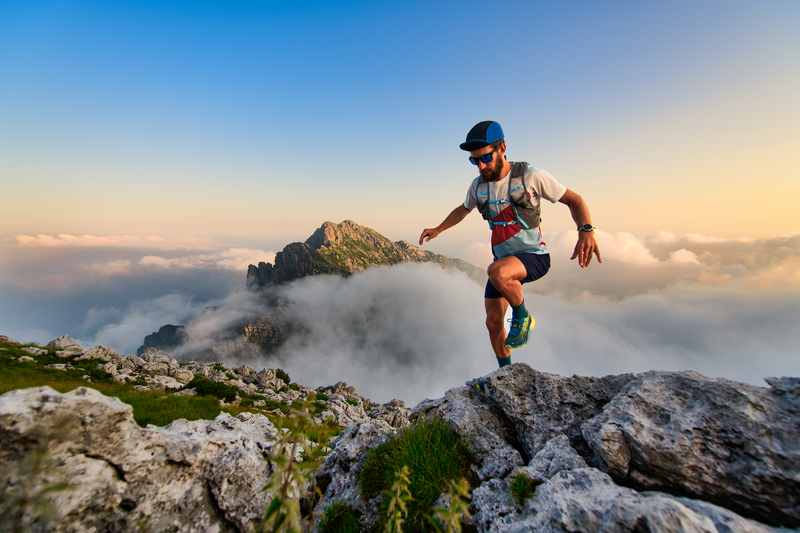
95% of researchers rate our articles as excellent or good
Learn more about the work of our research integrity team to safeguard the quality of each article we publish.
Find out more
ORIGINAL RESEARCH article
Front. Plant Sci. , 27 September 2022
Sec. Crop and Product Physiology
Volume 13 - 2022 | https://doi.org/10.3389/fpls.2022.920570
This article is part of the Research Topic Recent Advances on Nitrogen Use Efficiency in Crop Plants and Climatic Challenges View all 29 articles
Salinity stress (SS) is a challenging abiotic stress that limits crop growth and productivity. Sustainable and cost effective methods are needed to improve crop production and decrease the deleterious impacts of SS. Zinc (Zn) nano-particles (NPs) have emerged as an important approach to regulating plant tolerance against SS. However, the mechanisms of SS tolerance mediated by Zn-NPs are not fully explained. Thus, this study was performed to explore the role of Zn-NPs (seed priming and foliar spray) in reducing the deleterious impacts of SS on wheat plants. The study comprised different SS levels: control, 6 and 12 dS m−1, and different Zn-NPs treatments: control, seed priming (40 ppm), foliar spray (20 ppm), and their combination. Salinity stress markedly reduced plant growth, biomass, and grain yield. This was associated with enhanced electrolyte leakage (EL), malondialdehyde (MDA), hydrogen peroxide (H2O2), sodium (Na), chloride (Cl) accumulation, reduced photosynthetic pigments, relative water contents (RWC), photosynthetic rate (Pn), transpiration rate (Tr), stomata conductance (Gs), water use efficiency (WUE), free amino acids (FAA), total soluble protein (TSP), indole acetic acid (IAA), gibberellic acid (GA), and nutrients (Ca, Mg, K, N, and P). However, the application of Zn-NPs significantly improved the yield of the wheat crop, which was associated with reduced abscisic acid (ABA), MDA, H2O2 concentration, and EL, owing to improved antioxidant activities, and an increase in RWC, Pn, Tr, WUE, and the accumulation of osmoregulating compounds (proline, soluble sugars, TSP, and FAA) and hormones (GA and IAA). Furthermore, Zn-NPs contrasted the salinity-induced uptake of toxic ions (Na and Cl) and increased the uptake of Ca, K, Mg, N, and P. Additionally, Zn-NPs application substantially increased the wheat grain Zn bio-fortification. Our results support previous findings on the role of Zn-NPs in wheat growth, yield, and grain Zn bio-fortification, demonstrating that beneficial effects are obtained under normal as well as adverse conditions, thanks to improved physiological activity and the accumulation of useful compounds. This sets the premise for general use of Zn-NPs in wheat, to which aim more experimental evidence is intensively being sought. Further studies are needed at the genomic, transcriptomic, proteomic, and metabolomic level to better acknowledge the mechanisms of general physiological enhancement observed with Zn-NPs application.
Abiotic stresses undermine crop production and threaten global food security. Salinity stress (SS) is an emerging stress factor as it hampers crop growth and productivity, and global food security (Zafar et al., 2020). Globally, more than 33% of soils are salt-affected, and this figure is expected to increase up to 50% by the end of 2050 (Gopalakrishnan and Kumar, 2020). Salinity stress has a harmful impact on plant metabolic processes and imposes osmotic injuries due to salt-induced ionic toxicity (Egamberdieva et al., 2019; Kamran et al., 2019). SS also disturbs nutrient homeostasis, water uptake, stomata opening, and chlorophyll synthesis. It causes a significant reduction in photosynthetic performance (Zafar et al., 2021). A higher salt concentration in the plant root zone reduces the water uptake, which in turn causes salinity induced osmotic stress, altering normal plant functions (Sonam et al., 2014).
Salinity stress also reduces the uptake of the major cationic nutrients (Ca2+, K+, and Mg2+), and increases the uptake of undesirable ions (Na and Cl; Sharaf et al., 2016). The increase in such ions reduces membrane stability and increases ion toxicity, and decreases photosynthetic efficiency (Zafar et al., 2021) due to stomata closing, reduced chlorophyll synthesis, and carbon dioxide (CO2) assimilation, resulting in stunted plant growth (Shahid et al., 2011). Salinity stress also increases the production of reactive oxygen species (ROS), which damage protein, deoxyribonucleic acid (DNA), lipids, meristematic and photosynthetic activities, destruct chlorophyll, and disturb nutritional homeostasis (Sarker et al., 2018; Hassan et al., 2019a; Aslam et al., 2021; Qari et al., 2022; Rehman et al., 2022; Batool et al., 2022a,b). Moreover, SS also damages chloroplast ultra-structure and photo-system efficiency, electron transport and negatively affects gas exchange (Hurtado et al., 2019). Additionally, SS reduces the number of leaves per plant, root and shoot growth, and biomass production. As these changes can cause huge yield losses (Ramadan et al., 2019; Khan et al., 2022), appropriate measures to mitigate the adverse effects of SS on plants are urgently needed.
More than 40 million tons of agrochemicals are used annually globally for crop production, and this quantity will double by the end of 2050 to feed the 9.5 billion people (Kah et al., 2019). Thus, it is mandatory to introduce new techniques that can effectively achieve sustainable crop productivity. Nanotechnology has emerged as an important field and its use has substantially increased in many areas of life including agriculture. Plants can benefit from nano-particles (NPs) as nutrient sources that can also increase their tolerance against different stresses (Raliya et al., 2017). Various kinds of NPs are used in diverse fields of life; however, zinc (Zn) NPs are widely used in agriculture and different other sectors (Adrees et al., 2021).
Zinc is an important micro-nutrient for plants and has beneficial effects on plant growth; however, it also has some toxic effects at a higher concentration. Zn is involved in protein and chlorophyll synthesis and is also needed for metabolic turnover and enzymatic activities (Saravanakkumar et al., 2016). Not surprisingly, deficiency of Zn has a negative effect on plants, thus, the use of ZnO-NPs is an interesting approach to fulfilling the Zn needs of plants (Du et al., 2019). Approximately, 550 tons Zn-NPs are produced annually across the globe for different uses (Alabdallah and Alzahrani, 2020). The NPs of Zn are considered to be safe as compared to other metals (Kim et al., 2020). Zn nano-particles exert benefits on soil fertility, and crop productivity and they are an important source of Zn, which is an important nutrient for plant growth and protection from different stresses (Rossi et al., 2019; Esper Neto et al., 2020; Salim and Raza, 2020). The use of NPs could minimize the use of toxic and harsh chemicals for plant growth, helping to avoid damage to our environment and soil health (Maroufpour et al., 2020).
The application of NPs is reported to positively influence plant response to SS (Elsheery et al., 2020; Zulfiqar and Ashraf, 2021). NPs induce profound impacts on physiological and biochemical processes (Etesami et al., 2021). Zn-NPs regulate the SS tolerance in plants by improving membrane stability, anti-oxidant activities, ionic homeostasis, and gene expression (Noohpisheh et al., 2021). However, these effects can vary depending on plant species, environmental conditions, size and shape of NPs, and their rate of application (Etesami et al., 2021). Wheat is the staple food of more than 50% of people across the globe; however, it is considered only a moderately salt tolerant species (Spano and Bottega, 2016). Wheat is an important source of both macro and micro nutrients for humans. Among micro nutrients, Zn is an important nutrient needed for humans and plants; however, in both cases, Zn deficiency is an issue of major concern. Bio-fortification is an important strategy globally, to improve the grain Zn concentration and fulfill human needs (Hassan et al., 2019b). This approach involves the use of both agronomic and breeding practices to improve grain Zn (Hassan et al., 2021). Breeding approaches are costly and time demanding (Hassan et al., 2021), and agronomic approaches may be a quick solution to solve this problem (Hassan et al., 2021). The agronomic approach involves the use of micronutrients by different methods to enhance grain Zn concentration (Chattha et al., 2017; Hassan et al., 2019b).
