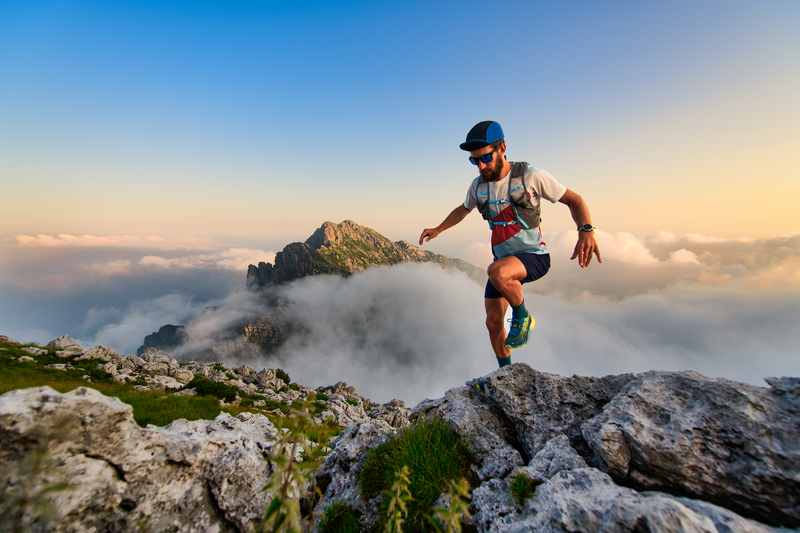
95% of researchers rate our articles as excellent or good
Learn more about the work of our research integrity team to safeguard the quality of each article we publish.
Find out more
REVIEW article
Front. Plant Sci. , 23 August 2022
Sec. Plant Cell Biology
Volume 13 - 2022 | https://doi.org/10.3389/fpls.2022.931979
This article is part of the Research Topic pH as a Signal and Secondary Messenger in Plant Cells View all 6 articles
Plant nutrition, growth, and response to environmental stresses are pH-dependent processes that are regulated at the apoplastic and subcellular levels. The root apoplastic pH is especially sensitive to external cues and can also be modified by intracellular inputs, such as hormonal signaling. Optimal crosstalk of the mechanisms involved in the extent and span of the apoplast pH fluctuations promotes plant resilience to detrimental biotic and abiotic factors. The fact that variations in local pHs are a standard mechanism in different signaling pathways indicates that the pH itself can be the pivotal element to provide a physiological context to plant cell regions, allowing a proportional reaction to different situations. This review brings a collective vision of the causes that initiate root apoplastic pHs variations, their interaction, and how they influence root response outcomes.
The concentration of H+ (protons) present in all aqueous compartments defines their pH and determines the physicochemical properties of the molecules embedded in the solution. The pH affects both the enzymatic and transporters activity and the protein-protein interactions since the structure and solubility of the proteins are dependent on their net charge and ionization of specific residues (Pace et al., 2009). For this reason, proton levels have a leading role in the development and growth of living organisms. Focusing on plants, pH impacts all essential aspects of their biology such as nutrient absorption by the root, control of the cell wall (CW) expansion, or stomatal movements (Barbez et al., 2017; Geilfus, 2017; Li et al., 2021; Zhang et al., 2021). The pH range found in plant cells is between 4.0 and 8.4, depending on the different subcellular compartments and the rhizosphere (Tsai and Schmidt, 2021). Extreme swings in pH have severe negative repercussions on plant fitness (Ratcliffe, 2003). Consequently, plants possess strong regulatory mechanisms to buffer proton concentration oscillations and to keep the pH within a range compatible with proper growth and development.
Regulation of pH is managed by all subcellular compartments, but it is within the apoplast where the pH (hereafter named pHapo) plays a more crucial role in regulating essential processes like intercellular signaling, plant–microbe interactions, plant response to abiotic stresses, and water and nutrient transport (Figure 1) (Monshausen et al., 2009, 2011; Barbez et al., 2017). Plants have different mechanisms to sustain apoplastic H+ ions levels in ranges in consonance with the plant physiology. This includes plasma membrane proton pumps, organic anion release, root respiration, redox-coupled process (Hinsinger et al., 2003), and the buffer capacity of the plant CW (Martinière et al., 2018). Although they do not act directly in buffering pH, phytohormones have a strong impact on the regulation of pHapo. While the role of auxin or abscisic acid (ABA) in pHapo has broadly been studied, other hormones are getting more attention as modulators of proton pump activity, such as cytokinin (Falhof et al., 2016).
Figure 1. pHapo as the hub for root response. pHapo forms a gradient along the rhizosphere and the different root cell layers, that favors nutrient and water uptake. In normal root growth conditions (left panel), low concentrations of auxin promote plasma proton pumps (AHA) activation lowering the pHapo which induces CW loosening and vacuole regulation for water uptake. This, together with nutrient uptake, boosts cell growth (acid growth theory). Acid pH induces the activation of CW modifying proteins (CWMPs) such as expansins, to promote the CW loosening easing root growth. Under stress situations, hormones such as ABA or ethylene inhibit the AHAs activity fostering the alkalinization of the epidermal pHapo and the rhizosphere. Microbe perception through microbe-associated molecular patterns (MAMPs) and damage-associated molecular pattern (DAMPs) also reduce the activity of AHAs leading to the pHapo alkalinization. In these alkaline conditions, peroxidases generate H2O2 (Reactive Oxygen Species, ROS), and the CWMPs are inactivated promoting the stiffness of the CW.
Environmental challenges such as high salinity, drought, anoxia, or microbes induce drastic pHapo changes provoking a systemic plant response (Felle et al., 2005; Minibayeva et al., 2015; Geilfus, 2017; Geilfus et al., 2017; Kesten et al., 2019). A specific example of this influence happens during high rainfalls. The dissolved atmospheric carbon dioxide (CO2) forms carbonic acid (H2CO3), which together with the atmospheric pollutants acidify the soil (Blake, 2005). This low external pH increases the availability of Mn2+ and/or Al3+ inhibiting plant growth (Msimbira and Smith, 2020). Interestingly, in some cases, the same stress induces different variations in the pHapo. The plant pathogen Fusarium oxysporum induces a fast root pHapo acidification during its first contact with the root, while the pHapo is alkalinized in later stages of the infection (Kesten et al., 2019). In this review, we revisit the role of the root pHapo in cellular signaling and how it is regulated. Root pHapo values are especially dynamic, changing more than two pH units upon certain stresses (Geilfus, 2017), likely because molecules diffusion between the root apoplast and the exterior is happening with high permeability. Additionally, the pHapo change depends on the intensity of the stress. For example, pHapo increases proportionally to the concentration of NaCl (Geilfus and Mühling, 2012). Importantly, as with other signaling mechanisms, the pHapo values tend to return to their steady state after their modification.
The acid growth theory is broadly assumed in the plant scientific community, which affirms that while acid pHapo enables cell growth, alkaline pHapo induces CW stiffness and protection against osmotic stress and pathogens ultimately arresting cell growth (Takahashi et al., 2012; Barbez et al., 2017; Du et al., 2020). The fact that so many different processes are linked to the pHapo brings to light the pivotal role of the H+ levels in the development of the plant. We consider that the root pHapo acts as a regulatory element, which uses the local levels of protons as a link between the external environment and plant physiology. The oscillation of the pHapo during different stresses would be a way through which plant development systems coordinate to react properly. Furthermore, pH levels coordinate with cytosolic secondary messengers such as Ca2+, allowing the translation and scalability of the external signalization to the cytosol (Behera et al., 2018). We present here, an overview of the processes that can modulate the root pHapo, how the rhizosphere and the phytohormones participate in this process, and how the connection among these elements determines root response (growth and nutrient absorption).
There are different methods to estimate the pH of the plant cell and its subcellular compartments, including the apoplast. The most used and accurate methods are based on the use of dyes (such as Bromocresol) in vivo pH-sensor based of fluorescent probes, H+-selective microelectrodes, or 31P nuclear magnetic resonance spectroscopy [reviewed in Geilfus (2017) and Tsai and Schmidt (2021)]. Thanks to these approaches, we know that the pH is not constant across the length and tissues of the root and additionally varies within the different subcellular compartments of the cell. Concretely, the root pHapo oscillates in an acidic range between 4.5 and 5.5, assuming normal growth conditions (Felle, 2001; Tsai and Schmidt, 2021). Ultimately, this allows for cell elongation. In contrast, the pH in the cytosol is slightly alkaline, with values around 7.5 (Felle, 2001). The cytosol has a stronger buffer capacity (20 to 80 mM H+ per pH unit) than the apoplast (low millimolar range per pH unit) (Oja et al., 1999; Felle, 2001), probably because dramatic pH changes within the cytosol would likely produce devastating effects on the major part of the cellular metabolism processes, small pH changes are enough to act as the signaling element (Kader and Lindberg, 2010). Thus, pHapo has a higher dynamic range than the cytosolic pH, which potentially might give the apoplast the capacity to create more complex signaling. The root apoplast is a continuum through different cell root layers. However, the pHapo of the endodermis and the stele are significantly more acidic than the pHapo of the root external cell layers in mature areas of the root (Martinière et al., 2018). Plants keep this radial variation in pH even when the culture media surrounding the plant is significantly more alkaline (Martinière et al., 2018). One possible explanation that could justify the differences in the pHapo along the radial root cell layers is the establishment of a pH gradient that allows for the directionality of the nutrients acquisition. However, it is unknown whether the pHapo of the inner layers of the root becomes more alkaline upon stress, as it happens within the epidermal cell upon treatment with microbe-associated molecular pattern such as chitin (Felle et al., 2009; Kesten et al., 2019).
