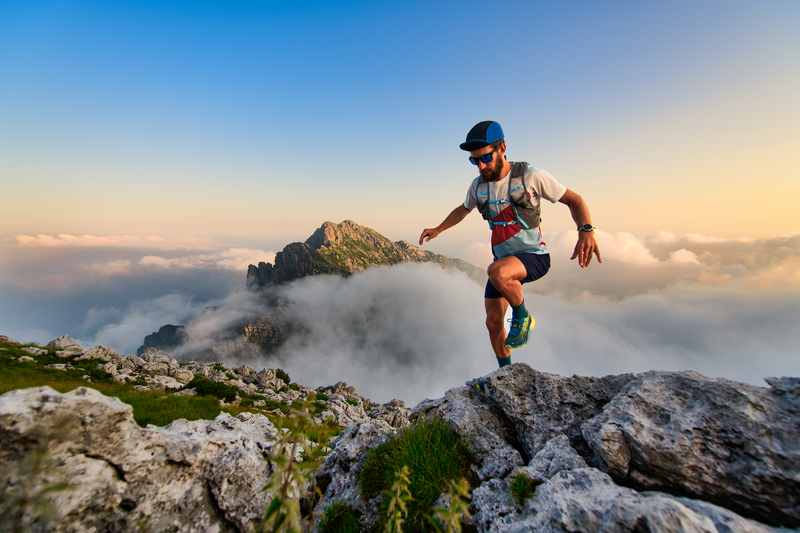
95% of researchers rate our articles as excellent or good
Learn more about the work of our research integrity team to safeguard the quality of each article we publish.
Find out more
ORIGINAL RESEARCH article
Front. Plant Sci. , 02 June 2022
Sec. Plant Abiotic Stress
Volume 13 - 2022 | https://doi.org/10.3389/fpls.2022.922564
This article is part of the Research Topic The Role of Stress Proteins in Plants Under Abiotic Stress View all 5 articles
SAPK/SnRK2 family genes play crucial roles in plant growth, development, and abiotic stress responses. The objective of this study was to identify and characterize the LpSAPK genes in perennial ryegrass (Lolium perenne L.). The results showed that there are 10 LpSAPKs in perennial ryegrass that could be classified into three groups with similar genic (exon–intron) structures to their orthologous genes in Arabidopsis and other grass species. Ka/Ks analysis suggested that the LpSAPKs and their orthologs were under purifying selection to maintain their conserved function during evolution. Nine out of ten LpSAPKs were localized in the cytoplasm and nucleus with the exception of LpSAPK5 which was only observed in the cytoplasm. Most LpSAPKs were responsive to various abiotic stress and hormonal (ABA, cytokinin, and ethylene) treatments but were downregulated in leaves and upregulated in roots, suggesting that there were unknown cis elements in promoters of these genes or unidentified post-transcriptional mechanism responsible for the tissue-dependent stress-regulated expression of these LpSAPKs. Furthermore, LpSAPK9 was identified as a candidate positive regulator in drought tolerance using a yeast ectopic expression system, and LpSAPK9 showed contrasting expression changes in drought-sensitive and -tolerant ryegrass varieties, suggesting that expression levels of LpSAPK9 were related to ryegrass drought tolerance. These results will facilitate further functional analysis of LpSAPKs for molecular breeding of ryegrass and other related grass species.
Protein phosphorylation and de-phosphorylation, catalyzed by kinases and phosphatases, comprise one important type of post-translational modification that plays in the regulation of plants’ adaptation to abiotic stresses (Couso et al., 2021). The stress-activated protein kinase (SAPK), also known as the SnRK2 (sucrose non-fermenting 1-related protein kinase 2 subfamily), is a plant-specific Ser/Thr protein kinase family that regulates plant growth, development, and abiotic stress responses via abscisic acid (ABA) dependent or independent signaling pathway (Pang et al., 2018; Chen et al., 2020b; Takahashi et al., 2020).
All SAPKs contain two domains: the N-terminal kinase domain and the C-terminal regulatory domain. The N-terminal kinase domain is conserved to the kinase domain of AMPK (AMP-activated protein kinase; Hrabak et al., 2003). The C-terminal domain consists of two subdomains, including Domain I and Domain II (Yoshida et al., 2006). The Domain I harbors about 30 amino acids, which exist in all SnRK2 members and are required for activation by osmotic stress; while the Domain II contains about 40 amino acids that mediate interaction with the clade A-type PP2Cs (2C Protein Phosphatases) for ABA signaling transduction (Kobayashi et al., 2004). Some SAPKs were identified as important regulators in plant tolerance to multi-environmental stresses. For example, in response to salt stress, AtSnRK2.4 and AtSnRK2.10 bind to phosphatidic acid (PA) through a 42 aa long domain, while the mutant proteins with the loss of PA interaction domains showed severe root inhibition under salt stress (Julkowska et al., 2015; Putta et al., 2020). Overexpression of AtSnRK2.8 and TaSnRK2.3/2.4/2.7 significantly enhanced drought stress tolerance in transgenic plants (Umezawa et al., 2004; Mao et al., 2010; Zhang et al., 2011; Tian et al., 2013). Several other SAPKs, such as ZmSAPK8, OsSAPK4, and PtSnRK2.5/2.7, act as positive regulators of multiple stress tolerances (Diédhiou et al., 2008; Ying et al., 2011; Song et al., 2016). Therefore, SAPKs are excellent candidate genes for stress tolerance improvement.
Perennial ryegrass (Lolium perenne L.) is an important cool-season perennial grass species widely cultivated in temperate regions throughout the world for its turf and forage purposes. It is important to further improve the drought stress tolerance of perennial ryegrass for better pasture and turf persistence where irrigation is often of major concern. The objectives of this study were (i) to classify and characterize SAPKs gene family in perennial ryegrass, and (ii) to identify SAPKs which can be used as candidate genes for perennial ryegrass stress tolerance.
