- 1Boyce Thompson Institute for Plant Research, Ithaca, NY, United States
- 2Department of Plant Pathology and Plant-Microbe Biology, Cornell University, Ithaca, NY, United States
- 3Department of Biochemistry, Christopher S. Bond Life Sciences Center, University of Missouri, Columbia, MO, United States
Pseudokinases are thought to lack phosphotransfer activity due to altered canonical catalytic residues within their kinase domain. However, a subset of pseudokinases maintain activity through atypical phosphotransfer mechanisms. The Arabidopsis ILK1 is a pseudokinase from the Raf-like MAP3K family and is the only known plant pseudokinase with confirmed protein kinase activity. ILK1 activity promotes disease resistance and molecular pattern-induced root growth inhibition through its stabilization of the HAK5 potassium transporter with the calmodulin-like protein CML9. ILK1 also has a kinase-independent function in salt stress suggesting that it interacts with additional proteins. We determined that members of the ILK subfamily are the sole pseudokinases within the Raf-like MAP3K family and identified 179 novel putative ILK1 protein interactors. We also identified 70 novel peptide targets for ILK1, the majority of which were phosphorylated in the presence of Mn2+ instead of Mg2+ in line with modifications in ILK1’s DFG cofactor binding domain. Overall, the ILK1-targeted or interacting proteins included diverse protein types including transporters (HAK5, STP1), protein kinases (MEKK1, MEKK3), and a cytokinin receptor (AHK2). The expression of 31 genes encoding putative ILK1-interacting or phosphorylated proteins, including AHK2, were altered in the root and shoot in response to molecular patterns suggesting a role for these genes in immunity. We describe a potential role for ILK1 interactors in the context of cation-dependent immune signaling, highlighting the importance of K+ in MAMP responses. This work further supports the notion that ILK1 is an atypical kinase with an unusual cofactor dependence that may interact with multiple proteins in the cell.
Introduction
Protein kinases form the basis of the phosphorylation cascades that transmit cellular signals by facilitating phosphotransfer from ATP to serine, threonine, tyrosine, and histidine residues in a substrate protein or peptide (Kwon et al., 2019). The highly conserved eukaryotic kinase domain contains three catalytic residues essential for binding and positioning ATP in conjunction with metal ion cofactors such as Mn2+ and Mg2+ to facilitate phosphotransfer (Adams, 2001). Approximately 5% of all plant genes encode proteins containing a kinase domain, and at least one-third of plant proteins are phosphorylated (Kwon et al., 2019). Based on bioinformatic analyses, eukaryotic kinases have been divided into canonical (catalytically active) kinases and pseudokinases, which are usually catalytically inactive due to a non-canonical catalytic residue (Kwon et al., 2019). The catalytic residues include aspartate in the DFG subdomain that binds divalent cations to co-ordinate β and γ phosphates of ATP, the lysine in the VAIK subdomain that anchors and orients ATP for substrate transfer and the aspartate in the HRD domain of the catalytic loop that is a base acceptor to achieve proton transfer (Adams, 2001). Over 17% of predicted protein kinases encoded in the Arabidopsis genome are classified as peudokinases and recent research on plant pseudokinases confirm that they perform distinct biological functions in plant defense against microbial pathogens through establishing protein-protein interactions, as opposed to phosphotransfer (Kwon et al., 2019). For example, several kinase catalytic subdomains of the ZED1 pseudokinase influences its binding to PBL proteins and the ZAR1 nucleotide-binding leucine-rich repeat (NLR) protein during activation of effector-triggered immunity (ETI) (Bastedo et al., 2019). The BIR2 pseudokinase regulates microbial-associated molecular pattern (MAMP)-induced signaling by binding the BAK1 co-receptor to prevent its untimely interaction with the FLS2 receptor which is involved in perception of the flagellin-derived flg22 (Halter et al., 2014). The GHR1 receptor pseudokinase is involved in inducing stomatal closure following MAMP treatment through its interactions with the calcium-responsive CDPK3 (Hua et al., 2012; Sierla et al., 2018).
While a majority of pseudokinases lack phospho-transfer activity, a small subset maintain atypical kinase activity through catalytic residue substitutions with similar amino acids elsewhere in the catalytic site (Kwon et al., 2019). For example, some pseudokinases including CASK do not require a cofactor to initiate phosphotransfer indicating the divergence of their mechanisms from typical protein kinases (Mukherjee et al., 2010; Lewis et al., 2014; Kwon et al., 2019). The integrin-liked kinase 1 (ILK1) from Medicago and Arabidopsis are the only plant pseudokinases that are active kinases in vitro and in vivo with demonstrated auto- and substrate-phosphorylation activity (Chinchilla et al., 2008; Nemoto et al., 2011; Brauer et al., 2016). ILK1 belongs to the ILK family of Raf-like MAP3Ks found in both metazoans and plants though the nature of ILK catalytic residue degeneration is distinct between kingdoms (Ichimura et al., 2002; Popescu et al., 2017). In animals, the ILKs lack the catalytic aspartate in the HRD domain and have variable changes in the DFG domain (Popescu et al., 2017). Several studies indicate that the metazoan ILKs are inactive, though some evidence suggests that they maintain kinase activity in vitro (Dagnino, 2011; Hannigan et al., 2011). In the plant kingdom, many species encode both pseudokinase ILKs and ILKs with canonical kinase domains, though more ancient lineages including the green algae only encode non-pseudokinase ILKs (Popescu et al., 2017). For example, in Arabidopsis, ILK1, ILK2, and ILK3 encode pseudokinases while ILK4, ILK5, and ILK6 encode kinases. The plant pseudokinase ILKs have a common GFG motif in place of the DFG motif and some species encode ILKs that lack the catalytic aspartate in the HRD domain (Popescu et al., 2017). This suggests that degradation of the ILK kinase domains occurred independently in plants and animals and that the GFG motif is maintained across evolutionary time in plants, potentially due to a link with ILK function. However, it remains unclear how frequently these atypical catalytic residues occur in the closely related Raf-like MAP3K family members.
Previous work on pseudokinase ILKs from Arabidopsis (ILK1) and Medicago (MsILK1) indicate that they are able to phosphorylate substrates in vitro with an unusual preference for the Mn2+ cofactor compared to Mg2+ in keeping with their modified cofactor-binding domain (DFG) (Chinchilla et al., 2008; Brauer et al., 2016). While mutation of the aspartate residue in the HRD catalytic loop to asparagine eliminates kinase activity in most protein kinases, this mutation increased ILK1 activity further demonstrating the uniqueness of its enzymatic mechanism (Strong et al., 2011; Brauer et al., 2016). Ectopic expression of the Medicago ILK1 containing the same HRD- > HRN mutation in Arabidopsis reduced lateral root formation relative to the native Medicago ILK1, suggesting a role for MsILK1 in root development (Chinchilla et al., 2008). Complementation of the ilk1 knock out mutant in Arabidopsis with kinase-dead or kinase-active ILK1 revealed that ILK1 activity is essential for pattern-triggered root growth inhibition in response to flg22, pep1, and elf18 (Brauer et al., 2016; Popescu et al., 2017). Kinase function of the ILK1 pseudokinase as also required for resistance to the bacterial pathogen Pseudomonas syringae pv. tomato DC3000 in the leaf (Brauer et al., 2016; Popescu et al., 2017). Our previous proteomic screens using functional protein microarrays (FPM) and a kinase client assay (KiC) revealed seven transporters that interacted with ILK1, including the HAK5 potassium transporter that mediates potassium uptake under limiting conditions (Qi et al., 2008). Further confirmation of the ILK1-HAK5 interaction in vivo and functional analysis of the hak5-3 knock out mutant indicate that the role of ILK1 in defense and MAMP response seems to be partly dependent on its maintenance of HAK5 potassium transporter stability in conjunction with the calmodulin-like protein CML9 (Brauer et al., 2016). Indeed, MAMP treatment triggers bulk loss of K+, Mn2+ and accumulation of P3–, S2–, Zn2+, and Fe3+ in Arabidopsis seedlings, where K+ and Mn2+ are influenced by ILK1 expression (Brauer et al., 2016). Recent work indicates that ILK1 also promotes transcriptional changes of cell wall modifying enzymes in response to MAMPs as well as lignification of the root xylem which has previously been linked to maintenance of ion transport throughout the plant (Boyce et al., 2004; Dimlioglu et al., 2022). Kinase-independent functions for ILK1 include the promotion of germination during salt and osmotic stresses suggesting that much like the metazoan ILKs that scaffold multiple proteins to promote cell adhesion, ILK1 may also interact with more than one protein in vivo (Brauer et al., 2016; Chastney et al., 2021). Together, this suggests a role for ILK1 in maintaining ion homeostasis and that this regulation is essential for plant adaptive responses to biotic and abiotic stress.
