- Instituto de Biología Molecular y Celular de Plantas, Universitat Politècnica de València-Consejo Superior de Investigaciones Científicas, Ciudad Politécnica de la Innovación, Valencia, Spain
Seed longevity is modulated by multiple genetic factors in Arabidopsis thaliana. A previous genome-wide association study using the Elevated Partial Pressure of Oxygen (EPPO) aging assay pinpointed a genetic locus associated with this trait. Reverse genetics identified the transcription factor DOF4.1 as a novel seed longevity factor. dof4.1 loss-of-function plants generate seeds exhibiting higher germination after accelerated aging assays. DOF4.1 is expressed during seed development and RNAseq data show several putative factors that could contribute to the dof4.1 seed longevity phenotype. dof4.1 has reduced seed permeability and a higher levels of seed storage proteins mRNAs (cruciferins and napins) in developing seeds, as compared to wild-type seeds. It has been reported that mutant lines defective in cruciferins or napins present reduced seed longevity. The improved longevity of dof4.1 is totally lost in the quadruple mutant dof4.1 cra crb crc, but not in a dof4.1 line depleted of napins, suggesting a prominent role for cruciferins in this process. Moreover, a negative regulation of DOF4.1 expression by the transcription factor DOF1.8 is suggested by co-inoculation assays in Nicotiana benthamiana. Indeed, DOF1.8 expression anticorrelates with that of DOF4.1 during seed development. In summary, modulation of DOF4.1 levels during seed development contributes to regulate seed longevity.
Introduction
Seed longevity is an essential trait for plants with economic importance. Prolonged storage reduces seed viability, with many crops having short life spans when stored in an uncontrolled environment (Leprince et al., 2017). Seed longevity is a multifactorial trait, and an increasing number of genetic factors have been identified, mainly in model species, using reverse genetics, genome-wide association studies or coexpression network analysis (Righetti et al., 2015; Renard et al., 2021). A main strategy to circumvent seed deterioration is embryo protection from oxidative damage of cellular components such as lipids, nucleic acids and proteins (Bailly et al., 2008; Rajjou and Debeaujon, 2008; Rajjou et al., 2008), that would decrease seed germination rate or increase frequency of abnormal seedlings. At the interface between the embryo and the external environment, the seed coat is the first front to minimize oxidation from outside. In this regard, seed coat lipidic barriers such as cutin and suberin, that confer impermeability, have proven to be essential for seed longevity (Renard et al., 2020a,2021). Moreover, the ability of some types of seeds to transform the cytoplasm into a glassy state, which reduces metabolic activity and hence oxidation (named orthodox seeds), allow them to survive for decades in extreme dehydration (Buitink et al., 2000; Rajjou and Debeaujon, 2008). This glassy cytoplasm is proposed to be stabilized by oligosaccharides (i.e., raffinose, RFOs) and by the highly hydrophilic late embryogenesis abundant (LEA) proteins. LEA accumulation correlates with longevity acquisition (Chatelain et al., 2012) and decreases with aging (Rajjou et al., 2008), and down-regulation of the Arabidopsis LEA14 gene affects seed longevity (Hundertmark et al., 2011). Small heat-shock-proteins sHSPs also play a role in seed longevity, likely preventing aggregation in the glassy-state and assisting in protein folding (Almoguera et al., 2012). Other protective mechanisms comprise passive antioxidant defenses, such as seed coat flavonoids (Debeaujon et al., 2000), tocopherols (Sattler et al., 2004), or glutathione (Kranner et al., 2006), as well as detoxifying enzymes, such as superoxide dismutases (Le et al., 2010; Lee et al., 2010) or the NADP-MALIC ENZYME 1, which reduces the levels of protein carbonylation during seed storage (Yazdanpanah et al., 2019).
Seed storage proteins (SSPs) accumulate significantly during seed maturation, and constitute the major source of reduced nitrogen for the growing seedlings. Seeds of A. thaliana contain two main classes of SSPs, cruciferins and napins. Cruciferins are 12S globulins synthesized as preproproteins, proteolytically cleaved into α and β subunits. Mature 12S globulins are 12-mers composed of six α and six β polypeptides (Adachi et al., 2003). Napins are 2S albumins that accumulate as heterodimers consisting also of two subunits generated by cleavage of a precursor (Krebbers et al., 1988). Arabidopsis thaliana contains four genes encoding cruciferins (CRUCIFERIN A (CRA, At5g44120), CRUCIFERIN B (CRB, At1g03880), CRUCIFERIN C (CRC, At4g28520), and CRUCIFERIN D (CRD, At1g03890) and five genes encoding napins, named as SESA1 to 5 (van der Klei et al., 1993; Wan et al., 2007). Comparative proteomics of near isogenic lines (NILs) of Arabidopsis with introgressed genomic regions at seed longevity QTLs (Nguyen et al., 2012) suggested that SSPs are important for seed longevity, and knock-out mutants lacking CRA, CRB, and CRC or reduced levels of napins presented reduced seed longevity (Nguyen et al., 2015). Due to their abundance and their high affinity for oxidation, SSPs are potent ROS buffering system, protecting other proteins from oxidation. In fact, the level of carbonylated proteins after aging is increased in a triple crua crub cruc mutant (Nguyen et al., 2015). Therefore, understanding the dynamics of seed storage protein accumulation and their regulators reveals as a novel way to modulate seed longevity.
SSPs are synthesized during seed filling, increasing steadily from 10 to 17 days after pollination, and accounting for nearly 60% of the Arabidopsis dry seed protein content (Baud et al., 2002). The B3-domain-containing transcription factors FUSCA3 (FUS3), ABSCISIC ACID INSENSITIVE3 (ABI3) and LEAFY COTYLEDON2 (LEC2) are master regulators of seed development controlling most seed maturation processes, including seed storage proteins synthesis (Verma et al., 2022). SSP promoters contain elements with which B3-domain proteins interact (Bäumlein et al., 1986; Ezcurra et al., 2000; Reidt et al., 2000) and most of them are direct targets of FUS3, LEC2, and ABI3 (Braybrook et al., 2006; Mönke et al., 2012; Wang and Perry, 2013). Consequently, abi3, lec2, and fus3 mutants exhibit reduction of SSP accumulation, and also reduced seed longevity (Parcy et al., 1997; Sugliani et al., 2009). LEAFY COTYLEDON1 (LEC1), another master regulator of seed development, activates the synthesis of SSPs via FUS3 and ABI3 (Kagaya et al., 2005). Gibberellins (GAs) negatively regulates the synthesis of SSPs. The DELLA protein RGL3 facilitates ABI3 transcriptional activation via protein–protein interaction, thus promoting the expression of SSP synthesis genes. Decrease of GAs and increase of abscisic acid during seed maturation (Yan and Chen, 2017) results in ABI3 and RGL3 accumulation and SSP synthesis.
SSPs expression arrest is also genetically regulated. At the end of the seed filling stage, SSPs genes are down-regulated and SSP accumulation ceases (Baud et al., 2002). Repression of the whole LEC1 network is performed by members of the VP1/ABI3-LIKE (VAL) family of B3 domain transcription factors (Suzuki et al., 2007) or by the chromatin-remodeling factor PICKLE (Ogas et al., 1999). A more specific mechanism is the repression of specific seed maturation genes while allowing continued expression of others. One example is the French bean ROM2 gene, a bZIP transcription factor expressed after storage protein expression. ROM2 binds to the phaseolin G-box promoter region and represses phaseolin expression (Chern et al., 1996). Seed storage protein synthesis is also linked to available nitrogen (Lhuillier-Sounde’le’ et al., 1999). During seed filling, seeds become sink tissues that rely on remobilization of nutrients, especially from senescent leaves, being nitrogen import into the seed a rate-limiting step (Havé et al., 2017). Several lines of evidence suggest the existence of sensors that monitor and adjust seed protein contents to nitrogen availability. For example, transgenic plants overexpressing storage proteins result in a compensatory reduction of the endogenous ones (Hagan et al., 2003; Scossa et al., 2008), and the TaNAM-B1 transcription factor present in ancestral wheat varieties accelerates leaf senescence and increases seed protein content (Uauy et al., 2006).
