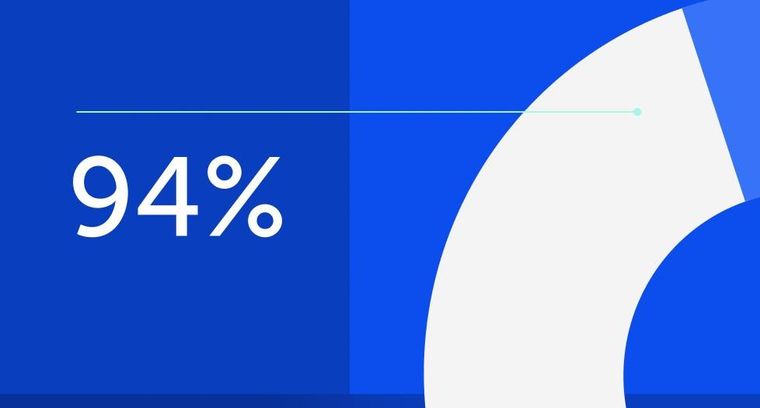
94% of researchers rate our articles as excellent or good
Learn more about the work of our research integrity team to safeguard the quality of each article we publish.
Find out more
REVIEW article
Front. Plant Sci., 27 September 2022
Sec. Functional Plant Ecology
Volume 13 - 2022 | https://doi.org/10.3389/fpls.2022.926535
This article is part of the Research TopicSilviculture and Agriculture Tree Growth Under Water Stress, Volume IIView all 5 articles
Considerable evidences highlight the occurrence of increasing widespread tree mortality as a result of global climate change-associated droughts. However, knowledge about the mechanisms underlying divergent strategies of various tree species to adapt to drought has remained remarkably insufficient. Leaf stomatal regulation and embolism resistance of stem xylem serves as two important strategies for tree species to prevent hydraulic failure and carbon starvation, as comprising interconnected physiological mechanisms underlying drought-induced tree mortality. Hence, the physiological and anatomical determinants of leaf stomatal regulation and stems xylem embolism resistance are evaluated and discussed. In addition, root properties related to drought tolerance are also reviewed. Species with greater investment in leaves and stems tend to maintain stomatal opening and resist stem embolism under drought conditions. The coordination between stomatal regulation and stem embolism resistance are summarized and discussed. Previous studies showed that hydraulic safety margin (HSM, the difference between minimum water potential and that causing xylem dysfunction) is a significant predictor of tree species mortality under drought conditions. Compared with HSM, stomatal safety margin (the difference between water potential at stomatal closure and that causing xylem dysfunction) more directly merge stomatal regulation strategies with xylem hydraulic strategies, illustrating a comprehensive framework to characterize plant response to drought. A combination of plant traits reflecting species’ response and adaptation to drought should be established in the future, and we propose four specific urgent issues as future research priorities.
Forests, as the dominant biomes of the global land, plays a crucial role in the biogeochemical cycle (Pan et al., 2011). A great amount of evidences highlights increasing forest mortality as a result of global climate change-associated severe drought, and such mortality can lead to large-scale detrimental effect on ecosystem structure and function, causing a conversion from the forests carbon sink into carbon source (Phillips et al., 2009; Hartmann et al., 2018). Global observations of drought-induced forest dieback have stressed a need to understand and predict the vulnerability of trees to more frequent drought in the future.
The mechanisms underlying divergent strategies of tree species to drought have remained remarkably difficult to study, leading to some uncertainty in forecasting the future of forests in the world (Arend et al., 2021). Moreover, differences among tree species in drought adaptability are also integral determinants of distributions and the probability of extinctions in the future (Bonan, 2008). At present, two interconnected physiological reasons underlying drought-induced tree death have evolved, hydraulic failure and carbon starvation (McDowell et al., 2008; McDowell, 2011; Choat et al., 2018; Brodribb et al., 2020).
Based on the cohesion-tension theory, water in plants is transported from the soil through the plant to the crown along a gradient of negative pressure (tension) in the conduits (tracheids or vessels) of the xylem (McDowell et al., 2008; Trugman et al., 2021). Nevertheless, during transpiration, embolism can occur when the pressure in the conduits becomes negative enough to cause air entry through pits into neighboring conduits (Tyree and Zimmermann, 2002). The conduit will become incapable of water transport if embolism occurs (Tyree and Zimmermann, 2002). The hydraulic failure is that drought-induced xylem embolism stops water flow, and subsequently plant tissues desiccate (McDowell et al., 2008). Therefore, tree’ xylem embolism resistance serves as an important strategy to prevent hydraulic failure (Pivovaroff et al., 2016). Another important hydraulic strategy for trees to cope with drought is the closure of stomata. The CO2 used for photosynthesis and the water lost in transpiration share the common pathway—the stomatal pores on leaf surfaces, and reduced transpiration through closuring stomata is inevitably accompanied by the expense of carbon gain. The term “carbon starvation” is that drought induced-stomatal closure (even leaf shedding) causes carbohydrates supply to drop off and the continued carbohydrates metabolism or even the impaired phloem conductance leads to plant tissues starve (McDowell et al., 2008; McDowell, 2011).
Therefore, xylem embolism resistance and stomatal regulation are closely related to hydraulic failure and carbon starvation. In other words, the risk of tree mortality is generally determined by the lethal thresholds of carbon depletion and/or hydraulic failure to which plants are exposed during drought. The lethal thresholds are mainly the thresholds of embolism resistance and stomatal regulation (McDowell et al., 2008; Choat et al., 2018; Blackman et al., 2019; Brodribb et al., 2020). In fact, previous studies have indeed shown that drought-induced mortality of a given species can be predicted by hydraulic thresholds (xylem pressure at which 50% loss of hydraulic conductance, P50, a hydraulic trait widely used to estimate hydraulic safety) and declining carbon availability (Choat et al., 2012; Anderegg et al., 2016; Anderegg et al., 2019 Chen et al., 2019a).
The water is limited in the soil, and the ability of terrestrial plants to resist embolism and regulate stoma is limited. A conifer species from arid regions of Western Australia, Callitris tuberculata, is the most embolism resistant tree species in the world to date (P50 = -18.8 MPa) (Larter et al., 2015). In addition, stomatal regulation is also a requisite condition for land plants evolved to adapt to the terrestrial environment and it is impossible that endless water from the soil to be transpired into the atmosphere. Therefore, tree species with different adaptation strategies are screened and survived in different environments, which also achieves colorful biodiversity in terrestrial environments with various moisture conditions.
In this review, we evaluate and discuss the physiological and anatomical determinant of xylem embolism resistance and leaf stomatal regulation. The coordination and trade-offs of the two drought adaptation strategies are also summarized. Root, as important organ for absorbing water and nutrients, and its coordination with leaf and stem are also reviewed. In addition, research prospects of trees’ drought adaptation strategies in the future are proposed.
Based on fossil record, stomata appear approximately 400 million years ago (Edwards et al., 1998; Brodribb and Holbrook, 2003). The first published stomatal measurement was about stomatal density (Sack and Buckley, 2016), and the recognition that stomata open and close by changes in guard cell turgor was in 1938 (Heath, 1938). Although the total area of stomatal pores occupies only a small fraction of the leaf surface, typically less than 3%, 98% of H2O and CO2 passes through these pores (Lawson and Blatt, 2014). The evolution of stoma is believed to be caused by the selective pressure of optimizing the ratio of CO2 uptake to water lost during gas exchange (Raven, 2002; Brodribb and Holbrook, 2003). When plants experience drought, stomatal closure will be the first response of plant to reduce transpiration (Bartlett et al., 2016).
Stomal opening or closing is controlled by inflating or deflating stomatal guard cells driven by influx or efflux of water, whose water potential is associated with chemical osmolytes or signals (such as pH, ABA, H+, K+, Cl2 and ethephon, etc.) and leaf water potential (Brodribb et al., 2014; Medeiros et al., 2015; Papanatsiou et al., 2019). Therefore, water potential plays a key role in determining stomatal movements. In addition, guard cell size (and geometry) also tends to affect the speed of stomatal movement, and larger stomata often exhibit slower responses (Lawson and Blatt, 2014). In this review, the summarization and discussion will focus on the effect of water status on stomatal opening and closure.
Significant variation in sensitivity and responsiveness of the opening and closing of stomata to external environmental and internal signaling cues to exist among species (Lawson and Blatt, 2014). Further, the species-specific differences in the relationship between stomatal behavior and dynamic variation in water conditions are important for understanding the species-specific differences in the performance of response and adaptation to the environment (Meinzer et al., 2016). Frequently, across species, stomatal regulation is classified along a continuum from isohydry to anisohydry (Brodribb and McAdam, 2013; Brodribb et al., 2014; Skelton et al., 2015; Meinzer et al., 2016; Chen et al., 2021b; Hartmann et al., 2021). This classification existed before the water potential concept (Slatyer and Taylor, 1960; Scholander et al., 1965) and initially focused more on the regulation of transpiration than on the maintaining stable leaf water potential (Ψleaf) per se (Martínez-Vilalta and Garcia-Forner, 2017). Now the definition of iso/anisohydric is usually based on Ψleaf, and anisohydric species is generally attributed to less stomatal sensitivity, allowing large variation in Ψleaf; isohydric species typically exhibit strong stomatal regulation, leading to relatively stable Ψleaf (Tardieu and Simonneau, 1998; Klein, 2014; Meinzer et al., 2017).
Three methods are generally adopted for the quantification of iso/anisohydric behavior: (1) the relationship between stomatal conductance (Gs) and Ψleaf; (2) the relationship between predawn water potential (Ψpd) and midday water potential (Ψmd); (3) the “hydroscape” method.
The quantification of iso/anisohydric behavior by definition refers to ascertain the sustainability of Gs in the decrease of Ψleaf during drought, so the water potential at stomatal closure (Ψclose) obtained by the Gs-Ψleaf curve is a suitably quantitative indicator of iso/anisohydric behavior (Brodribb and Holbrook, 2003; Skelton et al., 2015; Chen et al., 2021b; Bartlett and Sinclair, 2021a). This Gs-Ψleaf curve method need to measure a wide enough range of soil moisture or water potential and relevant Gs to construct intact curve for obtaining accurate Ψclose. In addition, Gs is also influenced by CO2 concentration, illumination, air temperature, etc., so these interference factors on Gs must be excluded as much as possible during the measurement. By analyzing Ψclose, many studies found that species-specific Ψclose formed a continuum, not simply dichotomy between isohydric and anisohydric (Klein, 2014; Martin-StPaul et al., 2017; Pivovaroff et al., 2018; Chen et al., 2019a; Henry et al., 2019). In a study of 20 co-occurring temperate broadleaf tree species, Ψclose was found to be in the range from -0.655 MPa to -5.54 MPa (Chen et al., 2019a). In a meta-analysis study gathered data from more than 100 species in different biomes, Ψclose varied from -1 to -4.3 MPa (Martin-StPaul et al., 2017). Noticeably, in a study conducted in southern California with a Mediterranean-type climate, Ψclose was found to be about -10 MPa in a chaparral species (Pivovaroff et al., 2018).
