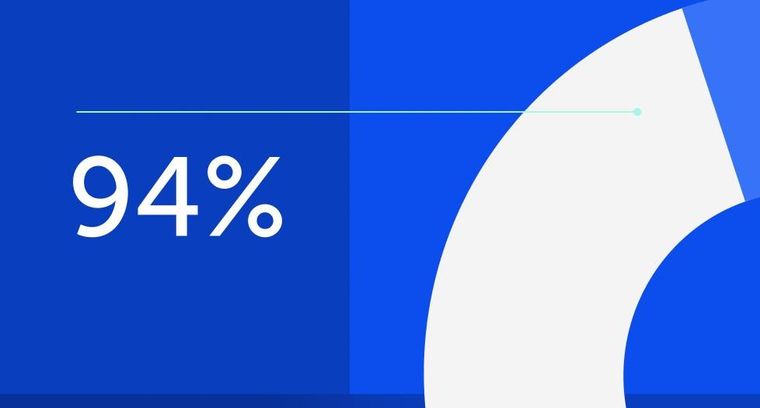
94% of researchers rate our articles as excellent or good
Learn more about the work of our research integrity team to safeguard the quality of each article we publish.
Find out more
REVIEW article
Front. Plant Sci., 07 July 2022
Sec. Plant Physiology
Volume 13 - 2022 | https://doi.org/10.3389/fpls.2022.929532
This article is part of the Research TopicCurrent Challenges in Photosynthesis: From Natural to Artificial, Volume IIView all 6 articles
The oxygen-evolving center (OEC) in photosystem II (PSII) of oxygenic photosynthetic organisms is a unique heterometallic-oxide Mn4CaO5-cluster that catalyzes water splitting into electrons, protons, and molecular oxygen through a five-state cycle (Sn, n = 0 ~ 4). It serves as the blueprint for the developing of the man-made water-splitting catalysts to generate solar fuel in artificial photosynthesis. Understanding the structure–function relationship of this natural catalyst is a great challenge and a long-standing issue, which is severely restricted by the lack of a precise chemical model for this heterometallic-oxide cluster. However, it is a great challenge for chemists to precisely mimic the OEC in a laboratory. Recently, significant advances have been achieved and a series of artificial Mn4XO4-clusters (X = Ca/Y/Gd) have been reported, which closely mimic both the geometric structure and the electronic structure, as well as the redox property of the OEC. These new advances provide a structurally well-defined molecular platform to study the structure–function relationship of the OEC and shed new light on the design of efficient catalysts for the water-splitting reaction in artificial photosynthesis.
Photosynthetic oxygen evolution is a unique function of oxygenic photosynthetic organisms, which takes place in photosystem II (PSII) of cyanobacteria, algae, and plants (Barber, 2009; Dau and Zaharieva, 2009; Cardona et al., 2012; Vinyard et al., 2013; Shen, 2015; Govindjee et al., 2017; Junge, 2019; Lubitz et al., 2019; Shevela et al., 2019; Blankenship, 2021). PSII is a multi-subunit membrane protein complex containing more than 20 subunits and hundreds of cofactors. The reaction center of PSII is shown in Figure 1A. Upon photo excitation, the primary electron donor (P680) donates one electron to the primary electron acceptor (Pheo) in a few picoseconds, producing the P680+• and Pheo-• at the donor side and acceptor side, respectively (Renger and Holzwarth, 2005; Rappaport and Diner, 2008; Cardona et al., 2012; Shevela et al., 2021). Pheo-• then delivers the electron to the primary plastoquinone (QA) and the secondary plastoquinone (QB) in sequence via the non-heme iron at the acceptor side (Petrouleas and Crofts, 2005; Cardona et al., 2012), where one bicarbonate anion coordinated on the non-heme iron is highly required for the efficient electron transfer between QA and QB (Shevela et al., 2012). P680+• with high redox potential (~1.25 V) abstracts one electron from the secondary electron donor (TyrZ), forming a neutral radical (TyrZ•) (Diner and Britt, 2005; Styring et al., 2012). The latter then drives the water-splitting reaction at the oxygen-evolving center (OEC) in milliseconds at the donor side (Tommos and Babcock, 1998; Zhang, 2007; Styring et al., 2012). The catalytic turnover of the OEC (Figure 1B) involves five different redox states (Sn, n = 0 ~ 4) (Kok et al., 1970; Dau and Haumann, 2007; Cox et al., 2014a; Yano and Yachandra, 2014), in which the S0 state is the initial and most reduced state, the S1 state is the dark-stable state, the S2 and S3 states are metastable and decay eventually to the dark stable S1 state, whereas the S4 state is a transient state that releases molecular oxygen and regenerates the S0 state. This catalytic water-splitting reaction provides electrons and protons to, ultimately, produce the biomass or biofuel, and molecular oxygen to maintain the oxygenic atmosphere of our planet (Blankenship et al., 2011; Barber, 2020), which serves as the blueprint to develop efficient man-made catalysts for the water-splitting reaction in artificial photosynthesis.
Figure 1. Structure of the reaction center and main cofactors involved in electron transfer in PSII (Umena et al., 2011) (A) and catalytic cycle of the OEC (Cox et al., 2020) (B). The oxidation states of four Mn ions in each S-state are shown within square boxes.
Due to broad interests in fundamental research and potential applications in artificial photosynthesis (Herrero et al., 2008; Gust et al., 2010; Andreiadis et al., 2011; Concepcion et al., 2012; Faunce et al., 2013; Kärkäs et al., 2014; Hunter et al., 2016; Najafpour et al., 2016; El-Khouly et al., 2017; Nocera, 2017; Ye et al., 2019; Zhang and Sun, 2019b; Zhang and Reisner, 2020; Kondo et al., 2021), the structure and catalytic mechanism of the OEC have attracted extensive attention during the last century (Junge, 2019; Cox et al., 2020). However, revealing the principle of the OEC has been one of the great and persistent challenges and a long-standing issue in the research field of photosynthesis.
Extensive biochemistry and biophysics studies have been performed to reveal the properties of the OEC in different S-states during the last several decades (Yano and Yachandra, 2014; Junge, 2019). It has been well demonstrated that OEC is composed of four Mn ions and one calcium ion, in which the calcium can be replaced by strontium (Debus, 1992; Yocum, 2008). Based on spectroscopic studies of the X-ray absorption spectroscopy (XAS) (Yano and Yachandra, 2014) and electron paramagnetic resonance (EPR) (Peloquin and Britt, 2001; Krewald et al., 2015) measurements of different S-states OEC, the oxidation states of the four Mn ions were generally suggested to be S0 (III, III, III, IV), S1 (III, III, IV, IV), S2 (III, IV, IV, IV), and S3 (IV, IV, IV, IV), respectively. However, some groups (Zheng and Dismukes, 1996; Gatt et al., 2012; Pace et al., 2012; Petrie et al., 2020) suggested that the oxidation states of the four Mn ions could be S0 (II, III, III, III), S1 (III, III, III, III), S2 (III, III, III, IV), and S3 (III, III, IV, IV), respectively. These two different assignments of the four Mn ions are labeled as the “high-oxidation paradigm” and the “low oxidation paradigm,” respectively (Krewald et al., 2015; Pantazis, 2018). The former has generally been used by most researchers, yet the unambiguous chemical evidence for the assignment of the oxidation states of the four Mn ions remains elusive.
The crystal structure information of the OEC has emerged since the beginning of this century (Zouni et al., 2001; Kamiya and Shen, 2003; Ferreira et al., 2004; Loll et al., 2005; Guskov et al., 2009; Tanaka et al., 2017; Graça et al., 2021; Kato et al., 2021). In 2001, Zouni et al. reported a crystal structure of PSII from a cyanobacterium at a resolution of 3.8 Å (Zouni et al., 2001). In 2004, Ferreira et al. (Ferreira et al., 2004) reported a resolution of 3.5 Å structure data of PSII and proposed that the OEC was a Mn3CaO4 cubane attached by a “dangler” Mn ion via one μ4-oxide bridge, forming a Mn4CaO4-cluster (Ferreira et al., 2004). In 2011, Umena et al. (Umena et al., 2011) reported the crystal structure of PSII at a resolution of 1.9 Å, which revealed the detailed structure of the OEC, as shown in Figure 2A. Here, the coordination ligands of the OEC are provided by six carboxylate groups from the amino acid residues of D1-Asp170, D1-Glu189, D1-Glu333, D1-Asp342, D1-Ala344, CP43-Glu354, one imidazole group from D1-His332, and four water molecules (two on calcium and two on dangler Mn, respectively). Further, an additional μ2-oxide bridge (O4) between Mn4 and Mn3 was observed (Umena et al., 2011), which is consistent with the proposal by Dau et al. (2008). The structure of the OEC, shown in Figure 2A, has been further confirmed by the X-ray free-electron laser (XFEL) data (Kupitz et al., 2014; Suga et al., 2015, 2017, 2019; Young et al., 2016; Kern et al., 2018; Ibrahim et al., 2020; Hussein et al., 2021) and the single-particle cryo-electron microscopy (Cryo-EM) (Wei et al., 2016; Kato et al., 2021; Xiao et al., 2021; Gisriel et al., 2022). The entire structure of the OEC is an asymmetric Mn4CaO5-cluster. In this cluster, calcium, a key component of the OEC, is located in the middle and connected to the four Mn ions through three oxide bridges and two carboxylate groups; this structural feature (see Figure 2B) is consistent with the proposal by Zhang et al. in 1999 (Zhang et al., 1999).
Figure 2. Scheme for the structure of the OEC (Umena et al., 2011) (A) and the theoretical model (Zhang et al. 1999) (B).