Two non-pseudokinases ILK4 and ILK5 have overlapping functions with ILK1 in regulating root lignification, secondary root formation and root growth during osmotic stress or following MAMP treatment in Arabidopsis (Dimlioglu et al., 2022). It is unclear if this functional overlap is due to similar phosphorylation targets or protein interactors between the ILKs. Recent advances place ILK5 as a true MAP3K functioning downstream of the extracellular ATP receptor P2K1 to promote pattern-triggered responses (Kim D. et al., 2022). Ligand-activated P2K1 phosphorylates ILK5 which in turn initiates the MKK5-MPK3/MPK6 phosphorylation cascade to induce stomatal closure and resistance to P. syringae DC3000 (Kim D. et al., 2022). Thus, the ILKs may contribute to plant responses to multiple patterns through the endogenous ATP signaling pathway which is activated in response to wounding and abiotic stress (Matthius et al., 2019). Externally applied ATP inhibits root elongation and increases cell wall lignification potentially through elevation of auxin (Matthius et al., 2019). A similar mechanism regulates root growth inhibition in response to the pep1 endogenous pattern, where pep1 triggers altered distribution of auxin efflux carriers and auxin accumulation which induces premature cell differentiation and inhibited root growth (Jing et al., 2019). Whether ATP plays a role in pep1 or microbial pattern-induced root growth inhibition remains unclear.
Understanding the plant ILK interactions and phosphorylation patterns would expand our comprehension of the active pseudokinases and facilitate future work on modeling alternative active sites. While our previous work focused on transporter interactors of ILK1, we expand this approach in this work by using two unbiased in vitro proteomic screens to identify putative ILK1 interacting proteins. The importance of the Mn2+ or Mg2+ cofactors on ILK1 phosphorylation of target peptides is also evaluated to determine the relationship between substrate selectivity and cofactor binding for these atypical kinases.
Results
Putative ILK1 Phosphorylation Targets
Our previous survey of plant ILKs indicates the DFG- > GFG substitution in the catalytic site occurred prior to the divergence between the gymnosperms and the angiosperms (Popescu et al., 2017). We surveyed variation within the three essential catalytic residues to determine if similar substitutions exist across the Raf-like MAP3K family in Arabidopsis. The ILKs were the only members of the Raf-like MAP3Ks containing altered catalytic residues in the DFG, VAIK and HRD subdomains indicating that they are the only pseudokinases (Supplementary Table 1). This suggests that the GFG substitution within the ILK pseudokinase family members developed independently, likely from a canonical ILK kinase (Supplementary Table 1). The ILKs are also non-RD kinases (HRD- > HCD) and are overrepresented alongside the B4 subfamily as non-RD kinases within Raf-like MAP3K family (Supplementary Table 1). Non-RD kinases do not require activation loop phosphorylation to become active and can instead be activated through other conformational changes and autophosphorylation sites in ILK1 are indeed outside of activation loop (Nolan et al., 2004; Brauer et al., 2016).
To further characterize the unusual kinase activity of ILK1, we identified phosphorylation targets of ILK1 using the KiC assay. This approach involves incubation of a purified protein kinase with a synthetic peptide library comprised of approximately 2,100 peptides, representing over 4,000 in vivo, experimentally mapped phosphorylation sites, and detection of phosphorylated sites by mass spectrometry (Huang et al., 2010; Huang and Thelen, 2012; Ahsan et al., 2013). We identified 32 and 18 phosphorylated peptides using either Mn2+ or Mg2+ as cofactors, respectively (Figure 1, Supplementary Tables 2, 3, and Supplementary Data 1). Four peptides were phosphorylated in both conditions, including one derived from a pectin methylesterase involved in cell wall-mediated defense (PME39, AT4G02300), the BYPASS1 protein which is required for root responses to Rhizobium infection, an ankyrin repeat protein (AT3G04470) and an RNA helicase family protein (AT1G08050) that is oxidized following flg22 or salicylic acid treatments (Bethke et al., 2009; Liu et al., 2015; Arthikala et al., 2018). The majority of the ILK1 peptide targets were localized to the nucleus or plasma membrane, consistent with previous observations that ILK1 can be found in the plasma membrane, the endoplasmic reticulum, and the nucleus (Brauer et al., 2016; Supplementary Figure 1A and Supplementary Data 1). Applying the KiC approach using the hyperactive ILK1D319N isoform revealed 26 and 16 phosphorylated peptides with Mn2+ or Mg2+, respectively, 15 of which were also identified as phosphorylated by ILK1. Altogether, 70 peptide targets were phosphorylated by ILK1 and ILK1D319N including transporters, protein kinases, enzymes involved in metabolite biosynthesis, and scaffold proteins (Supplementary Figure 1B). Notable potential targets included the HAK5 transporter which was confirmed as an ILK1-interacting protein previously, MEKK1, which promotes basal defense downstream of PRRs and MEKK7, which interacts with the FLS2 PRR to suppress flg22-induced immune responses (Brauer et al., 2016; Mithoe et al., 2016; Sun and Zhang, 2022).