More isohydric species show less variability in Ψmd, but Ψmd of anisohydric tree species present oppositely (Martínez-Vilalta and Garcia-Forner, 2017). Based on this definition, Martínez-Vilalta et al. (2014) classified the stomatal regulation behaviors of plants by the decline range of Ψmd with the decrease of Ψpd, i.e., the slope (σ) of the model. However, σ vary with soil water potential, which highlights that confirming iso/anisohydric regulation on the basis of differences between predawn and midday water potential is problematic (Hochberg et al., 2018). Furthermore, changes in Ψmd and Ψpd are also affected by hydraulic conductance and Gs, and the reductions in both have the opposite effects on Ψleaf (Martínez-Vilalta and Garcia-Forner, 2017). Thus, considering the large effect of the environment, for proper the degree of iso-anisohydry of the species’ genotypic effect, basic physiological characteristics (such as Ψclose or its proxy, turgor loss point) should be adopted rather than the degree of relationship between Ψpd and Ψmd (Hochberg et al., 2018).
Meinzer et al. (2016) coined a new metric, the “hydroscape”, to define tree species’ isohydricity. The hydroscape is a two-dimensional metric, defined as the area enclosed by the Ψpd and Ψmd regression lines, and species with larger hydroscapes are more anisohydric. The hydroscape integrates more comprehensive information and more accurately quantifies the iso/anisohydric degree than simple metrics such as σ (Meinzer et al., 2016). Furthermore, the strong correlation between hydroscape and hydraulic traits also suggests that the hydroscape may provide more information about overall drought tolerance than metrics involving the rate of change in Ψmd as Ψpd declines (Meinzer et al., 2016; Li et al., 2019).
In addition to the three mentioned main methods, turgor loss point (TLP) was also regarded as a potential proxy for species stomatal regulation strategy (Hochberg et al., 2018), which is directly and closely linked with stomatal regulation (Brodribb and Holbrook, 2003; Chen et al., 2021b; Figure 1).
Figure 1 The relationship between turgor loss point and the water potential at stomatal closure of 25 angiosperm speices. The data comes from Chen et al. (2021b) and Li et al. (2015).
Regardless of how iso/anisohydric behavior is defined, it has been shown to be associated with a variety of stem and leaf traits (Fu et al., 2019; Chen et al., 2021b). The determinants or relevant traits of stomatal behavior are mainly phytohormone ABA, hydraulic traits and structural investment as discussed below.
ABA can cause a reduction in guard cell turgor, subsequently closing stomata (Brodribb and McAdam, 2013). However, the earliest stomatal response was an passive-hydraulic regulation without the requirement of ABA. This can be found in the extant species of basal vascular plants, including the lycophytes, ferns, and gymnosperms in evolutionary terms (McAdam and Brodribb, 2015). The passive-hydraulic regulation in these species show that stomata are like valves closing or opening in response to, respectively, a decrease or increase in turgor (McAdam and Brodribb, 2015).
The traditional studies suggest that ABA is a root-related hormone which is transported to the foliage through the stem xylem (Davies and Zhang, 1991), but short-term responses of ABA cannot be made clear by xylem transport (Lee et al., 2006). Now mounting molecular evidence illustrates the capacity of foliage and even guard cells to generate ABA (Bauer et al., 2013), and the ABA concentration may increase after short-term exposure of foliage to drought (Waadt et al., 2014; McAdam and Brodribb, 2015). In addition, the vascular tissues, including the phloem cells, can also synthesize ABA and transport ABA to the guard cells (Okamoto et al., 2009; Kuromori et al., 2014).
Tardieu and Simonneau (1998) proposed that qualitative differences in ABA may be the source of the differences between anisohydric and isohydric plants. Among conifers, contrasting iso/anisohydric behaviors were found to be related to divergent trends in xylem embolism resistance and foliar ABA dynamics during drought, with a functional phylogeny type (Brodribb et al., 2014). The rise in ABA in the xylem or leaf can activate anion channels, causing a reduction of guard cell turgor and subsequent stomatal closure. This mode fits with some species, whose stomata were sensitive to ABA as drought stress intensified, but not fit with other species (Brodribb and McAdam, 2013).
The physiological evidences from 42 species of conifers showed that the evolutionary mechanism of stomatal regulation of gymnosperm species follows two distinct pathways (Brodribb et al., 2014). In gymnosperm species with isohydric behavior, foliar ABA levels rapidly rise during drought stress exposure, and high levels of foliar ABA induce complete stomatal closure at relatively high water potential (ABA-rising type) (Brodribb et al., 2014; Kollist et al., 2014). However, in some gymnosperm species with anisohydric behavior, stomatal initial closure is induced by increased ABA and then by a transition to water potential during drought, and the stomata could only be completely closed when the negative water potential pull the guard cell turgor low enough (Brodribb and McAdam, 2013; Brodribb et al., 2014). The similar results have been also obtained in angiosperms, and thus the relationship between ABA dynamics and iso/anisohydric behaviors may be commonplace in woody plants (Brodribb and McAdam, 2013; Nolan et al., 2017). Given that ABA is likely to be an important determinant of stomatal regulation type, the iso/anisohydric behaviors may be regulated by relatively few genes, namely those regulating ABA catabolism or synthesis (Brodribb and McAdam, 2013; Brodribb and McAdam, 2015).
Around the plant water use strategy of maximizing carbon gain and minimizing water loss, there are a set of hydraulic traits coordinated with iso/anisohydric behavior, which is not only the consequence of hydraulic regulation but also related to costs and gains.
Stomatal behavior directly affects plant water potential, and in previous research minimum midday water potential was strongly correlated with Ψclose, suggesting that Ψclose crucially affects water stress the leaves experience (Bartlett et al., 2016). TLP is an important trait reflecting drought stress tolerance of tree species (Zhu et al., 2018), and stomatal behavior is largely determined by leaf turgor (Figure 1). Lenz et al. (2006) found that TLP was more negative in species inhabited in lower rainfall sites and whose diurnal fluctuations in water potential were larger. Other leaf pressure–volume (P-V) traits, such as relative water content at TLP (RWCtlp) and osmotic potential at full turgor (Ψ100), were found to be strongly related to TLP and consequentially Ψclose. Meinzer et al. (2016) demonstrated that TLP and Ψ100 could serve as robust proxies for a species’ location along the iso/anisohydry continuum within a diverse group of woody species studied under similar conditions. Estimates of TLP and Ψ100 derived from osmometer measurement method could further rapidly streamline rankings of species’ iso/anisohydric stringency (Bartlett et al., 2012b).
The TLP is controlled by the accumulation of actively osmotic solutes (Kozlowski and Pallardy, 2002). A meta-analysis study found that Ψ100 was the main determinant of TLP (Bartlett et al., 2012a). In addition to Ψ100, the TLP is always related to the leaf modulus of elasticity (ϵ) (Meinzer et al., 2014). More negative Ψ100 and greater ϵ can contribute to the turgor maintenance as water content and water potential declines, which can maintain leaf physiological activity by maintaining protoplast volume, thus extending stomatal opening and photosynthesis with drying conditions (Meinzer et al., 2014; Johnson et al., 2018). Because stomata generally close around the TLP, stomata rarely closing at very negative water potential may reflect the finiteness of osmotic adjustment and the inability of foliage to maintain turgor at very negative Ψleaf (Bartlett et al., 2012a; Henry et al., 2019). Therefore, the position of species along the isohydric to anisohydric continuum would be scaled with species-specific variation in foliage osmotic properties (Meinzer et al., 2016; Hartmann et al., 2021).
The contribution of highly negative osmotic potentials to maintaining turgor is equivalent to more investment in high concentrations of compatible solutes (Johnson et al., 2018; Hartmann et al., 2021). The leaves of isohydric species would not undergo osmoregulation to maintain turgor during drought, which would decrease energy costs related to solute accumulation (Meinzer et al., 2014; Hartmann et al., 2021). However, excessive solute accumulation may result in the deleterious effects on leaf protein activity, which lead to a physiological limit to stomata opening, so osmotic potential is predicted to below the values of Ψclose (Martin-StPaul et al., 2017).
Stomatal conductance is directly linked to leaf hydraulic conductance (Kleaf), and stomatal closure have been shown to be related to a loss of Kleaf, aiming to prevent leaf desiccation (Brodribb and Holbrook, 2003; Woodruff et al., 2015). The coordination between Ψleaf50 (Ψleaf at 50% loss of conductivity) and TLP suggests that decline in Kleaf under drought should partially be a result of mesophyll cells losing turgor and shrinking (Scoffoni et al., 2014). Species with more negative TLP undergo less cell shrinkage and have slower declines in Kleaf during drought (Bartlett et al., 2016). Therefore, the Kleaf of more anisohydric species may be less vulnerable to drought (Nardini and Luglio, 2014), and more isohydric species were found to have lower hydraulic capacitance of leaves but higher Kleaf (Fu et al., 2019). Water storage through capacitance is also a factor in Ψclose (Hammond and Adams, 2019). Nevertheless, there is no consistent conclusion on the relationship between Ψclose and maximum Gs (Meinzer et al., 2017; Henry et al., 2019). Foliar water uptake was found to be an important water acquisition mechanism that can mitigate water deficits in some tree species, but the role of foliar water uptake on stomatal regulation remains unknown (Eller et al., 2013).
Stomatal behavior is regulated not only by water potential but also by structural adaptations that impact the demand and supply for water. Structural traits of leaf or stem such as vein density, specific leaf area (SLA), and wood density are essential to the exploring differential stomatal behaviors among species, which show the tradeoff between investment and stress tolerance (Figure 2).
Figure 2 The relationships between the iso/anisohydric behavior and functional traits of leaves and branches, which show how these traits vary with the Ψclose. The anisohydric species show stronger stress resistance, with greater investment in stems and leaves than the isohydric species to maintain stomatal opening under drought conditions. Ψclose (water potential at stomatal closure), TLP (turgor loss point), Ψ100 (osmotic potential at full turgor), RWCtlp (relative water content at TLP), Kleaf (leaf hydraulic conductance). This figure is changed from Chen et al. (2021).
Water flows from the petiole through leaf conduits that form a network of veins (McKown et al., 2010). However, vein ramification is costly in terms of material investment. Vein density was found to be significantly positively correlated with leaf carbon content and the degree of iso/anisohydry, which indicated that anisohydric tree species invested more carbon in leaves than did isohydric species (Chen et al., 2021b).
Specific leaf area (SLA) is the leaf area per unit of dry-mass investment, indicating high SLA requires less investment per unit area. More anisohydric plant species tend to have lower SLA (Pivovaroff et al., 2018; Chen et al., 2021b), and Ψclose was found to be significantly related to leaf carbon content (Chen et al., 2021b). Wood density is an integrative trait and a direct manifestation of investment in wood, which was found to be correlated with the stringency of iso/anisohydry (Klein, 2014; Fu and Meinzer, 2019; Fu et al., 2019). Therefore, it can be concluded that the anisohydric species showed greater investment in foliage and stem than the isohydric species (Chen et al., 2021b; Figure 2).
Seasonal adjustment in TLP was observed in lianas (Maréchaux et al., 2017), and leaf-level osmotic adjustment was observed in long-term drought experiment (Binks et al., 2016). Adjustments in ϵ have been also documented in the season (Meinzer et al., 2014). A more anisoyhdric species was observed that adjusted TLP during drought, whereas a co-occurring more isohydric species did not (Nolan et al., 2017). Meinzer et al. (2014) observed that the more anisohydric species had more negative TLP and Ψ100 with decreasing water availability, but TLP and Ψ100 in the more isohydric species did not change. In a anisohydric desert shrub, Larrea tridentata, TLP was observed to vary by up to 2 MPa over short periods (Meinzer et al., 1988; Johnson et al., 2018).