The rhizosphere is the volume of soil that has a direct exchange of molecules with the root. The nature of the root-soil interphase is influenced by the root secretions, the soil composition, and their associated microorganisms. There are substantial levels of molecule diffusion between the rhizosphere and the apoplast of the root outer layers which hints at the strong influence of the rhizosphere over the root pHapo (Alassimone et al., 2012). Interestingly, the pHapo close to the plasma membrane (PM) stays acid even when the pH of the media gets significantly more alkaline, highlighting the strong regulatory mechanisms involved in maintaining the pH stable (Martinière et al., 2018). The rhizosphere pH is an essential element in plant nutrient acquisition because it determines the solubility and availability of these nutrients. In general, plants are adapted to grow in soils with a pH in the range between 5.5 and 7.5 because in these conditions the nutrient availability is optimized (Msimbira and Smith, 2020) (Figure 1). Acid soils favor micronutrients such as iron (Fe) or manganese (Mn) acquisition, while neutral or slightly alkaline soils increase the availability of macronutrients such as nitrogen (N), phosphorous (P), or potassium (K). Nevertheless, the rhizosphere pH must be balanced to avoid the deficiency or toxicity, respectively, of some ions such as Al+3 or Fe2+ (Msimbira and Smith, 2020). Hence, plants have different mechanisms to actively change the pHapo, which will also influence the rhizosphere's pH. In fact, it has been observed that the overexpression of H+-ATPase in rice plants changes the rhizosphere's pH, enhances nutrient uptake, and increases yield production (Zhang et al., 2021). Thus, the modulation of root pHapo represents an interesting target in biotechnology.
The root pHapo is altered through endogenous and exogenous processes. Here, we will focus on the endogenous, plant-mediated processes which play a dominant role in the establishment of the pHapo, i.e., PM proton pumps activity, root respiration, organic anion release, redox-coupled process, and the CW (Figure 2).
Figure 2. Origin and consequences of pHapo changes. The root pHapo is determined by different cellular elements and processes, such as root respiration, redox-coupled reactions, CW structure, organic acids, and the activity of AHAs. Changes in pHapo affect the activity of CW modifying proteins (CWMPs), water and nutrient uptake, and the cellular response. The pKa is predicted to be more acidic in the CW than the apoplastic fluid. The AHA activity participates in the creation of the proton motive force (PMF), which is used by different transporters to internalize nutrients. Calcium ions (showed as Ca2+) contribute to CW structure by interacting with pectins and forming the egg- box in a pH-dependent manner. AHA activity is regulated by phytohormones and by CW integrity sensors after perceiving signals at the apoplast (like RALFs and DAMPs), leading to apoplast alkalization.
Extrusion of H+ by the proton pumps at the PM is the dominant mechanism used by plants to regulate the differential pH between the cytosol and the apoplast (Figures 1, 2). These pumps use the energy derived by the ATP to create a proton gradient that contributes to the generation of a proton motive force (PMF) (Haruta and Sussman, 2012; Wegner and Shabala, 2020). This PMF is used to then drive solute and water uptake into the cell (Cosse and Seidel, 2021). Among the 11 plasma proton pumps in Arabidopsis thaliana, named AUTOINHIBITED H+-ATPases (AHAs) (Axelsen and Palmgren, 2001), the most expressed ones are AHA1 and AHA2, that share an elevated level of functional complementation, and which importance is evidenced by the embryo lethality of the double mutant (Haruta et al., 2010). Importantly, the fact that the overexpression of AHAs does not show any obvious phenotypical effect suggests that the levels of these proteins are tightly controlled and/or the regulation of their activity is mainly acting posttranslationaly. AHA activity is regulated through the phosphorylation/de-phosphorylation of different amino acid residues by various proteinaceous interaction partners. These interactions are driven by phytohormones, lipids, and Ca2+ signaling, all of which are highly conserved among plants (Haruta et al., 2015; Falhof et al., 2016). This plethora of AHAs-activity regulators shows that these pumps have an extraordinary impact on many key aspects of plant biology and any disturbance could have disastrous consequences for plant fitness (Gévaudant et al., 2007). However, the identity of direct regulators of AHAs is still elusive. It is also unknown and unexplored whether the variations in the level of activation of the AHAs are part of a signaling cascade on their own, in addition to the role of the pH as a secondary messenger. Indeed, mutants displaying AHA overactivation, such as the mutant on the companion cellulose synthase (cc1cc2) or FERONIA (fer-4), show an upregulation of defense-related genes, making them more tolerant to pathogen attack and supporting the potential direct connection between AHA activity and plant defense (Masachis et al., 2016; Kesten et al., 2019).
Root respiration produces at least 50% of the CO2 present in the soil (Pregitzer et al., 2007). When there is high availability of N in the soil, plants can enhance their growth rate, increasing significantly the CO2 concentration in these soils (Pregitzer et al., 1998). The CO2 present in the soil interacts with the water forming weak acid carbonate (H2CO3) that, in neutral and alkaline environments, will release H+ and acidify the apoplast and the rhizosphere (Figure 2).
Malate, oxalate, fumarate, malonate, succinate, and oxalacetate are some of the organic anions found in root exudates (Vančura and Hovadík, 1965; Wegner et al., 2021). They are released by the cell in a deprotonated form because their pKa is usually lower than the pHapo (Hinsinger et al., 2003), helping the cell to buffer the proton extrusion generated by the AHAs when the pHapo becomes too acidic (Figure 2). For this reason, some of the organic anions transporters at the PM are activated upon extracellular pH drop (Liang et al., 2013). Moreover, these molecules are secreted by the roots to improve P acquisition (Lambers et al., 2006) or increase plant tolerance to elevated levels of aluminum (Al) (Yang et al., 2013), which might take place during rhizosphere acidification.
The oxidation state of key elements such as Fe, Mn, N, or Sulfur (S) is coupled with the pHapo because the direction of the reaction to change their oxidation state is conditioned by the amount of H+ present in the media (Hinsinger et al., 2003). For example, the chemical reduction of Fe3+ consumes H+ ions increasing the pHapo, while the chemical oxidation of Fe2+ acidifies the soil (van Breemen, 1987) (Figure 2).
The plant CW is mainly composed of the carbohydrates cellulose, hemicellulose, and pectin, with proteins embedded in it. Pectin is enriched in uronic acids, mostly galacturonic acids (GalAs), whose carboxyl group is negatively charged. Pectin is secreted to the apoplast and heavily methylated at their GalAs. Its demethylation at the CW and the potential link with Ca2+ ions to form the so-called egg-boxes alters the charge balance of the CW (Shomer et al., 2003). The pKa value of carboxyl groups in the GalAs is between 4.0 and 5.0, which means they behave as weak acids helping to maintain acidic apoplastic spaces (Meychik and Yermakov, 2001). The CW arabinogalactan proteins also contain uronic acids (Seifert and Roberts, 2007) which, potentially, could also contribute to the CW charge, although the quantitative importance of this possibility is not clear. It has been reported that the pH of the CW is more acid than the apoplastic fluid (Sentenac and Grignon, 1981, 1987), suggesting that the CW carboxyl groups might be highly protonated, based on their pKa, in a steady state apoplast (Figure 2), but this should be corroborated with more accurate methods.
All these mechanisms are linked to environmental conditions. Proton pumps are induced in Fe-deficient roots (Santi and Schmidt, 2009), and their overexpression enhances N and C uptake by rice (Zhang et al., 2021). Redox-coupled reactions with Fe, N, or S might cause temporal variation in the soil pH during seasonal flooding (van Breemen, 1987). Organic acids produce changes in soil pH increasing nutrient availability (Liu et al., 2022) and might contribute to microbial growth, which together with root respiration, will produce CO2 with a strong influence on plant biology in alkaline soils (Hinsinger et al., 2003). Overall, the plant mechanisms to modify pHapo have a critical role under stress conditions, such as high salinity or drought (Miao et al., 2022).
Phytohormone signaling pathways can be triggered in response to different stresses and help the plant to adjust to specific necessities. Some of the hormonal signaling pathways alter the pHapo as a consequence of their response mechanisms.
Abscisic acid (ABA) is considered a stress-response hormone whose signaling is triggered as a result of environmental stress including drought, high soil salinity, temperature, and metal soil contamination (Roberts and Snowman, 2000; Zörb et al., 2013; Urano et al., 2017). Treatment with ABA specifically inhibits the AHA activity, alkalizing the apoplast and impairing the hypocotyl elongation (Hayashi et al., 2014) or root growth (Planes et al., 2015). However, the downregulation of AHA activity induced by ABA in guard cells is determinant of stomatal closure (Bauer et al., 2013; Miao et al., 2022). Inversely, lower concentrations of ABA activate the AHAs' activity, stimulating the root growth (Miao et al., 2021). Certainly, there is controversy among published ABA data regarding its influence on pHapo, pointing out that the effect of ABA on pHapo might be concentration- and cell-type-dependent. ABA also exerts different effects on different plant species; for example, saline stress and ABA-mediated response cause the inhibition of the activity of proton pumps and pHapo alkalinization in tomato roots (Gronwald et al., 1990), whilst in cucumber roots, the same conditions activate the activity of the proton pumps (Janicka-Russak and Kłobus, 2007). The different effects that ABA has on the pHapo suggest that it is not the only factor influencing AHA activity and other signaling elements seem to be essential in regulating the pHapo.
Over the last years, it has been extensively discussed the role of auxin in plant cell growth. Auxin enhances AHA activity by mediating the phosphorylation of the penultimate threonine residue within the C-terminal autoinhibitory domain of AHAs (Takahashi et al., 2012). This auxin's AHA activation implies the induction of SAUR19 gene expression, which inhibits the PP2C-D phosphatase activity, required for the dephosphorylation of the C-terminal autoinhibitory domain of AHAs. Since PP2C-D is not active, the AHA's C-terminal autoinhibitory domain remains active (Spartz et al., 2014). The activation of AHAs allows for the loosening of the CW (read below, section the impact of pHapo on apoplastic biology) facilitating cell growth (Barbez et al., 2017; Du et al., 2020). The role of auxin in regulating the pHapo is biphasic and dependent on its concentration. The above mechanism describing AHA activation and the pHapo acidification by auxin is observed under endogenous auxin concentrations. However, high levels of auxin applied externally trigger a fast pHapo alkalinization that requires the FERONIA receptor activity and induces the concomitant inhibition of cell expansion (Barbez et al., 2017). Thus, a controversial aspect of the auxin effect on the pHapo has raised in the last years, where the auxin regulation of the pHapo seems to be dependent not only on its local concentration but also on the cell type or/and plant tissue where auxin acts. It has recently been reported that the same auxin concentration can promote growth in shoots while having the opposite effect on roots. This implies the existence of differential activity of auxin receptors, such as TIR1/AFB and TMK1, that is dependent on tissue localization (Li et al., 2021; Lin et al., 2021).