Notably, most crystallographic studies, in the past, were performed on the dark stable PSII sample, in which the OEC was generally in the S1 state. Recently, the structures of the OEC in other S-states have been reported (Suga et al., 2017, 2019; Kern et al., 2018; Ibrahim et al., 2020; Hussein et al., 2021; Li et al., 2021). Remarkably, one new oxygen atom (O6) coordinated to the Mn1 was observed in the S3 state. This new oxygen atom was suggested to serve as one of the substrates to form the O=O bond (Suga et al., 2017, 2019; Kern et al., 2018; Ibrahim et al., 2020). However, the existence of the new oxygen (O6) in the S3 state is still under debate. It was argued that both O6 and O5 in the reported S3 state OEC may belong to the same oxygen atom but in two possible positions (Petrie et al., 2020; Wang et al., 2021). Furthermore, there are some structural uncertainties due to the incoherent transition of the S-state of PSII samples (Askerka et al., 2015; Tanaka et al., 2017).
Compared with the structure revealed by the X-ray diffraction (XRD) (Ferreira et al., 2004; Loll et al., 2005; Umena et al., 2011; Tanaka et al., 2017), the structure of the OEC revealed by the XFEL (Kupitz et al., 2014; Suga et al., 2015, 2017, 2019; Young et al., 2016; Kern et al., 2018; Ibrahim et al., 2020; Hussein et al., 2021) has been considered to be more reliable due to the lack of significant radiation damage induced by the X-ray beam (Yano et al., 2005; Grabolle et al., 2006). However, as yet, there is consensus for the atomic positions of the S1 state OEC, as revealed by XFEL, certainly not for all structures with the results from EXAFS spectroscopy studies on the active sample used (Davis and Pushkar, 2015; Askerka et al., 2017). To check if those reported structure data were directly correlated with the native structures of the OEC in different S-states, we have carried out bond valence sum (BVS) calculations on these XFEL’s structures reported recently (Chen et al., 2019; Li et al., 2020b). We note that BVS calculation has been widely used to evaluate the oxidation valences of atoms in coordination complexes and in metalloenzymes (Brown, 2009). Table 1 lists the results of the BVS calculation on the XFEL’s structures of different S-states of the OEC reported recently. Surprisingly, we see that the oxidation states of the four Mn ions revealed by BVS calculations are significantly lower than that suggested by the spectroscopic studies (Peloquin and Britt, 2001; Dau and Haumann, 2007; Yano and Yachandra, 2014; Krewald et al., 2015) (Figure 1B), indicating that the reduction of the Mn ions with high valences would take place during the structural determination by XFEL (Yano et al., 2005; Grabolle et al., 2006; Amin et al., 2016). If so, one would expect that those reported structure data of the OEC would be different from the native structure. This opinion is consistent with the suggestion that structural modifications of the OEC induced by XFEL may take place and the position of the oxide bridge (eg., O5) could be significantly disturbed by XFEL (Amin et al., 2016). Therefore, the precise structure of the OEC in different S-states remains elusive.
Table 1. Bond valence sum (BVS) calculations on the structure of the OEC revealed by X-ray free-electron laser (XFEL) method at different resolutions in different S-states.
Based on biochemical, biophysical, and theoretical investigations, various hypotheses for the catalytic mechanisms of the O=O bond formation have been suggested by many researchers (Siegbahn, 2013; Askerka et al., 2017; Barber, 2017; Wang et al., 2017; Corry and O’Malley, 2018; Kawashima et al., 2018; Kern et al., 2018; Pushkar et al., 2018; Yamaguchi et al., 2018; Britt and Marchiori, 2019; Suga et al., 2019; Zhang and Sun, 2019a; Marchiori et al., 2020; Capone et al., 2021). Figure 3 shows four typical proposals.
Figure 3. Four typical mechanism models suggested in literatures. Components of the OEC involved in O=O bond formation are marked by red color. Roman numbers indicate the oxidation state of the Mn ion.
Figure 3A shows a possible mechanism proposed by Barber (Barber, 2017), in which two water molecules (W2 and W3) serve as substrates to form the O=O bond. The key feature of this mechanism is that the O=O bond is formed by a nucleophilic attack of a calcium ligated hydroxyl group onto an electrophilic oxo group of MnV ≡ O or MnIV-O• derived from the deprotonation of the second substrate water molecule. Similar proposals had also been suggested by others (Pecoraro et al., 1998; Vrettos et al., 2001; Chen et al., 2015; Vinyard et al., 2015). However, these proposals were not supported by recent theoretical calculations reported by Siegbahn group (Siegbahn, 2017). The second model (Figure 3B) was suggested by Ishikita group (Kawashima et al., 2018), in which the μ2-oxide bridge (O4) and one water molecule (W1) serve as two oxygen sources to form the O=O bond. The key feature of this proposal is that the O=O bond is formed through the coupling of the O4 oxide bridge and a MnIV-O• oxyl radical. However, the oxidation states of (III, IV, IV, IV) for the four Mn ions in the S3 state were not consistent with the widely accepted oxidation states of (IV, IV, IV, IV) (Peloquin and Britt, 2001; Dau and Haumann, 2008; Yano and Yachandra, 2014; Krewald et al., 2015). The third model (Figure 3C) was proposed by Sun group (Zhang and Sun, 2019a), in which one MnVII ion was suggested to be involved in the S4 state. This mechanism has been recently evaluated by a computational study that shows that the formation of the MnVII requires a much higher barrier for forming O2 than the earlier proposals with four MnIV atoms (Li et al., 2020a). The fourth model, originally proposed by Siegbahn (Siegbahn, 2013), and then other groups (Pecoraro et al., 1998; Cox et al., 2020), is where the μ4-oxide bridge (O5) and the newly inserted water (O6) are considered to serve as the oxygen source for the O=O bond (Figure 3D). This proposal has been widely used to explain the observation of the crystallographic data and a large number of spectroscopic observations (Cox et al., 2014b, 2020; Kern et al., 2018; Britt and Marchiori, 2019; Suga et al., 2019). According to this mechanism, the release of O2 from the S4 state would result in the formation of four unsaturated metal ions, namely three 5-coordinated manganese (i.e., Mn1, Mn3, and Mn4) and one 6-coordinated calcium, which would certainly require a much higher activation energy (Zhang and Kuang, 2018). Thus, one would expect that the molecular oxygen release could be the rate-limiting step during the catalytic cycle; however, this is inconsistent with the fast O2 release observed in PSII (Haumann et al., 2005; Davis et al., 2018).
As mentioned above, although various hypotheses for the mechanism of the water-splitting reaction of the OEC have been proposed (Siegbahn, 2013; Askerka et al., 2017; Barber, 2017; Wang et al., 2017; Corry and O’Malley, 2018; Kawashima et al., 2018; Kern et al., 2018; Pushkar et al., 2018; Yamaguchi et al., 2018; Britt and Marchiori, 2019; Suga et al., 2019; Zhang and Sun, 2019a; Marchiori et al., 2020; Capone et al., 2021), the detailed mechanism remains an open question mainly due to the lack of the unambiguous experimental evidence for the O=O bond formation and the precise geometric structure and electronic structure of the OEC in different S-states (Chen and Zhang, 2021).
To facilitate the understanding of the structure and properties of the OEC, as well as for developing efficient water-splitting catalysts, many research groups, during the last three decades, have attempted to synthesize the OEC (Wieghardt, 1989; Limburg et al., 1999; Mukhopadhyay et al., 2004; Mullins and Pecoraro, 2008; Dismukes et al., 2009; Gerey et al., 2016; Zhang and Sun, 2019b; Li et al., 2020b; Chen et al., 2021; Ezhov et al., 2021). It is a great challenge and a long-standing issue for chemists to synthesize the OEC in the laboratory (Zhang, 2015; Li et al., 2020b). During the last two decades, numerous multi-manganese complexes have been synthesized (Wieghardt, 1989; Limburg et al., 1999; Mukhopadhyay et al., 2004; Mullins and Pecoraro, 2008; Dismukes et al., 2009; Gerey et al., 2016; Chang et al., 2017). Significant advances for the mimicking of the OEC have emerged since 2011 (Tsui et al., 2013a; Chen et al., 2017; Paul et al., 2017). Further, Agapie group (Kanady et al., 2011) reported an artificial MnIV3CaO4-complex (1) using a multi-pyridylalkoxide ligand (Figures 4A,B). In addition, a series of analogs or derivatives of the cluster have been reported, by using a similar ligand (Tsui and Agapie, 2013; Kanady et al., 2014; Lin et al., 2015; Lionetti et al., 2019). In 2012, Christou group reported a MnIV3Ca2O4-complex (2) with one Ca2+ attached to the Mn3CaO4 cubane (Mukherjee et al., 2012) (Figures 4C,D). A similar Mn3Ca2O4-complex was also isolated as a by-product during the synthesis of Mn4CaO4-cluster (Chen et al., 2022). Here, the peripheral ligands of the Mn3Ca2O4-cluster are provided by pivalic anions or neutral pivalic acid, which resembles to that of the OEC in PSII (Umena et al., 2011). In 2014, Zhang group (Chen et al., 2014) reported an artificial (MnIV3SrO4)2O-complex (3) that contains both the heterometallic-oxide Mn3SrO4 cubane and all three types of oxide bridges (μ2-oxide, μ3-oxide, and μ4-oxide), as seen in the Sr2+-containing OEC (Koua et al., 2013) (Figures 4E,F).
Figure 4. Structures of three artificial complexes containing Mn3XO4 cubane (X = Ca/Sr). (A) Core of the Mn3CaO4-complex (1); (B) Structure of the Mn3CaO4-complex (1) (Kanady et al., 2011); (C) Core structure of the Mn3Ca2O4-complex (2); (D) Structure of the Mn3Ca2O4-complex (2) (Mukherjee et al., 2012); (E) Core of the (Mn3SrO4)2O-complex (3); (F) Structure of the (Mn3SrO4)2O-complex (3) (Chen et al., 2014). Distances are given in Å units; Mn, Ca, Sr., O, N, and C are shown in purple, green, cyan, orange, blue, and yellow, respectively. For clarity, all hydrogen atoms are not shown.