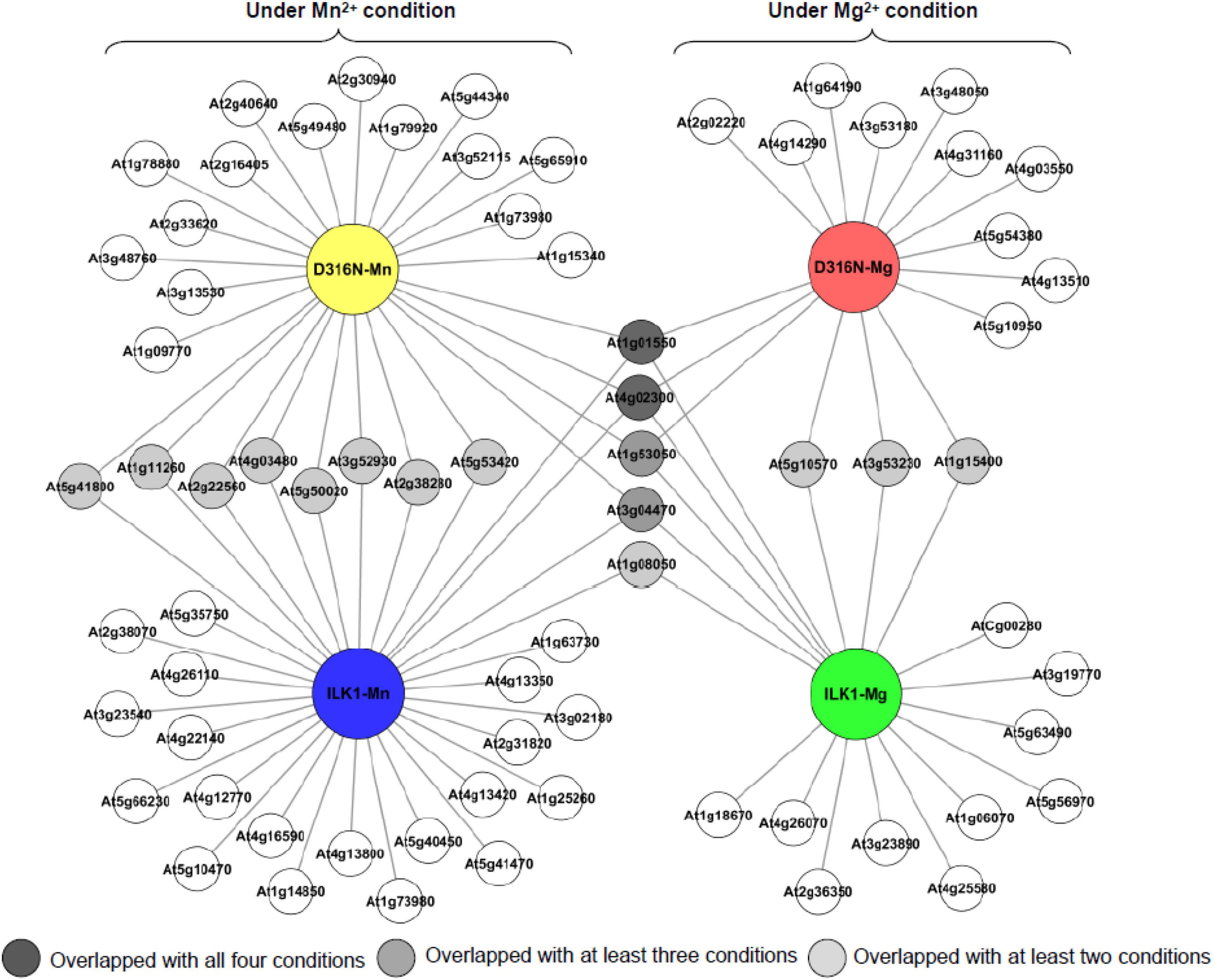
Figure 1. Topological relationship of the potential phosphorylation targets for ILK1 and the ILKD316N hyperactive mutant identified by screening the phosphorylation status of a synthetic peptide library after incubation with purified ILK1 protein. Cofactor conditions included the use of either 5 mM MgCl2 or MnCl2. The cartograph was assembled by Cytoscape 2.8.3 (http://www.cytoscape.org).
In general, both ILK1 and ILK1D319N kinases phosphorylated primarily serine and threonine residues, but tyrosine phosphorylation was also recorded on a small subset of peptides, including one derived from the HAK5 transporter (Supplementary Tables 2, 3). A higher number of targets was observed with Mn2+ as a cofactor compared to Mg2+ which is interesting considering that Mg2+ is preferred by most serine/threonine protein kinases while tyrosine kinases seem to prefer Mn2+ as a cofactor (Swarup et al., 1984; Bossemeyer et al., 1993). Thus, ILK1 appears to be an exception to these trends, being primarily a serine/threonine kinase with a preference for the Mn2+ cofactor.
Putative ILK1 Protein Interactors
To understand the protein-protein interaction specificity of ILK1, we surveyed half (15,000) of the predicted Arabidopsis proteome using functional protein microarrays to identify 179 putative interactors of our purified ILK1-V5 protein (Supplementary Data 2). Interactors were identified across three replicate arrays by subtracting the V5 signal from the background and normalizing to positive and negative control spots on the chip as described previously and identifying spots with significantly higher signal compared to control slides (Brauer et al., 2014). Most of the interactors localize to the nucleus or the cytosol, and are involved in metabolite conversion, protein modification, or transcriptional regulation (Supplementary Figure 1 and Supplementary Data 2). Interactors within the MAPK family included the MEKK3 that promotes MTI and signal transduction downstream of PRR activation (Sun and Zhang, 2022). We also identified MEKK19, and MPK19 as potential interactors, though the function of these kinases is unknown. Several transporters were also identified as potential ILK1 interactors including the KUP5 potassium transporter which is closely related to HAK5, and is expressed across diverse root cell types (Lhamo and Luan, 2021). This indicates that ILK1 can interact with a wide range of protein types in vitro, supporting the notion that additional ILK1-protein interactions beyond the CML9 and HAK5 proteins may exist in plant cells.
Trends Across Putative ILK1 Protein Interactors and Substrates
Overall, we identified 249 peptides or proteins which interacted with purified ILK1 in vitro and are not significantly enriched for specific biological or molecular functions. Interestingly, we did not identify the same ILK1 targets in both the KiC and functional protein microarray, though these approaches shared 709 peptides or fusion proteins derived from the same cognate protein (Popescu et al., 2007; Ahsan et al., 2013). Within the shared protein list, 28 ILK1-phosphorylated peptides had their cognate proteins spotted on the functional microarray but were not identified as ILK1-interacting proteins on the microarray. These included the HAK5 transporter that interacts with ILK1 in vivo, the MEKK1 immune signaling kinase, the STP1 sugar transporter, and the THE1 receptor kinase that detects cell wall modifications to promote resistance against necrotrophic pathogens (Brauer et al., 2016; Qu et al., 2017). This would suggest that protein microarrays may generate false negatives likely due to the influence of the interaction surface on the protein folding and interaction site availability. Indeed, previous analysis of protein microarray fidelity revealed false positive rates of 14% (Stiffler et al., 2006). Nevertheless the two assays give us complementary views of ILK1 as both an active kinase and protein scaffold potentially establishing protein-protein interactions through its ankyrin domain like the metazoan ILKs (Chastney et al., 2021). While further work is needed to confirm these interactions in vivo, combining proteomic approaches enabled us to assemble a calcium-responsive complex between CML9, ILK1, and HAK5 in addition to confirmed substrates for the P2K1 receptor kinase (Popescu et al., 2007; Brauer et al., 2016; Chen et al., 2017; Chen et al., 2021). Further, the genes transcribing 31 of the putative ILK1 interactors were transcriptionally responsive to MAMP treatments in roots or shoots suggesting a role in immunity (Figure 2 and Supplementary Table 4). These included three transporters, the cytokinin receptor AHK2 and the pectinesterase inhibitor PMEI4, both of which influence root growth (Hocq et al., 2017; Kubiasová et al., 2020). Thus, ILK1’s influence on MAMP-induced root growth inhibition may be linked to its effect on nutrient transport, cell wall modifications or cytokinin signaling and further functional work is needed to explore these possibilities.