The function and signaling of the gaseous hormone ethylene (ET) are intimately related to auxin in regulating plant development. ET is also a major regulator of stress responses (Vaseva et al., 2018), and the external application of ET or its precursor, the 1-aminocyclopropane-1-carboxylic acid, induces a fast apoplastic alkalization inhibiting the fast cell elongation of epidermal root cells (Staal et al., 2011). ET modulates the alkaline stress-mediated inhibition of root growth by increasing auxin accumulation via induction of auxin biosynthesis-related genes, control of local auxin biosynthesis, and the expression of the auxin transporter AUX1 (Li et al., 2015; Vaseva et al., 2018) (Figure 2). ET signaling can also modulate cellular responses involved in plant acclimation to acidic pH by regulating the activity of the class III peroxidases (CIII Prxs). The CIII-Prx produces CW modifications, such as hydroxyproline-rich glycoproteins crosslinking and callose deposition lead to higher CW stiffness, and higher tolerance to extremely low pH ultimately leads to an arrest in cell expansion during stress conditions (De Cnodder et al., 2005; Graças et al., 2021).
Cytokinin (CK) hormonal actions usually antagonize those of auxin effects and can counterpart its signaling. CK has a key role in root growth, intervening in the balance between cell division and cell differentiation within the root tip (Dello Ioio et al., 2007; Montesinos et al., 2020). CK participates in the establishment of the root transition zone, determining the root meristem size, through the activation of the AHAs that acidify the pHapo and the activation of the α-expansin EXPA1. The latter helps in the loosening of the CW, and the beginning of the cell expansion in the root elongation zone. Thus, the pHapo acidification of the root mediated by the CK activation of AHA1 and AHA2 proton pumps is necessary to control the initiation of cell differentiation (Pacifici et al., 2018). Curiously, although auxin and CK usually have opposing effects, they can promote similar cellular responses that are dependent on their concentration, signal duration, and cell type.
Brassinosteroids (BRs) are plant steroidal hormones that, similar to auxin and CK, participate in the regulation of plant growth and differentiation by controlling cell division and expansion. BRs can activate the AHAs during hypocotyl elongation by inducing the phosphorylation in their penultimate amino acid (Thr) (Minami et al., 2019). In this line, it was shown that the BR-receptor BRI1-BAK1 system interacts directly with AHA2 and AHA7 proteins in vivo. However, it remains unclear whether this interaction is enough to phosphorylate and activate these H+-ATPases (Miao et al., 2018, 2022; Yuan et al., 2018). Remarkably, little is known about how BRs can modify the pHapo in root cells and further investigation in this direction will be necessary for the coming years.
The fact that different phytohormones converge in regulating the pHapo supports the idea that pHapo might be a universal language among cells to coordinate cell responses.
Another plant's short-distance communication signaling mechanism is mediated by the RALF (Rapid Alkalinization Factor) small signaling peptides (Figure 2). RALFs peptides regulate plant patterning and development (Murphy and De Smet, 2014). This family of small peptides holds 34 members in A. thaliana, and as its name indicates, they have been associated with alkalinization of the extracellular medium (Pearce et al., 2001; Blackburn et al., 2020). Concretely, RALF1 interacts with the PM-localized receptor FERONIA (FER) to regulate the pHapo. This interaction leads to AHA2 phosphorylation reducing its activity and a fast alkalinization of the apoplast that inhibits root cell elongation (Haruta et al., 2014). RALF33 and RALFL36 treatment decreased by 3-fold the H+ pumping activity of H+-ATPases and increase the pHapo, inhibiting root growth (Gjetting et al., 2020). RALF23 overexpression leads to an alkalinization of the rhizosphere and inhibition of the root growth (Srivastava et al., 2009). RALF23 could exert part of its function by altering the nanoscale organization of the BRs-receptor BAK1, and its interaction with FER (Gronnier et al., 2022). Interestingly, pathogens like Fusarium oxysporum also secrete RALF-like peptides during their plant infection to produce a pHapo alkalinization that enables fungal colonization (Masachis et al., 2016).
Overall, having such a complex and evolving system that includes hormonal and small peptides regulation to modify pHapo suggests that pHapo is a way to escalate the cell response signal, acting as an element to regulate the activity and properties of many different proteins and molecules at once.
Changes in pHapo have a direct impact on the CW integrity and the properties of the proteins embedded in it. The balance of protonation in pectin carboxyl groups determines their capacity to interact with cations, mostly Ca2+ to form the egg-box, and affects the pectin–cellulose and pectin–pectin interactions, which has a significant impact on the structure of the CW (Meychik et al., 2021) (Figure 2). During plant cell elongation, precise and local acidification cycles of pH changes occur, changing the CW components' interaction and causing a CW-restructuring without compromising the cell integrity leads to unidirectional cell growth (Hocq et al., 2017; Arsuffi and Braybrook, 2018; Majda and Robert, 2018). The activity of several CW modifying proteins (CWMPs), such as expansins, is pH-dependent (Cosgrove, 1999; Sampedro and Cosgrove, 2005). The precise control of H+ fluxes and the spatio-temporal specificity of expansins expression and localization are essential in cell elongation (Samalova et al., 2022). In fact, salt stress alters the expansins expression pattern in wheat, affecting the cell wall extensibility under different pHapo (Shao et al., 2021). Expansins promote the loosening of the CW while the increase of the turgor pressure created by the enhanced intake of K+ allows the cells to grow (Channels, 1991; Mathur and Hülskamp, 2001; Hager, 2003; Velasquez et al., 2016; Phyo et al., 2019). On the other hand, alkaline pHs induce the activity of other CWMPs, such as extensins and peroxidases, which fortify the CW (Castilleux et al., 2021). Overall, we propose that the ability to create microdomains within the apoplast, by tightly regulating the pHapo oscillations, might allow the cell to precisely modify its CW and adapt its growth to its developmental needs. However, this idea needs further experimental validation.
The homeostatic balance of certain ions between the apoplast and the cytosol is a key element in plant development and is closely related to proton level regulation. The strong ion difference (SID) represents the net charge between the cations and anions and it has a direct influence on the pH (Gerendás and Schurr, 1999). Proton ATPases energize ions secondary transporters, such as K+, Mg2+, or Cl−, to balance the net charge created by proton transport and to permit a pH gradient (Good, 1988; Hangarter and Good, 1988; Gerendás and Schurr, 1999). K+ is one of the most abundant cations present in plants since it is essential for their growth, and it has a strong connection with the pHapo. In a situation with low levels of apoplastic K+, acidification of the extracellular space enhances K+ uptake, allowing for cell growth (Chen and Gabelman, 2000; Minjian et al., 2007). On other occasions, the pHapo is affected by high levels of toxic anions, such as Na+ which induces apoplastic alkalinization (Foster and Miklavcic, 2020) or NH4+ which produces apoplastic acidification (Liu and von Wirén, 2017). Likely, it is in those moments, in which the apoplastic balance ion is affected, when the pHapo might act as a signal to activate the cell response.
The CW also takes part in the apoplastic cation/anion balance in a pH-dependent manner. In standard growth conditions, pectin binds Ca2+ and B3+ (O'Neill et al., 2004; Phyo et al., 2019). However, those cations might be displaced by protons if the pH decreases significantly thus modifying the CW structure. Moreover, in the case of a significant increase for another cation, such as Na+ during saline stress, we hypothesize that Na+ could compete for the negative charges at the CW, and replace protons, boron, and calcium from the carboxyl groups of pectins and AGPs. In fact, during salt stress the apoplastic space becomes alkaline which might enhance the interaction of Na+ with the CW, allowing its compartmentalization and decreasing its toxicity. Interestingly, treatment with anions like SO or NO, which are abundant in nature, does not trigger apoplastic pH changes, suggesting a strong compensatory mechanism related to the presence of anions (Martinière et al., 2018).
The activity of several transporters is connected with the H+ flux across the PM (Zhou et al., 2021). In Arabidopsis, pH 6.6 seems to be optimal for AHA transport activity (Olivari et al., 1993; Hoffmann et al., 2019), although, at lower pHs, the activity of the AHAs is enhanced due to the cytosolic acidification (Liang et al., 2020). The Na+/H+ exchangers (NHXs) use the H+ influx to pump Na+ extracellularly (Aharon et al., 2003), and their activity might be enhanced with higher AHA activity (Fan et al., 2019). This leads us to think that the apoplastic alkalinization observed upon salt stress might facilitate AHA's activity. The cation/H+ exchangers (CHX) are hypothesized to use the H+ gradient in a similar way to that described for NHXs, but it needs to be experimentally demonstrated (Sze and Chanroj, 2018). The extracellular acidification also stimulates the activity of N transporters such as ammonium transporter (AMT) or nitrate transporter (NRT) (Søgaard et al., 2009; Fan et al., 2016). The activity of the different isoforms of phosphate transporters (PHT) has different optimal pHs (Ai et al., 2009; Sun et al., 2012; Wang et al., 2014). Interestingly, the loss of AHA2 function coincides with the downregulation of K transporters (Hoffmann et al., 2019). All these examples underline that the pHapo influences many of the most relevant families of ion transporters with significant consequences in plant physiology.
Plants need to adapt constantly to a dynamic environment, and for that, they require systems that monitor multiple stimuli and integrate this information to generate long-distance signals that serve for communication and generation of responses in different tissues and organs. The unification of these different cellular signaling systems has been an object of study in the last decades. The pHapo is closely related to electric signaling, glutamate, Ca2+, and ROS systems, interacting with each other to provide the appropriate duration, localization, and physiological context in response to the stimuli that generate the signals (Johns et al., 2021). Different examples illustrate how pHapo coordinates with Ca2+ and the generated electrical signals, also named slow wave potentials (SWPs). For example, the inactivation of the electrogenic proton pumps, and consequent alkalinization of the pHapo, have been implicated in the generation of SWPs (Kumari et al., 2019); or the constitutive activation of AHA1 inhibits both Ca2+ waves and SWP generation (Shao et al., 2020). Furthermore, the reactivation of the AHA1 activity is fundamental for the repolarization of the PM and the restoration of the membrane potential after the signal transmission (Kumari et al., 2019; Johns et al., 2021).
