- 1College of Life Science, Northeast Forestry University, Harbin, China
- 2School of Forestry, Northeast Forestry University, Harbin, China
- 3School of Resources and Environmental Science, Northeast Agricultural University, Harbin, China
The application of Ericoid mycorrhizal (ErM) fungi is considered to be an important strategy for increasing plant yield and drought resistance. In this study, we isolated and identified two ErM fungi that can promote the growth of lingonberry. We tried to understand the potential of these two ErM fungi to promote the growth of lingonberry and the strategies to help plants cope with water shortage. The use value of ErM fungi was evaluated by inoculating Oidiodendron maius FC (OmFC) or Lachnum pygmaeum ZL6 (LpZL6), well-watered (WW) and severe drought stress (SDS). The results showed that the mycelium of LpZL6 was denser than that of OmFC, and both ErM fungi significantly increased the biomass of lingonberry stems and roots. They also significantly increased the chlorophyll content by 65.6 and 97.8%, respectively. In addition, inoculation with LpZL6 fungi can improve drought resistance, promote root growth and increase root wet weight by 1157.6%. Drought reduced the chlorophyll content and soluble sugar content of lingonberry but increased significantly after inoculation with LpZL6. Inoculation with LpZL6 decreased lingonberry’s malondialdehyde (MDA) content but increased the superoxide dismutase (SOD) activity. Overall, these results indicated that the successful coexistence of ErM fungi and lingonberry alleviated the adverse effects of drought stress through higher secondary metabolites and photosynthetic pigment synthesis.
Introduction
Lingonberry (Vaccinium vitis-idaea L.) is a low-shrub wild plant growing in the temperate and subtropical regions of the Northern Hemisphere. Its leaves and fruits have antibacterial, anticancer, and antioxidant effects (Lin et al., 2019). The berries are rich in vitamins (vitamins A, B, and C), calcium, magnesium, potassium, and phosphorus and have unique polyphenols and a large number of anthocyanins (Ek et al., 2006). Lingonberry juice not only has anti-inflammatory and anti-atherosclerotic properties (Kivimäki et al., 2012), but can also reduce blood sugar and lipids (Eid et al., 2014; Marungruang et al., 2020; Ryyti et al., 2020). Since Vaccinium plants usually lack root hairs, most plants are symbiotic with mycorrhizal fungi under natural conditions. The fungi associated with lingonberry are called Ericoid mycorrhizal (ErM) fungi (Lukesova et al., 2015). ErM fungi are beneficial microorganisms for the growth of lingonberry, which can promote the growth and nutrient absorption of blueberry and reduce the damage to plants under drought, heavy metals, and saline-alkali environments (Baba et al., 2021; Cai et al., 2021; Mu et al., 2021).
Ascomycota is the main fungus involved in the formation of ErM. Oidiodendron maius is a member of Leotiomycetes and has been isolated from Rhododendron fortunei (Wei et al., 2020). Rhododendron fortunei seedlings inoculated with the Oidiodendron maius strain produced abundant roots (Wei et al., 2016a), and significantly increased root and stem biomass (Wei et al., 2016b). Lachnum pygmaeum is a member of Pezizomycetes and has been isolated from Vaccinium corymbosum (Bizabani and Dames, 2015). Previous studies have shown that Lachnum sp. can form ErM fungi in plant roots, but it does not form a specific mycorrhizal structure. It is only characterized by mycelial infection in cortical cells, which cannot cause plant disease but can promote plant growth (Bizabani and Dames, 2015; Yang et al., 2018). However, another study showed that Lachnum pygamaeum and Lachnum virgineum formed a typical crimp ErM (Walker et al., 2011). This indicates that Oidiodendron maius and Lachnum spp. have the potential to form ErM structures, although their host adaptability and the environmental adaptability of plants have not been well proven.
Ericoid mycorrhizal plays an important role in promoting plant growth, which not only increases plant stem length and leaf area but also promotes plant root growth. The inoculation of ErM fungi can also alleviate the damage caused by environmental stress because it secretes secondary metabolites or produces specific symbiotic structures to help the host recruit beneficial bacteria and promote water absorption and plant growth and development (Cheng et al., 2021; El-Nashar et al., 2021; Sun et al., 2021). ErM plants have two reasons for their ability to effectively tolerate water deficit. First, fungal mycelia enter the plant root cells, and on the other hand, they have a wide area to absorb more water to plants (Smith et al., 2010; Doubková et al., 2013). Second, after the formation of ErM, fungi can promote plant physiological changes and limit the excessive production of reactive oxygen species (ROS) by signal transduction to enhance plant chlorophyll content, superoxide dismutase (SOD), catalase (CAT), peroxidase (POD), malondialdehyde (MDA) and antioxidant enzymes activity to reduce the damage of plant water deficit (Caravaca et al., 2005; Wu et al., 2014).
The economic benefit and ecological function bottleneck of lingonberry is due to the lack of ErM research (Daghino et al., 2016; Perotto et al., 2018; Vohník, 2020). In this paper, we reported the isolation and identification of mycorrhizal fungi from wild lingonberry in the Greater Khingan Range and analyzed the mycelium morphology and mycorrhiza structure after in vitro synthesis. In addition, we studied the effects of ErM inoculation on plant growth. Finally, we assessed the beneficial value of ErM fungi in helping lingonberry alleviate the water deficit.
Materials and Methods
Sample Collection, Isolation, and Pure Culture
The lingonberry was taken from The Greater Khingan Range, and the coordinates were 124°5′1″E, 52°2′14″N, Harbin, China (Figure 1). The samples were original undamaged woodlands, and the main tree species were Larix gmelinii, Betula platyphylla, Pinus sylvestris var. mongolica, Populus davidiana, etc. The main shrubs are Rhododendron dauricum, Ledum palustre var. dilatatum, Vaccinium vitis-idaea, etc. The main herbs are Cyperaceae, Compositae, Leguminosae, Rosaceae, etc., pH 4.85 ± 0.49. The soil layer has a very thick humus layer; the main litter pine needles and moss are widely distributed. The mean annual temperature is −4.3°C. The annual rainfall is 497.7 mm. Maximum temperature 32.1°C. Minimum temperature −52.0°C. The average annual temperature difference is 49°C. The sunshine duration is 2,052.1 h (Figure 1). Organic matter 105.96 ± 10.21 g kg–1, total phosphorus 1.09 ± 0.11 g kg–1, total nitrogen 11.30 ± 1.13 g kg–1, available phosphorus 73.64 ± 6.79 mg kg–1, available nitrogen 182.67 ± 15.68 mg kg–1 (Table 1).
The collected lingonberry root samples were stored at 4°C and sent back to the laboratory for mycorrhizal fungi isolation. The lingonberry roots were rinsed with flowing water for 5 min (Figure 2A) and then rinsed with sterilized water three times. Next, the washed roots were sterilized in 75% alcohol solution for 15 s and washed three times with sterilized water. Then, the roots were placed into 2% sodium hypochlorite disinfectant for 2 min and rinsed five times with sterilized water. The samples were dried on sterile filter paper in petri dishes for 10 min. The dried roots were cut into segments (approximately 5 mm long each) on PDA medium for 1 week at 25°C in darkness (Figure 2B). The fungus mycelium was separated and purified (Figures 2C,D). The structure of the mycelium was photographed by microscopy (OLYMPUS BX43) and scanning electron microscopy (HITACHI TM3030).
