- State Key Laboratory of Hybrid Rice, College of Life Sciences, Wuhan University, Wuhan, China
The ELO family is involved in synthesizing very-long-chain fatty acids (VLCFAs) and VLCFAs play a crucial role in plant development, protein transport, and disease resistance, but the physiological function of the plant ELO family is largely unknown. Further, while nitric oxide synthase (NOS)-like activity acts in various plant environmental responses by modulating nitric oxide (NO) accumulation, how the NOS-like activity is regulated in such different stress responses remains misty. Here, we report that the yeast mutant Δelo3 is defective in H2O2-triggered cell apoptosis with decreased NOS-like activity and NO accumulation, while its Arabidopsis homologous gene ELO2 (ELO HOMOLOG 2) could complement such defects in Δelo3. The expression of this gene is enhanced and required in plant osmotic stress response because the T-DNA insertion mutant elo2 is more sensitive to the stress than wild-type plants, and ELO2 expression could rescue the sensitivity phenotype of elo2. In addition, osmotic stress-promoted NOS-like activity and NO accumulation are significantly repressed in elo2, while exogenous application of NO donors can rescue this sensitivity of elo2 in terms of germination rate, fresh weight, chlorophyll content, and ion leakage. Furthermore, stress-responsive gene expression, proline accumulation, and catalase activity are also repressed in elo2 compared with the wild type under osmotic stress. In conclusion, our study identifies ELO2 as a pivotal factor involved in plant osmotic stress response and reveals its role in regulating NOS-like activity and NO accumulation.
Introduction
Water availability, as an important environment factor, is tightly associated with plants' growth and survival (Wang et al., 2021). Drought, high salinity, and low temperature cause osmotic stress by limiting water availability to plants, and severely affect agricultural production through the suppression of germination, flowering, or senescence (Lozano-Juste et al., 2020; Zhang et al., 2020a; Fu et al., 2021; Yuan et al., 2021). According to recent data, about 90% of arable land worldwide is suffering from different kinds of abiotic stresses, and up to 70% of plants are facing the risk of yield decrease as a result of these environmental stresses (Fancy et al., 2017). Thus, novel insights into plant osmotic stress responses are in growing demand when we are facing current challenges in agriculture and food production.
As sessile growing organisms, plants have evolved diverse strategies to survive the threats to their existence caused by osmotic stress in continually changing circumstances, including morphological adaptations by inhibiting the growth of shoots and accelerating the development of roots, as well as adjusting the transport of ions like the elevation of cytosolic Ca2+ and metabolites such as the accumulation of abscisic acid (ABA) in cellular level responses, gene expression, and so on (Zhu et al., 1997; Xiong and Zhu, 2002; Fujita et al., 2011; Zhao et al., 2016). Besides, plants also release signaling molecules to initiate stress response as one of the protection strategies. Nitric oxide (NO), an important concentration-dependent gaseous compound, is reported as a vitally important molecule in many biological and physiological systems in living organisms (Liu et al., 2015; Zhao et al., 2015; Begara-Morales et al., 2018; Wong et al., 2020; Cui et al., 2021). Since 1992, when NO was named “Molecular of the Year” by the journal Science, there has been a plethora of research on the profound effects of NO on plant physiological processes such as germination, floral transition, and environmental responses (Guo et al., 2003; He et al., 2004; Shen et al., 2013; Zhao et al., 2015; Zhang et al., 2018a; Cui et al., 2021). For example, drought could promote NO production in maize, wheat, and barley, and exogenous application of NO donor SNP (sodium nitroprusside) could enhance drought tolerance by reducing water stress and inducing stomatal closure, while suppressing NO accumulation severely reducing plant drought stress tolerance, illustrating that NO is important to plant drought stress response (Gan et al., 2015; Majeed et al., 2020). NO is also promoted to a high level in salt-stressed plants (Uchida et al., 2002; Liu et al., 2015; Li et al., 2018). While decreasing NO in wild-type plants by L-NAME (Nω-nitro-L-arginine-methylester), a NO synthase (NOS) inhibitor, and in the noa1 mutant by mutating Nitric Oxide Associated 1 displays more sensitivity to high salinity, overexpressing rat neuronal NOS to increase NO accumulation notably enhances plant salt stress tolerance (Zhao et al., 2007; Shi et al., 2012a; Xie et al., 2013; Cai et al., 2015). As for osmotic stress, the supplement of SNP could alleviate over-accumulated ROS (reactive oxygen species)-caused oxidative damage as well as decrease the inhibition of root growth, chlorophyll content, and proline accumulation in osmotic stressed noa1 plants (Zhang et al., 2010). However, reducing NO accumulation by application of L-NAME leads to enhanced osmotic stress (Xing et al., 2004; Cao et al., 2019; Mohd Amnan et al., 2021). These findings show that NO can be induced when plants are subjected to various types of environmental stresses. In addition, the demonstration of the involvement of NOS-mediated NO synthesis in plant abiotic and biotic stress responses represents the growing attention attached to the roles of NOS-like activity in plant stress responses (Romero-Puertas et al., 2004; Tossi et al., 2009; Zhang et al., 2010; Kong et al., 2012; Cai et al., 2015). Nevertheless, little is known about how NOS-like activity is modulated in plants. NO synthesis in these plant stress responses is still waiting for further exploration (Foresi et al., 2010; Santolini et al., 2017; Astier et al., 2018). In mammals, three main NOS isoforms converting L-arginine to L-citrulline and NO have been well-described with different localizations and functions (Mayer and Hemmens, 1997; Wendehenne et al., 2001; Stuehr and Haque, 2019). However, the gene(s) involved in coding for NOS protein has yet to be identified in higher plants as well as yeast. Recently, two plant factors, Sorting Nexin 1 and WD40-REPEAT 5a, have been shown to affect NOS-like activity in plant responses to salt and heavy metal stresses (Li et al., 2018; Zhang et al., 2020b). But, whether and how NOS-like activity is regulated in plant osmotic stress response is still unknown.
VLCFAs, as hydrocarbon chains containing more than 20 carbon atoms, are the precursors of different kinds of lipids such as phospholipids and sphingolipids, and function in various physiological processes (Bach et al., 2011; Wang et al., 2018; Zhukov, 2018; Kim et al., 2021). Elo1, Elo2, and Elo3 are three fatty acid elongases in yeast. While Elo1 participates in the elongation of LCFAs (long-chain fatty acids), Elo2 and Elo3 take part in the elongation of LCFAs to VLCFAs (Toke and Martin, 1996; Oh et al., 1997). The loss of function in ELO1 (Δelo1) does not result in differences in fatty acid composition, but the double mutant Δelo2 Δelo3 is not viable in yeast, indicating the essential roles of these elongase-mediated VLCFAs in cell growth. In addition, Elo2 and Elo3 have crucial roles in yeast lipotoxicity, heat stress, and salt stress responses (Tvrdik et al., 2000; Randez-Gil et al., 2020; Zhu et al., 2020). In mammals, seven fatty acid elongase genes (ELOVL1-7) have been characterized and reported to be involved in several diseases such as ichthyosis, stargardt syndrome, and hepatic steatosis (Kihara, 2012, 2016; Agbaga, 2016; Nie et al., 2021; Tanno et al., 2021). In plants, four ELOs (ELO1-4) in Arabidopsis, homologs of yeast Elo2, have been biochemically identified for VLCFA synthesis (Nagano et al., 2019), but their physiological roles have not been elucidated.
In this study, we find that Elo3 is a putative mediator of NOS by screening yeast deletion mutants with changed NOS-like activity under H2O2 treatment. We also show that Arabidopsis ELO2 (ELO HOMOLOG 2), as the homolog of yeast Elo3, functions in the modulation of osmotic stress-promoted NO accumulation via plant NOS-like activity. While T-DNA insertion mutant elo2 shows decreased osmotic stress tolerance than the wild type, and ELO2 expression could rescue this sensitivity phenotype of the mutant. Furthermore, elo2 exhibits increased H2O2 accumulation and repressed stress-responsive gene expression compared with the wild type under osmotic stress. Taken together, ELO2, as a newly discovered factor, functions in modulating NO accumulation through NOS-like activity in osmotically stressed plants.
Materials and Methods
Strains and H2O2 Treatment
The yeast Saccharomyces cerevisiae strains used in this article including wild type strain BY4741 (MATα, his3Δ1, leu2Δ0, metΔ0, and ura3Δ0) and deletion mutant strain Δelo3 (YLR372W::kanMX4) were purchased from UROSCARF (Frankfurt, Germany). The yeast was cultured in YPD medium containing glucose (2%, m/v), yeast extract (1%, m/v), and peptone (2%, m/v), pH 5.8. The cells were treated with 4 mM H2O2 for 30 min at 28°C for H2O2 treatment.
Plants, Growth Conditions, and Germination Rate Analysis
The Arabidopsis thaliana line elo2 (SALK_080633C, Col-0) was obtained from Arashare (https://www.arashare.cn/index/) and confirmed by PCR. The sterilized and washed seeds were placed at 4°C for 3 d. For germination rate analysis, about 100 seeds were planted on 1/2 MS (Murashige and Skoog) medium supplemented with 0, 200, 250, and 300 mM mannitol at 23°C and 100 mol m−2 s−1 illumination under 16 h light/8 h dark conditions for 5 days, and those that penetrated the seed coat were regarded as germinated seeds as described (Zhao et al., 2020). The primers are listed in Supplementary Table 1.