Salt stress significantly reduces the growth and productivity of the wheat crop. Various practices are used globally to overcome the effects of SS on wheat crops. Nonetheless, the effect of Zn-NPs has not been sufficiently studied. Although a few studies are available about the effect of Zn-NPs on the performance of the wheat crops grown under SS, they have not fully explored the mechanism linked to Zn-NP induced SS tolerance. There is no information available related to the effect of Zn-NPs produced through a green approach to wheat growth, yield, and grain Zn concentration under SS.
We hypothesize that the application of Zn-NPs could alleviate the toxic effects of SS by improving antioxidant activities, physiological functioning, and accumulation of stress protecting hormones and osmolytes. The present study aimed to determine the impact of Zn-NPs on physiological attributes, antioxidant enzymes, ionic homeostasis, osmo-regulating compounds, yield, and grain Zn bio-fortification of wheat crops grown under SS.
The experiment was performed at the wire house of the Department of Agronomy, University of Agriculture Faisalabad, Pakistan, to determine the effects of zinc (Zn) nano-particles (NPs) applied by seed priming and foliar spray on the wheat crop under SS. During this period (November 2019 to April 2020), the average temperature of 17.6°C was recorded, whereas average humidity and total rainfall were observed at 71.1% and 28.4 mm. Salt sensitive variety of wheat, Punjab 2011 was used as a test crop. The study comprised three SS levels: control, 6 and 12 dS m−1, and three patterns of Zn-NPs treatment: control, seed priming (40 ppm Zn), foliar spray (20 ppm Zn), and the combination of seed priming (40 ppm) + foliar spray (20 ppm). The soil used in the experiment was taken from an experimental field using a spade. Afterward, debris and weeds were removed, and the soil was sieved and properly stored. The soil was recognized as sandy loam with pH 7.82, organic matter (OM) 0.83%, total nitrogen (N) 0.041%, available phosphorus (P) 6.7 mg kg−1, and exchangeable potassium (K) 158 mg kg−1.
Pots with a capacity of 8 kg and a diameter of 28 cm were then filled with a mixture of soil and sand (9:1). The desirable levels of soil salinity were achieved by adding Na2CO3 and NaCl. The concentration of salts was calculated with the formula below:
In this equation, TSS is total soluble salts that were measured with following formula:
EC1 was the original electrical conductivity (EC) of soil, whereas EC2 was the EC as per our treatments. The soil paste was prepared by adding the distilled water and allowed for 2 h to reach equilibrium. This mixture was then filtered with filter paper to obtain the extract. The soil mixture was then oven dried at 105°C and soil saturation was measured with the following formula:
To attain SS levels 6 and 12 dS m−1, NaCl was added at the rate of 1.179 and 2.58 g/kg, whereas Na2CO3 was added as; 0.023 and 0.50 g/kg, respectively.
Eight seeds were sown in each pot. For seed priming, 40 mg Zn-NPs were dissolved in 1,000 of distilled water, and seeds were soaked in this solution for 6 h before they were dried and sown in pots. For foliar application, 20 mg Zn-NPs were dissolved in 1,000 ml of water to prepare the solution for foliar spray. N and P fertilizers such as urea and di-ammonium phosphate (DAP) were applied at the rate of 1.46 and 1.56 g pot−1, respectively. The pots were regularly visited and irrigated as per crop needs. The irrigation was applied to each based on visual observations with the help of a hand sprayer. The weeds occurring in pots were manually pulled and no insect pest attack was observed; therefore, no pesticide was applied. The leaves of mulberry plants were washed with distilled water; after that, they were oven dried and milled to make powder. The powder samples (20 g) were mixed in distilled water (100 ml) and boiled at 75°C for 45 min (Saravanakkumar et al., 2016). After that, the extract was obtained and stored at 4°C. Later on, Zn-NPs were prepared by adding 20 ml extract to 80 ml of ZnSO4.7H2O. The solution was then sonicated for 1 h at 60°C, and the change in color to yellow showed the formation of Zn-NPs (Eya’ane Meva et al., 2016).
Three plants were chosen at random from each pot to determine growth traits. After that, the roots and shoots of the selected plants were oven dried to determine dry weights. Photosynthetic rate, (Pn), transpiration rate (Tr), stomata conductance (gs), and water use efficiency (WUE) were measured by using a portable infra-red gas analyzer (IRGA) from randomly selected plants from each pot. All these characteristics were recorded at 10–12 am at maximum sunlight.
For determination of chlorophyll contents, 0.5 g of fresh leaves were taken and ground in 80% solution of 5 ml acetone. The ground sample was centrifuged for 3 min. A clear supernatant was collected after centrifugation and absorbance were measured using a spectrophotometer at three wavelengths, i.e., 645 nm (Chl a), 663 nm (Chl. b), and 470 nm (carotenoids) as per methods of Arnon (1949). Subsequently, we took 5 ml of 50 mM potassium phosphate buffer, and an additional 0.5 g of fresh leaves were ground. The material was then centrifuged, the supernatant was placed in a cuvette, and the sample absorbance was measured using a spectrophotometer at 600 nm to determine anthocyanin contents (Kubo et al., 1999).
One gram of fresh leaves was taken and weighed on an electrical scale to determine fresh weight. After that, they were dipped in distilled water (H2O) for 24 h. Then leaves were removed from the water, excess water was wiped out and leaves were weighed again to determine turgid weight. Afterward, the leaves were sun-dried and packed in small paper bags and oven dried at 70°C for 24 h to determine the dry weight. Relative water content (RWC) was determined with this formula: RWC = (FW-DW)/(TW-DW) × 100.
Half a gram of fresh leaf sample (chopped into small pieces) was dipped in distilled water for half an hour, and EC1 was measured by using an EC meter. EC2 was recorded by heating the samples in a water bath at 90°C for 50 min. The final value of electrolyte leakage (EL; %) was determined with this formula:
Test tubes containing 100 μl of plant extract, H2O2 (5.9 mM), and 1,000 μl buffer solution were prepared, and we noted absorbance at 240 nm (Aebi, 1984). The technique of Zhang (1992) was used for the determination of peroxidase (POD) activity. We took 0.1 ml H2O2 added 2.7 ml phosphate buffer and shook it well. Then, 0.1 ml of guicol and 0.1 ml of enzyme extract were added and shaken again, and absorbance was recorded with a spectrophotometer at 470 nm. The mixture containing 100 μl enzyme extract, 100 μl ascorbate (7.5 mM), 100 μl H2O2 (300 mM), and 2.7 ml potassium buffer (25 mM) was prepared, and absorbance was taken at 290 nm for determining ascorbate peroxidase (APX) activity (Nakano and Asada, 1981). Lastly, for determination of POD; 400 μl H2O2, 25 ml buffer, 100 μl Tritoen, 50 μl nitroblue tetrazolium chloride (NBT), 50 μl sample, and 50 μl riboflavin were taken, and absorbance was recorded at 560 nm (Zhang, 1992).
The technique of Velikova et al. (2000) was used to determine the quantity of H2O2 in the sample. We ground 0.25 g of plant leaves in 5 ml of 5% TCA and centrifuged them for 5 min, and the extract was then collected. Then, 1 ml crude sample, 1 ml potassium iodide (1 M), and 100 ml phosphate buffer were added, and absorbance was recorded at 600 nm. For malondialdehyde (MDA) determination, a 0.5 g plant sample was ground in 5 ml of TCA and centrifuged for 15 min. Afterward, the mixture was heated for 30 min at 100°C and cooled rapidly and absorbance was recorded at 532 nm (Rao and Sresty, 2000).
A quantity of 0.5 g plant material was ground in 5 ml potassium buffer, centrifuged for 5 min at 10,000 rpm, and the supernatant was collected. Then, 1 ml 2% ninhydrin solution and 1 ml 10% pyridine solution were added to test tubes containing the extract and warmed for 30 min in a water bath at 90°C. Distilled water was added to maintain volume at 15 ml, and absorbance was recorded with a spectrophotometer at 570 nm (Hamilton and Van Slyke, 1943). In the case of TSP, 0.5 g of plant sample was ground in potassium phosphate buffer (50 mM) and centrifuged for 15 min and 1 ml of supernatant was added with 2 ml of Bradford reagent. This solution was left for 15 min at room temperature, and absorbance was read at 590 nm (Bradford, 1976). In the case of soluble sugars1–2 ml of supernatant was placed on the prism of the refractometer and brix percentage was noted. For proline content, a 0.5 g plant sample was extracted with 10 ml of 3% sulpho-salicylic acid and centrifuged at 10,000 rpm for 10 min. Acid-ninhydrin was then added to the supernatant and placed in a water bath (30 min), and absorbance was recorded at 520 nm (Bates et al., 1973).