Anisohydric species may have to possess the plasticity in leaf P-V traits to compensate that their relatively limited stomatal regulation of maintaining stable leaf water potential (Meinzer et al., 2014; Johnson et al., 2018). In addition to P-V traits, the Ψleaf50 of ansiohydric species also alters during the driest part of the summer (Johnson et al., 2018). Thus, it is reasonable to speculate that anisohydric species may have greater capacities to alter leaf biophysical properties, and individuals of some species may switch between isohydric and anisohydric behavior within species depending on developmental stages and environmental conditions (Meinzer et al., 2014; Meinzer et al., 2016; Johnson et al., 2018).
During a paleoclimatic crisis, hydraulic systems evolved under selective pressure to be highly resistant to embolism as increasing drought levels (Larter et al., 2017; Martin-StPaul et al., 2017), and species have evolved broad differences in embolism resistance under this strong selection (Choat et al., 2012).
Xylem safety threshold is the point in which a tree starts losing control over its hydraulic system. When the water potential is out of the safety threshold, continuing residual water loss can no longer be compensated by water uptake from the soil. Therefore, embolism resistance is one of the key traits responsible for predicting the drought tolerance of tree species (Brodribb and Cochard, 2009; Anderegg et al., 2016; Choat et al., 2018; Brodribb et al., 2020).
P50 is often used to characterize the xylem safety of trees (Brodribb and Cochard, 2009; Choat et al., 2012). When water potential is below P50, the water transport function will be markedly impaired (Choat et al., 2012; Choat et al., 2018; Arend et al., 2021). The hydraulic system of plants has evolved to the level of embolism resistance reaching P50 down to -18.8 MPa (Larter et al., 2015); and the most vulnerable species is found in tropical forest, whose P50 is as high as -0.18 MPa (Maherali et al., 2004).
Angiosperms exhibited higher intraspecific variation than gymnosperms in P50 (Anderegg, 2015). Higher angiosperm variability in P50 ought to result from anatomical differences between gymnosperms and angiosperms (Johnson et al., 2012; Anderegg, 2015). In addition, P50 is not the hydraulic safety threshold of all woody plants. Angiosperm species may be unable to recover if water potential dropped below P88 (the water potential inducing 88% xylem embolism), and P50 is considered more likely to be the hydraulic safety threshold of gymnosperms species (Urli et al., 2013). Environmental conditions and developmental stages may also affect the threshold to some degree. A recent field research of adult Norway spruce showed that processes leading to a rapid deterioration of tree hydraulic status and tree mortality occur in before P50 is reached (Arend et al., 2021; Li et al., 2022), suggesting that the vulnerability of some species to hydraulic failure may differ from previously expectations under different conditions.
The study of xylem embolism has a long history (Dixon and Joly, 1895; Ewart, 1906), and extensive research have been done in this field, including research methods (Cochard et al., 2013; Chen et al., 2021a). Thus, in this review, we do not dwell on the previous common description and only briefly describe the determinants of xylem embolism resistance from the perspective of anatomy.
Embolism occurs as ‘air-seeding’ enters the conduits through the pit pores, and pit area hypothesis proposes that the probability of embolism propagation in conduits increases with the total pit area of vessels (Tyree and Zimmermann, 2002; Wheeler et al., 2005). Larger vessels are more likely to have larger total pit area, so larger vessels may be more vulnerable to embolism (Jacobsen et al., 2007). Within species, smaller conduits were found to tend to be less vulnerable to embolism (Cai and Tyree, 2010; Fichot et al., 2015). However, the lack of comprehensive knowledge on the conduit size and pit traits further limits the understanding of the relationship between conduit dimensions and drought-induced embolism resistance (Cardoso et al., 2020). The porosity of pit membranes seems to be more important because pit membrane prevents bubble propagation (Tyree and Zimmermann, 2002). Thus, the importance of conduit size for embolism resistance remains a debate subject.
Pit membranes develop from the cell wall made of multiple cellulose layers, pectins and structural proteins, with a thickness between 140 nm and 1180 nm and including between 4 and 30 layers (Fichot et al., 2015; Kaack et al., 2021). The three-dimensional structure, hydration of pit membranes and their chemical composition play a key role in the resistance to embolism spread (Cardoso et al., 2020; Kaack et al., 2021). Perfusion experiments showed that cellulose and pectin are main components of pit membrane determining embolism resistance (Fichot et al., 2015).
Embolism spreading is related to the porosity of pit membrane, smaller pores tending to increase resistance (Choat et al., 2008; Fichot et al., 2015). Therefore, vulnerability to air-seeding is associated with the pit area, a bigger pit area implying an increased likelihood of having larger pores (Hacke et al., 2006). Thicker pit membrane is related to reduced porosity and therefore increasing resistance to embolism (Fichot et al., 2015; Figure 3). This link between embolism resistance and pit membrane thickness is also valid at the intrageneric (Scholz et al., 2013) and intraspecific level (Schuldt et al., 2016). Variation in pit membrane thickness is largely influenced by the number of microfibril layers, with thin pit membranes consisting of less microfibril layers than thick pit membranes (Kaack et al., 2021).
Figure 3 The relationship between water potential at which 50% loss of hydraulic conductance (P50) and intervessel pit membrane thickness of 75 angiosperm speices. The data comes from Scholz et al. (2013); Kaack et al. (2021) and Li et al. (2016).
Species with thicker conduits walls have less porous and thicker pit membranes and consequent stronger embolism resistance (Li et al., 2016). Thick conduit walls also facilitate strong wall reinforcement (conduit thickness/span), which would against deflection and even implosion of conduit walls and possible injury to pit membranes (Hacke and Sperry, 2001; Hacke et al., 2001). An extensive and supportive fiber matrix is also associated with embolism resistance. It was proposed that small diameter fibers can result in dense wood and provide mechanical strength, which facilitate resisting embolism (Jacobsen et al., 2005; Mccullon et al., 2012; Blackman et al., 2019; Chen et al., 2020). Some studies also found that the positive relationship between fiber wall thickness and embolism resistance may be caused by lignification and wood density of xylem (Jacobsen et al., 2005; Awad et al., 2012; Fichot et al., 2015; Torres-Ruiz et al., 2017). Moreover, vasicentric tracheids and fiber–tracheids could act as water reservoirs, thereby increasing tissue capacitance, which are associated with lower cavitation vulnerability (Barotto et al., 2016).
Embolism-resistant traits, such as thick pit membrane, thick conduit wall, high wood density and high fiber-wall area, all are costly in terms of carbon investment, highlighting the relationship between stress resistance and carbon investment (Figure 4).
Figure 4 General xylem traits that determine stem embolism resistance and the relationships between these traits and stem embolism resistance. The thicker end of each triangle represents the higher value of the corresponding trait, and the xylem with stronger embolism resistance has greater construction investment.
In general, the intra-specific P50 varied with more negative P50 values found in more xeric environments over space (Anderegg, 2015), and P50 values shifted toward more negative in response to drought stress (Wortemann et al., 2011). However, a few studies revealed that individuals exposed to drought stress were more vulnerable to embolism (Nardini and Luglio, 2014; Savi et al., 2015; Trugman et al., 2021). The explanation of this phenomenon is ‘cavitation fatigue’ for plants experienced chronic or extreme water stress (Hacke et al., 2001; Nardini and Luglio, 2014). Increased vulnerability to cavitation of the species, Quercus ilex L. (holm oak), exposed to long-lasting growing at urban sites under impervious pavements or 6-yr partial rainfall exclusion, might be explained as the hydraulic damage caused by cavitation fatigue (Limousin et al., 2010; Savi et al., 2015). In addition, cavitation fatigue has been also applied to explain the differences of embolism resistance between sapwood and heartwood (Fichot et al., 2015). A previous study demonstrated that conduits of the outer ring were still functional, whereas conduits of the older xylem were almost all embolized (Sperry et al., 1991).
The nutritional environment may also affect intra-specific variation of embolism resistance. High N availability tends to increase porosity of the pit membranes of vessel, and therefore, N-fertilized individuals have been shown to be more vulnerable to embolism (Plavcová and Hacke, 2012; Plavcová et al., 2013; Fichot et al., 2015). Additional P supply tends to increase embolism resistance, especially at high N concentrations (Harvey and Van Den Driessche, 1997; Hevia et al., 2019). As the xylem of species/genotypes more resistant to embolism needs more carbon investment and the carbon resources of shaded plants are limited, shaded individuals tend to be more vulnerable to cavitation (Schoonmaker et al., 2010; Plavcová et al., 2011). In addition, phloem conductance and water storage affect the recovery of embolism (Hammond and Adams, 2019). Nevertheless, intra-specific variation of vulnerability to embolism should be small, and a study of one widespread pine species (Pinus pinaster) showed low variation in genes of P50 across different populations (Lamy et al., 2014).
Plant root system plays a significant role in plant growth by exploiting soil resources through the uptake of water and nutrients (Wasaya et al., 2018). In general, resource absorption is undertaken by roots of terminal branch orders (usually the first- and second-order roots), whereas roots of the higher branch orders perform other functions, such as anchorage and storage (McCormack et al., 2015). Trees within the same forest are equipped with different rooting depths, so available water of trees varies with depth. In general, deep roots, i.e. deep-water access, may mitigate drought-induced mortality by limiting exposure to water stress. In a tropical forest, species exposure to drought stress exponentially declined with deeper root depth, which was relevant to drought resistance and resilience (Chitra-Tarak et al., 2021).
Root traits such as fine root diameter, specific root length, root angle, root length density and root hydraulic traits are considered to be linked with root water acquisition, water use efficiency, drought tolerance and access to nutrient, etc., which are important for understanding plant growth, survival and productivity (Nelson and Oliver, 2017; Wasaya et al., 2018; Tracy et al., 2020). The anatomical traits for the adaptations to soil water content can be determined by indices of the ratio of the root tissue areas (i.e. cortex to stele ratio, xylem to stele ratio) (Yamauchi et al., 2020). Root hairs are subcellular protrusions of the root epidermis, which extend into the soil and can improve the water and nutrient capture (Lynch, 2019). Importantly, root hydraulic traits are directly related to the capabilities and strategies of root organ and even whole plant to adapt to drought. Losing water from the root cells causes dehydrating roots to shrink, physically reduces hydraulic conductance at the soil-root interface, and causes roots to lose turgor which is crucial to root growth (Bartlett et al., 2021b). Roots with a more negative Ψ100 would exhibit a higher cell solute concentration and consequently more negative ΨTLP, and roots with a greater RWCTLP would retain more water at ΨTLP (Bartlett et al., 2021b). A lower root capacitance indicates that the roots retain more volume via less cell shrinkage, and thus maintain greater contact with the soil (Bartlett et al., 2021b). Hydraulic redistribution describes the passive flux of water through roots, for example from moist to dry soil layers (Hafner et al., 2020). Osmotic adjustment would strengthen the water potential gradient driving hydraulic redistribution, and higher hydraulic conductivities and larger conduits are also found to be related to higher hydraulic redistribution quantity (Hafner et al., 2020; Bartlett et al., 2021b). Root cortical lacunae usually precede root xylem embolism under drought stress, and embolism usually appears first in the fine roots and then in older, coarse roots (Cuneo et al., 2016). However, turgor and embolism recovery of roots occurs quickly upon exposure to water (c. 40 min-4 h) by absorbing inorganic ions and water (Shabala and Lew, 2002; Cuneo et al., 2018). Although the research on root hydraulic characteristics has made great progress, the research is still relatively backward compared with the aboveground part. Due to the inaccessibility of roots, new methods for root assaying need to be developed in the future (Nelson and Oliver, 2017).