In 2015, Zhang group reported an artificial Mn4CaO4-complex (4; Figures 5C,D) that was prepared through a two-step procedure (Zhang et al., 2015). The first step was to synthesize a precursor through a reaction of Ca(CH3CO2)2•H2O, Mn(CH3CO2)2•(H2O)4, nBu4NMnO4 (nBu = n-butyl), and tBuCO2H (tBu = tert-butyl; molar ratio of 1: 1: 4: 40) in boiling acetonitrile. The second step was to treat the precursor with 2% pyridine in ethyl acetate, leading to the formation of the final product, [Mn4CaO4(tBuCO2)8(tBuCO2H)2(C5H5N)] (4). This Mn4CaO4-complex contains a Mn3CaO4 cubane attached by a dangler Mn ion via one μ4-oxide bridge, forming an asymmetric Mn4CaO4-cluster. Its peripheral environment is provided by eight tBuCO2− anions and three neutral ligands on Ca and Mn4 (two pivalic acid molecules and one pyridine, respectively), which is remarkably similar to that in the OEC. BVS calculation confirms that the oxidation states of the four Mn ions are in (III, III, IV, IV).
Figure 5. Structural comparison of the OEC (Suga et al., 2015) and the artificial Mn4CaO4-complex (4) (Zhang et al., 2015). (A) Core of the OEC; (B) structure of the OEC; (C) core of 4; (D) structure of 4. The data for the OEC is taken from the first monomer in the crystal structure data of PSII with the Protein Data Bank code 4UB6. For clarity, the methyl groups and the hydrogen atoms are not shown. All other illustrations are the same as those in Figure 4.
The artificial Mn4CaO4-cluster (4) has a [MnIII2MnIV2]/[MnIIIMnIV3] redox couple of ~0.8 V (vs. normal hydrogen electrode, NHE), as shown in the cyclic voltammogram (CV) (Figure 6A), which is essentially the same as the estimated value (~ 0.8 V) for the S1 → S2 transition of the OEC (Vass and Styring, 1991; Dau and Zaharieva, 2009; Mandal et al., 2020). The oxidized Mn4CaO4-cluster displays two distinct electron paramagnetic resonance (EPR) signals (g = 4.9 and g = 2.0) (Figure 6B), which are similar to the g ≈ 4 and g = 2.0 EPR signals observed in the S2 state OEC (Peloquin and Britt, 2001; Pantazis et al., 2012). Furthermore, the artificial Mn4CaO4-cluster can catalyze the water-splitting reaction on the electron surface in the presence of a small amount of water in acetonitrile (Figure 6C).
Figure 6. Redox properties, EPR, and catalytic activity measurements of Mn4CaO4-complex (4) (Zhang et al., 2015). (A) Cyclic voltammogram (CV) measurement of 4 in dichloroethane; (B) EPR spectrum for the one-electron oxidized 4; (C) activity measurements of 4 in acetonitrile with different amounts of H2O. The inset in C shows the CV of 4 without H2O on a different scale.
The artificial Mn4CaO4-cluster (4) is the closest mimic of the OEC up to now, which resembles not only in the structure of the metal-oxide core and the peripheral ligands, but also in the redox potential and the catalytic function of the OEC. Considering the high similarity between the artificial Mn4CaO4-cluster and the OEC, we speculate that oxidation states (III, III, IV, IV) of the four Mn ions in this artificial cluster provide unambiguous chemical evidence to support the assignment of oxidation states of (III, III, IV, IV) for the four Mn ions in the S1 state OEC in PSII.
The mechanism of the synthesis of the artificial Mn4CaO4-cluster has been recently studied by characterizing the intermediate species during the synthesis of the Mn4CaO4-complex (4) (Chen et al., 2022). By using the high-resolution electrospray ionization (HR-ESI) mass spectroscopy, we have characterized the precursor of the Mn4CaO4-cluster and observed five key fragments with m/z− values at 1233.235, 1218.259, 875.118, 358.120, and 343.143 assigned to the [Mn4CaO4(tBuCO2)9]−, [Mn3Ca2O4(tBuCO2)9]−, [Mn3CaO4(tBuCO2)6]−, [Mn(tBuCO2)3]−, and [Ca(tBuCO2)3]−, respectively (Chen et al., 2022). More importantly, after extensive experimentation, three key intermediates, [Mn3CaO4(tBuCO2)6(tBuCO2H)3] (5), [nBu4NMn(tBuCO2)4] (6), and [Mn4CaO4(tBuCO2)8(tBuCO2H)3] (7), were successfully crystallized. The structures of these intermediates (5–7) are shown in Figure 7.
Figure 7. Structures of three intermediates for the synthesis of the artificial Mn4CaO4-cluster (Chen et al., 2022). (A) Core of [Mn3CaO4(tBuCO2)6(tBuCO2H)3] (5); (B) structure of 5; (C) Mn center in [nBu4NMn(tBuCO2)4] (6); (D) structure of the [Mn(tBuCO2)4]− anion in 6; (E) core structure of [Mn4CaO4(tBuCO2)8(tBuCO2H)3] (7); (F) structure of 7. All other illustrations are the same as those in Figure 5.
Based on the isolation and characterization of these intermediates for the synthesis of the Mn4CaO4-cluster, we suggest that the Mn4CaO4-cluster could be formed through a reaction between a thermodynamically stable Mn3CaO4-cluster and an unusual four-coordinated MnIII ion (Figure 8). The freshly formed Mn4CaO4-cluster (7) with carboxylate groups only is unstable, but it can be significantly stabilized by binding an organic base (e.g., pyridine) on the “dangler” Mn ion. Furthermore, we have found that the dangler Mn ion is flexible and can be replaced by calcium under weak acid conditions, giving rise to the Mn3Ca2O4-cluster (2) as shown in Figures 4C,D.
Figure 8. Possible synthesizing mechanism of the artificial Mn4CaO4-cluster (4) (Chen et al., 2022).
Considering the high similarity between the artificial Mn4CaO4-cluster and the OEC (Figure 5), we speculate that the synthesizing mechanism (Figure 8), described above, could provide chemical insights into the assembly of the OEC. In the biological system, both the assembly and the disassembly of the OEC frequently take place under physiological conditions. The disassembly of the OEC takes place after the photodamage and degradation of the D1 protein of PSII under high light flux. To achieve the water-splitting capability, the newly functional OEC must be properly assembled after the repairing of the D1 protein of PSII (Barber and Andersson, 1992; Dasgupta et al., 2008). In PSII, the early steps of the assembly of the OEC involving two Mn and one Ca ions have been studied for more than 50 years (Cheniae and Martin, 1971; Dasgupta et al., 2008; Bao and Burnap, 2016; Murray et al., 2020); on the other hand, the assembly of the third and the fourth Mn ions in OEC is fully unknown (Bao and Burnap, 2016; Avramov et al., 2020). Considering the observed thermodynamical stability of the fully carboxylic ligand coordinated Mn3CaO4-cluster (5) observed (Chen et al., 2022), we propose that a similar Mn3CaO4-cluster could be present during the synthesis of the OEC in PSII. If it was the case, a mono-nuclear Mn ion (similar to that in 6) would be necessary to be incorporated into the Mn3CaO4-cluster, followed by structural rearrangements to form the intact OEC, as has been suggested recently (Gisriel et al., 2020; Sato et al., 2021).
In order to improve the stability of the artificial Mn4CaO4-cluster, we have optimized its peripheral environment by replacing the two pivalic acid molecules on the calcium with organic solvent molecules (Chen et al., 2019). Structures of two new Mn4CaO4-complexes, [Mn4CaO4(tBuCO2)8(Py)(tBuCO2H) (CH3CN)] (8) and [Mn4CaO4(tBuCO2)8(Py)(DMF)2] (9) are shown in Figure 9. Interestingly, we have found that the change of these ligands on calcium does not affect neither the Mn4CaO4 core nor the oxidation states of the four Mn ions, as shown in Figure 9. This observation demonstrates that both the geometric structure and the electronic structure of the artificial Mn4CaO4-cluster are relatively stable, which provides chemical insights into the reason why the oxygenic photosynthetic organisms have selected the Mn4CaO4-cluster as the key structural unit to build the OEC in natural photosynthesis (Barber, 2020). Furthermore, the same oxidation states of the four Mn ions in 4, 7, 8, and 9 (Figures 5, 7, 9) further confirm the assignment of the oxidation stats of (III, III, IV, IV) in the S1 state OEC in PSII (Krewald et al., 2015).
Figure 9. Structural comparison of three artificial Mn4CaO4-complexes (4, 8, 9) (Zhang et al., 2015; Chen et al., 2019). (A) Core of 4; (B) structure of 4; (C) core of 8; (D) structure of 8; (E) core of 9; (F) structure of 9. All illustrations are the same as those in Figure 5.
The redox-inactive metal ion, Ca2+, is an indispensable component for the catalytic function of the OEC, and its depletion results in the complete loss of the water-splitting capability of PSII (Yocum, 2008). In the biological system, Ca2+ can only be functionally replaced by Sr2+ (Boussac et al., 2004; Yocum, 2008). It has been argued that the Lewis acidity of the redox-inactive metal ion could play a role in modulating the redox potentials of heterometallic-oxide clusters (Tsui et al., 2013b; Tsui and Agapie, 2013; Krewald et al., 2016; Saito et al., 2021). However, the detailed functional role of the Ca2+ in the OEC remains largely unknown because direct investigation of the calcium is severely restricted by the lack of controlled modifications of this redox-inactive metal ion without changing the core structure and the local protein environment of the OEC in the biological system (Krewald et al., 2016; Saito et al., 2021).