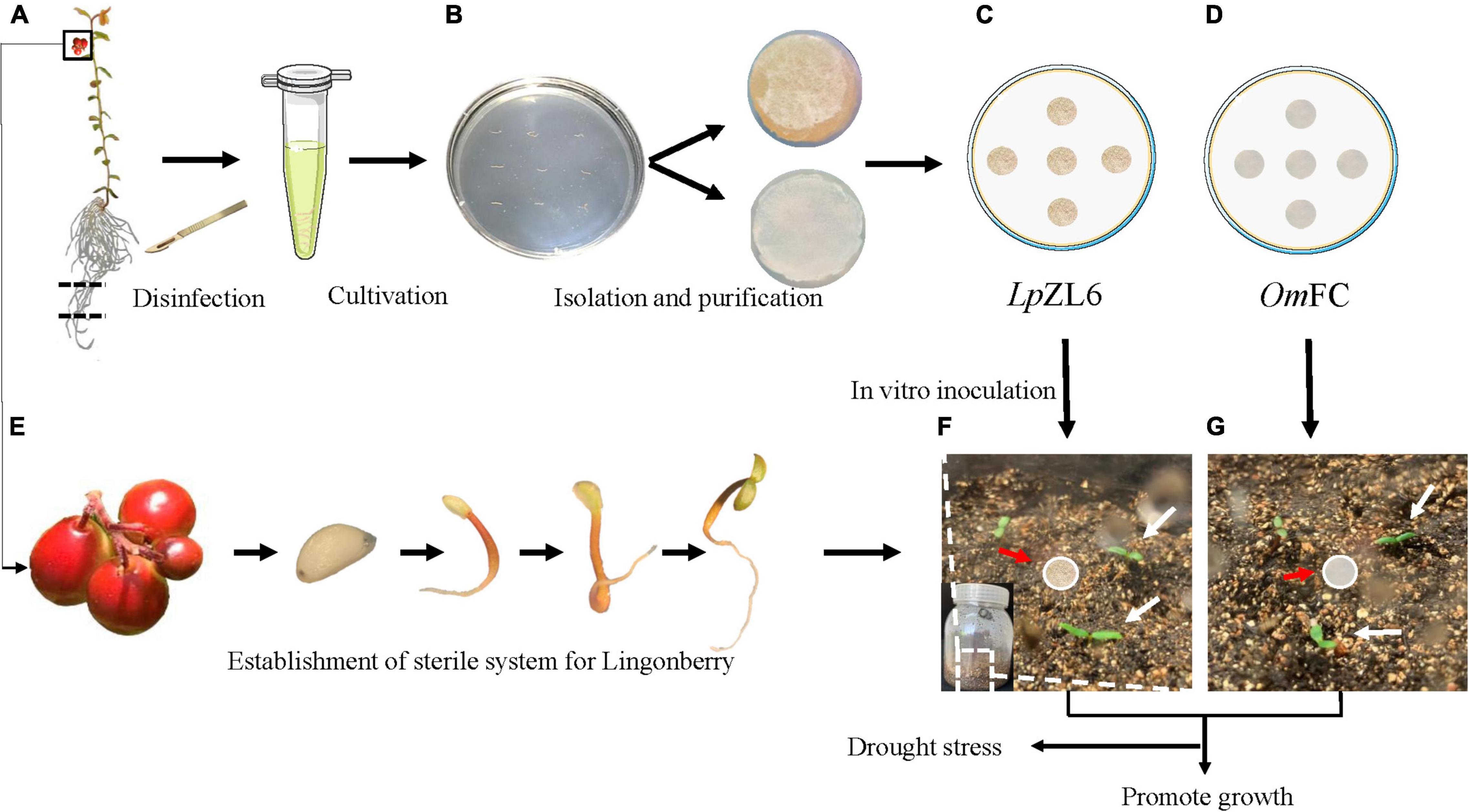
Figure 2. Obtaining aseptic seedlings of lingonberry and isolating ErM fungi. Disinfection of blueberry seedlings (A), isolation of ErM fungi (B), OmFC and LpZL6 fungi culture (C,D), obtain aseptic seedlings of lingonberry (E). Lingonberry inoculated with ErM fungi (F,G).
DNA Extraction, Sequencing and Phylogenetic Analysis
Total DNA was extracted from fungi by the CTAB method (Murray and Thompson, 1980). PCR used 2 μL DNA, equivalent to 50 ng total DNA, with a final volume of 20 μL. The 20 μL reaction system contained 1 μL of template DNA, 4 pmol of each primer ITS1 and ITS4, 2 μL of dNTPs (2.5 mM dATP, dTTP, dCTP, and dGTP), 2 μL of 10× TransTaq HiFi Buffer II, 0.2 μL of TransTaq HiFi DNA Polymerase (www.transgen.com.cn, product code: AP131) and enough ddH2O to reach a total volume of 20 μL. Primers ITS1 and ITS4 are shown in Supplementary Table 1. ITS PCR products were sent to Bosch Biotechnology Co., Ltd. (Harbin, China) for DNA sequencing. ITS sequences were compared with those in the NCBI database by BLAST. The phylogenetic tree based on the ITS sequence was constructed by using MEGA 7.0 software and maximum likelihood method analysis (Saitou and Nei, 1987). Sequence data can be obtained from the NCBI database and submitted to GenBank with accession numbers ON032317.1 (OmFC) and ON007175.1 (LpZL6).
Obtaining Aseptic Seedlings of Lingonberry and Synthesizing Mycorrhizae in vitro
Completely mature fruits were collected. The harvested lingonberry fruits were squeezed, and the mature seeds were stripped. The seeds were rinsed with clear water, dried, and placed in a 4°C refrigerator until use (Figure 2E). After a month, the seeds were sterilized in 75% alcohol solution for 30 seconds, washed three times with sterilized water, transferred to 2% sodium hypochlorite for 5 min, and washed five times with sterilized water. The samples were transferred to 1/2 MS medium supplemented with sucrose (30 g L–1) and agar (9 g L–1). The seedlings grew at low light intensity (30 μmol m–2s–1) in the culture room at 22 ± 2°C (Figure 2E). After 50 days, the lingonberry aseptic seedlings were planted in a sterile flask with soil: vermiculite = 2:1. The soil was put into the flask and cooled to room temperature after high-pressure sterilization. The seedlings were cultured at 22°C for two weeks under 16 h of light and 8 h of darkness for subsequent experiments (Figures 2F–J).
According to the method described by Wei et al. (2016a), in vitro mycorrhizal synthesis of LpZL6 and OmFC fungi and lingonberry was carried out and slightly modified. We used the staining method developed by Upson et al. to further investigate the morphology of hyphae curl and the identification of hyphae colonization in root cells (Upson et al., 2007). The simple step is to first clean the roots in FAA for 24 h. Then, the samples were rinsed with sterile water, soaked in 10% KOH solution for 60 min and kept in 90°C water. After removal, it was washed with distilled water until colorless, placed in 5% lactic acid solution for 4 min, and placed in trypan blue dye solution at room temperature overnight. Finally, the root segments were removed, soaked in glycerol lactate and observed under a microscope.
Study on the Growth-Promoting Activities of OmFC and LpZL6
The phosphorus utilization of fungi was measured by the P-V-Mo yellow colorimetric method. Fungi were cultured in an inorganic phosphorus liquid medium at 28°C for 5 days at 150 rpm. The supernatant fermentation broth (0.4 ml) was added to 2% citric acid solution (0.4 ml) and vanadium ammonium molybdate solution (4 ml), with deionized water volume to 10 ml after mixing. After standing at room temperature for 20 min, the absorbance of the sample at 456 nm wavelength was measured by UV spectrophotometer, and the content of available phosphorus in the fermentation broth was calculated according to the phosphorus standard curve. Fermentation broth samples were taken every 48 h, with three replicates per group.
The fungi were inoculated into liquid medium containing tryptophan and cultured at 28°C for 5 days at 150 rpm min–1. The indole-3-acetic acid (IAA) content in the fermentation broth was determined by Salkowski reagent. The operation was as follows: The supernatant was mixed with Salkowski reagent at a ratio of 1:2, and the sample was placed in the dark for 30 minutes. The absorbance of the sample at 530 nm was measured by a UV spectrophotometer, and the IAA content was calculated by an IAA standard curve. Fermentation broth samples were taken every 48 hours with 3 replicates per group.
Determination of Growth Parameters and Related Physiological Indexes of Lingonberry
Germ-free plants were grown in the culture room of Northeast Forestry University (45°43′45.71″ northern latitude, 126°38′11.04″ east longitude, Heilongjiang Province, China). The lingonberry was inoculated with OmFC, LpZL6, and no fungi in a germ-free tissue culture bottle. The inoculation process was to bury the activated fungus piece at 0.5 cm deep in the middle of the aseptic seedlings of lingonberry (Figures 2F,G). Drought stress experiments included well-watered (WW) 100% field capacity (FC), severe drought stress (SDS) 50% FC and inoculation of ErM fungi. The design included the following treatments: WW and plants without fungal inoculation, SDS and plants without fungal inoculation, SDS and inoculated ErM fungal plants and WW inoculated ErM fungal plants. The soil water content was examined every 2 days through soil quality change for 60 days. The seedlings grew in the culture room at 22 ± 2°C. The experiment was carried out in a 16/8-h light/dark mode under greenhouse conditions with a light intensity of 30 μmol m–2 s–1. Statistical analysis and physiological indexes were measured after 60 days.
Measurements of chlorophyll content were performed according to the method described by Lichtenthaler (1987), and photosynthetic pigments (carotenoids and chlorophyll) were extracted from fresh leaves with 80% acetone. After centrifugation at 6,000 rpm for 15 min, the absorbance of the supernatant was recorded by a spectrophotometer at 470,646 and 661 nm. Total soluble sugar (TSS) measurements were performed according to the method of Masuko et al. (2005). Dry leaves (0.1 g) were ground with 12.5 ml ethanol and centrifuged at 8000 rpm for 10 min. The TSS was estimated at 490 nm by the phenol-sulfate acid method using enzyme calibration (BioTek ELx808, United States). The TSS content was calculated by a standard curve. The proline content was measured by the ninhydrin colorimetric method described by Bates et al. (1973), and the absorbance at 520 nm was measured. The proline concentration was calculated using a standard curve.
Measurement of MDA content in leaves Referring to Velikova et al. (2000), 0.5 g leaves were ground into homogenate in 5 ml 0.1% (w v–1) trichloroacetic acid (TCA), centrifuged at 11,500 × g for 10 min. One milliliter supernatant was mixed with 4 ml thiobarbituric acid (TBA) reagent (0.5% TBA in 20% TCA). The samples were heated at 95°C for 30 min, cooled rapidly in an ice bath and centrifuged at 11,500 × g for 15 min. The amount of MDA-TBA complex was measured by spectrophotometry at 532 and 600 nm, and the MDA content was calculated. SOD activity was measured by the method of Monazzah et al. (2018) and Sairam et al. (2002). The reaction solution was composed of 40 mmol L–1 phosphate buffer (pH 7.8), 0.1 mmol L–1 EDTA, 75 lmol L–1 NBT, 2 lmol L–1 riboflavin, 13 mmol L–1 methionine, and tissue extract. The absorbance of the mixture was measured by a spectrophotometer at 560 nm. One unit of SOD activity was defined as the amount of enzyme required for 50% nitroblue tetrazole (NBT) photoreduction inhibition. SOD activity is expressed as the enzyme activity of fresh weight per gram leaf.
