- 1Institute of Ecological Environment Protection Research, Shanghai Academy of Agricultural Sciences, Shanghai, China
- 2Shanghai Key Laboratory of Protected Horticultural Technology, Shanghai, China
- 3Nicholas School of the Environment, Duke University, Durham, NC, United States
- 4Soil Science and Plant Nutrition, UWA School of Agriculture and Environment, The University of Western Australia, Perth, WA, Australia
- 5Institute for Adriatic Crops and Karst Reclamation, Split, Croatia
- 6Department of Soil Science of Temperate Ecosystems, University of Göttingen, Göttingen, Germany
- 7Department of Agricultural Soil Science, University of Göttingen, Göttingen, Germany
- 8Peoples Friendship University of Russia (RUDN University), Moscow, Russia
- 9Institute of Environmental Sciences, Kazan Federal University, Kazan, Russia
Plants adjust root morphological and/or exudation traits in response to phosphorus (P) mobilization mediated by microorganisms. We hypothesized that straw application coupled with P fertilization would influence microbial P and then root nutrient-acquisition strategies related to crop growth. Root morphological (length and average diameter) and exudation traits (acid phosphatase and carboxylates) of Brassica chinensis, Solanum lycopersicum, Lactuca sativa, and Vigna unguiculata in response to microbial P dynamics were characterized in no-P and P-fertilized soil with/without straw addition. Straw addition increased the growth of fungi and bacteria, stimulating microbial P immobilization at day 24. The high microbial abundance was associated with four tested crops having short roots in straw-amended compared with no-straw soil at day 24. In straw-amended soil, B. chinensis and S. lycopersicum shifted toward root P-acquisition strategies based on fast elongation and strong carboxylate exudation from days 24 to 40. Such effective root P-acquisition strategies together with microbial P release increased shoot P content in S. lycopersicum in straw-amended compared with those without straw at day 40. Conversely, L. sativa and V. unguiculata produced short roots in response to the stable (or even increased) microbial P after straw addition till day 40. In straw-amended soil, high P application stimulated root elongation and carboxylate exudation in L. sativa and V. unguiculata, whereas carboxylate exudation by S. lycopersicum was decreased compared with the straw-amended but non-fertilized treatment at day 40. In summary, root P-acquisition strategies in response to microbial P differed among the tested crop species. Phosphorus fertilization needs to be highlighted when returning straw to enhance P-use efficiency in vegetable cropping systems.
Introduction
Low availability of phosphorus (P) is often limiting plant growth in most soils (Hinsinger, 2001; Shen et al., 2011). Plant species adopt specific root morphological and/or exudation traits to enable efficient P acquisition (Lambers et al., 2006; Wen et al., 2019, 2022). For example, nutrient-acquisitive species with thin roots show rapid root elongation and explore a large soil volume to adapt to low P availability and enhance P acquisition (Li et al., 2017; Wen et al., 2017, 2019). In contrast, some crops with a slow root growth rate demonstrated intensive exudation of P-mobilizing compounds, such as carboxylates and acid phosphatase, in response to P deficiency (Wen et al., 2019, 2020; Koester et al., 2021). Microbial biomass turnover mediated by the growth/death of microorganisms, including phosphate-solubilizing microorganisms (PSM), is critical in increasing soil P availability (Bi et al., 2020; Huang et al., 2021; Raymond et al., 2021). Root morphological and/or exudation traits influencing P acquisition would differ in response to microbial P mobilization and among crop species.
Organic amendments, via providing available carbon (C) to microorganisms, trigger the growth of bacteria and fungi, stimulating P immobilization initially, followed by P release from necrotic microbial biomass (Jing et al., 2017; Chen et al., 2019). The dynamic growth and then death (P immobilization and then release) underpin a balance between root morphological and exudation traits related to high P-use efficiency (Marschner et al., 2011; Richardson et al., 2011). For instance, the high microbial abundance leads to intensive root–microbial competition and causes slow growth and short length of roots, suggesting P-conservative strategies underlying increased crop P utilization efficiency (Marschner et al., 2011; Richardson and Simpson, 2011; Cortois et al., 2016). In contrast, P release from necrotic microbial biomass governs acquisitive root morphological and/or exudation traits to enable crop P uptake (Richardson et al., 2011; Ma et al., 2018; Chen et al., 2019). Because of the interspecific variation in root P-acquisition strategies (Wen et al., 2019, 2022), clarifying the dynamics in microbial effects on root morphological and exudation traits among diverse crop species will elucidate the rhizosphere mechanisms underlying root–microbe interactions that influence crop P nutrition and growth.
Phosphorus fertilization increases microbial growth and abundance, driving a high flux of P into microbial biomass when sufficient amounts of available C are present (Chen et al., 2019; Teste et al., 2021). Given that root–microbe competition intensifies due to microbial growth after organic amendment, supplementing available P via fertilization would diminish microbial competition and drive the shifts toward acquisitive root strategies (Marschner et al., 2011; Richardson et al., 2011; Ma et al., 2018; Lynch, 2019). The high P release from necrotic microbial biomass as well as direct supplementation of available P expands the size of plant-available P pool in the P-fertilized compared with non-fertilized soil. Among species, crops with diverse types of root system exhibit distinct morphological and exudation plasticity in response to contrasting soil P conditions (Wen et al., 2019). Nevertheless, only very few studies investigated dynamics of root morphological and/or exudation traits related to crop P acquisition depending on the addition of organic materials and P fertilization.
Organic amendment is important to facilitate crop production because of stimulating microorganisms to decompose organic materials and enhance nutrient cycling (Luo et al., 2019; Huang et al., 2021). Such effects are significant for vegetable crops because the addition of organic materials can supply C to microorganisms in soils containing luxury P, but limited C (Yan et al., 2013), thus activating microbial growth and triggering microbially mediated P cycling (Čapek et al., 2018; Soong et al., 2020; Koester et al., 2021). Increasing microbially mediated P bioavailability stimulates fast and deep root growth (Ma et al., 2018; Lynch, 2019; Zhang et al., 2020).
Because a root diameter is one of the significant traits influencing the effects of microbial turnover to crop P acquisition (Hodge et al., 2000), we selected Brassica chinensis (B. chinensis), Solanum lycopersicum (S. lycopersicum), Lactuca sativa (L. sativa), and Vigna unguiculata (V. unguiculata) with distinct average root diameters as the targeted crops to investigate crop growth potential as influenced by microbial P mobilization. In order to clarify the mechanisms underlying root–microbe interactions related to crop P acquisition, root morphological and exudation traits of the four crops in response to microbial P were characterized in a pot experiment with various straw and P additions.
Specifically, we tested the following hypotheses: (1) Wide interspecific variation of root morphological and/or exudation traits exists when B. chinensis, S. lycopersicum, L. sativa, and V. unguiculata adapt to microbial growth/death accompanied by P immobilization/release in straw-amended compared with soil without straw and (2) P fertilization plays a major role in mediating root–microbe interactions, influencing benefits of microbial P mobilization to crop P acquisition and growth.
Materials and Methods
Experimental Setup
There were 16 treatment combinations of P supply (no-P and 100 mg P kg–1 soil) and straw (with and without), together with four common vegetable crops (B. chinensis, S. lycopersicum, L. sativa, and V. unguiculata) to examine the microbial influence on root morphological and/or exudation traits. Two sampling times (24 and 40 days) were set to assess the dynamics of root P-acquisition strategies in response to microbial P immobilization and mobilization after straw addition. Each treatment included four replicates for each sampling time. Therefore, 128 pots were arranged in the experiment.
Soil for the pot experiment was collected from the 0- to 20-cm depth of the paddy soil at Zhuanghang Experimental Station of Shanghai Academy of Agricultural Sciences (30°53′N, 121°22′E) in Shanghai, China. The collected soil was air-dried and passed through a 2-mm sieve. The soil was Gleysol with a bulk density of 1.4 g cm–3, 12.3 g kg–1 organic C, 1.1 g kg–1 total nitrogen (N), 0.8 g kg–1 total P, 43 mg kg–1 mineral N (NO3– + NH4+), 13 mg kg–1 NaHCO3-extractable P, 114 mg kg–1 NH4Ac-extractable potassium (K), and pH of 6.8 (soil/water = 1:5). The methods were according to Bao (2013). Each pot (24 cm diameter at the top, 11 cm at the bottom, and 13 cm high) was filled with 2.5 kg of air-dried soil.
Rice straw collected from farmers after harvesting was oven-dried to constant weight, crushed in a pulverizer, and passed through a 2-mm sieve. Straw was digested in a mixture of K2Cr2O7–H2SO4 to measure organic C (Bao, 2013). Total N, P, and K were determined by digesting 0.5 g of crushed straw using a mixture of 5 mL of concentrated sulfuric acid and 8 mL of 30% v/v H2O2 (Bao, 2013). The straw contained 306 g organic C kg–1, 4.4 g N kg–1, 0.75 g P kg–1, and 11.8 g K kg–1. The addition of straw with the high C/P ratio (408:1) would result in microbial C mineralization being limited by P, thus requiring P uptake from soil to maintain the optimal microbial C/P ratio ranging from 42:1 to 60:1 (Cleveland and Liptzin, 2007; Xu et al., 2013).