To study the possible function of the calcium ion in OEC and to develop robust artificial catalysts for the water-splitting reaction, tremendous efforts have been devoted to preparing calcium substituted Mn4XO4-clusters in our laboratory. In 2021, we successfully prepared the [Mn4YO4(tBuCO2)9(Napy)] (Napy = 1,8-naphthyridine) (10) and [Mn4GdO4(tBuCO2)9(Napy)] (11) (Yao et al., 2021). Surprisingly, as shown in Figure 10, both the two rare-earth element-containing Mn4XO4-clusters (X = Y, Gd) have nearly the same core structure and peripheral carboxylic ligands, as well as the oxidation states of the four Mn ions as those in the Mn4CaO4-cluster (4) and in the S1 state of the OEC (Umena et al., 2011). This observation clearly demonstrates that the substitution of the calcium by the rare-earth element does not affect neither the geometric structure nor the electronic structure of the Mn4XO4-clusters.
Figure 10. Structural comparison of the OEC (Suga et al., 2015), Mn4CaO4-cluster (4) (Zhang et al., 2015), the Mn4YO4-cluster (10) and Mn4GdO4-cluster (11) (Yao et al., 2021). See Figure 5 for further information.
CV measurements (Figure 11) show that both Mn4YO4-cluster (10) and Mn4GdO4-cluster (11) have a redox potential of +0.79 V for the [MnIII2MnIV2]/[MnIIIMnIV3] redox couple, which is nearly the same as that of the Mn4CaO4-cluster (4) (+0.8 V) (Zhang et al., 2015) and the estimated value for the S1 → S2 transition (∼ + 0.8 V) of the OEC (Dau and Zaharieva, 2009; Mandal et al., 2020). Moreover, the redox potentials of −0.05 V and + 1.3 V for the [MnIII3MnIV]/[MnIII2MnIV2] and [MnIIIMnIV3]/[MnIV4] irreversible redox couples can be estimated for both the Mn4YO4-cluster and the Mn4GdO4-cluster, respectively. These values are also close to that (−0.1 and + 1.25 V) observed in the Mn4CaO4-cluster (Zhang et al., 2015) as well. These results clearly show that the replacement of the calcium by rare-earth element does not significantly affect redox potentials of the heterometallic-oxide Mn4XO4-cluster although the Lewis acidity of Y3+, Gd3+, and Ca2+ is significantly different. This observation challenges the earlier view that the redox-inactive metal ion would modulate the redox potentials of the heterometallic-oxide cluster (Tsui et al., 2013b; Tsui and Agapie, 2013).
Figure 11. Redox potentials of the Mn4YO4-cluster (10) and Mn4GdO4-cluster (11) (Yao et al., 2021). (A) CV of Mn4YO4-cluster. (B) CV of Mn4GdO4-cluster. The possible oxidation states of the four Mn ions are shown in blue. The scan direction is indicated by the arrow. (C) Dependence of E1/2 of three redox couples on pKa of the X(aqua)n+ ion (Perrin, 1982) of three Mn4XO4-clusters. All potentials were referenced to NHE.
The above results suggest that rare-earth elements can structurally and energetically replace the calcium in artificial neutral Mn4XO4 clusters in a chemical system, which, indeed, sheds new light on the functional role of the calcium in the OEC and supports the idea that the redox-inactive metal ion could indeed play roles in maintaining the cluster’s integrity and stability instead of modulating the redox potential of the OEC. Obviously, these robust rare-earth element-containing Mn4XO4-clusters provide a structurally well-defined molecular platform to investigate the structure–function relationship of its biological paradigm and shed new light on the design of efficient water-splitting catalysts in artificial photosynthesis.
Although these Mn4XO4-clusters (X = Ca/Y/Gd) closely mimic the OEC in many aspects and provide new insights into the structure–function relationship of the biological catalyst, it remains a great challenge to overcome in order to discover a precise mimic of the structure and function of the OEC in a laboratory. In the first place, the μ2-oxide bridge (O4) seen in the S1 state of the OEC is still missing in all the current known Mn4XO4-clusters. Incorporating this last “missing puzzle” into artificial Mn4XO4-cluster is a great challenge for synthetic chemistry, which is urgently needed for the understanding of the functional role of this oxide bridge and of the catalytic mechanism for the O=O bond formation in the OEC. Further, all synthetic Mn4XO4-clusters display very poor solubility in aqueous solution because of the hydrophobic peripheral environment mainly provided by the pivalate groups (i. e. tBuCO2); thus, it is difficult to carry out its catalytic performance in aqueous solution as is the case with many other artificial catalysts reported thus far (Zhang and Sun, 2019b; Kondo et al., 2021). In addition, it has been found that many Mn complexes are not stable in the aqueous solution during the catalytic reaction (Hocking et al., 2011; Li et al., 2017); thus, it is crucial to develop a proper experimental condition for the catalytic performance of these OEC’s mimics. In the biological system, the Mn4CaO5-cluster is surrounded by non-aqueous protein environment with special channels for the delivery of protons, electrons, and the substrate (Shen, 2015; Hussein et al., 2021). Obviously, mimicking the first and the second coordination spheres of the OEC in PSII with functional channels is further required to achieve high reactivity in the future.
In summary, the crystallographic studies of PSII have revealed that the OEC is composed of an asymmetric Mn4CaO5-cluster; however, the detailed catalytic mechanism for the water-splitting reaction remains elusive due to the structural uncertainty of the different intermediate states of the OEC during its catalytic turnover. It is a great challenge to precisely mimic the OEC in the laboratory, yet a series of artificial Mn4XO4-clusters (X = Ca/Y/Gd) have been reported recently, which closely mimic both the geometric structure and the electronic structure, as well as the redox properties of the OEC in PSII. The investigation of these structurally well-defined chemical models provides distinct chemical insights into the understanding of the structure–function relationship of the OEC as well as the catalytic mechanism of the water-splitting reaction in natural photosynthesis. We list below several major take-home messages.
The oxidation states of the four Mn ions in all these Mn4XO4-clusters are (III, III, IV, IV), which provides the unambiguous chemical evidence for the “high-oxidation paradigm” assignment of the four Mn ions in the S1 state of the OEC (Krewald et al., 2015).
The preparation and reactivity of artificial Mn4CaO4-clusters clearly demonstrate that this cluster is thermodynamically stable, which supports the proposal that the Mn4CaO4-cluster could be an evolutionary origin of the natural OEC (Barber, 2016).
The finding that the rare-earth elements can structurally and energetically replace the calcium in the Mn4CaO4-cluster provides important chemical insight into the functional role of the calcium in the OEC. It indicates that the redox-inactive metal ion could play roles in maintaining the cluster’s integrity and stability instead of modulating the redox potential of the OEC.
Based on the characterization of artificial Mn4XO4-clusters, we clearly see that all μ3- and μ4- oxide bridges are tightly bound to the cluster, supporting that they may play roles in maintaining the cluster’s stability and integrity rather than as reactive sites for the O=O bond formation. However, we should point out that precise structural mimicking and functional mimicking are urgently required in the future to reveal the detailed catalytic mechanism and to achieve the high reactivity of water-splitting reaction in artificial photosynthesis. We believe that the further investigation of these robust artificial Mn4XO4-clusters would help to develop efficient man-made catalysts for the water-splitting reaction in artificial photosynthesis.
CZ conceived the project and designed experiments. YC carried out BVS calculation. CZ, CC, BX, and RY participated in the synthesis and characterization of artificial Mn4XO4-clusters involved in the paper. YC and CZ wrote the manuscript All authors contributed to the article and approved the submitted version.
This work was supported by the National Natural Science Foundation of China (Nos. 91961203 and 22001255), the National Key Research & Development Program of China (No. 2017YFA0503704), the Strategic Priority Research Program of the Chinese Academy of Sciences (Nos. XDA21010212 and XDB17030600), and the Youth Innovation Promotion Association CAS (No. 2022030).
The authors declare that the research was conducted in the absence of any commercial or financial relationships that could be construed as a potential conflict of interest.
All claims expressed in this article are solely those of the authors and do not necessarily represent those of their affiliated organizations, or those of the publisher, the editors and the reviewers. Any product that may be evaluated in this article, or claim that may be made by its manufacturer, is not guaranteed or endorsed by the publisher.
Amin, M., Badawi, A., and Obayya, S. S. (2016). Radiation damage in XFEL: case study from the oxygen-evolving complex of photosystem II. Sci. Rep. 6:36492. doi: 10.1038/srep36492
Andreiadis, E. S., Chavarot-Kerlidou, M., Fontecave, M., and Artero, V. (2011). Artificial photosynthesis: from molecular catalysts for light-driven water splitting to photoelectrochemical cells. Photochem. Photobiol. 87, 946–964. doi: 10.1021/acs.accounts.6b00405
Askerka, M., Brudvig, G. W., and Batista, V. S. (2017). The O2-evolving complex of photosystem II: recent insights from quantum mechanics/molecular mechanics (QM/MM), extended X-ray absorption fine structure (EXAFS), and femtosecond X-ray crystallography data. Acc. Chem. Res. 50, 41–48. doi: 10.1021/acs.biochem.5b00089
Askerka, M., Vinyard, D. J., Wang, J., Brudvig, G. W., and Batista, V. S. (2015). Analysis of the radiation-damage-free X-ray structure of photosystem II in light of EXAFS and QM/MM data. Biochemistry 54, 1713–1716. doi: 10.1021/acs.biochem.5b00089
Avramov, A. P., Hwang, H. J., and Burnap, R. L. (2020). The role of Ca2+ and protein scaffolding in the formation of nature’s water oxidizing complex. Proc. Natl. Acad. Sci. U. S. A. 117, 28036–28045. doi: 10.1073/pnas.2011315117
Bao, H., and Burnap, R. L. (2016). Photoactivation: The light-driven assembly of the water oxidation complex of photosystem II. Front. Plant Sci. 7:578. doi: 10.3389/fpls.2016.00578
Barber, J. (2009). Photosynthetic energy conversion: natural and artificial. Chem. Soc. Rev. 38, 185–196. doi: 10.1039/b802262n
Barber, J. (2016). Mn4Ca cluster of photosynthetic oxygen-evolving center: structure, function and evolution. Biochemistry 55, 5901–5906. doi: 10.1021/acs.biochem.6b00794
Barber, J. (2017). A mechanism for water splitting and oxygen production in photosynthesis. Nat. Plants 3:17041. doi: 10.1038/nplants.2017.41
Barber, J. (2020). Solar-driven water-splitting provides a solution to the energy problem underpinning climate change. Biochem. Soc. Trans. 48, 2865–2874. doi: 10.1042/BST20200758
Barber, J., and Andersson, B. (1992). Too much of good things: light can be bad for photosynthesis. Trends Biochem. Sci. 17, 61–66. doi: 10.1126/science.1200165
Blankenship, R. E. (2021). Molecular Mechanisms of Photosynthesis. Malden, USA: Blackwell Science Ltd.