Recent genomics and systems approaches have revealed the intricate regulatory network controlling seed maturation (Holdsworth et al., 2008; Santos-Mendoza et al., 2008; Lee et al., 2010; Sreenivasulu and Wobus, 2013), that includes the expression of sets of transcriptions factor acting in different seed compartments at different stages of development, regulating many of the abovementioned events. DOF transcription factors are plant-specific transcription factors containing an N-terminal DOF (DNA-binding one finger) domain and a C-terminal region for transcriptional regulation. Arabidopsis contains 37 potential DOF genes (Yanagisawa, 2002; Yang et al., 2006; Moreno-Risueno et al., 2007; Le Hir and Bellini, 2013; Noguero et al., 2013), classified into seven groups discerning mainly in their C-terminal part, which confers them a broad range of biological functions in different plant-specific scenarios, such as photosynthetic carbon assimilation, light-regulated gene expression, accumulation of seed storage proteins, germination, dormancy, response to phytohormones and flowering time (Gupta et al., 2015). In the recent years, DOF proteins have been identified that bind to plant genes and interact with other transcription factors involved in seed development (reviewed in Ruta et al., 2020). Among them, the maize P-box-containing DOF transcription factor regulates SSP accumulation by binding to the prolamin box in zein gene promoters, interacting with the bZIP transcriptional activator Opaque2 (Vicente-Carbajosa et al., 1997), the maize ZmDOF36 protein positively controls starch accumulation (Wu et al., 2019), and the soybean GmDof4 and GmDof11 genes enhance lipid content in Arabidopsis seeds (Wang et al., 2007). DOF transcription factors are also involved in other aspects of seed development, such as suberin biosynthesis in the outer integument, which further influence seed longevity. In Arabidopsis, the DOF1.5/COG1 gene is a negative regulator of light perception that controls the expression of the peroxidases PRX2 and PRX25, involved in the polymerization of suberin in the seed coat (Renard et al., 2020a). Also, the Arabidopsis DOF4.2 protein has been proposed to be involved in seed coat composition and mucilage production (Zou et al., 2013). Given the diversity in structure of the different DOF proteins, the discovery of more members of this family involved in seed development will be of great value to understand the molecular events controlling seed quality traits such as seed longevity.
In this study, we identified the transcription factor DOF4.1 as a negative regulator of seed longevity. dof4-1 loss-of-function mutant exhibited enhanced seed viability after artificial aging assays. Transcriptomic analysis of dof4-1 showed significant accumulation of transcripts of several longevity-related genes, especially SSPs. Seed permeability is reduced in dof4-1 mutants, and lipid polyester layer is thicker in these mutants, suggesting also a role for DOF4.1 in seed coat lipid barriers development. The enhanced seed longevity of dof4-1 is abolished in the quadruple mutant dof4.1 crua crub cruc, suggesting that increased cruciferin expression greatly contributes to the dof4-1 phenotype. Moreover, a functional link is suggested between DOF4.1 and DOF1.8 transcription factors. DOF1.8 repressed DOF4.1 expression in Nicotiana leaves and expression of both transcription factors anticorrelates during seed development. However, dof1.8 mutants present similar longevity than wild type, suggesting the existence of additional cellular factor controlling DOF4.1 expression.
Materials and Methods
Plant Material and Growth Conditions
dof4.1-1 (Salk_076064), dof4.1-3 (GABI_383E02), and dof1.8-1 (Salk_130584) mutants were obtained from the Nottingham Arabidopsis Stock Centre (NASC). cra crb crc (crabc) mutant and RNAi napin line (transgenic line for napin silencing through RNAi), generated by Withana-Gamage et al. (2013), were a gift from Dr. Bentsink laboratory.
Arabidopsis thaliana seeds were surface sterilized with 70% ethanol 0.1% Triton X-100 for 15 min and rinsed three times with sterile water. Seeds were stratified for 3 days at 4°C. Germination was carried out on plates containing 25 ml Murashige and Skoog (MS) salts with 1% (w/v) sucrose, 10 mM 2-(N-morpholino) ethanesulfonic acid and 0.9% (w/v) agar, pH was adjusted to 5.7. To obtain fresh seeds, Arabidopsis plants were grown under greenhouse conditions (16 h light/8 h dark, at 23 ± 2°C and 70 ± 5% relative humidity) in pots containing a 1:2 vermiculite:soil mixture. Control and mutant plants were grown simultaneously.
Artificial Aging Assays
Seeds used for testing were harvested at the same time and stored under the same conditions for at least 2 weeks prior to the experiment. A control germination assay without aging was used to discard genotypes with affected germination. The Elevated Partial Pressure of Oxygen (EPPO) (Groot et al., 2012), was performed with 5 bar O2 and 10% relative humidity of for 9 months. For the accelerated-aging treatment, imbibed seeds were aged at 40°C for 32–36 h. Natural seed aging treatment consisted of dry seed storage at room temperature (20–25°C, 40–60% RH) for 18 months. Except in accelerated aging, all treatments were performed prior to seed sterilization and stratification. After the treatment, seeds were sown on MS medium and seedling establishment with green cotyledons was scored after 9 days. All the assays were performed with three biological replicates using 50 seeds per replicate. T-tests were used to determine significant differences between genotypes.
Biochemical Staining Assays
Triphenyltetrazolium salt penetration assay was performed as described by Molina et al. (2008). Briefly, seeds were incubated in the dark in 1% (w/v) tetrazolium red at 30°C for 2 and 4 days. Then, formazan was extracted and quantified as the absorbance at 485 nm. For mucilage staining, seeds were first imbibed in water during 3 min and then incubated in 0.2% Ruthenium Red solution for 15 m with agitation. After washing with water, photographs were taken with a Nikon’s Eclipse E600 microscope.
Seed coat suberin staining was performed on mature seeds with Sudan Red 7B as described by Bueso et al. (2016).
Transcriptomic Assays and RNA Data Analysis
RNA was extracted from developing seeds (9 days after pollination, DAP), as described before (Oñate-Sánchez and Vicente-Carbajosa, 2008). Two replicates were performed for wild-type seeds, and three replicates for dof4.1-3 seeds. Twenty-million paired-ends 50 nt reads per library (40 million in total) were sequenced. After adaptor removal and low-quality trimming of raw reads with cutadapt (Martin, 2011), clean reads were quality assessed with FastQC and mapped to the TAIR10 Arabidopsis thaliana genome using HISAT2 (Kim et al., 2015). Gene counts were then obtained with htseq-count (Anders et al., 2015) and used for differential expression analysis with DESeq2 (Love et al., 2014). Significative genes (p-adjusted (FDR) < 0.05 and expression in dof4.1 at least two-fold higher or lower than in the wild type) were used for functional analysis using Panther (Mi et al., 2021). Redundant GO terms were removed using ReviGO (Supek et al., 2011) and remaining GO terms were visualized using bubble plots. Data have been submitted to GEO repository under the accession number GSE198206.
qRT-PCR Expression Assays
RNA was extracted from developing seeds (3-21 DAP) as above. DNA removal was performed with DNAse kit (E.Z.N.A). About 500 ng RNA was reverse-transcribed using the Maxima first-strand cDNA synthesis kit (Thermo Fisher Scientific) according to the manufacturer’s instructions. qRT-PCR was performed with the PyroTaq EvaGreen qPCR Mix Plus (ROX; Cultek S.L.U., Spain) in a total volume of 20 μl using an Applied Biosystems 7500 Real-Time PCR System (Thermo Fisher Scientific). Data are the mean of three replicates. Relative mRNA abundance compared to AT5G55840, ASAR1 was calculated, using the comparative ΔCt method. Primers for qRT-PCR are listed in Supplementary Table 1.
Constructs and Agrobacterium Transformation
To obtain pUBQ10:DOF4.1-HA construct, the cDNA of DOF4.1 was amplified from wild-type seeds cDNA, using the following primers, that include BamHI restriction sites at both ends of the gene: DOF4.1 Bam FP: TAGCGGATCCATGGACCATCATCAGTATCAT and DOF4.1 Bam RP: TGAGGGATCCCCATGTTGGTCCACCACTAT. After amplification, it was cloned into the pCR8 vector. Then, the cDNA was introduced into pTEX:2xHA vector using BamHI sites. Finally, the cDNA DOF4.1-HA fragment was obtained by digestion with SmaI and SalI and ligated into the pUBQ10 vector.
To obtain pDOF4.1:GFP construct, the promoter of DOF4.1 (1,500 bp upstream the start codon) was amplified using the following primers (pDOF.FP: CCAAAACCAAACAGAATTGATTCCC and pDOF.RP: AGTAATTAATCCCTGTAATAAGTATACGTATG), subcloned into pCR8 vector, and finally into pMDC107 through GATEWAY technology.
p35S:DOF1.8 was generated using the Goldenbraid (GB) technology (Sarrion-Perdigones et al., 2014). First, DOF1.8 cDNA sequence was domesticated and introduced in the pUPD2 vector following the instructions of GoldenBraid4.0 webpage. Primers for DOF1.8 domestication are listed in Supplementary Table 2. Then, a GoldenBraid multipartite assembly reaction was performed to join the generated DOF1.8 cDNA with the 35S promoter (GB0030) and a NOS terminator (GB0037) GB parts (in the pUPD2 vector) and to introduce the resulting transcriptional unit (TU) into the pDGB_α1 Goldenbraid destination vector. E. coli competent cells were transformed with the different constructs and proper cloning was proved by plasmid sequencing. The obtained constructs were introduced into Agrobacterium tumefaciens strain GV3101 for transient (in Nicotiana) or stable expression (in dof4.1-3 mutant in Arabidopsis, using the floral dipping method).