Plasmid Construction and Transformation
For yeast pGAL::ELO2 Δelo3 lines, the full-length ELO2 (AT3G06470) CDS was amplified and cloned into the pYES260 vector, then pYES260-AtELO2 was introduced into yeast mutant Δelo3. For Arabidopsis ELO2::ELO2 elo2 lines, the 2.6-kb genomic sequence of ELO2, including 1 kb upstream of the start codon, was amplified and cloned into the pCambia 1300 vector. The construct was transformed into an elo2 mutant using the floral-dip method (Clough and Bent, 1998). The primers are listed in Supplementary Table 1.
Detection of NO and NOS-Like Activity
NO content was detected with the NO-specific fluorescent probe DAF-FM DA (Beyotime, Haimen, China) as in our previous report (Shi et al., 2012b; Cai et al., 2015). NOS-like activity was assayed using a NOS assay kit based on DAF-FM DA (Beyotime, Haimen, China). To detect the NOS-like activity of plants, seedlings were frozen and ground with liquid nitrogen and resuspended in 1 ml of prepared extraction buffer (50 mM Tris–HCl, pH 7.4, 1 mM EDTA, 1 mM dithiothreitol, 1 mM leupeptin, 1 mM pepstatin, and 1 mM phenylmethylsulfonyl fluoride). After centrifuging at 12,000 g for 15 min at 4°C, the supernatant was used as the enzyme extract NOS-like activity was analyzed using the NOS assay kit mentioned before.
Determination of L-Arginine Content in Plants
L-arginine was extracted with cooled trichloroacetic acid (5%, w/v) and analyzed based on the method described in the previous report (Shi et al., 2013).
RT-qPCR Analysis
RNA extraction, first-strand cDNA synthesis, and RT-qPCR were carried out according to our previous report (Fu et al., 2021). ACTIN2 (AT3G18780) and UBQ10 (AT4G05320) were used as internal controls. The primers are listed in Supplementary Table 1.
Total Chlorophyll Content Assays
Total chlorophyll was extracted with 80% acetone (v/v). The absorbance at light wavelengths of 603, 645, and 663 nm was measured and total chlorophyll content was calculated with the formula as previously reported (Zhang et al., 2020a).
Measurement of Proline, MDA, and Ion Leakage Rate
The proline content was determined as reported (Zhang et al., 2020a). In short, the proline was extracted in sulfosalicylic acids (3%, m/v), then added to acetic acid and ninhydrin mixture (1:1). After boiling for 30 min, the absorbance of the supernatant was measured at 520 nm. The MDA was assessed as reported (Cai et al., 2015). The analysis of the ion leakage rate was performed based on the amounts of electrolytes released from plants before and after boiling in the de-ionized water. The seedlings were placed in de-ionized water and shaken for 30 min, the electrolyte leakage 1 (I1) was measured. After a water bath at 100°C for 30 min, then shaking for another 30 min, electrolyte leakage 2 (I2) was obtained. The ion leakage rate was calculated as I1/I2 × 100%.
DAB Staining and Detection of Catalase Activity
The solution used for DAB staining was freshly prepared with 1 mg/ml DAB and 0.1% Tween 20 in 10 mM Na2HPO4. Five-d-old plants were incubated in the solution for 8 h. To eliminate the chlorophyll, 70% ethanol was used to rinse the plants. The intensity of DAB staining was measured using Photoshop CS5 (Adobe). Catalase activity was assessed as described (Wang et al., 2021).
Results
Yeast Elo3 and Arabidopsis ELO2 Conservatively Act on H2O2-Induced NO Accumulation by Regulating NOS-Like Activity in Yeast
NO synthesis plays a crucial role in living organisms' adaptation to adverse conditions. However, the factor(s) modulating NOS-like activity to change NO levels in plants and yeast remain largely unknown. It is reported that H2O2 can induce NO synthesis and cause cell apoptosis in yeast, and this process is associated with the changes in NOS-like activity (Almeida et al., 2007). Thus, we searched for the player(s) regulating NOS-like activity in yeast, first, by treating the yeast deletion mutants with 4 mM H2O2 and assaying cell apoptosis, NO accumulation and NOS-like activity. Under the treatment of H2O2, Δelo3 exhibited significantly repressed cell apoptosis compared with the wild type (Supplementary Figure 1A), suggesting that H2O2-promoted NO accumulation was defective in the mutant yeast cell. Indeed, the NO accumulation of Δelo3 was much lower than that in the wild type using the staining assay of NO-specific fluorescence by DAF-FM DA when subjected to H2O2 treatment (Supplementary Figures 1B,C). Consistently, the mutant of ELO3 in yeast resulted in the repression of H2O2-induced NOS-like activity (Supplementary Figure 1D).
Then, we found that Arabidopsis ELO2 (ELO HOMOLOG 2) shares a 29.13% identity with yeast Elo3 (Supplementary Figure 2) and speculated that ELO2 may have a conservative role with yeast Elo3 in regulating NO accumulation. Thus, we constructed the plasmid pYES260-ELO2 to drive ELO2 expression under the control of the galactose-induced yeast GAL1 promoter, transformed it into Δelo3, and measured cell apoptosis, NO accumulation, and NOS-like activity. Our results demonstrated that upon H2O2 treatment, the expression of ELO2 in pGAL1::ELO2 Δelo3 induced by galactose completely rescued the cell apoptosis as well as NO content in Δelo3 (Supplementary Figures 3A–C). In addition, the NOS-like activity was also restored under the treatment of H2O2 by the induced expression of ELO2 in pGAL1::ELO2 Δelo3 via galactose (Supplementary Figure 3D), demonstrating that Arabidopsis ELO2 and yeast Elo3 conservatively function in H2O2-induced NO accumulation.
Arabidopsis ELO2 Acts in the Plant Osmotic Stress Response
Arabidopsis ELO2 belongs to the ELO family, which functions in VLCFAs' elongation (Nagano et al., 2019), but its physiological function is misty. To explore the participation of ELO2 in plant stress response, we obtained an ELO2 T-DNA insertion mutant SALK_080633 with dramatically reduced ELO2 expression and named it elo2 (Supplementary Figure 4). We then examined the sensitivity of elo2 to osmotic stress by adding different concentrations of mannitol in a 1/2 MS medium. While both elo2 and the wild type had comparable growth phenotypes in terms of seed germination, chlorophyll content, fresh weight, and ion leakage rate under normal growth conditions (Figures 1A–E), osmotic stress severely inhibited the seed germination of elo2 in comparison to the wild type when grown under different concentrations of mannitol (Figures 1A,B). After growing for 5 days on a medium containing 250 mM mannitol, over 80% of wild-type seeds but only 28% of elo2 germinated (Figures 1A,B). In addition, elo2 exhibited reduced fresh weight, lower chlorophyll content, and a higher ion leakage rate compared with wild type (Figures 1C–E). To confirm that such osmotic stress sensitivity of elo2 was due to decreased ELO2 gene expression, we generated the ELO2::ELO2 elo2 transgenic complement lines (Supplementary Figure 4C) and assayed its sensitivity to osmotic stress. Our results showed that ELO2::ELO2 elo2 displayed comparable phenotypes to wild-type plants based on our assays of seed germination, chlorophyll content, fresh weight, and ion leakage rate under both normal and stress conditions, respectively (Figures 1A–E). We also analyzed ELO2 expression by RT-qPCR and found that the transcription of ELO2 was up-regulated by osmotic stress (Figure 1F). Collectively, these results demonstrate that ELO2 is required in the plant's response to osmotic stress.