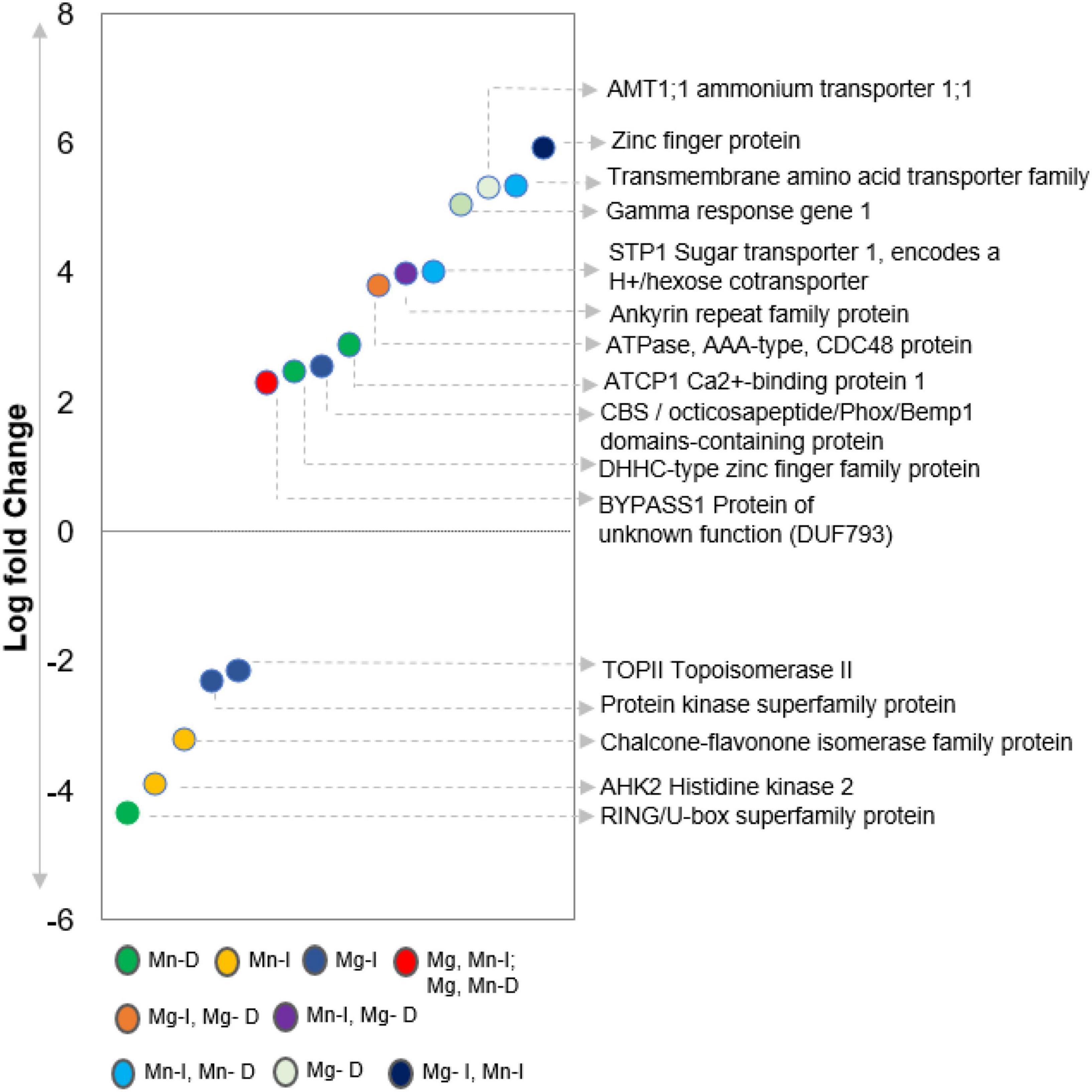
Figure 2. Differential expression of ILK1 targets in Arabidopsis leaves 3 h after 1 μM flg22 treatment relative to the 0 h time point (from Dimlioglu et al., 2022). All of the targets were identified by KiC in the presence of ILK1-V5 (I) of ILK1D319N-V5 (D) purified proteins with MnCl2 (Mn) or MgCl2 (Mg) as cofactors.
Discussion
Here, we describe the putative protein-protein interactions and phosphorylation activity of the atypical protein kinase ILK1. Members of the ILK family were the only pseudokinases identified within the Raf-like MAP3Ks and the ILK1 is one of the only pseudokinases in plants with demonstrated phosphotransfer activity demonstrated in vitro and in vivo (Brauer et al., 2016). In line with its unusual metal ion cofactor-binding domain, we demonstrate that ILK1 phosphorylates a broader range of peptides in the presence of Mn2+ compared to Mg2+ but that it primarily phosphorylates serine and threonine residues. Our survey of ILK1 protein interactors revealed interactions with diverse proteins, including transporters, protein kinases, cell wall modifying enzymes, and hormone receptors. Together, this indicates that ILK1 is an active kinase with a potentially broad range of interactions with diverse substrates. While its kinase-dependent role in MAMP-induced growth and defense responses seems to align with its regulation of the HAK5 potassium transporter, we cannot exclude the possibility that additional phosphorylation targets of ILK1 may exist in the cell. Indeed, it is increasingly apparent that protein kinases interact with a wide range of proteins in vitro and in vivo, suggesting that the functional specificity of kinases is related to their localization, protein complexing, and posttranslational modifications as opposed to interaction specificities (Popescu et al., 2009; Brauer et al., 2018).
Root growth is influenced by both potassium and MAMPs through ILK1 and HAK5 indicating shared components of potassium and immune signaling pathways (Qi et al., 2008; Brauer et al., 2016). Blocking K+ channels abolishes flg22-induced root growth inhibition which is counterintuitive if the function of K+ in this process is strictly to promote nutrition (Brauer et al., 2016; Shabala, 2017). The importance of potassium in defense is further underlined by the fact that the rice blast pathogen Magnaporthe oryzae targets the AKT1 potassium channel using the AvrPiz-t effector to promote virulence during infection (Shi et al., 2018). However, it remains unclear if the role of K+ in plant defense is due to a direct influence on signaling or if these observations reflect a broader scope of changes in ion homeostasis and their impact on immunity as K+ influences transport of diverse nutrients and influences membrane charge and fluidity (Nieves-Cordones et al., 2019). MAMP exposure induces K+ and Mn2+ loss and accumulation of P3– S2–, Zn2+, and Fe3+ in Arabidopsis seedlings within minutes of flg22 application though this shift is transient since Fe3+ accumulation returns to control levels by 6 h (Brauer et al., 2016; Nobori et al., 2018). Indeed, suppression of phosphate transport through the PHT1;4 uptake transporter in roots is regulated downstream of MAMP recognition by BIK1 and PBL1 receptor-like cytosolic kinases and influences infection by Ralstonia solanacearum (Dindas et al., 2022). On the pathogen side, P. syringae DC3000 sulfur starvation responses are induced when plants are pre-treated with flg22 indicating that bacteria may sense the nutritional shift in plants during immune activation (Lovelace et al., 2018). The pH of the plant rhizosphere is manipulated by bacterial release of gluconic acid and influenced by bacterial amino acid synthesis, which has a direct impact on root defense responses and microbiome profiles (Yu et al., 2019; Liu et al., 2021). This indicates the dynamic interplay between plant nutrition status and plant-microbe interactions.