To ensure that the supply of other nutrients was adequate for the plant growth, the soil was fertilized with basal nutrients at the following rates (mg kg–1 soil): Ca(NO3)2⋅4H2O, 2250; K2SO4, 267; MgSO4⋅7H2O, 87; Fe-EDTA, 11.7; MnSO4⋅H2O, 13.3; ZnSO4⋅7H2O, 20; CuSO4⋅5H2O, 4; H3BO3, 2.7; and Na2MoO4⋅5H2O, 0.33. Phosphorus in the high-P treatment was applied as Ca(H2PO4)2⋅H2O. Mineral nutrients together with 33 g of rice straw (thus adding 4 g C kg–1 soil) were mixed thoroughly with soil and packed into the pots.
Plant Growth
Based on the popular genotype in the south of China, the genotypes of the four crop species were B. chinensis L. Xiaqing3, S. lycopersicum L. Shenfen16, and L. sativa L. Shenxuan4 (provided by Horticultural Institute of Shanghai Academy of Agricultural Sciences), and V. unguiculata L. Fengchan8 was provided by Horticultural Institute of Guangdong Academy of Agricultural Sciences. Seeds were surface-sterilized with 10% v/v H2O2 for 20 min, rinsed with deionized water, soaked in CaSO4-saturated solution for 12 h, and then germinated in Petri dishes on wet filter paper at 22°C for 2 days. Four seedlings were planted per pot and later thinned to two plants.
The experiment was conducted in a sunlight glasshouse at Zhuanghang Experimental Station of Shanghai Academy of Agricultural Sciences. Glasshouse temperature was maintained at 21–25°C during the day and 13–16°C at night. We ventilated the glasshouse every day to remain natural humidity. The pots were arranged in a completely randomized design and were re-randomized weekly. The plants were gently watered every day by weight to maintain field capacity (30%, v/v) and were grown for 40 days.
Harvest and Measurements
In a preliminary experiment from April to May in the year of 2017, the dynamics of CO2 emissions indicated that the peak rate of mineralization of rice straw occurred 20 days after addition, with 13% of total straw-derived CO2–C released at that time (see also Zhu et al., 2018). Hence, plants were harvested at 24 days (after the maximum initial flush of C) and 40 days after sowing (prolonged release of C at a low rate; see also Zhu et al., 2018) and were separated into shoots and roots.
Shoot P Content and Root Traits
The shoots of B. chinensis, S. lycopersicum, L. sativa, and V. unguiculata were oven-dried at 105°C for 30 min and then at 65°C to constant weight for shoot biomass determination. Roots were carefully brushed, removed from the soil, and gently shaken to remove the loosely adhering soil (considered to be bulk soil), with the soil tightly adhering to roots being defined as rhizosphere soil. Roots with rhizosphere soil were then transferred to a vial containing 50 mL of 0.2 mM CaCl2 and gently shaken to dislodge the rhizosphere soil (Pearse et al., 2007). Care was taken to minimize root damage.
A subsample (8 mL) of the rhizosphere soil suspension was filtered immediately for the determination of rhizosphere carboxylates by high-performance liquid chromatography (modified from Shen et al., 2003). Two drops of microbial inhibitor Micropur (Sicheres Trinkwasser, Munich, Germany) at 0.01 g L–1 were added before storage at –20°C until analysis of carboxylates. When measuring, the filter liquor was passed through cation and then anion exchange resin columns. The eluant was concentrated in a rotary evaporator. The residue was dissolved in dilute HClO4 solution with pH of 2.1 and then separated on a Bio-Rad Aminex HPX-87h sulfonic column with eluent containing 5 mM L–1 H2SO4 at 50°C at a flow rate of 0.5 mL min–1. Carboxylates were measured spectrophotometrically at 210 nm. Total carboxylates in the rhizosphere soil were analyzed according to Cawthray (2003).
The activity of acid phosphatase in the rhizosphere soil was measured according to Neumann (2006). Two 0.5-mL aliquots of soil suspension were transferred into 2-mL Eppendorf tubes, with 0.4 mL sodium acetate buffer and 0.1 mL p-nitrophenyl phosphate (NPP) substrate added. One tube was supplemented with 0.5 mL of 0.5 M NaOH and then incubated at 30°C for 60 min, whereas the other tube was first incubated at 30°C for 60 min, and then, the reaction was terminated by adding 0.5 mL of 0.5 M NaOH. Absorption of the two samples was measured spectrophotometrically (UVmini-1240; Shimadzu, Kyoto, Japan) at 405 nm.
After dislodging the rhizosphere soil, roots were placed in an icebox for transport to the laboratory and were then rinsed with tap water. No nodule was found in V. unguiculata roots. The roots were scanned on an EPSON root scanner at 400 dot-per-inch resolution (Epson Expression 1600 pro, Model EU-35, Tokyo, Japan). The total root length (TRL) and average root diameter (RD) were analyzed by Win-RHIZO software (Regent Instruments Inc., Sainte-Foy, Quebec, QC, Canada).
The dried shoots were crushed in a pulverizer and passed through a 2-mm sieve. Phosphorus concentration in shoots was determined by digesting 0.5 g of crushed shoots using a mixture of 5 mL of concentrated sulfuric acid and 8 mL of 30% v/v H2O2 (Bao, 2013). Shoot P was analyzed by the molybdovanadophosphate method spectrophotometrically (UVmini-1240; Shimadzu, Kyoto, Japan) at 450 nm (Johnson and Ulrich, 1959).
Microbial Traits
At each harvest, two samples of fresh bulk soil from each pot were collected, thoroughly mixed, and grounded to pass through a 2-mm sieve. One sample of the fresh soil was used for the determination of microbial biomass C (MBC) and P content (MBP). The microbial C and P contents were determined using the chloroform fumigation–extraction method (Brookes et al., 1982; Wu et al., 1990). In brief, fresh soil equivalent to 10 g of air-dried soil was fumigated with 100 mL of ethanol-free CHCl3 at room temperature for 48 h. Simultaneously, another sample of fresh soil was treated in the same way, but without ethanol-free CHCl3. Organic C was extracted from the fumigated and non-fumigated samples in 40 mL of 0.5 M K2SO4 and was measured using a TOC/TN analyzer (Multi N/C 2100S, Analytik Jena AG, Jena, Germany). The value of 0.45 was used for the fraction of biomass C mineralized (Wu et al., 1990).
For MBP analysis, samples of fresh bulk soil equivalent to 5 g of air-dried soil were weighed into crucibles and fumigated in a desiccator as per the methods for measuring MBC detailed above. Dissolved P was extracted from the fumigated and non-fumigated samples using a solution of 0.5 M NaHCO3 with a 1:10 soil-to-solution ratio (Morel et al., 1996), and P was determined using the molybdenum blue assay (Murphy and Riley, 1962). The correction for adsorption of P during fumigation was made by simultaneously equilibrating non-fumigated soil with a series of P-containing standard solutions followed by extraction with NaHCO3 (Morel et al., 1996). The amount of microbial P was estimated by using a Kp factor of 0.4 (Brookes et al., 1982).
The bulk soil samples for examining quantity of genes in bacterial (16S rRNA) and fungal (ITS) microbiome as well as phosphate-solubilizing functional microbes encoding phoD and pqqC genes were stored at –80°C until DNA extraction and quantification. Copy numbers of the 16S rRNA (Janssen, 2006; Luo et al., 2020) and ITS (Anderson and Parkin, 2007; Luo et al., 2020) genes as well as the phoD-encoding phosphomonoesterase facilitating organic P mineralization (Fraser et al., 2015; Luo et al., 2019) and the ppqC-encoding pyrroloquinoline-quinone synthase catalyzing inorganic P dissolution (Zheng et al., 2019; Bi et al., 2020) were measured in three replicates in each sample. We extracted total genomic soil DNA from 0.25 g of soil using a DNeasy Power Soil DNA Isolation Kit (MoBio Laboratories, Carlsbad, CA, United States) according to the manufacturer’s protocols, and stored the extracted DNA at –20°C for subsequent quantitative PCR (qPCR) quantification.
The copies of 16S rRNA, ITS, phoD, and pqqC genes in bulk soil were measured according to Jing et al. (2017). Extracted DNA was examined with a NanoDrop spectrophotometer (NanoDrop ND-2000c Technologies, Wilmington, DE, United States) and visually checked on agarose gel (1%) before qPCR quantification. Quantitative PCR was carried out using SYBR Premix ExTaqTm Kit (Takara Biotech, Dalian, China). The primers and amplification protocol of genes are listed in Supplementary Table 1. The 25-μL reaction mixture contained 12.5 μL of SYBR matrix, 1 μL primer sets, 2.5 μL template DNA, and 8 μL ultrapure water. A single clone containing the correct insert was grown in Luria–Bertani media, and plasmid DNA was then extracted, purified, and quantified. A tenfold series of dilution of the plasmid DNA was then carried out to generate a standard curve covering six orders of magnitude from 103 to 1011 copies of the template per assay. Blanks were always run with water instead of DNA extract. The final gene quantities were obtained by calibrating against total DNA concentrations extracted from the soil.
