significantly (F9,20 = 3.78, p < 0.05, Table 2), which ranged from 2.40 to 7.26 cmol kg–1, with the highest in the sole mycorrhiza treatment and the lowest in PGPB+AMF+NPK. The post-planting magnesium content ranged from 1.60 to 8.30 cmol kg–1 of soil, and it was significantly higher in the PGPB+AMF+NPK treatment (F9,20 = 3.43, p < 0.05, Table 2).
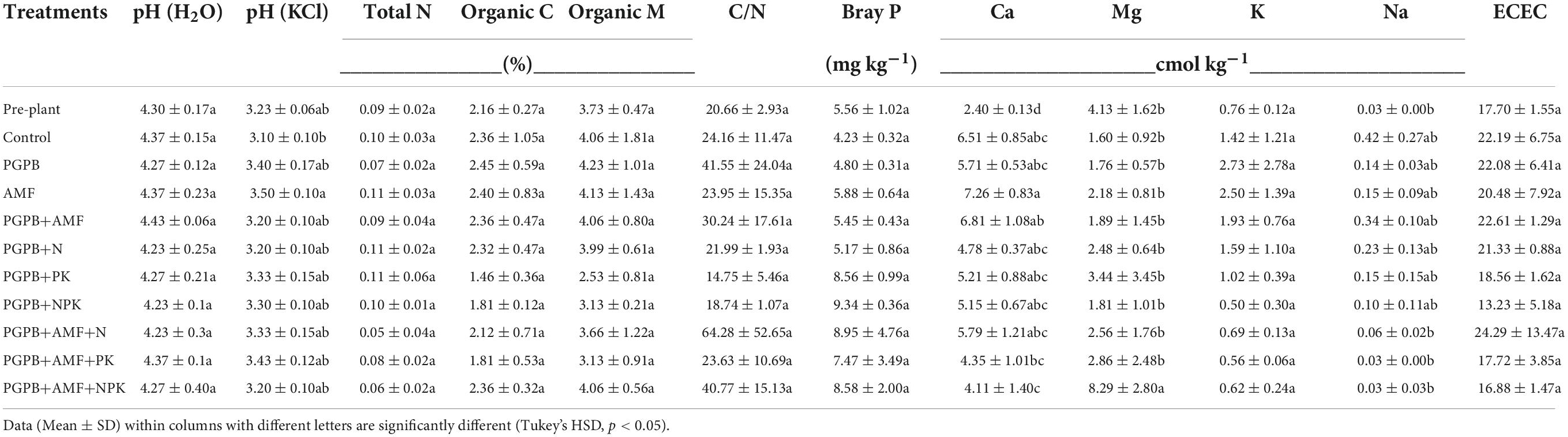
Table 2. Soil properties as influenced by inoculation of plant growth-promoting bacteria (PGPB) and arbuscular mycorrhiza fungi (AMF), with or without the addition of nitrogen (N), phosphorus (P), and potassium (K) fertilizers.
Mycorrhiza colonization and nodulation of soybean roots
The soybean roots were colonized by mycorrhiza at the rate of 51.1–56.7%, which was only observed in plant roots that were inoculated with AMF, but there was no significant difference between the treated plots (p > 0.05, Figure 3). Hence, the addition of PGPB or chemical NPK fertilizer to the AMF treatments did not influence the rate of colonization of soybean roots by AMF. The number of root nodules ranged from 2.9 to 9.1 with the highest in PGPB treatments followed by the sole AMF as compared to the control (F9,20 = 37.53, p < 0.001; Figure 4A). The inoculation of PGPB and AMF increased the number of root nodules by 67.2% and 57%, respectively, as compared to the control, with the PGPB performing 10.2% more than AMF in relation to the control. Meanwhile, the integration of PGPB and AMF increased the number of root nodules by 68.4% compared to the control. Furthermore, the treatments with co-application of biofertilizers (PGPB and AMF) and mineral NPK fertilizer significantly increased the number of root nodules at the range of 66.9–68.6% compared to the control, but there was no significant difference between the treated plots (Figure 4A). A similar trend of results was also observed for the number of effective nodules (F9,20 = 28.85, p < 0.001; Figure 4B). The highest nodulation rating occurred in PGPB treatments compared to the control (F9,20 = 8.36, p < 0.05; Supplementary Table 1). Nodule volume ranged from 0.16 to 0.40 ml per plant and differed significantly (F9,20 = 22.15, p < 0.05; Supplementary Table 1) with the highest in PGPB treatments. The nodule dry weight ranged from 0.017 to 0.038 g with the highest in PGPB treatments compared to the control (F9,20 = 43.38, p < 0.05; Supplementary Table 1). Overall, no additional effect of mineral NPK fertilizer application was detected in all the measured root nodulation parameters.
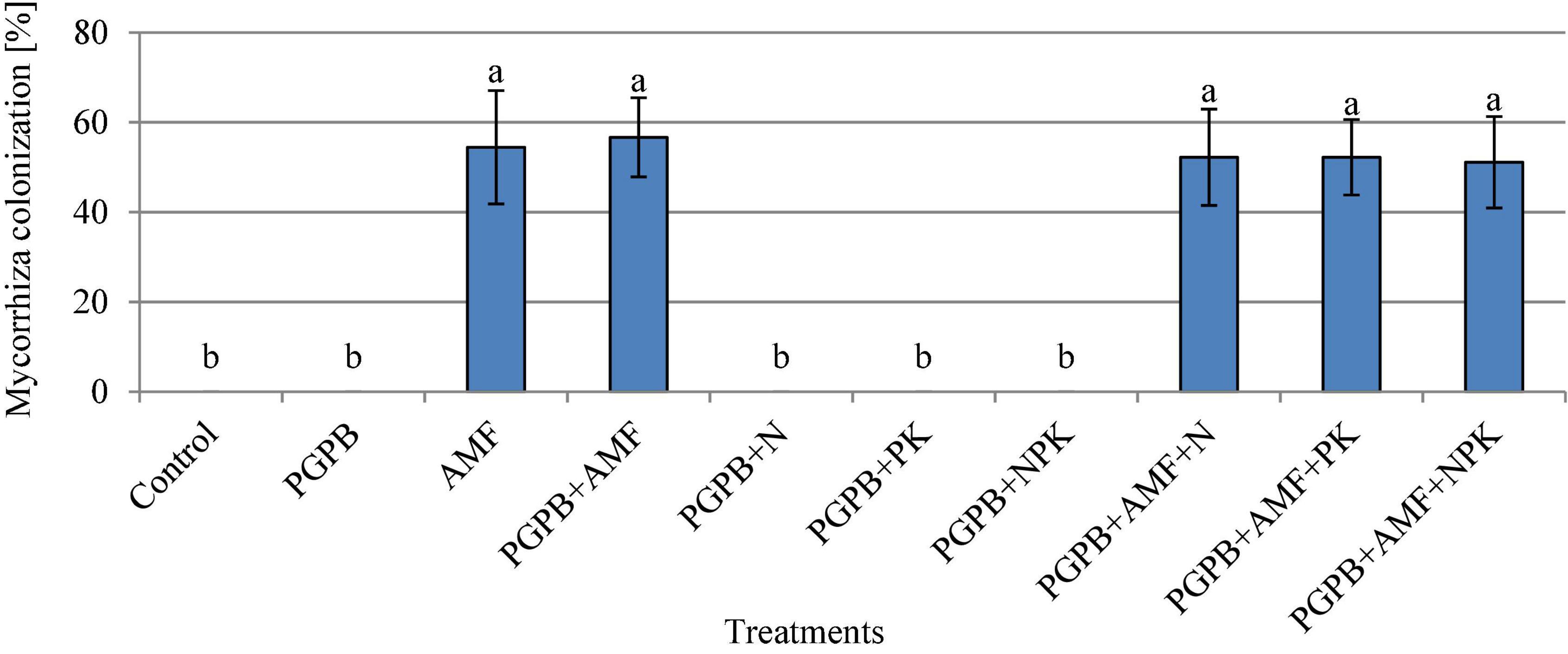
Figure 3. Mycorrhiza colonization of soybean roots affected by inoculation of plant growth-promoting bacteria (PGPB) and arbuscular mycorrhiza fungi (AMF), with or without the addition of nitrogen (N), phosphorus (P), and potassium (K) fertilizers. Data (Mean ± SD) with different letters are significantly different (Tukey’s HSD, p < 0.05).