Statistical Analysis
All experiments were completely randomized and repeated four times. In the weight measurement, the weight of 10 plants was counted randomly. The root and bud were separated, and the bud fresh weight (FW) was immediately reported. Then, the dry weight (DW) of branches was measured after drying in an oven (70°C, 48 h). We conducted a t-test to determine the significant difference (p < 0.05 or p < 0.01, depending on the experiment). In root length measurements, we used six plants for counting. SPSS software v 19.0 was used to analyze the data.
Results
Colony Morphology, Systematic Development and Mycorrhizal Formation
In this study, two mycorrhizal fungi were isolated and purified from the roots of lingonberry in The Greater Khingan Range, and the colony morphology after isolation and purification was consistent with the identification results. The OmFC was irregular in shape and gradually changed from white to gray-brown dull, and intact in the early stage. No aerial mycelium was produced within two weeks. LpZL6 showed regular white mycelium, no luster, transparent, and dry texture, and the edge of the mycelium was grainy (Figures 3A,B). Scanning electron microscopy showed that LpZL6 mycelium was denser than OmFC (Figure 3C). The phylogenetic tree showed that OmFC belonged to the Oidiodendron maius evolutionary branch (NCBI accession number: ON032317.1), while LpZL6 belonged to Lachnum spp. evolutionary branch (NCBI accession number: ON007175.1). However, several characteristics between the two branches were different (Figure 3D). LpZL6 grew slowly and formed regular colonies and was 10.43 ± 0.17 mm 7 day–1 on PDA medium. In contrast, OmFC grew faster and formed irregular colonies and was 19.76 ± 2.81 mm 7 day–1 on PDA medium.
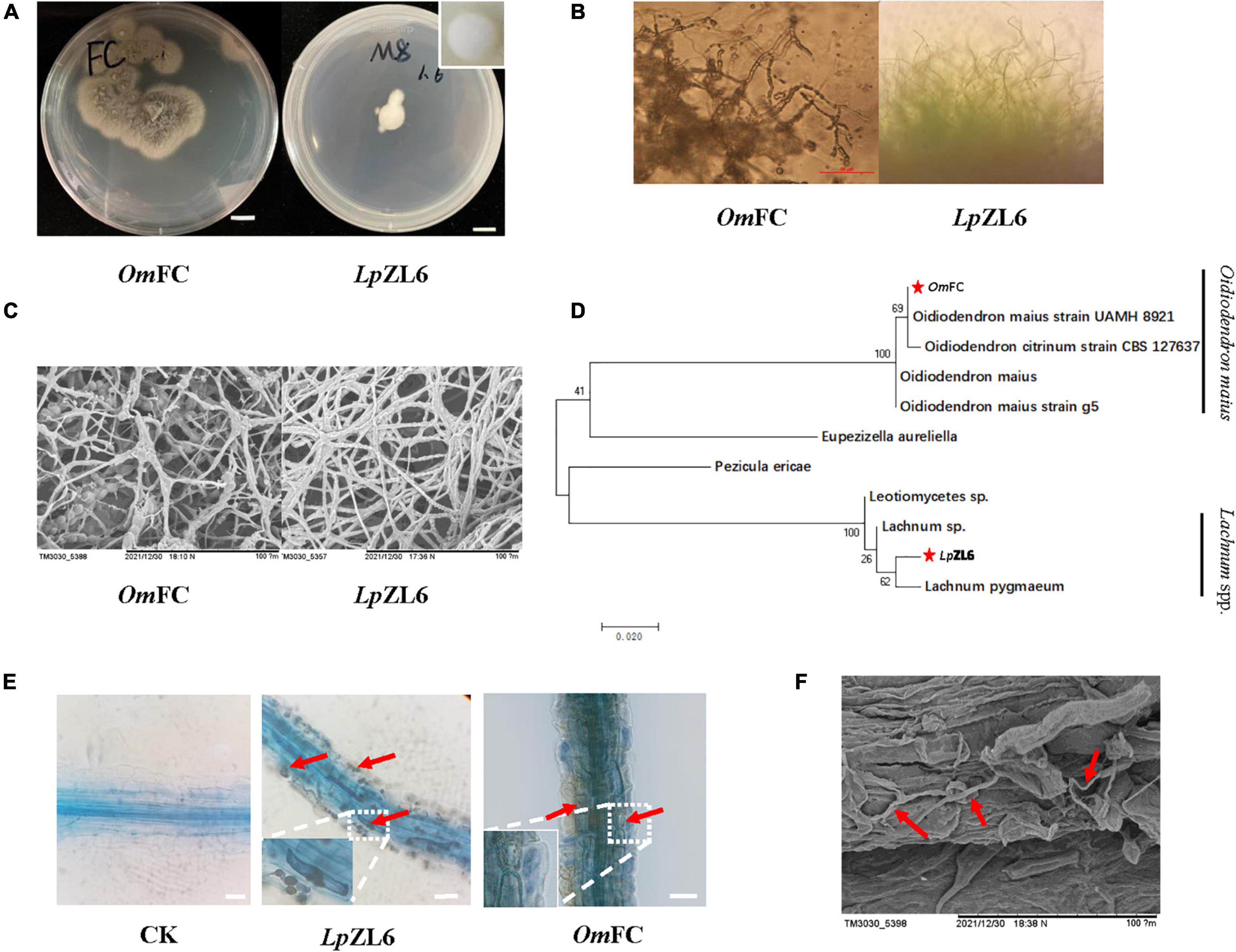
Figure 3. Fungi morphology and mycorrhizal synthesis. Identification of ErM fungi (A–C), phylogenetic analysis of ErM fungi (D), and ErM fungi inoculated plant root (E,F).
OmFC and LpZL6 were inoculated with lingonberry and were healthy before plant measurement. Lingonberry without fungal inoculation showed no fungal structure. All fungi colonized the fine roots of lingonberry seedlings and formed hyphae of typical ErM mycorrhiza fungi in important root cells (Figure 3E). The results showed that OmFC and LpZL6 successfully colonized the roots of lingonberry. Scanning electron microscopy showed hyphal colonization in the roots of lingonberry (Figure 3F).
OmFC and LpZL6 Promote Plant Growth
The growth test of lingonberry that evaluated the benefits of ErM fungal inoculation revealed significant differences between individual groups. Compared with no fungi inoculation, it has higher stemmed, more lush leaves and greener colors, OmFC and LpZL6 significantly increased the biomass of lingonberry (Figure 4A). It has higher stemmed, more lush leaves and greener colors. However, plants inoculated with OmFC (4.18 ± 1.39 cm) performed better than those inoculated with LpZL6 (3.45 ± 0.2 cm), and the stem length increased by 54.8 and 27.8% compared with WT, respectively (Figure 4C). The chlorophyll content of lingonberry inoculated with OmFC (1.78 ± 0.05 mg g–1) and LpZL6 (1.49 ± 0.06 mg g–1) increased by 97.8 and 65.6% compared with WT, respectively (Figure 4B). This confirms that the fungi OmFC and LpZL6 make lingonberry beneficial fungi and promote growth and chlorophyll accumulation.