Transient Expression Assays in Nicotiana benthamiana
Transient expression assays were performed by agroinfiltration of 4-weeks-old Nicotiana benthamiana leaves. Agrobacterium cultures containing pDOF4.1:GFP and p35S:DOF1.8 were grown until OD:0,2 and leaves were infiltrated with pDOF4.1:GFP with or without p35S:DOF1.8. p35S:YFP (GB0209) was employed as a positive control and p35S:DOF1.8 alone was used as negative control. In all cases, p35S:P19 silencing suppressor (GB0108) was coinfiltrated and pDGB1alpha1_SF (GB0106), a construct with a Solanum lycopersicum intergenic region (an “inert” DNA fragment), was used, when necessary, to equilibrate the final concentration of each Agrobacterium culture in the final mixes. 3 biological replicates were performed for positive and negative controls and 6 biological replicates for assaying DOF4.1 expression. After 5 days, GFP was observed in a confocal ZEISS-LSM710 microscope. Laser used was Argon with an excitation lambda of 488 nm, and an emission spectrum of 500–550 nm was registered.
Results
Loss-of-Function of the DOF4.1 Transcription Factor Increases Seed Longevity
Previously, a genome-wide association study on Arabidopsis had revealed a genomic region on chromosome 4 associated with seed aging after the Elevated Partial Pressure of Oxygen (EPPO) assay (Renard et al., 2020b). To identify the causal agent of this association, genes around the significant polymorphism (SNP) were selected as case studies for reverse genetic analysis. The associated SNP was in linkage disequilibrium with the AT4G00940 locus, encoding a Dof-type transcription factor (DOF4.1). The DOF4.1 protein belongs to the group III of DOF transcription factors of Arabidopsis, containing the binding domain in the N-terminal part, a characteristic C-terminal part shared by all members of this group, and a LPDLNP motif shared only by two DOF proteins of the group (DOF2.5/DAG2 and DOF3.7/DAG1), involved in seed germination (Gualberti et al., 2002; Yanagisawa, 2002). According to the Arabidopsis eFP Browser (Winter et al., 2007) DOF4.1 is expressed during seed development, making this gene a putative candidate for further analysis (Supplementary Figure 1). Two T-DNA alleles were selected for aging experiments. The dof4.1-1 allele has the T-DNA inserted in the leader intron of this gene (Ahmad et al., 2013). RT-PCR experiments on homozygous plants show that this allele still transcribes some DOF4.1 mRNA. However, this mRNA includes the T-DNA in the 5′-UTR (Supplementary Data Sheet 1), which will presumably interfere in ribosome scanning and translation of the DOF4.1 protein. The dof4.1-3 allele contains a T-DNA in the second exon (Figure 1A). RT-PCR experiments show that this mutant is not able to transcribe a mRNA, and we consider it a knock-out allele (Supplementary Data Sheet 1).
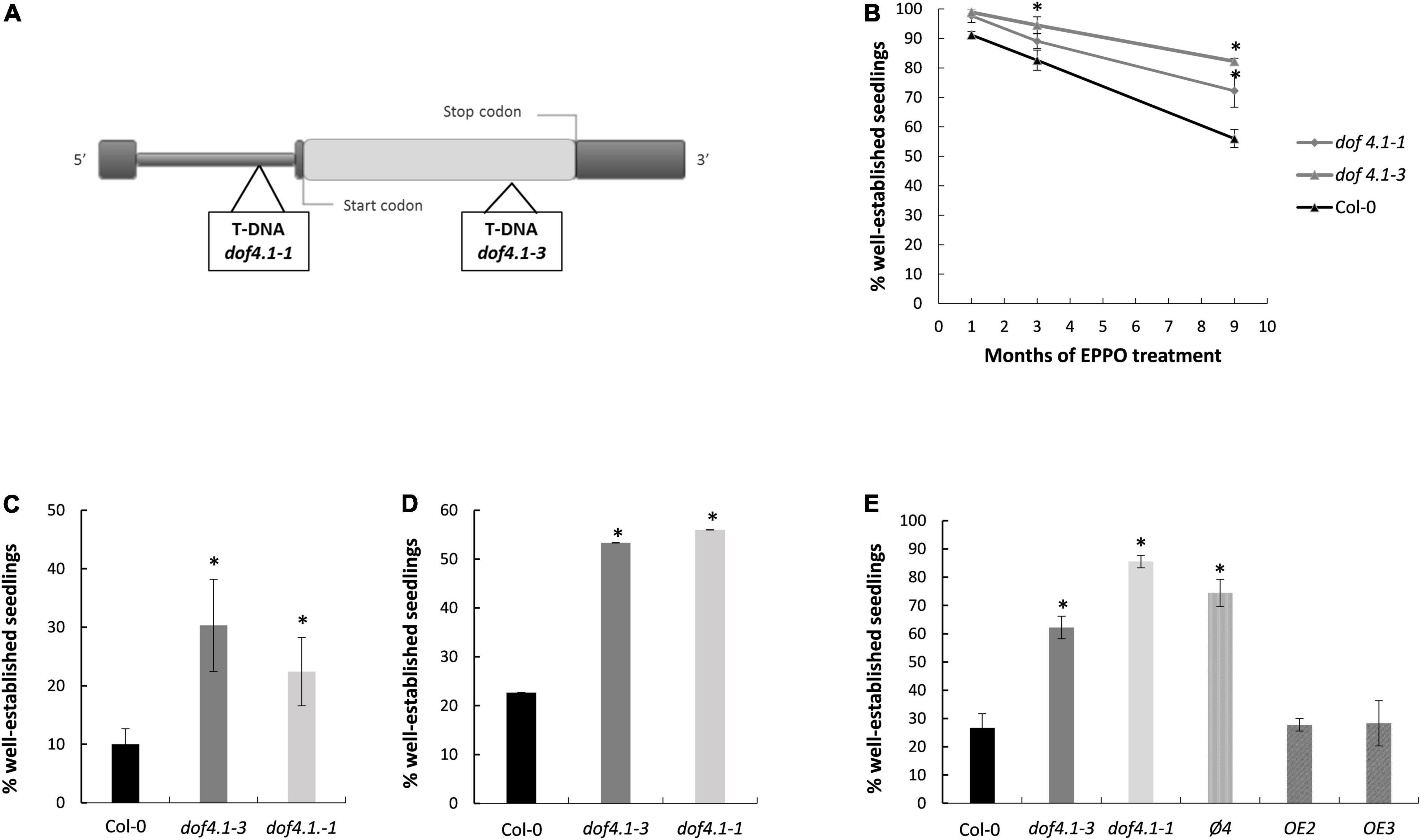
Figure 1. dof4.1 seeds exhibit increased longevity. (A) Scheme of DOF4.1 locus showing the localization of T-DNA insertions in dof4.1-1 and dof4.1-3 alleles. (B) Elevated Partial Pressure of Oxygen (EPPO) treatment: Seeds were sown on MS plates after maintaining them during 1, 3, or 9 months at 5 bar O2 and 10% RH. (C) Accelerating Aging Treatment: seeds were imbibed in water and incubated at 40°C for 36 h. (D) Natural seed aging treatment: dry seeds were maintained at room temperature and ambient humidity (20–25°C, 40–60% RH) for 18 months and sown on MS plates. (E) Accelerated aging treatment in dof4.1-3 lines overexpressing DOF4.1 under the control of pUBQ10 promoter. Seeds were imbibed in water and incubated at 40°C for 36 h. Percentage of established seedlings was recorded after 9 days growing on MS media. Bars represent the average and standard errors of three replicates with 50 seeds per line. Col-0, Columbia 0. φ4: Transgenic plant transformed with the empty pUBQ10 vector used to generate the overexpression lines. OE: overexpression lines. Asterisks indicate significant differences with WT (P < 0.05) between samples in two-tailed Student’s t-test.
Artificial aging assay (EPPO) over dof4.1-1 and dof4.1-3 seeds indicate that DOF4.1 function is hampering seed longevity. After 9 months on high oxygen conditions, 72% of dof4.1-1 and 83% of dof4.1-3 seeds could germinate, against only 56% of wild type Col-0 seeds (Figure 1B). A similar response was obtained under a wet-aging artificial assay (accelerated aging assay, Figure 1C) and also after naturally aging the seeds for 18 months on ambient conditions in the laboratory (Figure 1D). In these assays, seeds of both dof4.1 alleles are better storable than wild type, dof4.1-3 seed presenting slightly higher longevity than dof4.1-1 in EPPO and ambient assays. Complementation of the dof4.1-3 mutant with a wild-type DOF4.1 gene abolished the seed longevity resistance observed in the mutant, indicating that this phenotype is due to the lack of the DOF4.1 protein (Figure 1E). These results indicate that the presence in seeds of a functional DOF4.1 protein is detrimental for seed longevity under different storage conditions.