Statistical Analyzes
Analysis of variance (ANOVA) was conducted using SPSS version 26.0 (IBM SPSS Inc., Chicago, IL, United States) after normality test of the data. Significant differences among means were separated by Tukey’s test at 5% probability (p ≤ 0.05). Crop P content and shoot biomass, root morphological and exudation traits as well as microbial traits were subjected to two-way ANOVA to assess the effects of straw, soil P supply, and their interaction at days 24 and 40 separately. The Student t-test (p ≤ 0.05) was used to test the difference in each parameter between days 24 and 40.
Principal component analysis (PCA) was performed with the R “prcomp” function to test the difference in microbial effects on root morphological and exudation traits between with and without P fertilizer soils at 24 and 40 days. We used PCA to determine the multivariate ordination of the six soil microbial traits (MBC and MBP, bacterial and fungal microbial abundance as well as phosphate-solubilizing microorganisms in terms of phoD and pqqC gene abundance) with four root P-acquisition traits (total root length and average diameter as well as rhizosphere carboxylates and acid phosphatase) separately in the B. chinensis, S. lycopersicum, L. sativa, and V. unguiculata treatments. PCA plots were drawn with the FAC-TOEXTRA package in R (Kassambara and Mundt, 2017). Furthermore, we calculated the post hoc Spearman’s rank correlations to assess relationships between individual root traits and the six soil microbial traits.
Results
Shoot Biomass Accumulation and P Acquisition by the Four Crops
Straw addition and P fertilization influenced shoot biomass and P acquisition by B. chinensis, S. lycopersicum, and V. unguiculata, but not L. sativa, at day 24 (Figure 1 and Supplementary Tables 2, 3). Straw addition caused the low shoot biomass and P content in B. chinensis in no-P soil with compared to without straw amendment at day 24 (Figure 1A and Supplementary Tables 2, 3). Shoot biomass and P content in S. lycopersicum were decreased due to straw addition compared with the no-straw treatments regardless of P supply at day 24. Conversely, crop P acquisition by S. lycopersicum in no-P soil was increased after straw addition at day 40 (Figure 1B). Biomass accumulation and P acquisition by V. unguiculata were lower in the straw-amended than no-straw soil in both with and without P fertilizer at days 24 and 40 (Figure 1D). In straw-amended soil, high P application increased shoot biomass and shoot P content of V. unguiculata at day 40 when compared with non-fertilized crops (Figure 1D).
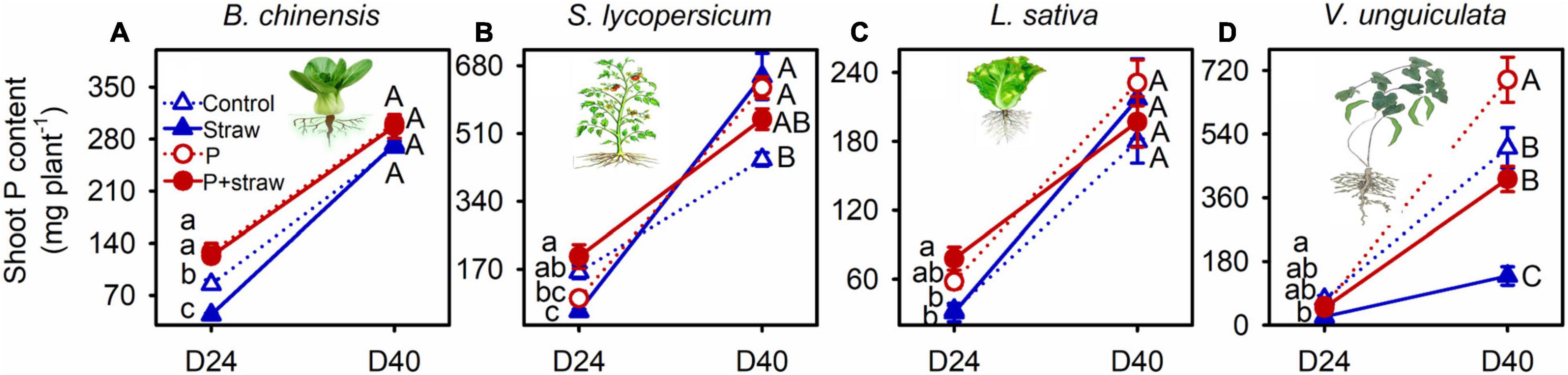
Figure 1. Effects of straw addition and P fertilization on shoot P content in B. chinensis (A), S. lycopersicum (B), L. sativa (C), and V. unguiculata (D) at days 24 and 40. Control: no fertilizer P or straw; straw: no P but with straw addition; P: fertilizer P at 100 mg P kg–1 soil; P + straw: fertilizer P at 100 mg P kg–1 soil together with straw addition. D24: 24 days after straw addition, and D40: 40 days after straw addition. Data are means ± SE (n = 4). For each crop species, the lowercase letters denote significant differences (p ≤ 0.05) among treatments without P or straw, with straw but no fertilizer P, with fertilizer P but no straw, and with fertilizer P and straw at day 24, and the capital letters denote significant differences (p ≤ 0.05) among the four treatments at day 40. Significant difference (p ≤ 0.001) in shoot P content of the four crops between days 24 and 40 was detected using Student’s t-tests (see Supplementary Table 3).
Root P-Acquisition Strategies of the Four Crops
Straw together with P application influenced root P-acquisition strategies of the four crops (Supplementary Table 2). B. chinensis produced short thick roots in response to straw addition in the no- and high-P soils at day 24 and modified the root traits toward strong exudation of carboxylates and acid phosphatase at day 40 (Figures 2A,B and Supplementary Tables 4, 5). Straw addition was associated with short roots in S. lycopersicum regardless of P supply at day 24; however, S. lycopersicum shifted root traits toward high root elongation accompanied by strong carboxylates and acid phosphatase exudation at day 40 in soil without P fertilizer (Figures 2C,D). Both L. sativa and V. unguiculata produced short roots in response to straw addition regardless of P fertilization at days 24 and 40 (except L. sativa in the high-P soil at day 24; Figures 2E,G). High P application in the straw-amended soil increased root length and exudation of carboxylates by L. sativa and V. unguiculata but decreased carboxylate exudation by S. lycopersicum compared with the no-P supply at day 40 (Figures 2D–H).
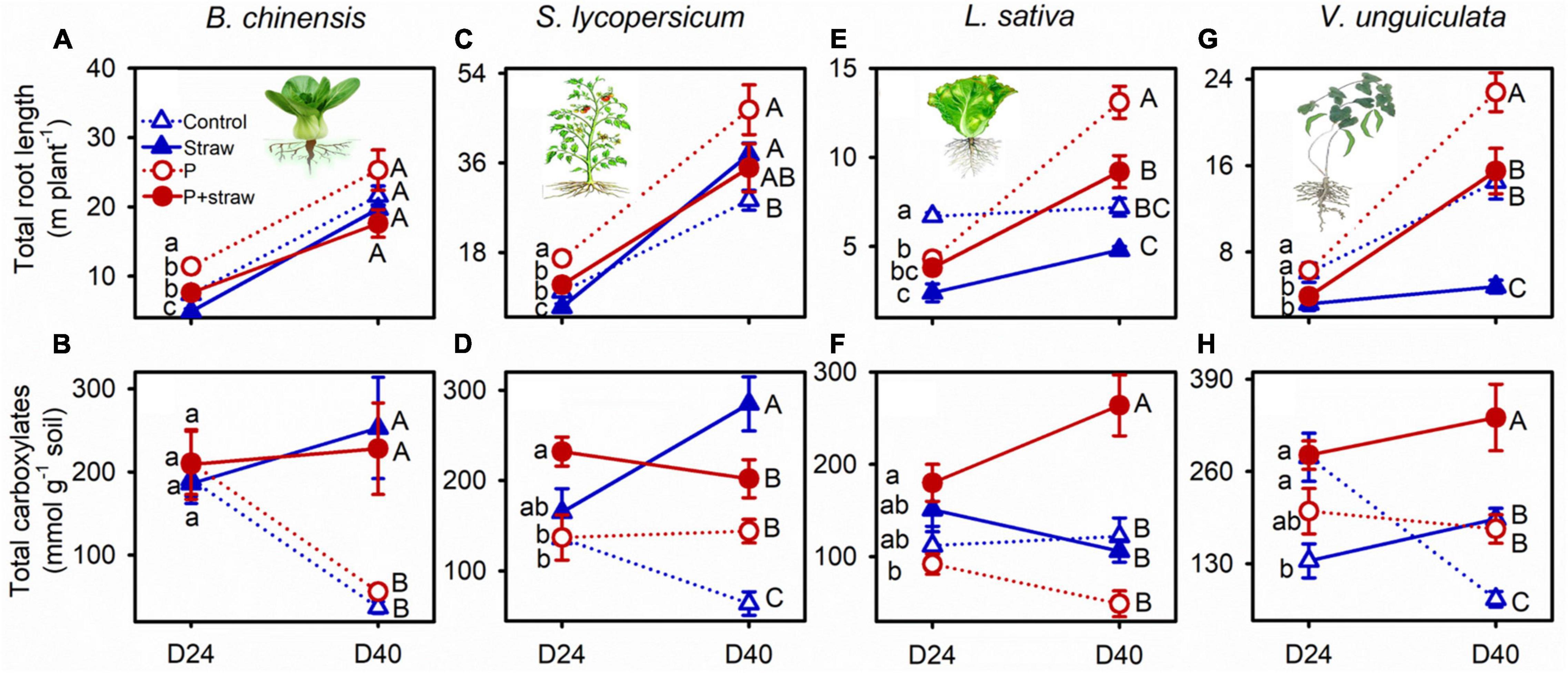
Figure 2. Effects of straw addition and P fertilization on root length and exudation of carboxylates in the rhizosphere of B. chinensis (A,B), S. lycopersicum (C,D), L. sativa (E,F), and V. unguiculata (G,H) at days 24 and 40. Control: no fertilizer P or straw; straw: no P but with straw addition; P: fertilizer P at 100 mg P kg–1 soil; P + straw: fertilizer P at 100 mg P kg–1 soil together with straw addition. D24: 24 days after straw addition, and D40: 40 days after straw addition. Data are means ± SE (n = 4). For each crop species and a given parameter, the lowercase letters denote significant differences (p ≤ 0.05) among treatments without P or straw, with straw but no fertilizer P, with fertilizer P but no straw, and with fertilizer P and straw at day 24, and the capital letters denote significant differences (p ≤ 0.05) among the four treatments at day 40.