The ability of maintaining high surface conductance to CO2 while avoiding desiccation is pivotal for survival in land plants. While reducing leaf Gs for reducing water loss has the cost of constraining photosynthetic carbon sequestration (Cowan and Farquhar, 1977). Therefore, the functional properties of the plant hydraulic system are integral to carbon and water balance (Drake et al., 2015; Figure 5A), and the ability of maximizing carbon gain and minimizing water loss has served as a powerful selective force on the evolution of functional and structural adaptations to drought in plants (Ambrose et al., 2009). Strong correlations between iso-anisohydric behavior and stem traits were observed in recent studies, highlighting the coordination of stomatal regulation and xylem function (Chen et al., 2021b; Figure 6). Many species with similar root syndromes display contrasting aboveground traits, which highlights the importance of including belowground organs to the whole-plant trait integration (Carmona et al., 2021). However, researches on the relationship between stem and leaf traits and root properties are still scarce, thus there is an important research task in the future in order to fully understand the coordination of tree water relations.
Figure 5 (A) Frame diagram characterizing the relationships between stomatal conductance, leaf/stem water potential and stem xylem embolism. (B) The survival rate of tree species with greater hydraulic safety margin (HSM) or stomatal safety margin (SSM) is higher under drought conditions.
Figure 6 The relationship between water potential at stomatal closure and water potential at which 50% loss of hydraulic conductance (P50) of 39 angiosperm speices. The data comes from Pivovaroff et al. (2018); Chen et al. (2019a) and Li et al. (2015).
The stomatal closure for preventing Ψleaf from falling below a set minimum value facilitates keeping the stem xylem from experiencing more serious embolism (Meinzer et al., 2014), and xylem embolism may also produce rapid hydraulic signals for initiating the stomatal response (Salleo et al., 2000). The hydraulic message triggered by embolism including ABA and pH changes in the xylem sap that travels to the shoots and contributes to the regulation of Gs, together with the direct effect of xylem water potential (Salleo et al., 2000; Tardieu, 2016). In other words, stomatal regulation and stem embolism are mutually linked by a negative feedback mechanism (Salleo et al., 2000).
The iso/anisohydric strategies combined with the corresponding xylem traits reflect the drought avoidance strategy and drought tolerance strategy. Drought avoidance and drought tolerance are two divergent strategies promoting plants adaptation to drought (Oliveira et al., 2021). The continuum of stomatal strategy from isohydry to anisohydric basically coincides with that from drought avoidance to drought tolerance. Drought-avoiding species close stomata rapidly responding to increased water deficit, and even directly shed leaves, which constrains carbon assimilation during dry periods; such drought avoiders with low wood density and high hydraulic capacitance appear to avoid xylem embolism via releasing of stored water to decrease Ψleaf fluctuations (Brodribb et al., 2014; Oliveira et al., 2021). On the contrary, species of drought tolerance with denser wood and low capacitance appear to rely on xylem structure to resist embolism and could maintain foliage over dry periods (Fu et al., 2019). Therefore, drought tolerators can sustain relatively photosynthesis, albeit at the cost of enhanced investment in structural reinforcement of embolism-resistant xylem and in osmoregulation to sustain leaf turgor (Meinzer et al., 2016). In general, anisohydric species appear to occupy more drought-prone habitats compared with isohydric species (McDowell et al., 2008).
The concept of HSM is generally described as the difference between a critical point (usually P50 or P88) on the vulnerability curve to embolism and the minimum seasonal xylem water potential (Ψmin). In view of the significant correlation between the Ψmin and Ψclose, Ψmin is often assumed to provide a measure of stomatal regulation of water potential (Pivovaroff et al., 2018; Chen et al., 2019a). As woody plants tend to maximize carbon sequestration relative to the investments of constructing or maintaining hydraulic support for foliage, woody species routinely operate at the catastrophic brink of xylem failure, highlighting the importance of the margin between xylem water potential and P50 or P88 (Tyree and Sperry, 1988; Bond and Kavanagh, 1999). Therefore, large positive HSM implies a relatively conservative response and small HSM (or even negative HSM) suggests a hydraulically risky response in the control of plant water relations (Skelton et al., 2015; Trugman et al., 2021). Although drought-induced tree death is complex and involves many physiological traits and processes, numerous previous studies showed that HSM was good (even only) predictor of mortality or branch dieback for tree species or shrub species (Adams et al., 2017; Chen et al., 2019a; Figure 5B).
According to the definition of HSM, HSM is determined by both Ψmin and embolism resistance. Although embolism resistance is strongly associated with the Ψmin across species, more embolism-resistant species have larger hydraulic safety margin than sensitive ones (Markesteijn and Poorter, 2011; Fu and Meinzer, 2019). HSM also affects species-specific distribution, and species that inhabit more arid environments usually possess larger HSM than those occupying wetter ones (Garzón et al., 2018). In addition, there appears to be a tendency for coniferous species to have larger HSMs compared with most angiosperm species (Choat et al., 2012), with Mediterranean climate angiosperms being a potential exception (Meinzer and McCulloh, 2013). Pioneer species usually operate lower HSM than shade-tolerant species which usually have more conservative strategies, and strong differences in HSM were also found between co-occurring deciduous and evergreen species (Markesteijn and Poorter, 2011). To offset a shorter growing season, deciduous species generally maximize photosynthetic carbon intake in the growth season (Wright et al., 2004; Ishida et al., 2006), and would thus operate a greater danger in hydraulic failure to maximize productivity in short growth season (Markesteijn and Poorter, 2011).
Unfortunately, for acquiring HSM, datasets of Ψmin in field conditions are typically patchy because of the laborious onsite measurement techniques (Breshears et al., 2009; Choat et al., 2018), and long-term measurement of Ψmin across seasonal scales is very time consuming. In addition, the hydraulic resistance in leaves is dynamic over a day timescale, which would lead to pronounced transpiration-induced disequilibrium between leaf and stem water potential in transpiring shoots (Meinzer et al., 2009). Therefore, stem xylem water potential of field grown plants must be measured on non-transpiring (shading treatment) leaves or shoot tips attached to transpiring shoots (Meinzer et al., 2009), which also increases the difficulty of measuring the Ψmin especially in tall trees.
Stomatal safety margin (SSM) refers to the difference between Ψclose and the water potential causing xylem dysfunction (usually P50). Compared with HSM, SSM uses Ψclose instead of Ψmin, which offsets the difficulty of obtaining the Ψmin data mentioned above. In addition, SSM more directly integrates the “safety” of stomatal response to water potential and xylem to drought-induced embolism (Skelton et al., 2015; Figure 5A).
Stomatal regulation preventing leaf water potential from falling below a set minimum value facilitates constraining excessive loss of stem hydraulic conductivity. This is in agreement with the hydraulic segmentation hypothesis, i.e., more distal components such as foliage protect stems by earlier embolism under drought (Tyree and Ewers, 1991; Nolf et al., 2015). Large positive SSM represent that leaves stomatal closure occurs before stem severe embolism, whereas negative SSM indicate stomatal closure subsequent to P50 (Skelton et al., 2015).
Ψclose varied from -0.655 MPa to -5.54 MPa (Martin-StPaul et al., 2017; Chen et al., 2019a), with the outlier about -10 MPa in a chaparral species in Mediterranean-type climate (Pivovaroff et al., 2018), spanning a range of variation about one-third that for embolism resistance (P50, -0.18 MPa to -18.8 MPa) (Maherali et al., 2004; Larter et al., 2015). Meta-analysis showed that most species have Ψclose values that are higher than their P50 (negative value) (Martin-StPaul et al., 2017; Figure 6). However, in the published data, it is found that the stomatal closure of a little tree species occurs after the occurrence of large xylem embolism, and such outliers are easy to die during drought (Skelton et al., 2015; Pivovaroff et al., 2018; Chen et al., 2019a; Chen et al., 2019b). In addition, there are species with strong embolism-resistant stems ultimately experience high mortality, which may result from the terrible coordination between stomatal regulation and xylem embolism resistance (Anderegg et al., 2019). There are clear evidences of partial and complete mortality correlated with hydraulic failure in both isohydric and anisohydric plants (McDowell, 2011), which further highlight the importance of coordination between stomatal regulation and xylem embolism resistance.
After stomatal closure, it still shows a decline of water potential, which is likely driven by cuticular and residual stomatal conductance. Nonetheless, the time of desiccation of species with wider SSM was longer (Blackman et al., 2019; Cardoso et al., 2020), and species that closing stomata relatively late also assume risky safety margin (Skelton et al., 2015). In general, SSM increased continuously with increasing embolism resistance (Martin-StPaul et al., 2017), and SSM is correlated with the HSM (Chen et al., 2019a). Most importantly, merging stomatal regulation strategies with xylem embolism resistance strategies contributes to a more comprehensive framework to manifest plant adaptation to drought (Skelton et al., 2015).
The dialogue between leaves, stems and roots is important in water uptake, transport and utilization. Water uptake occurs when the water potential of root system is higher than leaf water potential, thus establishing a gradient for water flux. In general, there is a linkage between the circadian oscillations in root hydraulic conductance and the daily variations in the transpiration, with significant consequences on water uptake (Tardieu et al., 2017; Trugman et al., 2021).
In terms of coordination between roots and leaf stomata, varieties in soil-plant hydraulic conductance would drive stomatal closure (Cai et al., 2022). Root-produced signaling may also affect stomatal conductance, and split root experiments have the potential to enable the investigation of the water uptake pattern of root systems (Koebernick et al., 2015). Previous studies found that when the dry part of the root system is at an intermediate soil water status, signal output from the entire root system is maximized, such that further soil drying actually decreases root-to-shoot signaling (Dodd et al., 2010). Alternatively, root capacitance might also impact gas exchange. Roots with a lower capacitance, which indicates that roots retain greater volume as water potentials decline, tend to maintain greater gas exchange through smaller declines in root volume and less ABA production (Bartlett et al., 2021b).
In terms of coordination between roots and stem hydraulic traits, there may be a trade-off between root depth and stem xylem embolism resistance. A study found that species exposure to drought stress declined with deeper root depth indicating that trees compensate for drought stress-related mortality risk through deep-water access, whereas species with deeper root had lower stem xylem embolism resistance and narrower stem hydraulic safety margins (Chitra-Tarak et al., 2021; Trugman et al., 2021). Species with investment in deep roots can access reliable deep-water resources, which ensures that hydraulic risk may not realize for them. Nevertheless, shallow-rooted species may tend to pay the cost of stem hydraulic safety, adapting for an environment in which hydraulic risk may be prone to occur, as extreme droughts usually occur in shallow soil layers (Chitra-Tarak et al., 2021). In addition, root systems have metabolic costs of respiration, another trade-off therefore may exist between the carbon cost of the root system and the growth of other organs (for example, stem) (Tardieu et al., 2017).
As mentioned above, sound knowledge of tree hydraulic function is crucial to understand drought-induced tree mortality, and it is necessary to extend and integrate other functional traits and processes to establish a more comprehensive framework.
Stomatal regulation and xylem embolism resistance are likely to interact with other important traits and processes, e.g., leaf area, plant water storage, root properties, hydraulic segmentation, minimum bark conductance and metabolic status, in the plant responses to drought (Hammond and Adams, 2019; Trugman et al., 2021). We can take stomatal regulation and xylem embolism resistance as the core, expand other important traits, including the relationship between investment of matter allocation and stress resistance, and incorporate the thoughts of the ‘fast-slow’ plant economics spectrum to establish a more comprehensive combination of traits that reflects tree species’ response and adaptation to drought. In functionally diverse communities, an integrated traits combination may produce more diverse drought adaptation strategies (Martínez-Vilalta and Garcia-Forner, 2017) and will accurately improve our prediction of how plants to adapt to spatio-temporal changes in environment conditions, which facilitates to model drought-related tree mortality and changes in plant demography in the future (Rowland et al., 2021).