Transcriptomic Experiments in dof4.1-3 Seeds Suggest a Role for DOF4.1 in Seed Development
To gain insight into the mechanisms by which DOF4.1 is affecting seed longevity, the transcriptome of 10 days after fertilization (DAP) dof4.1-3 seeds was compared to wild-type‘s. 259 and 623 genes were found up-regulated and down-regulated in dof4.1-3, respectively (Supplementary Table 2). The top 20 significant categories (gene ontology, biological process) among the two group of genes are shown in Figure 2A. Although their link to seed longevity is not straightforward at first glimpse, it is remarkable the enrichment of categories such as “seed maturation” (GO: 0010431) among the upregulated genes, and “seed coat development” (GO: 0010214), among the downregulated genes, suggesting a role for the DOF4.1 gene in seed-related processes. A concerted upregulation was found among seed storage protein genes (cruciferins and napins), namely the ones encoding the CRA (3.0-fold), CRB (3.83-fold), CRC (3.6-fold) and CRD (5.7-fold) globulins, as well as in those encoding the SESA1 (AT4G27140) (5.7-fold), SESA2 (AT4G27150) (2.7-fold) and SESA5 (AT5G54740) (3.0-fold) 2S albumins. The SESA4 gene (AT4G27170) was also upregulated (2.26-fold), but slightly below the criteria we used for significance (adjusted p-value 0.06). Moreover, the SSP-inductor ABI3 gene was also up-regulated in the dof4.1-3 mutant (1.85-fold). Surprisingly, the gene encoding the master regulator LEC1 was significantly repressed (2.65-fold), perhaps obeying to an SSP-dependent feedback mechanism. Moreover, also enriched on the upregulated genes was the category lipid droplet (GO: 0005811), specialized organelles acting as seed lipidic reservoirs. Other upregulated genes involved in seed maturation with a putative link with seed longevity are the DELAY OF GERMINATION (DOG1), the ABCG6 gene, encoding a suberin transporter and four late embryogenesis abundant (LEA) genes. Finally, we found upregulated genes involved in oxidative stress mitigation, such as the superoxide dismutase MSD2, the peroxiredoxin PER1, the orthologous gene of the rice OsLpa1 gene, involved in phytic acid biosynthesis, or the VTC5 gene, involved in the synthesis of ascorbic acid, as well as the RF4 raffinose synthase and the NADP-Malic enzyme 1(NADP-ME1) genes. Any of them could potentially contribute to the extended longevity phenotype found in this mutant.
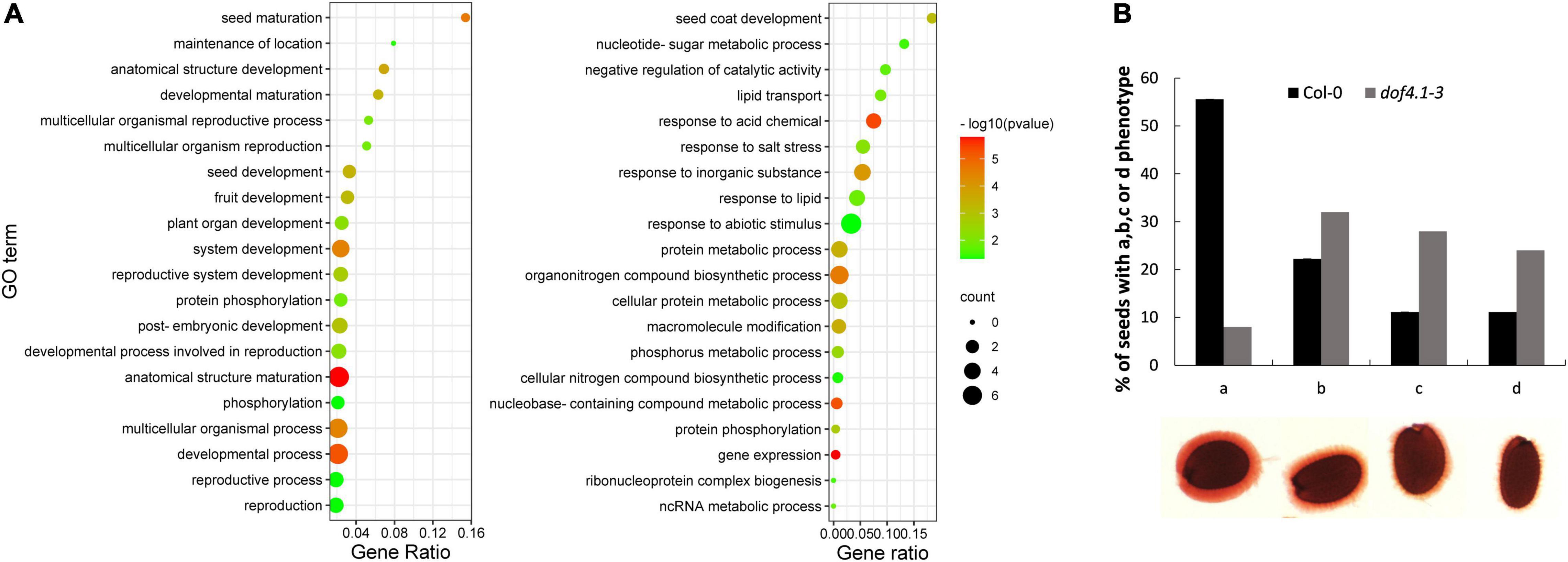
Figure 2. Seed development is affected in dof4.1-3. (A) Top-20 non-redundant enriched gene-ontology (GO) categories in dof4.1-3 upregulated (left panel) and downregulated (right panel) genes. Biological Process enrichments were obtained using Panther and filtered using REVIGO to remove semantically redundant terms. Terms were pruned by REVIGO frequency (terms with frequency > 15% were removed) and ranked by dispensability. Gene ratio: Ratio of upregulated genes in a given category divided by total number of genes in this category. Counts: Number of upregulated genes in a given category. -log p (p-value in log scale after false discovery rate correction). (B) Histogram showing visual scoring of mucilage phenotypes, from perfect (a) to severely affected mucilage (d), after staining with ruthenium red in Col-0 and dof4.1-3 seeds. 70 seeds were analyzed per genotype. Col-0, Columbia 0.
Among the dof4.1-3 down-regulated genes included in the enriched “seed coat development” category, we found up to seven genes related to mucilage development, such as the MYB61 and KNAT7 transcription factors and the MUM4, MUM5, MUCI21, MUCI10, and URGT2 genes. Moreover, the enriched category “negative regulation of catalytic activity” (GO: 0043086) includes ten downregulated pectin methylesterase inhibitor-related proteins (PMEI). Mucilage is mainly composed of pectin, and seed PMEIs regulate the degree of methylesterification of homogalacturonan, which affect mucilage properties. Among them, the PMEI6 gene, specifically expressed in seed coat epidermal cells and necessary for proper mucilage development (Saez-Aguayo et al., 2013), was also downregulated in dof4.1-3. We then investigated the ability of dof4.1 seeds to properly develop mucilage, using ruthenium red staining. When Arabidopsis wild-type seeds were hydrated, the seed coat mucilage swelled rapidly, and a dense pink-stained capsule was observed after staining. In contrast, dof4.1 displayed a lighter staining and reduced thickness of the inner mucilage layer, suggesting a looser pectin network in this mutant (Figure 2B). These results suggest that DOF4.1 is involved in different aspects of embryo and seed coat development.
Reduced Seed Permeability and Seed Storage Protein Accumulation in dof4.1 Mutants Explain Their Longevity Phenotype
Seed coat lipid barriers are important structure contributing to seed longevity, limiting the diffusion of oxidizing molecules between the embryo and the surrounding environment. Given the involvement of DOF4.1 in seed coat development, we quantified seed coat permeability of dof4.1 mutants, measuring tetrazolium salt uptake using the triphenyltetrazolium reduction method (Molina et al., 2008). As shown in Figure 3A, both dof4.1-1 and dof4.1-3 alleles accumulated lower levels of red formazans compared with the wild-type control, suggesting that seed permeability is reduced in these mutants, which could be a mechanism for their extended seed longevity. Next, the ability of dof4.1 mutants to synthesize a lipid polyester layer in the seed coat was investigated using biochemical assay. After staining seeds with Sudan red to visualize seed coat suberized cell walls, wild-type seeds showed the characteristic pink layer in the outer integument of the seed coat. This layer was clearly thicker in dof4.1 mutants, suggesting that lipid polyester deposition in seeds is enhanced when DOF4.1 is not present (Figure 3B). We next evaluated the contribution of dof4.1 SSP levels on seed longevity. The effect of these proteins on seed longevity was reported in napins and cruciferins depleted lines, which were sensitive to artificial aging (Nguyen et al., 2015). To ascertain whether the increased expression of cruciferins observed in the dof4.1-3 mutant could contribute to its extended seed longevity, a quadruple mutant dof4.1-3 cra crb crc was generated. Similarly, to investigate the contribution of napins increased expression in dof4.1, we crossed dof4.1-3 with an RNAi line depleted in napins (Withana-Gamage et al., 2013). Accelerate aging assays show that the positive effect on longevity of the dof4.1-3 mutant is abolished when all cruciferins are depleted (Figure 3C), indicating that a major contribution to longevity of the dof4.1 mutants rely on the increased expression of cruciferins and that other putative contributing factors cannot compensate for the total depletion of these proteins. In the napins-depleted dof4.1 mutant, the dof4.1 background still can confer an advantage in seed longevity (Figure 3D), likely by the increased expression of cruciferins of this mutant, or by additional factors. In summary, these experiments indicate that loss-of-function of DOF4.1 affects positively to seed impermeability. It also promotes napins and cruciferins accumulation. Cruciferin overaccumulation is determinant for the extended seed longevity observed in dof4.1 mutants, although additional SSP-independent contributions are not discarded.