For the determination of plant hormones, a 0.5 g plant sample was ground in 80% methanol (2 ml) with 40 mg of butylated hydroxytoluene. Lastly, the obtained extract was dissolved in 0.1% gelatin (pH 7.5), and 0.1% Tween-20 consisting of 2 ml of phosphate-buffered and the concentration of indole-3-acetic acid (IAA) and abscisic acid (ABA) was determined with the method of Weiler et al. (1981). For determination of gibberellic acid (GA) content, 0.1 g plant was taken and extracted by using 3 ml of 96%, ethanol, and absorbance was taken at 254 nm (Berríos et al., 2004).
The plant samples were collected, washed, oven dried (65°C), and ground to make powder. After that, 0.5 g samples were digested in the acid mixture (HCl:HNO3, 1:2) at 180°C for 10 min. The concentration of Na and K was determined by a flame photometer (Sultan et al., 2021). Moreover, for the determination of Cl content, a chloride analyzer was used on the prepared extract. Lastly, the ground wheat grains were taken and digested in the acid mixture (HCl:HNO3, 1:2), then absorbance was recorded using atomic absorption spectrophotometer to determine grain Zn concentration (Sultan et al., 2021).
Randomly selected three plants from each pot were taken to determine the number of tillers, spike length, grains/spike, and spikelets/spike. Then, plant samples were manually harvested, threshed, and grains were separated from the spikes. 1,000-grain weight was recorded by randomly taking 1,000 seeds.
The experiment was conducted in a completely randomized design. The 3 × 3 factorial combinations of SS and Zn-NPs per three replicates made a total of 27 pots. The data on different traits were submitted to the ANOVA for the main factors and their interaction. The LSD test at p ≤ 0.05 was used for determining significant differences among means (Steel et al., 1997). Moreover, figures were created by using the SigmaPlot-8 software.
Salinity stress caused a marked reduction in growth traits of wheat (Table 1). The root and shoot length and biomass were significantly decreased under strong SS (12 dSm−1) as compared to moderate SS (6 dS m−1). In response to this, the application of Zn-NPs significantly mitigated the salinity induced toxic effects and appreciably improved the growth traits and biomass production. However, the application of Zn-NPs by seed priming and foliar spray markedly improved the growth traits as compared to the other methods of single Zn-NPs application. Seed priming (40 ppm) + foliar spray (20 ppm) of Zn-NPS appreciably improved the shoot length (SL: 30.9%), shoot fresh weight (SFW: 54.4%), shoot dry weight (SDW: 48.3%), root fresh weight (RFW: 14.9%), root dry weight (RDW: 19.5%), and leaves per plant (LPP: 12%) as compared to control under strong SS (12 dS m−1; Table 1).
Table 1. Effect of Zn-NPs application on growth attributes of wheat plants grown under salinity stress.
Salinity stress induced a deleterious impact on the photosynthetic pigments (Table 2). The increasing concentration of salts in the growing medium up to 12 dS m−1 significantly reduced the chlorophyll, carotenoid, and anthocyanin contents (Table 2). Nonetheless, the application of Zn-NPs markedly improved the synthesis of all the aforementioned photosynthetic pigments under control and the two SS levels. The combined application of Zn-NPs by seed priming and foliar spray was shown the top performer, and it significantly increased the chlorophyll-a, chlorophyll-b, carotenoid, and anthocyanin by a respective 66.7, 76.6, 54.2, and 93.8% under strong SS (12 dS m−1), as compared to control (Table 2). SS also induced a distinctive impact on all the gas exchange parameters (Figure 1). The increased salt concentration in the growing medium up to 12 dS m−1 decreased the Pn, Tr, Gs, and WUE by 60.6, 34.4, 44.4, and 49.2%, respectively, as compared to the control (Figure 1). The application of Zn-NPS appreciably improved all the gas exchange parameters under SS (Figure 1). However, the combined use of Zn-NPs by seed priming and foliar spray was proved the top performer in improving the Pn, Tr, Gs, and WUE, as compared to other methods of Zn-NPs application and control (Figure 1).
Table 2. Effect of Zn-NPs application on photosynthetic pigments and anthocyanin contents of wheat plants grown under salinity stress.
Figure 1. Effect of Zn-NPs on photosynthetic rate (A), transpiration rate (B), stomata conductance (C), and water use efficiency (D) of wheat plants grown under salinity stress. Data depicted indicate means ± SE (n = 4). Different letters indicate significant differences at p < 0.05.
The RWC of wheat plants grown under SS was significantly decreased, as compared to control plants (Figure 2). An RWC reduction of 14.8 and 25.1%, respectively, was recorded at moderate (6 dS m−1) and strong SS (12 dS m−1), as compared to the control. Zn-NPs markedly improved the RWC of wheat plants under both control and SS conditions. The application of Zn-NPs by seed priming + foliar spray appreciably improved the leaf RWC, as compared to control and other methods of single Zn-NPs application (Figure 2). SS significantly increased the EL, and the MDA and H2O2 accumulation in wheat plants (82, 66.9, and 54.7%, respectively) under a SS level of 12 dS m−1 (Figure 2). The application of Zn-NPs significantly reduced the concentration of all the oxidative stress markers. In this context, the combined application of Zn-NPs by seed priming and foliar spray was once more shown as the top performed, and it significantly reduced the EL (37.6 and 52.5%), and the MDA (21.1 and 28.0%) and H2O2 content (19.0 and 33.9%) under the respective moderate and strong SS (Figure 2).
Figure 2. Effect of Zn-NPs on relative water content (A), electrolyte leakage (B), malondialdeide (C), and hydrogen peroxide (D) of wheat plants grown under salinity stress. Data depicted indicate means ± SE (n = 4). Different letters indicate significant differences at p < 0.05.
The activities of APX, CAT, POD, and SOD were markedly increased under SS. The application of Zn-NPs by all the methods significantly increased the activities of all antioxidants (Figure 3). However, the combined use of Zn-NPs with seed priming and foliar spray was portrayed as the top performer, and it appreciably increased the activity of APX, CAT, POD, and SOD by 14.4, 32.4, 16.4, and 26.4%, respectively, under strong SS as compared to control (Figure 3).
Figure 3. Effect of Zn-NPs on antioxidant activities: catalase (A), peroxidase (B), ascorbate peroxidase (C), and superoxide dismutase (D) of wheat plants grown under salinity stress. Data depicted indicate means ± SE (n = 4). Different letters indicate significant differences at p < 0.05.
A distinctive response of osmoregulating compounds and hormones was recorded under SS and with the application of Zn-NPs. SS significantly reduced the concentration of TSP and FAA, and maximum reduction was recorded under strong SS (Table 3). Moreover, the concentration of total soluble sugars (TSS) and proline showed a significant increase under SS (Table 3). The application of Zn-NPs significantly increased the accumulation of TSP, FAA, TSS, and proline, and the combined use of Zn-NPs by seed priming and foliar spray was shown to the top performed, and it significantly increased the accumulation of all these protective osmolytes (Table 3).
Table 3. Effect of Zn-NPs application on hormones and osmolytes contents of wheat plants grown under salinity stress.
Moreover, an increase in IAA, GA, and ABA accumulation was seen under SS. The application of Zn-NPs also increased the accumulation of IAA and GA, whereas it markedly reduced the accumulation of ABA. The combined application of Zn-NPs by seed priming and foliar spray increased the IAA and GA concentration by 22.6 and 17.7%, respectively, whereas it reduced the ABA concentration by 19.5% under strong SS (Table 3).
The concentration of all the ions was significantly affected by the SS and application of Zn-NPs. SS markedly increased the accumulation of Na and Cl in plant roots and shoots while it reduced the accumulation of K in plant parts (Table 4). Zn-NPs reduced Na and Cl concentration, while they increased the accumulation of K in plant parts grown under SS (Table 4). Likewise, SS also reduced the concentration of Ca, Mg, N, P, and Zn in wheat roots and shoots, and the maximum reduction was noted under strong SS (Tables 4, 5). Zn-NPs appreciably improved the concentration of Ca, Mg, N, P, and Zn in the two plant portions. However, the combined application of Zn-NPs as seed priming and foliar spray significantly increased the concentration of Ca, Mg, N, P, and Zn, as compared to the single seed priming or foliar spray application (Tables 4, 5).
Table 4. Effect of Zn-NPs application on nutrient contents in root and shoot of wheat plants grown under salinity stress.