In addition, we propose four specific urgent issues as future research priorities:
(1) We should expand future works to not only quantify empirical thresholds for hydraulic failure and associated HSM or SSM but also understand species specific variations over time to reach the thresholds (P50, Ψclose, and so on), which requires further knowledge of a range of other traits such as rooting depth, phloem conductance and plant water storage, and so on (Hammond and Adams, 2019).
(2) There is uncertainty in the mechanisms about the refilling of embolized xylem and the point of no return along P50, Ψclose, HSM or SSM, which limits our ability to understand and predict the legacies of successive drought events. This uncertainty is also related to the lack of knowledge about the interdependence of hydraulic failure and carbon starvation. Increased embolism and associated xylem tensions would cause declining carbon gain, transport, utilization, and subsequent feedbacks by which declining NSC availability impacts embolism through refilling or water homeostasis, but studies in this area is still lacking (McDowell, 2011; Hammond and Adams, 2019).
(3) It is not fully understood how iso/anisohydric behavior, xylem embolism resistance and HSM or SSM are affected by the root elements, especially root depth and root capacitance. Tree species with different iso/anisohydric behaviors and HSM or SSM may have different investments in above-ground woody stem and below-ground root growth (Ambrose et al., 2015). In addition, it is not clear that how chemical messages originating from roots affect canopy stomatal regulation under field conditions, especially for tall trees (Tardieu, 2016), and how roots as storage organs compensate for aboveground death by resprouting.
(4) The increase of the air temperature is the main hallmark of global climate change and is coupled with droughts, hence directly or indirectly affecting drought adaptation/resistance/resilience strategies of tree species. Some species exhibited higher stomatal conductance and stomatal density in the wet season in warming experiment (Wu et al., 2020). However, there is a lack of research on the effect of long-term warming on iso/anisohydric regulation, stem xylem embolism resistance and HSM or SSM, and thus future research in this area should be carried out.
ZC, XW and SRL conceived the ideas. ZC, SL, and SRL led the writing of the manuscript. ZC and XW revised the manuscript. All authors contributed to the article and gave final approval for publication.
The research was supported by the Fundamental Research Funds of Chinese Academy of Forestry (CAFYBB2020QB009), the Ministry of Science and Technology of China for Key R&D Program (2021YFD2200405) and the National Natural Science Foundation of China (31800513).
We would like to thank Baotianman Forest Ecosystem Research Station for the assistance in the previous research related to the ideas of this paper.
The authors declare that the research was conducted in the absence of any commercial or financial relationships that could be construed as a potential conflict of interest.
All claims expressed in this article are solely those of the authors and do not necessarily represent those of their affiliated organizations, or those of the publisher, the editors and the reviewers. Any product that may be evaluated in this article, or claim that may be made by its manufacturer, is not guaranteed or endorsed by the publisher.
The Supplementary Material for this article can be found online at: https://www.frontiersin.org/articles/10.3389/fpls.2022.926535/full#supplementary-material
Adams, H. D., Zeppel, M. J.B., Anderegg, W. R.L., Landhäusser, S. M., Tissue, D. T. (2017). A multi-species synthesis of physiological mechanisms in drought-induced tree mortality. Nat Ecol Evol. 1, 1285–1291.
Ambrose, A. R., Baxter, W. L., Wong, C. S., Næsborg, R. R., Williams, C. B., Dawson, T. E. (2015). Contrasting drought-response strategies in California redwoods. Tree Physiol. 35, 453–469. doi: 10.1093/treephys/tpv016
Ambrose, A. R., Sillett, S. C., Dawson, T. E. (2009). Effects of tree height on branch hydraulic, leaf structure and gas exchange in California redwood. Plant Cell Environ. 32, 743–757. doi: 10.1111/j.1365-3040.2009.01950.x
Anderegg, W. R. L. (2015). Spatial and temporal variation in plant hydraulic traits and their relevance for climate change impacts on vegetation. New Phytol. 205, 1008–1014. doi: 10.1111/nph.12907
Anderegg, W. R. L., Klein, T., Bartlett, M., Sack, L., Pellegrini, A. F. A., Choat, B., et al. (2016). Meta-analysis reveals that hydraulic traits explain cross-species patterns of drought-induced treemortality across the globe. Proc. Natl. Acad. Sci. U.S.A. 113), 5024–5029. doi: 10.1073/pnas.1525678113
Anderegg, W. R. L., Trugman, A. T., Bowling, D. R., Salvucci., G., Tuttle, S. E. (2019). Plant functional traits and climate influence drought intensification and land–atmosphere feedbacks. Proc. Natl. Acad. Sci. U.S.A. 116, 14071–14076. doi: 10.1073/pnas.1904747116
Arend, M., Link, R. M., Patthey, R., Hoch, G., Schuldt, B., Kahmen, A. (2021). Rapid hydraulic collapse as cause of drought-induced mortality in conifers. Proc. Natl. Acad. Sci. U.S.A. 118, e2025251118. doi: 10.1073/pnas.2025251118
Awad, H., Herbette, S., Brunel, N., Tixier, A., Pilate, G., Cochard, H., et al. (2012). No trade-off between hydraulic and mechanical properties in several transgenic poplars modified for lignins metabolism. Environ. Exp. Bot. 77, 185–195. doi: 10.1016/j.envexpbot.2011.11.023
Barnes, C. R. (1904). The ascent of water in trees. Science. 20, 179–179. doi: 10.1126/science.20.501.179.b
Barotto, A. J., Fernandez, M. E., Gyenge, J., Meyra, A., Martinez-Meier, A., Monteoliva, S. (2016). First insights into the functional role of vasicentric tracheids and parenchyma in eucalyptus species with solitary vessels: Do they contribute to xylem efficiency or safety? Tree Physiol. 36, 1485–1497. doi: 10.1093/treephys/tpw072
Bartlett, M. K., Klein, T., Jansen, S., Choat, B., Sack, L. (2016). The correlations and sequence of plant stomatal, hydraulic, and wilting responses to drought. Proc. Natl. Acad. Sci. U.S.A. 113, 13098–13103. doi: 10.1073/pnas.1604088113
Bartlett, M. K., Scoffoni, C., Ardy, R., Zhang, Y., Sun, S., Cao, K., et al. (2012b). Rapid determination of comparative drought tolerance traits: Using an osmometer to predict turgor loss point. Methods Ecol. Evol. 3, 880–888. doi: 10.1111/j.2041-210X.2012.00230.x
Bartlett, M. K., Scoffoni, C., Sack, L. (2012a). The determinants of leaf turgor loss point and prediction of drought tolerance of species and biomes: A global meta-analysis. Ecol. Lett. 15, 393–405. doi: 10.1111/j.1461-0248.2012.01751.x
Bartlett, M. K., Sinclair, G. (2021a). Temperature and evaporative demand drive variation in stomatal and hydraulic traits across grape cultivars. J. Exp. Bot. 72, 1995–2009. doi: 10.1093/jxb/eraa577
Bartlett, M. K., Sinclair, G., Fontanesi, G., Knipfer, T., Walker, M. A., McElrone, A. J. (2021b). Root pressure-volume curve traits capture rootstock drought tolerance. Ann. Bot-London 129, 389–402. doi: 10.1093/aob/mcab132
Bauer, H., Ache, P., Lautner, S., Fromm, J., Hartung, W., Al-Rasheid, K. A., et al. (2013). The stomatal response to reduced relative humidity requires guard cellautonomous ABA synthesis. Curr. Biol. 23, 53–57. doi: 10.1016/j.cub.2012.11.022
Binks, O., Meir, P., Rowland, L., da Costa, A. C.L., Vasconcelos, S.S., de Oliveira, A. A.R., et al. (2016). Plasticity in leaf-level water relations of tropical rainforest trees in response to experimental drought. New Phytol. 211, 477–488. doi: 10.1111/nph.13927
Blackman, C. J., Li, X., Choat, B., Rymer, P. D., De Kauwe, M. G., Duursma, R. A., et al. (2019). Desiccation time during drought is highly predictable across species of eucalyptus from contrasting climates. New Phytol. 224, 632–643. doi: 10.1111/nph.16042
Bonan, G. B. (2008). Forests and climate change: Forcings, feedbacks, and the climate benefits of forests. Science. 320, 1444–1449. doi: 10.1126/science.1155121
Bond, B. J., Kavanagh, K. L. (1999). Stomatal behavior of four woody species in relation to leaf-specific hydraulic conductance and threshold water potential. Tree Physiol. 19, 503—510. doi: 10.1093/treephys/19.8.503
Breshears, D. D., Myers, O. B., Meyer, C. W., Barnes, F. J., Zou, C. B.. (2009). Tree die-off in response to global change-type drought: Mortality insights from a decade of plant water potential measurements. Front. Ecol. Environ. 7, 185–189. doi: 10.1890/080016
Brodribb, T. J., Cochard, H. (2009). Hydraulic failure defines the recovery and point of death in water-stressed conifers. Plant Physiol. 149, 575–584. doi: 10.1104/pp.108.129783
Brodribb, T. J., Holbrook, N. M. (2003). Stomatal closure during leaf dehydration, correlation with other leaf physiological traits. Plant Physiol. 132, 2166–2173. doi: 10.1104/pp.103.023879
Brodribb, T. J., McAdam, S. A. M. (2013). Abscisic acid mediates a divergence in the drought response of two conifers. Plant Physiol. 162, 1370–1377. doi: 10.1104/pp.113.217877
Brodribb, T. J., McAdam, S. A. M. (2015). Evolution in the smallest valves (stomata) guides even the biggest trees. Tree Physiol. 35, 451–452. doi: 10.1093/treephys/tpv042
Brodribb, T. J., McAdam, S. A. M., Jordan., G. J., Martins, S. C. V. (2014). Conifer species adapt to low-rainfall climates by following one of two divergent pathways. Proc. Natl. Acad. Sci. U.S.A. 111, 14489–14493. doi: 10.1073/pnas.1407930111
Brodribb, T. J., Powers, J., Cochard, H., Choat, B. (2020). Hanging by a thread? forests and drought. Science. 368, 261–266. doi: 10.1126/science.aat7631
Cai, G., Ahmed, M., Abdalla, M., Carminati, A. (2022). Root hydraulic phenotypes impacting water uptake in drying soils. Plant Cell Environ. 45, 650–663. doi: 10.1111/pce.14259
Cai, J., Tyree, M. T. (2010). The impact of vessel size on vulnerability curves: Data and models for within-species variability in saplings of aspen, Populus tremuloides michx. Plant Cell Environ. 33, 1059–1069. doi: 10.1111/j.1365-3040.2010.02127.x
Cardoso, A. A., Billon, L., Fanton Borges, A., Fernández-de-Uña, L., Gersony, J. T., Güney, A., et al. (2020). New developments in understanding plant water transport under drought stress. New Phytol. 227, 1025–1027. doi: 10.1111/nph.16663
Carmona, C. P., Bueno, C. G., Toussaint, A., Träger, S., Díaz, S., Moora, M., et al. (2021). Fine-root traits in the global spectrum of plant form and function. Nature 597, 683–687. doi: 10.1038/s41586-021-03871-y
Chen, Y., Choat, B., Sterck, F., Maenpuen, P., Katabuchi, M., Zhang, S., et al. (2021a). Hydraulic prediction of drought-induced plant dieback and top-kill depends on leaf habit and growth form. Ecol. Lett. 24, 2350–2363. doi: 10.1111/ele.13856
Chen, Z., Li, S., Luan, J., Zhang, Y., Zhu, S., Wan, X., et al. (2019a). Prediction of temperate broadleaf tree species mortality in arid limestone habitats with stomatal safety margins. Tree Physiol. 39, 1428–1437. doi: 10.1093/treephys/tpz045
Chen, Z., Liu, S., Lu, H., Wan, X. (2019b). Interaction of stomatal behaviour and vulnerability to xylem cavitation determines the drought response of three temperate tree species. AoB Plants 11, plz058. doi: 10.1093/aobpla/plz058
Chen, Z., Zhang, Y., Yuan, W., Zhu, S., Pan, R., Wan, X., et al. (2021b). Coordinated variation in stem and leaf functional traits of temperate broadleaf tree species in the isohydric-anisohydric spectrum. Tree Physiol. 41, 1601–1610. doi: 10.1093/treephys/tpab028
Chen, Z., Zhu, S., Zhang, Y., Luan, J., Li, S., Sun, P., et al. (2020). Tradeoff between storage capacity and embolism resistance in the xylem of temperate broadleaf tree species. Tree Physiol. 40, 1029–1042. doi: 10.1093/treephys/tpaa046
Chitra-Tarak, R., Xu, C., Aguilar, S., Anderson-Teixeira, K. J., Chambers, J., Detto, M., et al. (2021). Hydraulically-vulnerable trees survive on deep-water access during droughts in a tropical forest. New Phytol. 231, 1798–1813. doi: 10.1111/nph.17464
Choat, B., Brodribb, T. J., Brodersen, C. R., Duursma, R. A., López, R., Medlyn, B. E. (2018). Triggers of tree mortality under drought. Nature 558, 531–539. doi: 10.1038/s41586-018-0240-x
Choat, B., Cobb, A. R., Jansen, S. (2008). Structure and function of bordered pits: new discoveries and impacts on whole-plant hydraulic function. New Phytol. 177, 608–625. doi: 10.1111/j.1469-8137.2007.02317.x
Choat, B., Jansen, S., Brodribb, T. J. (2012). Global convergence in the vulnerability of forests to drought. Nature. 491, 752–756. doi: 10.1038/nature11688
Cochard, H., Badel, E., Herbette, S., Delzon, S., Choat, B., Jansen, S. (2013). Methods for measuring plant vulnerability to cavitation: a critical review. J. Exp. Bot. 64, 4779–4791. doi: 10.1093/jxb/ert193
Cowan, I. R., Farquhar, G. D. (1977). Stomatal function in relation to leaf metabolism and environment. Symp. Soc. Exp. Biol. 31, 475–505.