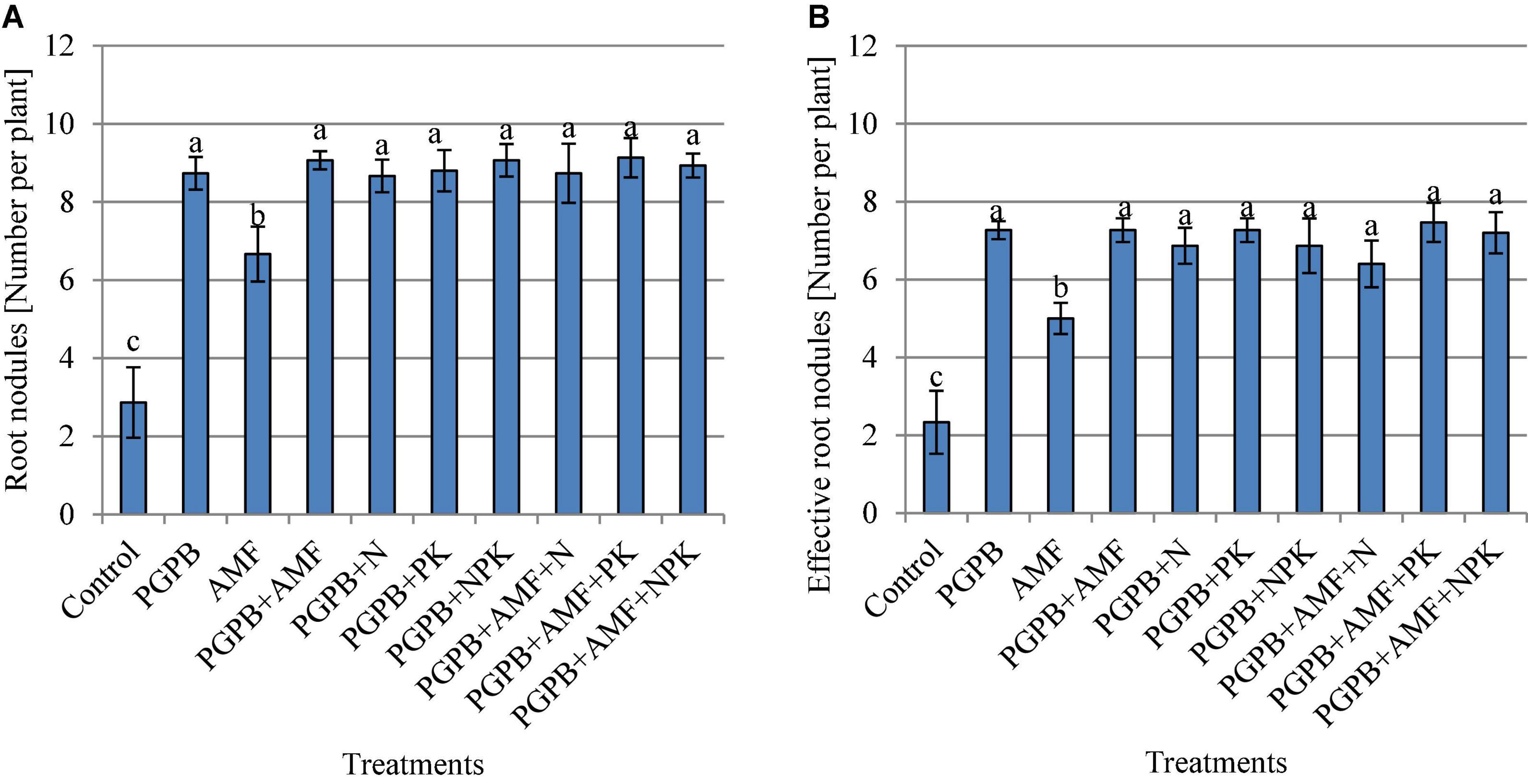
Figure 4. The total number of root nodules per plant (A) and the number of effective root nodules per plant (B) as affected by inoculation of plant growth-promoting bacteria (PGPB) and arbuscular mycorrhiza fungi (AMF), with or without the addition of nitrogen (N), phosphorus (P), and potassium (K) fertilizers. Data (Mean ± SD) with different letters are significantly different (Tukey’s HSD, p < 0.001).
Acid phosphatase activity in the soybean rhizosphere
The acid phosphatase activity in the soybean rhizosphere ranged from 46.1 to 85.1 mg h–1 kg–1, which differed significantly across treatments (F9,20 = 13.25, p < 0.001; Figure 5), with the lowest phosphatase activity recorded in the soybean roots of the control without any microbial or mineral fertilizer application, while the highest occurred in the sole PGPB treatment as compared to all the other treatments. All the microbial treatments almost doubled the acid phosphatase activity in the soybean rhizosphere as compared to the control, with a 45.8% and 27% increase in acid phosphatase activity for PGPB and AMF, respectively, as compared to the control, while the PGPB performed 18.8% more than AMF in relation to the control. Meanwhile, the integration of PGPB and AMF increased the acid phosphatase activity by 37.6% compared to the control, but this was 8.2% lower than the sole PGPB application. Furthermore, the treatments with co-application of biofertilizers (PGPB and AMF) and mineral NPK fertilizer significantly increased the acid phosphatase activity in the range of 26.2–37.2% compared to the control, but there was no significant difference between the NPK fertilizer–treated plots (Figure 5). Meanwhile, the co-application of biofertilizers (PGPB and AMF) and mineral NPK fertilizer significantly reduced the acid phosphatase activity by 13.7–26.5% as compared to the sole PGPB treatment.
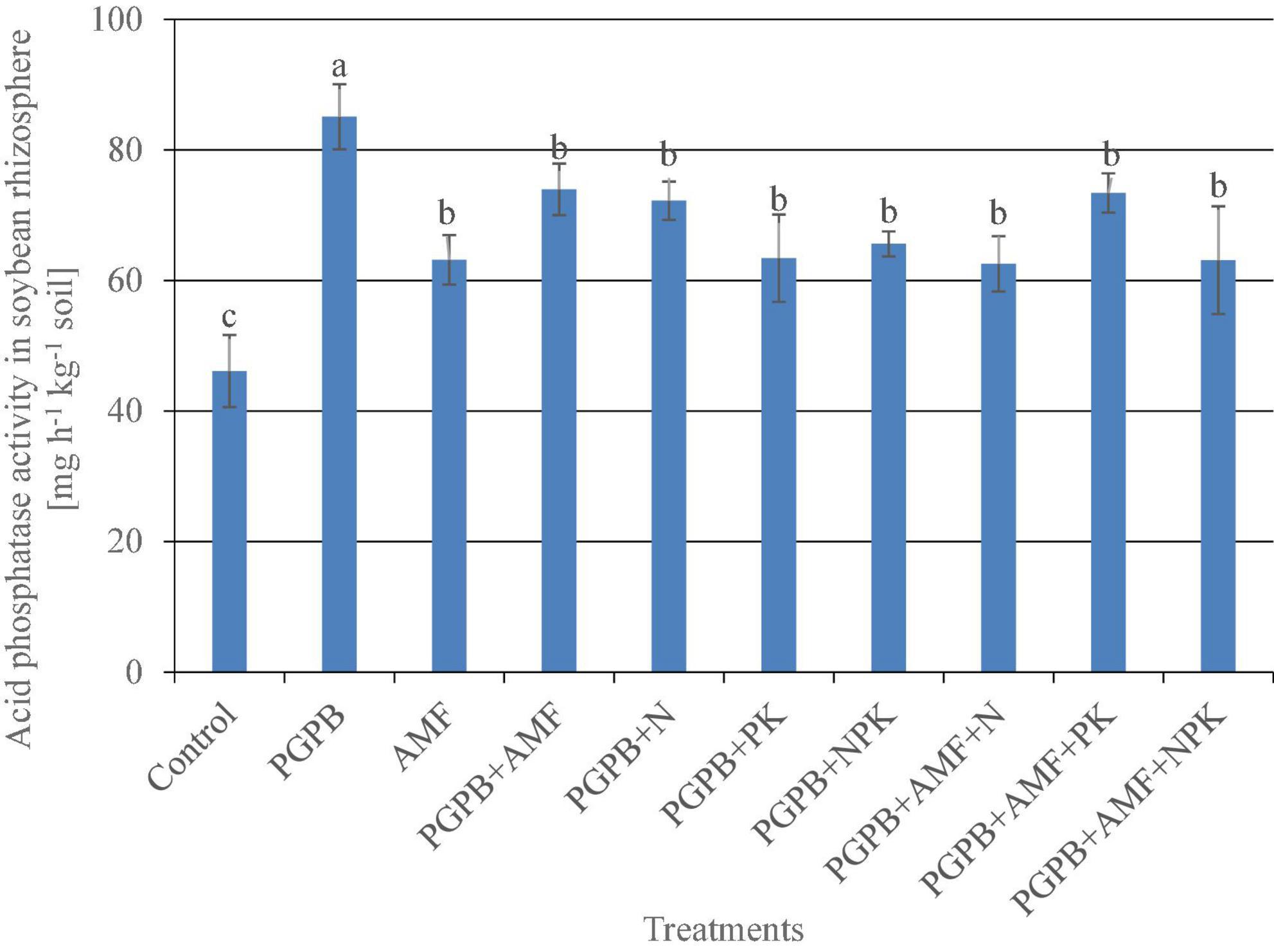
Figure 5. Acid phosphatase activity in the rhizosphere of soybean plants affected by inoculation of plant growth-promoting bacteria (PGPB) and arbuscular mycorrhiza fungi (AMF), with or without the addition of nitrogen (N), phosphorus (P), and potassium (K) fertilizers. Data (Mean ± SD) with different letters are significantly different (Tukey’s HSD, p < 0.001).