Seeds of perennial ryegrass (cv. “Buena vista”) were sown in a cell tray filled with fritted clay and maintained in a greenhouse at Nanjing Agricultural University (Nanjing, China). 20 days old seedlings were transplanted and cultivated hydroponically for 2 weeks in Hoagland’s nutrient solution and maintained in a growth chamber set at a photoperiod of 14/10 h, the controlled temperature of 25/20°C (day/night), relative humidity of 70%, and photosynthetically active radiation (PAR) of 750 μmol photos m−2 s−1. The Hoagland’s nutrient solution was replaced with a fresh solution twice a week to ensure adequate oxygen and nutrient supply.
To determine the expression patterns of LpSAPKs involved in stress and hormone responses, plants were exposed to cold (4°C), heat (38°C), osmotic stress (15% PEG6000, w/v), NaCl (255 μm), AlCl3 (1.6 mm), CdCl2 (200 μm), 6-BA (25 μm), ABA (50 μm), and ethephon (200 μm, an ethylene releaser) treatments, respectively. Leaf and root samples were collected at 0, 0.5, 2, 6, 12, 24, & 48 h under each treatment, frozen in liquid nitrogen, and kept at −80°C for RNA extractions. Tissues including the root, crown, stem, leaf sheath, expanding leaf (the 2nd leaf from the top), mature leaf (the 3rd leaf from the top), and senescent leaf (the 4th leaf from the top) were collected from adult plants separately for RNA extractions and used for organ-specific expression analysis.
For drought tolerance assessment of perennial ryegrass lines, seeds of one commercial cultivar “Buena vista” and two selected lines bred in our research program (cv. “XiaLu-4” and “XiaLu-6,” previously named as “R2-4” and “R2-6,” respectively; Zhang et al., 2022a) were germinated on wetted paper towels with water and 10% PEG6000 in a growth chamber. 1 week later, the plant height, root length, and fresh weight of seedlings were measured, and the samples of leaf and root were collected to examine the expression level of LpSAPK9. Plant height was measured manually from the base to the shoot tip of each plant.
Coding sequence (CDS) of ten OsSAPKs in rice was obtained from NCBI1 based on the accession number reported by Zhang et al. (2018). All LpSAPKs gene sequences were identified using the local BLAST program to screen the perennial ryegrass genome database (Daniel et al., 2021) and our transcriptome database (Xu et al., 2019; Zhang et al., 2022b) with ten OsSAPKs sequences as quires. The E-value threshold for the BLAST program was set at 1e−10 to obtain the candidate dataset of SAPK genes. The local perennial ryegrass genome database and transcriptome databases were built using Bioedit software.2 Sequences of all LpSAPKs were examined using the Conserved Domain Database3 to confirm whether these proteins have typical features of the SAPKs. The biophysical properties of the LpSAPKs, such as Molecular weight (Mw), Isoelectric point (pI), and Grand average of hydropathy (GRAVY), were calculated using the ProtParam tool.4
Total mRNA was extracted from different tissues of perennial ryegrass using the Plant RNA Kit (Omega Bio-Tek, United States). The first-strand cDNA was synthesized using a cDNA Reverse Transcription Kit (TaKaRa, Dalian, China) and was used for gene cloning. All confirmed LpSAPK genes were cloned by using primers designed according to the sequences flanking their open reading frame (ORF; Supplementary Table S1). PCR was performed in a 50 μl reaction volume using Phanta Super-Fidelity DNA Polymerase from Vazyme (Vazyme Biotech Co., Ltd., Nanjing, China) for gene cloning. The PCR products were purified using the Gel Extraction Kit (Omega Bio-Tek, United States) and cloned into a modified pEND-linker vector for sequencing.
A phylogenetic tree was constructed using the neighbor-joining (NJ) method in the MEGA 7.0 software program with 1,000 bootstrap replicates, p-distance model/method, uniform rates among sites, partial deletion with 50% Cutoff gaps/missing data treatment, and the number of threads is seven. We have added this information in the revised MS. In addition to LpSAPKs from perennial ryegrass, their orthologous proteins (SAPK/SnRK2) from Arabidopsis (Arabidopsis thaliana), rice (Oryza sativa), maize (Zea mays), and brachypodium (Brachypodium distachyon) were used for the phylogenetic analysis. Multiple amino acid sequence alignment of LpSAPKs was performed using online Multiple Sequence Alignment tool.5 The gene structures of the LpSAPKs were determined based on the comparison of their coding sequences (CDS) and genomic sequences. The online MEME program (version 5.4.1)6 was used to identify the conserved motifs of the LpSAPK proteins. The Ka/Ks analysis was performed using TBtools (Chen et al., 2020a). Two kb nucleotide sequences upstream from the transcription initiation site were considered as promoters of each gene. The cis elements of LpSAPK promoters were obtained from the PLACE database.7
The CDS of the LpSAPKs were amplified with gene-specific primers (Supplementary Table S1) and cloned into the pEarleyGate103 vector to generate LpSAPK-GFP fusion genes and transformed into Agrobacterium tumefaciens strain “AGL1.” Then, the resuspended Agrobacterium at OD600 = 0.6 in 1/4 MS salt solution was injected into the leaves of 4 to 6-week-old Nicotiana benthamiana plants. The injected leaves were incubated in the dark for 12 h and then moved to a normal growth environment for 3 days, and then examined the GFP fluorescence signal using a Zeiss LSM 800 laser scanning confocal microscope (Carl Zeiss SAS, Jena, Germany). The subcellular localizations of LpSAPKs were also predicted by using the online PSORT software.8
For qRT-PCR analysis, the same procedure was used as reported previously (Zhang et al., 2016b, 2021). Detailed information on primers used for the qRT-PCR analysis was listed in Supplementary Table S1, and LpeIHF4A was selected as the reference gene (Huang et al., 2014). The relative expression levels of LpSAPK genes were calculated using the 2−ΔΔCT method.