Blankenship, R. E., Tiede, D. M., Barber, J., Brudvig, G. W., Fleming, G., Ghirardi, M., et al. (2011). Comparing photosynthetic and photovoltaic efficiencies and recognizing the potential for improvement. Science 332, 805–809. doi: 10.1126/science.1200165
Boussac, A., Rappaport, F., Carrier, P., Verbavatz, J. M., Gobin, R., Kirilovsky, D., et al. (2004). Biosynthetic Ca2+/Sr2+ exchange in the photosystem II oxygen-evolving enzyme of Thermosynechococcus elongatus. J. Biol. Chem. 279, 22809–22819. doi: 10.1074/jbc.M401677200
Britt, R. D., and Marchiori, D. A. (2019). Photosystem II, poised for O2 formation. Science 366, 305–306. doi: 10.1126/science.aaz4522
Brown, I. D. (2009). Recent developments in the methods and applications of the bond valence model. Chem. Rev. 109, 6858–6919. doi: 10.1021/cr900053k
Capone, M., Narzi, D., and Guidoni, L. (2021). Mechanism of oxygen evolution and Mn4CaO5 cluster restoration in the natural water-oxidizing catalyst. Biochemistry 60, 2341–2348. doi: 10.1021/acs.biochem.1c00226
Cardona, T., Sedoud, A., Cox, N., and Rutherford, A. W. (2012). Charge separation in photosystem II: A comparative and evolutionary overview. Biochim. Biophys. Acta 1817, 26–43. doi: 10.1016/j.bbabio.2011.07.012
Chang, W., Chen, C., Dong, H., and Zhang, C. (2017). Artificial Mn4-oxido complexes mimic the oxygen-evolving center in photosynthesis. Sci. Bull. 62, 665–668. doi: 10.1016/j.scib.2017.04.005
Chen, C., Chen, Y., Yao, R., Li, Y., and Zhang, C. (2019). Artificial Mn4Ca clusters with exchangeable solvent molecules mimicking the oxygen-evolving center in photosynthesis. Angew. Chem. Int. Ed. 58, 3939–3942. doi: 10.1002/anie.201814440
Chen, Q. F., Guo, Y. H., Yu, Y. H., and Zhang, M. T. (2021). Bioinspired molecular clusters for water oxidation. Coord. Chem. Rev. 448:214164. doi: 10.1016/j.ccr.2021.214164
Chen, C., Li, Y., Zhao, G., Yao, R., and Zhang, C. (2017). Natural and artificial Mn4Ca cluster for the water splitting reaction. Chem. Sus. Chem 10, 4403–4408. doi: 10.1002/cssc.201701371
Chen, C., Xu, B., Yao, R., Chen, Y., and Zhang, C. (2022). Synthesizing mechanism of the Mn4Ca-cluster mimicking the oxygen-evolving center in photosynthesis. Chem. Sus. Chem. 15:e202102661. doi: 10.1002/cssc.202102661
Chen, C., and Zhang, C. (2021). Mn4Ca-cluster: photosynthetic water-splitting catalyst. Comprehensive Coordination Chemistry III 2, 454–465. doi: 10.1016/B978-0-12-409547-2.14830-9
Chen, C., Zhang, C., Dong, H., and Zhao, J. (2014). A synthetic model for the oxygen-evolving complex in Sr2+-containing photosystem II. Chem. Commun. 50, 9263–9265. doi: 10.1039/c4cc02349h
Chen, C., Zhang, C., Dong, H., and Zhao, J. (2015). Artificial synthetic MnIVCa-oxido complexes mimic the oxygen-evolving complex in photosystem II. Dalton Trans. 44, 4431–4435. doi: 10.1039/c4dt03459g
Cheniae, G. M., and Martin, I. F. (1971). Photoactivation of the manganese catalyst of O2 evolution. I. Biochemical and kinetic aspects. Biochim. Biophys. Acta 253, 167–181. doi: 10.1016/0005-2728(71)90242-8
Concepcion, J. J., House, R. L., Papanikolas, J. M., and Meyer, T. J. (2012). Chemical approaches to artificial photosynthesis. Proc. Natl. Acad. Sci. U. S. A. 109, 15560–15564. doi: 10.1073/pnas.1212254109
Corry, T. A., and O’Malley, P. J. (2018). Evidence of O-O bond formation in the final metastable S3 state of nature’s water oxidizing complex implying a novel mechanism of water oxidation. J. Phys. Chem. Lett. 9, 6269–6274. doi: 10.1021/acs.jpclett.8b02793
Cox, N., Pantazis, D. A., and Lubitz, W. (2020). Current understanding of the mechanism of water oxidation in photosystem II and its relation to XFEL data. Annu. Rev. Biochem. 89, 795–820. doi: 10.1146/annurev-biochem-011520104801
Cox, N., Pantazis, D. A., Neese, F., and Lubitz, W. (2014a). Biological water oxidation. Acc. Chem. Res. 46, 1588–1596. doi: 10.1021/ar3003249
Cox, N., Retegan, M., Neese, F., Pantazis, D. A., Boussac, A., and Lubitz, W. (2014b). Electronic structure of the oxygen-evolving complex in photosystem II prior to O-O bond formation. Science 345, 804–808. doi: 10.1126/science.1254910
Dasgupta, J., Ananyev, G. M., and Dismukes, G. C. (2008). Photoassembly of the water-oxidizing complex in photosystem II. Coord. Chem. Rev. 252, 347–360. doi: 10.1016/j.ccr.2007.08.022
Dau, H., Grundmeier, A., Loja, P., and Haumann, M. (2008). On the structure of the manganese complex of photosystem II: extended-range EXAFS data and specific atomic-resolution models for four S-states. Phil. Trans. R. Soc. Lond. B 363, 1237–1244. doi: 10.1098/rstb.2007.2220
Dau, H., and Haumann, M. (2007). Eight steps preceding O-O bond formation in oxygenic photosynthesis—A basic reaction cycle of the photosystem II manganese complex. Biochim. Biophys. Acta 1767, 472–483. doi: 10.1016/j.bbabio.2007.02.022
Dau, H., and Haumann, M. (2008). The manganese complex of photosystem II in its reaction cycle—basic framework and possible realization at the atomic level. Coord. Chem. Rev. 252, 273–295. doi: 10.1016/j.ccr.2007.09.001
Dau, H., and Zaharieva, I. (2009). Principles, efficiency and blueprint character of solar-energy conversion in photosynthetic water oxidation. Acc. Chem. Res. 42, 1861–1870. doi: 10.1021/ar900225y
Davis, K. M., and Pushkar, Y. N. (2015). Structure of the oxygen evolving complex of photosystem II at room temperature. J. Phys. Chem. B 119, 3492–3498. doi: 10.1021/acs.jpcb.5b00452
Davis, K. M., Sullivan, B. T., Palenik, M. C., Yan, L., Purohit, V., Robison, G., et al. (2018). Rapid evolution of the photosystem II electronic structure during water splitting. Phys. Rev. X. 8:041014. doi: 10.1103/PhysRevX.8.041014
Debus, R. J. (1992). The manganese and calcium ions of photosynthetic oxygen evolution. Biochim. Biophys. Acta 1102, 269–352. doi: 10.1016/0167-4838(92)90520-N
Diner, B. A., and Britt, R. D. (2005). “The redox-active Tyrosines YZ and YD,” in Photosystem II: The Light-Driven Water: Plastoquinone Oxidoreductase. eds. T. J. Wydrzynski and K. Satoh (Dordrecht, The Netherlands: Springer), 207–233.