Ion channels like the GLUTAMATE RECEPTOR–LIKE (GLR) family act as sensors that convert the external signal into an increase in intracellular Ca2+ concentration, generating Ca2+ waves and the SWPs, that propagate to distant organs (Toyota et al., 2018). The fact that the GLRs (GLR3.3 and GLR3.6) are only active when the pHapo is above 6.5, indicates that alkaline pHapo is an essential condition for these channels for opening (Shao et al., 2020). Furthermore, the constant activation of H+-ATPases by fusicoccin generates an increase in the extracellular protons, which impairs the depolarization phase of glutamate-induced SWPs (Shao et al., 2020), illustrating once more, the high dependency on the activity of these transporters on pHapo. Other calcium transporters such as CNGC14 also show the existing connection between Ca2+ signaling and pHapo, since the loss-of-function cngc14 mutant losses the capacity to alkalinize the apoplast after high auxin concentration treatments (Shih et al., 2015). In general, different abiotic stresses produce different hallmarks in pHapo and Ca2+ dynamics in the cytosol and the apoplast, this variety of signaling signatures allows the cell to respond accordingly to the specific stimulus (Gao et al., 2004).
Reactive oxygen species (ROS) waves can propagate through different plant tissues similarly to electric and calcium waves during long-distance signaling (Johns et al., 2021). The increased levels of intracellular Ca2+ can activate the RESPIRATORY BURST OXIDASE HOMOLOG D (RBOHD) PM-localized enzymes, that synthesize ROS, such as H2O2, in the apoplastic space during the plant electrical/ionic system signaling (Gilroy et al., 2016; Johns et al., 2021). Likewise, pHapo affects ROS production, being the alkalization of the apoplast an essential step for the generation of H2O2 (Bolwell et al., 1995; Monshausen et al., 2007). The PM is permeant to H2O2 and thereby variations in pHapo can be translated to the interior of the cell. The presence of H2O2 will amplify the signaling by interacting with proteins with redox-sensitive moieties in the cytosol or other subcellular compartments (Antunes and Brito, 2017; Rampon et al., 2018; Janku et al., 2019). Moreover, oscillations in cytosolic H2O2 might modulate the AHA activity and participate in pHapo regulation (Mangano et al., 2018). While pHapo alkalinization contributes to CW stiffening through H2O2 production, low pHapo levels can help the protonation of superoxide anion radicals (i.e., •OH), which promote CW loosening (Pottosin et al., 2014). Contrary to what one might think, active oxygen species production does not seem to contribute to alkalinization of the apoplast by H+ consumption, since in tobacco cells have been observed that the NtrbohD oxidase activity does not affect the extracellular pH (Simon-Plas et al., 2002).
Environmental events affect simultaneously the rhizosphere and the pHapo. Some of them promote acidification, like high rainfall, ammonium-based fertilizers, and plant growth on its own (Msimbira and Smith, 2020). On the other hand, irrigation with water containing prominent levels of bicarbonates or drought tends to alkalinize the rhizosphere in a long term (Odutola Oshunsanya, 2019). Saline stress (NaCl) is one of the most common severe stresses faced by plants, producing pHapo alkalinization because of two events happening concomitantly: Cl− ions are internalized via symport with 2 H+ using the proton gradient generated by the AHAs (Geilfus, 2017) and salt-induced ABA-increase downregulates the AHA activity (Falhof et al., 2016; Geilfus, 2017). Nevertheless, ABA should have a secondary role since the levels of ABA are still increasing while the pH starts to drop in the apoplast (Zhang et al., 2016). Importantly, alkaline soils induce similar effects on plants as salt stress and both stresses have a synergistic effect exacerbating the plant responses when both are present (Xu et al., 2013), likely because the plants tend to accumulate more Na+ in alkaline substrates (Guo et al., 2010).
Moderate water stress enhances H+ efflux in the root apoplast to facilitate water uptake (Siao et al., 2020), but alkalinization of the apoplast is observed in the leaf (Geilfus, 2017). The same stimulus having an opposite effect on pHapo fluctuation in different plant tissues suggests that pHapo might originate the signal to the plant response but the direction of the pHapo changes provides the meaning of the pH signaling.
pHapo regulation during biotic interactions is time, spatial and microbial dependent, thus generalizations are not possible. Some fungal pathogens, such as the root vascular fungus F. oxysporum (Fo), induce fast apoplastic acidification in the first stages of contact by increasing the AHA activity, which seems to be required for plant defense (Kesten et al., 2019). Later, a strong transcriptional downregulation of the AHAs and an upregulation of their inhibitors (Menna et al., 2021), together with the secretion by Fo of RALF peptide, provokes an apoplastic alkalinization that favor Fo infection (Masachis et al., 2016). However, it remains to be explained how the fungus advances through a CW which might become stiffer upon alkalinization. Conversely, other fungal pathogens, like Sclerotinia sclerotiorum, induce acidification by the secretion of organic acids, which induce cell death (Cessna et al., 2000).
Microbes and small herbivores cause wounds and mechanical stress to plants. The response to these alterations includes the synthesis in situ of the plant hormone Jasmonic Acid (JA). JA triggers a cellular response that can travel quickly from the wound to distal cells and from the root to the shoot. In this process, the alteration of the pHapo is essential to create the intracellular signaling that includes the SWPs, glutamate, Ca2+, and ROS response. Concretely, during wound response, the activity of AHA1 is transiently inhibited at the beginning of the signaling, alkalinizing the pHapo. The apoplastic proton variance is required to generate the PM depolarization and to propagate the SWP. The AHA activity is also important during the PM repolarization phase, where its activity is recovered (Kumari et al., 2019; Shao et al., 2020).
Soil pH alters the microbiota of the rhizosphere that can potentially interact with the root. In general, acid soils enhance fungal over bacterial growth, which might favor fungal pathogens spreading, and alkaline soils favor bacterial growth (Rousk et al., 2009, 2010). However, acidification of the rhizosphere has also been reported to enhance the spread of soil-borne bacterial pathogens (Li et al., 2017).
Rhizosphere and pHapo proton levels influence the growth of both the plant and the intruder and their defense and virulence, respectively. Nevertheless, it is the timing and the dynamic of the pHapo changes that have a leading role in biotic interactions, and the organism that controls or tolerates better those pH oscillations prevails. We propose that those pHapo changes which return to basal levels, such as the strong and fast acidification seen upon Fo contact, would have the potential to contribute to cell signaling. On the other hand, stress-induced long-term pHapo changes would be a structural situation as a product of the earlier signaling.
pHapo fluxes in response to physiological needs or environmental stress might have strong consequences for plant cells beyond the ones described so far. Based on different lines of evidence discussed through this review, we predict that the intensity, duration, direction, and tissue/cellular localization of the pHapo changes could act as a signal for the root to cope with those different and very challenging stresses. Therefore, in this last section, we discuss potential areas of research to undertake in the next years.
The apoplast is a heterogenous and dynamic compartment and so is its pH. Although some works have shown those differences in the pHapo within different apoplastic regions (Sentenac and Grignon, 1981, 1987; Martinière et al., 2018), pHapo measurements are presumably an average of different subdomains and future experiments should be focused on revealing the dynamics of those pH microdomains in vivo. It is particularly interesting to resolve the participation of specific components of the CW in pHapo since CW has a different composition even in different areas of the same cell.
An interesting concept to explore in the future is the alteration that the pHapo exerts on the cell cortex pH. AHA activation, and the consequent apoplastic acidification, modify the pH on the internal side of the PM, while the rest of the cytosol is able to keep the pH stable (Kesten et al., 2019). Thus, the pH in the cell cortex area is slightly different from the rest of the cytosol and presumably should be tightly regulated to maintain the PMF (Figure 2). Moreover, variations in the cell cortex pH should alter the important cellular processes that occur in this area, such as the cortical microtubules polymerization and depolymerization, and the activation of cell surface receptors and transporters (Lomin et al., 2012; Kour et al., 2021; Lanassa Bassukas et al., 2022). However, we still need to understand this regulation.
Different stresses tend to activate more than one hormonal pathway and, in some cases, the transcriptional responses from each hormone are specific to the stress (Nemhauser et al., 2006). Interestingly, one of the common points in this interplay is the AHA-dependent pHapo changes, which happen concomitantly with the action of the specific plant hormone. Understanding how those phytohormone-dependent pHapo changes are connected with a certain response of the plant might provide a potential biotechnological tool to enhance plant resilience under different stresses.
Higher pHapo together with the increase in ROS, certain plant hormones, or cytosolic Ca2+ waves stimulate the deposition of callose, lignin, and suberin in the CW. These highly resilient molecules help to protect the cell against abiotic and biotic stresses (Miedes et al., 2014; Geilfus et al., 2017; Bacete et al., 2018; Vaahtera et al., 2019), but plant cell growth becomes seriously compromised. Nowadays, the use of microbial and plant CW-derived molecules is a potential strategy to prime plant tolerance to pathogens (Molina et al., 2021), although their impact on crop yield remains a problem to be solved. We envision that the identification of molecules that modulate the pHapo in a more localized, transient, and weaker way will be an interesting biotechnological solution to promote plant stress tolerance with lower collateral effects on growth.
Environment and internal signaling integration provides the plant with the proper perception of its biological status. In this sense, pHapo is a ubiquitous element that emerges as a perfect candidate to unify the different inputs and generate a homogenous response. The pHapo is connected with well-described cell messengers: plant hormones, ROS, and cytosolic Ca2+. Although many aspects remain unsettled, pHapo might be the general switch that plant physiology relies on.
FMG-A, CS-R, and JCM conceived the review. FMG-A and JCM wrote the review with comments and edits from CS-R. All authors contributed to the article and approved the submitted version.
The work described in this review was supported by the Vontobel Foundation to FMG-A and CS-R, and the ETH Zurich Career Seed Grant (SEED-09 21-1) to FMG-A and JCM. Open access funding provided by ETH Zurich.
The authors declare that the research was conducted in the absence of any commercial or financial relationships that could be construed as a potential conflict of interest.
All claims expressed in this article are solely those of the authors and do not necessarily represent those of their affiliated organizations, or those of the publisher, the editors and the reviewers. Any product that may be evaluated in this article, or claim that may be made by its manufacturer, is not guaranteed or endorsed by the publisher.