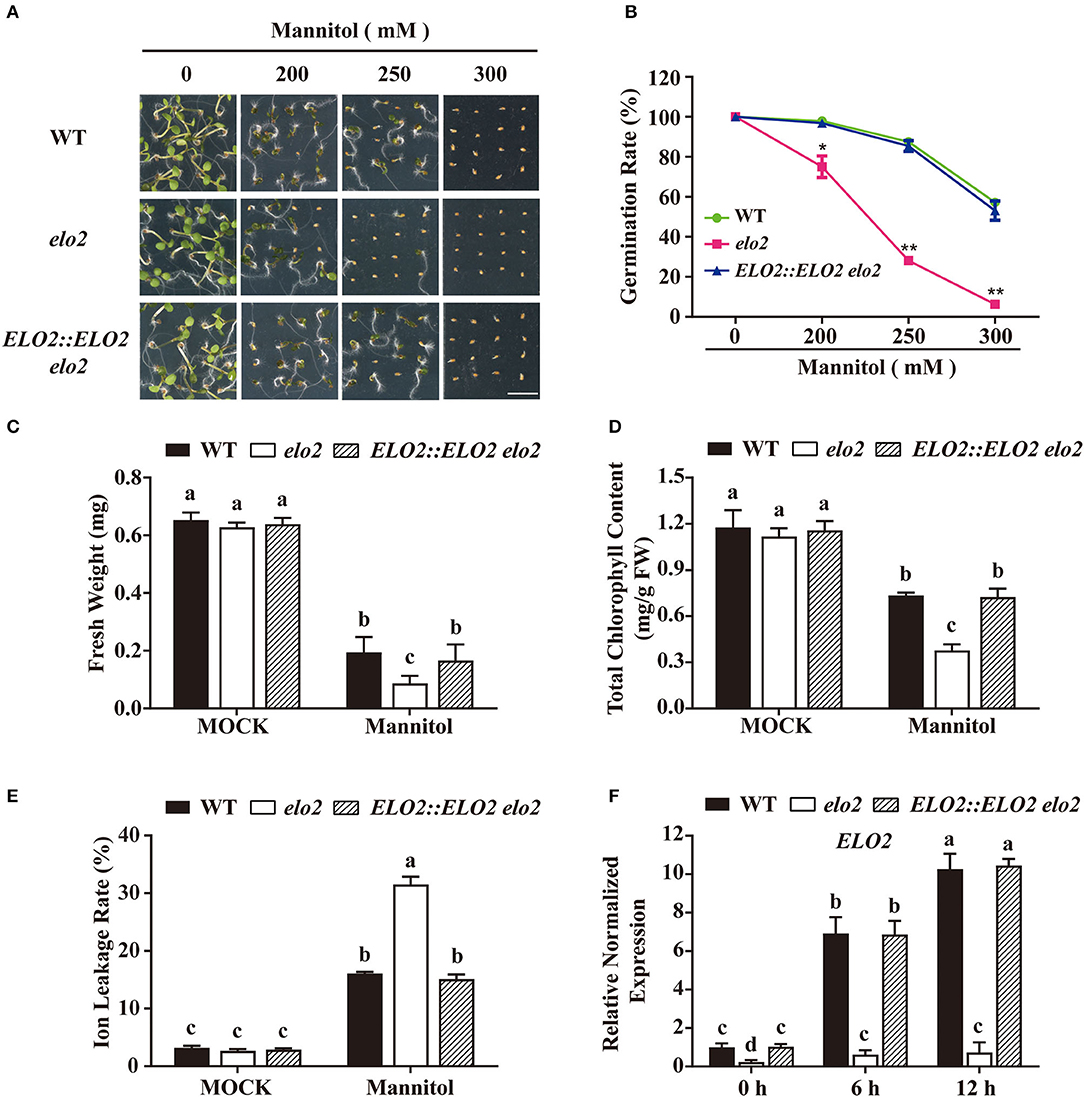
Figure 1. Arabidopsis ELO2 acts in plant osmotic stress response. (A,B) Phenotypes (A) and germination rate (B) of WT, elo2, and ELO2::ELO2 elo2 plants under the treatment of 200, 250, or 300 mM mannitol for 5 days Scale bars = 0.5 cm. (C) Fresh weight of WT, elo2, and ELO2::ELO2 elo2 plants under the treatment of 250 mM mannitol for 5 days. (D,E) Total chlorophyll content (D) and ion leakage rate (E) of 5-day-old WT, elo2, and ELO2::ELO2 elo2 seedlings under the treatment of 250 mM mannitol for 12 h. FW: fresh weight. (F) Relative normalized expression of ELO2 in 5-day-old WT, elo2, and ELO2::ELO2 elo2 seedlings under the treatment of 250 mM mannitol for 0, 6, and 12 h. The data obtained for ELO2::ELO2 elo2 #1 are shown. Data shown are means ± SD of three independent biological replicates. Asterisks indicate significant differences from the wild type (Student's t-test): *P < 0.05; **P < 0.01. Different letters indicate significantly different values (P < 0.05 by Tukey's test).
ELO2 Affects NOS-Like Activity to Modulate Osmotic Stress-Induced NO Accumulation
As per our above data, galactose-induced expression of Arabidopsis ELO2 in yeast Δelo3 could rescue its reduced NOS-like activity under H2O2 treatment (Supplementary Figure 3D). Whether ELO2 also participates in plant osmotic stress response by regulating NO levels needs further exploration. Therefore, first, we detected NO content in mannitol-treated and untreated the wild type and elo2 plants, we found that osmotic stress promoted NO content in wild-type seedlings as reported (Cao et al., 2019), but this promotion was significantly repressed in elo2 (Figures 2A,B). Then, we analyzed whether the decreased NO content in elo2 resulted from the change of NOS-like activity or the lack of its substrate by examining NOS-like activity and the content of L-arginine in wild type and elo2. It is shown in our results that there was no noticeable difference in L-arginine content between wild-type and elo2 plants treated with mannitol (Figure 2C). However, the osmotic stress-promoted NOS-like activity in the wild type was largely repressed in elo2 (Figure 2D).
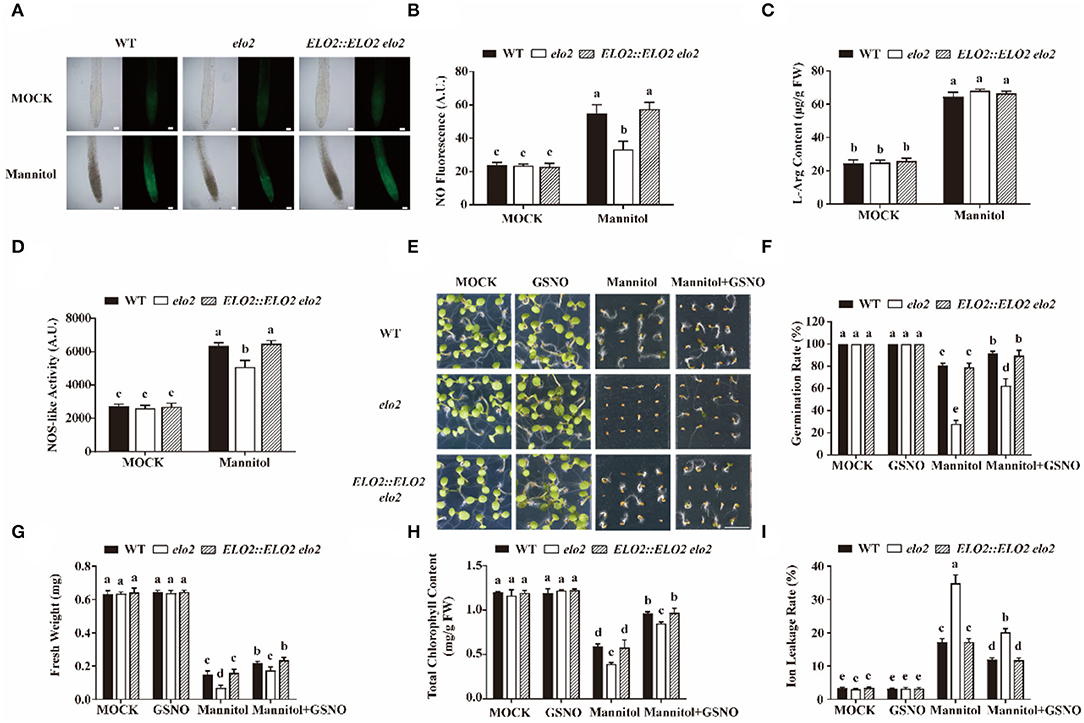
Figure 2. ELO2 affects NOS-like activity to modulate osmotic stress-induced NO accumulation. (A,B) Representative images (A) and fluorescence (B) of DAF-FM DA staining in 5-day-old WT, elo2, and ELO2::ELO2 elo2 plants under the treatment of 250 mM mannitol for 12 h. Scale bars = 200 μm. (C,D) L-Arg content (C) and NOS-like activity (D) of 5-day-old WT, elo2, and ELO2::ELO2 elo2 seedlings under the treatment of 250 mM mannitol for 12 h. FW: fresh weight. (E–G) Phenotypes (E), germination rate (F), and fresh weight (G) of wild-type, elo2, and ELO2::ELO2 elo2 plants under the treatment of 50 μM GSNO, 250 mM mannitol, and 250 mM mannitol plus 50 μM GSNO for 5 days Scale bars = 0.5 cm. (H,I) Total chlorophyll content (H) and ion leakage rate (I) of 5-day-old WT, elo2, and ELO2::ELO2 elo2 seedlings treated with or without 50 μM GSNO, 250 mM mannitol, and 250 mM mannitol plus 50 μM GSNO for 12 h. FW: fresh weight. A.U. indicates the pixel intensity arbitrary units of DAF-FM DA fluorescence. Data shown are means ± SD of three independent biological replicates. Different letters indicate significantly different values (P < 0.05 by Tukey's test).