Soybean grain yield and nutrient contents
The soybean grain yield ranged between 0.50 and 1.16 tons ha–1 and increased significantly in all applied treatments compared to the non-treated control (F9,20 = 8.83, p < 0.001; Figure 6). The soybean grain yield increased significantly by 46.1–57.1% for all treated plots (sole PGPB or AMF and their combination with NPK fertilizer) in relation to the control, but there was no significant difference between the treated plots. In contrast to soybean grain yield that was not influenced by the integrated application of biofertilizers (PGPB or AMF) and chemical NPK fertilizers, the nutrient contents of soybean grains increased significantly in the integrated biofertilizer and NPK fertilizer treatments as compared to the sole application of biofertilizers (Table 3). All treatments, including biofertilizers alone and their combinations with chemical NPK fertilizers, significantly increased the contents of carbohydrate, protein, zinc, and iron in the soybean grains (Table 3). The carbohydrate content ranged between 20.1 and 22.7% (F9,20 = 5926.53, p < 0.001) and protein ranged between 31 and 39.9% (F9,20 = 3977.2, p < 0.001) across treatments, with the highest in PGPB+AMF+NPK treatment, as compared to the lowest values in the control (Table 3). Inoculating PGPB and AMF with or without NPK fertilizers significantly enhanced the protein content of soybean (F9,20 = 3977.18, p < 0.001; Table 3), which ranged from 31 to 39.9% with the highest in PGPB+AMF+NPK treatment and the lowest in the control. The zinc content ranged between 2.7 and 4.9 mg 100 g–1 (F9,20 = 1842.11, p < 0.001; Table 3) and iron ranged between 16.8 and 19.9 mg 100 g–1 across treatments, again with the highest in PGPB+AMF+NPK addition, as compared to the lowest in the control.
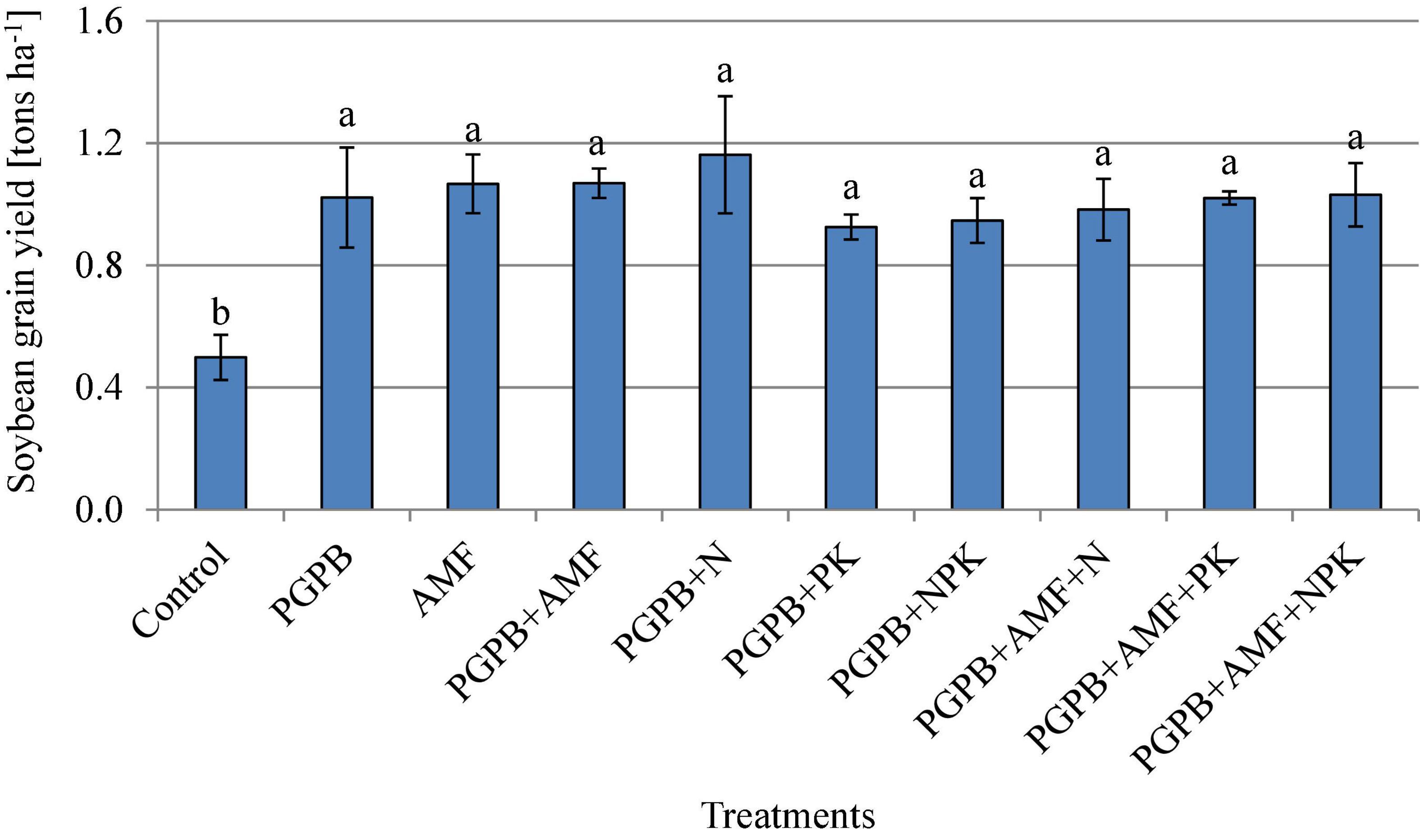
Figure 6. Soybean grain yield (tons ha1) affected by inoculation of plant growth-promoting bacteria (PGPB) and arbuscular mycorrhiza fungi (AMF), with or without the addition of nitrogen (N), phosphorus (P), and potassium (K) fertilizers. Data (Mean ± SD) with different letters are significantly different (Tukey’s HSD, p < 0.001).
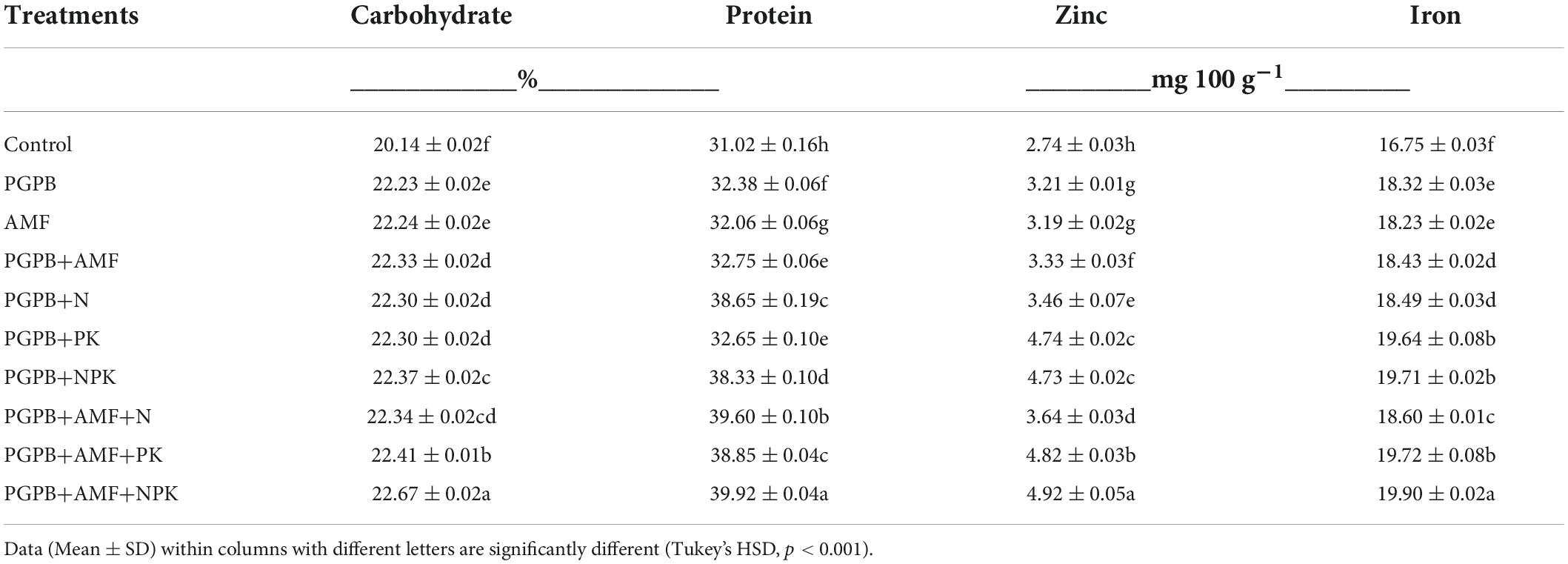
Table 3. Nutrient contents of soybean grains as affected by inoculation of plant growth-promoting bacteria (PGPB) and arbuscular mycorrhiza fungi (AMF), with or without the addition of nitrogen (N), phosphorus (P), and potassium (K) fertilizers.