Aharon, G. S., Apse, M. P., Duan, S., Hua, X., and Blumwald, E. (2003). Characterization of a family of vacuolar Na+/H+ antiporters in Arabidopsis thaliana. Plant Soil 253, 245–256. doi: 10.1023/A:1024577205697
Ai, P., Sun, S., Zhao, J., Fan, X., Xin, W., Guo, Q., et al. (2009). Two rice phosphate transporters, OsPht1;2 and OsPht1;6, have different functions and kinetic properties in uptake and translocation. Plant J. 57, 798–809. doi: 10.1111/j.1365-313X.2008.03726.x
Alassimone, J., Roppolo, D., Geldner, N., and Vermeer, J. E. M. (2012). The endodermis–development and differentiation of the plant's inner skin. Protoplasma 249, 433–443. doi: 10.1007/s00709-011-0302-5
Antunes, F., and Brito, P. M. (2017). Quantitative biology of hydrogen peroxide signaling. Redox Biol. 13, 1–7. doi: 10.1016/j.redox.2017.04.039
Arsuffi, G., and Braybrook, S. A. (2018). Acid growth: an ongoing trip. J. Exp. Bot. 69, 137–146. doi: 10.1093/jxb/erx390
Axelsen, K. B., and Palmgren, M. G. (2001). Inventory of the superfamily of P-type ion pumps in Arabidopsis. Plant Physiol. 126, 696–706. doi: 10.1104/pp.126.2.696
Bacete, L., Mélida, H., Miedes, E., and Molina, A. (2018). Plant cell wall-mediated immunity: cell wall changes trigger disease resistance responses. Plant J. 93, 614–636. doi: 10.1111/tpj.13807
Barbez, E., Dünser, K., Gaidora, A., Lendl, T., and Busch, W. (2017). Auxin steers root cell expansion via apoplastic pH regulation in Arabidopsis thaliana. Proc. Natl. Acad. Sci. U S A. 114, E4884–E4893. doi: 10.1073/pnas.1613499114
Bauer, H., Ache, P., Lautner, S., Fromm, J., Hartung, W., Al-Rasheid, K. A. S., et al. (2013). The stomatal response to reduced relative humidity requires guard cell-autonomous ABA synthesis. Curr. Biol. 23, 53–57. doi: 10.1016/j.cub.2012.11.022
Behera, S., Zhaolong, X., Luoni, L., Bonza, M. C., Doccula, F. G., De Michelis, M. I., et al. (2018). Cellular Ca2+ signals generate defined pH signatures in plants. Plant Cell 30, 2704–2719. doi: 10.1105/tpc.18.00655
Blackburn, M. R., Haruta, M., and Moura, D. S. (2020). Twenty years of progress in physiological and biochemical investigation of RALF peptides. Plant Physiol. 182, 1657–1666. doi: 10.1104/pp.19.01310
Blake, L. (2005). “Acid Rain and Soil Acidification,” in Encyclopedia of Soils in the Environment, ed D. Hillel (Oxford: Elsevier), 1–11. doi: 10.1016/B0-12-348530-4/00083-7
Bolwell, G. P., Butt, V. S., Davies, D. R., and Zimmerlin, A. (1995). The origin of the oxidative burst in plants. Free Radic. Res. 23, 517–532. doi: 10.3109/10715769509065273
Castilleux, R., Plancot, B., Vicré, M., Nguema-Ona, E., and Driouich, A. (2021). Extensin, an underestimated key component of cell wall defence? Ann. Bot. 127, 709–713. doi: 10.1093/aob/mcab001
Cessna, S. G., Sears, V. E., Dickman, M. B., and Low, P. S. (2000). Oxalic acid, a pathogenicity factor for Sclerotinia sclerotiorum, suppresses the oxidative burst of the host plant. Plant Cell 12, 2191–2200. doi: 10.1105/tpc.12.11.2191
Channels, P. (1991). Electrogenic transport properties of growing Arabidopsis root Hairs1. Plant Physiol. 97, 1527–1534. doi: 10.1104/pp.97.4.1527
Chen, J., and Gabelman, W. H. (2000). Morphological and physiological characteristics of tomato roots associated with potassium-acquisition efficiency. Sci. Hortic. 83, 213–225. doi: 10.1016/S0304-4238(99)00079-5
Cosgrove, D. J. (1999). Enzymes and other agents that enhance cell wall extensibility. Annu Rev. Plant Physiol. Plant Mol. Biol. 50, 391–417. doi: 10.1146/annurev.arplant.50.1.391
Cosse, M., and Seidel, T. (2021). Plant proton pumps and cytosolic pH-Homeostasis. Front. Plant Sci. 12:672873. doi: 10.3389/fpls.2021.672873
De Cnodder, T., Vissenberg, K., Van Der Straeten, D., and Verbelen, J.-P. (2005). Regulation of cell length in the Arabidopsis thaliana root by the ethylene precursor 1-aminocyclopropane- 1-carboxylic acid: a matter of apoplastic reactions. N. Phytol. 168, 541–550. doi: 10.1111/j.1469-8137.2005.01540.x
Dello Ioio, R., Linhares, F. S., Scacchi, E., Casamitjana-Martinez, E., Heidstra, R., Costantino, P., et al. (2007). Cytokinins determine Arabidopsis root-meristem size by controlling cell differentiation. Curr. Biol. 17, 678–682. doi: 10.1016/j.cub.2007.02.047
Du, M., Spalding, E. P., and Gray, W. M. (2020). Rapid auxin-mediated cell expansion. Annu. Rev. Plant Biol. 71, 379–402. doi: 10.1146/annurev-arplant-073019-025907
Falhof, J., Pedersen, J. T., Fuglsang, A. T., and Palmgren, M. (2016). Plasma membrane H(+)-ATPase regulation in the center of plant physiology. Mol. Plant 9, 323–337. doi: 10.1016/j.molp.2015.11.002
Fan, X., Tang, Z., Tan, Y., Zhang, Y., Luo, B., Yang, M., et al. (2016). Overexpression of a pH-sensitive nitrate transporter in rice increases crop yields. Proc. Natl. Acad. Sci. U.S.A. 113, 7118–7123. doi: 10.1073/pnas.1525184113
Fan, Y., Yin, X., Xie, Q., Xia, Y., Wang, Z., Song, J., et al. (2019). Co-expression of SpSOS1 and SpAHA1 in transgenic Arabidopsis plants improves salinity tolerance. BMC Plant Biol. 19:74. doi: 10.1186/s12870-019-1680-7
Felle, H. H. (2001). PH: signal and messenger in plant cells. Plant Biol. 3, 577–591. doi: 10.1055/s-2001-19372
Felle, H. H., Herrmann, A., Hückelhoven, R., and Kogel, K.-H. (2005). Root-to-shoot signalling: apoplastic alkalinization, a general stress response and defence factor in barley (Hordeum vulgare). Protoplasma 227, 17–24. doi: 10.1007/s00709-005-0131-5
Felle, H. H., Waller, F., Molitor, A., and Kogel, K.-H. (2009). The mycorrhiza fungus Piriformospora indica induces fast root-surface pH signaling and primes systemic alkalinization of the leaf apoplast upon powdery mildew infection. Mol. Plant. Microbe. Interact. 22, 1179–1185. doi: 10.1094/MPMI-22-9-1179
Foster, K. J., and Miklavcic, S. J. (2020). A comprehensive biophysical model of ion and water transport in plant roots. III. Quantifying the energy costs of ion transport in salt-stressed roots of Arabidopsis. Front. Plant Sci. 11:865. doi: 10.3389/fpls.2020.00865
Gao, D., Knight, M. R., Trewavas, A. J., Sattelmacher, B., and Plieth, C. (2004). Self-reporting Arabidopsis expressing pH and [Ca2+] indicators unveil ion dynamics in the cytoplasm and in the apoplast under abiotic stress. Plant Physiol. 134, 898–908. doi: 10.1104/pp.103.032508
Geilfus, C.-M., and Mühling, K. H. (2012). Transient alkalinization in the leaf apoplast of Vicia faba L. depends on NaCl stress intensity: an in situ ratio imaging study. Plant Cell Environ. 35, 578–587. doi: 10.1111/j.1365-3040.2011.02437.x
Geilfus, C.-M., Tenhaken, R., and Carpentier, S. C. (2017). Transient alkalinization of the leaf apoplast stiffens the cell wall during onset of chloride salinity in corn leaves. J. Biol. Chem. 292, 18800–18813. doi: 10.1074/jbc.M117.799866
Geilfus, C. M. (2017). The pH of the apoplast: dynamic factor with functional impact under stress. Mol. Plant 10, 1371–1386. doi: 10.1016/j.molp.2017.09.018
Gerendás, J., and Schurr, U. (1999). Physicochemical aspects of ion relations and pH regulation in plants—a quantitative approach. J. Exp. Bot. 50, 1101–1114. doi: 10.1093/jxb/50.336.1101
Gévaudant, F., Duby, G., von Stedingk, E., Zhao, R., Morsomme, P., and Boutry, M. (2007). Expression of a constitutively activated plasma membrane H+-ATPase alters plant development and increases salt tolerance. Plant Physiol. 144, 1763–1776. doi: 10.1104/pp.107.103762
Gilroy, S., Białasek, M., Suzuki, N., Górecka, M., Devireddy, A. R., Karpiński, S., et al. (2016). ROS, Calcium, and Electric signals: key mediators of rapid systemic signaling in plants. Plant Physiol. 171, 1606–1615. doi: 10.1104/pp.16.00434
Gjetting, S. K., Mahmood, K., Shabala, L., Kristensen, A., Shabala, S., Palmgren, M., et al. (2020). Evidence for multiple receptors mediating RALF-triggered Ca2+ signaling and proton pump inhibition. Plant J. 104, 433–446. doi: 10.1111/tpj.14935
Good, N. E. (1988). Active transport, ion movements, and pH changes: I. The chemistry of pH changes. Photosynth. Res. 19, 225–236. doi: 10.1007/BF00046875
Graças, J. P., Belloti, M., Lima, J. E., Peres, L. E. P., Burlat, V., Jamet, E., et al. (2021). Low pH-induced cell wall disturbances in Arabidopsis thaliana roots lead to a pattern-specific programmed cell death in the different root zones and arrested elongation in late elongation zone. Environ. Exp. Bot. 190:104596. doi: 10.1016/j.envexpbot.2021.104596