Dismukes, G. C., Brimblecombe, R., Felton, G. A. N., Pryadun, R. S., Sheats, J. E., Spiccia, L., et al. (2009). Development of bioinspired Mn4O4-cubane water oxidation catalysts: lessons from photosynthesis. Acc. Chem. Res. 42, 1935–1943. doi: 10.1021/ar900249x
El-Khouly, M. E., El-Mohsnawy, E., and Fukuzumi, S. (2017). Solar energy conversion: From natural to artificial photosynthesis. J Photochem Photobiol C: Photochem Rev 31, 36–83. doi: 10.1016/j.jphotochemrev.2017.02.001
Ezhov, R., Ravari, A. K., Bury, G., Smith, P. F., and Pushkar, Y. (2021). Do multinuclear 3d metal catalysts achieve O–O bond formation via radical coupling or via water nucleophilic attack? WNA leads the way in [Co4O4]n+. Chem Catalysis 1, 407–422. doi: 10.1016/j.checat.2021.03.013
Faunce, T. A., Lubitz, W., Rutherford, A. W., MacFarlane, D., Moore, G. F., Yang, P., et al. (2013). Energy and environment policy case for a global project on artificial photosynthesis. Energy Environ. Sci. 6, 695–698. doi: 10.1039/c3ee00063j
Ferreira, K. N., Iverson, T. M., Maghlaoui, K., Barber, J., and Iwata, S. (2004). Architecture of the photosynthetic oxygen-evolving center. Science 303, 1831–1838. doi: 10.1126/science.1093087
Gatt, P., Petrie, S., Stranger, R., and Pace, R. J. (2012). Rationalizing the 1.9 Å crystal structure of photosystem II — A remarkable Jahn-teller balancing act induced by a single proton transfer. Angew. Chem. Int. Ed. 51, 12025–12028. doi: 10.1002/anie.201206316
Gerey, B., Goure, E., Fortage, J., Pecaut, J., and Collomb, M. N. (2016). Manganese-calcium/strontium heterometallic compounds and their relevance for the oxygen-evolving center of photosystem II. Coord. Chem. Rev. 319, 1–24. doi: 10.1016/j.ccr.2016.04.002
Gisriel, C. J., Wang, J., Liu, J., Flesher, D. A., Reiss, K. M., Huang, H. L., et al. (2022). High-resolution cryo-electron microscopy structure of photosystem II from the mesophilic cyanobacterium, Synechocystis sp. PCC 6803. Proc. Natl. Acad. Sci. U. S. A. 119:e2116765118. doi: 10.1073/pnas.2116765118
Gisriel, C. J., Zhou, K., Huang, H. L., Debus, R. J., Xiong, Y., and Brudvig, G. W. (2020). Cryo-EM structure of monomeric photosystem II from synechocystis sp. PCC 6803 lacking the water-oxidation complex. Joule 4, 2131–2148. doi: 10.1016/j.joule.2020.07.016
Govindjee, G., Shevela, D., and Björn, L. O. (2017). Evolution of the Z-scheme of photosynthesis: a perspective. Photosyn. Res. 133, 5–15. doi: 10.1007/s11120-016-0333-z
Grabolle, M., Haumann, M., Müller, C., Liebisch, P., and Dau, H. (2006). Rapid loss of structural motifs in the manganese complex of oxygenic photosynthesis by X-ray irradiation at 10-300K. J. Biol. Chem. 281, 4580–4588. doi: 10.1074/jbc.M509724200
Graça, A. T., Hall, M., Persson, K., and Schröder, W. P. (2021). High-resolution model of Arabidopsis photosystem II reveals the structural consequences of digitonin-extraction. Sci. Rep. 11:15534. doi: 10.1038/s41598-021-94914-x
Guskov, A., Kern, J., Gabdulkhakov, A., Broser, M., Zouni, A., and Saenger, W. (2009). Cyanobacterial photosystem II at 2.9-Å resolution and the role of quinones, lipids, channels and chloride. Nat. Struct. Mol. Biol. 16, 334–342. doi: 10.1038/nsmb.1559
Gust, D., Moore, T. A., and Moore, A. L. (2010). Solar fuels via artificial photosynthesis. Acc. Chem. Res. 42, 1890–1898. doi: 10.1021/ar900209b
Haumann, M., Liebisch, P., Muller, C., Barra, M., Grabolle, M., and Dau, H. (2005). Photosynthetic O2 formation tracked by time-resolved x-ray experiments. Science 310, 1019–1021. doi: 10.1126/science.1117551
Herrero, C., Lassalle-Kaiser, B., Leibl, W., Rutherford, A. W., and Aukauloo, A. (2008). Artificial systems related to light driven electron transfer processes in PSII. Coord. Chem. Rev. 252, 456–468. doi: 10.1016/j.ccr.2007.09.002
Hocking, R. K., Brimblecombe, R., Chang, L. Y., Singh, A., Cheah, M. H., Glover, C., et al. (2011). Water-oxidation catalysis by manganese in a geochemical-like cycle. Nat. Chem. 3, 461–466. doi: 10.1038/NCHEM.1049
Hunter, B. M., Gray, H. B., and Müller, A. M. (2016). Earth-abundant heterogeneous water oxidation catalysts. Chem. Rev. 116, 14120–14136. doi: 10.1021/acs.chemrev.6b00398
Hussein, R., Ibrahim, M., Bhowmick, A., Simon, P. S., Chatterjee, R., Lassalle, L., et al. (2021). Structural dynamics in the water and proton channels of photosystem II during the S2 to S3 transition. Nat. Commun. 12:6531. doi: 10.1038/s41467-021-26781-z
Ibrahim, M., Fransson, T., Chatterjee, R., Cheah, M. H., Hussein, R., Lassalle, L., et al. (2020). Untangling the sequence of events during the S2 → S3 transition in photosystem II and implications for the water oxidation mechanism. Proc. Natl. Acad. Sci. U. S. A. 117, 12624–12635. doi: 10.1073/pnas.2000529117
Junge, W. (2019). Oxygenic photosynthesis: history, status and perspective. Q. Rev. Biophys. 52:e1. doi: 10.1017/S0033583518000112
Kamiya, N., and Shen, J. R. (2003). Crystal structure of oxygen-evolving photosystem II from Thermosynechococcus vulcanus at 3.7 Å resolution. Proc. Natl. Acad. Sci. U. S. A. 100, 98–103. doi: 10.1073/pnas.0135651100
Kanady, J. S., Lin, P. H., Carsch, K. M., Nielsen, R. J., Takase, M. K., Goddard, W. A., et al. (2014). Toward models for the full oxygen-evolving complex of photosystem II by ligand coordination to lower the symmetry of the Mn3CaO4 cubane: demonstration that electronic effects facilitate binding of a fifth metal. J. Am. Chem. Soc. 136, 14373–14376. doi: 10.1021/ja508160x
Kanady, J. S., Tsui, E. Y., Day, M. W., and Agapie, T. (2011). A synthetic model of the Mn3Ca subsite of the oxygen-evolving complex in photosystem II. Science 333, 733–736. doi: 10.1126/science.1206036
Kärkäs, M. D., Verho, O., Johnston, E. V., and Åkermark, B. (2014). Artificial photosynthesis: molecular systems for catalytic water oxidation. Chem. Rev. 114, 11863–12001. doi: 10.1021/cr400572f
Kato, K., Miyazaki, N., Hamaguchi, T., Nakajima, Y., Akita, F., Yonekura, K., et al. (2021). High-resolution cryo-EM structure of photosystem II reveals damage from high-dose electron beams. Commun. Biol. 4:382. doi: 10.1038/s42003-021-01919-3
Kawashima, K., Takaoka, T., Kimura, H., Saito, K., and Ishikita, H. (2018). O2 evolution and recovery of the water-oxidizing enzyme. Nat. Commun. 9:1247. doi: 10.1038/s41467-018-03545-w
Kern, J., Chatterjee, R., Young, I. D., Fuller, F. D., Lassalle, L., Ibrahim, M., et al. (2018). Structures of the intermediates of Kok’s photosynthetic water oxidation clock. Nature 563, 421–425. doi: 10.1038/s41586-018-0681-2
Kok, B., Forbush, B., and McGloin, M. (1970). Cooperation of charges in photosynthetic O2 evolution. I. A linear four step mechanism. Photochem. Photobiol. 11, 457–475. doi: 10.1111/j.1751-1097.1970.tb06017.x
Kondo, M., Tatewaki, H., and Masaoka, S. (2021). Design of molecular water oxidation catalysts with earth-abundant metal ions. Chem. Soc. Rev. 50, 6790–6831. doi: 10.1039/d0cs01442g
Koua, F. H. M., Umena, Y., Kawakami, K., and Shen, J. R. (2013). Structure of Sr-substituted photosystem II at 2.1 Å resolution and its implications in the mechanism of water oxidation. Proc. Natl. Acad. Sci. U. S. A. 110, 3889–3894. doi: 10.1073/pnas.1219922110
Krewald, V., Neese, F., and Pantazis, D. A. (2016). Redox potential tuning by redox-inactive cations in nature’s water oxidizing catalyst and synthetic analogues. Phys. Chem. Chem. Phys. 18, 10739–10750. doi: 10.1039/c5cp07213a
Krewald, V., Retegan, M., Cox, N., Messinger, J., Lubitz, W., DeBeer, S., et al. (2015). Metal oxidation states in biological water splitting. Chem. Sci. 6, 1676–1695. doi: 10.1039/c4sc03720k