Correlation of soil parameters and soybean performance
The soil phosphorus correlated significantly (p < 0.05; Table 4) with protein (r = 0.46) and carbohydrate (r = 0.41) contents in soybean grains. The effective root nodules correlated significantly (p < 0.001) with acid phosphatase (r = 0.67; Figure 7A) and soybean yield (r = 0.66; Figure 7B). Acid phosphatase correlated significantly (p < 0.001) with soybean yield (r = 0.63; Figure 7C) and carbohydrate (r = 0.61; Table 4) contents in the grains. The effective root nodules correlated significantly with carbohydrate (r = 0.87, p < 0.001), protein (r = 0.46, p < 0.01), zinc (r = 0.59, p < 0.001), and iron (r = 0.77, p < 0.01) contents in soybean grains (Table 4). These correlations indicate higher root nodulation with increased acid phosphatase activity in the rhizosphere of soybean plants, and the influence of symbiotic N2 fixation and plant available phosphorus in soil on the nutrient contents of soybean grains. Overall, these results highlight strong relationships between farm management practices, rhizosphere microbial activities, and soybean grain bio-fortification.
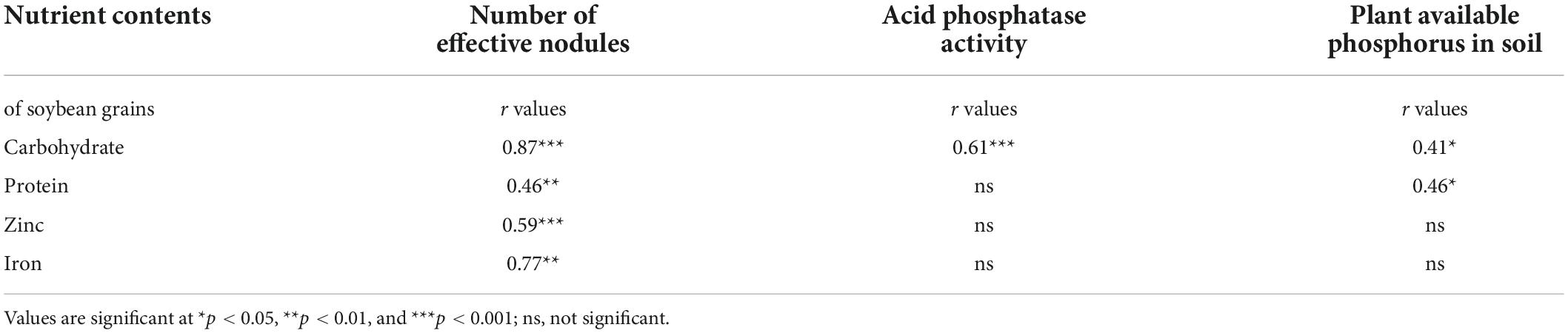
Table 4. Correlation of nutrient value of soybean grains with the number of effective root nodules, acid phosphatase activity in the rhizosphere, and plant available phosphorus in soil.
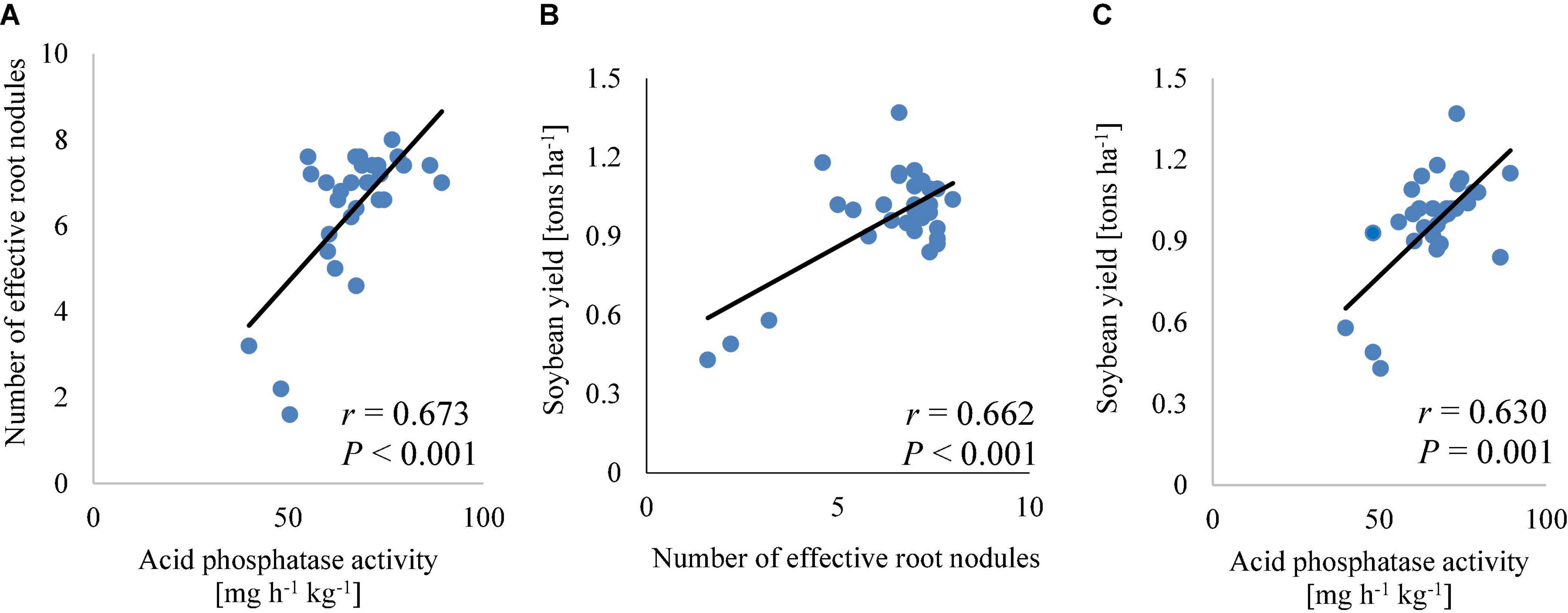
Figure 7. Correlation of the number of effective root nodules and acid phosphatase activity (A), soybean yield and number of effective root nodules (B), or acid phosphatase activity (C).
Discussion
Mycorrhization and root nodulation
Although soil N and P did not differ significantly across treatments, increased microbial activities (root nodulation, mycorrhization, and acid phosphatase) in the rhizosphere resulting from the influence of inoculated microorganisms probably increased plant nutrient uptake, which resulted in greater yield (Rawat et al., 2020). The fact that mycorrhiza colonization was not observed in plants that were not inoculated with AMF likely reflects low native AMF presence in soil, while a consistent level of AMF colonization for all inoculated treatments reflects positive plant–AMF symbiosis, especially under low native AMF status. However, the addition of PGPB and NPK fertilizer did not affect the rate of AMF colonization compared to the sole AMF treatment, indicating a strong boost of the inoculated AMF to compensate for low native AMF presence, irrespective of other inputs. Chen et al. (2018) and Powell and Rillig (2018) also described such a low AMF colonization rate as an indication of low soil health status. This could be attributed to intensive and unsustainable farm management practices such as chemical inputs and soil tillage under rotational cropping systems that may affect the soil biota (Wakam et al., 2015; Begoude et al., 2016). The observed high root colonization in AMF inoculated plants demonstrates the capacity of the inoculated mycorrhiza to compete with other rhizosphere microbiota and survive, which is an important characteristic of efficient bio-inoculants in enhancing soil fertility and productivity.
The high root nodulation achieved with inoculation of the PGPB consortium comprising Bradyrhizobium suggests successful symbiosis between the inoculated indigenous rhizobia and the soybean roots (Korir et al., 2017; Soumare et al., 2020). Accordingly, improved soybean root nodulation and N2 fixation were reported with the inoculation of Bradyrhizobium species and fertilizer addition (Ulzen et al., 2016; Leggett et al., 2017; Dabesa and Tana, 2021). Moreover, the high root nodulation in soybean rhizosphere inoculated with PGPB comprising symbiotic Bradyrhizobium and non-symbiotic bacteria species is consistent with Jabborova et al. (2021) who reported higher root nodulation and nitrogen-fixing capacity following co-inoculation of B. japonicum and Pseudomonas putida compared to uninoculated plants. Additionally, Akley et al. (2022) reported a positive residual effect of Bradyrhizobium inoculation on soybean root nodulation after three cropping seasons. Nonetheless, root nodulation by soybean plants was not favored by inoculation of mycorrhiza, which is not consistent with other reports on the contribution of mycorrhiza to the N2 fixing process (Daniel et al., 2020; Novais et al., 2020). The poor root nodulation in the control can be attributed to a low density of native N2 fixing symbiotic rhizobium and low soil phosphorus content (Rosenblueth et al., 2018; Soumare et al., 2020). Although high plant available N in the soil can reduce or inhibit the symbiotic effectiveness of introduced rhizobia strains (Kasper et al., 2019), the addition of N to PGPB-inoculated soybean plants did not reduce root nodulation. However, the present results do not support our hypothesis that co-inoculating plant growth-promoting bacteria and mycorrhiza will enhance soybean root nodulation. This may be due to nutrient deficiencies especially low soil phosphorus as Püschel et al. (2017) reported that the contribution of AMF to N2 fixation by rhizobium can be affected by environmental factors and nutrient availability including soil phosphorus.