Microbial Biomass and Functionality
Straw addition stimulated bacterial and fungal proliferation at day 24 (except fungi in the no-P soil in which S. lycopersicum and V. unguiculata grew), independent of P fertilization (Supplementary Table 6). The abundance of phosphate-solubilizing microorganisms encoding phoD gene in the no-P soil (as well as in the P-fertilized soil with B. chinensis and S. lycopersicum) and the functional microorganisms encoding pqqC gene in the P-fertilized soil was increased after straw addition (Supplementary Table 7). The abundance of bacteria and fungi as well as phosphate-solubilizing functional microbes encoding phoD and pqqC genes remained stable (or even increased) in the straw-amended soil regardless of P fertilization from days 24 to 40 (Supplementary Tables 6, 7).
At day 24, the amounts of microbial biomass C and P were high in the P-fertilized and non-fertilized soils compared to without straw amendment regardless of crop species (Figure 3 and Supplementary Table 8). Under B. chinensis and S. lycopersicum, microbial P decreased in the straw-amended soil regardless of P fertilization (Figures 3B,D and Supplementary Table 8). Conversely, microbial P remained stable in straw soil but increased in the straw and P soil in which L. sativa and V. unguiculata grew from days 24 to 40 (Figures 3F,H and Supplementary Table 7).
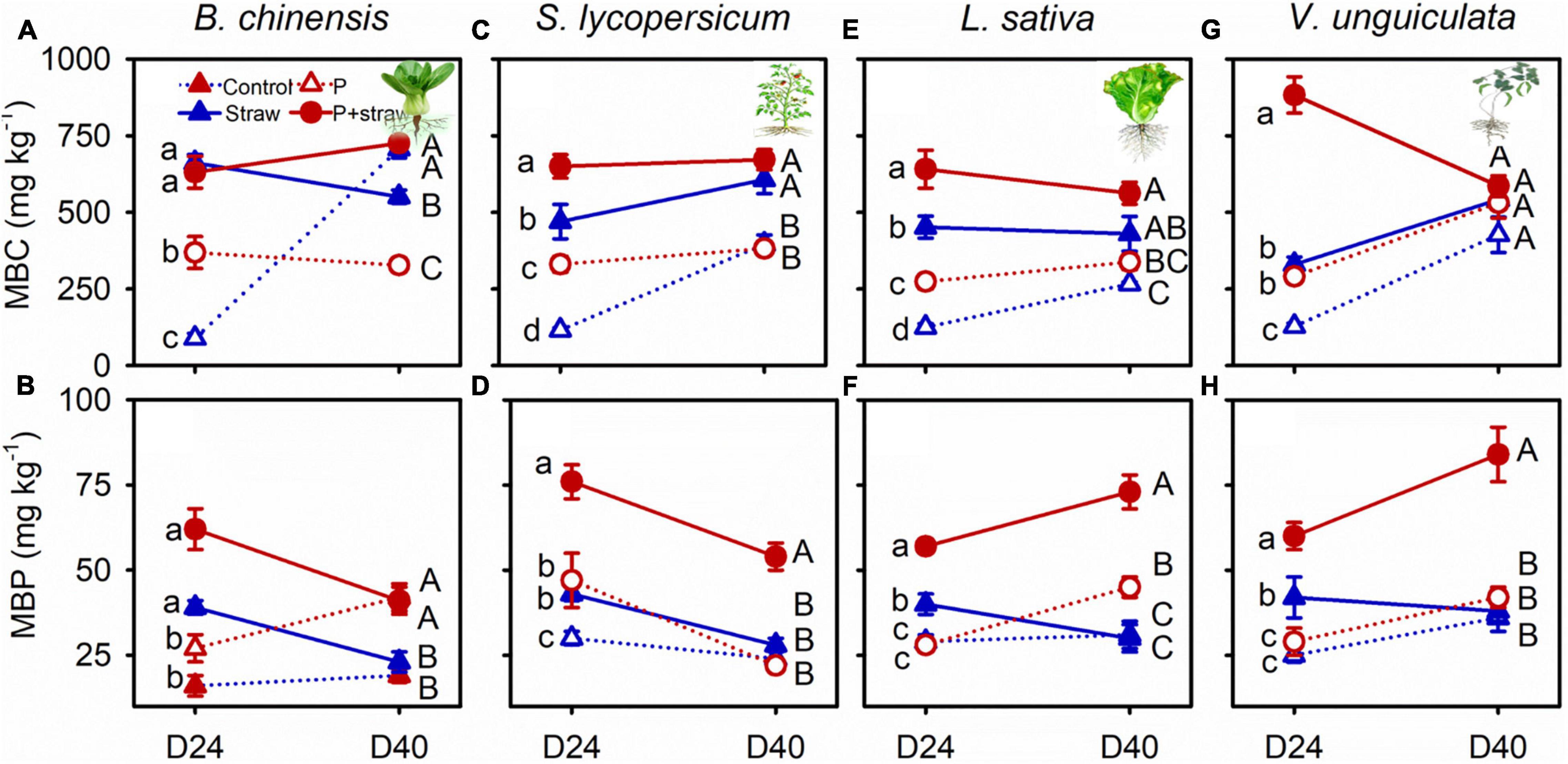
Figure 3. Effects of straw addition and P fertilization on MBC (microbial biomass C) and MBP (microbial biomass P) in soil with B. chinensis (A,B), S. lycopersicum (C,D), L. sativa (E,F), and V. unguiculata (G,H) after days 24 and 40 days. Control: no fertilizer P or straw; straw: no P but with straw addition; P: fertilizer P at 100 mg P kg–1 soil; P + straw: fertilizer P at 100 mg P kg–1 soil together with straw addition. D24: 24 days after straw addition, and D40: 40 days after straw addition. Data are means ± SE (n = 4). For each crop species and a given parameter, the lowercase letters denote significant differences (p ≤ 0.05) among treatments without P or straw, with straw but no fertilizer P, with fertilizer P but no straw, and with fertilizer P and straw at day 24, and the capital letters denote significant differences (p ≤ 0.05) among the four treatments at day 40.
Multivariate Coordination
The abundance of bacteria and fungi that included phosphate-solubilizing microorganisms encoding phoD and pqqC genes was positively associated with exudation of carboxylates at days 24 and 40 (Figure 4). The total root length was governed by a high abundance of bacteria in soil at day 24 after straw addition. Microbial C was positively correlated with exudation of carboxylates and acid phosphatase, and microbial P was positively associated with carboxylate exudation at day 40. Microbial P was negatively correlated with the total root length (p = 0.068), but positively correlated with a root diameter at day 40. Specifically, the root diameter and microbial P were scattered in the direction of L. sativa and V. unguiculata, whereas the total root length was located in the direction of B. chinensis and S. lycopersicum at day 40.