Cuneo, I. F., Knipfer, T., Brodersen, C. R., McElrone, A. J. (2016). Mechanical failure of fine root cortical cells initiates plant hydraulic decline during drought. Plant Physiol. 172, 1669–1678. doi: 10.1104/pp.16.00923
Cuneo, I. F., Knipfer, T., Mandal, P., Brodersen, C. R., McElrone, A. J. (2018). Water uptake can occur through woody portions of roots and facilitates localized embolism repair in grapevine. New Phytol. 218, 506–516. doi: 10.1111/nph.15032
Davies, W. J., Zhang, J. (1991). Root signals and the regulation of growth and development of plants in drying soil. Annu. Rev. Plant Physiol. Mol. Biol. 42, 55–76. doi: 10.1146/annurev.pp.42.060191.000415
Dixon, H. H., Joly, J. (1895). On the ascent of sap. Philosophical Transactions of the Royal Society of London B, 186, 563–576.
Dodd, I. C., Egea, G., Watts, C. W., Whalley, W. R. (2010). Root water potential integrates discrete soil physical properties to influence ABA signalling during partial rootzone drying. J. ExpBot. 61, 3543–3551. doi: 10.1093/jxb/erq195
Drake, P. L., Price, C. A., Poot, P., Veneklaas, E. J. (2015). Isometric partitioning of hydraulic conductance between leaves and stems: balancing safety and efficiency in different growth forms and habitats. Plant Cell Environ. 38, 1628–1636. doi: 10.1111/pce.12511
Edwards, D., Kerp, H., Hass, H. (1998). Stomata in early land plants: An anatomical and ecophysiological approach. J. Exp. Bot. 49, 255–278. doi: 10.1093/jxb/49.Special_Issue.255
Eller, C. B., Lima, A. L., Oliveira, R. S. (2013). Foliar uptake of fog water and transport belowground alleviates drought effects in the cloud forest tree species, drimys brasiliensis (Winteraceae). New Phytol. 199, 151–162. doi: 10.1111/nph.12248
Fichot, R., Brignolas, F., Cochard, H., Ceulemans, R. (2015). Vulnerability to drought-induced cavitation in poplars: synthesis and future opportunities. Plant Cell Environ. 38, 1233–1251. doi: 10.1111/pce.12491
Fu, X., Meinzer, F. C. (2019). Metrics and proxies for stringency of regulation of plant water status (iso/anisohydry): A global data set reveals coordination and tradeoffs among water transport traits. Tree Physiol. 39, 122–134. doi: 10.1093/treephys/tpy087
Fu, X., Meinzer, F. C., Woodruff, D. R., Liu, Y., Smith, D. D., McCulloh, K. A., et al. (2019). Coordination and trade-offs between leaf and stem hydraulic traits and stomatal regulation along a spectrum of isohydry to anisohydry. Plant Cell Environ. 42, 2245–2258. doi: 10.1111/pce.13543
Garzón, M. B., González Muñoz, N., Wigneron, J., Moisy, C., Fernández-Manjarrés, J., Delzon, S. (2018). The legacy of water deficit on populations having experienced negative hydraulic safety margin. Global Ecol. Biogeogr. 27, 346–356. doi: 10.1111/geb.12701
Hacke, U. G., Sperry, J. S. (2001). Functional and ecological xylem anatomy. Perspect. Plant Ecol. 4, 97–115. doi: 10.1078/1433-8319-00017
Hacke, U. G., Sperry, J. S., Wheeler, J. K., Castro, L. (2006). Scaling of angiosperm xylem structure with safety and efficiency. Tree Physiol. 26, 689–701. doi: 10.1093/treephys/26.6.689
Hacke, U. G., Stiller, V., Sperry, J. S., Pittermann, J., McCulloh, K. A. (2001). Cavitation fatigue. embolism and refilling cycles can weaken the cavitation resistance of xylem. Plant Physiol. 125, 779–786. doi: 10.1104/pp.125.2.779
Hafner, B. D., Hesse, B. D., Bauerle, T. L., Grams, T. E. E. (2020). Water potential gradient, root conduit size and root xylem hydraulic conductivity determine the extent of hydraulic redistribution in temperate trees. Funct. Ecol. 34, 561–574. doi: 10.1111/1365-2435.13508
Hammond, W. M., Adams, H. D. (2019). Dying on time: Traits influencing the dynamics of tree mortality risk from drought. Tree Physiol. 39, 906–909. doi: 10.1093/treephys/tpz050
Hartmann, H., Moura, C. F., Anderegg, W. R. L., Ruehr, N. K., Salmon, Y., Allen, C. D., et al. (2018). Research frontiers for improving our understanding of drought-induced tree and forest mortality. New Phytol. 218, 15–28. doi: 10.1111/nph.15048
Hartmann, H., Roman, M. L., Schuldt, B. (2021). A whole-plant perspective of isohydry: stem-level support for leaf-level plant water regulation. Tree Physiol. 41, 901–905. doi: 10.1093/treephys/tpab011
Harvey, H. P., Van Den Driessche, R. (1997). Nutrition, xylem cavitation and drought resistance in hybrid poplar. Tree Physiol. 17, 647–654. doi: 10.1093/treephys/17.10.647
Heath, O. V. S. (1938). An experimental investigation of the mechanism of stomatal movement, with some preliminary observations upon the response of the guard cells to “shock.” New Phytol. 37, 385–395. doi: 10.1111/j.1469-8137.1938.tb06954.x
Hedwig, J. (1793). Sammlung seiner zerstreuten abhandlungen und beobachtungen über botanisch-ökonomische gegenstände (Leipzig, Germany: Band 1. Siegfried Lebrecht Crucius).