Table 5. Effect of Zn-NPs application on nutrient contents in root and shoot of wheat plants grown under salinity stress.
Salinity stress and application of Zn-NPs significantly influenced wheat yield traits (Table 6). SS determined sharp reductions of 23.2, 71.1, and 64.8%, respectively, in spike length, spikelets/spike, and grains/spike (Table 6). The application of Zn-NPs mitigated this reduction and significantly increased the aforementioned yield traits under control and SS conditions. Moreover, SS also significantly reduced the 1,000 grain weight and grain yield/pot. The maximum reduction in 1,000 grain weight and grain yield/pot was recorded in 12 dS m−1 SS as compared to 6 dS m−1 SS (Table 6). The combined use of Zn-NPs by seed priming and foliar spray markedly improved the 1,000 grain weight (32.6 and 12.2%, respectively) and grain yield/pot (23.2 and 71.1%, respectively) under moderate and strong SS (Table 6). The increase in salinity significantly decreased the grain Zn concentration (Figure 4), in response to which, the application of Zn-NPs significantly improved the grain Zn concentration. Nonetheless, the Zn-NPs application by combined seed priming and foliar spray significantly increased the grain Zn contents as compared to the other two methods of single Zn-NPs application, and control (Figure 4).
Figure 4. Effect of Zn-NPs on grain Zn concentration of wheat plants grown under salinity stress. Data depicted indicate means ± SE (n = 4). Different letters indicate significant differences at p < 0.05.
Soil salinity is a serious abiotic stress that limits crop productivity across the globe. Wheat is considered a salt sensitive crop, and SS can cause huge growth and yield losses in wheat crops. Therefore, increasing crop production on salt affected soils is a serious challenge (Zhanwu et al., 2014). The present study was planned to avert the effects of SS on the wheat crop by exogenous application of Zn-NPs. SS significantly decreased plant growth (Table 1). Excessive concentration of salts in the growing medium causes ionic toxicity and osmotic imbalance, and disturbs nutrient and water uptake and plant photosynthetic efficiency. All this results in reduced growth and biomass productivity (Lv et al., 2013). The high Na concentration (Table 4) reduced K+ accumulation which in turn serves to reduce cell turgid pressure; therefore, cells become rigid and cannot reach their maximum size (Lv et al., 2013; Abdelgawad et al., 2016; Fatma et al., 2021). However, the application of Zn-NPs significantly improved wheat growth (Table 1). The application of Zn maintained membrane integrity, improved K+ uptake (Table 4), gas exchange parameters (Figure 1), antioxidants (Figure 3), and reduced the uptake of Na, resulting in overall improvement of plant growth. The application of Zn-NPs improved the auxin biosynthesis (Table 3), chlorophyll production, nutrient uptake (Table 4), and reduced the salinity induced stomata closure by decreasing ABA (Table 3), which also contributed to significant increases in growth and biomass productivity (Khaghani and Saffari, 2016; Rajput et al., 2018).
Chlorophyll plays a key role in photosynthesis, and a reduction in chlorophyll content reduces the photosynthetic rate and the accumulation of photosynthates in plant organs (Taiz and Zeiger, 2006). SS increases the activities of enzymes involved in chlorophyll degradation, therefore, contributes to decreasing chlorophyll content (Rao and Rao, 2013). SS also reduces the uptake of Mg2+ ions, which are considered to be building blocks of the chlorophyll molecule; therefore, a decrease in Mg2+ uptake causes a marked reduction in chlorophyll synthesis (Fatma et al., 2021). Moreover, salinity induced oxidative stress damages the chloroplast structure and causes chlorophyll degradation, owing to an increase in chlorophyllase enzyme activity (Latef and Chaoxing, 2011; Latef et al., 2014). However, Zn-NPs reduced the negative impacts of SS and enhanced chlorophyll content (Table 2). The application of Zn-NPs reduced the activities of chlorophyll degradation enzymes and resultantly increased the chlorophyll content and subsequent plant growth under SS (Mehrabani et al., 2018; Sturikova et al., 2018). Zn-NPs can penetrate the plant cells by leaf stomata and improve the plant metabolic activities and synthesis of photosynthetic pigments under stress conditions (Venkatachalam et al., 2017; Alabdallah and Alzahrani, 2020). Zn is crucial for the formation of protochlorophyllide, it positively affects chloroplasts and repairs PS-II, and therefore, causes an increase in photosynthetic pigments under SS (Hänsch and Mendel, 2009; Salama et al., 2019). In our experiment, the application of Zn also positively regulated the Mg2+ uptake, which is considered to be an important component of chlorophyll structure; hence, Zn application increased the chlorophyll content of wheat plants. SS also caused a significant decrease in anthocyanin content (Table 2); however, Zn-NPs increased the anthocyanin contents (Table 2). Anthocyanin regulates ROS accumulation and maintains photosynthetic efficiency (Xu and Rothstein, 2018). Therefore, Zn-NPs mediated increase in anthocyanin content might protect the photosynthetic apparatus and therefore improve the chlorophyll content and plant photosynthetic efficiency.
Salinity stress significantly reduced stomata conductance, transpiration rate, intercellular CO2, and WUE (Figure 1). The reduction in stomata conductance under SS generally occurs due to reductions in vapor pressure, leaf turgor potential, and root generated chemical signals (Chaves et al., 2009). Moreover, a reduction in stomata conductance (Figure 1) significantly reduced the transpiration owing to closing stomata. Additionally, salinity induced accumulation of undesirable ions (Na and Cl), decreases the rate of photosynthesis by damaging the photosynthetic apparatus (Xu and Zhou, 2008; Biswal et al., 2011; Xu and Rothstein, 2018). However, the application of Zn-NPs significantly improved the entire gas exchange parameters under SS, in accordance with the results obtained by Torabian et al. (2016), who found significant improvements in the aforementioned gas exchange parameters with the application of Zn-NPs. Zn application regulated the stomata movements by maintaining higher K+ (Table 4) in guard cells, which maintain higher stomata conductance under SS. Moreover, Zn-NPs also reduced the ABA accumulation (Table 3) that might favor stomata opening and, therefore, maintain higher Tr and WUE in wheat plants under SS. The membrane damage is measured by MDA content, and in the present study, SS caused a significant increase in MDA accumulation (Figure 2). Salinity induced ion toxicity that leads to a higher accumulation of MDA owing to membrane damage. The excessive Na participates in ROS production, which causes damage to cellular membranes and increases the MDA accumulation under SS (Sultan et al., 2021). Zn-NPs alleviated the membrane damage by increasing in anti-oxidant activities (Figure 3), therefore reducing the MDA accumulation under SS (Hussein et al., 2017). RWC was considerably decreased under SS Figure 2); however, the application of Zn contrasted this reduction in leaf RWC (Figure 2). SS induced osmotic stress which reduces water uptake, therefore, reduces the leaf RWC (Kim et al., 2020; Fatma et al., 2021). The present increase in RWC by Zn-NPs may be attributed to improved membrane integrity, stomata conductance, and Tr (Figure 1), as well as an increase in water uptake due to better root growth, which helps the plants to maintain higher leaf RWC. The present study indicates that SS caused a significant increase in Na accumulation in plant parts, while it reduced the K accumulation. The accumulation of Na in plant cells causes ionic imbalance because above a modest concentration Na is a toxic inorganic ion (Chen et al., 2012). The increase in SS increased the Na+ accumulation which inhibited the K uptake (Table 4), with a consequential effect on stomata guard cells (Munns and Tester, 2008; Saddiq et al., 2021). Because of the low concentration of external protons, SS reduces the ability of Na/K antiporters to exclude excessive Na which, therefore, increases the Na accumulation at the expense of K accumulation in plant cells (Munns and Tester, 2008). However, the application of Zn-NPs significantly reduced the Na accumulation while maintaining a higher K concentration in all plant parts (Table 4). Possibly, Zn application maintained the structural integrity and controlled the permeability of root cell membranes; therefore, reduced the excessive Na uptake and consequently reduced the Na accumulation while maintaining higher K accumulation in wheat plants.