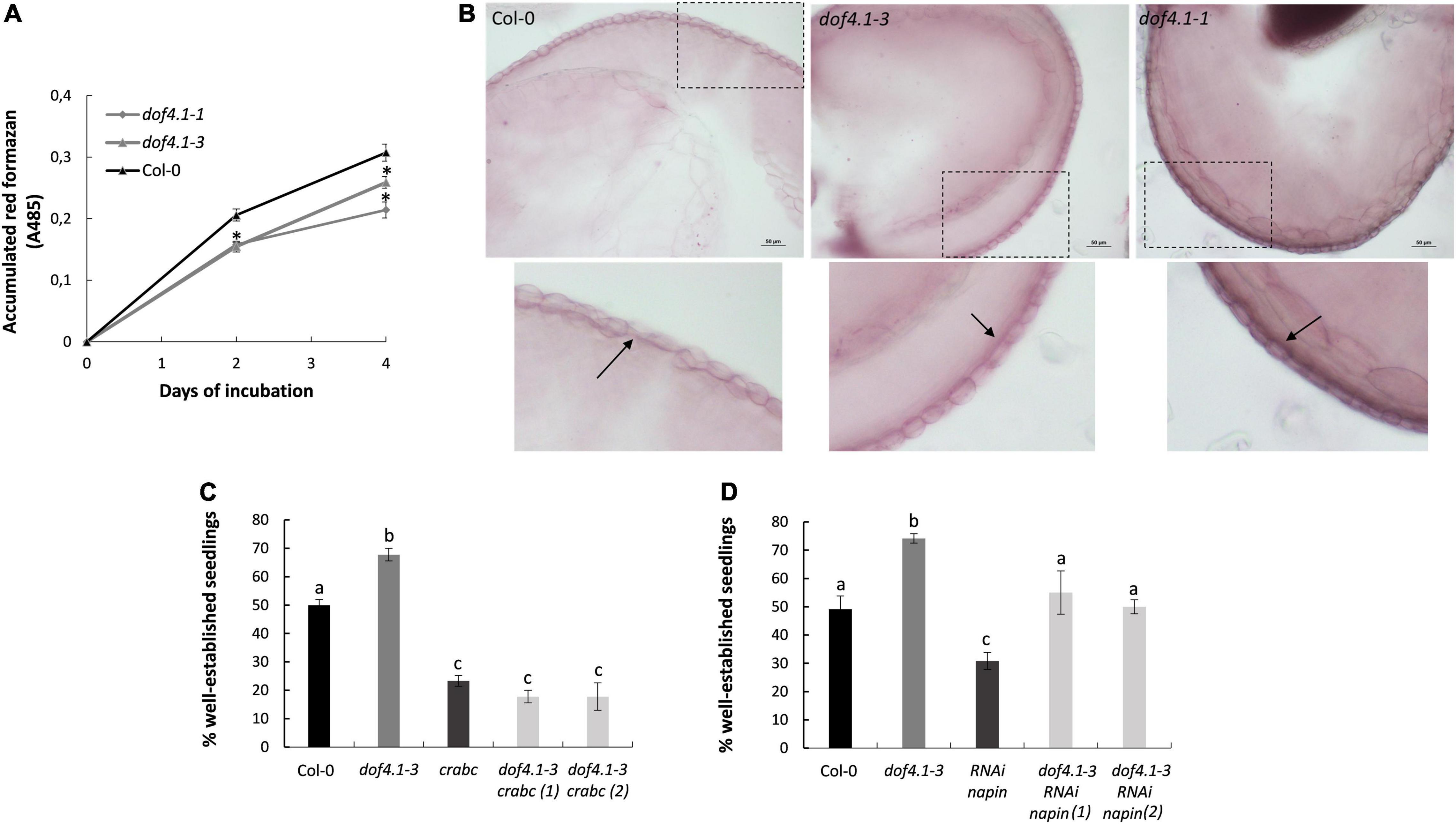
Figure 3. dof4.1mutants present reduced seed permeability and dof4.1-3 increased SSPs content determine its higher longevity. (A) Seed permeability of dof4.1 mutant. Quantitative time course of formazan accumulation in Col-0, dof4.1-1, and dof4.1-3 seeds. Wild type (Col-0) and mutant seeds were incubated during 2 or 4 days in 1% tetrazolium at 30°C and then formazan was extracted and quantified. Data (absorbance at 485 nM) are the mean and standard error of three biological replicates. Asterisks indicate significant differences with WT (P < 0.05) between samples in two-tailed Student’s t-test. (B) Sudan Red 7B staining of the subepidermal layer of the seed coat in wild type (Col-0), dof4.1-3 and dof4.1-1 dry seeds. Scale bars correspond to 50 μM. Lower panels amplify the indicated area to show a detail of the staining. Arrows point to the suberin layer Accelerating Aging Treatment over a quadruple mutant dof4.1-3 cra crb crc (C) and a transgenic RNAi line for napins in dof4.1-3 background (D). Seeds were imbibed in water and incubated at 40°C for 32 h. Percentage of established seedlings was recorded after 9 days growing on MS media. Different letters indicate significant differences with WT (P < 0.05) between samples in two-tailed Student’s t-test. Col-0, Columbia 0. crabc, cra crb crc. RNAi napin: transgenic line silenced in napins by RNA interference. Data are the mean and standard error of three biological replicates.
DOF4.1 as a Modulator of Seed Storage Proteins Expression During Seed Development
To understand the role of DOF4.1 in the seed, we first monitored the effect of its loss-of-function on SSP accumulation along seed development. Primers for RT-PCRs were designed for conserved regions of all four cruciferins, or for conserved regions of napins SESA1, 2 and 5 (see Supplementary Methods), to amplify cruciferins or napins in a single reaction. As shown in Figures 4A,B, expression of napins and cruciferins at 9 DAP followed the same profile as our RNA-seq results, with higher expression of the genes encoding both types of SSPs in dof4.1-3 seeds as compared to wild type. This difference in expression is maintained or even increased at 14 and 21 DAP for both types of SSPs genes. We next studied the expression of DOF4.1 along seed development. As shown in Figure 4C, DOF4.1 start being expressed after 9 DAP, being progressively accumulated at 14 and 21 DAP. This indicates that expression of this transcription factor is triggered at later stages of seed development, when storage reserve accumulation in the embryo is reaching to an end and the seed is reaching its mature stage, suggesting that DOF4.1 is involved in reducing the expression of SSP genes at later stages of development.