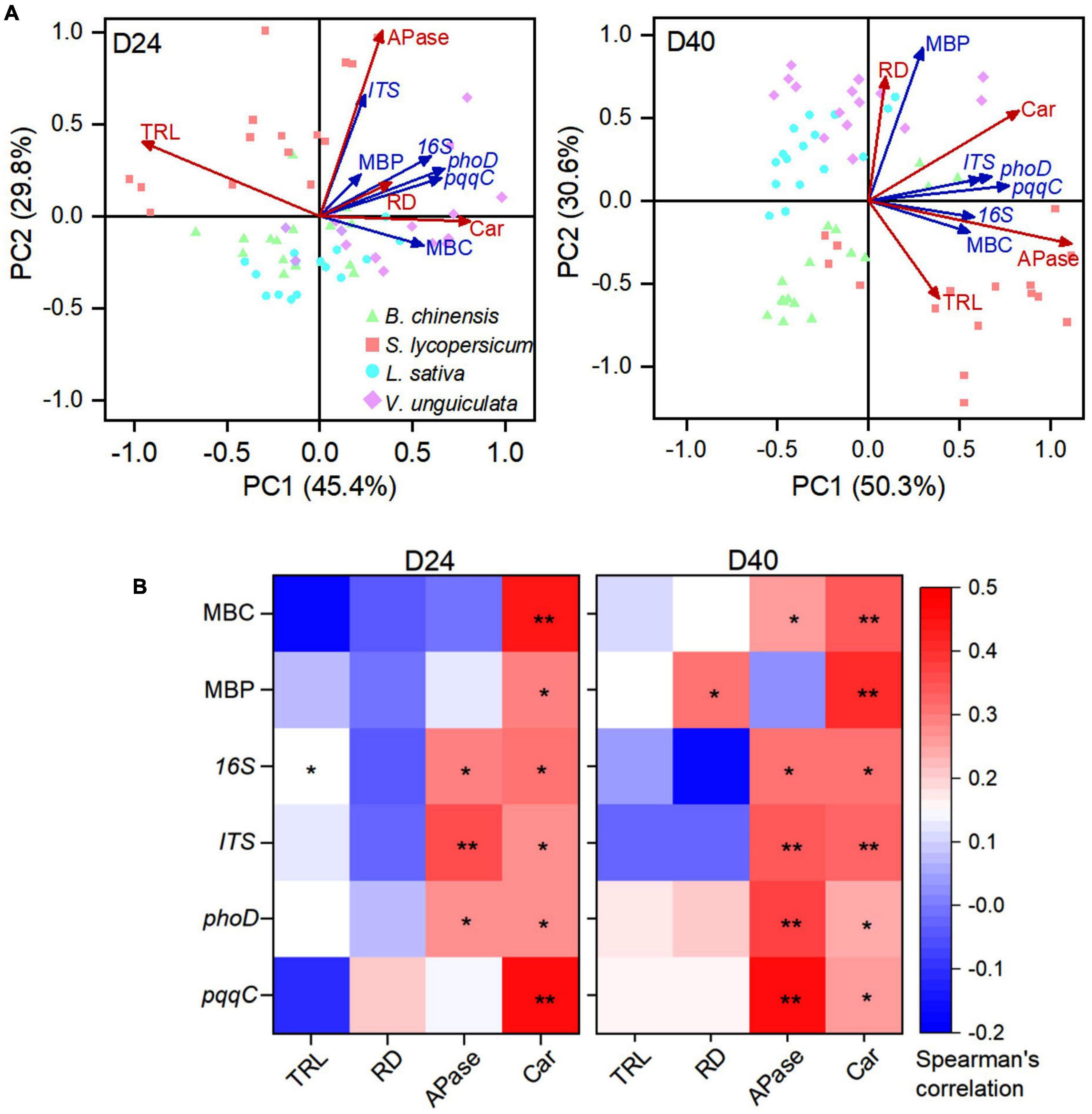
Figure 4. (A) Principal component analysis (PCA) and (B) spearman’s rank correlation matrices between four root traits and six soil microbe traits B. chinensis, S. lycopersicum, L. sativa, and V. unguiculata after 24 and 40 days. D24: 24 days after straw addition, and D40: 40 days after straw addition. Root trait abbreviations: TRL, total root length; RD, average root diameter; Car, concentration of carboxylates in the rhizosphere soil; and APase, acid phosphatase activity in the rhizosphere soil. Microbial trait abbreviations: MBC: microbial biomass C content; MBP: microbial biomass P content; 16S: abundance of bacteria; ITS: abundance of fungi; phoD: abundance of phoD gene; and pqqC: abundance of pqqC gene. Asterisks in (B) indicate statistical significance (**, p ≤ 0.01; *, p ≤ 0.05).
Discussion
Straw Application Coupled With P Fertilization Increased Microbial P
The abundance of bacteria and fungi including phosphate-solubilizing microbial groups encoding phoD and pqqC genes was increased at day 24 after straw addition (Supplementary Tables 6, 7). This was mainly because organic materials supplied C to stimulate the growth of bacteria and fungi that included phosphate-solubilizing functional groups (Jing et al., 2017; Chen et al., 2019; Huang et al., 2021). Microbial growth immobilized orthophosphate in soil (Chen et al., 2019), resulting in high microbial P in straw-amended compared with no-straw soil at day 24 (Figure 3 and Supplementary Table 8). Microbial cells turn over more rapidly than plant roots do, therefore releasing nutrient back into the soil, whereas plants are able to retain captured nutrient to a greater extent (Hodge et al., 2000). Although organic C is depleted in soil over long time, carboxylates and acid phosphatase represent C-containing substrates to rhizosphere microbes (Bicharanloo et al., 2020; Ding et al., 2021), underpinning stable microbial biomass C in the straw-amended compared with no-straw soil from days 24 to 40 (Figures 2, 3, 4 and Supplementary Tables 5, 8). Phosphorus acquisition in B. chinensis and S. lycopersicum was associated with a decline in microbial P in straw-amended soil from days 24 to 40 (Figures 1A,B, 3B,D and Supplementary Table 8). In contrast, microbial P remained stable in straw-amended soil under L. sativa and V. unguiculata, resulting from the slow and small P acquisition in the two crops during days 24 to 40 (Figures 1C,D, 3F,H and Supplementary Table 8).
Phosphorus fertilization maximized microbial P pool when there was sufficient available C (Chen et al., 2019), resulting in high microbial P in soil amended with straw and P compared to that without P fertilizer during the first 24 days (Figures 3B,D,F,H). Because high amounts of available P supplemented via fertilization satisfied nutrient demand for crops and microbes from days 24 to 40 (Figure 1), in straw soil microbial P remained high in P-fertilized compared to non-fertilized treatments at day 40 (Figures 3B,D,F,H). The results indicated that straw together with P fertilizer governed high microbial P to determine large crop P acquisition.
Root P-Acquisition Strategies in Response to Microbial P Differed Among Crops
Microbial growth and P immobilization indicated intensive competition between roots and microbes (Marschner et al., 2011; Richardson et al., 2011). The tested four crops produced short roots in the straw-amended compared with no-straw soil during the first 24 days (Figures 2A,C,E,G), suggesting P-conservative root traits based on slow elongation under microbial growth (McCormack et al., 2015; Cortois et al., 2016). The slow root growth conferred stress resistance by reducing C demand for roots, thereby allowing for great investment in defense traits (e.g., high root tissue density) to tolerate microbial proliferation (Chapin et al., 1993; Valverde-Barrantes et al., 2017). Furthermore, the slow growth of roots indicated crops produced roots with long life span and low turnover rate in order to decrease P loss via shed cells and increase nutrient conservation for high use efficiency in shoots (McCormack et al., 2015). Crops, regardless of species, decreased root elongation to avoid microbial competition and conserve P for the growth during the first 24 days after straw addition.
The bacterial abundance remained high in straw-amended compared with no-straw soil B. chinensis growing at days 24 and 40 (Supplementary Table 6). Conversely, the fungal abundance was high in straw than no-straw soil at day 24, but became the same level as those in no-straw soil at day 40 (Supplementary Table 6). The results indicated a decline in root-microbe competition in straw-amended soil under B. chinensis at day 40 compared with day 24. In straw-amended soil under S. lycopersicum, L. sativa, and V. unguiculata, the bacterial and fungal abundance remained the same level as those without straw added at day 40 (Supplementary Table 6). The results indicated root–microbial competition was weak to determine root traits in straw-amended soil at day 40 compared with day 24.
Microbial P turnover was important in increasing plant-available P (Raymond et al., 2021; Lu et al., 2022). In soil with weak root-microbe competition at day 40, soil P availability became important to determine root P-acquisition strategies (Figure 4). In straw-amended soil, B. chinensis and S. lycopersisucm produced roots to reach or exceed crops in no-straw soil at day 40, indicating fast growth during days 24 to 40 to compensate the short length at day 24 (Figures 2A,C). Absorptive long roots and strong carboxylate exudation are involved in efficient nutrient acquisition, especially in competition with microorganisms (Lynch, 2019). Crop P content of thick-root species mainly relied on exudation of P-mobilizing compounds under P deficiency (Shen et al., 2011; Wen et al., 2020). Strong carboxylate exudation by L. sativa and V. unguiculata indicated crops adopted P-acquisitive root traits in straw-amend soil compared with those in no-straw soil at day 40 (Figure 2). In summary, the four tested crops adopted conservative strategies based on short length during the first 24 days after straw addition, whereas each tested crop species adopted specific root P-acquisition strategies in response to microbial P turnover during days 24 to 40 (Figure 5).