Henry, C., John, G. P., Pan, R., Bartlett, M. K., Fletcher, L. R., Scoffoni, C., et al. (2019). A stomatal safety-efficiency trade-off constrains responses to leaf dehydration. Nat. Commun. 10, 1–9. doi: 10.1038/s41467-019-11006-1
Hevia, A., Sánchez-Salguero, R., Julio Camarero, J., Querejeta, J. I., Sangüesa-Barreda, G, Gazol, A. (2019). Long-term nutrient imbalances linked to drought-triggered forest dieback. Sci. Total Environ. 690, 1254–1267. doi: 10.1016/j.scitotenv.2019.06.515
Hochberg, U., Rockwell, F. E., Holbrook, N. M., Cochard, H. (2018). Iso/Anisohydry: A plant–environment interaction rather than a simple hydraulic trait. Trends Plant Sci. 23, 112–120. doi: 10.1016/j.tplants.2017.11.002
Ishida, A., Diloksumpun, S., Ladpala, P., Staporn, D., Panuthai, S., Gamo, M., et al. (2006). Contrasting seasonal leaf habits of canopy trees between tropical dry-deciduous and evergreen forests in Thailand. Tree Physiol. 26, 643–656. doi: 10.1093/treephys/26.5.643
Jacobsen, A. L., Agenbag, L., Esler, K. J., Pratt, R. B., Ewers, F. W., Davis, S. D. (2007). Xylem density, biomechanics and anatomical traits correlate with water stress in 17 evergreen shrub species of the Mediterranean-type climate region of south. Africa. J. Ecol. 95, 171–183. doi: 10.1111/j.1365-2745.2006.01186.x
Jacobsen, A. L., Ewers, F. W., Pratt, R. B., Paddock, W. A., Davis, S. D. (2005). Do xylem fibers affect vessel cavitation resistance? Plant Physiol. 139, 546–556. doi: 10.1104/pp.104.058404
Johnson, D. M., Berry, Z. C., Baker, K. V., Smith, D. D., McCulloh, K. A., Domec, J. (2018). Leaf hydraulic parameters are more plastic in species that experience a wider range of leaf water potentials. Funct. Ecol. 32, 894–903. doi: 10.1111/1365-2435.13049
Johnson, D. M., McCulloh, K. A., Woodruff, D. R., Meinzer, F. C. (2012). Hydraulic safety margins and embolism reversal in stems and leaves: why are conifers and angiosperms so different? Plant Sci. 195, 48–53. doi: 10.1016/j.plantsci.2012.06.010
Kaack, L., Weber, M., Isasa, E., Karimi, Z., Li, S., Pereira, L., et al. (2021). Pore constrictions in intervessel pit membranes provide a mechanistic explanation for xylem embolism resistance in angiosperms. New Phytol. 230, 1829–1843. doi: 10.1111/nph.17282
Klein, T. (2014). The variability of stomatal sensitivity to leaf water potential across tree species indicates a continuum between isohydric and anisohydric behaviours. Funct. Ecol. 28, 1313–1320. doi: 10.1111/1365-2435.12289
Koebernick, N., Huber, K., Kerkhofs, E., Vanderborght, J., Mathieu, J., Vereecken, H., et al. (2015). Unraveling the hydrodynamics of split root water uptake experiments using CT scanned root architectures and three dimensional flow simulations. Front. Plant Sci. 6, 370. doi: 10.3389/fpls.2015.00370
Kollist, H., Nuhkat, M., Roelfsema, M. R. G. (2014). Closing gaps: Linking elements that control stomatal movement. New Phytol. 203, 44–62. doi: 10.1111/nph.12832
Kozlowski, T. T., Pallardy, S. G. (2002). Acclimation and adaptive responses of woody plants to environmental stresses. Bot. Rev. 68, 270–334. doi: 10.1663/0006-8101(2002)068[0270:AAAROW]2.0.CO;2
Kuromori, T., Sugimoto, E., Shinozaki, K. (2014). Intertissue signal transfer of abscisic acid from vascular cells to guard cells. Plant Physiol. 164, 1587–1592. doi: 10.1104/pp.114.235556
Lamy, J. B., Delzon, S., Bouche, P. S., Alia, R., Giuseppe Vendramin, G., Cochard, H., et al. (2014). Limited genetic variability and phenotypic plasticity detected for cavitation resistance in a Mediterranean pine. New Phytol. 201, 874–886. doi: 10.1111/nph.12556
Larter, M., Brodribb, T. J., Pfautsch, S., Burlett, R., Cochard, H., Delzon, S. (2015). Extreme aridity pushes trees to their physical limits. Plant Physiol. 168, 804–807. doi: 10.1104/pp.15.00223
Larter, M., Pfautsch, S., Domec, J., Trueba, S., Nagalingum, N., Delzon, S. (2017). Aridity drove the evolution of extreme embolism resistance and the radiation of conifer genus callitris. New Phytol. 215, 97–112. doi: 10.1111/nph.14545
Lawson, T., Blatt, M. R. (2014). Stomatal size, speed, and responsiveness impact on photosynthesis and water use efficiency. Plant Physiol. 164, 1556–1570. doi: 10.1104/pp.114.237107
Lee, K. H., Piao, H. L., Kim, H. Y., Choi, S. M., Jiang, F., Hartung, W., et al. (2006). Activation of glucosidase via stressinduced polymerization rapidly increases active pools of abscisic acid. Cell. 126, 1109–1120. doi: 10.1016/j.cell.2006.07.034
Lenz, T. I., Wright, I. J., Westoby, M. (2006). Interrelations among pressure-volume curve traits across species and water availability gradients. Physiol. Plant 127, 423–433. doi: 10.1111/j.1399-3054.2006.00680.x
Li, X., Blackman, C. J., Peters, J. M. R., Choat, B., Rymer, P. D., Medlyn, B. E., et al. (2019). More than iso/anisohydry: Hydroscapes integrate plant water use and drought tolerance traits in 10 eucalypt species from contrasting climates. Funct. Ecol. 33, 1035–1049. doi: 10.1111/1365-2435.13320
Li, S., Feifel, M., Karimi, Z., Schuldt, B., Choat, B., Jansen, S. (2015). Leaf gas exchange performance and the lethal water potential of five European species during drought. Tree Physiol. 36, 179–192. doi: 10.1093/treephys/tpv117
Li, S., Lens, F., Espino, S., Karimi, Z., Klepsch, M. M., Schenk, H. J., et al. (2016). Intervessel pit membrane thickness as a key determinant of embolism resistance in angiosperm xylem. IAWA J. 37, 152–171. doi: 10.1163/22941932-20160128
Li, S., Li, X., Wang, J., Chen, Z., Lu, S., Wan, X., et al. (2022). Hydraulic traits are coupled with plant anatomical traits under drought-rewatering cycles in Ginkgo biloba l. Tree Physiol. 42, 1216–1227. doi: 10.1093/treephys/tpab174
Limousin, J. M., Longepierre, D., Huc, R., Rambal, S. (2010). Change in hydraulic traits of Mediterranean quercus ilex subjected to long-term throughfall exclusion. Tree Physiol. 30, 1026–1036. doi: 10.1093/treephys/tpq062
Lynch, J. P. (2019). Root phenotypes for improved nutrient capture: An underexploited opportunity for global agriculture. New Phytol. 223, 548–564. doi: 10.1111/nph.15738
Maherali, H., Pockman, W., Jackson, R. (2004). Adaptive variation in the vulnerability of woody plants to xylem cavitation. Ecology. 85, 2184–2199. doi: 10.1890/02-0538
Maréchaux, I., Bartlett, M. K., Iribar, A., Sack, L., Chave, J. (2017). Stronger seasonal adjustment in leaf turgor loss point in lianas than trees in an Amazonian forest. Biol. Lett. 13, 20160819. doi: 10.1098/rsbl.2016.0819
Markesteijn, L., Poorter, L. (2011). Ecological differentiation in xylem cavitation resistance is associated with stem and leaf structural traitspc. Plant Cell Environ. 34, 137–148. doi: 10.1111/j.1365-3040.2010.02231.x
Martínez-Vilalta, J., Garcia-Forner, N. (2017). Water potential regulation, stomatal behaviour and hydraulic transport under drought: Deconstructing the iso/anisohydric concept. Plant Cell Environ. 40, 962–976. doi: 10.1111/pce.12846
Martínez-Vilalta, J., Rafael, P., Aguadé, D., Javier, R., Mencuccini, M. (2014). A new look at water transport regulation in plants. New Phytol. 204, 105–115. doi: 10.1111/nph.12912
Martin-StPaul, N., Delzon, S., Cochard, H. (2017). Plant resistance to drought depends on timely stomatal closure. Ecol. Lett. 20, 1437–1447. doi: 10.1111/ele.12851
McAdam, S. A. M., Brodribb, T. J. (2015). The evolution of mechanisms driving the stomatal response to vapor pressure deficit. Plant Physiol. 167, 833–843. doi: 10.1104/pp.114.252940
McCormack, M. L., Dickie, I. A., Eissenstat, D. M., Fahey, T. J., Fernandez, C. W., Guo, D., et al. (2015). Redefining fine roots improves understanding of below-ground contributions to terrestrial biosphere processes. New Phytol. 207, 505–518. doi: 10.1111/nph.13363
Mccullon, K. A., Johnson, D. M., Meinzer, F. C. (2012). Hydraulic architecture of two species differing in wood density: Opposing strategies in co-occurring tropical pioneer trees. Plant Cell Environ. 35, 116–125. doi: 10.1111/j.1365-3040.2011.02421.x
McDowell, N. G. (2011). Mechanisms linking drought, hydraulics, carbon metabolism, and vegetation mortality. Plant Physiol. 155, 1051–1059. doi: 10.1104/pp.110.170704
McDowell, N., Pockman, W. T., Allen, C. D., Breshears, D. D., Cobb, N., Kolb, T., et al. (2008). Mechanisms of plant survival and mortality during drought: Why do some plants survive while others succumb to drought? New Phytol. 178, 719–739. doi: 10.1111/j.1469-8137.2008.02436.x
McKown, A. D., Cochard, H., Sack, L. (2010). Decoding leaf hydraulics with a spatially explicit model: principles of venation architecture and implications for its evolution. Am. Nat. 175, 447–460. doi: 10.1086/650721
Medeiros, D. B., Daloso, D. M., Fernie, A. R., Nikoloski, Z., Araújo, W. L. (2015). Utilizing systems biology to unravel stomatal function and the hierarchies underpinning its control. Plant Cell Environ. 38, 1457–1470. doi: 10.1111/pce.12517
Meinzer, F. C., Johnson, D. M., Lachenbruch, B., McCulloh, K. A., Woodruff, D. R. (2009). Xylem hydraulic safety margins in woody plants: coordination of stomatal control of xylem tension with hydraulic capacitance. Funct. Ecol. 23, 922–930. doi: 10.1111/j.1365-2435.2009.01577.x
Meinzer, F. C., McCulloh, K. A. (2013). Xylem recovery from drought-induced embolism: where is the hydraulic point of no return? Tree Physiol. 33, 331–334. doi: 10.1093/treephys/tpt022
Meinzer, F. C., Sharifi, M. R., Nilsen, E. T., Rundel, P. W. (1988). Effects of manipulation of water and nitrogen regime on the water relations of the desert shrub Larrea tridentata. Oecologia. 77, 480–486. doi: 10.1007/BF00377263
Meinzer, F. C., Smith, D. D., Woodruff, D. R., Marias, D. E., McCulloh, K. A., Howard, A. R., et al. (2017). Stomatal kinetics and photosynthetic gas exchange along a continuum of isohydric to anisohydric regulation of plant water status. Plant Cell Environ. 40, 1618–1628. doi: 10.1111/pce.12970
Meinzer, F. C., Woodruff, D. R., Marias, D. E., McCulloh, K. A., Sevanto, S. (2014). Dynamics of leaf water relations components in co-occurring iso-and anisohydric conifer species. Plant Cell Environ. 37, 2577–2586. doi: 10.1111/pce.12327
Meinzer, F. C., Woodruff, D. R., Marias, D. E., Smith, D. D., McCulloh, K. A., Howard, A. R., et al. (2016). Mapping ‘hydroscapes’ along the iso- to anisohydric continuum of stomatal regulation of plant water status. Ecol. Lett. 19, 1343–1352. doi: 10.1111/ele.12670
Nardini, A., Luglio, J. (2014). Leaf hydraulic capacity and drought vulnerability: Possible trade-offs and correlations with climate across three major biomes. Funct. Ecol. 28, 810–818. doi: 10.1111/1365-2435.12246
Nelson, S. K., Oliver, M. J. (2017). A soil-plate based pipeline for assessing cereal root growth in response to polyethylene glycol (PEG)-induced water deficit stress. Front. Plant Sci. 8, 1272. doi: 10.3389/fpls.2017.01272
Nolan, R. H., Tarin, T., Santini, N. S., McAdam, S. A. M., Ruman, R., Eamus, D. (2017). Differences in osmotic adjustment, foliar abscisic acid dynamics and stomatal regulation between an isohydric and anisohydric woody angiosperm during drought. Plant Cell Environ. 40, 3122–3134. doi: 10.1111/pce.13077