Based on our above results, we speculated that the sensitivity of elo2 to osmotic stress was due to decreased NOS-like activity and thus reduced NO content. If this was the case, it is expected that an exogenously applied NO donor could rescue this stress sensitivity of elo2. Therefore, we assayed the seed germination of mannitol-treated wild type and elo2 with the application of GSNO or SNP, two widely-used NO donors (Cai et al., 2015), and we found that GSNO could decrease the sensitivity of elo2 to osmotic stress in terms of the enhanced seed germination of elo2 from 28 to 62% compared with wide-type plants from 82 to 91% (Figures 2E,F). Likewise, fresh weight, chlorophyll content, and ion leakage of elo2 were also partially restored by the addition of GSNO compared with untreated control (Figures 2G–I). Consistently, the application of exogenous SNP brought the similar results (Figure 3). To further confirm our speculation, we also conducted the experiments with the treatments of NOS inhibitor L-NAME and NO scavenger 2-(4-carboxyphenyl)-4,4,5,5-tetramethylimidazoline-1-oxyl-3-oxide (cPTIO) under osmotic stress and observed the germination phenotypes of treated plants. Our results showed that both L-NAME and cPTIO can further inhibit the germination of mannitol-treated elo2 mutant and the wild type, but the further inhibition was alleviated in elo2 from about 28 to 25% compared with the wild type from 80 to 58% because the NO content is lower in elo2 than the wild type (Supplementary Figure 5). Taken together, our results reveal that Arabidopsis ELO2 participates in osmotic stress response by modulating NOS-like activity to change NO content.
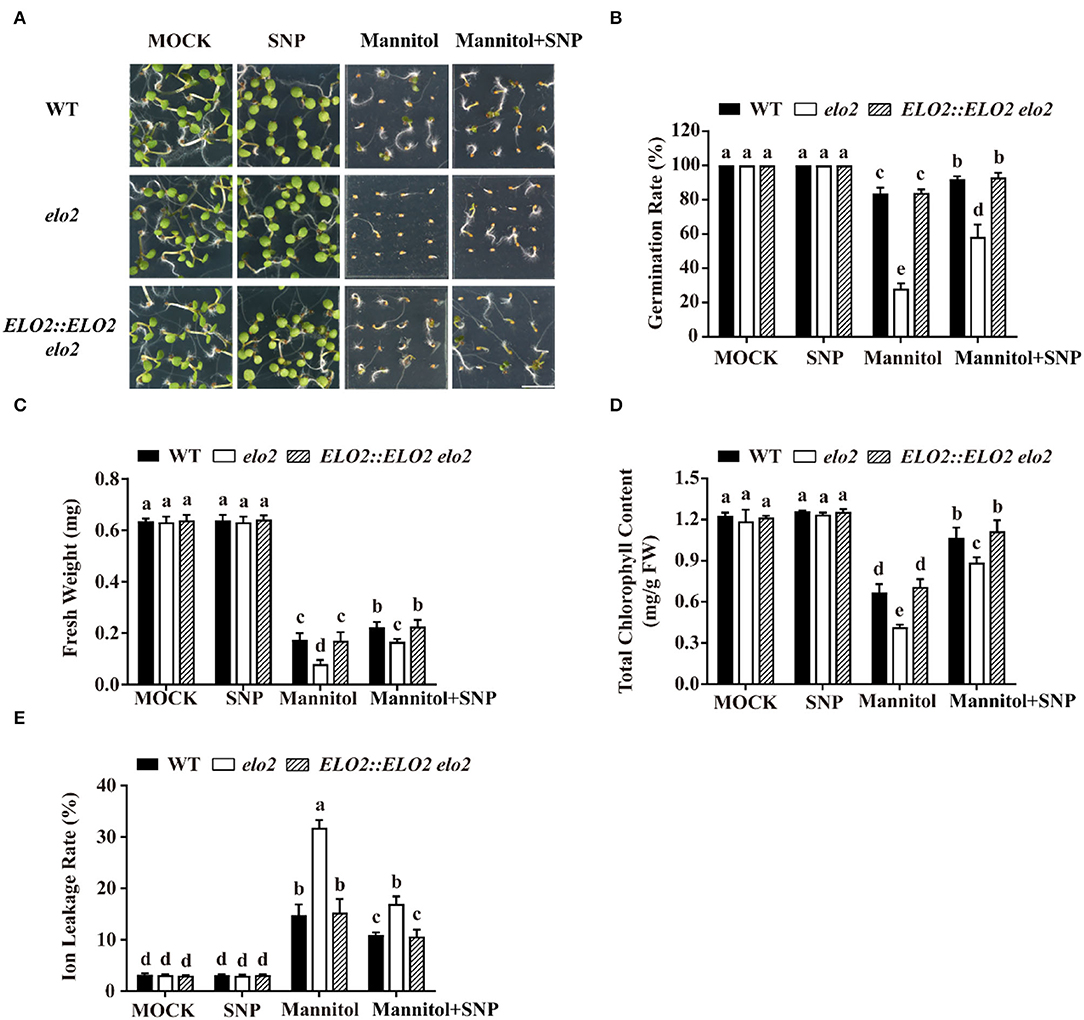
Figure 3. SNP alleviates the sensitivity of elo2 to osmotic stress. (A–C) Phenotypes (A), germination rate (B), and fresh weight (C) of WT, elo2, and ELO2::ELO2 elo2 plants under the treatment of 5 μM SNP, 250 mM mannitol, and 250 mM mannitol plus 5 μM SNP for 5 days. (D,E) Total chlorophyll content (D) and ion leakage rate (E) of 5-day-old WT, elo2, and ELO2::ELO2 elo2 seedlings treated with or without 5 μM SNP, 250 mM mannitol, and 250 mM mannitol plus 5 μM SNP for 12 h. FW: fresh weight. Scale bars = 0.5 cm. Data shown are means ± SD of three independent biological replicates. Different letters indicate significantly different values (P < 0.05 by Tukey's test).
ELO2 Is Involved in the Expression of Stress-Responsive Genes and Proline Synthesis in Plant Osmotic Stress Response
It has been reported in our previous study that expressing rat neuronal NOS to promote NO synthesis in rice enhanced abiotic stress tolerance by elevating stress-responsive gene transcription and proline accumulation (Cai et al., 2015). The low NO content in elo2 may repress the transcription of stress-responsive genes and the proline accumulation for its decreased tolerance to osmotic stress. Thus, we assessed the transcript levels of some stress-responsive genes in mannitol treated or untreated elo2 seedlings, and the data showed that the expression of COR15A, COR47, KIN2, and RD22 in wild-type plants was significantly elevated under osmotic stress, but this promotion was repressed in mannitol-treated elo2 seedlings (Figures 4A–D). We also detected the proline content in wild-type and elo2 and found that proline accumulation was severely repressed in stressed elo2 compared with the stressed wild type (Figure 4E), prompting us to further explore whether the reduced proline content was caused by the decreased expression of proline biosynthetic genes. Indeed, the transcript levels of P5CR, P5CS1, and P5CS2, three important genes that function in proline biosynthesis, were much lower in elo2 than in the wild type when treated with mannitol (Figures 4F–H). These data suggest that ELO2 participates in plant osmotic stress response by altering the transcription of stress-responsive genes and proline biosynthetic genes.
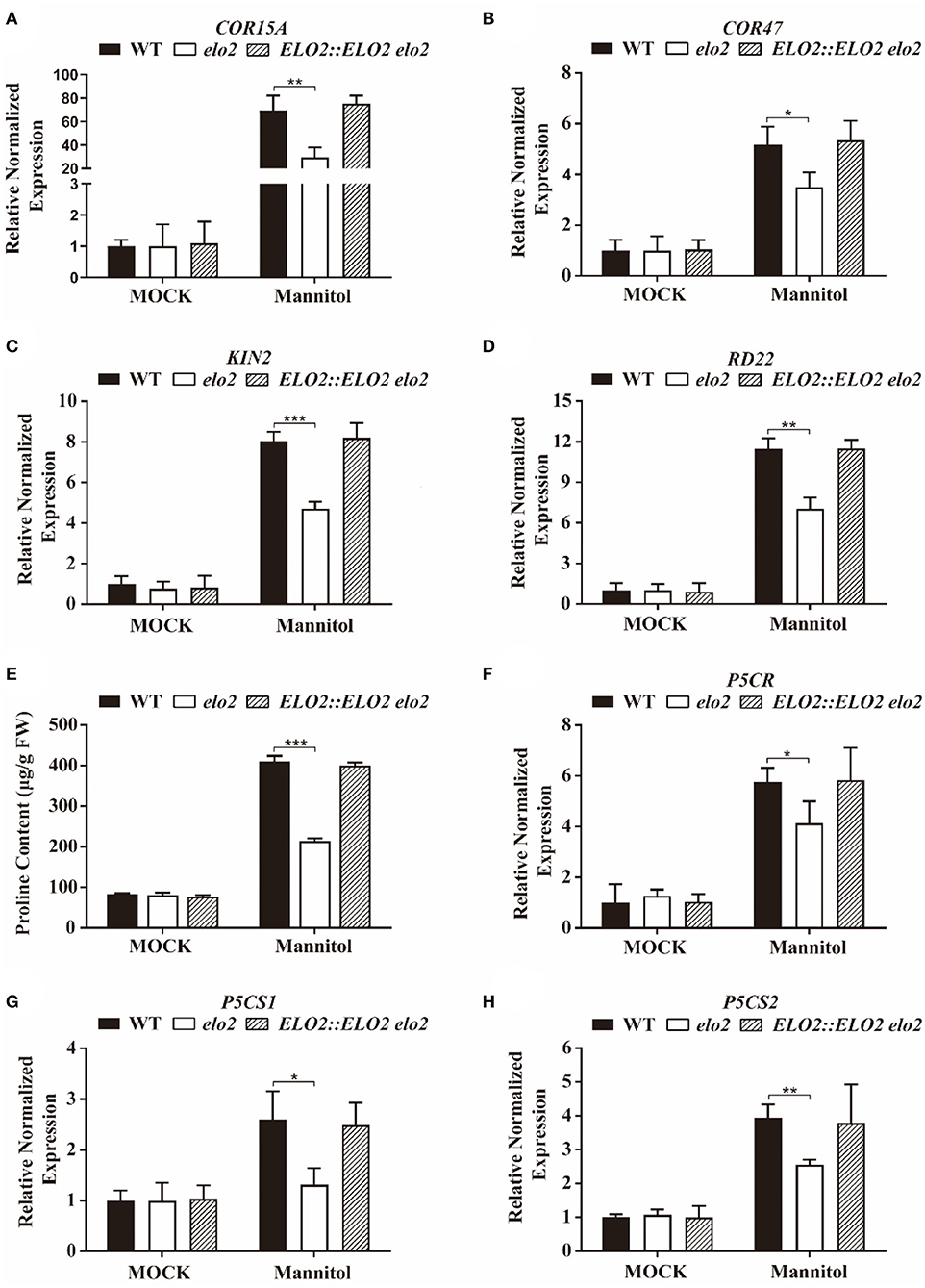
Figure 4. ELO2 is involved in the expression of stress-responsive genes and proline synthesis in plant osmotic stress response. (A–D) Relative normalized expression of COR15A (A), COR47 (B), KIN2 (C), and RD22 (D) in 5-day-old WT, elo2, and ELO2::ELO2 elo2 seedlings under 250 mM mannitol treatment for 12 h or not. (E–H) Proline content (E) and relative normalized expression of proline biosynthetic genes, P5CR (F), P5CS1 (G), P5CS2 (H) in mannitol-treated and untreated elo2, and ELO2::ELO2 elo2. Data shown are means ± SD of three independent biological replicates. Asterisks indicate significant differences from the osmotic stressed wild-type plant (Student's t-test): *P < 0.05; **P < 0.01; ***P < 0.001.