Two Saccharomyces cerevisiae yeast mutant strains, △hog1 (Winkler et al., 2002) and △G19 (Quintero et al., 1996), that were sensitive to drought and salt treatments, were used to screen stress-related LpSAPKs. The CDS of the LpSAPKs were cloned into the pGAD426 vector and then transformed to the yeast strains using the Frozen-EZ Yeast Transformation Kit (Zymo Research, United States). The transformed yeast strains were grown in the synthetic dropout uracil (SD-Ura) or synthetic dropout uracil histidine (SD-Ura-His) medium. The pGAD426-GUS plasmid was used as the negative control. For drought tolerance assessment, 10 μl △hog1 yeast solution (OD600 = 1) was spotted on solidified SD-Ura medium containing 0.75 M sorbitol and incubated at 28°C for 3 days. The growth of transformants in SD-Ura liquid medium containing 0.75 M sorbitol was determined by measuring OD600 at 24 h intervals. The transformed yeast mutant strain△G19 was used for salt tolerance by assessing their growth in SD-Ura-His medium containing 500 mm NaCl.
Data in this study were statistically analyzed by the LSD and Duncan test program at a significance level of 0.05 using the SPSS software (Version 12, SPSS Inc., Chicago, IL). Data were expressed as means ± standard error (SE).
Ten LpSAPK genes were isolated from the perennial ryegrass genome and transcriptome databases and named as LpSAPK1-10 after corresponding to their orthologous proteins in rice. Subsequently, their CDSs were cloned and sequenced (GenBank accession numbers were listed in Supplementary Table S2). The CDS of the LpSAPKs vary from 1,017 bp (LpSAPK3) to 1,143 bp (LpSAPK9) and their encoded proteins range from 338 aa to 380 aa with molecular weights of 38.30 kDa (LpSAPK3) to 43.52 kDa (LpSAPK9). All LpSAPKs have isoelectric points (pI) less than 7.0, indicating they are acidic hydrophilic proteins (GRAVY <0) according to the GRAVY analysis (Supplementary Table S2).
Furthermore, all LpSAPKs have typical domains of the SAPK family, including the ATP-binding region (DI/LGXGXFGVA) and protein kinase-activating domain (I/VCHRDLKLENTLLD) in N-terminal regions that constitute the serine/threonine kinase domain, and the domain I and domain II in the C-terminal regions (Figure 1). It is interesting to note that domain I is present in all LpSAPKs, while domain II is specific to LpSAPK8 and LpSAPK10 (Figure 1).
Figure 1. Multiple sequence alignment of LpSAPKs. The protein kinase ATP-binding region signature and the serine/threonine-protein kinase active-site signature were indicated by a red box and a blue box, respectively. Domain I and Domain II at the C-terminus were marked by a green box and a yellow box, respectively.
To illustrate the evolutionary relationship among SAPK/SnRK2 homologs in different plants, a phylogenetic tree was constructed comprising 10 LpSAPKs in perennial ryegrass, 10 OsSAPKs in rice, 11 ZmSnRK2s in maize, 10 BdSAPKs in brachypodium, and 10 AtSnRK2s in Arabidopsis (Figure 2; Supplementary Table S3). The SAPKs were clustered into three subclasses, namely, groups 1, 2, and 3 (Figure 2). Group 1 comprised 5 LpSAPKs, including LpSAPK4/5/6/7/9; the group 2 comprised 3 ones, namely, LpSAPK1/2/3; while the rest two (LpSAPK8/10) were classified in the group 3.
Figure 2. Phylogenetic analysis of SAPK/SnRK2 proteins from Arabidopsis (AtSnRK2s), rice (OsSAPKs), and maize (ZmSnRK2s), brachypodium (BdSAPKs), and perennial ryegrass (LpSAPKs). The phylogenetic tree was constructed using the neighbor-joining (NJ) method with 1,000 bootstrap replicates in MEGA7.0. The Genebank accession numbers of LpSAPK, AtSnRK2, OsSAPK2, ZmSnRK2, and BdSAPK proteins are listed in Supplementary Tables S2, S3.
Most LpSAPKs exhibit similar exon–intron organizations that LpSAPK1/2/3/4/6/7/8/9 have 9 exons, LpSAPK10 has 8 exons, while LpSAPK5 only has 3 exons (Figure 3A). A total of 12 conserved motifs were identified by MEME analysis (Figure 3B). The motifs 1–5 and 7–9 were found in all LpSAPK proteins, while the motif 11 was specific to the group 3 LpSAPKs, and motifs 10 & 12 were specific to the group 1 LpSAPKs. Moreover, the identified motifs 1, 4, and 5 were the protein kinase-activating domain, the domain I, and the ATP-binding region, respectively.
Figure 3. Phylogenetic relationships, genic structures, and conserved motif composition of LpSAPKs. (A) Phylogenetic relationships and exon–intron organizations of the LpSAPKs. Exons and introns are shown by filled boxes and single lines, respectively. (B) Motif analysis of LpSAPKs. Each colored box represents a motif in the protein, with the motif name indicated at the bottom of the figure.
Since the phylogenetic classification and genic structures of LpSAPKs were similar to those in other plant species, we speculated that the LpSAPKs might be under purifying selection during evolution to maintain their conserved functions. To verify this hypothesis, we performed the Ka/Ks analysis using LpSAPKs and their close orthologous genes in rice and brachypodium. As shown in Table 1 all ten LpSAPKs and their compared orthologs had ka/ks ≦ 0.2, suggesting that all of these genes were under purifying selection.
Table 1. Purifying selection of LpSAPKs and their orthologous genes in rice (OsSAPKs) and brachypodium (BdSAPKs).
Using the PSORT software, we predicted that all LpSAPKs had relatively high affirmatives to the nucleus and cytosol (Supplementary Table S4). Consistently, we observed that the subcellular localization of LpSAPKs-GFP was in both the cytoplasm and the nucleus of leaf epidermal cells, while the only exception went for LpSAPK5 which was mainly detected in the cytoplasm of guard cells but not in the nucleus (Figure 4).