The results indicated that SS significantly up-regulated activities of APX, CAT, POD, and SOD; application of Zn-NPs also improved antioxidant activities (Figure 3). SOD is considered to be an important enzyme that decomposes the O2−radical into H2O2, and SOD is also considered the first line of defense under SS. These findings are in agreement with the previous findings of El-Esawi et al. (2018) and who also found a significant increase in anti-oxidant activities (APX, CAT, POD, and SOD) under SS. In the present work, the application of Zn-NPs significantly improved antioxidant activities, which is consistent with the findings of Rizwan et al. (2019), who also found a significant increase in anti-oxidant activities with application of Zn-NPs under SS. The application of Zn in the form of NPs improves the expression of anti-oxidant genes (SOD and CAT), and therefore maintains the higher activity of SOD and CAT, and minimizes the salinity induced oxidative stress (Pavithra et al., 2017). Zn deficiency reduced Zn-SOD activity; however, Zn supply restores enzymatic activity because Zn is a structural component of Zn-SOD (Cakmak, 2000). Zn is also needed for the higher activity of CAT which also plays an important role in H2O2 detoxification (Wani et al., 2013). The application of Zn decomposes O2; therefore, it maintains higher CAT activity under SS (Sharaf et al., 2016). The application of Zn also increases the activity of APX, which is attributed to the ability of Zn to facilitate the synthesis of this antioxidant (Cakmak, 2000; Amini et al., 2016). Lastly, an increase in CAT activity following the application of Zn-NPs also indicates that the Zn-NPs improve CAT activity to mitigate the adverse effects of SS.
A higher concentration of proline under SS suggests that plants accumulate proline as a defensive osmolyte against SS (Hmidi et al., 2018). SS also reduced the IAA concentration, which could be due to over-accumulation of nitric oxide (NO) (Liu et al., 2015). It is well known that ABA increases under SS and handles stomata closure; nonetheless, this trade-off reduces plant growth and photosynthesis due to limitations in gas exchange (Fujita et al., 2005; Fatma et al., 2021). The increase in ABA under SS induced stomata closure and reduced the Tr (Figure 1), thereby reducing WUE and overall photosynthetic efficiency (Tanaka et al., 2013). Plants also accumulate soluble sugars. Which appreciably maintains the turgor pressure and enhances the membrane stability under SS by scavenging ROS (Hussein et al., 2017). Zn-NPs maintained a higher proline and soluble sugar accumulation (Table 3). This increase can be attributed to an increase in the activities of enzymes involved in proline biosynthesis. The application of Zn-NPs maintained a higher IAA level. Zn is known to be a co-enzyme needed for tryptophan synthesis that is a precursor to the formation of IAA (Waraich et al., 2011). Therefore, Zn induces an increase in IAA that can be attributed to an increase in tryptophan synthesis under SS. The increase in IAA improves root growth, which facilitates nutrient and water uptake; therefore, IAA improves plant growth under SS conditions (Waraich et al., 2011). The application of Zn-NPs also improved GA concentration, which also leads to a substantial increase in growth (Table 1) by regulating plant osmotic balance (Jamil et al., 2018). The application of Zn-NPs significantly reduced the ABA accumulation, which could be an important mechanism of Zn induced SS tolerance in plants. However, more studies at the metabolomic, proteomic, and transcriptomic level are needed to explore how Zn-NPs application reduces the accumulation of ABA under SS.
The results indicated that SS caused a significant reduction in yield and yield traits of wheat plants. SS induced ionic, osmotic, and oxidative stresses which damage the photosynthetic apparatus and alter plant physiological functioning as stomata closure, reduction in leaf expansion, and altered gas exchange parameters, which in turn reduce the final productivity (Zahra et al., 2018; Javaid et al., 2019). The higher concentration of Na causes water deficiency, which is a major reason for a substantial reduction in crop yield across the globe (Iqbal et al., 2019; Yadav et al., 2020). However, in this experiment, the Zn application significantly improved the yield and yield traits under SS, thanks to improved plant physiological functioning, gas exchange parameters (Figure 1), metabolic activities, chlorophyll synthesis, antioxidant activity, and accumulation of osmolytes (Hassan et al., 2020). SS significantly reduced the grain Zn content, which could be attributed to excessive Na concentration in the growing medium reducing the Zn uptake. However, Zn-NPs determined a noticeable bio-fortification of wheat grain. The application of Zn-NPs reduced Na uptake while maintaining a higher uptake of Zn, which contributed to a significant improvement in grain Zn content. Moreover, the results indicated that different methods of Zn-NPs application significantly affected the grain Zn content; nonetheless, the combined application of seed priming + foliar spray significantly improved the grain Zn concentration (Figure 4). The foliar applied Zn markedly increased the grain Zn content, although a little quantity of Zn is applied in this method as compared to soil application (Cakmak et al., 2010). The foliar applied Zn maintains higher Zn availability within plant tissues during reproductive stages; therefore, it substantially increased the grain Zn concentration (Chattha et al., 2017).
Salinity stress markedly affected the wheat plant by altering the photosynthetic performance, nutrient uptake, osmolyte, and hormone accumulation. However, the application of Zn-NPs offset the salinity induced toxic effects, and improved wheat growth and final yield. The application of Zn-NPs showed a reduction in EL, MDA, H2O2, and ABA accumulation, in exchange for an increase in photosynthetic pigments, leaf gas exchange parameters, hormone, and osmolyte accumulation, and antioxidant activities, resulting in a substantial improvement in plant growth functions. Further, the beneficial role of Zn-NPs was evidenced by the restricted entry of toxic ions (Na and Cl), and improved uptake of major nutrients as the sodium contrasting cations Ca, K, and Mg, plus N and P. Based on our results, the application of Zn-NPs can be seen an effective approach to improve wheat growth, final yield and obtain grain Zn bio-fortification under salinity stress as well as normal conditions. Therefore, it is evinced that Zn-NPs use, especially the double seed priming and foliar spray application, could be a winning strategy under many circumstances, although further evidence is needed in support of this. Additionally, further studies are needed at a genomic, transcriptomic, proteomic, and metabolomic level to explore the mechanisms of Zn-NPs in inducing the general enhancement in physiological functions that, especially under SS, appear a valuable means to face stress. Lastly, more investigations are needed to optimize the rate of Zn-NPs for the wheat crop under variable cropping systems and climate conditions.
The original contributions presented in the study are included in the article/supplementary material.
MUC and IK: conceptualization. TA: data collection. MUC and MUH: writing original draft. IK, MN, MBC, HMA, RYG, NRA, SA and LB review and editing. All authors contributed to the article and approved the submitted version.
This research was funded by the Researchers Support Project (RSP-2021/123), King Saud University, Riyadh, Saudi Arabia.
The authors would like to extend their sincere appreciation to the Researchers Supporting Project (RSP-2021/123) at King Saud University, Riyadh, Saudi Arabia.
The authors declare that the research was conducted in the absence of any commercial or financial relationships that could be construed as a potential conflict of interest.
All claims expressed in this article are solely those of the authors and do not necessarily represent those of their affiliated organizations, or those of the publisher, the editors and the reviewers. Any product that may be evaluated in this article, or claim that may be made by its manufacturer, is not guaranteed or endorsed by the publisher.
Abdelgawad, H., Zinta, G., Hegab, M. M., Pandey, R., Asard, H., and Abuelsoud, W. (2016). High salinity induces different oxidative stress and antioxidant responses in maize seedlings organs. Front. Plant Sci. 7:276. doi: 10.3389/fpls.2016.00276
Adrees, M., Khan, Z. S., Hafeez, M., Rizwan, M., Hussain, K., Asrar, M., et al. (2021). Foliar exposure of zinc oxide nanoparticles improved the growth of wheat (Triticum aestivum L.) and decreased cadmium concentration in grains under simultaneous cd and water deficient stress. Ecotoxicol. Environ. Saf. 208:111627. doi: 10.1016/j.ecoenv.2020.111627
Aebi, H. (1984). “[13] Catalase in vitro,” in Methods in Enzymology. Vol. 105. (Academic press), 121–126.
Alabdallah, N. M., and Alzahrani, H. S. (2020). Impact of ZnO nanoparticles on growth of cowpea and okra plants under salt stress conditions. Biosci. Biotech. Res. Asia 17, 329–340. doi: 10.13005/bbra/2836
Amini, S., Ghadiri, H., Chen, C., and Marschner, P. (2016). Salt-affected soils, reclamation, carbon dynamics, and biochar: a review. J. Soils Sediments 16, 939–953. doi: 10.1007/s11368-015-1293-1
Arnon, D. I. (1949). Copper enzymes in isolated chloroplasts Polyphenoloxidase in Beta vulgaris. Plant Physiol. 24, 1–15.
Aslam, A., Khan, S., Ibrar, D., Irshad, S., Bakhsh, A., Gardezi, S. T. R., et al. (2021). Defensive impact of foliar applied potassium nitrate on growth linked with improved physiological and Antioxidative activities in sunflower (Helianthus annuus L.) hybrids grown under salinity stress. Agronomy 11, 2076. doi: 10.3390/agronomy11102076
Bates, L. S., Waldren, R. P., and Teare, I. (1973). Rapid determination of free proline for water-stress studies. Plant Soil 39, 205–207. doi: 10.1007/BF00018060
Batool, M., El-Badri, A. M., Hassan, M. U., Haiyun, Y., Chunyun, W., Zhenkun, Y., et al. (2022a). Drought stress in Brassica napus: effects, tolerance mechanisms, and management strategies. J. Plant Growth Regul., 1–25. doi: 10.1007/s00344-021-10542-9
Batool, M., El-Badri, A. M., Wang, Z., Mohamed, I. A., Yang, H., Ai, X., et al. (2022b). Rapeseed morpho-physio-biochemical responses to drought stress induced by PEG-6000. Agronomy 12, 579. doi: 10.3390/agronomy12030579
Berríos, J., Illanes, A., and Aroca, G. (2004). Spectrophotometric method for determining gibberellic acid in fermentation broths. Biotechnol. Lett. 26, 67–70. doi: 10.1023/B:BILE.0000009463.98203.8b
Biswal, B., Joshi, P., Raval, M., and Biswal, U. (2011). Photosynthesis, a global sensor of environmental stress in green plants: stress signalling and adaptation. Curr. Sci. 101, 47–56.