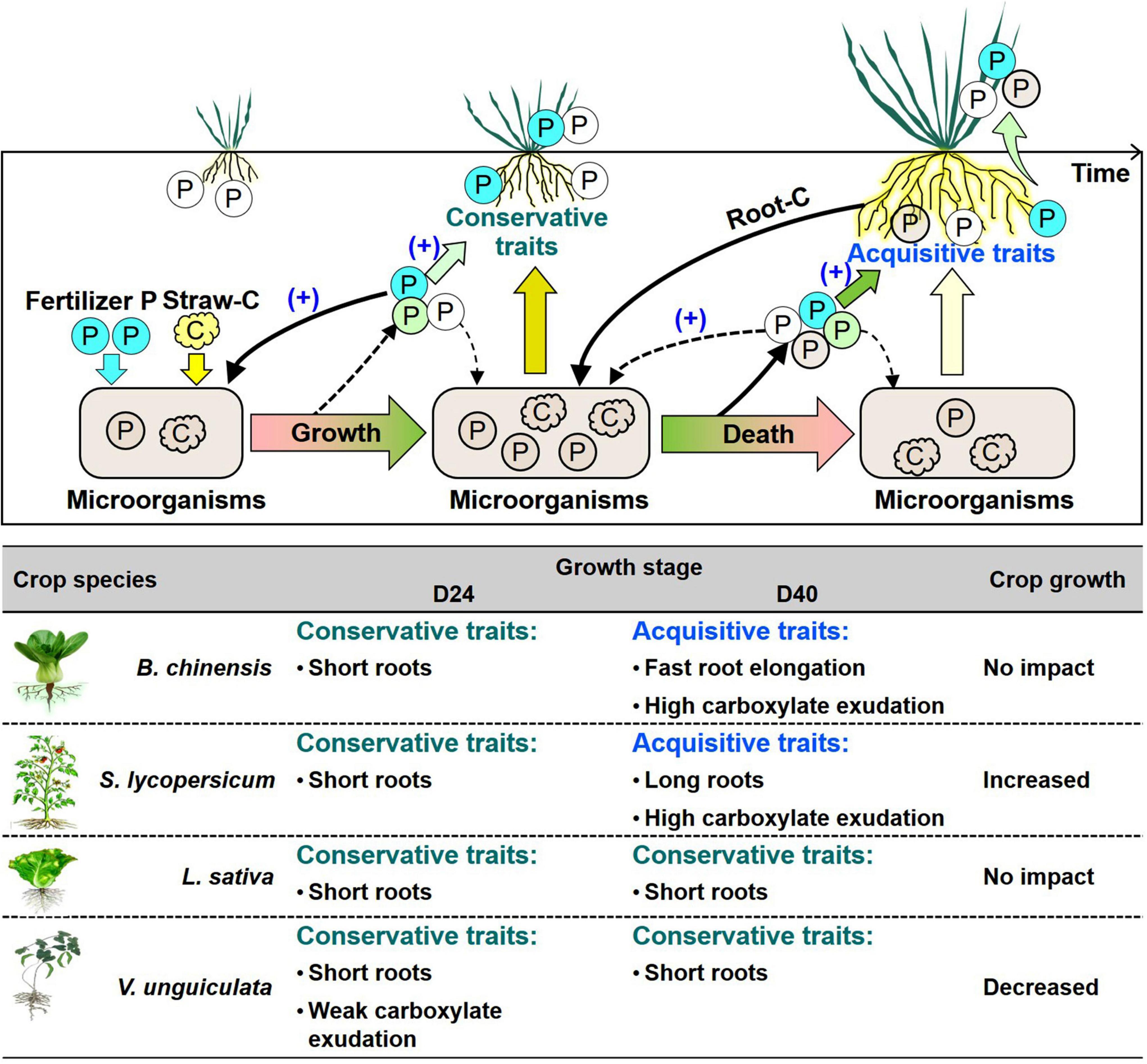
Figure 5. Framework of the root-microbe interactions driven by straw addition and P fertilization as well as the role of these interactions in P uptake by B. chinensis, S. lycopersicum, L. sativa, and V. unguiculata. The solid-line black arrows indicate the strong interactions among soil P, microbes, and roots influencing plant growth, whereas the dashed arrows denote the relatively weak effects. The white circles indicate the available P in soil; the blue circles denote the added fertilizer P; and the pink circles represent the processes of P release from microbial biomass to the plant-available P pool, but no supporting data are available so far. The table denotes the changes in root morphological and exudation traits with straw addition in comparison with roots without straw addition after day 24 (D24) and 40 days (D40). A dark green arrow indicates the strong influence of soil P on root traits, whereas a light green one indicates the weak influence. A light brown arrow indicates the strong influence of microbes on root traits, whereas a light yellow one denotes the weak influence. The plus in parentheses indicates the P fertilization increases the role of P in governing root traits related to crop P acquisition and microbial P immobilization.
Soil P availability was significant to root P-acquisitive traits but differed highly among crop species at day 40 after straw addition (Figure 4). For instance, P fertilization in the straw-amended soil governed fast root elongation and strong exudation of carboxylates by L. sativa and V. unguiculata at day 40, determining efficiency P-acquisitive traits to recovery from microbial competition (Figures 2E–H). In contrast, when soil P was sufficiency to crops, in straw-amended soil P fertilization diminished exudation of carboxylates and acid phosphatase by S. lycopersicum (Figures 2C,D). Root–microbe interactions under straw amendment highly depended on P fertilization (Figure 5).
Phosphorus Acquisition Underlying Root–Microbe Interactions Determined by Straw Amendment, P Fertilization, and Crop Species
Short roots and microbial P immobilization decreased shoot P content in B. chinensis and S. lycopersicum in straw compared to those without straw at day 24 (Figures 1A,B). Root elongation accompanied by strong carboxylate exudation together with microbial P release suggested efficient P acquisition and shoot accumulation (Lynch, 2019; Raymond et al., 2021; Lu et al., 2022), such as in B. chinensis and S. lycopersicum with straw treatments from days 24 to 40 (Figures 2A–2D, 3B,D). Such high crop P acquisition compensated the low shoot P content before day 24, eliciting similar crop P content in B. chinensis and even larger shoot P content in S. lycopersicum in the straw-amended compared with no-straw soil at day 40 (Figures 1A,B). The short roots and low carboxylate exudation resulted in low shoot P content in V. unguiculata in the straw-amended compared with no-straw soil across the experiment, whereas L. sativa was influenced little due to having the lowest P demand among the four crops (Figures 1C,D).
A large pool of plant-available P (in the high-P compared with no-P soil) eliminated P limitation imposed by microbially derived P immobilization (Spohn and Widdig, 2017; Chen et al., 2019), underpinning crop P acquisition by the four tested species under microbial competition (Figure 1). Root elongation accompanied by strong exudation of carboxylates indicated high P acquisition by L. sativa and V. unguiculata in the P + straw compared with the straw without P soil at day 40 (Figure 1D; Lynch, 2019). By contrast, sufficient P decreased carboxylate exudation of S. lycopersicum at day 40 (Figures 2C,D), potentially limiting benefits to crop growth from mobilization of microbial P. In conclusion, straw application coupled with P fertilization governed P acquisition (influenced by root–microbe interactions) differently in the four tested crop species.
Highlights
Crop species, straw amendment, and P fertilization need to be considered simultaneously for high P-use efficiency in vegetable cropping systems. S. lycopersicum benefited from microbial P mobilization in no-P soil after straw addition (Figure 2), indicating a high potential of straw-governed microbial P mobilization for increasing P-use efficiency. By contrast, the low shoot P content of thick-rooted V. unguiculata highlighted specific management of P fertilization coupled with straw returning needed to be considered to increase crop P acquisition (Figure 2). The match between fertilizer P application and crop species is critical in increasing P-acquisition efficiency and saving fertilizer via organic material amendments. Further and detailed research is needed to examine how to maximize the benefits of microbial P mobilization to crop P-use efficiency.
The microbial populations in the rhizosphere and bulk soil can differ significantly (Edwards et al., 2018). It could draw attention to provide adequate justification for disparity of microbes between rhizosphere and bulk soil as well as the role in governing dynamic root-microbe interactions. Mycorrhizal symbiosis is the key player in aiding plant nutrient uptake although the dependency of plants on this symbiosis tends to vary (Wen et al., 2019, 2022). Further studies need to clarify how rhizosphere microbial composition governs tradeoffs among root morphology, exudation, and mycorrhizal symbioses for phosphorus-acquisition strategies. The key microbial taxa or microbe-derived phytohormones are important in influencing root morphological and exudation traits related to crop nutrient acquisition (Huang et al., 2021; Sun et al., 2021; Mamet et al., 2022; Xu et al., 2022). It is necessary to clarify the mechanisms of dynamic root-microbe interactions underlying rhizosphere microbial composition or phytohormones in soils treated with organic material and P fertilization.
Conclusion
Straw addition triggered the growth of bacteria and fungi, including phosphate-solubilizing functional groups, to increase microbial P. The high abundance of microorganisms was associated with short roots of the four tested species. In straw-amended soil, B. chinensis and S. lycopersicum shifted from short roots in response to microbial P immobilization to fast root elongation accompanied by strong carboxylate exudation upon release of microbial P. Conversely, after straw addition, L. sativa and V. unguiculata tended to adopt conservative strategies based on short roots. In straw-amended soil, alleviation of P deficiency via fertilization stimulated root growth and carboxylate exudation by L. sativa and V. unguiculata, whereas efficiency in root P-acquisition by S. lycopersicum was decreased compared with the non-fertilized soil. In summary, root P-acquisition strategies in response to microbial P dynamics after straw addition differed among the four crops tested in the present experiment. High-P fertilization may need to be considered together with returning straw to ensure strong crop growth.