Figure 4. Subcellular localization of the LpSAPK proteins. The vector 35S::GFP was used as the control. All LpSAPKs were infusion with a GFP tag and visualized as green fluorescence in leaf cells of Nicotiana benthamiana.
Promoters of all LpSAPKs were amplified from perennial ryegrass and sequenced for cis elements analysis. As shown in Table 2, these LpSAPKs promoters have multiple cis elements involved in stress and hormone signaling pathways. For example, the ABRE (MACGYGB) and the CGTCA-motif were found in all LpSAPKs promoters. The ABRE cis element was the core DNA-binding sequence by ABI3 and ABF4 that were key transcription factors in the ABA signaling pathway. Similarly, the CGTCA-motif was one major cis-acting regulatory element involved in the MeJA (methyl jasmonate) pathway and was also identified in all LpSAPK promoters. The ethylene-responsive element (ERE) was found in the promoters of LpSAPK3/4/5/6/7/9. The gibberellin-responsive (GARE) was present in the promoters of LpSAPK1/2/7/9. The TGA element, involved in the auxin signaling pathway, was present in the promoters of LpSAPK5/6/7/8/9. The MYB binding site was found in the promoters of LpSAPK5/6/7/9. The TC-rich repeat, a defense and stress-responsive element, was found in the promoters of LpSAPK6/8/9. And the low-temperature responsive element (LTR) was identified in the promoters of LpSAPK5/7 (Table 2).
Expression patterns of 10 LpSAPKs were analyzed in seven tissues, including root, crown, stem, leaf sheath, expanding, mature and senescent leaves. As shown in Figure 5, five LpSAPKs (LpSAPK1/2/5/6/8) had similar expression patterns that were higher in leaves than in stem, crown, and root; expression levels of LpSAPK6 and LpSAPK7 were gradually and significantly increased along with leaf aging, while the contrary was true for LpSAPK9; and LpSAPK3&4 showed similar expression levels in all tested tissues.
Figure 5. Relative expression levels of LpSAPKs in different organs. Data are means ± SE (n = 4). Different letters above bars represent significant differences at p ≤ 0.05.
Then, relative expression levels of LpSAPKs were analyzed under six different abiotic stress conditions (PEG, NaCl, CdCl2, AlCl3, cold, and heat) at seven-time points. In general, these six stress treatments led to suppressed expression of eight LpSAPKs (except for LpSAPK5 and LpSAPK6) in leaves, and suppressed expression of LpSAPK1/2/3/9 but upregulated expression of LpSAPK4/5/7/8 in roots over two-fold changes (Figure 6). It is also notable that the transcriptional responses of these LpSAPKs to these stress treatments were more dramatic in roots than in leaves. For example, the expression levels of LpSAPK7/8 increased by 10- to 120-fold in roots but decreased by less than 20 folds in leaves in response to different abiotic stress (Figure 6).
Figure 6. Relative expression levels of LpSAPKs in leaves and roots under six different abiotic stresses. (A,B) osmotic stress with 15% PEG6000; (C,D) salt stress; (E–H) heavy mental stress (200 μm CdCl2 and 1.6 Mm AlCl3); (I,J) cold stress (4°C); (K,L) heat stress (38°C). Data are means ± SE (n = 4). *, **, and *** above the bars represents significant difference compared to the values at 0 h at p ≤ 0.05, 0.01, and 0.001, respectively.
We also analyzed the relative expression levels of LpSAPKs in response to ABA, cytokinin (6-BA), and ethephon (ETH) treatments. As shown in Figure 7, nine out of ten LpSAPKs (except LpSAPK5) were responsive to ABA treatment, among which eight were downregulated but the LpSAPK6 was upregulated in leaves. The contrary trend was observed in roots that nine LpSAPKs (except LpSAPK9) were upregulated by ABA treatments. In response to 6-BA treatment, expression levels of LpSAPK3/4/7/8/9 were downregulated in leaves, while nine LpSAPKs (except LpSAPK9) were upregulated by 6-BA in roots. Under ethephon treatment, nine LpSAPKs (except LpSAPK5) were downregulated in leaves; while in roots, LpSAPK1/2/6/9/10 were suppressed, and LpSAPK4/5/7/8 were upregulated (Figure 7).
Figure 7. Relative expression levels of LpSAPKs in leaves and roots under three hormone treatments. (A,B) ABA treatment; (C,D) cytokinin (6-BA) treatment; (E,F) ethephon treatment. Data are means ± SE (n = 4). *, **, and *** above the bars represent significant differences compared to 0 h at p ≤ 0.05, 0.01, and 0.001, respectively.
To screen potential LpSAPKs regulating drought and salt tolerance, we expressed each of these genes in two yeast mutant strains that were susceptible to drought or salt stress. As shown in Figure 8, the ∆hog1 cells harboring LpSAPK9 exhibited remarkably enhanced growth when compared with the GUS control on the SD-Ura agar medium with 0.75 M sorbitol (Figure 8A). Quantitatively, the LpSAPK9/∆hog1 lines grew at significantly higher rates than the GUS/∆hog1 control lines after 24, 48, and 72 h of incubation in SD-Ura liquid medium with 0.75 M sorbitol (Figure 8B). Yet, LpSAPK9/∆G19 lines grew at significantly slower rates than the GUS/∆G19 control lines when the medium was supplemented with 500 mm NaCl (Figures 8C,D). For the rest LpSAPK genes, no significant effect was observed on drought or salt tolerance of the yeast mutants.
Figure 8. Drought and salt tolerance assessment of yeast cells with heterologous overexpressed of LpSAPKs. (A,B) The growth of ∆hog1 yeast mutants transformed with the GUS gene (negative control) or with all the LpSAPKs on SD-Ura medium with or without sorbitol. (C,D) The growth of ∆G19 yeast mutants transformed with the GUS gene or with all the LpSAPKs on SD-Ura-His medium with or without NaCl. Data in b and c are means ± SE (n = 4). Different letters above bars represent significant differences at p ≤ 0.05.