Bradford, M. M. (1976). A rapid and sensitive method for the quantitation of microgram quantities of protein utilizing the principle of protein-dye binding. Anal. Biochem. 72, 248–254. doi: 10.1016/0003-2697(76)90527-3
Cakmak, I. (2000). Tansley review no. 111: possible roles of zinc in protecting plant cells from damage by reactive oxygen species. New Phytol. 146, 185–205. doi: 10.1046/j.1469-8137.2000.00630.x
Cakmak, I., Pfeiffer, W. H., and Mcclafferty, B. (2010). Biofortification of durum wheat with zinc and iron. Cereal Chem. 87, 10–20. doi: 10.1094/CCHEM-87-1-0010
Chattha, M. U., Hassan, M. U., Khan, I., Chattha, M. B., Mahmood, A., Chattha, M. U., et al. (2017). Biofortification of wheat cultivars to combat zinc deficiency. Front. Plant Sci. 8:281. doi: 10.3389/fpls.2017.00281
Chaves, M. M., Flexas, J., and Pinheiro, C. (2009). Photosynthesis under drought and salt stress: regulation mechanisms from whole plant to cell. Ann. Bot. 103, 551–560. doi: 10.1093/aob/mcn125
Chen, S., Xing, J., and Lan, H. (2012). Comparative effects of neutral salt and alkaline salt stress on seed germination, early seedling growth and physiological response of a halophyte species Chenopodium glaucum. Afri. J. Biotech. 11, 9572–9581. doi: 10.5897/AJB12.320
Du, W., Yang, J., Peng, Q., Liang, X., and Mao, H. (2019). Comparison study of zinc nanoparticles and zinc sulphate on wheat growth: From toxicity and zinc biofortification. Chemosphere 227, 109–116. doi: 10.1016/j.chemosphere.2019.03.168
Egamberdieva, D., Wirth, S., Bellingrath-Kimura, S. D., Mishra, J., and Arora, N. K. (2019). Salt-tolerant plant growth promoting rhizobacteria for enhancing crop productivity of saline soils. Front. Microbiol. 10:2791. doi: 10.3389/fmicb.2019.02791
El-Esawi, M. A., Alaraidh, I. A., Alsahli, A. A., Alzahrani, S. M., Ali, H. M., Alayafi, A. A., et al. (2018). Serratia liquefaciens KM4 improves salt stress tolerance in maize by regulating redox potential, ion homeostasis, leaf gas exchange and stress-related gene expression. Int. J. Mol. Sci. 19, 3310. doi: 10.3390/ijms19113310
Elsheery, N. I., Helaly, M. N., El-Hoseiny, H. M., and Alam-Eldein, S. M. (2020). Zinc oxide and silicone nanoparticles to improve the resistance mechanism and annual productivity of salt-stressed mango trees. Agronomy 10, 558. doi: 10.3390/agronomy10040558
Esper Neto, M., Britt, D. W., Lara, L. M., Cartwright, A., Dos Santos, R. F., Inoue, T. T., et al. (2020). Initial development of corn seedlings after seed priming with nanoscale synthetic zinc oxide. Agronomy 10, 307. doi: 10.3390/agronomy10020307
Etesami, H., Fatemi, H., and Rizwan, M. (2021). Interactions of nanoparticles and salinity stress at physiological, biochemical and molecular levels in plants: A review. Ecotoxicol. Environ. Saf. 225:112769. doi: 10.1016/j.ecoenv.2021.112769
Eya’ane Meva, F., Segnou, M. L., Ebongue, C. O., Ntoumba, A. A., Kedi, P. B. E., Deli, V., et al. (2016). Spectroscopic synthetic optimizations monitoring of silver nanoparticles formation from Megaphrynium macrostachyum leaf extract. Rev. Bras 26, 640–646. doi: 10.1016/j.bjp.2016.06.002
Fatma, M., Iqbal, N., Gautam, H., Sehar, Z., Sofo, A., D’ippolito, I., et al. (2021). Ethylene and sulfur coordinately modulate the antioxidant system and ABA accumulation in mustard plants under salt stress. Plan. Theory 10, 180. doi: 10.3390/plants10010180
Fujita, Y., Fujita, M., Satoh, R., Maruyama, K., Parvez, M. M., Seki, M., et al. (2005). AREB1 is a transcription activator of novel ABRE-dependent ABA signaling that enhances drought stress tolerance in Arabidopsis. Plant Cell 17, 3470–3488. doi: 10.1105/tpc.105.035659
Gopalakrishnan, T., and Kumar, L. (2020). Modeling and mapping of soil salinity and its impact on Paddy lands in Jaffna peninsula, Sri Lanka. Sustain. For. 12, 8317. doi: 10.3390/su12208317
Hamilton, P., and Van Slyke, D. (1943). Amino acid determination with ninhydrin. J. Biol. Chem. 150, 231–250. doi: 10.1016/S0021-9258(18)51268-0
Hänsch, R., and Mendel, R. R. (2009). Physiological functions of mineral micronutrients (Cu, Zn, Mn, Fe, Ni, Mo, B, Cl). Curr. Opin. Plant Biol. 12, 259–266. doi: 10.1016/j.pbi.2009.05.006
Hassan, M. U., Aamer, M., Chattha, M. U., Haiying, T., Shahzad, B., Barbanti, L., et al. (2020). The critical role of zinc in plants facing the drought stress. Agriculture 10, 396. doi: 10.3390/agriculture10090396
Hassan, M. U., Aamer, M., Nawaz, M., Rehman, A., Aslam, T., Afzal, U., et al. (2021). Agronomic bio-fortification of wheat to combat zinc deficiency in developing countries. Pak. J. Agric. Res. 34, 201–217. doi: 10.17582/journal.pjar/2021/34.1.201.217
Hassan, M. U., Chattha, M. U., Khan, I., Chattha, M. B., Aamer, M., Nawaz, M., et al. (2019a). Nickel toxicity in plants: reasons, toxic effects, tolerance mechanisms, and remediation possibilities—a review. Environ. Sci. Pollut. Res. 26, 12673–12688. doi: 10.1007/s11356-019-04892-x
Hassan, M. U., Chattha, M. U., Ullah, A., Khan, I., Qadeer, A., Aamer, M., et al. (2019b). Agronomic biofortification to improve productivity and grain Zn concentration of bread wheat. Int. J. Agric. Biol. 21, 615–620.
Hmidi, D., Abdelly, C., Athar, H.-U.-R., Ashraf, M., and Messedi, D. (2018). Effect of salinity on osmotic adjustment, proline accumulation and possible role of ornithine-δ-aminotransferase in proline biosynthesis in Cakile maritima. Physiol. Mol. Biol. Plants 24, 1017–1033. doi: 10.1007/s12298-018-0601-9
Hurtado, A. C., Chiconato, D. A., De Mello Prado, R., Junior, G. D. S. S., and Felisberto, G. (2019). Silicon attenuates sodium toxicity by improving nutritional efficiency in sorghum and sunflower plants. Plant Physiol. Biochem. 142, 224–233. doi: 10.1016/j.plaphy.2019.07.010
Hussein, M., Embiale, A., Husen, A., Aref, I. M., and Iqbal, M. (2017). Salinity-induced modulation of plant growth and photosynthetic parameters in faba bean (Vicia faba) cultivars. Pak. J. Bot. 49, 867–877.
Iqbal, S., Basra, S. M., Afzal, I., Wahid, A., Saddiq, M. S., Hafeez, M. B., et al. (2019). Yield potential and salt tolerance of quinoa on salt-degraded soils of Pakistan. J. Agron. Crop Sci. 205, 13–21. doi: 10.1111/jac.12290
Jamil, M., Ahamd, M., Anwar, F., Zahir, Z. A., Kharal, M. A., and Nazli, F. (2018). Inducing drought tolerance in wheat through combined use of l-tryptophan and Pseudomonas fluorescens. Pak. J. Agric. Sci. 55, 331–337.