Nolf, M., Creek, D., Duursma, R., Holtum, J., Mayr, S., Choat, B. (2015). Stem and leaf hydraulic properties are finely coordinated in three tropical rainforest tree species. Plant Cell Environ. 38, 2652–2661. doi: 10.1111/pce.12581
Okamoto, M., Tanaka, Y., Abrams, S. R., Kamiya, Y., Seki, M., Nambara, E. (2009). High humidity induces abscisic acid 89-hydroxylase in stomata and vasculature to regulate local and systemic abscisic acid responses in arabidopsis. Plant Physiol. 149, 825–834. doi: 10.1104/pp.108.130823
Oliveira, R. S., Eller, C. B., Barros, F. V., Hirota, M., Brum, M., Bittencourt, P. (2021). Linking plant hydraulics and the fast-slow continuum to understand resilience to drought in tropical ecosystems. New Phytol. 230, 904–923. doi: 10.1111/nph.17266
Pan, Y., Birdsey, R. A., Fang, J., Houghton, R., Kauppi, P. E., Kurz, W. A., et al. (2011). A large and persistent carbon sink in the world’s forests. Science. 333, 988–993. doi: 10.1126/science.1201609
Papanatsiou, M., Petersen, J., Henderson, L., Wang, Y., Christie, J. M., Blatt, M. R. (2019). Optogenetic manipulation of stomatal kinetics improves carbon assimilation, water use, and growth. Science. 363, 1456–1459. doi: 10.1126/science.aaw0046
Phillips, O. L., Aragão, L. E. O. C., Lewis, S. L., Fisher, J. B., Lloyd, J., López-González, G., et al. (2009). Drought sensitivity of the amazon rainforest. Science. 323, 1344–1347. doi: 10.1126/science.1164033
Pivovaroff, A. L., Cook, V. M. W., Santiago, L. S. (2018). Stomatal behaviour and stem xylem traits are coordinated for woody plant species under exceptional drought conditions. Plant Cell Environ. 41, 2617–2626. doi: 10.1111/pce.13367
Pivovaroff, A. L., Pasquini, S. C., De Guzman, M. E., Alstad, K. P., Stemke, J. S., Santiago, L. S. (2016). Multiple strategies for drought survival among woody plant species. Funct. Ecol. 30, 517–526. doi: 10.1111/1365-2435.12518
Plavcová, L., Hacke, U. G. (2012). Phenotypic and developmental plasticity of xylem in hybrid poplar saplings subjected to experimental drought, nitrogen fertilization, and shading. J. Exp. Bot. 63, 6481–6491. doi: 10.1093/jxb/ers303
Plavcová, L., Hacke, U. G., Almeida-Rodriguez, A. M., Li, E., Douglas, C. J. (2013). Gene expression patterns underlying changes in xylem structure and function in response to increased nitrogen availability in hybrid poplar. Plant Cell Environ. 36, 186–199. doi: 10.1111/j.1365-3040.2012.02566.x
Plavcová, L., Hacke, U. G., Sperry, J. S. (2011). Linking irradiance-induced changes in pit membrane ultrastructure with xylem vulnerability to cavitation. Plant Cell Environ. 34, 501–513. doi: 10.1111/j.1365-3040.2010.02258.x
Raven, J. A. (2002). Selection pressures on stomatal evolution. New Phytol. 153, 371–386. doi: 10.1046/j.0028-646X.2001.00334.x
Rowland, L., Martínez-Vilalta, J., Mencuccini, M. (2021). Hard times for high expectations from hydraulics: Predicting drought-induced forest mortality at landscape scales remains a challenge. New Phytol. 230, 1685–1687. doi: 10.1111/nph.17317
Sack, L., Buckley, T. N. (2016). The developmental basis of stomatal density and flux. Plant Physiol. 171, 2358–2363. doi: 10.1104/pp.16.00476
Salleo, S., Nardini, A., Pitt, F., Lo Gullo, M. A. (2000). Xylem cavitation and hydraulic control of stomatal conductance in laurel (Laurus nobilis l.). Plant Cell Environ. 23, 71–79. doi: 10.1046/j.1365-3040.2000.00516.x
Savi, T., Bertuzzi, S., Branca, S., Tretiach, M., Nardini, A. (2015). Drought-induced xylem cavitation and hydraulic deterioration: Risk factors for urban trees under climate change? New Phytol. 205, 1106–1116. doi: 10.1111/nph.13112
Scholander, P. F., Bradstreet, E. D., Hemmingsen, E. A., Hammel, H. T. (1965). Sap pressure in vascular plants. Science. 148, 339–346. doi: 10.1126/science.148.3668.339
Scholz, A., Rabaey, D., Stein, A., Cochard, H., Smets, E., Jansen, S. (2013). The evolution and function of vessel and pit characters with respect to cavitation resistance across 10 prunus species. Tree Physiol. 33, 684–694. doi: 10.1093/treephys/tpt050
Schoonmaker, A. L., Hacke, U. G., Landhäusser, S. M., Lieffers, V. J., Tyree, M. T. (2010). Hydraulic acclimation to shading in boreal conifers of varying shade tolerance. Plant Cell Environ. 33, 382–393. doi: 10.1111/j.1365-3040.2009.02088.x
Schuldt, B., Knutzen, F., Delzon, S., Jansen, S., Muller-Haubold, H., Burlett, R., et al. (2016). How adaptable is the hydraulic system of European beech in the face of climate change-related precipitation reduction? New Phytol. 210, 443–458. doi: 10.1111/nph.13798
Scoffoni, C., Vuong, C., Diep, S., Cochard, H., Sack, L. (2014). Leaf shrinkage with dehydration: coordination with hydraulic vulnerability and drought tolerance. Plant Physiol. 164, 1772–1788. doi: 10.1104/pp.113.221424
Shabala, S. N., Lew, R. R. (2002). Turgor regulation in osmotically stressed arabidopsis epidermal root cells. direct support for the role of inorganic ion uptake as revealed by concurrent flux and cell turgor measurements. Plant Physiol. 129, 290–299. doi: 10.1104/pp.020005
Skelton, R. P., Westa, A. G., Dawson, T. E. (2015). Predicting plant vulnerability to drought in biodiverse regions using functional traits. Proc. Natl. Acad. Sci. U.S.A. 112, 5744–5749. doi: 10.1073/pnas.1503376112
Slatyer, R. O., Taylor, S. A. (1960). Terminology in plant- and soil-water relations. Nature. 187, 922–924. doi: 10.1038/187922a0
Sperry, J. S., Perry, A. H., Sullivan, J. E. M. (1991). Pit membrane degradation and air-embolism formation in ageing xylem vessels of Populus tremuloides michx. J. Exp. Bot. 42, 1399–1406. doi: 10.1093/jxb/42.11.1399
Tardieu, F. (2016). Too many partners in root-shoot signals. does hydraulics qualify as the only signal that feeds back over time for reliable stomatal control? New Phytol. 212, 802–804. doi: 10.1111/nph.14292
Tardieu, F., Draye, X., Javaux, M. (2017). Root water uptake and ideotypes of the root system: Whole-plant controls matter. Vadose Zone J. 16, 9. doi: 10.2136/vzj2017.05.0107
Tardieu, F., Simonneau, T. (1998). Variability among species of stomatal control under fluctuating soil water status and evaporative demand: modelling isohydric and anisohydric behaviours. J. Exp. Bot. 49, 419–432. doi: 10.1093/jxb/49.Special_Issue.419
Torres-Ruiz, J. M., Cochard, H., Fonseca, E., Badel, E., Gazarini, L., Vaz, M. (2017). Differences in functional and xylem anatomical features allow cistus species to co-occur and cope differently with drought in the Mediterranean region. Tree Physiol. 37, 755–766. doi: 10.1093/treephys/tpx013
Tracy, S. R., Nagel, K. A., Postma, J. A., Fassbender, H., Wasson, A., Watt, M. (2020). Crop improvement from phenotyping roots: Highlights reveal expanding opportunities. Trends Plant Sci. 25, 105–118. doi: 10.1016/j.tplants.2019.10.015
Trugman, A. T., Anderegg, L. D. L., Anderegg, W. R. L., Das, A. J., Stephenson, N. L. (2021). Why is tree drought mortality so hard to predict? Trends Ecol. Evol. 36, 520–532. doi: 10.1016/j.tree.2021.02.001
Tyree, M. T., Ewers, F. W. (1991). The hydraulic architecture of trees and other woody plants. New Phytol. 119, 345–360. doi: 10.1111/j.1469-8137.1991.tb00035.x
Tyree, M. T., Sperry, J. S. (1988). Do woody plants operate near the point of catastrophic xylem dysfunction caused by dynamic water stress? Plant Physiol. 88, 574–580. doi: 10.1104/pp.88.3.574
Tyree, M. T., Zimmermann, M. H. (2002). Xylem structure and the ascent of sap, 2nd edn (Berlin, Germany: Springer).
Urli, M., Porté, A. J., Cochard, H., Guengant, Y., Burlett, R., Delzon, S. (2013). Xylem embolism threshold for catastrophic hydraulic failure in angiosperm trees. Tree Physiol. 33, 672–683. doi: 10.1093/treephys/tpt030
Waadt, R., Hitomi, K., Nishimura, N., Hitomi, C., Adams, S. R., Getzoff, E. D., et al. (2014). FRET-based reporters for the direct visualization of abscisic acid concentration changes and distribution in arabidopsis. eLife. 3, e01739. doi: 10.7554/eLife.01739
Wasaya, A., Zhang, X., Fang, Q., Yan, Z. (2018). Root phenotyping for drought tolerance: A review. Agronomy 8, 241. doi: 10.3390/agronomy8110241
Wheeler, J. K., Sperry, J. S., Hacke, U. G., Hoang, N. (2005). Inter-vessel pitting and cavitation in woody rosaceae and other vesselled plants: A basis for a safety versus efficiency trade-off in xylem transport. Plant Cell Environ. 28, 800–812. doi: 10.1111/j.1365-3040.2005.01330.x
Woodruff, D. R., Meinzer, F. C., Marias, D. E., Sevanto, S., Jenkins, M. W., McDowell, N. G. (2015). Linking nonstructural carbohydrate dynamics to gas exchange and leaf hydraulic behavior in pinus edulis and juniperus monosperma. New Phytol. 206, 411–421. doi: 10.1111/nph.13170
Wortemann, R., Herbette, S., Barigah, T. S., Fumanal, B., Alia, R., Ducousso, A., et al. (2011). Genotypic variability and phenotypic plasticity of cavitation resistance in fagus sylvatica l. across Europe. Tree Physiol. 31, 1175–1182. doi: 10.1093/treephys/tpr101
Wright, I. J., Reich, P. B., Westoby, M., Ackerly, D. D., Baruch, Z., Bongers, F., et al. (2004). The worldwide leaf economics spectrum. Nature. 428, 821–827. doi: 10.1038/nature02403
Wu, T., Tissue, D. T., Li, X., Liu, S., Chu, G., Zhou, G., et al. (2020). Long-term effects of 7-year warming experiment in the field on leaf hydraulic and economic traits of subtropical tree species. Glob. Change Biol. 26, 7144–7157. doi: 10.1111/gcb.15355
Yamauchi, T., Pedersen, O., Nakazono, M., Tsutsumi, N. (2020). Key root traits of poaceae for adaptation to soil water gradients. New Phytol. 229, 3133–3140. doi: 10.1111/nph.17093
Keywords: climate change, hydraulic traits, hydraulic failure, carbon starvation, stomatal safety margin, tree mortality
Citation: Chen Z, Li S, Wan X and Liu S (2022) Strategies of tree species to adapt to drought from leaf stomatal regulation and stem embolism resistance to root properties. Front. Plant Sci. 13:926535. doi: 10.3389/fpls.2022.926535
Received: 22 April 2022; Accepted: 12 September 2022;
Published: 27 September 2022.
Edited by:
Teemu Hölttä, University of Helsinki, FinlandReviewed by:
Edgard Picoli, Universidade Federal de Viçosa, BrazilCopyright © 2022 Chen, Li, Wan and Liu. This is an open-access article distributed under the terms of the Creative Commons Attribution License (CC BY). The use, distribution or reproduction in other forums is permitted, provided the original author(s) and the copyright owner(s) are credited and that the original publication in this journal is cited, in accordance with accepted academic practice. No use, distribution or reproduction is permitted which does not comply with these terms.
*Correspondence: Shirong Liu, bGl1c3JAY2FmLmFjLmNu
Disclaimer: All claims expressed in this article are solely those of the authors and do not necessarily represent those of their affiliated organizations, or those of the publisher, the editors and the reviewers. Any product that may be evaluated in this article or claim that may be made by its manufacturer is not guaranteed or endorsed by the publisher.
Research integrity at Frontiers
Learn more about the work of our research integrity team to safeguard the quality of each article we publish.