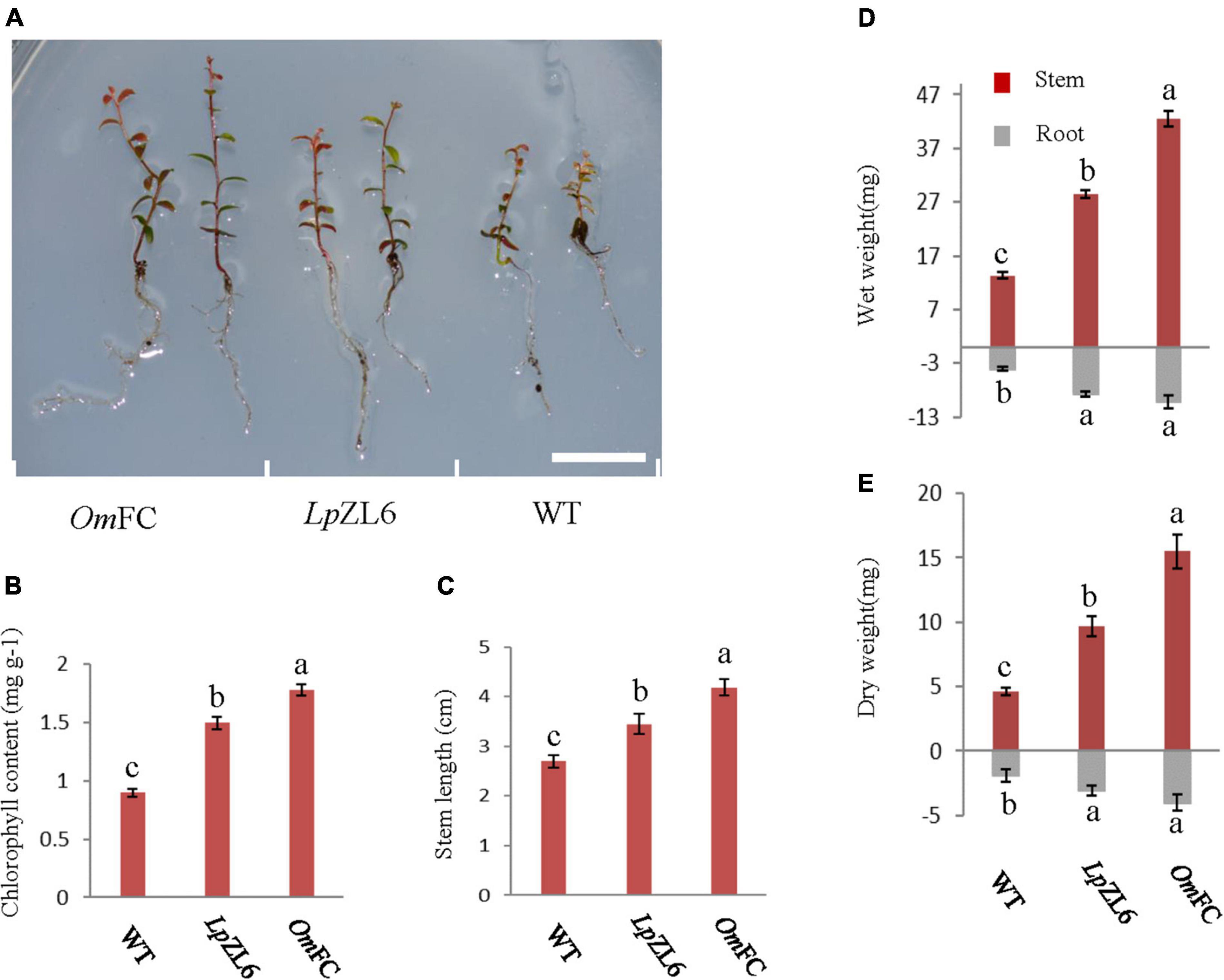
Figure 4. OmFC and LpZL6 promote plant growth. Inoculated OmFC and LpZL6 to lingonberry seedlings (A). Inoculated OmFC and LpZL6 fungal chlorophyll content changes (B). Changes in stem length of inoculated OmFC and LpZL6 fungi (C). Inoculation with OmFC and LpZL6 increased the wet and dry weight of lingonberry stems and roots (D,E). (Scale bar in a = 1 cm).
ErM fungi were inoculated into the roots of lingonberry, and the results showed that OmFC and LpZL6 could significantly promote the biomass of lingonberry plants. The stem wet weight of lingonberry inoculated with OmFC (42.53 ± 0.17 mg) and LpZL6 (28.56 ± 0.76 mg) increased by 217.8 and 113.5%, respectively, compared with that of lingonberry without inoculation. The stem dry weight of lingonberry inoculated with OmFC (15.5 ± 1.29 mg) and LpZL6 (9.71 ± 0.78 mg) increased by 236.2 and 110.6%, respectively, compared with that of lingonberry without inoculation (Figure 4D). Inoculation with ErM fungi not only increased the biomass of aboveground stems but also increased the biomass of roots. lingonberry had more and longer roots. The root wet weight of lingonberry inoculated with OmFC (10.26 ± 1.18 mg) and LpZL6 (8.75 ± 0.49 mg) increased by 154.6 and 117.1%, respectively, compared with that of non-inoculated fungi. The root dry weight of lingonberry inoculated with OmFC and LpZL6 was 113.2 and 61.9% higher than that of non-inoculated fungi, respectively (Figure 4E). These results indicated that inoculation with OmFC and LpZL6 promoted the accumulation of wet and dry weight of lingonberry.
OmFC and LpZL6 Have the Characteristics of Producing IAA and Phosphate Solubilization
Studies suggest that the ability of fungi to produce IAA and phosphate solubilization is positively correlated with plant growth. To explore the growth-promoting ability of fungi, we analyzed IAA content and phosphate solubilization in mycelia of OmFC and LpZL6. The results showed that phosphate solubilization increased gradually from the first day to the eleventh day after inoculation with LpZL6, while phosphate solubilization first decreased then increased after inoculation with OmFC (Figure 5A). The IAA content produced by LpZL6 inoculation reached a high peak on the third day but began to decline on the fifth day and then gradually increased. IAA increased gradually from the first day to the eleventh day after inoculation with OmFC fungi (Figure 5B). OmFC and LpZL6 no significant difference in phosphate solubility.
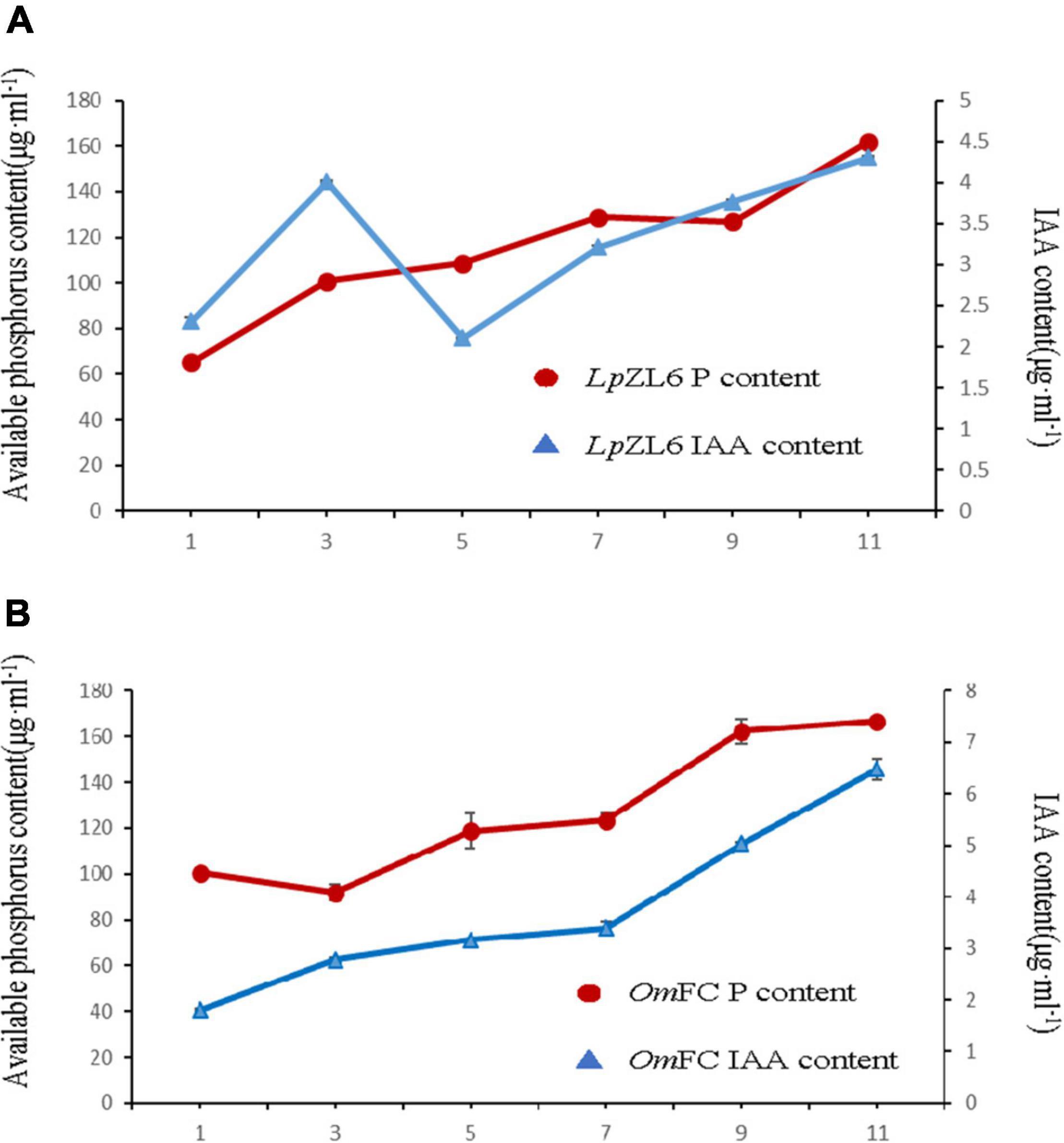
Figure 5. OmFC and LpZL6 have the characteristics of producing IAA and phosphate solubilization. 0–11 days LpZL6 to producing IAA and phosphate solubilization (A). 0–11 days OmFC to producing IAA and phosphate solubilization (B).
Inoculation With LpZL6 Promoted the Growth of Lingonberry and Alleviated Drought Stress Damage
Under drought stress, the biomass of roots and stems of lingonberry plants inoculated with ErM fungi increased compared with those of non-inoculated plants (Figure 6A). The stem wet weight (6.17 ± 0.45 mg) and root wet weight (7.42 ± 0.63 mg) of lingonberry inoculated with LpZL6 were increased by 32.1% and 1157.6%, respectively (Figure 6B). Interestingly, the development of inoculated LpZL6 fungal roots is exciting. Water deficit stress not only significantly reduced the fresh weight of stems and roots but also reduced the dry weight of stems and roots (p < 0.001). SDS reduced stem dry weight and root dry weight by 8.6 and 741.7%, respectively, compared with WW (Figure 6C), and non-inoculated ErM fungi lingonberry seedlings even died directly. These results showed that under the condition of water deficit, inoculation with LpZL6 fungi could not only alleviate the damage caused by drought stress on lingonberry seedlings but also significantly promote the growth and development of roots. The above results indicated that inoculation with LpZL6 fungi could promote root development and alleviate the damage caused by the water deficit in lingonberry. However, OmFC did not alleviate the water deficit.