Javaid, T., Farooq, M. A., Akhtar, J., Saqib, Z. A., and Anwar-Ul-Haq, M. (2019). Silicon nutrition improves growth of salt-stressed wheat by modulating flows and partitioning of Na+, Cl− and mineral ions. Plant Physiol. Biochem. 141, 291–299. doi: 10.1016/j.plaphy.2019.06.010
Kah, M., Tufenkji, N., and White, J. C. (2019). Nano-enabled strategies to enhance crop nutrition and protection. Nat. Nanotechnol. 14, 532–540. doi: 10.1038/s41565-019-0439-5
Kamran, M., Parveen, A., Ahmar, S., Malik, Z., Hussain, S., Chattha, M. S., et al. (2019). An overview of hazardous impacts of soil salinity in crops, tolerance mechanisms, and amelioration through selenium supplementation. Int. J. Mol. Sci. 21, 148. doi: 10.3390/ijms21010148
Khaghani, S., and Saffari, J. (2016). Microwave-assisted chemical preparation of ZnO nanoparticles and its application on the improving grain yield, quantity and quality of safflower (Carthamus tinctorius L.). J. Nanostrac. 6, 43–48. doi: 10.7508/JNS.2016.01.007
Khan, I., Chattha, M. U., Skalicky, M., Chattha, M. B., Ahsin, A., Rizwan, M. A., et al. (2022). Mitigation of salinity induced oxidative damage, growth and yield reduction in fine rice by sugarcane press-mud application. Front. Plant Sci. 13:840900. doi: 10.3389/fpls.2022.840900
Kim, I., Viswanathan, K., Kasi, G., Thanakkasaranee, S., Sadeghi, K., and Seo, J. (2020). ZnO nanostructures in active antibacterial food packaging: preparation methods, antimicrobial mechanisms, safety issues, future prospects, and challenges. Food Rev. Int. 38, 537–565. doi: 10.1080/87559129.2020.1737709
Kubo, H., Peeters, A. J. M., Aarts, M. G. M., Pereira, A., and Koornneef, M. (1999). ANTHOCYANINLESS2, a homeobox gene affecting anthocyanins distribution and root development in Arabidopsis. Plant Cell 11, 1217–1226.
Latef, A., Alhmad, M., and Sallam, M. (2014). Comparative study on the physiological response of two wheat cultivars to salinity stress. Assiut. Univ. J. Bot. 43, 55–69.
Latef, A. H. A., and Chaoxing, H. (2011). Effect of arbuscular mycorrhizal fungi on growth, mineral nutrition, antioxidant enzymes activity and fruit yield of tomato grown under salinity stress. Sci. Hortic. 127, 228–233. doi: 10.1016/j.scienta.2010.09.020
Liu, W., Li, R.-J., Han, T.-T., Cai, W., Fu, Z.-W., and Lu, Y.-T. (2015). Salt stress reduces root meristem size by nitric oxide-mediated modulation of auxin accumulation and signaling in Arabidopsis. Plant Physiol. 168, 343–356. doi: 10.1104/pp.15.00030
Lv, B. S., Li, X. W., Ma, H. Y., Sun, Y., Wei, L. X., Jiang, C. J., et al. (2013). Differences in growth and physiology of rice in response to different saline-alkaline stress factors. Agron. J. 105, 1119–1128. doi: 10.2134/agronj2013.0017
Maroufpour, N., Mousavi, M., Abbasi, M., and Ghorbanpour, M. (2020). “Biogenic nanoparticles as novel sustainable approach for plant protection,” in Biogenic Nano-Particles and their Use in Agro-Ecosystems eds. M. Ghorbanpour, P. Bhargava, A. Varma, and D. K. Choudhary (Springer Nature Singapore Pte Ltd.), 161–172.
Mehrabani, L. V., Hassanpouraghdam, M. B., and Shamsi-Khotab, T. (2018). The effects of common and nano-zinc foliar application on the alleviation of salinity stress in Rosmarinus officinalis L. Acta Sci. Pol. Hortorum Cultus 17, 65–73. doi: 10.24326/asphc.2018.6.7
Munns, R., and Tester, M. (2008). Mechanisms of salinity tolerance. Annu. Rev. Plant Biol. 59, 651–681. doi: 10.1146/annurev.arplant.59.032607.092911
Nakano, Y., and Asada, K. (1981). Hydrogen peroxide is scavenged by ascorbate-specific peroxidase in spinach chloroplasts. Plant Cell Physiol. 22, 867–880.
Noohpisheh, Z., Amiri, H., Mohammadi, A., and Farhadi, S. (2021). Effect of the foliar application of zinc oxide nanoparticles on some biochemical and physiological parameters of Trigonella foenum-graecum under salinity stress. Plant biosystems-An Int. J. Deal. Aspects Plant Biol. 155, 267–280. doi: 10.1080/11263504.2020.1739160
Pavithra, G., Rajashekar Reddy, B., Salimath, M., Geetha, K., and Shankar, A. (2017). Zinc oxide nano particles increases Zn uptake, translocation in rice with positive effect on growth, yield and moisture stress tolerance. Indian J. Plant Physiol. 22, 287–294. doi: 10.1007/s40502-017-0303-2
Qari, S. H., Hassan, M. U., Chattha, M. U., Mahmood, A., Naqve, M., Nawaz, M., et al. (2022). Melatonin induced cold tolerance in plants: physiological and molecular responses. Front. Plant Sci. 13:843071. doi: 10.3389/fpls.2022.843071
Rajput, V. D., Minkina, T. M., Behal, A., Sushkova, S. N., Mandzhieva, S., Singh, R., et al. (2018). Effects of zinc-oxide nanoparticles on soil, plants, animals and soil organisms: a review. Environ. Nanotech. Monit. Manag. 9, 76–84. doi: 10.1016/j.enmm.2017.12.006
Raliya, R., Saharan, V., Dimkpa, C., and Biswas, P. (2017). Nanofertilizer for precision and sustainable agriculture: current state and future perspectives. J. Agric. Food Chem. 66, 6487–6503. doi: 10.1021/acs.jafc.7b02178
Ramadan, A. A., Abd Elhamid, E. M., and Sadak, M. S. (2019). Comparative study for the effect of arginine and sodium nitroprusside on sunflower plants grown under salinity stress conditions. Bull. Nat. Res. Cent. 43, 1–12. doi: 10.1186/s42269-019-0156-0
Rao, G., and Rao, G. (2013). Pigment composition and chlorophyllase activity in pigeon pea (Cajanus indicus Spreng) and Gingelley (Sesamum indicum L.) under NaCl salinity (1981). Indian J. Exp. Biol. 19, 768–770.
Rao, K. M., and Sresty, T. (2000). Antioxidative parameters in the seedlings of pigeonpea (Cajanus cajan (L.) Millspaugh) in response to Zn and Ni stresses. Plant Sci. 157, 113–128.
Rehman, S., Chatta, M. U., Imran, K., Athar, M., Hassan, M. U., Asma, A. A. H., et al. (2022). Exogenously applied trehalose augments cadmium stress tolerance and yield of mung bean (Vigna radiata L.) grown in soil and hydroponic systems through reducing cd uptake and enhancing photosynthetic efficiency and antioxidant defense systems. Plan. Theory 11, 822. doi: 10.3390/plants11060822
Rizwan, M., Ali, S., Ur Rehman, M. Z., Adrees, M., Arshad, M., Qayyum, M. F., et al. (2019). Alleviation of cadmium accumulation in maize (Zea mays L.) by foliar spray of zinc oxide nanoparticles and biochar to contaminated soil. Environ. Pollut. 248, 358–367. doi: 10.1016/j.envpol.2019.02.031
Rossi, L., Fedenia, L. N., Sharifan, H., Ma, X., and Lombardini, L. (2019). Effects of foliar application of zinc sulfate and zinc nanoparticles in coffee (Coffea arabica L.) plants. Plant Physiol. Biochem. 135, 160–166. doi: 10.1016/j.plaphy.2018.12.005
Saddiq, M. S., Afzal, I., Iqbal, S., Hafeez, M. B., and Raza, A. (2021). Low leaf sodium content improves the grain yield and physiological performance of wheat genotypes in saline-sodic soil. Pesquisa Agrop. Trop. 51, 1–10. doi: 10.1590/1983-40632021v5167663
Salama, D. M., Osman, S. A., Abd El-Aziz, M., Abd Elwahed, M. S., and Shaaban, E. (2019). Effect of zinc oxide nanoparticles on the growth, genomic DNA, production and the quality of common dry bean (Phaseolus vulgaris). Biocat. Agric. Biotech. 18:101083. doi: 10.1016/j.bcab.2019.101083
Salim, N., and Raza, A. (2020). Nutrient use efficiency (NUE) for sustainable wheat production: a review. J. Plant Nutr. 43, 297–315. doi: 10.1080/01904167.2019.1676907
Saravanakkumar, D., Sivaranjani, S., Umamaheswari, M., Pandiarajan, S., and Ravikumar, B. (2016). Green synthesis of ZnO nanoparticles using Trachyspermum ammi seed extract for antibacterial investigation. Der Phar. Chem. 8, 173–180.