ELO2 Modulates Catalase Activity and Thus H2O2 Accumulation in Plant Osmotic Stress Response
It is reported that higher mannitol causes osmotic stress and hurts plant cells partially by enhancing ROS accumulation (Zhang et al., 2021). Thus, we also assayed whether elo2 with decreased NO accumulation has higher ROS accumulation under the treatment of mannitol using 3,3-diaminobenzidine (DAB) staining. The data revealed that both wild-type and elo2 plants had increased H2O2 accumulation after the treatment of mannitol (Figures 5A,B), however, the H2O2 content in the elo2 was much higher than that in the wide-type challenged with osmotic stress (Figures 5A,B). Consistently, MDA content, as the indicator for lipid peroxidation, was also higher in elo2 than the wild type stressed with mannitol (Figure 5C).
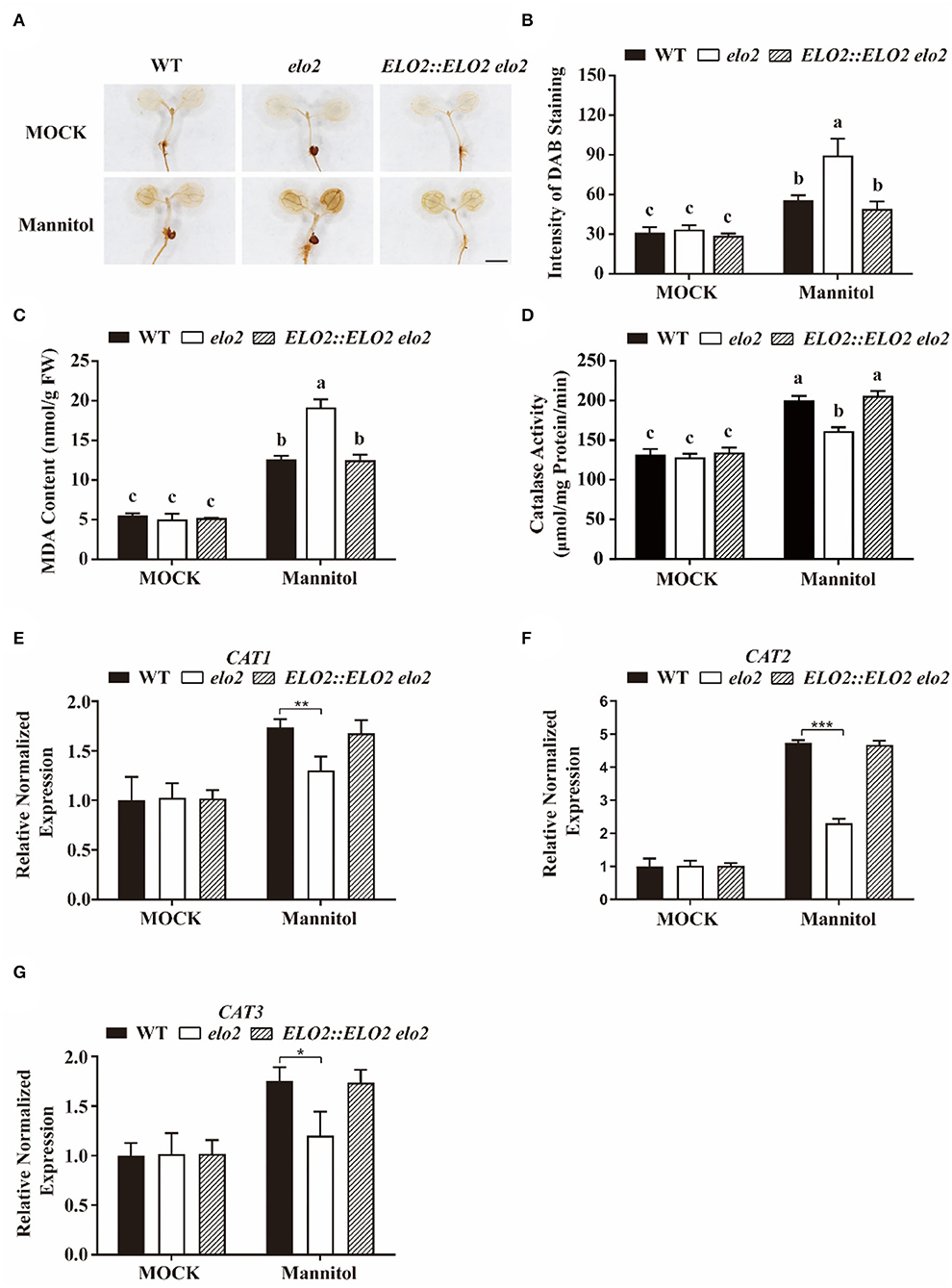
Figure 5. ELO2 modulates catalase activity and thus H2O2 accumulation in plant osmotic stress response. (A,B) Representative images (A) and intensity of DAB staining (B–G) MDA content (C), catalase activity (D), and relative normalized expression of CAT1 (E), CAT2 (F), CAT3 (G) in 5-day-old WT, elo2, and ELO2::ELO2 elo2 seedlings under the treatment of 250 mM mannitol for 12 h or not. Scale bars = 1 mm. Data shown are means ± SD of three independent biological replicates. Different letters indicate significantly different values (P < 0.05 by Tukey's test). Asterisks indicate significant differences from the osmotic stressed wild-type plant (Student's t-test): *P < 0.05; **P < 0.01; ***P < 0.001.
Catalase (CAT) is the key H2O2-scavenging enzyme that contributes to maintaining ROS homeostasis by catalyzing the degradation of H2O2 in plant cells. To figure out the relationship between over-accumulated H2O2 and CAT in elo2, we first determined catalase activity and found that the catalase activity in elo2 was significantly inhibited compared with wild-type plants under the treatment of mannitol (Figure 5D). Then, the expression of genes encoding CATs (CAT1, CAT2, and CAT3) was measured. Consistent with repressed catalase activity in elo2, the transcription of CATs was largely compromised in mannitol-treated elo2 (Figures 5E–G). These results indicate that ELO2 affects catalase activity and thus H2O2 accumulation in plant osmotic stress response.
Discussion
Over the past 20 years, NO has been known as a crucial molecule in plants. Since the 1990s, there have emerged numerous studies with regard to NO-mediated biotic/abiotic stress response (Shi et al., 2012a; Liu et al., 2015; Castillo et al., 2018; Khan et al., 2019; Hasanuzzaman et al., 2021; Jedelska et al., 2021). Although many advances in comprehending the functions of NO have been made, there still exist a large number of mysteries that need further exploration, among them, the most attractive one revolves around NO synthesis in plants.
In mammals, NO is generated dominantly by NOSs with L-arginine as substrate (Mayer and Hemmens, 1997; Wendehenne et al., 2001; Stuehr and Haque, 2019). However, in plants, nitrite reduction has been identified as the most explicit synthesis route because the gene(s) coding for NOS in higher plants have yet to be discovered, although a NOS from green algae, Ostreococcus tauri, was found to be similar to human NOSs (Foresi et al., 2010). Further, while the role of NOS-dependent NO synthesis has been implied in diverse plant stress responses, the mediators of NOS activity are still waiting for further exploration (Cai et al., 2015; Liu et al., 2015; Li et al., 2018).