Gronnier, J., Franck, C. M., Stegmann, M., DeFalco, T. A., Abarca, A., von Arx, M., et al. (2022). Regulation of immune receptor kinase plasma membrane nanoscale organization by a plant peptide hormone and its receptors. Elife 11:e74162. doi: 10.7554/eLife.74162.sa2
Gronwald, J. W., Suhayda, C. G., Tal, M., and Shannon, M. C. (1990). Reduction in plasma membrane ATPase activity of tomato roots by salt stress. Plant Sci. 66, 145–153. doi: 10.1016/0168-9452(90)90198-W
Guo, R., Shi, L., Ding, X., Hu, Y., Tian, S., Yan, D., et al. (2010). Effects of saline and alkaline stress on germination, seedling growth, and ion balance in wheat. Agron. J. 102, 1252–1260. doi: 10.2134/agronj2010.0022
Hager, A. (2003). Role of the plasma membrane H+-ATPase in auxin-induced elongation growth: historical and new aspects. J. Plant Res. 116, 483–505. doi: 10.1007/s10265-003-0110-x
Hangarter, R. P., and Good, N. E. (1988). Active transport, ion movements, and pH changes: II. Changes of pH and ATP synthesis. Photosynth. Res. 19, 237–250. doi: 10.1007/BF00046876
Haruta, M., Burch, H. L., Nelson, R. B., Barrett-Wilt, G., Kline, K. G., Mohsin, S. B., et al. (2010). Molecular characterization of mutant Arabidopsis plants with reduced plasma membrane proton pump activity. J. Biol. Chem. 285, 17918–17929. doi: 10.1074/jbc.M110.101733
Haruta, M., Gray, W. M., and Sussman, M. R. (2015). Regulation of the plasma membrane proton pump (H(+)-ATPase) by phosphorylation. Curr. Opin. Plant Biol. 28, 68–75. doi: 10.1016/j.pbi.2015.09.005
Haruta, M., Sabat, G., Stecker, K., Minkoff, B. B., and Sussman, M. R. (2014). A peptide hormone and its receptor protein kinase regulate plant cell expansion. Science 343, 408–411. doi: 10.1126/science.1244454
Haruta, M., and Sussman, M. R. (2012). The effect of a genetically reduced plasma membrane protonmotive force on vegetative growth of Arabidopsis. Plant Physiol. 158, 1158–71. doi: 10.1104/pp.111.189167
Hayashi, Y., Takahashi, K., Inoue, S.-I., and Kinoshita, T. (2014). Abscisic acid suppresses hypocotyl elongation by dephosphorylating plasma membrane H(+)-ATPase in Arabidopsis thaliana. Plant Cell Physiol. 55, 845–853. doi: 10.1093/pcp/pcu028
Hinsinger, P., Plassard, C., Tang, C., and Jaillard, B. (2003). Origins of root-mediated pH changes in the rhizosphere and their responses to environmental constraints: a review. Plant Soil 248, 43–59. doi: 10.1023/A:1022371130939
Hocq, L., Pelloux, J., and Lefebvre, V. (2017). Connecting Homogalacturonan-Type pectin remodeling to acid growth. Trends Plant Sci. 22, 20–29. doi: 10.1016/j.tplants.2016.10.009
Hoffmann, R. D., Olsen, L. I., Ezike, C. V., Pedersen, J. T., Manstretta, R., López-Marqués, R. L., et al. (2019). Roles of plasma membrane proton ATPases AHA2 and AHA7 in normal growth of roots and root hairs in Arabidopsis thaliana. Physiol. Plant. 166, 848–861. doi: 10.1111/ppl.12842
Janicka-Russak, M., and Kłobus, G. (2007). Modification of plasma membrane and vacuolar H+ -ATPases in response to NaCL and ABA. J. Plant Physiol. 164, 295–302. doi: 10.1016/j.jplph.2006.01.014
Janku, M., Luhová, L., and Petrivalský, M. (2019). On the origin and fate of reactive oxygen species in plant cell compartments. Antioxidants 8:105. doi: 10.3390/antiox8040105
Johns, S., Hagihara, T., Toyota, M., and Gilroy, S. (2021). The fast and the furious: rapid long-range signaling in plants. Plant Physiol. 185, 694–706. doi: 10.1093/plphys/kiaa098
Kader, M. A., and Lindberg, S. (2010). Cytosolic calcium and pH signaling in plants under salinity stress. Plant Signal. Behav. 5, 233–238. doi: 10.4161/psb.5.3.10740
Kesten, C., Gámez-Arjona, F. M., Menna, A., Scholl, S., Dora, S., Huerta, A. I., et al. (2019). Pathogen-induced pH changes regulate the growth-defense balance in plants. EMBO J. 38:e101822. doi: 10.15252/embj.2019101822
Kour, J., Kohli, S. K., Khanna, K., Bakshi, P., Sharma, P., Singh, A. D., et al. (2021). Brassinosteroid signaling, crosstalk and, physiological functions in plants under heavy metal stress. Front. Plant Sci. 12:608061. doi: 10.3389/fpls.2021.608061
Kumari, A., Chételat, A., Nguyen, C. T., and Farmer, E. E. (2019). Arabidopsis H+-ATPase AHA1 controls slow wave potential duration and wound-response jasmonate pathway activation. Proc. Natl. Acad. Sci. U S A. 116, 20226–20231. doi: 10.1073/pnas.1907379116
Lambers, H., Shane, M. W., Cramer, M. D., Pearse, S. J., and Veneklaas, E. J. (2006). Root structure and functioning for efficient acquisition of phosphorus: matching morphological and physiological traits. Ann. Bot. 98, 693–713. doi: 10.1093/aob/mcl114
Lanassa Bassukas, A. E., Xiao, Y., and Schwechheimer, C. (2022). Phosphorylation control of PIN auxin transporters. Curr. Opin. Plant Biol. 65:102146. doi: 10.1016/j.pbi.2021.102146
Li, G., Song, H., Li, B., Kronzucker, H. J., and Shi, W. (2015). Auxin Resistant1 and PIN-FORMED2 protect lateral root formation in Arabidopsis under iron stress. Plant Physiol. 169, 2608–2623. doi: 10.1104/pp.15.00904
Li, L., Verstraeten, I., Roosjen, M., Takahashi, K., Rodriguez, L., Merrin, J., et al. (2021). Cell surface and intracellular auxin signalling for H+ fluxes in root growth. Nature 599, 273–277. doi: 10.1038/s41586-021-04037-6
Li, S., Liu, Y., Wang, J., Yang, L., Zhang, S., Xu, C., et al. (2017). Soil acidification aggravates the occurrence of bacterial wilt in South China. Front. Microbiol. 8:703. doi: 10.3389/fmicb.2017.00703
Liang, C., Ma, Y., and Li, L. (2020). Comparison of plasma membrane H+-ATPase response to acid rain stress between rice and soybean. Environ. Sci. Pollut. Res. Int. 27, 6389–6400. doi: 10.1007/s11356-019-07285-2
Liang, C., Piñeros, M. A., Tian, J., Yao, Z., Sun, L., Liu, J., et al. (2013). Low pH, aluminum, and phosphorus coordinately regulate malate exudation through GmALMT1 to improve soybean adaptation to acid soils. Plant Physiol. 161, 1347–1361. doi: 10.1104/pp.112.208934
Lin, W., Zhou, X., Tang, W., Takahashi, K., Pan, X., Dai, J., et al. (2021). TMK-based cell-surface auxin signalling activates cell-wall acidification. Nature 599, 278–282. doi: 10.1038/s41586-021-03976-4
Liu, Y., Evans, S. E., Friesen, M. L., and Tiemann, L. K. (2022). Root exudates shift how N mineralization and N fixation contribute to the plant-available N supply in low fertility soils. Soil Biol. Biochem. 165:108541. doi: 10.1016/j.soilbio.2021.108541
Liu, Y., and von Wirén, N. (2017). Ammonium as a signal for physiological and morphological responses in plants. J. Exp. Bot. 68, 2581–2592. doi: 10.1093/jxb/erx086
Lomin, S. N., Krivosheev, D. M., Steklov, M. Y., Osolodkin, D. I., and Romanov, G. A. (2012). Receptor properties and features of cytokinin signaling. Acta Nat. 4, 31–45. doi: 10.32607/20758251-2012-4-3-31-45
Majda, M., and Robert, S. (2018). The role of auxin in cell wall expansion. Int. J. Mol. Sci. 19:951. doi: 10.3390/ijms19040951
Mangano, S., Martínez Pacheco, J., Marino-Buslje, C., and Estevez, J. M. (2018). How does pH fit in with oscillating polar growth? Trends Plant Sci. 23, 479–489. doi: 10.1016/j.tplants.2018.02.008
Martinière, A., Gibrat, R., Sentenac, H., Dumont, X., Gaillard, I., and Paris, N. (2018). Uncovering pH at both sides of the root plasma membrane interface using noninvasive imaging. Proc. Natl. Acad. Sci. U.S.A. 115, 6488–6493. doi: 10.1073/pnas.1721769115
Masachis, S., Segorbe, D., Turrà, D., Leon-Ruiz, M., Fürst, U., El Ghalid, M., et al. (2016). A fungal pathogen secretes plant alkalinizing peptides to increase infection. Nat. Microbiol. 1:10643. doi: 10.1038/nmicrobiol.2016.43
Mathur, J., and Hülskamp, M. (2001). Cell growth: how to grow and where to grow. Curr. Biol. 11, R402–R404. doi: 10.1016/S0960-9822(01)00219-6
Menna, A., Dora, S., Sancho-Andrés, G., Kashyap, A., Meena, M. K., Sklodowski, K., et al. (2021). A primary cell wall cellulose-dependent defense mechanism against vascular pathogens revealed by time-resolved dual transcriptomics. BMC Biol. 19:161. doi: 10.1186/s12915-021-01100-6
Meychik, N., Nikolaeva, Y., and Kushunina, M. (2021). The significance of ion-exchange properties of plant root cell walls for nutrient and water uptake by plants. Plant Physiol. Biochem. 166, 140–147. doi: 10.1016/j.plaphy.2021.05.048
Meychik, N. R., and Yermakov, I. P. (2001). Ion exchange properties of plant root cell walls. Plant Soil 234, 181–193. doi: 10.1023/A:1017936318435