Data Availability Statement
The raw data supporting the conclusions of this article will be made available by the authors, without undue reservation.
Author Contributions
DZ, SX, SC, and HZ conceived the ideas and collected and analyzed the data. All authors interpreted the data and revised the manuscript.
Funding
DZ was supported by Shanghai Agriculture Applied Technology Development Program (T20210202), National Natural Science Foundation of China (31801946), Chengdu Science and Technology Bureau Key R&D Program (2021-YF05-01472-SN), and SAAS Program for Excellent Research Team (2022(008)). YK was supported by Government Program of Competitive Growth of Kazan Federal University and the RUDN University Strategic Academic Leadership Program.
Conflict of Interest
The authors declare that the research was conducted in the absence of any commercial or financial relationships that could be construed as a potential conflict of interest.
Publisher’s Note
All claims expressed in this article are solely those of the authors and do not necessarily represent those of their affiliated organizations, or those of the publisher, the editors and the reviewers. Any product that may be evaluated in this article, or claim that may be made by its manufacturer, is not guaranteed or endorsed by the publisher.
Supplementary Material
The Supplementary Material for this article can be found online at: https://www.frontiersin.org/articles/10.3389/fpls.2022.924154/full#supplementary-material
References
Anderson, I. C., and Parkin, P. I. (2007). Detection of active soil fungi by RT-PCR amplification of precursor rRNA molecules. J. Microbiol. Meth. 68, 248–253. doi: 10.1016/j.mimet.2006.08.005
Bao, S. D. (2013). Soil Agricultural Chemistry Analysis. Third version. Beijing: China Agriculture Press.
Bi, Q. F., Li, K. J., Zheng, B. X., Liu, X. P., Li, H. Z., Jin, B. J., et al. (2020). Partial replacement of inorganic phosphorus (P) by organic manure reshapes phosphate mobilizing bacterial community and promotes P bioavailability in a paddy soil. Sci. Total Environ. 703:134977. doi: 10.1016/j.scitotenv.2019.134977
Bicharanloo, B., Shirvan, B. M., Keitel, C., and Dijkstra, F. A. (2020). Rhizodeposition mediates the effect of nitrogen and phosphorous availability on microbial carbon use efficiency and turnover rate. Soil Biol. Biochem. 142:107705. doi: 10.1016/j.soilbio.2020.107705
Brookes, P. C., Powlson, D. S., and Jenkinson, D. S. (1982). Measurement of microbial biomass phosphorus in soil. Soil Biol. Biochem. 14, 319–329.
Čapek, P., Manzoni, S., Kotas, P., Kaštovská, E., Wild, B., Diáková, K., et al. (2018). A plant–microbe interaction framework explaining nutrient effects on primary production. Nat. Ecol. Evol. 2, 1588–1596. doi: 10.1038/s41559-018-0662-8
Cawthray, G. R. (2003). An improved reversed-phase liquid chromatographic method for the analysis of low-molecular mass organic acids in plant root exudates. J. Chromatogr. A. 1011, 233–240. doi: 10.1016/S0021-9673(03)01129-4
Chapin, F. S., Autumn, K., and Pugnaire, F. (1993). Evolution of suites of traits in response to environmental stress. Am. Nat. 142:S78–S92. doi: 10.1086/285524
Chen, J., Seven, J., Zilla, T., Dippold, M. A., Blagodatskaya, E., and Kuzyakov, Y. (2019). Microbial C:N:P stoichiometry and turnover depend on nutrient availability in soil: a 14C, 15N and 33P triple labelling study. Soil Biol. Biochem. 131, 206–216. doi: 10.1016/j.soilbio.2019.01.017
Cleveland, C. C., and Liptzin, D. (2007). C:N:P stoichiometry in soil: is there a “Redfield ratio” for the microbial biomass? Biogeochemistry 85, 235–252. doi: 10.1007/s10533-007-9132-0
Cortois, R., Schröder-Georgi, T., Weigelt, A., van der Putten, W. H., De Deyn, G. B., and van der Heijden, M. (2016). Plant-soil feedbacks: role of plant functional group and plant traits. J. Ecol. 104, 1608–1617. doi: 10.1111/1365-2745.12643
Ding, W., Cong, W.-F., and Lambers, H. (2021). Plant phosphorus-acquisition and -use strategies affect soil carbon cycling. Trends Ecol. Evol. 36, 899–906. doi: 10.1016/j.tree.2021.06.005
Edwards, J. A., Santos-Medellín, C. M., Liechty, Z. S., Nguyen, B., Lurie, E., Eason, S., et al. (2018). Compositional shifts in root-associated bacterial and archaeal microbiota track the plant life cycle in field-grown rice. PLoS Biol. 16:e2003862. doi: 10.1371/journal.pbio.2003862
Fraser, T. D., Lynch, D. H., Bent, E., Entz, M. H., and Dunfield, K. E. (2015). Soil bacterial phoD gene abundance and expression in response to applied phosphorus and long-term management. Soil Biol. Biochem. 88, 137–147. doi: 10.1016/j.soilbio.2015.04.014
Hinsinger, P. (2001). Bioavailability of soil inorganic P in the rhizosphere as affected by root-induced chemical changes: a review. Plant Soil 237, 173–195. doi: 10.1023/A:1013351617532
Hodge, A., Robinson, D., and Fitter, A. (2000). Are microorganisms more effective than plants at competing for nitrogen? Trends Plant Sci. 5, 304–308. doi: 10.1016/S1360-1385(00)01656-3
Huang, Y., Dai, Z., Lin, J., Li, D., Ye, H., Dahlgren, R. A., et al. (2021). Labile carbon facilitated phosphorus solubilization as regulated by bacterial and fungal communities in Zea mays. Soil Biol. Biochem. 163:108465. doi: 10.1016/j.soilbio.2021.108465
Janssen, P. H. (2006). Identifying the dominant soil bacterial taxa in libraries of 16S rRNA and 16S rRNA genes. App. Environ. Microb. 72, 1719–1728. doi: 10.1128/AEM.72.3.1719-1728.2006
Jing, Z., Chen, R., Wei, S., Feng, Y., Zhang, J., and Lin, X. (2017). Response and feedback of C mineralization to P availability driven by soil microorganisms. Soil Biol. Biochem. 105, 111–120. doi: 10.1016/j.soilbio.2016.11.014
Johnson, C. M., and Ulrich, A. (1959). Analytical Methods for Use in Plant Analysis. Berkeley, CA, USA: University of California, Agricultural Experiment Station.
Kassambara, A., and Mundt, F. (2017). Factoextra: extract and visualize the results of multivariate data analyses. R package version 1.0.5. Available online at https://cran.r-project.org/web/packages/factoextra (accessed on 12 Jun. 2018).
Koester, M., Stock, S., Nájera, F., Abdallah, K., Gorbushina, A., Prietzel, J., et al. (2021). From rock eating to vegetarian ecosystems — disentangling processes of phosphorus acquisition across biomes. Geoderma 388:114827. doi: 10.1016/j.geoderma.2020.114827
Lambers, H., Shane, M. W., Cramer, M. D., Pearse, S. J., and Veneklaas, E. J. (2006). Root structure and functioning for efficient acquisition of phosphorus: matching morphological and physiological traits. Ann. Bot. 98, 693–713. doi: 10.1093/aob/mcl114
Li, H., Liu, B., McCormack, M. L., Ma, Z., and Guo, D. (2017). Diverse belowground resource strategies underlie plant species coexistence and spatial distribution in three grasslands along a precipitation gradient. N. Phytol. 216, 1140–1150. doi: 10.1111/nph.14710
Lu, J. L., Jia, P., Feng, S. W., Wang, Y. T., Zheng, J., Ou, S. N., et al. (2022). Remarkable effects of microbial factors on soil phosphorus bioavailability: a country-scale study. Glob. Change Biol. 28, 4459–4471. doi: 10.1111/gcb.16213
Luo, G., Sun, B., Li, L., Li, M., Liu, M., Zhu, Y., et al. (2019). Understanding how long-term organic amendments increase soil phosphatase activities: insight into phoD- and phoC-harboring functional microbial populations. Soil Biol. Biochem. 139:107632. doi: 10.1016/j.soilbio.2019.107632
Luo, G., Xue, C., Jiang, Q., Xiao, Y., Zhang, F., Guo, S., et al. (2020). Soil carbon, nitrogen, and phosphorus cycling microbial populations and their resistance to global change depend on soil C: N: P stoichiometry. mSystems 5:e162–e120. doi: 10.1128/mSystems.00162-20
Lynch, J. P. (2019). Root phenotypes for improved nutrient capture: an underexploited opportunity for global agriculture. N. Phytol. 223, 548–564. doi: 10.1111/nph.15738
Ma, X., Zarebanadkouki, M., Kuzyakov, Y., Blagodatskaya, E., Pausch, J., and Razavi, B. S. (2018). Spatial patterns of enzyme activities in the rhizosphere: effects of root hairs and root radius. Soil Biol. Biochem. 118, 69–78. doi: 10.1016/j.soilbio.2017.12.009
Mamet, S. D., Helgason, B. L., Lamb, E. G., McGillivray, A., Stanley, K. G., Robinson, S. J., et al. (2022). Phenology-dependent root bacteria enhance yield of Brassica napus. Soil Biol. Biochem. 166:108468. doi: 10.1016/j.soilbio.2021.108468
Marschner, P., Crowley, D., and Rengel, Z. (2011). Rhizosphere interactions between microorganisms and plants govern iron and phosphorus acquisition along the root axis – model and research methods. Soil Biol. Biochem. 43, 883–894. doi: 10.1016/j.soilbio.2011.01.005
McCormack, M. L., Dickie, I. A., Eissenstat, D. M., Fahey, T. J., Fernandez, C. W., Guo, D., et al. (2015). Redefining fine roots improves understanding of below-ground contributions to terrestrial biosphere processes. N. Phytol 207, 505–518. doi: 10.1111/nph.13363
Morel, C. H., Tiessen, J., and Stewart, W. B. (1996). Correction for P-sorption in the measurement of soil microbial biomass P by CHCl3 fumigation. Soil Biol. Biochem. 28, 1699–1706. doi: 10.1016/S0038-0717(96)00245-3
Murphy, J., and Riley, J. P. (1962). A modified single solution methods for determination of phosphorus in natural water. Anal. Chem. Acta 27, 31–36.