To find out the factors implicated in regulating the NOS activity in plants, we screened the yeast mutants and found that H2O2-induced yeast cell apoptosis was significantly repressed in Δelo3. Its homologous gene ELO2 in Arabidopsis could complement such defects in Δelo3 (Supplementary Figure 2). It's reported that NO synthesis participates in various stress responses (Shi et al., 2012a; Liu et al., 2015), we showed that osmotic stress-promoted NOS-like activity and NO accumulation in the wild type are significantly repressed in elo2. Furthermore, treatment of NO donors with GSNO/SNP can rescue this sensitivity of the mutant. In brief, ELO2 could serve as the mediator of NOS-like in plants to regulate NO content under osmotic stress.
However, the mechanism by which ELO2 modulates NOS-like activity remains mysterious. Recently, WD40-REPEAT 5a and Sorting Nexin 1 have been reported to regulate NOS-like activity in heavy metal and salt-stressed plants, respectively with unknown mechanisms (Li et al., 2018; Zhang et al., 2020b). We speculate that ELO2 may impact NOS-like activity by directly interacting with NOS, which hasn't been identified yet in higher plants. Thus, searching for ELO2 interacting proteins containing cofactor-binding sites for flavin adenine dinucleotide (FAD), flavin mononucleotide (FMN), 6R-tetra-hydrobiopterin (BH4), and calmodulin (CaM) as well as L-arginine and NADPH-binding sites, like NOSs in mammals, could be an interesting direction (Zemojtel et al., 2006; Santolini et al., 2017; Astier et al., 2018; Hancock and Neill, 2019; Stuehr and Haque, 2019). Alternatively, ELO2 may modulate NOS activity indirectly by interacting with NOS regulator(s).
Proline is associated with plants' viability under stress conditions (Xiong and Zhu, 2002; Zhao et al., 2016; Wu et al., 2020). Many reports indicate the participation of NO in proline accumulation by exogenously increasing or decreasing NO content under abiotic stresses (Shi et al., 2007; Arasimowicz-Jelonek et al., 2009; Naser Alavi et al., 2014). It is shown in our results that proline content and proline biosynthetic genes were prominently prompted by osmotic stress in the wild type, but the induction was inhibited in elo2 seedlings (Figure 4), revealing that ELO2 participates in regulating the proline biosynthetic gene expression and thus proline content in plant osmotic stress response.
NO is also thought to play a role in changes in oxidative compound accumulation in plants (Xiong et al., 2010; Shivaraj et al., 2020; Mohd Amnan et al., 2021). For instance, exogenous SNP alleviates stress-caused damage to plants along with a decrease in H2O2 (Shi et al., 2012a; Liu et al., 2015; Zhang et al., 2018b; Mohd Amnan et al., 2021). Our previous report also indicated that the rat neuronal NOS-overexpressing rice with higher NO accumulated less H2O2 under stress conditions (Cai et al., 2015). Our results showed that when challenged with osmotic stress, elo2 with decreased NO accumulation has higher H2O2, possibly by repressing CAT expression and thus decreasing catalase activity (Figure 5).
Moreover, it is well-known that the ELO family is involved in synthesizing VLCFAs, which are essential for plant growth and disease resistance (Nagano et al., 2019; Batsale et al., 2021). However, the physiological function of the ELO family in plants is rarely known. Here, we report that ELO2, a member of the ELO family, functions in plant osmotic stress response.
Taken together, our study identifies ELO2 as a novel factor involved in plant osmotic stress response by modulating NOS-like activity and thus NO accumulation (Figure 6).
Data Availability Statement
The raw data supporting the conclusions of this article will be made available by the authors, without undue reservation.
Author Contributions
S-QZ and Y-TL conceived and designed the project and analyzed the data and wrote the manuscript. S-QZ and Z-WF performed the experiments. All authors contributed to the article and approved the submitted version.
Funding
This work was supported by the National Natural Science Foundation of China (31830007) to Y-TL.
Conflict of Interest
The authors declare that the research was conducted in the absence of any commercial or financial relationships that could be construed as a potential conflict of interest.
Publisher's Note
All claims expressed in this article are solely those of the authors and do not necessarily represent those of their affiliated organizations, or those of the publisher, the editors and the reviewers. Any product that may be evaluated in this article, or claim that may be made by its manufacturer, is not guaranteed or endorsed by the publisher.
Acknowledgments
The authors sincerely thank AraShare for providing seeds.
Supplementary Material
The Supplementary Material for this article can be found online at: https://www.frontiersin.org/articles/10.3389/fpls.2022.924064/full#supplementary-material
References
Agbaga, M. P. (2016). Different mutations in ELOVL4 affect very long chain fatty acid biosynthesis to cause variable neurological disorders in humans. Adv. Exp. Med. Biol. 854, 129–135. doi: 10.1007/978-3-319-17121-0_18
Almeida, B., Buttner, S., Ohlmeier, S., Silva, A., Mesquita, A., Sampaio-Marques, B., et al. (2007). NO-mediated apoptosis in yeast. J. Cell. Sci. 120(Pt 18), 3279–3288. doi: 10.1242/jcs.010926
Arasimowicz-Jelonek, M., Floryszak-Wieczorek, J., and Kubi,ś, J. (2009). Involvement of nitric oxide in water stress-induced responses of cucumber roots. Plant Sci. 177, 682–690. doi: 10.1016/j.plantsci.2009.09.007
Astier, J., Gross, I., and Durner, J. (2018). Nitric oxide production in plants: an update. J. Exp. Bot. 69, 3401–3411. doi: 10.1093/jxb/erx420
Bach, L., Gissot, L., Marion, J., Tellier, F., Moreau, P., Satiat-Jeunemaitre, B., et al. (2011). Very-long-chain fatty acids are required for cell plate formation during cytokinesis in Arabidopsis thaliana. J. Cell Sci. 124(Pt 19), 3223–3234. doi: 10.1242/jcs.074575
Batsale, M., Bahammou, D., Fouillen, L., Mongrand, S., Joubes, J., and Domergue, F. (2021). Biosynthesis and functions of very-long-chain fatty acids in the responses of plants to abiotic and biotic stresses. Cells 10, 1284. doi: 10.3390/cells10061284
Begara-Morales, J. C., Chaki, M., Valderrama, R., Sanchez-Calvo, B., Mata-Perez, C., Padilla, M. N., et al. (2018). Nitric oxide buffering and conditional nitric oxide release in stress response. J. Exp. Bot. 69, 3425–3438. doi: 10.1093/jxb/ery072
Cai, W., Liu, W., Wang, W. S., Fu, Z. W., Han, T. T., and Lu, Y. T. (2015). Overexpression of rat neurons nitric oxide synthase in rice enhances drought and salt tolerance. PLoS ONE 10, e0131599. doi: 10.1371/journal.pone.0131599
Cao, X., Zhu, C., Zhong, C., Zhang, J., Wu, L., Jin, Q., et al. (2019). Nitric oxide synthase-mediated early nitric oxide burst alleviates water stress-induced oxidative damage in ammonium-supplied rice roots. BMC Plant Biol. 19, 108. doi: 10.1186/s12870-019-1721-2
Castillo, M. C., Coego, A., Costa-Broseta, A., and Leon, J. (2018). Nitric oxide responses in Arabidopsis hypocotyls are mediated by diverse phytohormone pathways. J. Exp. Bot. 69, 5265–5278. doi: 10.1093/jxb/ery286
Clough, S. J., and Bent, A. F. (1998). Floral dip: a simplified method for Agrobacterium-mediated transformation of Arabidopsis thaliana. Plant J. 16, 735–743. doi: 10.1046/j.1365-313x.1998.00343.x
Cui, B., Xu, S., Li, Y., Umbreen, S., Frederickson, D., Yuan, B., et al. (2021). The Arabidopsis zinc finger proteins SRG2 and SRG3 are positive regulators of plant immunity and are differentially regulated by nitric oxide. New Phytol. 230, 259–274. doi: 10.1111/nph.16993