At the cellular level, MAMP recognition induces transient influx of Ca2+, H+, and efflux of K+, Cl–, and NO3– (Jeworutzki et al., 2010). Cation transport contributes to the rate but not the extent of membrane depolarization which is primarily mediated by anions (Jabs et al., 1997; Jeworutzki et al., 2010; Brauer et al., 2016). Cation fluxes through La3+-sensitive channels are essential to trigger MAMP-triggered plasma membrane depolarization and defense compound production (Jabs et al., 1997; Jeworutzki et al., 2010). While these effects are attributed to Ca2+-depending signaling, La3+ blocks both Ca2+ and K+ channels in plants and thus a more nuanced understanding of the relationship between cation fluxes and immune signaling is warranted (Terry et al., 1992). For example, K+ levels influence the sensitivity of the plasma membrane to flg22, potentially through its influence on membrane polarity (Chi et al., 2021). Mn2+ homeostasis also changes in response to flg22 which could impact cofactor availability for ILK1 as well as tyrosine phosphorylation of pattern recognition receptors such as the lipid MAMP-binding LORE receptor and the elf18-binding EFR receptor (Macho et al., 2015; Luo et al., 2020). In mammals, cation leakage from pore-forming toxins or atypical cation pore formation is detected by the cell to induce MAPK signaling (Kloft et al., 2009; Olabisi et al., 2016). In plants, recent work indicates that the NB-LRRs form cation-conducting channels in the plasma membrane upon avirulence factor recognition to activate ETI (Kim N.H. et al., 2022). These resistosome channels can conduct calcium, but may also conduct other cations (Kim N.H. et al., 2022). The signaling mechanisms connecting cation efflux with downstream activation of ETI signaling remains unclear but could involve similar mechanisms as PTI. For example, cation-induced changes in plasma membrane polarity influences the voltage-regulated vacuolar two-pore channels and potassium channels including GORK and SKOR (Kurusu et al., 2005; Wu et al., 2018). Membrane polarity also influences membrane fluidity and the microdomains in which immune signaling proteins are recruited and thus disruption of these microdomains or protein interactions therein could trigger signaling pathways leading to defense induction (Malinsky et al., 2016). Alternatively, cations movement may be sensed directly as has been recently demonstrated during salt stress, where GIPC sphingolipids bind Na+ ions to activate Ca2+ influx channels and downstream signaling pathways regulating root growth (Jiang et al., 2019). Our understanding of the influence of cation fluxes on inducible immunity remains unclear, but the recent breakthroughs in our understanding of resistosomes will no doubt advance our progress in this area.
This work improves our understanding of the atypical protein kinases and reinforces the notion that functionally relevant alternative protein kinase mechanisms exist. Further characterization of the ILK family and their role as either scaffolding proteins or protein kinases will help to illuminate the function of these pseudokinases in plant defense and stress responses.
Methods
Protein Purification and Protein Microarray
The ILK1-V5 and ILK1D319N-V5 proteins were purified as described previously and protein purity was validated by mass spectrometry indicating a lack of background kinases within the samples (Brauer et al., 2014, 2016). Both Mn2+ and Mg2+ (5 mM) were included during ILK1 incubation on the arrays as cofactors and the experiment was conducted in triplicate as described in Brauer et al. (2016). Briefly, protein microarrays (AtPMA-5000 and AtPMA-10000) were blocked in TBS-T [20 mM Tris, 137 mM NaCl, 0.1% (v/v) Tween-20) with 1% (w/v) BSA for 1 h, drained, and kept in a humid chamber. 150 μl of purified ILK1 or cleavage buffer (both mixed with 1% (w/v) BSA and 5 mM MgCl2 and MnCl2) were applied to the slides for 1.5 h. Slides were washed in washing buffer (PBS, 5 mM MgCl2, and MnCl2, 0.05% (v/v) Triton X-100, 5% (v/v) glycerol, 1% (w/v) BSA], incubated with 500 μl Cy5-conjugated anti-V5 antibody (1:8,000, Agrisera) for 30 min, washed and incubated with 500 μl α-rabbit-DyLight649 (1:10,000, Jackson Immuno Research) for 30 min and washed with the same buffer once again. Slides were dipped in water, spun-dried, and scanned in a Scanarray® express scanner (Perkin Elmer); images were processed using GenePix® Pro 7 Acquisition and Analysis Software (Molecular Devices) as described in Popescu et al. (2009). We performed three experiments using ILK1 as a probe and two experiments without probe as a control.
Protein Microarray Bioinformatics
To analyze candidates with stronger binding signals than the control we implemented in Matlab a cross-array normalization method followed by a statistical testing method. All microarrays (probe and control) datasets were normalized using a linear regression model as follows: (1) background median intensity was subtracted from signal median intensity; (2) linear regression coefficients for each probe and control pairs were calculated: , where N is the number of proteins printed on the microarray; 3) “between arrays” normalization was performed using a linear transformation of probe datasets (using the estimated regression coefficients) and scaling of control datasets: , / , j = 1..M, the number of (probe, control) pair datasets. To select probe binding candidates we used a one-side t-test: , where is the standard error of the difference between the means; using pooled variance and 5% significance level (due to the small sample set). We controlled type II errors by applying an FDR method (Storey et al., 2002).
Kinase Client Assay
The same ILK1 purified proteins that were used for the protein microarray were also used for the KiC as described previously (Ahsan et al., 2013; Brauer et al., 2016). Peptide spectral matches were evaluated primarily using the XCorr scoring function of SEQUEST, employing a 1% false discovery rate for a randomized database of the peptide library. Phosphorylation-site localization was performed using phosphoRS (pRS) (Proteome Discoverer, v1.0.3, Thermo Fisher). An empty vector was used as a control against the 2.1k peptide library, peptides that overlapped with the empty vector control were excluded as potential clients, as these likely represented “background” kinase activities as described in Brauer et al. (2016).
Annotation and Enrichment Analysis
To determine functional enrichment for ILK1 interactors or ILK1 phosphorylation targets within a dataset, the PANTHER GO Term Enrichment1 was used with a cut-off value of p < 0.05.
Enrichment was measured against both the entire Arabidopsis genome as the background or using the genes specifically present in the KiC library or protein microarray.
Data Availability Statement
The original contributions presented in this study are included in the article/Supplementary Material, further inquiries can be directed to the corresponding author/s.
Author Contributions
EB and SP conceived of the study and designed the experiments. EB and NA conducted the proteomic experiments. EB, NA, and GP analyzed the data. EB wrote the manuscript. All authors interpreted the data and contributed to writing the manuscript.
Funding
This work was supported by a post-graduate fellowship to EB from the National Science and Engineering Research Council of Canada and by the National Science Foundation under project grants no. IOS-1025642 to SP and no. IOS-2048410 to JT. The USDA-National Institute of Food and Agriculture Hatch project MIS-145120 and 043040 and the Mississippi Agricultural & Forestry Experiment Station to SP provided funding for this work. GP acknowledges the support from the USDA-Agricultural Research Unit through the Big Data: Biocomputing, Bioinformatics, and Biological Discovery project 6066-21310-004-25S.
Conflict of Interest
The authors declare that the research was conducted in the absence of any commercial or financial relationships that could be construed as a potential conflict of interest.
Publisher’s Note
All claims expressed in this article are solely those of the authors and do not necessarily represent those of their affiliated organizations, or those of the publisher, the editors and the reviewers. Any product that may be evaluated in this article, or claim that may be made by its manufacturer, is not guaranteed or endorsed by the publisher.