Acid phosphatase activity
Soil phosphatase enzymes can be produced by plant roots or rhizosphere microorganisms and they play key roles in catalyzing reactions associated with organic matter decomposition and P cycling, while their quick response to changes in soil management is considered a useful biological indicator (Chodak and Nikliñska, 2012; Rejsek et al., 2012; Behera et al., 2017). The low acid phosphatase activity recorded in the rhizosphere of non-inoculated soybean plants is likely the contributions of soybean roots and the indigenous soil microbial community, while high phosphatase activity in the inoculated plants reflects the additional influence of the inoculated microbes that are involved in mineralizing organic to inorganic P (Rawat et al., 2020), or solubilizing inorganic phosphates (Tchakounté et al., 2020). The high acid phosphatase activity in sole PGPB treatment compared to the control and sole AMF treatments highlights the potential of inoculated plant growth-promoting bacteria. Some microbes in the inoculant biofertilizer used in this study were isolated from the rhizosphere of maize plants in Cameroon, with demonstrated ability to solubilize rock phosphate, fix N2, and produce siderophores (Tchakounté et al., 2018, 2020). Therefore, acid phosphatase activity in the rhizosphere can be explored as a possible mode of action of the inoculated microbes to induce nutrient dynamics that may enhance crop productivity. The superior acid phosphatase activity in the soybean rhizosphere inoculated with PGPB comprising symbiotic Bradyrhizobium and non-symbiotic bacteria species is in line with Jabborova et al. (2021) study which reported a higher acid phosphatase activity following co-inoculation of B. japonicum and P. putida. The increase in phosphatase activity following mycorrhiza inoculation could have been due to the production of glycoproteins (e.g., glomalin-related soil proteins) that increased microbial and enzymatic activities (Agnihotri et al., 2022). The observed decrease in acid phosphatase activity in the rhizosphere of PGPB-inoculated soybean plants and amended with NPK is in line with the demonstrated ability of NPK fertilizers to reduce soil microbial functions and acid phosphatase activity as reported by Mndzebele et al. (2020). These results are consistent with the second hypothesis that inoculating plant growth-promoting bacteria and arbuscular mycorrhiza fungi will enhance acid phosphatase activity in the rhizosphere of soybean plants, although their co-inoculation did not produce greater effects.
Soybean grain yield and nutrient contents
The soybean grain yield in the control plots for this study is within the range of 448–709 kg ha–1 that was previously reported by Wendt and Atemkeng (2004), across the first and second planting seasons in the humid forest ecosystem of Cameroon. However, treatment applications in this study increased the soybean grain yield relative to the control and the previously reported yield by Wendt and Atemkeng (2004). Inoculating PGPB and AMF with or without NPK fertilizer significantly increased soybean yield as compared to the control, which supports the third hypothesis that PGPB and AMF will increase soybean grain yield and nutrient contents (Thioub et al., 2017). Ksiȩżak and Bojarszczuk (2022) also reported higher soybean yield following inoculation with Bradyrhizobium and fertilizer addition. This increased soybean yield is consistent with the role of inoculated PGPB that comprise some Bacillus strains in nutrient solubilization and siderophore production as reported by Tchakounté et al. (2018, 2020). This is supported by Radhakrishnan et al. (2017) who highlighted the role of Bacillus strains in mediating crop growth via secretion of metabolites, drought tolerance, and protection against pests and diseases. The inoculated microbes in this study likely facilitated the conversion of complex forms of essential nutrients (e.g., P and N) to simple available forms for uptake by plant roots leading to enhanced growth and yield (Kang et al., 2015; Kuan et al., 2016). Furthermore, the secretion of phosphatase and organic acids by Bacillus spp. probably facilitated the conversion of inorganic phosphate into plant available phosphate for root uptake (Kang et al., 2014, 2015). Additionally, the inoculated microbes may have released ammonia from nitrogenous organic matter in the soil or their nifH genes produced nitrogenase for N2 fixation and uptake by plant roots to enhance growth and yield as reported for Bacillus spp. (Ding et al., 2005; Hayat et al., 2010; Kuan et al., 2016). The inoculation of mycorrhiza likely enhanced the production of glycoproteins that improved soil quality via increased aggregation and carbon sequestration, water-holding capacity, nutrient storage and availability, microbial and enzymatic activities, and production of extracellular polysaccharides, which increased crop performance (Agnihotri et al., 2022). The role of microbial inoculation in the present study is supported by correlations of soybean grain yield with microbial activities in the rhizosphere such as acid phosphatase and root nodulation, which is consistent with Lamptey et al. (2014). The superior performance of soybean plants that were inoculated with the consortium of PGPB comprising Bradyrhizobium is supported by Leggett et al. (2017), who reported improved soybean growth, nodulation and N2 fixation, and grain yield following inoculation with Bradyrhizobium. Meanwhile, the lack of grain yield increase when NPK fertilizers were added to PGPB or AMF indicates that the additional NPK inputs were not enough to cause a significant difference in this study. This finding opens up avenues for further investigation on the appropriate NPK fertilizer amounts to integrate with microbial inoculants.
The higher nutrient content in soybean grains inoculated with PGPB comprising symbiotic Bradyrhizobium and non-symbiotic bacteria species is in line with Jabborova et al. (2021) study which reported superior plant nutrients following inoculation of B. japonicum and P. putida compared to uninoculated plants. Akley et al. (2022) also reported a positive residual effect of Bradyrhizobium inoculation on soybean yield after three cropping seasons. The high nutrient contents in soybean grains from plants inoculated with PGPB and AMF, with NPK fertilizer addition in this study indicates soil nutrient enhancement and their effective uptake by plants that eventually accumulated in the grains. Similar results were reported by Sogut (2016), Marro et al. (2020), and Zhang et al. (2020). Beneficial microorganisms may directly enhance nutrient uptake and accumulation or indirectly stimulate other biochemical processes that are involved in the mobilization and deposition of nutrients in the grains (Lamptey et al., 2014; Marro et al., 2020). Overall, the improved nutrient contents in soybean grains following co-inoculation of plant growth-promoting bacteria and AMF with or without NPK fertilizer addition likely results from a combination of increased biochemical processes as reported by Sheteiwy et al. (2021), and supports the concept of integrated soil fertility management (Vanlauwe et al., 2010). These results confirm the positive impact of microbial inoculation and fertilizers on grain yield and protein content of maize (Martins et al., 2017) and soybeans (Marro et al., 2020; Alemayehu and Tana, 2021; Ksiȩżak and Bojarszczuk, 2022). Yasmin et al. (2020) also reported higher nutrient contents (e.g., N, P, K, Ca, Mg) in sweet potato roots following inoculation with Klebsiella sp. and N fertilizer addition. Nitrogen is an important component that is responsible for several physiological and biochemical processes in plants, being a structural constituent of chlorophyll molecules, proteins, enzymes, and nucleic acids (Nunes-Nesi et al., 2010). Hence, plants with proper nutrition via the application of chemical and biofertilizers likely had higher chlorophyll content with increased photosynthesis and production of photoassimilates, grain filling, and chemical composition, which increased soybean yield and nutritive contents. Ramesh et al. (2014) and Khande et al. (2017) reported that Bacillus species substantially influenced the mobilization of zinc and its concentration in soybean, which can be utilized as bio-inoculants for bio-fertilization and bio-fortification. Iron chelation by Bacillus spp. via siderophore production facilitates iron solubilization from minerals and organic compounds in the rhizosphere by binding Fe3+ in complex substances and reducing them to Fe2+ for uptake by plants (Walker and Connolly, 2008; Nadeem et al., 2012). These reports support the potential of our locally produced inoculant biofertilizer comprising three Bacillus strains with the potential to produce siderophore that likely increased mobilization of zinc for uptake by soybean plants.