Miao, R., Russinova, E., and Rodriguez, P. L. (2022). Tripartite hormonal regulation of plasma membrane H+-ATPase activity. Trends Plant Sci. 27, 588–600. doi: 10.1016/j.tplants.2021.12.011
Miao, R., Wang, M., Yuan, W., Ren, Y., Li, Y., Zhang, N., et al. (2018). Comparative analysis of Arabidopsis ecotypes reveals a role for brassinosteroids in root hydrotropism. Plant Physiol. 176, 2720–2736. doi: 10.1104/pp.17.01563
Miao, R., Yuan, W., Wang, Y., Garcia-Maquilon, I., Dang, X., Li, Y., et al. (2021). Low ABA concentration promotes root growth and hydrotropism through relief of ABA INSENSITIVE 1-mediated inhibition of plasma membrane H+-ATPase 2. Sci. Adv. 7:eabd4113. doi: 10.1126/sciadv.abd4113
Miedes, E., Vanholme, R., Boerjan, W., and Molina, A. (2014). The role of the secondary cell wall in plant resistance to pathogens. Front. Plant Sci. 5:358. doi: 10.3389/fpls.2014.00358
Minami, A., Takahashi, K., Inoue, S.-I., Tada, Y., and Kinoshita, T. (2019). Brassinosteroid induces phosphorylation of the plasma membrane H+-ATPase during Hypocotyl Elongation in Arabidopsis thaliana. Plant Cell Physiol. 60, 935–944. doi: 10.1093/pcp/pcz005
Minibayeva, F., Beckett, R. P., and Kranner, I. (2015). Roles of apoplastic peroxidases in plant response to wounding. Phytochemistry 112, 122–129. doi: 10.1016/j.phytochem.2014.06.008
Minjian, C., Haiqiu, Y., Hongkui, Y., and Chunji, J. (2007). Difference in tolerance to potassium deficiency between two maize inbred lines. Plant Prod. Sci. 10, 42–46. doi: 10.1626/pps.10.42
Molina, A., Miedes, E., Bacete, L., Rodríguez, T., Mélida, H., Denancé, N., et al. (2021). Arabidopsis cell wall composition determines disease resistance specificity and fitness. Proc. Natl. Acad. Sci. U.S.A. 118:e2010243118. doi: 10.1073/pnas.2010243118
Monshausen, G. B., Bibikova, T. N., Messerli, M. A., Shi, C., and Gilroy, S. (2007). Oscillations in extracellular pH and reactive oxygen species modulate tip growth of Arabidopsis root hairs. Proc. Natl. Acad. Sci. U.S.A. 104, 20996–21001. doi: 10.1073/pnas.0708586104
Monshausen, G. B., Bibikova, T. N., Weisenseel, M. H., and Gilroy, S. (2009). Ca2+ regulates reactive oxygen species production and pH during mechanosensing in Arabidopsis roots. Plant Cell 21, 2341–2356. doi: 10.1105/tpc.109.068395
Monshausen, G. B., Miller, N. D., Murphy, A. S., and Gilroy, S. (2011). Dynamics of auxin-dependent Ca2+ and pH signaling in root growth revealed by integrating high-resolution imaging with automated computer vision-based analysis. Plant J. 65, 309–318. doi: 10.1111/j.1365-313X.2010.04423.x
Montesinos, J. C., Abuzeineh, A., Kopf, A., Juanes-Garcia, A., Ötvös, K., Petrášek, J., et al. (2020). Phytohormone cytokinin guides microtubule dynamics during cell progression from proliferative to differentiated stage. EMBO J. 39:e104238. doi: 10.15252/embj.2019104238
Msimbira, L. A., and Smith, D. L. (2020). The roles of plant growth promoting microbes in enhancing plant tolerance to acidity and alkalinity stresses. Front. Sustain. Food Syst. 4:106. doi: 10.3389/fsufs.2020.00106
Murphy, E., and De Smet, I. (2014). Understanding the RALF family: a tale of many species. Trends Plant Sci. 19, 664–671. doi: 10.1016/j.tplants.2014.06.005
Nemhauser, J. L., Hong, F., and Chory, J. (2006). Different plant hormones regulate similar processes through largely nonoverlapping transcriptional responses. Cell 126, 467–475. doi: 10.1016/j.cell.2006.05.050
Odutola Oshunsanya, S. (2019). “Introductory chapter: Relevance of soil pH to agriculture,” in Soil pH for Nutrient Availability and Crop Performance (IntechOpen). doi: 10.5772/intechopen.82551
Oja, V. V, Savchenko, G., Jakob, B., and Heber, U. (1999). pH and buffer capacities of apoplastic and cytoplasmic cell compartments in leaves. Planta 209, 239–249. doi: 10.1007/s004250050628
Olivari, C., Pugliarello, M. C., Rasi-Caldogno, F., and De Michelis, M. I. (1993). Characteristics and regulatory properties of the H+-ATPase in a plasma membrane fraction purified from Arabidopsis thaliana. Bot. Acta 106, 13–19. doi: 10.1111/j.1438-8677.1993.tb00332.x
O'Neill, M. A., Ishii, T., Albersheim, P., and Darvill, A. G. (2004). Rhamnogalacturonan II: structure and function of a borate cross-linked cell wall pectic polysaccharide. Annu. Rev. Plant Biol. 55, 109–139. doi: 10.1146/annurev.arplant.55.031903.141750
Pace, C. N., Grimsley, G. R., and Scholtz, J. M. (2009). Protein ionizable groups: pK values and their contribution to protein stability and solubility. J. Biol. Chem. 284, 13285–13289. doi: 10.1074/jbc.R800080200
Pacifici, E., Di Mambro, R., Dello Ioio, R., Costantino, P., and Sabatini, S. (2018). Acidic cell elongation drives cell differentiation in the Arabidopsis root. EMBO J. 37:e99134. doi: 10.15252/embj.201899134
Pearce, G., Moura, D. S., Stratmann, J., and Ryan, C. A. Jr (2001). RALF, a 5-kDa ubiquitous polypeptide in plants, arrests root growth and development. Proc. Natl. Acad. Sci. U.S.A. 98, 12843–12847. doi: 10.1073/pnas.201416998
Phyo, P., Gu, Y., and Hong, M. (2019). Impact of acidic pH on plant cell wall polysaccharide structure and dynamics: insights into the mechanism of acid growth in plants from solid-state NMR. Cellulose 26, 291–304. doi: 10.1007/s10570-018-2094-7
Planes, M. D., Niñoles, R., Rubio, L., Bissoli, G., Bueso, E., García-Sánchez, M. J., et al. (2015). A mechanism of growth inhibition by abscisic acid in germinating seeds of Arabidopsis thaliana based on inhibition of plasma membrane H+-ATPase and decreased cytosolic pH, K+, and anions. J. Exp. Bot. 66, 813–825. doi: 10.1093/jxb/eru442
Pottosin, I., Velarde-Buendía, A. M., Bose, J., Zepeda-Jazo, I., Shabala, S., and Dobrovinskaya, O. (2014). Cross-talk between reactive oxygen species and polyamines in regulation of ion transport across the plasma membrane: implications for plant adaptive responses. J. Exp. Bot. 65, 1271–1283. doi: 10.1093/jxb/ert423
Pregitzer, K. S., Laskowski, M. J., Burton, A. J., Lessard, V. C., and Zak, D. R. (1998). Variation in sugar maple root respiration with root diameter and soil depth. Tree Physiol. 18, 665–670. doi: 10.1093/treephys/18.10.665
Pregitzer, K. S., Zak, D. R., Loya, W. M., Karberg, N. J., King, J. S., and Burton, A. J. (2007). “CHAPTER 7 - The Contribution of Root – Rhizosphere Interactions to Biogeochemical Cycles in a Changing World,” in The Rhizosphere, eds Z. G. Cardon and J. L. Whitbeck (Burlington: Academic Press), 155–178. doi: 10.1016/B978-012088775-0/50009-4
Rampon, C., Volovitch, M., Joliot, A., and Vriz, S. (2018). Hydrogen peroxide and redox regulation of developments. Antioxidants 7:159. doi: 10.3390/antiox7110159
Ratcliffe, R. G. (2003). Rengel, Z. (ed.) Handbook of plant growth. pH as the master variable. Ann. Bot. 92:165. doi: 10.1093/aob/mcg112
Roberts, S. K., and Snowman, B. N. (2000). The effects of ABA on channel-mediated K(+) transport across higher plant roots. J. Exp. Bot. 51, 1585–1594. doi: 10.1093/jexbot/51.350.1585
Rousk, J., Bååth, E., Brookes, P. C., Lauber, C. L., Lozupone, C., Caporaso, J. G., et al. (2010). Soil bacterial and fungal communities across a pH gradient in an arable soil. ISME J. 4, 1340–1351. doi: 10.1038/ismej.2010.58
Rousk, J., Brookes, P. C., and Bååth, E. (2009). Contrasting soil pH effects on fungal and bacterial growth suggest functional redundancy in carbon mineralization. Appl. Environ. Microbiol. 75, 1589–1596. doi: 10.1128/AEM.02775-08
Samalova, M., Gahurova, E., and Hejatko, J. (2022). Expansin-mediated developmental and adaptive responses: a matter of cell wall biomechanics? Quant. Plant Biol. 3:E11. doi: 10.1017/qpb.2022.6
Sampedro, J., and Cosgrove, D. J. (2005). The expansin superfamily. Genome Biol. 6:242. doi: 10.1186/gb-2005-6-12-242
Santi, S., and Schmidt, W. (2009). Dissecting iron deficiency-induced proton extrusion in Arabidopsis roots. N. Phytol. 183, 1072–1084. doi: 10.1111/j.1469-8137.2009.02908.x
Seifert, G. J., and Roberts, K. (2007). The biology of arabinogalactan proteins. Annu. Rev. Plant Biol. 58, 137–161. doi: 10.1146/annurev.arplant.58.032806.103801
Sentenac, H., and Grignon, C. (1981). A model for predicting ionic equilibrium concentrations in cell walls. Plant Physiol. 68, 415–419. doi: 10.1104/pp.68.2.415