Supplementary Material
The Supplementary Material for this article can be found online at: https://www.frontiersin.org/articles/10.3389/fpls.2022.931324/full#supplementary-material
Footnotes
References
Adams, J. A. (2001). Kinetic and catalytic mechanisms of protein kinases. Chem. Rev. 101, 2271–2290.
Ahsan, N., Huang, Y., Tovar-Mendez, A., Swatek, K. N., Zhang, J., Miernyk, J. A., et al. (2013). A versatile mass spectrometry-based method to both identify kinase client-relationships and characterize signaling network topology. J. Proteome Res. 12, 937–948. doi: 10.1021/pr3009995
Arthikala, M.-K., Nanjareddy, K., and Lara, M. (2018). In BPS1 downregulated roots, the BYPASS1 signal disrupts the induction of cortical cell divisions in bean-Rhizobium symbiosis. Genes 9:11. doi: 10.3390/genes9010011
Bastedo, D. P., Khan, M., Martel, A., Seto, D., Kireeva, I., Zhang, J., et al. (2019). Perturbations of the ZED1 pseudokinase activate plant immunity. PLoS Pathog. 15:e1007900. doi: 10.1371/journal.ppat.1007900
Bethke, G., Unthan, T., Uhrig, J. F., Pöschl, Y., Gust, A. A., Scheel, D., et al. (2009). Flg22 regulates the release of an ethylene response factor substrate from MAP kinase 6 in Arabidopsis thaliana via ethylene signaling. Proc. Natl. Acad. Sci. U.S.A. 106, 8067–8072. doi: 10.1073/pnas.0810206106
Bossemeyer, D., Engh, R. A., Kinzel, V., Ponstingl, H., and Huber, R. (1993). Phosphotransferase and substrate binding mechanism of the cAMP-dependent protein kinase catalytic subunit from porcine heart as deduced from the 2.0 A structure of the complex with Mn2+ adenylyl imidodiphosphate and inhibitor peptide PKI(5-24). EMBO J. 12, 849–859. doi: 10.1002/j.1460-2075.1993.tb05725.x
Boyce, C. K., Zwieniecki, M. A., Cody, G. D., Jacobsen, C., Wirick, S., Knoll, A. H., et al. (2004). Evolution of xylem lignification and hydrogel transport regulation. Proc. Natl. Acad. Sci. U.S.A. 101, 17555–17558. doi: 10.1073/pnas.0408024101
Brauer, E. K., Ahsan, N., Dale, R., Kato, N., Coluccio, A. E., Piñeros, M. A., et al. (2016). The Raf-like kinase ILK1 and the high affinity K+ transporter HAK5 are required for innate immunity and abiotic stress response. Plant Physiol. 171, 1470–1484. doi: 10.1104/pp.16.00035
Brauer, E. K., Popescu, G. V., Singh, D. K., Calviño, M., Gupta, K., Gupta, B., et al. (2018). Integrative network-centric approach reveals signaling pathways associated with plant resistance and susceptibility to Pseudomonas syringae. PLoS Biol. 16:e2005956. doi: 10.1371/journal.pbio.2005956
Brauer, E., Popescu, S., and Popescu, G. (2014). “Experimental and analytical approaches to characterize plant kinases using protein microarrays,” in Plant MAP Kinases. Methods in Molecular Biology, Vol. 1171, eds G. Komis and J. Šamaj (New York, NY: Springer), 217–235. doi: 10.1007/978-1-4939-0922-3_17
Chastney, M. R., Conway, J. R. W., and Ivaska, J. (2021). Integrin adhesion complexes. Curr. Biol. 31, R536–R542.
Chen, D., Cao, Y., Li, H., Kim, D., Ahsan, N., Thelen, J., et al. (2017). Extracellular ATP elicits DORN1-mediated RBOHD phosphorylation to regulate stomatal aperture. Nat. Commun. 8:2265. doi: 10.1038/s41467-017-02340-3
Chen, D., Hao, F., Mu, H., Ahsan, N., Thelen, J. J., and Stacey, G. (2021). S-acylation of P2K1 mediates extracellular ATP-induced immune signaling in Arabidopsis. Nat. Commun. 12:2750. doi: 10.1038/s41467-021-22854-1
Chi, Y., Wang, C., Wang, M., Wan, D., Huang, F., Jiang, Z., et al. (2021). Flg22-induced Ca2+ increases undergo desensitization and resensitization. Plant. Cell Environ. 44, 3793–3805. doi: 10.1111/pce.14186
Chinchilla, D., Frugier, F., Raices, M., Merchan, F., Giammaria, V., Gargantini, P., et al. (2008). A mutant ankyrin protein kinase from Medicago sativa affects Arabidopsis adventitious roots. Funct. Plant Biol. 35, 92–101. doi: 10.1071/FP07209
Dagnino, L. (2011). Integrin-linked kinase: a Scaffold protein unique among its ilk. J. Cell Commun. Signal. 5, 81–83. doi: 10.1007/s12079-011-0124-4
Dimlioglu, G., Nejat, N., Cooley, E., Srivastava, H., Stevens, J., Bokros, N., et al. (2022). Integrin-linked kinases 1, 4, and 5 converge to regulate the cell wall integrity and innate immunity to leaf and root pathogens. Plant Science
Dindas, J., DeFalco, T. A., Yu, G., Zhang, L., David, P., Bjornson, M., et al. (2022). Direct inhibition of phosphate transport by immune signaling in Arabidopsis. Curr. Biol. 32, 488–495. doi: 10.1016/j.cub.2021.11.063
Halter, T., Imkampe, J., Mazzotta, S., Wierzba, M., Postel, S., Bücherl, C., et al. (2014). The leucine-rich repeat receptor kinase BIR2 is a negative regulator of BAK1 in plant immunity. Curr. Biol. 24, 134–143. doi: 10.1016/j.cub.2013.11.047
Hannigan, G. E., McDonald, P. C., Walsh, M. P., and Dedhar, S. (2011). Integrin-linked kinase: not so ‘pseudo’after all. Oncogene 30, 4375–4385. doi: 10.1038/onc.2011.177
Hocq, L., Sénéchal, F., Lefebvre, V., Lehner, A., Domon, J.-M., Mollet, J.-C., et al. (2017). Combined experimental and computational approaches reveal distinct pH dependence of pectin methylesterase inhibitors. Plant Physiol. 173, 1075–1093. doi: 10.1104/pp.16.01790
Hua, D., Wang, C., He, J., Liao, H., Duan, Y., Zhu, Z., et al. (2012). A plasma membrane receptor kinase, GHR1, mediates abscisic acid-and hydrogen peroxide-regulated stomatal movement in Arabidopsis. Plant Cell 24, 2546–2561. doi: 10.1105/tpc.112.100107
Huang, Y., and Thelen, J. J. (2012). KiC assay: a quantitative mass spectrometry-based approach for kinase client screening and activity analysis. Methods Mol. Biol. 893, 359–370. doi: 10.1007/978-1-61779-885-6_22
Huang, Y., Houston, N. L., Tovar-Mendez, A., Stevenson, S. E., Miernyk, J. A., Randall, D. D., et al. (2010). A quantitative mass spectrometry-based approach for identifying protein kinase –clients and quantifying kinase activity. Anal. Biochem. 402, 69–76. doi: 10.1016/j.ab.2010.03.028