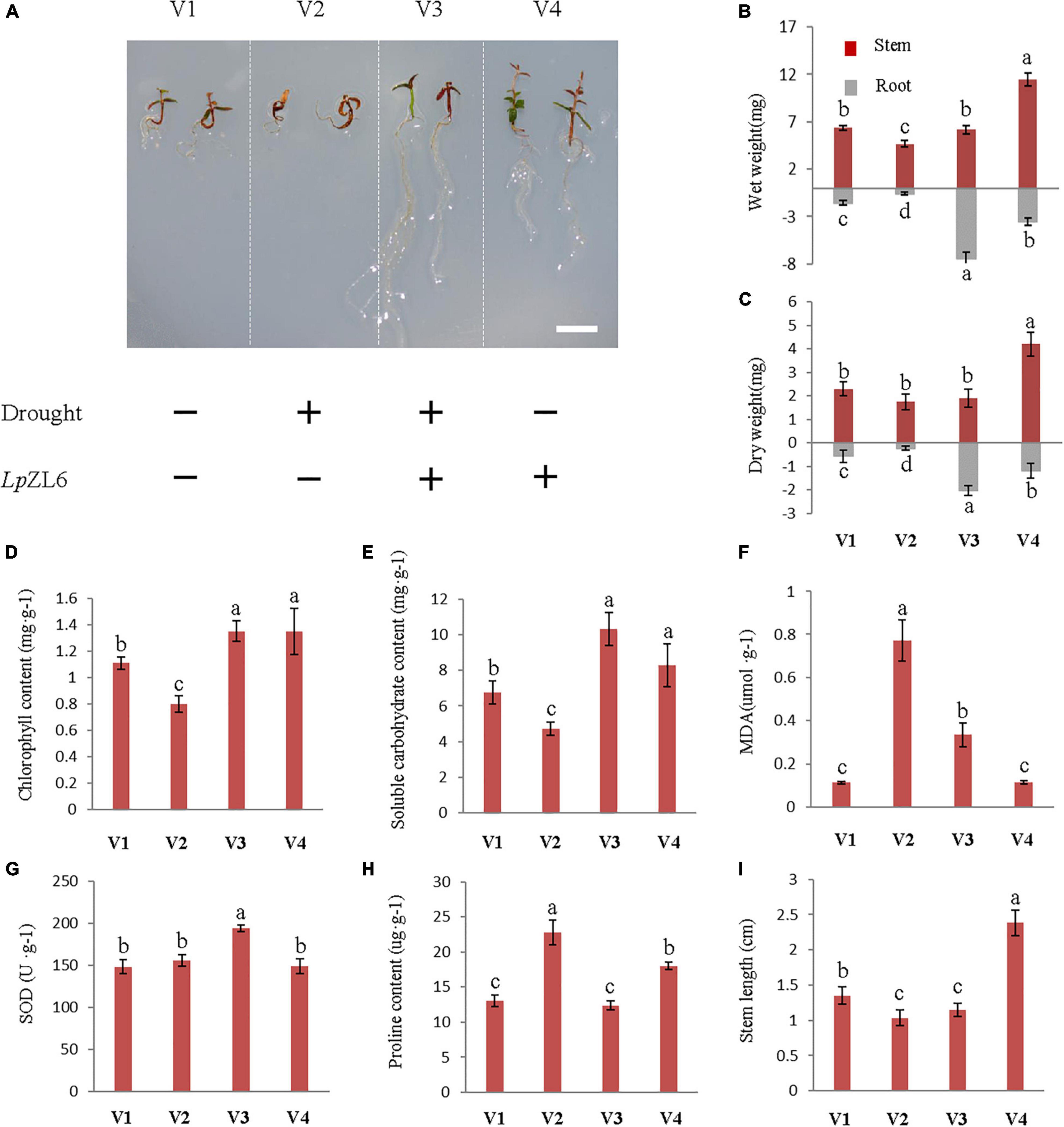
Figure 6. Inoculation with LpZL6 promoted the growth of lingonberry and alleviated drought stress damage. V1: Wild type; V2: + Drought; V3: + Drought + LpZL6; V4: + LpZL6. Drought stress inoculated with LpZL6 lingonberry seedlings (A). Drought stress inoculated with LpZL6 fungi increased the wet weight and dry weight of lingonberry stems and roots (B,C). Changes in chlorophyll content of LpZL6 inoculated fungi (D). Changes in TSS content of LpZL6 inoculated fungi (E). Changes in MDA content of LpZL6 inoculated fungi (F). Changes in SOD activity of LpZL6 inoculated fungi (G). Changes of proline content in LpZL6 fungi (H). Changes of stem length of LpZL6 inoculated fungi (I). [Scale bar in panel (A) = 0.5 cm].
Statistical analysis showed that drought stress significantly affected the chlorophyll content (p < 0.001). Compared with WW, the total chlorophyll concentration of non-inoculated plants (0.8 ± 0.06 mg g–1 FW) grown under SDS decreased by 38.8%, but inoculation with LpZL6 fungi (1.35 ± 0.08 mg g–1 FW) increased by 21.6% (Figure 6D). This indicated that the symbiosis between lingonberry and LpZL6 fungi alleviated the negative impact of drought stress. Variance analysis showed that under drought stress, LpZL6 inoculation and non-inoculation had significant effects on proline and TSS content (Figures 6E,H). The proline content of LpZL6-inoculated plants was significantly lower than that of non-inoculated plants. Under drought stress, the proline content of lingonberry inoculated with LpZL6 fungi decreased by 84.1% compared with the control. In contrast, the TSS content was significantly increased by 119.5% compared with non-inoculated LpZL6 fungi (Figure 6H).
In this study, LpZL6 fungal inoculation significantly increased TSS in lingonberry leaves (Figure 6E) by 119.5% under drought stress. Therefore, water deficiency can lead to the accumulation of soluble carbohydrates in plants. Under drought stress, compared with non-inoculated fungi, the MDA content of LpZL6 fungi decreased by 57.1% (Figure 6F). Mycorrhizal inoculation led to an increase in SOD activity in plant tissues, which was 19.8% higher than that without LpZL6 inoculation (Figure 6G).
Discussion
Vaccinium berries are widely praised worldwide for their medical value. Different countries have conducted systematic investigations on lingonberry berries, such as the United States (Grace et al., 2014), Canada (Kalt et al., 2008), Italy (Prencipe et al., 2014), and Finland (Latti et al., 2011). The results showed that wild lingonberry berries obviously had higher anthocyanin content and antioxidant activity than their varieties (Feng et al., 2016). Our experimental material lingonberry (Figure 1) is a red berry, also known as mountain cranberry, cowberry. Sweet and sour taste, leaves and fruits can treat urethritis, cystitis, enteritis, dysentery and other diseases (Kowalska, 2021; Parnanen et al., 2021; Rohrl et al., 2021). These berries are mainly developed and utilized in the United States and European countries. In contrast, there are many kinds of wild berry resources in China, and there are few reports. The natural distribution area of wild berries in Northeast China is the largest, approximately 15 million hectares, with the largest number of berries, especially Rhododendron. In addition, most of these berries are medicinal species (Feng et al., 2016). Therefore, research on ErM fungi and lingonberry roots can develop the cultivation ability of wild lingonberry in Northeast China on a large scale and improve the economic benefits of lingonberry.
Lingonberry mycorrhizal fungi are usually diverse. Studies have shown that Lachnum spp. is the dominant species in specific habitats, and specific colonization occurs on a certain type of plant (Korkama-Rajala et al., 2008). By estimating the diversity of mycorrhizal fungi in two herbaceous plants, two ascomycetes, Hymenoscyphus sp. and Lachnum sp. without mycorrhizal structure were found in mycorrhizal fungi, indicating that Lachnum spp. may also coexist with mycorrhizal fungi (Gao and Yang, 2010). Other studies have found that Lachnum sp. can colonize the root but does not form any specific mycorrhizal structure. Only mycelial infection in cortical cells is characterized by intracellular and intercellular colonization, which has no pathogenic effect on plants and can promote the growth of plant branches (Bizabani and Dames, 2015). However, another study found that Lachnum sp. was symbiotic with other ErM fungi in blueberry plants by high-throughput sequencing technology, and it was classified as a mycorrhizal fungus, suggesting that it may play a role in the colonization of mycorrhizal fungi (Yang et al., 2018). Oidiodendron maius fungi not only promote plant growth but also induce a large number of root developments. The mechanism is that Oidiodendron maius fungi can produce high concentrations of Trp (tryptophan) for IAA synthesis through IPA (indole-3-pyruvate) and IAA to promote mycelium growth. IAA may also induce adventitious root formation in micro-cutting and promote IAA production in plants (Wei et al., 2020). Our results showed that OmFC and LpZL6 colonized the roots of lingonberry and formed mycorrhizal structures (Figure 3E).