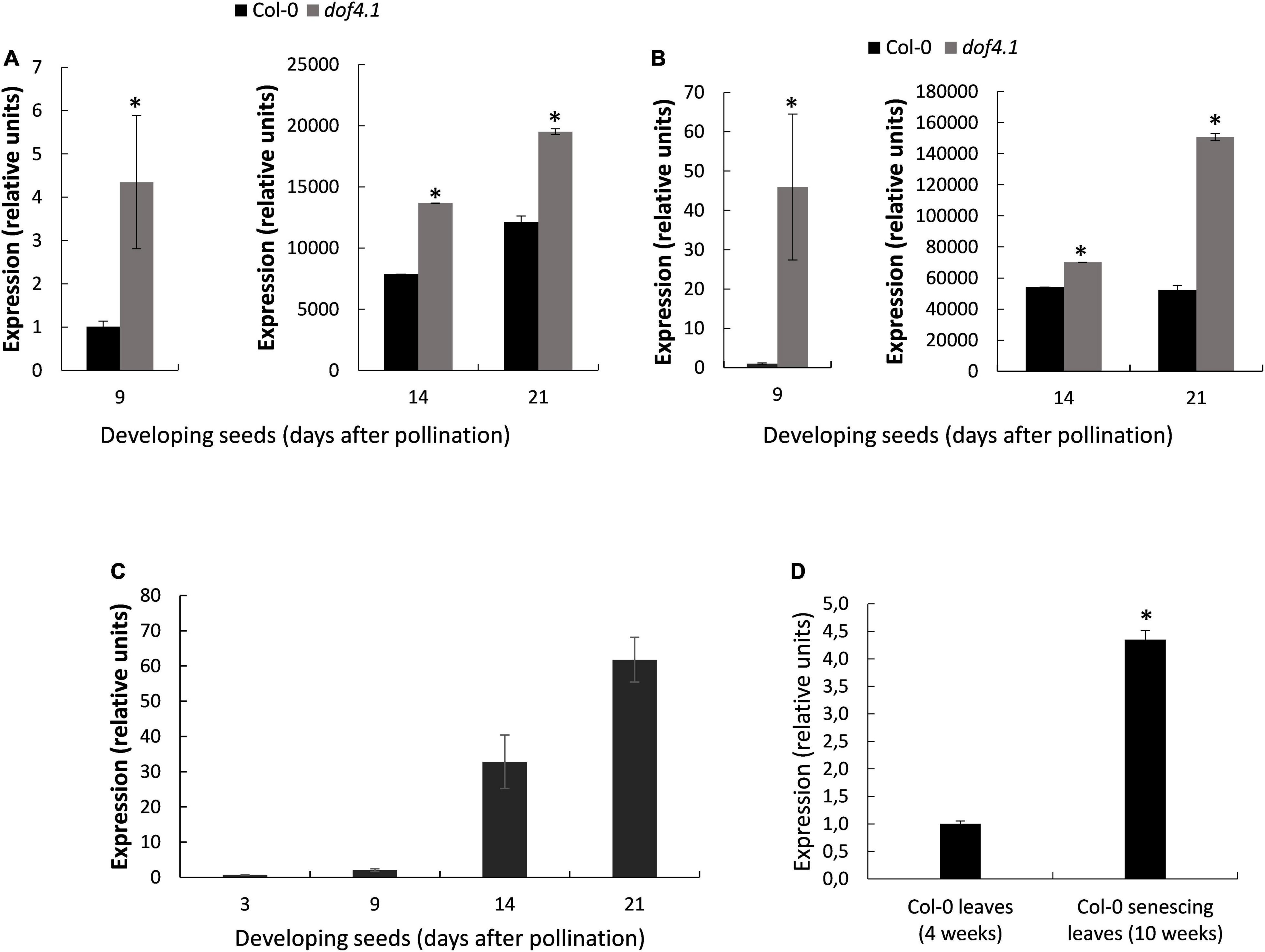
Figure 4. SSPs expression levels during seed development and dof4.1 expression in developing seeds and senescing leaves. Expression levels of cruciferins (A) and napins (B) were determined by real-time quantitative PCR (qRT-PCR) in developing seeds (9, 14, and 21 DAP) of Col-0 and dof4.1-3. (C) Expression level of DOF4.1 was determined by qRT-PCR in developing seeds (3, 9, 14, and 21 DAP) and in senescing leaves (10 weeks after transferring to soil) compared to non-senescing leaves (4 weeks after transferring to soil) (D). Data are the mean of three replicates. Significantly differing from Col-0 at P < 0.05 (*) using Student’s t-test. Col-0 = Columbia 0. DAP, Days After Pollination.
Nitrogen availability is a rate-limiting step for SSPs synthesis. Leaf senescence is another process induced by nitrogen deficiency (Balazadeh et al., 2014). In order to ascertain whether DOF4.1 could be also expressed in this nitrogen-dependent scenario, we compared expression of this gene in green well-expanded leaves vs. senescing leaves. As shown in Figure 4D, DOF4.1 expression is 4.35-fold higher in senescing leaves, suggesting that this transcription factor could be involved in nitrogen sensing processes in different organs of the plant.
DOF4.1 Expression Is Repressed by DOF1.8
DOF1.8 was identified as a putative regulator of DOF4.1 expression in a high-throughput one-hybrid experiment (Brady et al., 2011), and transient expression analysis in Arabidopsis seedlings using a GAL4-binding assay suggested that DOF1.8 has transcriptional repressor activity (Ramachandran et al., 2020). To ascertain whether DOF1.8 could be acting as a transcriptional repressor of DOF4.1, a fusion plasmid containing the green-fluorescent protein (GFP) under the control of DOF4.1 promoter (1.5 kb upstream of translational start) (pDOF4.1:GFP) was constructed and co-infiltrated into N. benthamiana epidermal cells together with a p35S:DOF1.8 construct. At 5 dpi, GFP fluorescence was observed in the nuclei of plants infiltrated with pDOF4.1-GFP alone, indicating that the DOF4.1 promoter is active in Nicotiana leaves. This signal was clearly reduced when this constructs was co-infiltrated with p35S:DOF1.8, suggesting that DOF1.8 repressed DOF4.1 expression (Figure 5A). We next studied the expression of DOF1.8 during seed development. As shown in Figure 5B, DOF1.8 is expressed at 9 DAP, being progressively repressed at 14 and 21 DAP, consistent with the increased expression of DOF4.1 in these time points. This anticorrelated expression support the hypothesis that DOF1.8 could be repressing DOF4.1 transcription in seeds, although whether DOF1.8 is directly binding to the DOF4.1 promoter or the repression is mediated by an intermediate factor is not known. To investigate whether DOF1.8 could also be involved in seed longevity via DOF4.1 regulation, we conducted aging assay on a loss-of-function dof1.8 allele containing a T-DNA in the second exon (named dof1.8-1). Accelerated aging assays show that dof1.8-1 seeds present similar longevity than wild type, whereas dof1.8-1 dof4.1-3 double mutant maintains the reduced longevity observed for dof4.1-3 (Figure 5C). This suggest the existence of redundant cellular factors that can substitute DOF1.8 to repress DOF4.1 in seeds in a dof1.8 background.
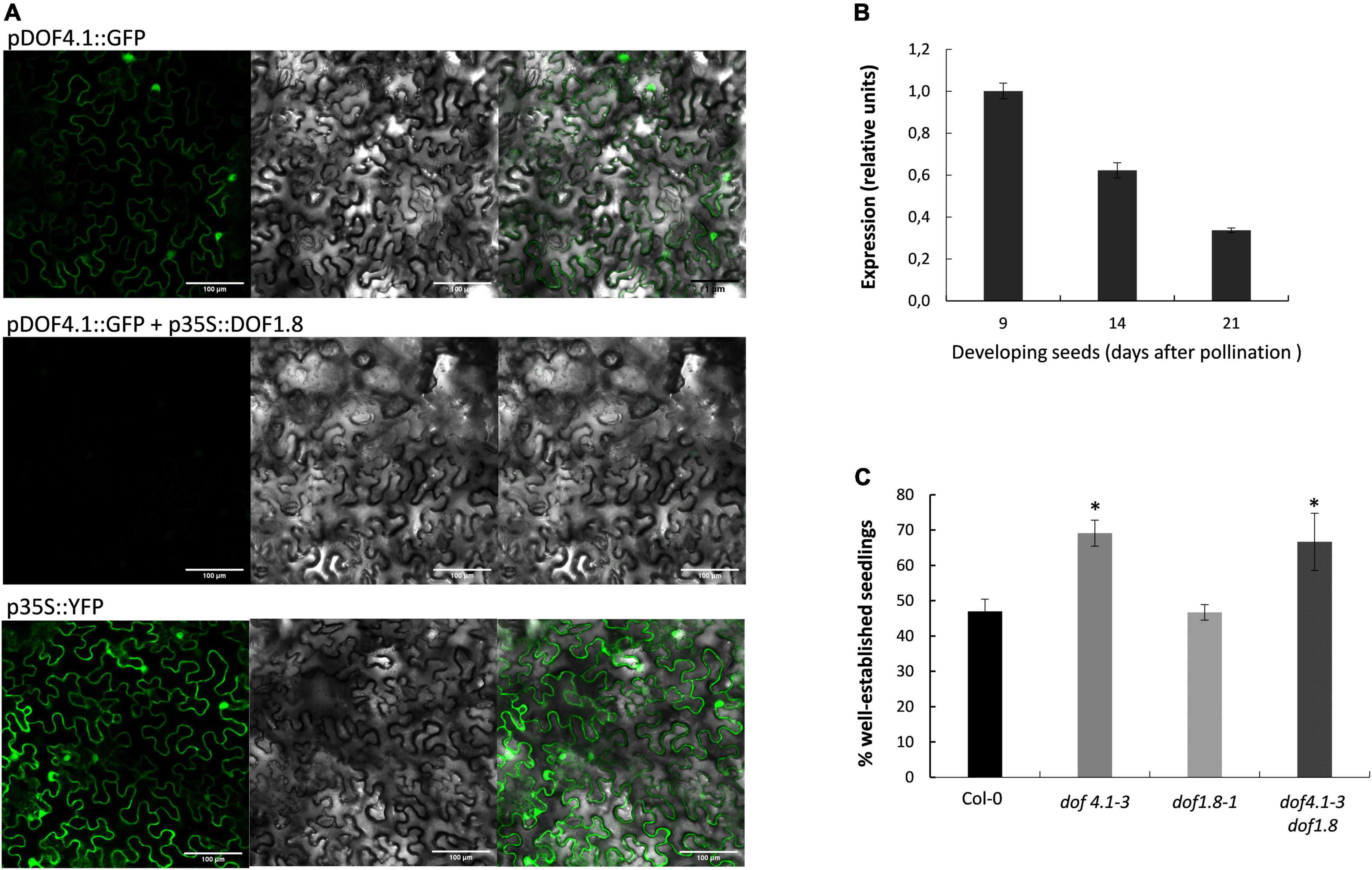
Figure 5. DOF1.8 transcription factor represses DOF4.1. (A) Representative images showing the transient expression of pDOF4.1:GFP in Nicotiana benthamiana leaves 5 days after agroinfiltration in the absence (upper panel) or in the presence (middle panel) of p35S:DOF1.8. The lower panel shows the expression of the positive control p35S: YFP. Scale bars 100 μM. (B) Expression level of DOF1.8 was determined by qRT-PCR in developing seeds (9, 14, and 21 DAP). DAP, days after polinization. (C) Accelerated aging assay of dof1.8-1 mutant and dof4.1-3 dof1.8-1 double mutant. Seeds were imbibed in water and incubated at 40°C during 32 h. The percentage of established seedlings (with green cotyledons) was recorded after 9 days growing on MS media. Data are the mean and standard error of three biological replicates. Asterisks indicate significant differences with wild type (P < 0.05) in two-tailed Student’s t-test. Col-0, Columbia 0.