Conclusion
The absence of mycorrhizal colonization in non–AMF-inoculated plants compared to the inoculated ones demonstrates very low content of native AMF strains in the Yaoundé field site in Cameroon, but there was no significant difference between the AMF-inoculated treatments. The successful mycorrhizal colonization of soybean roots for plants inoculated with AMF coupled with the significantly higher soybean yield highlights the potential of AMF inoculation to boost soybean productivity as compared to the control. Sole inoculation of PGPB or AMF-enhanced root nodulation and acid phosphatase activities in the rhizosphere of soybean plants, but their co-inoculation was not significantly different from the sole inoculations. The inoculation of PGPB and AMF increased soybean grain yield and bio-fortification, while NPK fertilizer addition enhanced soybean grain bio-fortification. Overall, these results open up important pathways for further investigation on local strategies for sustainable integrated soil fertility management to boost the productivity of soybean.
Data availability statement
The original contributions presented in this study are included in the article/Supplementary material, further inquiries can be directed to the corresponding author.
Author contributions
CN conceived the study, performed data analysis and literature searches, and prepared the first manuscript draft. BT participated in conceiving the study, established the field experiment, performed literature searches, and reviewed the manuscript. MO produced the inoculant formulations of plant growth-promoting bacteria, contributed in establishing the field experiment and literature searches, and reviewed the manuscript. CS participated in conceiving the study and coordinated the establishment and management of the field experiment. RN participated in the field establishment and literature searches and read the manuscript draft. MN participated in literature searches and read the manuscript draft. DA participated in data processing and analysis and literature searches and read the manuscript draft. GT isolated and plant growth-promoting bacteria and coordinated the production of microbial inoculum, and read the manuscript draft. SR coordinated the study and read the manuscript draft. All authors read the draft manuscript and approved the final manuscript.
Funding
This study was funded by the German Ministry of Education and Research (BMBF) through the MicBioTec (01DG20008) research grant.
Acknowledgments
We express gratitude to the Faculty of Agriculture and Veterinary Medicine of the University of Buea, and the Ministry of Higher Education of Cameroon for supporting the corresponding author. We also appreciate the support of Ndakwe Abigail Tifuh of the Rhizobiology Laboratory of the University of Buea for producing the PGPB consortium.
Conflict of interest
The authors declare that the research was conducted in the absence of any commercial or financial relationships that could be construed as a potential conflict of interest.
Publisher’s note
All claims expressed in this article are solely those of the authors and do not necessarily represent those of their affiliated organizations, or those of the publisher, the editors and the reviewers. Any product that may be evaluated in this article, or claim that may be made by its manufacturer, is not guaranteed or endorsed by the publisher.
Supplementary material
The Supplementary Material for this article can be found online at: https://www.frontiersin.org/articles/10.3389/fpls.2022.934339/full#supplementary-material
References
Agnihotri, R., Sharmaa, M. P., Prakash, A., Ramesh, A., Bhattacharjya, S., Patra, A. K., et al. (2022). Glycoproteins of arbuscular mycorrhiza for soil carbon sequestration: review of mechanisms and controls. Sci. Tot. Environ. 806:150571. doi: 10.1016/j.scitotenv.2021.150571
Akley, E. K., Rice, C. W., Adotey, N., Ampim, P. A. Y., Prasad, P. V. V., Danquah, E. O., et al. (2022). Residual Bradyrhizobium inoculation effects on soybean performance and selected soil health parameters. Agron. J. 114, 1627–1641. doi: 10.1002/agj2.21037
Alam, F., Bhuiyan, M. A. H., Alam, S. S. A., Waghmode, T. R., Kim, P. J., and Lee, Y. B. (2015). Effect of Rhizobium sp. BARIRGm901 inoculation on nodulation, nitrogen fixation and yield of soybean (Glycine max) genotypes in gray terrace soil. Biosci. Biotechnol. Biochem. 79, 1660–1668. doi: 10.1080/09168451.2015.1044931
Alemayehu, D., and Tana, T. (2021). Response of Soybean (Glycine max L. (Merrill)) to Bradyrhizobium inoculation, lime, and phosphorus applications at Bako, Western Ethiopia. Int. J. Agron. 2021:6686957. doi: 10.1155/2021/6686957
Ambassa-Kiki, R., and Nill, D. (1999). Effects of different land management techniques on selected topsoil properties of a forest ferralsol. Soil Tillage Res. 59, 259–264. doi: 10.1016/S0167-1987(99)00067-7
AOAC (2005). Official Methods of Analysis of the Association of Analytical Chemists, 18th Edn. Gathersburg, MD: AOAC International.
Atieno, M., Herrmann, L., Okalebo, R., and Lesueur, D. (2012). Efficiency of different formulations of Bradyrhizobium japonicum and effect of co-inoculation of Bacillus subtilis with two different strains of Bradyrhizobium japonicum. World J. Microbiol. Biotechnol. 28, 2541–2550. doi: 10.1007/s11274-012-1062-x
Backer, R., Rokem, J. S., Ilangumaran, G., Lamont, J., Praslickova, D., Ricci, E., et al. (2018). Plant growth-promoting rhizobacteria: context, mechanisms of action, and road map to commercialization of biostimulants for sustainable agriculture. Front. Plant Sci. 9:1465–1473. doi: 10.3389/fpls.2018.01473
Bechtaoui, N., Raklami, A., Benidire, L., Tahiri, A., Göttfert, M., and Oufdou, K. (2020). Effects of PGPR co-inoculation on growth, phosphorus nutrition and phosphatase/phytase activities of faba bean under different phosphorus availability condition. Polish J. Environ. Stud. 29, 1557–1565. doi: 10.15244/pjoes/110345
Becker, M., Patz, S., Becker, Y., Berger, B., Drungowski, M., Bunk, B., et al. (2018). Comparative genomics reveal a flagellar system, a type VI secretion system and plant growth-promoting gene clusters unique to the endophytic bacterium Kosakonia radicincitans. Front. Microbiol. 9:1997. doi: 10.3389/fmicb.2018.01997
Begoude, D. A. B., Sarr, P. S., Mpon, T. L. Y., Owona, D. A., Kapeua, M. N., and Araki, S. (2016). Composition of Arbuscular mycorrhizal fungi associated with cassava (Manihot esculenta Crantz) cultivars as influenced by chemical fertilization and tillage in Cameroon. J. Appl. Biosci. 98, 9270–9283. doi: 10.4314/jab.v98i1.4
Behera, B. C., Yadav, H., Singh, S. K., Mishra, R. R., Sethi, B. K., Dutta, S. K., et al. (2017). Phosphate solubilization and acid phosphatase activity of Serratia sp. isolated from mangrove soil of Mahanadi River Delta, Odisha, India. J. Genet. Eng. Biotechnol. 15, 169–178. doi: 10.1016/j.jgeb.2017.01.003
Bello, S. K., Yusuf, A. A., and Cargele, M. (2018). Performance of cowpea as influenced by native strain of rhizobia, lime and phosphorus in Samaru, Nigeria. Symbiosis 75, 167–176. doi: 10.1007/s13199-017-0507-2
Bender, S. F., Wagg, C., and van der Heijden, M. G. (2016). An underground revolution: biodiversity and soil ecological engineering for agricultural sustainability. Trends Ecol. Evol. 31, 440–452. doi: 10.1016/j.tree.2016.02.016
Bremner, J. M., and Mulvaney, C. S. (1982). “Nitrogen-Total,” in Methods of Soil Analysis. Part 2. Chemical and Microbiological Properties, eds A. L. Page, R. H. Miller, and D. R. Keeney (Madison, WI: American Society of Agronomy), 595–624. doi: 10.2134/agronmonogr9.2.2ed.c31
Chen, M., Arato, M., Borghi, L., Nouri, E., and Reinhardt, D. (2018). Beneficial services of arbuscular mycorrhizal fungi–from ecology to application. Front. Plant Sci. 9:1270. doi: 10.3389/fpls.2018.01270
Chodak, M., and Nikliñska, M. (2012). Development of microbial biomass and enzyme activities in mine soils. Polish J. Environ. Stud. 21:569.
Dabesa, A., and Tana, T. (2021). Response of Soybean (Glycine max L. (Merrill)) to Bradyrhizobium inoculation, lime, and phosphorus applications at Bako, Western Ethiopia. Int. J. Agron. 2021:6686957.
Daniel, M. M., Ezekiel, M. N., Methuselah, M. N., and John, M. M. (2020). Arbuscular mycorrhizal fungi and Bradyrhizobium co-inoculation enhances nitrogen fixation and growth of green grams (Vigna radiata L.) under water stress. J. Plant Nutr. 43, 1036–1047. doi: 10.1080/01904167.2020.1711940
David, T. P. (1978). An Introduction to Practical Biochemistry, 2nd Edn. London: Mc Graw-Hill, 183–184.