Kupitz, C., Basu, S., Grotjohann, I., Fromme, R., Zatsepin, N. A., Rendek, K. N., et al. (2014). Serial time-resolved crystallography of photosystem II using a femtosecond X-ray laser. Nature 513, 261–265. doi: 10.1038/nature13453
Li, J., Güttinger, R., Moré, R., Song, F., Wan, W., and Patzke, G. R. (2017). Frontiers of water oxidation: the quest for true catalysts. Chem. Soc. Rev. 46, 6124–6147. doi: 10.1039/c7cs00306d
Li, X. C., Li, J., and Siegbahn, P. E. M. (2020a). A theoretical study of the recently suggested MnVII mechanism for O-O bond formation in photosystem II. J. Phys. Chem. A 124, 8011–8018. doi: 10.1021/acs.jpca.0c05135
Li, H., Nakajima, Y., Nomura, T., Sugahara, M., Yonekura, S., Chan, S. K., et al. (2021). Capturing structural changes of the S1 to S2 transition of photosystem II using time-resolved serial femtosecond crystallography. IUCrJ 8, 431–443. doi: 10.1107/S2052252521002177
Li, Y., Yao, R., Chen, Y., Xu, B., Chen, C., and Zhang, C. (2020b). Mimicking the catalytic center for the water-splitting reaction in photosystem II. Catalysts 10:185. doi: 10.3390/catal10020185
Limburg, J., Vrettos, J. S., Liable-Sands, L. M., Rheingold, A. L., Crabtree, R. H., and Brudvig, G. W. (1999). A functional model for O-O bond formation by the O2-evolving complex in photosystem II. Science 283, 1524–1527. doi: 10.1126/science.283.5407.1524
Lin, P. H., Takase, M. K., and Agapie, T. (2015). Investigations of the effect of the non-manganese metal in heterometallic-oxido cluster models of the oxygen evolving complex of photosystem II: lanthanides as substitutes for calcium. Inorg. Chem. 54, 59–64. doi: 10.1021/ic5015219
Lionetti, D., Suseno, S., Tsui, E. Y., Lu, L., Stich, T. A., Carsch, K. M., et al. (2019). Effects of Lewis acidic metal ions (M) on oxygen-atom transfer reactivity of heterometallic Mn3MO4 cubane and Fe3MO(OH) and Mn3MO(OH) clusters. Inorg. Chem. 58, 2336–2345. doi: 10.1021/acs.inorgchem.8b02701
Loll, B., Kern, J., Saenger, W., Zouni, A., and Biesiadka, J. (2005). Towards complete cofactor arrangement in the 3.0Ǻ resolution structure of photosystem II. Nature 438, 1040–1044. doi: 10.1038/nature04224
Lubitz, W., Chrysina, M., and Cox, N. (2019). Water oxidation in photosystem II. Photosynth. Res. 142, 105–125. doi: 10.1007/s11120-019-00648-3
Mandal, M., Kawashima, K., Saito, K., and Ishikita, H. (2020). Redox potential of the oxygen-evolving complex in the electron transfer cascade of photosystem II. J. Phys. Chem. Lett. 11, 249–255. doi: 10.1021/acs.jpclett.9b02831
Marchiori, D. A., Debus, R. J., and Britt, R. D. (2020). Pulse EPR spectroscopic characterization of the S3 state of the oxygen-evolving complex of photosystem II isolated from synechocystis. Biochemistry 59, 4864–4872. doi: 10.1021/acs.biochem.0c00880
Mukherjee, S., Stull, J. A., Yano, J., Stamatatos, T. C., Pringouri, K., Stich, T. A., et al. (2012). Synthetic model of the asymmetric [Mn3CaO4] cubane core of the oxygen-evolving complex of photosystem II. Proc. Natl. Acad. Sci. U. S. A. 109, 2257–2262. doi: 10.1073/pnas.1115290109
Mukhopadhyay, S., Mandal, S. K., Bhaduri, S., and Armstrong, W. H. (2004). Manganese clusters with relevance to photosystem II. Chem. Rev. 104, 3981–4026. doi: 10.1021/cr0206014
Mullins, C. S., and Pecoraro, V. L. (2008). Reflections on small molecule manganese models that seek to mimic photosynthetic water oxidation chemistry. Coord. Chem. Rev. 252, 416–443. doi: 10.1016/j.ccr.2007.07.021
Murray, J. W., Rutherford, A. W., and Nixon, P. J. (2020). Photosystem II in a state of disassembly. Joule 4, 2082–2084. doi: 10.1016/j.joule.2020.09.014
Najafpour, M. M., Renger, G., Hołynska, M., Moghaddam, A. N., Aro, E. M., Carpentier, R., et al. (2016). Manganese compounds as water-oxidizing catalysts: from the natural water-oxidizing complex to nanosized manganese oxide structures. Chem. Rev. 116, 2886–2936. doi: 10.1021/acs.chemrev.5b00340
Nocera, D. G. (2017). Solar fuels and solar chemicals industry. Acc. Chem. Res. 50, 616–619. doi: 10.1021/acs.accounts.6b00615
Pace, R. J., Jin, L., and Stranger, R. (2012). What spectroscopy reveals concerning the Mn oxidation levels in the oxygen evolving complex of photosystem II: X-ray to near infra-red. Dalton Trans. 41, 11145–11160. doi: 10.1039/c2dt30938f
Pantazis, D. A. (2018). Missing pieces in the puzzle of biological water oxidation. ACS Catal. 8, 9477–9507. doi: 10.1021/acscatal.8b01928
Pantazis, D. A., Ames, W., Cox, N., Lubitz, W., and Neese, F. (2012). Two interconvertible structures that explain the spectroscopic properties of the oxygen-evolving complex of photosystem II in the S2 state. Angew. Chem. Int. Ed. 51, 9935–9940. doi: 10.1002/anie.201204705
Paul, S., Neese, F., and Pantazis, D. A. (2017). Structural models of the biological oxygen-evolving complex: achievements, insights, and challenges for biomimicry. Green Chem. 19, 2309–2325. doi: 10.1039/c7gc00425g
Pecoraro, V. L., Baldwin, M. J., Caudle, M. T., Hsieh, W. Y., and Law, N. A. (1998). A proposal for water oxidation in photosystem II. Pure Appl. Chem. 70, 925–929. doi: 10.1351/pac199870040925
Peloquin, J. M., and Britt, R. D. (2001). EPR/ENDOR characterization of the physical and electronic structure of the OEC Mn-cluster. Biochim. Biophys. Acta 1503, 96–111. doi: 10.1016/S0005-2728(00)00219-X
Perrin, D. D. (1982). Ionisation Constants of Inorganic Acids and Bases in Aqueous Solution. Oxford: Pergamon Press.
Petrie, S., Terrett, R., Stranger, R., and Pace, R. J. (2020). Rationalizing the geometries of the water oxidising complex in the atomic resolution, nominal S3 state crystal structures of photosystem II. ChemPhysChem 21, 785–801. doi: 10.1002/cphc.201901106
Petrouleas, V., and Crofts, A. R. (2005). “The iron-quinone acceptor complex,” in Photosystem II: The light-driven water: Plastoquinone Oxidoreductase. eds. T. J. Wydrzynski and K. Satoh (Dordrecht, The Netherlands: Springer), 177–206.
Pushkar, Y., Davis, K. M., and Palenik, M. C. (2018). Model of the oxygen evolving complex which is highly predisposed to O-O bond formation. J. Phys. Chem. Lett. 9, 3525–3531. doi: 10.1021/acs.jpclett.8b00800
Rappaport, F., and Diner, B. A. (2008). Primary photochemistry and energetics leading to the oxidation of the Mn4Ca cluster and to the evolution of molecular oxygen in photosystem II. Coord. Chem. Rev. 252, 259–272. doi: 10.1016/j.ccr.2007.07.016
Renger, G., and Holzwarth, A. R. (2005). “Primary electron transfer,” in Photosystem II: The light-driven water: Plastoquinone Oxidoreductase. eds. T. J. Wydrzynski and K. Satoh (Dordrecht, The Netherlands: Springer), 139–175.
Saito, K., Nakagawa, M., Mandal, M., and Ishikita, H. (2021). Role of redox-inactive metals in controlling the redox potential of heterometallic manganese-oxido clusters. Photosynth. Res. 148, 153–159. doi: 10.1007/s11120-021-00846-y
Sato, A., Nakano, Y., Nakamura, S., and Noguchi, T. (2021). Rapid-scan time-resolved ATR-FTIR study on the photoassembly of the water-oxidizing Mn4CaO5 cluster in photosystem II. J. Phys. Chem. B 125, 4031–4045. doi: 10.1021/acs.jpcb.1c01624
Shen, J. R. (2015). The structure of photosystem II and the mechanism of water oxidation in photosynthesis. Annu. Rev. Plant Biol. 66, 23–48. doi: 10.1146/annurev-arplant-050312-120129
Shevela, D., Bjorn, L., and Govindjee, G. (2019). Photosynthesis: Solar Energy for Life. Singapore: World Scientific.
Shevela, D., Eaton-Rye, J. J., Shen, J. R., and Govindjee, G. (2012). Photosystem II and the unique role of bicarbonate: A historical perspective. Biochim. Biophys. Acta 1817, 1134–1151. doi: 10.1016/j.bbabio.2012.04.003
Shevela, D., Kern, J. F., Govindjee, G., Whitmarsh, J., and Messinger, J. (2021). Photosystem II. Encyclopedia of Life Sciences. Hoboken: Wiley.