Fancy, N. N., Bahlmann, A.-K., and Loake, G. (2017). Nitric oxide function in plant abiotic stress. Plant Cell Environ. 40, 462–472. doi: 10.1111/pce.12707
Foresi, N., Correa-Aragunde, N., Parisi, G., Calo, G., Salerno, G., and Lamattina, L. (2010). Characterization of a nitric oxide synthase from the plant kingdom: NO generation from the green alga Ostreococcus tauri is light irradiance and growth phase dependent. Plant Cell 22, 3816–3830. doi: 10.1105/tpc.109.073510
Fu, Z. W., Li, J. H., Feng, Y. R., Yuan, X., and Lu, Y. T. (2021). The metabolite methylglyoxal-mediated gene expression is associated with histone methylglyoxalation. Nucleic Acids Res. 49, 1886–1899. doi: 10.1093/nar/gkab014
Fujita, Y., Fujita, M., Shinozaki, K., and Yamaguchi-Shinozaki, K. (2011). ABA-mediated transcriptional regulation in response to osmotic stress in plants. J. Plant Res. 124, 509–525. doi: 10.1007/s10265-011-0412-3
Gan, L., Wu, X., and Zhong, Y. (2015). Exogenously applied nitric oxide enhances the drought tolerance in hulless barley. Plant Prod. Sci. 18, 52–56. doi: 10.1626/pps.18.52
Guo, F.-Q., Okamoto, M., and Crawford, N. M. (2003). Identification of a plant nitric oxide synthase gene involved in hormonal signaling. Science 302, 100–103. doi: 10.1126/science.1086770
Hancock, J. T., and Neill, S. J. (2019). Nitric oxide: its generation and interactions with other reactive signaling compounds. Plants (Basel) 8, 41. doi: 10.3390/plants8020041
Hasanuzzaman, M., Inafuku, M., Nahar, K., Fujita, M., and Oku, H. (2021). Nitric oxide regulates plant growth, physiology, antioxidant defense, and ion homeostasis to confer salt tolerance in the mangrove species, kandelia obovata. Antioxidants (Basel) 10, 611. doi: 10.3390/antiox10040611
He, Y., Tang, R. H., Hao, Y., Stevens, R. D., Cook, C. W., Ahn, S. M., et al. (2004). Nitric oxide represses the Arabidopsis floral transition. Science 305, 1968–1971. doi: 10.1126/science.1098837
Jedelska, T., Luhova, L., and Petrivalsky, M. (2021). Nitric oxide signalling in plant interactions with pathogenic fungi and oomycetes. J. Exp. Bot. 72, 848–863. doi: 10.1093/jxb/eraa596
Khan, M., Imran, Q. M., Shahid, M., Mun, B. G., Lee, S. U., Khan, M. A., et al. (2019). Nitric oxide- induced AtAO3 differentially regulates plant defense and drought tolerance in Arabidopsis thaliana. BMC Plant Biol. 19, 602. doi: 10.1186/s12870-019-2210-3
Kihara, A. (2012). Very long-chain fatty acids: elongation, physiology and related disorders. J. Biochem. 152, 387–395. doi: 10.1093/jb/mvs105
Kihara, A. (2016). Synthesis and degradation pathways, functions, and pathology of ceramides and epidermal acylceramides. Prog. Lipid Res. 63, 50–69. doi: 10.1016/j.plipres.2016.04.001
Kim, J., Kim, R. J., Lee, S. B., and Chung Suh, M. (2021). Protein-protein interactions in fatty acid elongase complexes are important for very-long-chain fatty acid synthesis. J. Exp. Bot. 73, 3004–3017. doi: 10.1093/jxb/erab543
Kong, W., Huang, C., Chen, Q., Zou, Y., and Zhang, J. (2012). Nitric oxide alleviates heat stress-induced oxidative damage in Pleurotus eryngii var. tuoliensis. Fungal Genet. Biol. 49, 15–20. doi: 10.1016/j.fgb.2011.12.003
Li, T. T., Liu, W. C., Wang, F. F., Ma, Q. B., Lu, Y. T., and Yuan, T. T. (2018). SORTING NEXIN 1 functions in plant salt stress tolerance through changes of NO accumulation by regulating NO synthase-like activity. Front. Plant Sci. 9, 1634. doi: 10.3389/fpls.2018.01634
Liu, W., Li, R. J., Han, T. T., Cai, W., Fu, Z. W., and Lu, Y. T. (2015). Salt stress reduces root meristem size by nitric oxide-mediated modulation of auxin accumulation and signaling in Arabidopsis. Plant Physiol. 168, 343–356. doi: 10.1104/pp.15.00030
Lozano-Juste, J., Alrefaei, A. F., and Rodriguez, P. L. (2020). Plant osmotic stress signaling: MAPKKKs meet SnRK2s. Trends Plant Sci. 25, 1179–1182. doi: 10.1016/j.tplants.2020.09.003
Majeed, S., Nawaz, F., Naeem, M., Ashraf, M. Y., Ejaz, S., Ahmad, K. S., et al. (2020). Nitric oxide regulates water status and associated enzymatic pathways to inhibit nutrients imbalance in maize (Zea mays L.) under drought stress. Plant Physiol. Biochem. 155, 147–160. doi: 10.1016/j.plaphy.2020.07.005
Mayer, B., and Hemmens, B. (1997). Biosynthesis and action of nitric oxide in mammalian cells. Trends Biochem. Sci. 22, 477–481. doi: 10.1016/S0968-0004(97)01147-X
Mohd Amnan, M. A., Pua, T. L., Lau, S. E., Tan, B. C., Yamaguchi, H., Hitachi, K., et al. (2021). Osmotic stress in banana is relieved by exogenous nitric oxide. PeerJ. 9, e10879. doi: 10.7717/peerj.10879
Nagano, M., Kakuta, C., Fukao, Y., Fujiwara, M., Uchimiya, H., and Kawai-Yamada, M. (2019). Arabidopsis bax inhibitor-1 interacts with enzymes related to very-long-chain fatty acid synthesis. J. Plant Res. 132, 131–143. doi: 10.1007/s10265-018-01081-8
Naser Alavi, S. M., Arvin, M. J., and Manoochehri Kalantari, K. (2014). Salicylic acid and nitric oxide alleviate osmotic stress in wheat (Triticum aestivum L.) seedlings. J. Plant Interact. 9, 683–688. doi: 10.1080/17429145.2014.900120
Nie, L., Pascoa, T. C., Pike, A. C. W., Bushell, S. R., Quigley, A., Ruda, G. F., et al. (2021). The structural basis of fatty acid elongation by the ELOVL elongases. Nat. Struct. Mol. Biol. 28, 512–520. doi: 10.1038/s41594-021-00605-6
Oh, C. S., Toke, D. A., Mandala, S., and Martin, C. E. (1997). ELO2 and ELO3, homologues of the Saccharomyces cerevisiae ELO1 gene, function in fatty acid elongation and are required for sphingolipid formation. J. Biol. Chem. 272, 17376–17384. doi: 10.1074/jbc.272.28.17376
Randez-Gil, F., Prieto, J. A., Rodriguez-Puchades, A., Casas, J., Sentandreu, V., and Estruch, F. (2020). Myriocin-induced adaptive laboratory evolution of an industrial strain of Saccharomyces cerevisiae reveals its potential to remodel lipid composition and heat tolerance. Microb. Biotechnol. 13, 1066–1081. doi: 10.1111/1751-7915.13555
Romero-Puertas, M. C., Perazzolli, M., Zago, E. D., and Delledonne, M. (2004). Nitric oxide signalling functions in plant-pathogen interactions. Cell. Microbiol. 6, 795–803. doi: 10.1111/j.1462-5822.2004.00428.x
Santolini, J., Andre, F., Jeandroz, S., and Wendehenne, D. (2017). Nitric oxide synthase in plants: where do we stand? Nitric Oxide 63, 30–38. doi: 10.1016/j.niox.2016.09.005
Shen, Q., Wang, Y. T., Tian, H., and Guo, F. Q. (2013). Nitric oxide mediates cytokinin functions in cell proliferation and meristem maintenance in Arabidopsis. Mol. Plant 6, 1214–1225. doi: 10.1093/mp/sss148
Shi, H., Ye, T., Chen, F., Cheng, Z., Wang, Y., Yang, P., et al. (2013). Manipulation of arginase expression modulates abiotic stress tolerance in Arabidopsis: effect on arginine metabolism and ROS accumulation. J. Exp. Bot. 64, 1367–1379. doi: 10.1093/jxb/ers400
Shi, H. T., Li, R. J., Cai, W., Liu, W., Fu, Z. W., and Lu, Y. T. (2012a). In vivo role of nitric oxide in plant response to abiotic and biotic stress. Plant Signal. Behav. 7, 437–439. doi: 10.4161/psb.19219
Shi, H. T., Li, R. J., Cai, W., Liu, W., Wang, C. L., and Lu, Y. T. (2012b). Increasing nitric oxide content in Arabidopsis thaliana by expressing rat neuronal nitric oxide synthase resulted in enhanced stress tolerance. Plant Cell Physiol. 53, 344–357. doi: 10.1093/pcp/pcr181
Shi, Q., Ding, F., Wang, X., and Wei, M. (2007). Exogenous nitric oxide protect cucumber roots against oxidative stress induced by salt stress. Plant Physiol. Biochem. 45, 542–550. doi: 10.1016/j.plaphy.2007.05.005
Shivaraj, S. M., Vats, S., Bhat, J. A., Dhakte, P., Goyal, V., Khatri, P., et al. (2020). Nitric oxide and hydrogen sulfide crosstalk during heavy metal stress in plants. Physiol. Plant. 168, 437–455. doi: 10.1111/ppl.13028
Stuehr, D. J., and Haque, M. M. (2019). Nitric oxide synthase enzymology in the 20 years after the Nobel Prize. Br. J. Pharmacol. 176, 177–188. doi: 10.1111/bph.14533