Ding, Y., Wang, J., Liu, Y., and Chen, S. (2005). Isolation and identification of nitrogen-fixing bacilli from plant rhizospheres in Beijing region. J. Appl. Microbiol. 99, 1271–1281. doi: 10.1111/j.1365-2672.2005.02738.x
Hayat, R., Ali, S., Amara, U., Khalid, R., and Ahmed, I. (2010). Soil beneficial bacteria and their role in plant growth promotion: a review. Ann. Microbiol. 60, 579–598. doi: 10.1007/s13213-010-0117-1
Herliana, O., Harjoso, T., Anwar, A. H. S., and Fauzi, A. (2019). The effect of Rhizobium and N fertilizer on growth and yield of black soybean (Glycine max (L) Merril). IOP Conf. Ser. Earth Environ. Sci. 255:2015. doi: 10.1088/1755-1315/255/1/012015
Islam, M. S., Ahmed, M., Hossain, M. S., Akter, H., and Aktar, S. (2017). Response of soybean to Rhizobium biofertilizer under different levels of phosphorus. Prog. Agric. 28, 302–315. doi: 10.3329/pa.v28i4.36370
Jabborova, D., Kannepalli, A., Davranov, K., Narimanov, A., Enakiev, Y., Syed, A., et al. (2021). Co-inoculation of rhizobacteria promotes growth, yield, and nutrient contents in soybean and improves soil enzymes and nutrients under drought conditions. Sci. Rep. 11:22081. doi: 10.1038/s41598-021-01337-9
Kalayu, G. (2019). Phosphate solubilizing microorganisms: promising approach as biofertilizers. Int. J. Agron. 2019:4917256. doi: 10.1155/2019/4917256
Kalra, Y. P., and Maynard, D. G. (1991). Methods Manual for Forest Soil and Plant Analysis. Information Report NOR-X-319. Edmonton, AB: Forestry Canada, 116.
Kang, S.-M., Radhakrishnan, R., Lee, K.-E., You, Y.-H., Ko, J.-H., Kim, J.-H., et al. (2015). Mechanism of plant growth promotion elicited by Bacillus sp. LKE15 in oriental melon. Acta Agric. Scand. B Soil Plant Sci. 65, 637–647. doi: 10.1080/09064710.2015.1040830
Kang, S.-M., Radhakrishnan, R., You, Y.-H., Joo, G.-J., Lee, I.-J., Lee, K.-E., et al. (2014). Phosphate solubilizing Bacillus megaterium mj1212 regulates endogenous plant carbohydrates and amino acids contents to promote mustard plant growth. Indian J. Microbiol. 54, 427–433. doi: 10.1007/s12088-014-0476-6
Kanomanyanga, J., Jiao, X., Mudare, S., Mabasa, S., and Tang, L. (2021). Meta-analysis of the effects of Rhizobia inoculants and phosphorus fertilizer on soybean nodulation in Africa. Afr. J. Plant Sci. 15, 206–224. doi: 10.5897/AJPS2021.2168
Kasper, S., Christoffersen, B., Soti, P., and Racelis, A. (2019). Abiotic and biotic limitations to nodulation by leguminous cover crops in South Texas. Agriculture 9:209. doi: 10.3390/agriculture9100209
Khande, R. K., Sharma, S. K., Ramesh, A., and Sharma, M. P. (2017). Zinc solubilizing Bacillus strains that modulate growth, yield and zinc biofortification of soybean and wheat. Rhizosphere 4, 126–138. doi: 10.1016/j.rhisph.2017.09.002
Korir, H., Mungai, N. W., Thuita, M., Hamba, Y., and Masso, C. (2017). Co-inoculation effect of rhizobia and plant growth promoting rhizobacteria on common bean growth in a low phosphorus soil. Front. Plant Sci. 8:141. doi: 10.3389/fpls.2017.00141
Ksiȩżak, J., and Bojarszczuk, J. (2022). The effect of mineral N fertilization and Bradyrhizobium japonicum seed inoculation on productivity of soybean (Glycine max (L.) Merrill). Agriculture 12:110. doi: 10.3390/agriculture12010110
Kuan, K. B., Othman, R., Rahim, K. A., and Shamsuddin, Z. H. (2016). Plant growth-promoting rhizobacteria inoculation to enhance vegetative growth, nitrogen fixation and nitrogen remobilisation of maize under greenhouse conditions. PLoS One 11:e0152478. doi: 10.1371/journal.pone.0152478
Lamptey, S., Ahiabor, B. D. K., Yeboah, S., and Asamoah, C. (2014). Response of soybean (Glycine max) to Rhizobial inoculation and phosphorus application. J. Exp. Biol. Agric. Sci. 2, 73–77.
Leggett, M., Diaz-Zorita, M., Koivunen, M., Bowman, R., Pesek, R., Stevenson, C., et al. (2017). Soybean response to inoculation with Bradyrhizobium japonicum in the United States and Argentina. Agron. J. 109, 1031–1038. doi: 10.2134/agronj2016.04.0214
Mahmud, K., Makaju, S., Ibrahim, R., and Missaoui, A. (2020). Current progress in nitrogen fixing plants and microbiome research. Plants 9:97. doi: 10.3390/plants9010097
Marro, N. A., Cofréa, M. N., Grilli, G., Alvarez, C., Labuckas, D. O., Maestri, D., et al. (2020). Soybean yield, protein content and oil quality in response to interaction of Arbuscular mycorrhizal fungi and native microbial populations from mono and rotation-cropped soils. Appl. Soil Ecol. 152:8. doi: 10.1016/j.apsoil.2020.103575
Martins, M. R., Jantalia, C. P., Reis, V. M., Döwich, I., and Polidoro, L. C. (2017). Impact of plant growth-promoting bacteria on grain yield, protein content, and urea-15 N recovery by maize in a Cerrado Oxisol. Plant Soil 422, 239–250. doi: 10.1007/s11104-017-3193-1
Mndzebele, B., Ncube, B., Fessehazion, M., Mabhaudhi, T., Amoo, S., Plooy, C., et al. (2020). Effects of cowpea-amaranth intercropping and fertiliser application on soil phosphatase activities, available soil phosphorus, and crop growth response. Agronomy 10:79. doi: 10.3390/agronomy10010079
Nadeem, S. M., Shaharoona, B., Arshad, M., and Crowley, D. E. (2012). Population density and functional diversity of plant growth promoting rhizobacteria associated with avocado trees in saline soils. Appl. Soil Ecol. 62, 147–154. doi: 10.1016/j.apsoil.2012.08.005
Nanganoa, L. T., Ngome, F. A., Suh, C., and Basga, S. D. (2020). Assessing soil nutrients variability and adequacy for the cultivation of maize, cassava, and sorghum in selected agroecological zones of Cameroon. Int. J. Agron. 2020:8887318. doi: 10.1155/2020/8887318
Ngeno, J., Chemining’wa, G. N., Muthomi, J. W., and Shibairo, S. I. (2012). Effect of Rhizobium inoculation and nitrogen fertilizer application on growth, nodulation and yield of two garden pea genotypes. J. Anim. Plant Sci. 15, 2147–2156.
Ngosong, C., Bongkisheri, V., Tanyi, C. B., Nanganoa, L. T., and Tening, A. S. (2019). Optimizing nitrogen fertilization regimes for sustainable maize (Zea mays L.) production on the volcanic soils of Buea Cameroon. Adv. Agric. 2019:4681825. doi: 10.1155/2019/4681825
Novais, C. B., Sbrana, C., Jesus, C. E., Rouws, L. F. M., Giovannetti, M., Avio, L., et al. (2020). Mycorrhizal networks facilitate the colonization of legume roots by a symbiotic nitrogen-fixing bacterium. Mycorrhiza 30, 389–396. doi: 10.1007/s00572-020-00948-w
Ntambo, M. S., Isaac, S. C., Taruvinga, A., Hafeez, S., Anwar, T., Sharif, R., et al. (2017). The effect of Rhizobium inoculation with nitrogen fertilizer on growth and yield of soybeans (Glycine max L.). Int. J. Biosci. 10, 163–172. doi: 10.12692/ijb/10.3.163-172
Nunes-Nesi, A., Fernie, A. R., and Stitt, M. (2010). Metabolic and signaling aspects underpinning the regulation of plant carbon nitrogen interactions. Mol. Plant 3, 973–996. doi: 10.1093/mp/ssq049
Nyahnone, T. J. (2017). Le Développement De La Culture Du Soja Dans Les Fronts D’extensions Cotonniers du Nord-Cameroun: Le cas du Mayo-Rey. Mémoire De Master De Géographie, Université de Ngaoundéré, Yaounde, 135.
Nzossié, E. J. F., and Bring, C. (2020). Soybean (Glycine max (L.) Merr.) Production in the Cameroonian Cotton Basin between the Dynamics of Structuring an Agricultural Value Chain and Sustainability Issues. London: Intechopen, 93981.