To further verify whether LpSAPK9 was involved in plant drought tolerance, we measured its expression levels in three varieties of perennial ryegrass differing in drought tolerance. Notably, “Xialu-4” and “XiaLu-6” bred in our own program had exceptional drought tolerance. Consistently, the PEG-induced growth inhibitions on “XiaLu-4” and “XiaLu-6” were significantly lower than that in “Buena vista” with less decreased plant height, root length, and fresh weight (Figures 9A–D). Under the control condition, the expression level of LpSAPK9 in “Buena vista” was higher than those in “XiaLu-4” and “XiaLu-6”; yet under PEG treatment, the expression of LpSAPK9 decreased in “Buena vista” but was significantly increased in “XiaLu-4” and “Xialu-6” (Figure 9E).
Figure 9. Expression of LpSAPK9 to drought stress in three perennial ryegrass cultivars differing in drought tolerance. (A) The phenotype of three perennial ryegrass cultivars under control and drought stress conditions. (B–D) Growth traits (e.g., plant height, root length, and above-ground biomass) of three perennial ryegrass cultivars under control and drought stress conditions. “BV” and “X” in (D) represent “Buena Vista” and “XiaLu.” (E) Expression levels of LpSAPK9 of three perennial ryegrass cultivars under control and drought stress conditions. Data are means ± SE (n = 4). Different letters above bars represent significant differences at p ≤ 0.05.
Several SAPKs/SnRK2s were identified as important regulators in plant growth and development, ABA signaling transduction, and stress tolerance (Kobayashi et al., 2004; Diédhiou et al., 2008; Huai et al., 2008; Bai et al., 2017). Yet, the SAPK family gene(s) has not been characterized in perennial ryegrass before. In this study, we identified and cloned 10 LpSAPKs in perennial ryegrass using the transcriptomic and genomic databases, characterized expression patterns of LpSAPKs, and screened out a potential positive regulator (LpSAPK9) in plant drought tolerance.
The number of SAPKs proteins in perennial ryegrass was similar to other plant species, such as Arabidopsis (10 SAPKs/SnRK2s; Boudsocq et al., 2004), rice (10; Kobayashi et al., 2004), maize (11; Huai et al., 2008), and brachypodium (10; Wang et al., 2015), in spite of the big variation of their genome sizes. The phylogenetic trees of LpSAPKs in ryegrass, ZmSnRK2s in maize, OsSAPKs in rice, and AtSnRK2s in Arabidopsis were highly similar in three subgroups (Boudsocq et al., 2004; Kobayashi et al., 2004; Huai et al., 2008). And all SAPKs/SnRK2s in cotton, Arabidopsis, and rice were found to have similar genic structures with nine exons (Boudsocq et al., 2004; Huai et al., 2008; Liu et al., 2017). In LpSAPKs, eight have nine exons with two exceptions (LpSAPK5 and LpSAPK10). The coding sequenced-based phylogenetic analysis and full genic sequence-based gene structural (exon–intron) analysis revealed that the SAPKs was a conserved family across dicot and monocot species. Furthermore, the Ka/Ks analysis showed that all LpSAPKs and their orthologs in rice and brachypodium were less than 0.205, suggesting that these genes were under strong purifying selection to keep their conserved functions across different plant species. During evolution, fixation of advantageous mutations would lead to evolutionary innovations causing plants’ divergence and plants’ adaptation to new or constantly changing environments; while removal of deleterious mutations was essential for plants to maintain their “essential” functions (Paterson et al., 2004). Purifying selection of all LpSAPKs suggested that these genes were essential or even indispensable for plants’ survival in nature and their orthologous genes should have similar functions across different plant species.
The C-terminal domain of SnRKs consisted of two subdomains, Domain I and Domain II. Since Domain II was only found in a few specific SnRK2s, it might be responsible for their functional diversity, for example, through interaction with PP2Cs for ABA signaling transduction (Kobayashi et al., 2004; Fujita et al., 2013). In the present study, all the LpSAPKs consist of Domain I, while only LpSAPK8 and LpSAPK10 harbor Domain II. At the transcriptional level, LpSAPK8/10 also responded to ABA treatments. Together, this result indicated that LpSAPK8&10 were likely involved in ABA signaling transduction.
LpSARKs are responsive to hormones and various abiotic stress treatments and are often in the opposite trend in roots and in leaves (Figures 7, 8). Similar results were also found in other plant species (Boudsocq et al., 2004, 2007; Kobayashi et al., 2004; Fujita et al., 2009). For example, most Arabidopsis SnRK2s (except AtSnRK2.9) were responsive to sucrose, mannitol, sorbitol, and NaCl treatments; AtSnRK2.2/2.3/2.6/2.7/2.8 were responsive to ABA treatment; but no AtSnRK2 gene was responsive to low temperature (Boudsocq et al., 2004). Notably, nearly all LpSAPKs responded to stress and hormone treatments in contrasting trends at the transcriptional level. For example, LpSAPK5 was not responsive to all tested treatments in leaves but was upregulated by all tested treatments in roots. This contrasting result for LpSARKs in leaves and roots suggested that these protein kinases should be involved in plant stress-inducible development alternations. For example, under mild drought conditions, plants could develop deeper root systems with reduced leaf sizes. It would be interesting to carry out more functional tests on these LpSAPKs in the future.
On the other hand, the promoter analysis of these genes revealed a number of cis elements associated with hormone and stress responses, such as the ABA-response element (ABRE), gibberellin-response element (GARE), ethylene-responsive elements (ERE), methyl jasmonate response element (CGTCA), low-temperature stress response element (LTRE), stress response element (TC-rich repeats), and MYB binding site for drought-inducible element (MBS). Distinct distributions of cis elements were found on promoters of LpSAPKs. For example, no ERE was identified in the promoters of LpSAPK1/2//10, although these genes were responsive to the ethephon treatment. And no MBS and LTR element was found on promoters of LpSAPK1/2/3/4/8/10 although these genes were responsive to drought and cold stress treatments. Similar results were also found in wheat (Zhang et al., 2016a), tea (Zhang et al., 2018), and soybean (Zhao et al., 2017). These findings indicated that there were likely other unrecognized stress-related cis elements or post-transcriptional regulation (e.g., by small RNAs) involved in the regulation of LpSAPKs.