After ErM inoculation, the growth status and chlorophyll content of lingonberry changed (Sharma et al., 2020; Baba and Hirose, 2021). Studies have shown that the interaction between mycorrhizal fungi and plants occurs through the secretion of secondary metabolites or the formation of specific symbiotic structures to help the host recruit beneficial colonies and promote water absorption and plant growth and development (Cheng et al., 2021; El-Nashar et al., 2021; Sun et al., 2021). This was the same as our results. After inoculation with OmFC and LpZL6, the stem and root biomass of lingonberry increased significantly. However, plants can also balance endophytic fungi through nutritional distribution, secretion of specific substances and other ways to prevent their excessive proliferation (Liu et al., 2020).
Under drought stress, mycorrhizal fungi can assist plants in coping with water shortage environments, enhance plant water and nutrient absorption, and promote photosynthesis and transpiration (Amiri et al., 2017; Grelet et al., 2017; Ghanbarzadeh et al., 2019). The activation of the plant antioxidative stress system reduces oxidative damage, maintains plant cell stability, promotes plant uptake of nitrogen, phosphorus and other elements, and helps plants resist drought and soil pathogens (Abdel-Salam et al., 2018; Li et al., 2019). This is highly consistent with our results. It is exciting that under drought stress, LpZL6 inoculation can significantly increase the growth of lingonberry roots, up to 1157.6% (Figure 6B). This result is consistent with in Rhododendron fortunei; ErM fungi can significantly increase root biomass (Wei et al., 2020).
This study showed that water stress reduced growth parameters (Figure 7). This may be due to water and nutrient absorption and a decrease in plant photosynthetic capacity (Quiroga et al., 2018). Our results showed that inoculation of lingonberry with LpZL6 improved plant growth parameters under drought stress (Figure 6A). This may be because LpZL6 fungi provide more water for plants by expanding hyphae and developing roots (Figure 7), resulting in more dry matter production and accumulation in plants (Attarzadeh et al., 2019; Khajeeyan et al., 2019).
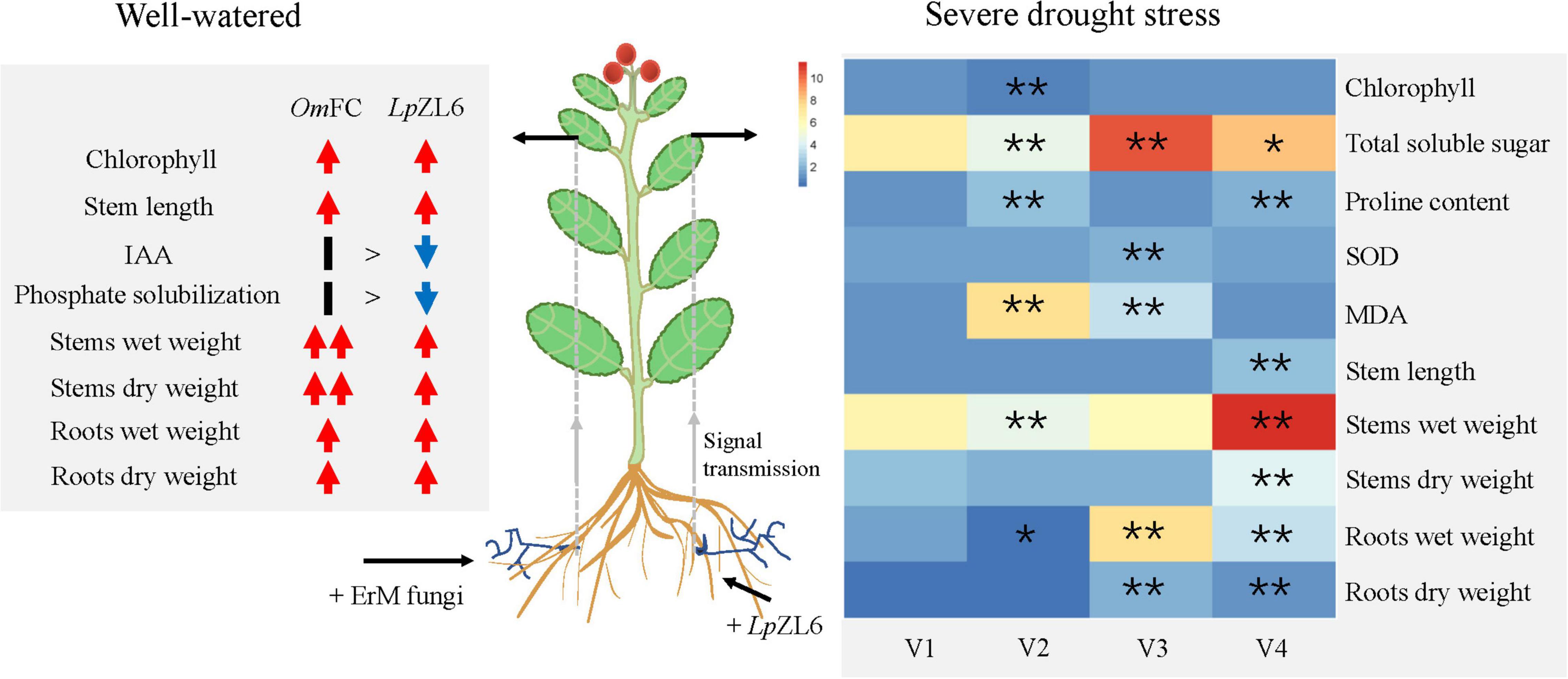
Figure 7. The mechanism of promoting plant growth and alleviating drought stress damage in lingonberry inoculated with ErM fungi. (Left) Inoculation with OmFC and LpZL6 increased chlorophyll content, stem and root weight. (Right) Inoculation LpZL6 increased the chlorophyll content, TSS content, and SOD activity of plants through colonization of plant roots and signal transduction, and decreased the proline content and MDA content to alleviate the damage of drought stress on plants. V1: Wild type; V2: + Drought; V3: + Drought + LpZL6; V4: + LpZL6.
Water deficiency stress can reduce the growth of plants and affect the biochemical and physiological processes of plants. In this study, water deficiency led to the accumulation of TSS in plants, which was due to the increased photosynthate and free carbohydrates (Lee et al., 2008). The improvement of TSS in lingonberry may be attributed to increased carbon fixation and enzyme activation (Figure 7). Our study showed that in plants under drought stress, inoculation with LpZL6 fungi could increase chlorophyll content and photosynthetic activity, thus increasing the content of TSS (Byrne and Mitchell, 2004; Lin et al., 2011; Asrar et al., 2012). Drought-resistant plants can maintain more chlorophyll content under water stress, which is consistent with our experimental results (Fang and Xiong, 2015). Under the condition of water shortage, the chlorophyll content of lingonberry leaves increased, and light energy was used more effectively (Figure 7).
Plants inoculated with LpZL6 showed reduced proline accumulation in response to severe drought stress (Figure 6H). This was consistent with the results of Ghanbarzadeh et al., where proline content in uninoculated leaves increased significantly under drought stress, while proline accumulation in inoculated fungal plants decreased (Ghanbarzadeh et al., 2020). To protect plants from potential damage under water shortage conditions, proline can become an effective scavenger of ROS to protect the plant cell membrane and other structures from damage (Rapparini and Peñuelas, 2014). In our study, LpZL6 inoculation reduced the MDA content of lingonberry under drought stress but increased the SOD activity, which had the same results in the study of geranium (Amiri et al., 2015). The above results showed that mycorrhizal fungi could help plants resist water stress by changing the physiological and biochemical processes of plants (Figure 7).
Data Availability Statement
The datasets presented in this study can be found in online repositories. The names of the repository/repositories and accession number(s) can be found in the article/Supplementary Material.
Author Contributions
HL, LS, and JZ designed the experiments and wrote the manuscript. HL, CG, and BF analyzed these data. Others participated in the experiments. All authors read and approved the final manuscript.
Funding
This work was supported by the National Science Foundation of China (32071777).
Conflict of Interest
The authors declare that the research was conducted in the absence of any commercial or financial relationships that could be construed as a potential conflict of interest.
The reviewer AW declared a shared affiliation with the author HS to the handling editor at the time of review.
Publisher’s Note
All claims expressed in this article are solely those of the authors and do not necessarily represent those of their affiliated organizations, or those of the publisher, the editors and the reviewers. Any product that may be evaluated in this article, or claim that may be made by its manufacturer, is not guaranteed or endorsed by the publisher.
Acknowledgments
We thank Zhili Liu, Huiying Cai, Manyi Bao, and Jili Zhang for help in the field sampling and laboratory analyses. Some material comes from smart (https://smart.servier.com).
Supplementary Material
The Supplementary Material for this article can be found online at: https://www.frontiersin.org/articles/10.3389/fpls.2022.920338/full#supplementary-material
References
Abdel-Salam, E., Alatar, A., and El-Sheikh, M. A. (2018). Inoculation with arbuscular mycorrhizal fungi alleviates harmful effects of drought stress on damask rose. Saudi J. Biol. Sci. 25, 1772–1780. doi: 10.1016/j.sjbs.2017.10.015
Amiri, R., Nikbakht, A., and Etemadi, N. (2015). Alleviation of drought stress on rose geranium [Pelargonium graveolens (L.) Herit.] in terms of antioxidant activity and secondary metabolites by mycorrhizal inoculation. Sci. Hortic. 197, 373–380.