Sentenac, H., and Grignon, C. (1987). Effect of H excretion on the surface pH of corn root cells evaluated by using weak acid influx as a pH probe. Plant Physiol. 84, 1367–1372. doi: 10.1104/pp.84.4.1367
Shao, Q., Gao, Q., Lhamo, D., Zhang, H., and Luan, S. (2020). Two glutamate- and pH-regulated Ca2+ channels are required for systemic wound signaling in Arabidopsis. Sci. Signal. 13:eaba1453. doi: 10.1126/scisignal.aba1453
Shao, Y., Feng, X., Nakahara, H., Irshad, M., Eneji, A. E., Zheng, Y., et al. (2021). Apical-root apoplastic acidification affects cell wall extensibility in wheat under salinity stress. Physiol. Plant. 173, 1850–1861. doi: 10.1111/ppl.13527
Shih, H.-W., DePew, C. L., Miller, N. D., and Monshausen, G. B. (2015). The cyclic nucleotide-gated channel CNGC14 regulates root gravitropism in Arabidopsis thaliana. Curr. Biol. 25, 3119–3125. doi: 10.1016/j.cub.2015.10.025
Shomer, I., Novacky, A. J., Pike, S. M., Yermiyahu, U., and Kinraide, T. B. (2003). Electrical potentials of plant cell walls in response to the ionic environment. Plant Physiol. 133, 411–422. doi: 10.1104/pp.103.024539
Siao, W., Coskun, D., Baluška, F., Kronzucker, H. J., and Xu, W. (2020). Root-apex proton fluxes at the centre of soil-stress acclimation. Trends Plant Sci. 25, 794–804. doi: 10.1016/j.tplants.2020.03.002
Simon-Plas, F., Elmayan, T., and Blein, J.-P. (2002). The plasma membrane oxidase NtrbohD is responsible for AOS production in elicited tobacco cells. Plant J. 31, 137–147. doi: 10.1046/j.1365-313X.2002.01342.x
Søgaard, R., Alsterfjord, M., Macaulay, N., and Zeuthen, T. (2009). Ammonium ion transport by the AMT/Rh homolog TaAMT1;1 is stimulated by acidic pH. Pflugers Arch. 458, 733–743. doi: 10.1007/s00424-009-0665-z
Spartz, A. K., Ren, H., Park, M. Y., Grandt, K. N., Lee, S. H., Murphy, A. S., et al. (2014). SAUR inhibition of PP2C-D phosphatases activates plasma membrane H+-ATPases to promote cell expansion in Arabidopsis. Plant Cell 26, 2129–2142. doi: 10.1105/tpc.114.126037
Srivastava, R., Liu, J.-X., Guo, H., Yin, Y., and Howell, S. H. (2009). Regulation and processing of a plant peptide hormone, AtRALF23, in Arabidopsis. Plant J. 59, 930–939. doi: 10.1111/j.1365-313X.2009.03926.x
Staal, M., De Cnodder, T., Simon, D., Vandenbussche, F., Van der Straeten, D., Verbelen, J.-P., et al. (2011). Apoplastic alkalinization is instrumental for the inhibition of cell elongation in the Arabidopsis root by the ethylene precursor 1-aminocyclopropane-1-carboxylic acid. Plant Physiol. 155, 2049–2055. doi: 10.1104/pp.110.168476
Sun, S., Gu, M., Cao, Y., Huang, X., Zhang, X., Ai, P., et al. (2012). A constitutive expressed phosphate transporter, OsPht1;1, modulates phosphate uptake and translocation in phosphate-replete rice. Plant Physiol. 159, 1571–1581. doi: 10.1104/pp.112.196345
Sze, H., and Chanroj, S. (2018). Plant endomembrane dynamics: studies of K+/H+ antiporters provide insights on the effects of pH and ion homeostasis. Plant Physiol. 177, 875–895. doi: 10.1104/pp.18.00142
Takahashi, K., Hayashi, K.-I., and Kinoshita, T. (2012). Auxin activates the plasma membrane H+-ATPase by phosphorylation during hypocotyl elongation in Arabidopsis. Plant Physiol. 159, 632–641. doi: 10.1104/pp.112.196428
Toyota, M., Spencer, D., Sawai-Toyota, S., Jiaqi, W., Zhang, T., Koo, A. J., et al. (2018). Glutamate triggers long-distance, calcium-based plant defense signaling. Science 361, 1112–1115. doi: 10.1126/science.aat7744
Tsai, H.-H., and Schmidt, W. (2021). The enigma of environmental pH sensing in plants. Nat. Plants 7, 106–115. doi: 10.1038/s41477-020-00831-8
Urano, K., Maruyama, K., Jikumaru, Y., Kamiya, Y., Yamaguchi-Shinozaki, K., and Shinozaki, K. (2017). Analysis of plant hormone profiles in response to moderate dehydration stress. Plant J. 90, 17–36. doi: 10.1111/tpj.13460
Vaahtera, L., Schulz, J., and Hamann, T. (2019). Cell wall integrity maintenance during plant development and interaction with the environment. Nat. Plants 5, 924–932. doi: 10.1038/s41477-019-0502-0
van Breemen, N. (1987). Effects of redox processes on soil acidity. NJAS 35, 271–279. doi: 10.18174/njas.v35i3.16724
Vančura, V., and Hovadík, A. (1965). Root exudates of plants: II. Composition of root exudates of some vegetables. Plant Soil 22, 21–32. doi: 10.1007/BF01377686
Vaseva, I. I., Qudeimat, E., Potuschak, T., Du, Y., Genschik, P., Vandenbussche, F., et al. (2018). The plant hormone ethylene restricts Arabidopsis growth via the epidermis. Proc. Natl. Acad. Sci. U S A. 115, E4130–E4139. doi: 10.1073/pnas.1717649115
Velasquez, S. M., Barbez, E., Kleine-Vehn, J., and Estevez, J. M. (2016). Auxin and cellular elongation. Plant Physiol. 170, 1206–1215. doi: 10.1104/pp.15.01863
Wang, X., Wang, Y., Piñeros, M. A., Wang, Z., Wang, W., Li, C., et al. (2014). Phosphate transporters OsPHT1;9 and OsPHT1;10 are involved in phosphate uptake in rice. Plant Cell Environ. 37, 1159–1170. doi: 10.1111/pce.12224
Wegner, L. H., Li, X., Zhang, J., Yu, M., Shabala, S., and Hao, Z. (2021). Biochemical and biophysical pH clamp controlling Net H+ efflux across the plasma membrane of plant cells. N. Phytol. 230, 408–415. doi: 10.1111/nph.17176
Wegner, L. H., and Shabala, S. (2020). Biochemical pH clamp: the forgotten resource in membrane bioenergetics. New Phytol. 225, 37–47. doi: 10.1111/nph.16094
Xu, W., Jia, L., Shi, W., Baluska, F., Kronzucker, H. J., Liang, J., et al. (2013). The Tomato 14-3-3 protein TFT4 modulates H+ efflux, basipetal auxin transport, and the PKS5-J3 pathway in the root growth response to alkaline stress. Plant Physiol. 163, 1817–1828. doi: 10.1104/pp.113.224758
Yang, L.-T., Qi, Y.-P., Jiang, H.-X., and Chen, L.-S. (2013). Roles of organic acid anion secretion in aluminium tolerance of higher plants. Biomed Res. Int. 2013:173682. doi: 10.1155/2013/173682
Yuan, W., Li, Y., Li, L., Siao, W., Zhang, Q., Zhang, Y., et al. (2018). BR-INSENSITIVE1 regulates hydrotropic response by interacting with plasma membrane H+-ATPases in Arabidopsis. Plant Signal. Behav. 13:e1486147. doi: 10.1080/15592324.2018.1486147
Zhang, J., Yu, H., Zhang, Y., Wang, Y., Li, M., Zhang, J., et al. (2016). Increased abscisic acid levels in transgenic maize overexpressing AtLOS5 mediated root ion fluxes and leaf water status under salt stress. J. Exp. Bot. 67, 1339–1355. doi: 10.1093/jxb/erv528
Zhang, M., Wang, Y., Chen, X., Xu, F., Ding, M., Ye, W., et al. (2021). Plasma membrane H+-ATPase overexpression increases rice yield via simultaneous enhancement of nutrient uptake and photosynthesis. Nat. Commun. 12:735. doi: 10.1038/s41467-021-20964-4
Zhou, J.-Y., Hao, D.-L., and Yang, G.-Z. (2021). Regulation of cytosolic pH: the contributions of plant plasma membrane H+-ATPases and multiple transporters. Int. J. Mol. Sci. 22:12998. doi: 10.3390/ijms222312998
Keywords: pH, apoplast, root, signaling, plants
Citation: Gámez-Arjona FM, Sánchez-Rodríguez C and Montesinos JC (2022) The root apoplastic pH as an integrator of plant signaling. Front. Plant Sci. 13:931979. doi: 10.3389/fpls.2022.931979
Received: 29 April 2022; Accepted: 26 July 2022;
Published: 23 August 2022.
Edited by:
Wenxiu Ye, Peking University, ChinaReviewed by:
Christoph Martin Geilfus, Humboldt University of Berlin, GermanyCopyright © 2022 Gámez-Arjona, Sánchez-Rodríguez and Montesinos. This is an open-access article distributed under the terms of the Creative Commons Attribution License (CC BY). The use, distribution or reproduction in other forums is permitted, provided the original author(s) and the copyright owner(s) are credited and that the original publication in this journal is cited, in accordance with accepted academic practice. No use, distribution or reproduction is permitted which does not comply with these terms.
*Correspondence: Juan Carlos Montesinos, am1vbnRlc2lub3NAZXRoei5jaA==; Francisco M. Gámez-Arjona, Z2FtZXphcmZAZXRoei5jaA==
†These authors share last authorship
Disclaimer: All claims expressed in this article are solely those of the authors and do not necessarily represent those of their affiliated organizations, or those of the publisher, the editors and the reviewers. Any product that may be evaluated in this article or claim that may be made by its manufacturer is not guaranteed or endorsed by the publisher.
Research integrity at Frontiers
Learn more about the work of our research integrity team to safeguard the quality of each article we publish.