Several functionally characterized SAPKs/SnRK2s were known for their regulatory roles in plant abiotic stress tolerance. For example, overexpression of AtSnRK2s, OsSAPKs, or TaSnRK2s enhanced plant tolerance to abiotic stresses (Diédhiou et al., 2008; Umezawa et al., 2009; Mao et al., 2010; Zhang et al., 2011). In order to quickly assess their roles in stress tolerance, a yeast heterologous expression system was used. It was found that heterologous expression of the LpSAPK9 increased drought tolerance, but decreased salt tolerance to yeast. However, heterologous expression of the rest LpSAPKs showed no obvious effect on drought or salt stress tolerance in yeast. Furthermore, the expression of LpSAPK9 in different ryegrass cultivars differing in their drought tolerance showed contrasting changes, that is, decreased in leaves of the drought-sensitive variety but increased in the more drought-tolerant varieties. These contrasting expression changes of LpSAPK9 coincided with the drought tolerance degrees of the varieties, suggesting that this gene might be one important regulator in ryegrass drought tolerance. On the other hand, this diversified expression pattern of LpSAPK9 in different ryegrass varieties also reiterated the possibility that this SAPK gene(s) could have been selected and used in ryegrass domestication and breeding.
In the present study, the SAPK/SnRK2 genes were identified and characterized using both in silico and experimental approaches in perennial ryegrass. The LpSAPKs were under strong purifying selection that showed similar genic structures and phylogenetic relationships to their orthologous genes in closely related species. Yet, the promoter regions of these LpSAPKs as well as their expression patterns were different from their orthologs, suggesting that even though their functions were conserved but still could have diverged at the transcriptional level. Furthermore, LpSAPK9 was screened out as a potential positive regulator in ryegrass drought tolerance. Our results will facilitate further functional analysis of LpSAPK family genes for molecular breeding of ryegrass.
The datasets presented in this study can be found in online repositories. The names of the repository/repositories and accession number(s) can be found in the article/Supplementary Material.
JZ and BX conceived the project. JX, RZ, QZ, XH, and TY performed the experiments and analyzed the data. JX and JZ wrote the paper. BX revised the manuscript. All authors contributed to the article and approved the submitted version.
This work was supported by Jiangsu Agriculture Science and Technology Innovation Fund [grant no. CX(21)3004] and National Natural Science Foundation of China (Grant no. 31971757).
The authors declare that the research was conducted in the absence of any commercial or financial relationships that could be construed as a potential conflict of interest.
All claims expressed in this article are solely those of the authors and do not necessarily represent those of their affiliated organizations, or those of the publisher, the editors and the reviewers. Any product that may be evaluated in this article, or claim that may be made by its manufacturer, is not guaranteed or endorsed by the publisher.
The Supplementary Material for this article can be found online at: https://www.frontiersin.org/articles/10.3389/fpls.2022.922564/full#supplementary-material
1. ^https://www.ncbi.nlm.nih.gov/
2. ^https://bioedit.software.informer.com/
3. ^http://www.ncbi.nlm.nih.gov/Structure/cdd/wrpsb.cgi
4. ^https://web.expasy.org/protparam/
5. ^https://www.genome.jp/tools-bin/clustalw
6. ^http://meme-suite.org/tools/meme
Bai, J. P., Mao, J., Yang, H. Y., Khan, A., Fan, A. Q., Liu, S. Y., et al. (2017). Sucrose non-ferment 1 related protein kinase 2 (SnRK2) genes could mediate the stress responses in potato (Solanum tuberosum L.). BMC Genet. 18:41. doi: 10.1186/s12863-017-0506-6
Boudsocq, M., Barbier-Brygoo, H., and Laurière, C. (2004). Identification of nine sucrose nonfermenting1related protein kinases2 activated by hyperosmotic and saline stresses in Arabidopsis thaliana. J. Biol. Chem. 279, 41758–41766. doi: 10.1074/jbc.M405259200
Boudsocq, M., Droillard, M. J., Barbier-Brygoo, H., and Laurière, C. (2007). Different phosphorylation mechanisms are involved in the activation of sucrose nonfermenting1 related protein kinases2 by osmotic stresses and abscisic acid. Plant Mol. Biol. 63, 491–503. doi: 10.1007/s11103-006-9103-1