Neumann, G. (2006). “Quantitative determination of acid phosphatase activity in the rhizosphere and on the root surface,” in Handbook of Methods used in Rhizosphere Research, eds J. Luster and R. Finlay (Birmensdorf, Switzerland: Swiss Federal Research Institute WSL), 426–427.
Pearse, S. J., Veneklaas, E. J., Cawthray, G., Bolland, M. D., and Lambers, H. (2007). Carboxylate composition of root exudates does not relate consistently to a crop species’ ability to use phosphorus from aluminium, iron or calcium phosphate sources. N. Phytol. 173, 181–190. doi: 10.1111/l.1469-8137.2006.01897.x
Raymond, N. S., Gómez-Muñoz, B., Bom, F. J. T., Nybroe, O., Jensen, L. S., and Müller-Stöver, D. S. (2021). Phosphate-solubilising microorganisms for improved crop productivity: a critical assessment. N. Phytol. 229, 1268–1277. doi: 10.1111/nph.16924
Richardson, A. E., Lynch, J. P., Ryan, P. R., Delhaize, E., Smith, F. A., Smith, S. E., et al. (2011). Plant and microbial strategies to improve the phosphorus effi- ciency of agriculture. Plant Soil 349, 121–156. doi: 10.1007/s11104-011-0950-4
Richardson, A. E., and Simpson, R. J. (2011). Soil microorganisms mediating phosphorus availability update on microbial phosphorus. Plant Physiol. 156, 989–996. doi: 10.1104/pp.111.175448
Shen, J., Rengel, Z., Tang, C., and Zhang, F. (2003). Role of phosphorus nutrition in development of cluster roots and release of carboxylates in soil-grown Lupinus albus. Plant Soil 248, 199–206. doi: 10.1023/A:1022375229625
Shen, J., Yuan, L., Zhang, J., Li, H., Bai, Z., Chen, X., et al. (2011). Phosphorus dynamics: from soil to plant. Plant Physiol. 156, 997–1005. doi: 10.1104/pp.111.175232
Soong, J. L., Fuchslueger, L., Maranon-Jimenez, S., Torn, M. S., Janssens, I. A., Penuelas, J., et al. (2020). Microbial carbon limitation: the need for integrating microorganisms into our understanding of ecosystem carbon cycling. Glob. Change Biol. 26, 1953–1961. doi: 10.1111/gcb.14962
Spohn, M., and Widdig, M. (2017). Turnover of carbon and phosphorus in the microbial biomass depending on phosphorus availability. Soil Biol. Biochem. 113, 53–59. doi: 10.1016/j.soilbio.2017.05.017
Sun, A., Jiao, X. Y., Chen, Q., Wu, A. L., Zheng, Y., Lin, Y. X., et al. (2021). Microbial communities in crop phyllosphere and root endosphere are more resistant than soil microbiota to fertilization. Soil Biol. Biochem. 153:108113. doi: 10.1016/j.soilbio.2020.108113
Teste, F. P., Lambers, H., Enowashu, E. E., Laliberté, E., Marhan, S., and Kandeler, E. (2021). Soil microbial communities are driven by the declining availability of cations and phosphorus during ecosystem retrogression. Soil Biol. Biochem. 163:108430. doi: 10.1016/j.soilbio.2021.108430
Valverde-Barrantes, O. J., Freschet, G. T., Roumet, C., and Blackwood, C. B. (2017). A worldview of root traits: the influence of ancestry, growth form, climate and mycorrhizal association on the functional trait variation of fine-root tissues in seed plants. New Phytol. 215:15621573. doi: 10.1111/nph.14571
Wen, Z., Li, H., Shen, J., and Rengel, Z. (2017). Maize responds to low shoot P concentration by altering root morphology rather than increasing root exudation. Plant Soil 416, 377–389. doi: 10.1007/s11104-017-3214-0
Wen, Z., Li, H., Shen, Q., Tang, X., Xiong, C., Li, H., et al. (2019). Tradeoffs among root morphology, exudation and mycorrhizal symbioses for phosphorus-acquisition strategies of 16 crop species. N. Phytol. 223, 882–895. doi: 10.1111/nph.15833
Wen, Z., Pang, J., Tueux, G., Liu, Y., Shen, J., Ryan, M. H., et al. (2020). Contrasting patterns in biomass allocation, root morphology and mycorrhizal symbiosis for phosphorus acquisition among 20 chickpea genotypes with different amounts of rhizosheath carboxylates. Funct. Ecol. 34, 1311–1324. doi: 10.1111/1365-2435.13562
Wen, Z., White, P. J., Shen, J., and Lambers, H. (2022). Linking root exudation to belowground economic traits for resource acquisition. N. Phytol. 233, 1620–1635. doi: 10.1111/nph.17854
Wu, J., Joergensen, R. G., Pommerening, B., Chaussod, R., and Brookes, P. C. (1990). Measurement of soil microbial biomass C by fumigation-extraction–an automated procedure. Soil Biol. Biochem. 22, 1167–1169. doi: 10.1016/0038-0717(90)90046-3
Xu, L., Cao, H., Li, C., Wang, C., He, N., Hu, S., et al. (2022). The importance of rare versus abundant phoD-harboring subcommunities in driving soil alkaline phosphatase activity and available P content in Chinese steppe ecosystems. Soil Biol. Biochem. 164:108491. doi: 10.1016/j.soilbio.2021.108491
Xu, X., Thornton, P. E., and Post, W. M. (2013). A global analysis of soil microbial biomass carbon, nitrogen and phosphorus in terrestrial ecosystems. Glob. Ecol. Biogeogr. 22, 737–749. doi: 10.1111/geb.12029
Yan, Z., Liu, P., Li, Y., Ma, L., Alva, A., Dou, Z., et al. (2013). Phosphorus in China’s intensive vegetable production systems: overfertilization, soil enrichment, and environmental implications. J. Environ. Qual. 42, 982–989. doi: 10.2134/jeq2012.0463
Zhang, Q., Song, Y., Wu, Z., Yan, X., Gunina, A., Kuzyakov, Y., et al. (2020). Effects of six-year biochar amendment on soil aggregation, crop growth, and nitrogen and phosphorus use efficiencies in a rice-wheat rotation. J. Clean. Prod. 242:118435. doi: 10.1016/j.jclepro.2019.118435
Zheng, B. X., Ding, K., Yang, X. R., Wadaan, M. A. M., Hozzein, W. N., Penuelas, J., et al. (2019). Straw biochar increases the abundance of inorganic phosphate solubilizing bacterial community for better rape (Brassica napus) growth and phosphate uptake. Sci. Total Environ. 647, 1113–1120. doi: 10.1016/j.scitotenv.2018.07.454
Keywords: root morphological and exudation traits, root–microbe interaction, fungi, bacteria, phosphate-solubilizing microorganisms, crop P-use efficiency
Citation: Zhang D, Zhang Y, Zhao Z, Xu S, Cai S, Zhu H, Rengel Z and Kuzyakov Y (2022) Carbon–Phosphorus Coupling Governs Microbial Effects on Nutrient Acquisition Strategies by Four Crops. Front. Plant Sci. 13:924154. doi: 10.3389/fpls.2022.924154
Received: 20 April 2022; Accepted: 07 June 2022;
Published: 05 July 2022.
Edited by:
Nicola Tomasi, University of Udine, ItalyReviewed by:
Lucia Cavalca, University of Milan, ItalyThangavelu Muthukumar, Bharathiar University, India
Copyright © 2022 Zhang, Zhang, Zhao, Xu, Cai, Zhu, Rengel and Kuzyakov. This is an open-access article distributed under the terms of the Creative Commons Attribution License (CC BY). The use, distribution or reproduction in other forums is permitted, provided the original author(s) and the copyright owner(s) are credited and that the original publication in this journal is cited, in accordance with accepted academic practice. No use, distribution or reproduction is permitted which does not comply with these terms.
*Correspondence: Haitao Zhu, aGFpdGFvemh1QDE2My5jb20=