Ichimura, K., Shinozaki, K., Tena, G., Sheen, J., Henry, Y., Champion, A., et al. (2002). Mitogen-activated protein kinase cascades in plants: a new nomenclature. Trends Plant Sci. 7, 301–308. doi: 10.1016/s1360-1385(02)02302-6
Jabs, T., Tschöpe, M., Colling, C., Hahlbrock, K., and Scheel, D. (1997). Elicitor-stimulated ion fluxes and O2– from the oxidative burst are essential components in triggering defense gene activation and phytoalexin synthesis in parsley. Proc. Natl. Acad. Sci. U.S.A. 94, 4800–4805. doi: 10.1073/pnas.94.9.4800
Jeworutzki, E., Roelfsema, M. R. G., Anschütz, U., Krol, E., Elzenga, J. T. M., Felix, G., et al. (2010). Early signaling through the Arabidopsis pattern recognition receptors FLS2 and EFR involves Ca2+-associated opening of plasma membrane anion channels. Plant J. 62, 367–378. doi: 10.1111/j.1365-313X.2010.04155.x
Jiang, Z., Zhou, X., Tao, M., Yuan, F., Liu, L., Wu, F., et al. (2019). Plant cell-surface GIPC sphingolipids sense salt to trigger Ca2+ influx. Nature 572, 341–346. doi: 10.1038/s41586-019-1449-z
Jing, Y., Zheng, X., Zhang, D., Shen, N., Wang, Y., Yang, L., et al. (2019). Danger-associated peptides interact with PIN-dependent local auxin distribution to inhibit root growth in Arabidopsis. Plant Cell 31, 1767–1787. doi: 10.1105/tpc.18.00757
Kim, D., Chen, D., Ahsan, N., Thelen, J. J., and Stacey, G. (2022). The mitogen-activated protein kinase kinase kinase, ILK5, regulates plant purinergic receptor-mediated, innate immunity. bioRxiv [Preprint] doi: 10.1101/2022.04.19.488815
Kim, N. H., Jacob, P., and Dangl, J. L. (2022). Con-Ca2+-tenating plant immune responses via calcium-permeable cation channels. New Phytol. 234, 813–818. doi: 10.1111/nph.18044
Kloft, N., Busch, T., Neukirch, C., Weis, S., Boukhallouk, F., Bobkiewicz, W., et al. (2009). Pore-forming toxins activate MAPK p38 by causing loss of cellular potassium. Biochem. Biophys. Res. Commun. 385, 503–506. doi: 10.1016/j.bbrc.2009.05.121
Kubiasová, K., Montesinos, J. C., Šamajová, O., Nisler, J., Mik, V., Semerádová, H., et al. (2020). Cytokinin fluoroprobe reveals multiple sites of cytokinin perception at plasma membrane and endoplasmic reticulum. Nat. Commun. 11:4285. doi: 10.1038/s41467-020-17949-0
Kurusu, T., Yagala, T., Miyao, A., Hirochika, H., and Kuchitsu, K. (2005). Identification of a putative voltage-gated Ca2+ channel as a key regulator of elicitor-induced hypersensitive cell death and mitogen-activated protein kinase activation in rice. Plant J. 42, 798–809. doi: 10.1111/j.1365-313X.2005.02415.x
Kwon, A., Scott, S., Taujale, R., Yeung, W., Kochut, K. J., Eyers, P. A., et al. (2019). Tracing the origin and evolution of pseudokinases across the tree of life. Sci. Signal. 12:eaav3810. doi: 10.1126/scisignal.aav3810
Lewis, J. D., Lo, T., Bastedo, P., Guttman, D. S., and Desveaux, D. (2014). The rise of the undead: pseudokinases as mediators of effector-triggered immunity. Plant Signal. Behav. 9, 18722–18727. doi: 10.4161/psb.27563
Lhamo, D., and Luan, S. (2021). Potential networks of nitrogen-phosphorus-potassium channels and transporters in Arabidopsis roots at a single cell resolution. Front. Plant Sci. 12:689545. doi: 10.3389/fpls.2021.689545
Liu, P., Zhang, H., Yu, B., Xiong, L., and Xia, Y. (2015). Proteomic identification of early salicylate-and flg22-responsive redox-sensitive proteins in Arabidopsis. Sci. Rep. 5:8625. doi: 10.1038/srep08625
Liu, Y., Han, J., Wilson, A. J., O’Sullivan, L., and Haney, C. H. (2021). Amino acid availability determines plant immune homeostasis in the rhizosphere microbiome. bioRxiv [Preprint]. doi: 10.1101/2021.12.20.473424
Lovelace, A. H., Smith, A., and Kvitko, B. H. (2018). Pattern-triggered immunity alters the transcriptional regulation of virulence-associated genes and induces the sulfur starvation response in Pseudomonas syringae pv. tomato DC3000. Mol. Plant Microbe Interact. 31, 750–765. doi: 10.1094/MPMI-01-18-0008-R
Luo, X., Wu, W., Liang, Y., Xu, N., Wang, Z., Zou, H., et al. (2020). Tyrosine phosphorylation of the lectin receptor-like kinase LORE regulates plant immunity. EMBO J. 39:e102856. doi: 10.15252/embj.2019102856
Maathuis, F. J. M. (2006). The role of monovalent cation transporters in plant responses to salinity. J. Exp. Bot. 57, 1137–1147. doi: 10.1093/jxb/erj001
Macho, A. P., Lozano-Durán, R., and Zipfel, C. (2015). Importance of tyrosine phosphorylation in receptor kinase complexes. Trends Plant Sci. 20, 269–272. doi: 10.1016/j.tplants.2015.02.005
Malinsky, J., Tanner, W., and Opekarova, M. (2016). Transmembrane voltage: potential to induce lateral microdomains. Biochim. Biophys. Acta 1861, 806–811. doi: 10.1016/j.bbalip.2016.02.012
Matthius, E., Sun, J., Wang, L., Bhat, M. G., Mohammad-Sidik, A. B., Wilkins, K. A., et al. (2019). DORN1/P2K1 and purino-calcium signalling in plants: making waves with extracellular ATP. Ann. Bot. 124, 1227–1242. doi: 10.1093/aob/mcz135
Mithoe, S. C., Ludwig, C., Pel, M. J. C., Cucinotta, M., Casartelli, A., Mbengue, M., et al. (2016). Attenuation of pattern recognition receptor signaling is mediated by a MAP kinase kinase kinase. EMBO Rep. 17, 441–454. doi: 10.15252/embr.201540806
Mukherjee, K., Sharma, M., Jahn, R., Wahl, M. C., and Südhof, T. C. (2010). Evolution of CASK into a Mg2+-sensitive kinase. Sci. Signal. 3:ra33. doi: 10.1126/scisignal.2000800
Nemoto, K., Seto, T., Takahashi, H., Nozawa, A., Seki, M., Shinozaki, K., et al. (2011). Autophosphorylation profiling of Arabidopsis protein kinases using the cell-free system. Phytochemistry 72, 1136–1144. doi: 10.1016/j.phytochem.2011.02.029
Nieves-Cordones, M., Ródenas, R., Lara, A., Martínez, V., and Rubio, F. (2019). The combination of K+ deficiency with other environmental stresses: what is the outcome? Physiol. Plant. 165, 264–276. doi: 10.1111/ppl.12827
Nobori, T., Velásquez, A. C., Wu, J., Kvitko, B. H., Kremer, J. M., Wang, Y., et al. (2018). Transcriptome landscape of a bacterial pathogen under plant immunity. Proc. Natl. Acad. Sci. U.S.A. 115, E3055–E3064.