Tanno, H., Sassa, T., Sawai, M., and Kihara, A. (2021). Production of branched-chain very-long-chain fatty acids by fatty acid elongases and their tissue distribution in mammals. Biochim. Biophys. Acta Mol. Cell Biol. Lipids 1866, 158842. doi: 10.1016/j.bbalip.2020.158842
Toke, D. A., and Martin, C. E. (1996). Isolation and characterization of a gene affecting fatty acid elongation in Saccharomyces cerevisiae. J. Biol. Chem. 271, 18413–18422. doi: 10.1074/jbc.271.31.18413
Tossi, V., Lamattina, L., and Cassia, R. (2009). An increase in the concentration of abscisic acid is critical for nitric oxide-mediated plant adaptive responses to UV-B irradiation. New Phytol. 181, 871–879. doi: 10.1111/j.1469-8137.2008.02722.x
Tvrdik, P., Westerberg, R., Silve, S., Asadi, A., Jakobsson, A., Cannon, B., et al. (2000). Role of a new mammalian gene family in the biosynthesis of very long chain fatty acids and sphingolipids. J. Cell Biol. 149, 707–718. doi: 10.1083/jcb.149.3.707
Uchida, A., Jagendorf, A. T., Hibino, T., Takabe, T., and Takabe, T. (2002). Effects of hydrogen peroxide and nitric oxide on both salt and heat stress tolerance in rice. Plant Sci. 163, 515–523. doi: 10.1016/S0168-9452(02)00159-0
Wang, L. F., Li, T. T., Zhang, Y., Guo, J. X., Lu, K. K., and Liu, W. C. (2021). CAND2/PMTR1 is required for melatonin-conferred osmotic stress tolerance in Arabidopsis. Int. J. Mol. Sci. 22, 4014. doi: 10.3390/ijms22084014
Wang, Q., Du, X., Ma, K., Shi, P., Liu, W., Sun, J., et al. (2018). A critical role for very long-chain fatty acid elongases in oleic acid-mediated Saccharomyces cerevisiae cytotoxicity. Microbiol. Res. 207, 1–7. doi: 10.1016/j.micres.2017.11.001
Wendehenne, D., Pugin, A., Klessig, D. F., and Durner, J. (2001). Nitric oxide: comparative synthesis and signaling in animal and plant cells. Trends Plant Sci. 6, 177–183. doi: 10.1016/S1360-1385(01)01893-3
Wong, A., Donaldson, L., Portes, M. T., Eppinger, J., Feijo, J. A., and Gehring, C. (2020). Arabidopsis DIACYLGLYCEROL KINASE4 is involved in nitric oxide-dependent pollen tube guidance and fertilization. Development 147, dev183715. doi: 10.1242/dev.183715
Wu, J., Zhang, N., Liu, Z., Liu, S., Liu, C., Lin, J., et al. (2020). The AtGSTU7 gene influences glutathione-dependent seed germination under ABA and osmotic stress in Arabidopsis. Biochem. Biophys. Res. Commun. 528, 538–544. doi: 10.1016/j.bbrc.2020.05.153
Xie, Y., Mao, Y., Lai, D., Zhang, W., Zheng, T., and Shen, W. (2013). Roles of NIA/NR/NOA1-dependent nitric oxide production and HY1 expression in the modulation of Arabidopsis salt tolerance. J. Exp. Bot. 64, 3045–3060. doi: 10.1093/jxb/ert149
Xing, H., Tan, L., An, L., Zhao, Z., Wang, S., and Zhang, C. (2004). Evidence for the involvement of nitric oxide and reactive oxygen species in osmotic stress tolerance of wheat seedlings: inverse correlation between leaf abscisic acid accumulation and leaf water loss. Plant Growth Regul. 42, 61–68. doi: 10.1023/B:GROW.0000014894.48683.1b
Xiong, J., Fu, G., Tao, L., and Zhu, C. (2010). Roles of nitric oxide in alleviating heavy metal toxicity in plants. Arch. Biochem. Biophys. 497, 13–20. doi: 10.1016/j.abb.2010.02.014
Xiong, L., and Zhu, J. K. (2002). Molecular and genetic aspects of plant responses to osmotic stress. Plant Cell Environ. 25, 131–139. doi: 10.1046/j.1365-3040.2002.00782.x
Yuan, T. T., Xiang, Z. X., Li, W., Gao, X., and Lu, Y. T. (2021). Osmotic stress represses root growth by modulating the transcriptional regulation of PIN-FORMED3. New Phytol. 232, 1661–1673. doi: 10.1111/nph.17687
Zemojtel, T., Frohlich, A., Palmieri, M. C., Kolanczyk, M., Mikula, I., Wyrwicz, L. S., et al. (2006). Plant nitric oxide synthase: a never-ending story? Trends Plant Sci. 11, 524–525; author reply 526–528. doi: 10.1016/j.tplants.2006.09.008
Zhang, B., Wang, H., Wang, P., and Zhang, H. (2010). Involvement of nitric oxide synthase-dependent nitric oxide and exogenous nitric oxide in alleviating NaCl induced osmotic and oxidative stress in Arabidopsis thaliana. Afr. J. Agri. Res. 5, 1713–1721. doi: 10.5897/AJAR09.743
Zhang, L., Li, G., Wang, M., Di, D., Sun, L., Kronzucker, H. J., et al. (2018a). Excess iron stress reduces root tip zone growth through nitric oxide-mediated repression of potassium homeostasis in Arabidopsis. New Phytol. 219, 259–274. doi: 10.1111/nph.15157
Zhang, Q., Cai, W., Ji, T. T., Ye, L., Lu, Y. T., and Yuan, T. T. (2020a). WRKY13 Enhances cadmium tolerance by promoting D-CYSTEINE DESULFHYDRASE and hydrogen sulfide production. Plant Physiol. 183, 345–357. doi: 10.1104/pp.19.01504
Zhang, Y., Su, J., Cheng, D., Wang, R., Mei, Y., Hu, H., et al. (2018b). Nitric oxide contributes to methane-induced osmotic stress tolerance in mung bean. BMC Plant Biol. 18, 207. doi: 10.1186/s12870-018-1426-y
Zhang, Y., Tian, Y. Y., Wang, L. F., Li, Y. H., Li, T. T., and Liu, W. C. (2020b). WDR5a functions in cadmium-inhibited root meristem growth by regulating nitric oxide accumulation in Arabidopsis. Planta 252, 78. doi: 10.1007/s00425-020-03486-z
Zhang, Y., Wang, L. F., Li, T. T., and Liu, W. C. (2021). Mutual promotion of LAP2 and CAT2 synergistically regulates plant salt and osmotic stress tolerance. Front. Plant Sci. 12, 672672. doi: 10.3389/fpls.2021.672672
Zhao, H., Nie, K., Zhou, H., Yan, X., Zhan, Q., Zheng, Y., et al. (2020). ABI5 modulates seed germination via feedback regulation of the expression of the PYR/PYL/RCAR ABA receptor genes. New Phytol. 228, 596–608. doi: 10.1111/nph.16713
Zhao, M. G., Tian, Q. Y., and Zhang, W. H. (2007). Nitric oxide synthase-dependent nitric oxide production is associated with salt tolerance in Arabidopsis. Plant Physiol. 144, 206–217. doi: 10.1104/pp.107.096842
Zhao, X., Wang, J., Yuan, J., Wang, X. L., Zhao, Q. P., Kong, P. T., et al. (2015). NITRIC OXIDE-ASSOCIATED PROTEIN1 (AtNOA1) is essential for salicylic acid-induced root waving in Arabidopsis thaliana. New Phytol. 207, 211–224. doi: 10.1111/nph.13327
Zhao, Y., Chan, Z., Gao, J., Xing, L., Cao, M., Yu, C., et al. (2016). ABA receptor PYL9 promotes drought resistance and leaf senescence. Proc. Natl. Acad. Sci. U.S.A. 113, 1949–1954. doi: 10.1073/pnas.1522840113
Zhu, G., Yin, N., Luo, Q., Liu, J., Chen, X., Liu, L., et al. (2020). Enhancement of sphingolipid synthesis improves osmotic tolerance of Saccharomyces cerevisiae. Appl. Environ. Microbiol. 86, e02911–e02919. doi: 10.1128/AEM.02911-19
Zhu, J.-K., Hasegawa, P. M., Bressan, R. A., and Bohnert, H. J. (1997). Molecular aspects of osmotic stress in plants. Crit. Rev. Plant Sci. 16, 253–277. doi: 10.1080/07352689709701950
Keywords: osmotic stress, ELO2, NOS-like activity, NO accumulation, H2O2-hydrogen peroxide
Citation: Zheng S-Q, Fu Z-W and Lu Y-T (2022) ELO2 Participates in the Regulation of Osmotic Stress Response by Modulating Nitric Oxide Accumulation in Arabidopsis. Front. Plant Sci. 13:924064. doi: 10.3389/fpls.2022.924064
Received: 20 April 2022; Accepted: 06 June 2022;
Published: 13 July 2022.
Edited by:
Shuhua Zhu, Shandong Agricultural University, ChinaReviewed by:
Wenjun Hu, Zhejiang Academy of Agricultural Sciences, ChinaHaitao Shi, Hainan University, China
Copyright © 2022 Zheng, Fu and Lu. This is an open-access article distributed under the terms of the Creative Commons Attribution License (CC BY). The use, distribution or reproduction in other forums is permitted, provided the original author(s) and the copyright owner(s) are credited and that the original publication in this journal is cited, in accordance with accepted academic practice. No use, distribution or reproduction is permitted which does not comply with these terms.
*Correspondence: Ying-Tang Lu, eWluZ3RsdSYjeDAwMDQwO3dodS5lZHUuY24=