Amiri, R., Nikbakht, A., Rahimmalek, M., and Hosseini, H. (2017). Variation in the Essential Oil Composition, Antioxidant Capacity, and Physiological Characteristics of Pelargonium graveolens L. Inoculated with Two Species of Mycorrhizal Fungi Under Water Deficit Conditions. J. Plant Growth Regul. 36, 502–515.
Asrar, A. A., Abdel-Fattah, G. M., and Elhindi, K. M. (2012). Improving growth, flower yield, and water relations of snapdragon (Antirhinum majus L.) plants grown under well-watered and water-stress conditions using arbuscular mycorrhizal fungi. Photosynthetica 50, 305–316.
Attarzadeh, M., Balouchi, H., Rajaie, M., Movahhedi Dehnavi, M., and Salehi, A. (2019). Improvement of Echinacea purpurea performance by integration of phosphorus with soil microorganisms under different irrigation regimes. Agric. Water Manag. 221, 238–247.
Baba, T., and Hirose, D. (2021). Slow-growing fungi belonging to the unnamed lineage in Chaetothyriomycetidae form hyphal coils in vital ericaceous rhizodermal cells in vitro. Fungal Biol. 125, 1026–1035. doi: 10.1016/j.funbio.2021.07.003
Baba, T., Janošík, L., Koukol, O., and Hirose, D. (2021). Genetic variations and in vitro root-colonizing ability for an ericaceous host in Sarcoleotia globosa (Geoglossomycetes). Fungal Biol. 125, 971–979. doi: 10.1016/j.funbio.2021.08.005
Bates, L. S., Waldren, R. P., and Teare, I. D. (1973). Rapid determination of free proline for water-stress studies. Plant Soil 39, 205–207.
Bizabani, C., and Dames, J. (2015). Effects of inoculating Lachnum and Cadophora isolates on the growth of Vaccinium corymbosum. Microbiol. Res. 181, 68–74. doi: 10.1016/j.micres.2015.08.005
Byrne, K., and Mitchell, D. T. (2004). Responses of mycorrhizal and non-mycorrhizal Erica cinerea and Vaccinium macrocarpon to Glomus mosseae. Mycorrhiza 14, 31–36. doi: 10.1007/s00572-003-0273-2
Cai, B., Vancov, T., Si, H., Yang, W., Tong, K., Chen, W., et al. (2021). Isolation and Characterization of Endomycorrhizal Fungi Associated with Growth Promotion of Blueberry Plants. J. Fungi 7:584. doi: 10.3390/jof7080584
Caravaca, F., Alguacil, M. M., and Hernandez, J. (2005). Involvement of antioxidant enzyme and nitrate reductase activities during water stress and recovery of mycorrhizal Myrtus communis and Phillyrea angustifolia plants. Plant Sci. 169, 191–197.
Cheng, H.-Q., Giri, B., Wu, Q.-S., Zou, Y.-N., and Kuèa, K. (2021). Arbuscular mycorrhizal fungi mitigate drought stress in citrus by modulating root microenvironment. Arch. Agron. Soil Sci. [Epub ahead of print]. doi: 10.1080/03650340.2021.1878497
Daghino, S., Martino, E., and Perotto, S. (2016). Model systems to unravel the molecular mechanisms of heavy metal tolerance in the ericoid mycorrhizal symbiosis. Mycorrhiza 26, 263–274. doi: 10.1007/s00572-015-0675-y
Doubková, P., Vlasáková, E., and Sudová, R. (2013). Arbuscular mycorrhizal symbiosis alleviates drought stress imposed on Knautia arvensis plants in serpentine soil. Plant Soil 370, 149–161.
Eid, H. M., Ouchfoun, M., Brault, A., Vallerand, D., Musallam, L., Arnason, J. T., et al. (2014). Lingonberry (Vaccinium vitis-idaea L.) Exhibits Antidiabetic Activities in a Mouse Model of Diet-Induced Obesity. Evid. Based Complement. Altern. Med. 2014:645812. doi: 10.1155/2014/645812
Ek, S., Kartimo, H., Mattila, S., and Tolonen, A. (2006). Characterization of phenolic compounds from lingonberry (Vaccinium vitis-idaea). J. Agric. Food Chem. 54, 9834–9842. doi: 10.1021/jf0623687
El-Nashar, Y. I., Hassan, B. A., and Aboelsaadat, E. M. (2021). Response of Nemesia (Nemesia × hybridus) plants to different irrigation water sources and arbuscular mycorrhizal fungi inoculation. Agric. Water Manag. 243:106416.
Fang, Y., and Xiong, L. (2015). General mechanisms of drought response and their application in drought resistance improvement in plants. Cell. Mol. Life Sci. 72, 673–689. doi: 10.1007/s00018-014-1767-0
Feng, C., Su, S., Wang, L., Wu, J., Tang, Z., Xu, Y., et al. (2016). Antioxidant capacities and anthocyanin characteristics of the black-red wild berries obtained in Northeast China. Food Chem. 204, 150–158. doi: 10.1016/j.foodchem.2016.02.122
Gao, Q., and Yang, Z. L. (2010). Ectomycorrhizal fungi associated with two species of Kobresia in an alpine meadow in the eastern Himalaya. Mycorrhiza 20, 281–287. doi: 10.1007/s00572-009-0287-5
Ghanbarzadeh, Z., Mohsenzadeh, S., Rowshan, V., and Moradshahi, A. (2019). Evaluation of the growth, essential oil composition and antioxidant activity of Dracocephalum moldavica under water deficit stress and symbiosis with Claroideoglomus etunicatum and Micrococcus yunnanensis. Sci. Hortic. 256:108652.
Ghanbarzadeh, Z., Mohsenzadeh, S., Rowshan, V., and Zarei, M. (2020). Mitigation of water deficit stress in Dracocephalum moldavica by symbiotic association with soil microorganisms. Sci. Hortic. 272:109549.
Grace, M. H., Esposito, D., Dunlap, K. L., and Lila, M. A. (2014). Comparative analysis of phenolic content and profile, antioxidant capacity, and anti-inflammatory bioactivity in wild Alaskan and commercial Vaccinium berries. J. Agric. Food Chem. 62, 4007–4017. doi: 10.1021/jf403810y
Grelet, G.-A., Ba, R., Goeke, D. F., Houliston, G. J., Taylor, A. F. S., and Durall, D. M. (2017). A plant growth-promoting symbiosis between Mycena galopus and Vaccinium corymbosum seedlings. Mycorrhiza 27, 831–839. doi: 10.1007/s00572-017-0797-5
Kalt, W., MacKinnon, S., McDonald, J., Vinqvist, M., Craft, C., and Howell, A. (2008). Phenolics of Vaccinium berries and other fruit crops. J. Sci. Food Agric. 88, 68–76.
Khajeeyan, R., Salehi, A., Dehnavi, M. M., Farajee, H., and Kohanmoo, M. A. (2019). Physiological and yield responses of Aloe vera plant to biofertilizers under different irrigation regimes. Agric. Water Manag. 225:105768.
Kivimäki, A. S., Ehlers, P. I., Siltari, A., Turpeinen, A. M., Vapaatalo, H., and Korpela, R. (2012). Lingonberry, cranberry and blackcurrant juices affect mRNA expressions of inflammatory and atherothrombotic markers of SHR in a long-term treatment. J. Funct. Foods 4, 496–503.
Korkama-Rajala, T., Muller, M. M., and Pennanen, T. (2008). Decomposition and fungi of needle litter from slow- and fast-growing Norway spruce (Picea abies) clones. Microb. Ecol. 56, 76–89. doi: 10.1007/s00248-007-9326-y
Kowalska, K. (2021). Lingonberry (Vaccinium vitis-idaea L.) Fruit as a Source of Bioactive Compounds with Health-Promoting Effects-A Review. Int. J. Mol. Sci. 22:5126. doi: 10.3390/ijms22105126
Latti, A. K., Riihinen, K. R., and Jaakola, L. (2011). Phenolic compounds in berries and flowers of a natural hybrid between bilberry and lingonberry (Vaccinium x intermedium Ruthe). Phytochemistry 72, 810–815. doi: 10.1016/j.phytochem.2011.02.015
Lee, B. R., Jin, Y. L., Jung, W. J., Avice, J. C., Morvan-Bertrand, A., Ourry, A., et al. (2008). Water-deficit accumulates sugars by starch degradation–not by de novo synthesis-in white clover leaves (Trifolium repens). Physiol. Plant 134, 403–411. doi: 10.1111/j.1399-3054.2008.01156.x
Li, J., Meng, B., Chai, H., Yang, X., Song, W., Li, S., et al. (2019). Arbuscular Mycorrhizal Fungi Alleviate Drought Stress in C3 (Leymus chinensis) and C4 (Hemarthria altissima) Grasses via Altering Antioxidant Enzyme Activities and Photosynthesis. Front. Plant Sci. 10:499. doi: 10.3389/fpls.2019.00499
Lichtenthaler, H. K. (1987). Chlorophylls and carotenoids: pigments of photosynthetic biomembranes. Methods Enzymol. 148, 350–382. doi: 10.1515/znc-2001-11-1225
Lin, L.-C., Lee, M.-J., and Chen, J.-L. (2011). Decomposition of organic matter by the ericoid mycorrhizal endophytes of Formosan rhododendron (Rhododendron formosanum Hemsl.). Mycorrhiza 21, 331–339. doi: 10.1007/s00572-010-0342-2
Lin, L. C., Ye, Y. S., and Lin, W. R. (2019). Characteristics of root-cultivable endophytic fungi from Rhododendron ovatum Planch. Braz. J. Microbiol. 50, 185–193. doi: 10.1007/s42770-018-0011-8
Liu, B., Wang, S., Wang, J., Zhang, X., Shen, Z., Shi, L., et al. (2020). The great potential for phytoremediation of abandoned tailings pond using ectomycorrhizal Pinus sylvestris. Sci. Total Environ. 719:137475. doi: 10.1016/j.scitotenv.2020.137475
Lukesova, T., Kohout, P., Vetrovsky, T., and Vohnik, M. (2015). The potential of Dark Septate Endophytes to form root symbioses with ectomycorrhizal and ericoid mycorrhizal middle European forest plants. PLoS One 10:e0124752. doi: 10.1371/journal.pone.0124752
Marungruang, N., Kovalenko, T., Osadchenko, I., Voss, U., Huang, F., and Burleigh, S. (2020). Lingonberries and their two separated fractions differently alter the gut microbiota, improve metabolic functions, reduce gut inflammatory properties, and improve brain function in ApoE-/- mice fed high-fat diet. Nutr. Neurosci. 23, 600–612. doi: 10.1080/1028415X.2018.1536423
Masuko, T., Minami, A., Iwasaki, N., Majima, T., Nishimura, S.-I., and Lee, Y. C. (2005). Carbohydrate analysis by a phenol–sulfuric acid method in microplate format. Anal. Biochem. 339, 69–72. doi: 10.1016/j.ab.2004.12.001
Monazzah, M., Rabiei, Z., and Enferadi, S. T. (2018). The Effect of Oxalic Acid, the Pathogenicity Factor of Sclerotinia Sclerotiorum on the Two Susceptible and Moderately Resistant Lines of Sunflower. Iran. J. Biotechnol. 16:e1832.