Discussion
The three compartments of developing seeds (embryo, endosperm and seed coat) undergo rapid reprogramming events from fertilization until maturation in a complex regulatory network, comprising the activation by transcription factors of a precise set of target genes involved in multiple cellular processes (Gutierrez et al., 2007; North et al., 2010). Seed longevity, acquired at late stages of seed maturation, is a multifactorial trait that is regulated at different levels in these three compartments. Therefore, transcription factors involved in different aspects of seed development are likely to modulate seed longevity via their impact on downstream responses contributing to this trait. Mutants of the master regulators of seed development FUS3, LEC1, LEC2, and ABI3 are badly storable, likely by their inability to activate seed storage proteins, raffinose oligosaccharides (RFOs), and by their lack of chlorophyll degradation (Sugliani et al., 2009). APETALA2 is also involved in more than one compartment, and ap2 mutants have reduced seed longevity, probably by multiple effects on the seed (Leon-Kloosterziel et al., 1994; Ohto et al., 2009). In other cases, the precise cause affecting longevity is more defined, as for example for the homeobox HB25, mediating apoplastic lipid barriers (Renard et al., 2021) for the flavonoid biosynthesis regulators, TT2, TT8, or TT16 (Debeaujon et al., 2000) or for the HEAT SHOCK FACTOR HSF9, activating small heat-shock-proteins (Prieto-Dapena et al., 2006; Tejedor-Cano et al., 2010). More transcription factors impacting seed longevity have been described, although their role is still uncertain. For example, WRKY3 and NFXL1 were identified a coexpression network analysis (Righetti et al., 2015), and KNAT7, SEPALLATE 3 or MYB47 in a genome-wide association study (Renard et al., 2020b). Loss-of-function mutants of these genes all exhibit reduced seed longevity. Here, we introduce a new player in the intricate regulatory network governing seed development, and suggest possible causes for the enhanced seed longevity of their loss-of-function mutant. The better storability of dof4.1 seeds suggest that DOF4.1 acts negatively on processes that foster seed longevity. Analysis of the transcriptome suggest that this could be happening at least via the seed coat and the embryo. The reduced permeability of dof4.1 mutants and the thickness of their lipid layers suggest that DOF4.1 could be negatively regulating seed coat lipid barriers, although whether this factor is regulating lipid levels or composition is not known. Increased expression of DOG1 or NADP-ME1 could be additional components contributing for the increased storability of dof4.1 seeds. DOG1 is involved in multiple aspects of seed maturation and longevity, including the increase of compounds of the RFO pathway or accumulation of LEA and heat-shock proteins (Dekkers et al., 2016) and dog1 mutants exhibits reduced seed longevity (Bentsink et al., 2006). Seeds of the NADP-ME1 loss-of-function mutant have reduced seed viability and display higher levels of protein carbonylation than those of the wild type. NADP-ME1 catalyzes the oxidative decarboxylation of malate to pyruvate producing NADPH, and is proposed to protect seeds against oxidation (Yazdanpanah et al., 2019). Likely, all these factors contribute to the extended longevity found in dof4.1 seeds. However, a major contribution for the dof4.1 phenotype seems to be the increased expression of cruciferins. The importance of these reserves for longevity is demonstrated in the quadruple mutant dof4.1 cra crb crc, which completely abolished the enhanced longevity of dof4.1. The same effect was not observed in a dof4.1 mutant depleted in napins, confirming that cruciferins, and not napins, are a major target for carbonylation, acting as buffers during storage and protecting other proteins from oxidation (Nguyen et al., 2015). This is consistent with the phenotype observed for the transparent testa glabra 1 (ttg1) mutant, which present reduced seed longevity (Debeaujon et al., 2000) despite accumulating more napins than wild type (Chen et al., 2015). The low longevity of this mutant is probably related to the function of TTG1 in seed coat development and flavonoid biosynthesis. DOF4.1, as well as TTG1 (Gonzalez et al., 2009), are positively regulating mucilage development; however, this structure is apparently not involved in seed longevity (Renard et al., 2021), and will not be discussed further in this study.
Dof transcription factors contain the characteristic one-finger domain (a multifunctional domain involved not only in DNA binding, but also in interactions with other proteins and in cell-to-cell trafficking), and a potential transcriptional activation or repression domain, normally in the C-terminal part of the protein (Yanagisawa, 2016). The predicted domain structure of DOF4.1 (Yanagisawa, 2002; Chen et al., 2013) do not allow to conclude whether this transcription factor is an activator or a repressor, which will require additional molecular studies. Negative regulation of longevity responses mediated by DOF4.1 could be achieved by direct repression of target genes, by activation of downstream negative regulators, or by interaction with signaling proteins. This last mechanism could be the case for MPK10, a mitogen-activated protein kinase involved in endosperm development and seed size (Xi et al., 2021) which interacts with WRKY10 and inhibits the expression of its target genes. Indeed, DOF4.1 interacts with MPK10 (Popescu et al., 2009), perhaps mediating these downstream responses in the endosperm.
This study positions DOF4.1 as an important player mediating the feedback regulation of the regulatory network governing accumulation of seed storage proteins. SSPs genes are directly regulated by the LEC2/FUS3/ABI3 and LEC1 master regulators of seed development, increasing their expression progressively during seed maturation. FUS3 suppresses TTG1 expression, which inhibits the expression of 2S precursors. TTG1 expression increases during late maturation, consistently with FUS3 decrease, and consequently, SSP expression also decrease. Similar to the profile of SSP accumulation observed here for dof4.1, ttg1 mutants also present enhanced accumulation of SSPs (napins, but not cruciferins) along seed development (Chen et al., 2015). DOF4.1 transcript levels also increase progressively along seed development, contributing to the inhibition of SSPs expression that take place at this late stages of seed development (napins and cruciferins). DOF4.1, however, is not a direct target of FUS3 (Wang and Perry, 2013), ABI3 (Mönke et al., 2012; Tian et al., 2020), nor LEC1 (Pelletier et al., 2017), although DOF4.1 expression is repressed in fus3 and lec1 loss-of-function mutants (Yamamoto et al., 2014), which could be a new FUS3-dependent mechanism aimed to avoid excessive accumulation of SSPs at later stages of seed development. Interestingly, DOF4.1 is highly expressed in senescing leaves. The nitrogen content of a leaf reaches the maximum level at completion of leaf expansion, and gradually decreases in the course of leaf senescence (Mae, 2004). Storage protein accumulation in seeds depends strongly on nitrogen availability (Lhuillier-Sounde’le’ et al., 1999; Miranda et al., 2001; Rolletschek et al., 2005), explaining the reserve compensation effect observed in biotechnological approaches aimed to increase the amount of seed proteins (Hagan et al., 2003; Scossa et al., 2008). It is tempting to speculate that seed nitrogen depletion at later stages of seed development could also be a signal activating DOF4.1, which would inhibit SSP expression independent of the FUS3 network. Interestingly, in a high-throughput interactome of nitrogen-associated metabolism, DOF4.1 was shown to bind the promoter of the GLUTAMATE DEHYDROGENASE 2 (GDH2) gene (Gaudinier et al., 2018), encoding a key enzyme involved in the incorporation of ammonia into organic compounds when remobilization of nutrients is required (Skopelitis et al., 2006), reinforcing the hypothesis of DOF4.1 as a nitrogen sensor.