Powell, J. R., and Rillig, M. C. (2018). Biodiversity of arbuscular mycorrhizal fungi and ecosystem function. New Phytol. 220, 1059–1075. doi: 10.1111/nph.15119
Püschel, D., Janoušková, M., Voøíšková, A., Gryndlerová, H., Vosátka, M., and Jansa, J. (2017). Arbuscular mycorrhiza stimulates biological nitrogen fixation in two Medicago spp. through improved phosphorus acquisition. Front. Plant Sci. 8:390. doi: 10.3389/fpls.2017.00390
Radhakrishnan, R., Hashem, A., and Abd Allah, E. F. (2017). Bacillus: a biological tool for crop improvement through bio-molecular changes in adverse environments. Front. Physiol. 8:667. doi: 10.3389/fphys.2017.00667
Ramesh, A., Sharma, S. K., Sharma, M. P., Yadav, N., and Joshi, O. P. (2014). Zinc solubilizing Bacillus cereus and related species modulates growth, yield and zinc biofortification of soybean and wheat seeds cultivated in central India. Appl. Soil Ecol. 73, 87–96. doi: 10.1016/j.apsoil.2013.08.009
Rawat, P., Das, S., Shankhdhar, D., and Shankhdhar, S. C. (2020). Phosphate-solubilizing microorganisms: mechanism and their role in phosphate solubilization and uptake. J. Soil Sci. Plant Nutr. 21, 49–68. doi: 10.1007/s42729-020-00342-7
Rejsek, K., Vronova, V., Pavelka, M., and Formanek, P. (2012). Acid phosphomonoesterase (E.C. 3.1.3.2.) location in soil. J. Plant Nutr. Soil Sci. 175:196. doi: 10.1002/jpln.201000139
Rosenblueth, M., Orrillo, O. E., López, L. A., Rogel, M. A., Hernández, R. B. J., Romero, M. J. C., et al. (2018). Nitrogen fixation in cereals. Front. Microbiol. 9:1794. doi: 10.3389/fmicb.2018.01794
Rowell, D. L. (1994). Soil Science: Methods and Applications. London: Addison Wesley Longman Singapore Publishers (Pte) Ltd.
Ruppel, S., and Merbach, W. (1995). Effects of different nitrogen sources on nitrogen fixation and bacterial growth of Pantoea agglomerans and Azospirillum sp. in bacterial pure culture: an investigation using 15N2 incorporation and acetylene reduction measures. Microbiol. Res. 150, 409–418. doi: 10.1016/S0944-5013(11)80023-6
Sheteiwy, M. S., Elgawad, H. A., Xiong, Y.-C., Macovei, A., Brestic, M., Skalicky, M., et al. (2021). Inoculation with Bacillus amyloliquefaciens and mycorrhiza confers tolerance to drought stress and improve seed yield and quality of soybean plant. Physiol. Plant. 172, 2153–2169. doi: 10.1111/ppl.13454
Sogut, T. (2016). Rhizobium inoculation improves yield and nitrogen accumulation in soybean (Glycine max) cultivars better than fertilizer. N. Z. J. Crop Hortic. Sci. 342, 115–120. doi: 10.1080/01140671.2006.9514395
Soumare, A., Diedhiou, A. G., Thuita, M., Hafidi, M., Ouhdouch, Y., Gopalakrishnan, S., et al. (2020). Exploiting biological nitrogen fixation: a route towards a sustainable agriculture. Plants 9:1011. doi: 10.3390/plants9081011
Tamiru, S., Mohan, L. P., and Angaw, T. (2012). Effects of inoculation by Bradyrhizobium japonicum strains on nodulation, nitrogen fixation, and yield of soybean (Glycine max L. Merill) varieties on nitisols of Bako, Western Ethiopia. Int. Sch. Res. Notices 2012:261475. doi: 10.5402/2012/261475
Tchakounté, T. G.-V., Berger, B., Patz, S., Henri, F., and Ruppel, S. (2018). Community structure and plant growth-promoting potential of cultivable bacteria isolated from Cameroon soil. Microbiol. Res. 214, 47–59. doi: 10.1016/j.micres.2018.05.008
Tchakounté, V. T. G., Berger, G., Patz, S., Becker, M., Ková, V. T., Novák, O., et al. (2020). The response of maize to inoculation with Arthrobacter sp. and Bacillus sp. in phosphorus-deficient, salinity-affected soil. Microorganisms 8:1005. doi: 10.3390/microorganisms8071005
Tening, A. S., Foba-Tendo, J. N., Yakum-Ntaw, S. Y., and Tchuenteu, F. (2013). Phosphorus fixing capacity of a volcanic soil on the slope of mount Cameroon. Agric. Biol. J. North Am. 4, 166–174. doi: 10.5251/abjna.2013.4.3.166.174
Thioub, M., Ewusi-Mensah, N., Sarkodie-Addo, J., and Adjei-Gyapong, J. (2017). Arbuscular mycorrhizal fungi inoculation enhances phosphorus use efficiency and soybean productivity on a Haplic Acrisol. Soil Tillage Res. 192, 174–186. doi: 10.1016/j.still.2019.05.001
Uko, A. E., Oko, B. F. B., and Ndon, B. A. (2002). Yield response of soybeans to levels of nitrogen and potassium fertilizer in the humid tropics. Glob. J. Agric. Sci. 1, 101–109. doi: 10.4314/gjass.v1i2.2189
Ulzen, J., Abaidoo, R. C., Mensah, N. E., Masso, C., and AbdelGadir, A. H. (2016). Bradyrhizobium inoculants enhance grain yields of soybean and cowpea in northern Ghana. Front. Plant Sci. 7:1770. doi: 10.3389/fpls.2016.01770
Van Reeuwijk, L. P. (1992). Procedures for Soil Analysis, 3rd Edn. Wageningen: International Soil Reference and Information Centre (ISRIC).
Vanlauwe, B., Bationo, A., Chianu, J., Giller, K. E., Merckx, R., Mokwunye, U., et al. (2010). Integrated soil fertility management: operational definition and consequences for implementation and dissemination. Outlook Agric. 39, 17–24. doi: 10.5367/000000010791169998
Venneman, J., Audenaert, K., Verwaeren, J., Baert, G., Boeckx, P., Moango, A. M., et al. (2017). Congolese rhizospheric soils as a rich source of new plant growth-promoting endophytic Piriformospora Isolates. Front. Microbiol. 8:212. doi: 10.3389/fmicb.2017.00212
Wakam, L. N., Nanhou, A. P., Fokom, R., and Tchameni, S. N. (2015). Effects of nitrogen levels and arbuscular mycorrhizal fungi on biomass production, mineral nutrition, sugar and total phenolic content of two Zea mays Cultivars. Am. J. Exp. Agric. 9, 1–11. doi: 10.9734/AJEA/2015/18088
Walker, E. L., and Connolly, E. L. (2008). Time to pump iron: iron-deficiency-signaling mechanisms of higher plants. Curr. Opin. Plant Biol. 11, 530–535. doi: 10.1016/j.pbi.2008.06.013
Wendt, J., and Atemkeng, M. (2004). Soybean, cowpea, groundnut, and pigeonpea response to soils, rainfall, and cropping season in the forest margins of Cameroon. Plant Soil 263, 121–132. doi: 10.1023/B:PLSO.0000047731.35668.e0
Yasmin, F., Othman, R., and Maziz, M. N. H. (2020). Yield and nutrient content of sweet potato in response of plant growth-promoting rhizobacteria (PGPR) inoculation and N fertilization. Jordan J. Biol. Sci. 13, 117–122.
Keywords: fertilizer, N2-fixation, P-solubilization, phosphatase, rhizosphere
Citation: Ngosong C, Tatah BN, Olougou MNE, Suh C, Nkongho RN, Ngone MA, Achiri DT, Tchakounté GVT and Ruppel S (2022) Inoculating plant growth-promoting bacteria and arbuscular mycorrhiza fungi modulates rhizosphere acid phosphatase and nodulation activities and enhance the productivity of soybean (Glycine max). Front. Plant Sci. 13:934339. doi: 10.3389/fpls.2022.934339
Received: 02 May 2022; Accepted: 29 August 2022;
Published: 26 September 2022.
Edited by:
Eduardo V. Soares, Instituto Superior de Engenharia do Porto (ISEP), PortugalReviewed by:
Mohamed Ait El Mokhtar, Université Hassan II Mohammedia, MoroccoMilan Skalicky, Czech University of Life Sciences Prague, Czechia
Roxana Vidican, University of Agricultural Sciences and Veterinary Medicine of Cluj-Napoca, Romania
Christel Baum, University of Rostock, Germany
Sushil K. Sharma, National Institute of Biotic Stress Management, India
Aketi Ramesh, Indian Council of Agricultural Research (ICAR), India
Copyright © 2022 Ngosong, Tatah, Olougou, Suh, Nkongho, Ngone, Achiri, Tchakounté and Ruppel. This is an open-access article distributed under the terms of the Creative Commons Attribution License (CC BY). The use, distribution or reproduction in other forums is permitted, provided the original author(s) and the copyright owner(s) are credited and that the original publication in this journal is cited, in accordance with accepted academic practice. No use, distribution or reproduction is permitted which does not comply with these terms.
*Correspondence: Christopher Ngosong, bmdvc29uZy5jaHJpc3RvcGhlckB1YnVlYS5jbQ==