Chen, C., Chen, H., Zhang, Y., Thomas, H. R., Frank, M. H., He, Y., et al. (2020a). TBtools: an integrative toolkit developed for interactive analyses of big biological data. Mol. Plant 13, 1194–1202. doi: 10.1016/j.molp.2020.06.009
Chen, S. S., Jia, H. L., Wang, X. F., Shi, C., Wang, X., Ma, P. Y., et al. (2020b). Hydrogen sulfide positively regulates abscisic acid signaling through persulfidation of SnRK2.6 in guard cells. Mol. Plant 13, 732–744. doi: 10.1016/j.molp.2020.01.004
Couso, I., Smythers, A. L., Ford, M. M., Umen, J. G., Crespo, J. L., and Hicks, L. M. (2021). Inositol polyphosphates and TOR kinase signaling govern photosystem II protein phosphorylation and photosynthetic function under light stress in Chlamydomonas. New Phytol. 232, 2011–2025. doi: 10.1111/nph.17741
Daniel, F., Elisabeth, V., Daniel, G., Ingrid, S.-S., Aki, M., Rie, S. I., et al. (2021). Ultralong oxford nanopore reads enable the development of a reference-grade perennial ryegrass genome assembly. Genome Biol. Evol. 13: evb159. doi: 10.1093/gbe/evab159
Diédhiou, C. J., Popova, O. V., Dietz, K. J., and Golldack, D. (2008). The SNF1-type serine-threonine protein kinase SAPK4 regulates stress-responsive gene expression in rice. BMC Plant Biol. 8:49. doi: 10.1186/1471-2229-8-49
Fujita, Y., Nakashima, K., Yoshida, T., Katagiri, T., Kidokoro, S., Kanamori, N., et al. (2009). Three SnRK2 protein kinases are the main positive regulators of abscisic acid signaling in response to water stress in Arabidopsis. Plant Cell Physiol. 50, 2123–2132. doi: 10.1093/pcp/pcp147
Fujita, Y., Yoshida, T., and Yamaguchi-Shinozaki, K. (2013). Pivotal role of the AREB/ABF SnRK2 pathway in ABRE-mediated transcription in response to osmotic stress in plants. Physiol. Plant. 147, 15–27. doi: 10.1111/j.1399-3054.2012.01635.x
Hrabak, E. M., Chan, C. W., Gribskov, M., Harper, J. F., Choi, J. H., Halford, N., et al. (2003). The Arabidopsis CDPK-SnRK superfamily of protein kinases. Plant Physiol. 132, 666–680. doi: 10.1104/pp.102.011999
Huai, J. L., Wang, M., He, J. G., Zheng, J., Dong, Z. G., Lv, H. K., et al. (2008). Cloning and characterization of the SnRK2 gene family from Zea mays. Plant Cell Rep. 27, 1861–1868. doi: 10.1007/s00299-008-0608-8
Huang, L. K., Yan, H. D., Jiang, X. M., Yin, G. H., Zhang, X. Q., Qi, X., et al. (2014). Identification of candidate reference genes in perennial ryegrass for quantitative rt-pcr under various abiotic stress conditions. Plos One 9:4. doi: 10.1371/journal.pone.0093724
Julkowska, M. M., Mcloughlin, F., Galvan-Ampudia, C. S., Rankenberg, J. M., Kawa, D., Klimecka, M., et al. (2015). Identification and functional characterization of the Arabidopsis Snf1-related protein kinase SnRK2.4 phosphatidic acid-binding domain. Plant Cell Environ. 38, 614–624. doi: 10.1111/pce.12421
Kobayashi, Y., Yamamoto, S., Minami, H., Kagaya, Y., and Hattori, T. (2004). Differential activation of the rice sucrose nonfermenting1-related protein kinase2 family by hyperosmotic stress and abscisic acid. Plant Cell 16, 1163–1177. doi: 10.1105/tpc.019943
Liu, Z., Ge, X. Y., Yang, Z. R., Zhang, C. J., Zhao, G., Chen, E. Y., et al. (2017). Genome-wide identification and characterization of SnRK2 gene family in cotton (Gossypium hirsutum L.). BMC Genet. 18:54. doi: 10.1186/s12863-017-0517-3
Mao, X., Zhang, H., Tian, S., Chang, X., and Jing, R. (2010). TaSnRK2.4, anSNF1-type serine/threonine protein kinase of wheat (Triticum aestivum L.), confers enhanced multistress tolerance in Arabidopsis. J. Exp. Bot. 61, 683–696. doi: 10.1093/jxb/erp331
Pang, C. H., Li, S. Y., Xia, Y., Yang, Y., and Liu, S. F. (2018). Research progress of plant ABA-independent SnRK2. Plant Physiol. 54, 19–24. doi: 10.13592/j.cnki.ppj.2017.0428
Paterson, A., Bowers, J., and Chapman, B. (2004). Ancient polyploidization predating divergence of the cereals, and its consequences for comparative genomics. P Natl. Acad. Sci. USA 101, 9903–9908. doi: 10.1073/pnas.0307901101
Putta, P., Creque, E., Piontkivska, H., and Kooijman, E. E. (2020). Lipid-protein interactions for ECA1 an N-ANTH domain protein involved in stress signaling in plants. Chem. Phys. Lipids 231:104919. doi: 10.1016/j.chemphyslip.2020.104919
Quintero, F. J., Garciadeblás, B., and Rodríguez-Navarro, A. (1996). The SAL1 gene of Arabidopsis, encoding an enzyme with 3′(2′), 5′-bisphosphate nucleotidase and inositol 1-phosphatase activities, increases salt tolerance in yeast. Plant Cell 8, 529–537.