Mu, D., Du, N., and Zwiazek, J. J. (2021). Inoculation with Ericoid Mycorrhizal Associations Alleviates Drought Stress in Lowland and Upland Velvetleaf Blueberry (Vaccinium myrtilloides) Seedlings. Plants 10:2786. doi: 10.3390/plants10122786
Murray, M. G., and Thompson, W. F. (1980). Rapid isolation of high molecular weight plant DNA. Nucleic Acids Res. 8, 4321–4326. doi: 10.1093/nar/8.19.4321
Parnanen, P., Lahteenmaki, H., Tervahartiala, T., Raisanen, I. T., and Sorsa, T. (2021). Lingonberries-General and Oral Effects on the Microbiome and Inflammation. Nutrients 13:3738. doi: 10.3390/nu13113738
Perotto, S., Daghino, S., and Martino, E. (2018). Ericoid mycorrhizal fungi and their genomes: another side to the mycorrhizal symbiosis? New Phytol. 220, 1141–1147. doi: 10.1111/nph.15218
Prencipe, F. P., Bruni, R., Guerrini, A., Rossi, D., Benvenuti, S., and Pellati, F. (2014). Metabolite profiling of polyphenols in Vaccinium berries and determination of their chemopreventive properties. J. Pharm. Biomed. Anal. 89, 257–267. doi: 10.1016/j.jpba.2013.11.016
Quiroga, G., Erice, G., Aroca, R., Zamarreño, ÁM., García-Mina, J. M., and Ruiz-Lozano, J. M. (2018). Arbuscular mycorrhizal symbiosis and salicylic acid regulate aquaporins and root hydraulic properties in maize plants subjected to drought. Agric. Water Manag. 202, 271–284.
Rapparini, F., and Peñuelas, J. (2014). “Mycorrhizal Fungi to Alleviate Drought Stress on Plant Growth,” in Use of Microbes for the Alleviation of Soil Stresses, Volume 1, ed. M. Miransari (New York, NY: Springer), 21–42.
Rohrl, C., Steinbauer, S., Bauer, R., Roitinger, E., Otteneder, K., and Wallner, M. (2021). Aqueous extracts of lingonberry and blackberry leaves identified by high-content screening beneficially act on cholesterol metabolism. Food Funct. 12, 10432–10442. doi: 10.1039/d1fo01169c
Ryyti, R., Hamalainen, M., Peltola, R., and Moilanen, E. (2020). Beneficial effects of lingonberry (Vaccinium vitis-idaea L.) supplementation on metabolic and inflammatory adverse effects induced by high-fat diet in a mouse model of obesity. PLoS One 15:e0232605. doi: 10.1371/journal.pone.0232605
Sairam, R. K., Rao, K. V., and Srivastava, G. C. (2002). Differential response of wheat genotypes to long term salinity stress in relation to oxidative stress, antioxidant activity and osmolyte concentration. Plant Sci. 163, 1037–1046.
Saitou, N., and Nei, M. (1987). The neighbor-joining method: a new method for reconstructing phylogenetic trees. Mol. Biol. Evol. 4, 406–425.
Sharma, S., Compant, S., Ballhausen, M.-B., Ruppel, S., and Franken, P. (2020). The interaction between Rhizoglomus irregulare and hyphae attached phosphate solubilizing bacteria increases plant biomass of Solanum lycopersicum. Microbiol. Res. 240:126556. doi: 10.1016/j.micres.2020.126556
Smith, S. E., Facelli, E., Pope, S., and Andrew Smith, F. (2010). Plant performance in stressful environments: interpreting new and established knowledge of the roles of arbuscular mycorrhizas. Plant Soil 326, 3–20.
Sun, T., Tan, W., Yang, Y., and Mu, H. (2021). AM Fungi and Piriformospora indica Improve Plant Growth of Pinus elliottii Seedlings. Phyton 90, 171–178.
Upson, R., Read, D. J., and Newsham, K. K. (2007). Widespread association between the ericoid mycorrhizal fungus Rhizoscyphus ericae and a leafy liverwort in the maritime and sub-Antarctic. New Phytol. 176, 460–471. doi: 10.1111/j.1469-8137.2007.02178.x
Velikova, V., Yordanov, I., and Edreva, A. (2000). Oxidative stress and some antioxidant systems in acid rain-treated bean plants: protective role of exogenous polyamines. Plant Sci. 151, 59–66.
Vohník, M. (2020). Ericoid mycorrhizal symbiosis: theoretical background and methods for its comprehensive investigation. Mycorrhiza 30, 671–695. doi: 10.1007/s00572-020-00989-1
Walker, J. F., Aldrich-Wolfe, L., Riffel, A., Barbare, H., Simpson, N. B., Trowbridge, J., et al. (2011). Diverse Helotiales associated with the roots of three species of Arctic Ericaceae provide no evidence for host specificity. New Phytol. 191, 515–527. doi: 10.1111/j.1469-8137.2011.03703.x
Wei, X., Chen, J., Zhang, C., Liu, H., Zheng, X., and Mu, J. (2020). Ericoid mycorrhizal fungus enhances microcutting rooting of Rhododendron fortunei and subsequent growth. Hortic. Res. 7:140.
Wei, X., Chen, J., Zhang, C., and Pan, D. (2016a). Differential Gene Expression in Rhododendron fortunei Roots Colonized by an Ericoid Mycorrhizal Fungus and Increased Nitrogen Absorption and Plant Growth. Front. Plant Sci. 7:1594. doi: 10.3389/fpls.2016.01594
Wei, X., Chen, J., Zhang, C., and Pan, D. (2016b). A New Oidiodendron maius Strain Isolated from Rhododendron fortunei and its Effects on Nitrogen Uptake and Plant Growth. Front. Microbiol. 7:1327. doi: 10.3389/fmicb.2016.01327
Wu, Q.-S., Zou, Y.-N., and Fathi Abd-Allah, E. (2014). “Chapter 15 - Mycorrhizal Association and ROS in Plants,” in Oxidative Damage to Plants, ed. P. Ahmad (San Diego: Academic Press), 453–475.
Keywords: symbiosis, drought stress, photosynthetic pigment, Ericoid mycorrhiza, growth
Citation: Lou H, Guo C, Fan B, Fu R, Su H, Zhang J and Sun L (2022) Lingonberry (Vaccinium vitis-idaea L.) Interact With Lachnum pygmaeum to Mitigate Drought and Promote Growth. Front. Plant Sci. 13:920338. doi: 10.3389/fpls.2022.920338
Received: 14 April 2022; Accepted: 10 May 2022;
Published: 09 June 2022.
Edited by:
Shaojun Dai, Shanghai Normal University, ChinaReviewed by:
Maria Vasilyevna Klenova, St. Petersburg State Agrarian University, RussiaAoxue Wang, Northeast Agricultural University, China
Tong Lin, South China Normal University, China
Copyright © 2022 Lou, Guo, Fan, Fu, Su, Zhang and Sun. This is an open-access article distributed under the terms of the Creative Commons Attribution License (CC BY). The use, distribution or reproduction in other forums is permitted, provided the original author(s) and the copyright owner(s) are credited and that the original publication in this journal is cited, in accordance with accepted academic practice. No use, distribution or reproduction is permitted which does not comply with these terms.
*Correspondence: Jie Zhang, emhhbmdqaWUyMDE1QG5lZnUuZWR1LmNu; Long Sun, c3VubG9uZzM2NUAxMjYuY29t
†ORCID: Hu Lou, orcid.org/0000-0002-5617-6940