Sarker, U., Islam, M. T., and Oba, S. (2018). Salinity stress accelerates nutrients, dietary fiber, minerals, phytochemicals and antioxidant activity in Amaranthus tricolor leaves. PLoS One 13:e0206388. doi: 10.1371/journal.pone.0206388
Shahid, M. A., Pervez, M. A., Balal, R. M., Ahmad, R., Ayyub, C. M., Abbas, T., et al. (2011). Salt stress effects on some morphological and physiological characteristics of okra (Abelmoschus esculentus L.). Soil Environ. 30, 66–73.
Sharaf, E., Sofy, A., Sofy, M., and Mabrouk, A. (2016). Effect of zinc on antioxidant enzyme activities of common bean under drought stress. J. Biol. Chem. Res. 33, 669–676.
Sonam, S., Anirudha, R., and Subhash, C. (2014). Effect of short term salt stress on chlorophyll content, protein and activities of catalase and ascorbate peroxidase enzymes in pearl millet. Am. J. Plant Physiol. 9, 32–37. doi: 10.3923/ajpp.2014.32.37
Spano, C., and Bottega, S. (2016). Durum wheat seedlings in saline conditions: salt spray versus root-zone salinity. Estuar. Coast. Shelf Sci. 169, 173–181. doi: 10.1016/j.ecss.2015.11.031
Steel, R. G. D., Torrie, J. H., and Dicky, D. A. (1997). Principles and Procedures of Statistics: A Biometrical Approach, 3rd Edn. New York, NY, USA: McGraw Hill, Inc., Book Co., 352–358.
Sturikova, H., Krystofova, O., Huska, D., and Adam, V. (2018). Zinc, zinc nanoparticles and plants. J. Hazard. Mater. 349, 101–110. doi: 10.1016/j.jhazmat.2018.01.040
Sultan, I., Khan, I., Chattha, M. U., Hassan, M. U., Barbanti, L., Calone, R., et al. (2021). Improved salinity tolerance in early growth stage of maize through salicylic acid foliar application. Ital. J. Agron. 16, 1810. doi: 10.4081/ija.2021.1810
Taiz, L., and Zeiger, E. (2006). Plant Physiology, 4th Edn. Sunderland, MA, USA: Sinauer Associates, Inc., Publishers, 764
Tanaka, Y., Nose, T., Jikumaru, Y., and Kamiya, Y. (2013). ABA inhibits entry into stomatal-lineage development in A rabidopsis leaves. Plant J. 74, 448–457. doi: 10.1111/tpj.12136
Torabian, S., Zahedi, M., and Khoshgoftar, A. H. (2016). Effects of foliar spray of two kinds of zinc oxide on the growth and ion concentration of sunflower cultivars under salt stress. J. Plant Nutr. 39, 172–180. doi: 10.1080/01904167.2015.1009107
Velikova, V., Yordanov, I., and Edreva, A. (2000). Oxidative stress and some antioxidant systems in acid rain-treated bean plants: protective role of exogenous polyamines. Plant Sci. 151, 59–66. doi: 10.1016/S0168-9452(99)00197-1
Venkatachalam, P., Priyanka, N., Manikandan, K., Ganeshbabu, I., Indiraarulselvi, P., Geetha, N., et al. (2017). Enhanced plant growth promoting role of phycomolecules coated zinc oxide nanoparticles with P supplementation in cotton (Gossypium hirsutum L.). Plant Physiol. Biochem. 110, 118–127. doi: 10.1016/j.plaphy.2016.09.004
Wani, A. S., Ahmad, A., Hayat, S., and Fariduddin, Q. (2013). Salt-induced modulation in growth, photosynthesis and antioxidant system in two varieties of Brassica juncea. Saudi J. Biol. Sci. 20, 183–193. doi: 10.1016/j.sjbs.2013.01.006
Waraich, E. A., Ahmad, R., and Ashraf, M. (2011). Role of mineral nutrition in alleviation of drought stress in plants. Aust. J. Crop. Sci. 5, 764–777.
Weiler, E., Jourdan, P., and Conrad, W. (1981). Levels of indole-3-acetic acid in intact and decapitated coleoptiles as determined by a specific and highly sensitive solid-phase enzyme immunoassay. Planta 153, 561–571. doi: 10.1007/BF00385542
Xu, Z., and Rothstein, S. J. (2018). ROS-induced anthocyanin production provides feedback protection by scavenging ROS and maintaining photosynthetic capacity in Arabidopsis. Plant Signal. Behav. 13, 1364–1377. doi: 10.1080/15592324.2018.1451708
Xu, Z., and Zhou, G. (2008). Responses of leaf stomatal density to water status and its relationship with photosynthesis in a grass. J. Exp. Bot. 59, 3317–3325. doi: 10.1093/jxb/ern185
Yadav, T., Kumar, A., Yadav, R., Yadav, G., Kumar, R., and Kushwaha, M. (2020). Salicylic acid and thiourea mitigate the salinity and drought stress on physiological traits governing yield in pearl millet-wheat. Saudi J. Biol. Sci. 27, 2010–2017. doi: 10.1016/j.sjbs.2020.06.030
Zafar, S., Akhtar, M., Perveen, S., Hasnain, Z., and Khalil, A. (2020). Attenuating the adverse aspects of water stress on wheat genotypes by foliar spray of melatonin and indole-3-acetic acid. Physiol. Mol. Biol. Plants 26, 1751–1762. doi: 10.1007/s12298-020-00855-6
Zafar, S., Hasnain, Z., Aslam, N., Mumtaz, S., Jaafar, H. Z., Wahab, P. E. M., et al. (2021). Impact of Zn nanoparticles synthesized via green and chemical approach on okra (Abelmoschus esculentus L.) growth under salt stress. Sustain. For. 13, 3694. doi: 10.3390/su13073694
Zahra, N., Mahmood, S., and Raza, Z. A. (2018). Salinity stress on various physiological and biochemical attributes of two distinct maize (Zea mays L.) genotypes. J. Plant Nutr. 41, 1368–1380. doi: 10.1080/01904167.2018.1452939
Zhang, X. (1992). “The measurement and mechanism of lipid peroxidation and SOD, POD and CAT activities in biological system,” in Research Ethodology of Crop Physiology (Beijing: Agriculture Press), 208–211.
Zhanwu, G., Jiayu, H., Chunsheng, M., Jixiang, L., Xiaoyu, L., Lidong, L., et al. (2014). Effects of saline and alkaline stresses on growth and physiological changes in oat (Avena sativa L.) seedlings. Not. Bot. Horti Agrobot. Cluj-Nap. 42, 357–362. doi: 10.15835/nbha4229441
Keywords: bio-fortification, hormones, osmolytes, nutrients, salinity stress, wheat, zinc
Citation: Chattha MU, Amjad T, Khan I, Nawaz M, Ali M, Chattha MB, Ali HM, Ghareeb RY, Abdelsalam NR, Azmat S, Barbanti L and Hassan MU (2022) Mulberry based zinc nano-particles mitigate salinity induced toxic effects and improve the grain yield and zinc bio-fortification of wheat by improving antioxidant activities, photosynthetic performance, and accumulation of osmolytes and hormones. Front. Plant Sci. 13:920570. doi: 10.3389/fpls.2022.920570
Received: 14 April 2022; Accepted: 24 June 2022;
Published: 27 September 2022.
Edited by:
Muhammad Azhar Nadeem, Sivas University of Science and Technology, TurkeyReviewed by:
Ali Raza, Fujian Agriculture and Forestry University, ChinaCopyright © 2022 Chattha, Amjad, Khan, Nawaz, Ali, Chattha, Ali, Ghareeb, Abdelsalam, Azmat, Barbanti and Hassan. This is an open-access article distributed under the terms of the Creative Commons Attribution License (CC BY). The use, distribution or reproduction in other forums is permitted, provided the original author(s) and the copyright owner(s) are credited and that the original publication in this journal is cited, in accordance with accepted academic practice. No use, distribution or reproduction is permitted which does not comply with these terms.
*Correspondence: Muhammad Nawaz, ZG1uYXdhekBrZnVlaXQuZWR1LnBr; Muhammad Umair Hassan, bXVoYXNzYW51YWZAZ21haWwuY29t
Disclaimer: All claims expressed in this article are solely those of the authors and do not necessarily represent those of their affiliated organizations, or those of the publisher, the editors and the reviewers. Any product that may be evaluated in this article or claim that may be made by its manufacturer is not guaranteed or endorsed by the publisher.
Research integrity at Frontiers
Learn more about the work of our research integrity team to safeguard the quality of each article we publish.