Song, X., Yu, X., Chiaki, H., Taku, D., Misato, O., and Qiang, Z. (2016). Heterologous overexpression of poplar SnRK2 genes enhanced salt stress tolerance in Arabidopsis thaliana. Front. Plant Sci. 7:612. doi: 10.3389/fpls.2016.00612
Takahashi, Y., Zhang, J., Hsu, P. K., Ceciliato, P. H. O., Zhang, L., Dubeaux, G., et al. (2020). MAP3 kinase-dependent SnRK2-kinase activation is required for abscisic acid signal transduction and rapid osmotic stress response. Nat. Commun. 11, 1–12. doi: 10.1038/s41467-019-13875-y
Tian, S. J., Mao, X. G., Zhang, H. Y., Chen, S. S., Zhai, C. C., Yang, S. M., et al. (2013). Cloning and characterization of TaSnRK2.3, a novel SnRK2 gene in common wheat. J. Exp. Bot. 64, 2063–2080. doi: 10.1093/jxb/ert072
Umezawa, T., Sugiyama, N., Mizoguchi, M., Hayashi, S., Myouga, F., Yamaguchi-Shinozaki, K., et al. (2009). Type 2C protein phosphatases directly regulate abscisic acid-activated protein kinases in Arabidopsis. Proc. Natl. Acad. Sci. U. S. A. 106, 17588–17593. doi: 10.1073/pnas.0907095106
Umezawa, T., Yoshida, R., Maruyama, K., and Shinozaki, K. (2004). SRK2C, a SNF1-related protein kinase 2, improves drought tolerance by controlling stress responsive gene expression in Arabidopsis thaliana. Proc. Natl. Acad. Sci. U. S. A. 101, 17306–17311. doi: 10.1073/pnas.0407758101
Wang, L. Z., Hu, W., Sun, J. T., Liang, X. Y., Yang, X. Y., Wei, S. Y., et al. (2015). Genome-wide analysis of SnRK gene family in Brachypodium distachyon and functional characterization of BdSnRK2.9. Plant Sci. 237, 33–45. doi: 10.1016/j.plantsci.2015.05.008
Winkler, A., Arkind, C., Mattison, C. P., Burkholder, A., and Ota, I. (2002). Heat stress activates the yeast high-osmolarity glycerol mitogen-activated protein kinase pathway, and protein tyrosine phosphatases are essential under heat stress. Eukaryot. Cell 1, 163–173. doi: 10.1128/EC.1.2.163-173.2002
Xu, B., Yu, G. H., Li, H., Xie, Z. N., Wen, W. W., Zhang, J., et al. (2019). Knockdown of STAYGREEN in perennial ryegrass (Lolium perenne L.) leads to transcriptomic alterations related to suppressed leaf senescence and improved forage quality. Plant Cell Physiol. 60, 202–212. doi: 10.1093/pcp/pcy203
Ying, S., Zhang, D. F., Li, H. Y., Liu, Y. H., Shi, Y. S., Song, Y. C., et al. (2011). Cloning and characterization of a maize SnRK2 protein kinase gene confers enhanced salt tolerance in transgenic Arabidopsis. Plant Cell Rep. 30, 1683–1699. doi: 10.1007/s00299-011-1077-z
Yoshida, R., Umezawa, T., Mizoguchi, T., Takahashi, S., Takahashi, F., and Shinozaki, K. (2006). The regulatory domain of SRK2E/OST1/SnRK2.6 interacts with ABI1 and integrates abscisic acid (ABA) and osmotic stress signals controlling stomatal closure in Arabidopsis. J. Biol. Chem. 281, 5310–5318. doi: 10.1074/jbc.M509820200
Zhang, J., Li, H., Huang, X. R., Xing, J., Yao, J. M., Yin, T. C., et al. (2022b). STAYGREEN-mediated chlorophyll a catabolism is critical for photosystem stability during heat-induced leaf senescence in perennial ryegrass. Plant Cell Environ. 45, 1412–1427. doi: 10.1111/pce.14296
Zhang, H. Y., Li, W. Y., Mao, X. G., Jing, R. L., and Jia, H. F. (2016a). Differential activation of the wheat SnRK2 family by abiotic stresses. Front. Plant Sci. 7:420. doi: 10.3389/fpls.2016.00420
Zhang, H., Mao, X., Jing, R., Chang, X., and Xie, H. (2011). Characterization of a common wheat (Triticum aestivum L.) TaSnRK2.7 gene involved in abiotic stress responses. J. Exp. Bot. 62, 975–988. doi: 10.1093/jxb/erq328
Zhang, Y. H., Wan, S. Q., Wang, W. D., Chen, J. F., Huang, L. L., Duan, M. S., et al. (2018). Genome-wide identification and characterization of the CsSnRK2 family in Camellia sinensis. Plant. Physiol. Bioch. 132, 287–296. doi: 10.1016/j.plaphy.2018.09.021
Zhang, J., Yu, G., Wen, W., Ma, X., Xu, B., and Huang, B. (2016b). Functional characterization and hormonal regulation of the PHEOPHYTINASE gene LpPPH controlling leaf senescence in perennial ryegrass. J. Exp. Bot. 67, 935–945. doi: 10.1093/jxb/erv509
Zhang, C. C., Zeng, L. S., Zhang, J., and Xu, B. (2022a). Analysis of phenophase and morphological traits of perennial ryegrass germplasm. Acta. Pratac. Sin. 39, 290–299. doi: 10.11829/j.issn.1001-0629.2021-0365
Zhang, J., Zhang, Q., Xing, J., Li, H. B., Miao, J. M., and Xu, B. (2021). Acetic acid mitigated salt stress by alleviating ionic and oxidative damages and regulating hormone metabolism in perennial ryegrass (Lolium perenne L.). Grass Res. 1, 1–10. doi: 10.48130/GR-2021-0003
Keywords: perennial ryegrass, LpSAPK genes, abiotic stress, plant hormone, drought tolerance
Citation: Xing J, Zhao R, Zhang Q, Huang X, Yin T, Zhang J and Xu B (2022) Genome-Wide Identification and Characterization of the LpSAPK Family Genes in Perennial Ryegrass Highlight LpSAPK9 as an Active Regulator of Drought Stress. Front. Plant Sci. 13:922564. doi: 10.3389/fpls.2022.922564
Received: 18 April 2022; Accepted: 06 May 2022;
Published: 02 June 2022.
Edited by:
Peng Zhou, Shanghai Jiao Tong University, ChinaReviewed by:
Ke Teng, Beijing Academy of Agricultural and Forestry Sciences, ChinaCopyright © 2022 Xing, Zhao, Zhang, Huang, Yin, Zhang and Xu. This is an open-access article distributed under the terms of the Creative Commons Attribution License (CC BY). The use, distribution or reproduction in other forums is permitted, provided the original author(s) and the copyright owner(s) are credited and that the original publication in this journal is cited, in accordance with accepted academic practice. No use, distribution or reproduction is permitted which does not comply with these terms.
*Correspondence: Jing Zhang, bmF1empAbmphdS5lZHUuY24=; Bin Xu, YmlueHVAbmphdS5lZHUuY24=
Disclaimer: All claims expressed in this article are solely those of the authors and do not necessarily represent those of their affiliated organizations, or those of the publisher, the editors and the reviewers. Any product that may be evaluated in this article or claim that may be made by its manufacturer is not guaranteed or endorsed by the publisher.
Research integrity at Frontiers
Learn more about the work of our research integrity team to safeguard the quality of each article we publish.