Siegbahn, P. E. M. (2013). Water oxidation mechanism in photosystem II, including oxidations, proton release pathways, O-O bond formation and O2 release. Biochim. Biophys. Acta 1827, 1003–1019. doi: 10.1016/j.bbabio.2012.10.006
Siegbahn, P. E. M. (2017). Nucleophilic water attack is not a possible mechanism for O-O bond formation in photosystem II. Proc. Natl. Acad. Sci. U. S. A. 114, 4966–4968. doi: 10.1073/pnas.1617843114
Styring, S., Sjöholm, J., and Mamedov, F. (2012). Two tyrosines that changed the world: interfacing the oxidizing power of photochemistry to water splitting in photosystem II. Biochim. Biophys. Acta 1817, 76–87. doi: 10.1016/j.bbabio.2011.03.016
Suga, M., Akita, F., Hirata, K., Ueno, G., Murakami, H., Nakajima, Y., et al. (2015). Native structure of photosystem II at 1.95Å resolution revealed by a femtosecond X-ray laser. Nature 517, 99–103. doi: 10.1038/nature13991
Suga, M., Akita, F., Sugahara, M., Kubo, M., Nakajima, Y., Nakane, T., et al. (2017). Light-induced structural changes and the site of O=O bond formation in PSII caught by XFEL. Nature 543, 131–135. doi: 10.1038/nature21400
Suga, M., Akita, F., Yamashita, K., Nakajima, Y., Ueno, G., Li, H., et al. (2019). An oxyl/oxo mechanism for oxygen-oxygen coupling in PSII revealed by an x-ray free-electron laser. Science 366, 334–338. doi: 10.1126/science.aax6998
Tanaka, A., Fukushima, Y., and Kamiya, N. (2017). Two different structures of the oxygen-evolving complex in the same polypeptide frameworks of photosystem II. J. Am. Chem. Soc. 139, 1718–1721. doi: 10.1021/jacs.6b09666
Tommos, C., and Babcock, G. T. (1998). Oxygen production in nature: A light-driven metalloradical enzyme process. Acc. Chem. Res. 31, 18–25. doi: 10.1021/ar9600188
Tsui, E. Y., and Agapie, T. (2013). Reduction potentials of heterometallic manganese–oxido cubane complexes modulated by redox-inactive metals. Proc. Natl. Acad. Sci. U. S. A. 110, 10084–10088. doi: 10.1073/pnas.1302677110
Tsui, E. Y., Kanady, J. S., and Agapie, T. (2013a). Synthetic cluster models of biological and heterogeneous manganese catalysts for O2 evolution. Inorg. Chem. 52, 13833–13848. doi: 10.1021/ic402236f
Tsui, E. Y., Tran, R., Yano, J., and Agapie, T. (2013b). Redox-inactive metals modulate the reduction potential in heterometallic manganese-oxido clusters. Nat. Chem. 5, 293–299. doi: 10.1038/nchem.1578
Umena, Y., Kawakami, K., Shen, J. R., and Kamiya, N. (2011). Crystal structure of oxygen-evolving photosystem II at a resolution of 1.9Å. Nature 473, 55–60. doi: 10.1038/nature09913
Vass, I., and Styring, S. (1991). pH-dependent charge equilibria between tyrosine-D and the S states in photosystem II. Estimation of relative midpoint redox potentials. Biochemistry 30, 830–839. doi: 10.1021/bi00217a037
Vinyard, D. J., Ananyev, G. M., and Dismukes, G. C. (2013). Photosystem II: the reaction center of oxygenic photosynthesis. Annu. Rev. Biochem. 82, 577–606. doi: 10.1146/annurev-biochem-070511-100425
Vinyard, D. J., Khan, S., and Brudvig, G. W. (2015). Photosynthetic water oxidation: binding and activation of substrate water for O-O bond formation. Faraday Discuss. 185, 37–50. doi: 10.1039/c5fd00087d
Vrettos, J. S., Limburg, J., and Brudvig, G. W. (2001). Mechanism of photosynthetic water oxidation: combining biophysical studies of photosystem II with inorganic model chemistry. Biochim. Biophys. Acta 1503, 229–245. doi: 10.1016/S0005-2728(00)00214-0
Wang, J., Armstrong, W. H., and Batista, V. S. (2021). Do crystallographic XFEL data support binding of a watermolecule to the oxygen-evolving complex of photosystem II exposed to two flashes of light? Proc. Natl. Acad. Sci. U. S. A. 118:e2023982118. doi: 10.1073/pnas.2023982118
Wang, J., Askerka, M., Brudvig, G. W., and Batista, V. S. (2017). Crystallographic data support the carousel mechanism of water supply to the oxygen-evolving complex of photosystem II. ACS Energy Lett. 2, 2299–2306. doi: 10.1021/acsenergylett.7b00750
Wei, X., Su, X., Cao, P., Liu, X., Chang, W., Li, M., et al. (2016). Structure of spinach photosystem II–LHCII supercomplex at 3.2Å resolution. Nature 534, 69–74. doi: 10.1038/nature18020
Wieghardt, K. (1989). The active-sites in manganese-containing metalloproteins and inorganic model complexes. Angew. Chem. Int. Ed. 28, 1153–1172. doi: 10.1002/anie.198911531
Xiao, Y., Huang, G., You, X., Zhu, Q., Wang, W., Kuang, T., et al. (2021). Structural insights into cyanobacterial photosystem II intermediates associated with Psb28 and Tsl0063. Nat. Plants 7, 1132–1142. doi: 10.1038/s41477-021-00961-7
Yamaguchi, K., Shoji, M., Isobe, H., Yamanaka, S., Kawakami, T., Yamada, S., et al. (2018). Theory of chemical bonds in metalloenzymes XXI. Possible mechanisms of water oxidation in oxygen evolving complex of photosystem II. Mol. Phys. 116, 717–745. doi: 10.1080/00268976.2008.1428375
Yano, J., Kern, J., Irrgang, K. D., Latimer, M. J., Bergmann, U., Glatzel, P., et al. (2005). X-ray damage to the Mn4Ca complex in single crystals of photosystem II: A case study for metalloprotein crystallography. Proc. Natl. Acad. Sci. U. S. A. 102, 12047–12052. doi: 10.1073/pnas.0505207102
Yano, J., and Yachandra, V. K. (2014). Mn4Ca-cluster in photosynthesis: where and how water is oxidized to dioxygen. Chem. Rev. 114, 4175–4205. doi: 10.1021/cr4004874
Yao, R., Li, Y., Chen, Y., Xu, B., Chen, C., and Zhang, C. (2021). Rare-earth elements can structurally and energetically replace the calcium in a synthetic Mn4CaO4-cluster mimicking the oxygen-evolving center in photosynthesis. J. Am. Chem. Soc. 143, 17360–17365. doi: 10.1021/jacs.1c09085
Ye, S., Ding, C., Liu, M., Wang, A., Huang, Q., and Li, C. (2019). Water oxidation catalysts for artificial photosynthesis. Adv. Mater. 31:1902069. doi: 10.1002/adma.201902069
Yocum, C. F. (2008). The calcium and chloride requirements of the O2 evolving complex. Coord. Chem. Rev. 252, 296–305. doi: 10.1016/j.ccr.2007.08.010
Young, I. D., Ibrahim, M., Chatterjee, R., Gul, S., Fuller, F. D., Koroidov, S., et al. (2016). Structure of photosystem II and substrate binding at room temperature. Nature 540, 453–457. doi: 10.1038/nature20161
Zhang, C. (2007). Low-barrier hydrogen bond plays key role in active photosystem II - a new model for photosynthetic water oxidation. Biochim. Biophys. Acta 1767, 493–499. doi: 10.1016/j.bbabio.2006.12.008
Zhang, C. (2015). The first artificial Mn4Ca-cluster mimicking the oxygen-evolving center in photosystem II. Sci. Chin. Life Sci. 58, 816–817. doi: 10.1007/s11427-015-4889-1
Zhang, C., Chen, C., Dong, H., Shen, J. R., Dau, H., and Zhao, J. (2015). A synthetic Mn4Ca-cluster mimicking the oxygen-evolving center of photosynthesis. Science 348, 690–693. doi: 10.1126/science.aaa6550
Zhang, C., and Kuang, T. (2018). A new milestone for photosynthesis. Natl. Sci. Rev. 5, 444–445. doi: 10.1093/nsr/nwx087
Zhang, C., Pan, J., Li, L., and Kuang, T. (1999). New structure model of oxygen-evolving center and mechanism for oxygen evolution in photosynthesis. Chin. Sci. Bull. 44, 2209–2215. doi: 10.1007/BF02885923
Zhang, J. Z., and Reisner, E. (2020). Advancing photosystem II photoelectrochemistry for semi-artificial photosynthesis. Nat. Rev. Chem. 4, 6–21. doi: 10.1038/s41570-019-0149-4
Zhang, B., and Sun, L. (2019a). Across the board: Licheng Sun on the mechanism of O-O bond formation in photosystem II. ChemSusChem 12, 3401–3404. doi: 10.1002/cssc.201901438
Zhang, B., and Sun, L. (2019b). Artificial photosynthesis: opportunities and challenges of molecular catalysts. Chem. Soc. Rev. 48, 2216–2264. doi: 10.1039/c8cs00897c
Zheng, M., and Dismukes, G. C. (1996). Orbital configuration of the valence electrons, ligand field symmetry, and manganese oxidation states of the photosynthetic water oxidizing complex: analysis of the S2 state multiline EPR signals. Inorg. Chem. 35, 3307–3319. doi: 10.1021/ic9512340
Keywords: photosystem II, oxygen-evolving center, Mn4CaO4-cluster, artificial photosynthesis, water-splitting reaction
Citation: Chen Y, Xu B, Yao R, Chen C and Zhang C (2022) Mimicking the Oxygen-Evolving Center in Photosynthesis. Front. Plant Sci. 13:929532. doi: 10.3389/fpls.2022.929532
Received: 27 April 2022; Accepted: 20 June 2022;
Published: 07 July 2022.
Edited by:
Harvey J. M. Hou, Alabama State University, United StatesReviewed by:
Mohammad Mahdi Najafpour, Institute for Advanced Studies in Basic Sciences (IASBS), IranCopyright © 2022 Chen, Xu, Yao, Chen and Zhang. This is an open-access article distributed under the terms of the Creative Commons Attribution License (CC BY). The use, distribution or reproduction in other forums is permitted, provided the original author(s) and the copyright owner(s) are credited and that the original publication in this journal is cited, in accordance with accepted academic practice. No use, distribution or reproduction is permitted which does not comply with these terms.
*Correspondence: Changhui Chen, Y2hlbmNoYW5naHVpQGljY2FzLmFjLmNu; Chunxi Zhang, Y2h1bnhpemhhbmdAaWNjYXMuYWMuY24=
†These authors have contributed equally to this work
Disclaimer: All claims expressed in this article are solely those of the authors and do not necessarily represent those of their affiliated organizations, or those of the publisher, the editors and the reviewers. Any product that may be evaluated in this article or claim that may be made by its manufacturer is not guaranteed or endorsed by the publisher.
Research integrity at Frontiers
Learn more about the work of our research integrity team to safeguard the quality of each article we publish.