Nolan, M. A., Babcock, D. F., Wennemuth, G., Brown, W., Burton, K. A., and McKnight, G. S. (2004). Sperm-specific protein kinase A catalytic subunit Cα2 orchestrates cAMP signaling for male fertility. Proc. Natl. Acad. Sci. U.S.A. 101, 13483–13488. doi: 10.1073/pnas.0405580101
Olabisi, O. A., Zhang, J. Y., VerPlank, L., Zahler, N., DiBartolo, S., Heneghan, J. F., et al. (2016). APOL1 kidney disease risk variants cause cytotoxicity by depleting cellular potassium and inducing stress-activated protein kinases. Proc. Natl. Acad. Sci. U.S.A. 113, 830–837. doi: 10.1073/pnas.1522913113
Popescu, S. C., Brauer, E. K., Dimlioglu, G., and Popescu, G. V. (2017). Insights into the structure, function, and ion-mediated signaling pathways transduced by plant integrin-linked kinases. Front. Plant Sci. 8:376. doi: 10.3389/fpls.2017.00376
Popescu, S. C., Popescu, G. V., Bachan, S., Zhang, Z., Gerstein, M., Snyder, M., et al. (2009). MAPK target networks in Arabidopsis thaliana revealed using functional protein microarrays. Genes Dev. 23, 80–92. doi: 10.1101/gad.1740009
Popescu, S. C., Popescu, G. V., Bachan, S., Zhang, Z., Seay, M., Gerstein, M., et al. (2007). Differential binding of calmodulin-related proteins to their targets revealed through high-density Arabidopsis protein microarrays. Proc. Natl. Acad. Sci. U.S.A. 104, 4730–4735. doi: 10.1073/pnas.0611615104
Qi, Z., Hampton, C. R., Shin, R., Barkla, B. J., White, P. J., and Schachtman, D. P. (2008). The high affinity K+ transporter AtHAK5 plays a physiological role in planta at very low K+ concentrations and provides a caesium uptake pathway in Arabidopsis. J. Exp. Bot. 59, 595–607. doi: 10.1093/jxb/erm330
Qu, S., Zhang, X., Song, Y., Lin, J., and Shan, X. (2017). THESEUS1 positively modulates plant defense responses against Botrytis cinerea through GUANINE EXCHANGE FACTOR4 signaling. J. Integr. Plant Biol. 59, 797–804. doi: 10.1111/jipb.12565
Rich-Griffin, C., Eichmann, R., Reitz, M. U., Hermann, S., Woolley-Allen, K., Brown, P. E., et al. (2020). Regulation of cell type-specific immunity networks in Arabidopsis roots. Plant Cell 32, 2742–2762. doi: 10.1105/tpc.20.00154
Shabala, S. (2017). Signalling by potassium: another second messenger to add to the list? J. Exp. Bot. 68, 4003–4007.
Shi, X., Long, Y., He, F., Zhang, C., Wang, R., Zhang, T., et al. (2018). The fungal pathogen Magnaporthe oryzae suppresses innate immunity by modulating a host potassium channel. PLoS Pathog. 14:e1006878. doi: 10.1371/journal.ppat.1006878
Sierla, M., Hõrak, H., Overmyer, K., Waszczak, C., Yarmolinsky, D., Maierhofer, T., et al. (2018). The receptor-like pseudokinase GHR1 is required for stomatal closure. Plant Cell 30, 2813–2837. doi: 10.1105/tpc.18.00441
Stiffler, M. A., Grantcharova, V. P., Sevecka, M., and MacBeath, G. (2006). Uncovering quantitative protein interaction networks for mouse PDZ domains using protein microarrays. J. Am. Chem. Soc. 128, 5913–5922.
Storey, J. D. (2002). A direct approach to false discovery rates. J. R. Stat. Soc. Ser. B Stat. Methodol. 64, 479–498.
Strong, T. C., Kaur, G., and Thomas, J. H. (2011). Mutations in the catalytic loop HRD motif alter the activity and function of Drosophila Src64. PLoS One 6:e28100. doi: 10.1371/journal.pone.0028100
Sun, T., and Zhang, Y. (2022). MAP kinase cascades in plant development and immune signaling. EMBO Rep. 23:e53817.
Swarup, G., Dasgupta, J. D., and Garbers, D. L. (1984). Tyrosine-specific protein kinases of normal tissues. Adv. Enzyme Regul. 22, 267–288. doi: 10.1016/0065-2571(84)90018-9
Terry, B. R., Findlay, G. P., and Tyerman, S. D. (1992). Direct effects of Ca2+-channel blockers on plasma membrane cation channels of Amaranthus tricolor protoplasts. J. Exp. Bot. 43, 1457–1473.
Wu, H., Zhang, X., Giraldo, J. P., and Shabala, S. (2018). It is not all about sodium: revealing tissue specificity and signalling roles of potassium in plant responses to salt stress. Plant Soil 431, 1–17.
Keywords: pseudokinase, Raf-like MAP3K, integrin-linked kinase, cation signaling, innate immunity, microbe-associated molecular pattern
Citation: Brauer EK, Ahsan N, Popescu GV, Thelen JJ and Popescu SC (2022) Back From the Dead: The Atypical Kinase Activity of a Pseudokinase Regulator of Cation Fluxes During Inducible Immunity. Front. Plant Sci. 13:931324. doi: 10.3389/fpls.2022.931324
Received: 28 April 2022; Accepted: 27 May 2022;
Published: 11 August 2022.
Edited by:
Jens Staal, Ghent University, BelgiumReviewed by:
Thomas Dandekar, Julius Maximilian University of Würzburg, GermanyCopyright © 2022 Brauer, Ahsan, Popescu, Thelen, Popescu and Her Majesty the Queen in Right of Canada, as represented by the Minister of Agriculture and Agri-Food Canada for the contribution of Elizabeth Brauer. This is an open-access article distributed under the terms of the Creative Commons Attribution License (CC BY). The use, distribution or reproduction in other forums is permitted, provided the original author(s) and the copyright owner(s) are credited and that the original publication in this journal is cited, in accordance with accepted academic practice. No use, distribution or reproduction is permitted which does not comply with these terms.
*Correspondence: Elizabeth K. Brauer, ZWxpemFiZXRoLmJyYXVlckBhZ3IuZ2MuY2E=; Sorina C. Popescu, c3BvcGVzY3VAYmNoLm1zc3RhdGUuZWR1
†ORCID: Elizabeth K. Brauer, orcid.org/0000-0002-6957-266X; Nagib Ahsan, orcid.org/0000-0002-6813-7527; George V. Popescu, orcid.org/0000-0002-7580-6792; Jay J. Thelen, orcid.org/0000-0001-5995-1562; Sorina C. Popescu, orcid.org/0000-0001-5780-8252
‡Present addresses: Elizabeth K. Brauer, Ottawa Research and Development centre, Agriculture and Agri-Food Canada, Ottawa, ON, Canada; Department of Biology, University of Ottawa, Ottawa, ON, Canada; Nagib Ahsan, Department of Chemistry and Biochemistry, The University of Oklahoma, Norman, OK, United States; Mass Spectrometry, Proteomics, and Metabolomics Core Facility, Stephenson Life Sciences Research Center, The University of Oklahoma, Norman, OK, United States; George V. Popescu, Institute for Genomics, Biocomputing and Biotechnology, Mississippi State University, Starkville, MS, United States; Sorina C. Popescu, Department of Biochemistry, Molecular Biology, Plant Pathology, and Entomology, Mississippi State University, Starkville, MS, United States