DOF1.8 is able to repress DOF4.1 expression (although this repression may be direct or mediated by an intermediate factor), and the anticorrelated expression of both genes in seeds suggest that this interaction could be biologically relevant in vivo. Where in the seed this interaction might be taking place is speculative and further studied will be needed, but the pattern of expression of these genes can envisaged some possibilities. DOF1.8 expression is mainly restricted to vascular tissues (Ramachandran et al., 2020). The unique maternal vascular bundle in the Arabidopsis seed terminates at the end of the funiculus and releases its content into the testa (Robinson-Beers et al., 1992), suggesting that the molecular repression of DOF4.1 by DOF1.8 in the seed coat could be taking place in this region. The symplastic connectivity within seed coat integuments (Stadler et al., 2005) would allow DOF4.1 to move to distal cells and regulate downstream processes in the outer integument, such as mucilage or lipidic barriers development, where DOF4.1 seems to be acting. Selective trafficking of transcription factors is widespread in plants (Han et al., 2014; Kitagawa and Jackson, 2017) and an intercellular trafficking motif has been reported as necessary for targeting proteins to plasmodesmata for cell-to-cell movement. Based on this, up to eight DOF transcription factors have been potentially considered as mobile (Lee and Zhou, 2012) and DOF4.1 is the canonical protein where this intercellular trafficking motif was discovered, allowing it to move between cells in roots and shoots tissues, and therefore opening the possibility to travel between cells through plasmodesmata also in seeds (Lee et al., 2006; Chen et al., 2013). A similar scenario could be hypothesized for the embryo up to globular stage, where a unique symplastic region exist, or for more developed embryos, with symplastic connectivity remaining between groups of cells belonging to similar tissues (Stadler et al., 2005).
Unfortunately, we could not observe a clear seed longevity phenotype in a dof1.8 background. This could be explained by another DOF factor substituting DOF1.8 when this is not present. In fact, both DOF1.8 and DOF4.6, the most similar DOF factor to DOF1.8 in Arabidopsis, present a similar pattern of expression (Ramachandran et al., 2020). Similar examples have been described for other proteins of the DOF family. For example, mucilage content and composition are altered in transgenic plants overexpressing the DOF4.2 transcription factor, but mucilage development is normal in dof4.2 (Zou et al., 2013). The redundancy in the DOF family, and the strong similarity among Dof DNA-binding domains suggests that Dof proteins display similar DNA-binding specificity, making plausible this hypothesis between members of the same sub-group of DOF proteins (Gupta et al., 2015; Yanagisawa, 2002). Alternatively, compensatory effects could maintain normal DOF4.1 levels in the cell, even in a mutant background.
This work represents a step forward in the knowledge of the complex transcriptional network regulating seed coat development and seed storage protein accumulation, identifies DOF4.1 as a novel player contributing to seed longevity and opens a door to further studying the role of its negative regulator, DOF1.8, in seed deterioration.
Data Availability Statement
The datasets presented in this study can be found in online repositories. The names of the repository/repositories and accession number(s) can be found below: https://www.ncbi.nlm.nih.gov/geo/, GSE198206.
Author Contributions
JG and RN conceived to the original idea, designed the experiments, and wrote the manuscript. RN, PA-M, JR, RM, JC, and CR-P performed the experiments. RN, EB, RS, and JG discussed the results and redesigned the experiments. All authors contributed to the article and approved the submitted version.
Funding
This study was funded by the Spanish Ministry of Science and Education, action BIO2014-52621-R.
Conflict of Interest
The authors declare that the research was conducted in the absence of any commercial or financial relationships that could be construed as a potential conflict of interest.
Publisher’s Note
All claims expressed in this article are solely those of the authors and do not necessarily represent those of their affiliated organizations, or those of the publisher, the editors and the reviewers. Any product that may be evaluated in this article, or claim that may be made by its manufacturer, is not guaranteed or endorsed by the publisher.
Acknowledgments
We thank Dr. Javier Forment and the Bioinformatic facility of the IBMCP for technical assistance in the RNA seq analysis.
Supplementary Material
The Supplementary Material for this article can be found online at: https://www.frontiersin.org/articles/10.3389/fpls.2022.915184/full#supplementary-material
Supplementary Figure 1 | Output image from the eFP (electronic fluorescent pictographic) browser showing the expression pattern of DOF4.1 in the Developmental series generated by Schmid et al. (2005, Map of Arabidopsis Development) and colleagues from Detlef Weigel’s group in Tübingen. Each pictograph is colored according to the level of expression for the gene on of interest (Absolute) (http://bar.utoronto.ca/efp2/Arabidopsis/Arabidopsis_eFPBrowser2.html).
Supplementary Table 1 | List of primers used in this study.
Supplementary Table 2 | Lists of differentially-expressed genes in seeds at 10 DAP between dof4.1-3 and wild type. Genes more expressed in dof4.1-3 (sheet1) or in wild type (sheet 2) seeds. p-adj: adjusted p-value using false discovery rate correction (FDR).
References
Adachi, M., Kanamori, J., Masuda, T., Yagasaki, K., Kitamura, K., Mikami, B., et al. (2003). Crystal structure of soybean 11S globulin: glycinin A3B4 homohexamer. Proc. Natl. Acad. Sci. U.S.A. 100, 7395–7400. doi: 10.1073/pnas.0832158100
Ahmad, M., Rim, Y., Chen, H., and Kim, J. Y. (2013). Functional characterization of Arabidopsis Dof transcription factor AtDof4.1. Russ. J. Plant Physiol. 60, 116–123.
Almoguera, C., Prieto-Dapena, P., Personat, J. M., Tejedor-Cano, J., Lindahl, M., Diaz-Espejo, A., et al. (2012). Protection of the photosynthetic apparatus from extreme dehydration and oxidative stress in seedlings of transgenic tobacco. PLoS One 2012:e51443–e51451. doi: 10.1371/journal.pone.0051443
Anders, S., Pyl, P. T., and Huber, W. (2015). HTSeq–a Python framework to work with high-throughput sequencing data. Bioinformatics 31, 166–169. doi: 10.1093/bioinformatics/btu638
Bailly, C., El-Maarouf-Bouteau, H., and Corbineau, F. (2008). From intracellular signaling networks to cell death: the dual role of reactive oxygen species in seed physiology. C R Biol. 331, 806–814. doi: 10.1016/j.crvi.2008.07.022
Balazadeh, S., Schildhauer, J., Araújo, W. L., Munné-Bosch, S., Fernie, A. R., and Proost, S. (2014). Reversal of senescence by N resupply to N-starved Arabidopsis thaliana: transcriptomic and metabolomic consequences. J. Exp. Bot. 65, 3975–3992. doi: 10.1093/jxb/eru119
Baud, S., Boutin, J. P., Miquel, M., Lepiniec, L., and Rochat, C. (2002). An integrated overview of seed development in Arabidopsis thaliana ecotype WS. Plant Physiol. Biochem. 40, 151–160.
Bäumlein, H., Wobus, U., Pustell, J., and Kafatos, F. C. (1986). The legumin gene family: structure of a B type gene of Vicia faba and a possible legumin gene specific regulatory element. Nucleic Acids Res. 14, 2707–2720. doi: 10.1093/nar/14.6.2707
Bentsink, L., Jowett, J., Hanhart, C. J., and Koornneef, M. (2006). Cloning of DOG1, a quantitative trait locus controlling seed dormancy in Arabidopsis. Proc. Natl. Acad. Sci. U.S.A. 103, 17042–17047. doi: 10.1073/pnas.0607877103
Brady, S. M., Zhang, L., Megraw, M., Martinez, N. J., Jiang, E., Yi, C. S., et al. (2011). A stele-enriched gene regulatory network in the Arabidopsis root. Mol. Syst. Biol. 7:459. doi: 10.1038/msb.2010.114
Braybrook, S. A., Stone, S. L., Park, S., Bui, A. Q., Le, B. H., Fischer, R. L., et al. (2006). Genes directly regulated by LEAFY COTYLEDON2 provide insight into the control of embryo maturation and somatic embryogenesis. Proc. Natl. Acad. Sci. U.S.A. 103, 3468–3473. doi: 10.1073/pnas.0511331103
Bueso, E., Muñoz-Bertomeu, J., Campos, F., Martínez, C., Tello, C., Martínez-Almonacid, I., et al. (2016). Arabidopsis COGWHEEL1 links light perception and gibberellins with seed tolerance to deterioration. Plant J. 87, 583–596. doi: 10.1111/tpj.13220
Buitink, J., Hemminga, M. A., and Hoekstra, F. A. (2000). Is there a role for oligosaccharides in seed longevity? An assessment of intracellular glass stability. Plant Physiol. 122, 1217–1224. doi: 10.1104/pp.122.4.1217
Chatelain, E., Hundertmark, M., Leprince, O., Le Gall, S., Satour, P., Deligny-Penninck, S., et al. (2012). Temporal profiling of the heat-stable proteome during late maturation of Medicago truncatula seeds identifies a restricted subset of late embryogenesis abundant proteins associated with longevity. Plant Cell Environ. 35, 1440–1455. doi: 10.1111/j.1365-3040.2012.02501.x
Chen, H., Ahmad, M., Rim, Y., Lucas, W. J., and Kim, J. Y. (2013). Evolutionary and molecular analysis of Dof transcription factors identified a conserved motif for intercellular protein trafficking. New Phytol. 198, 1250–1260. doi: 10.1111/nph.12223
Chen, M., Zhang, B., Li, C., Kulaveerasingam, H., Chew, F. T., and Yu, H. (2015). Transparent testa glabra1 regulates the accumulation of seed storage reserves in Arabidopsis. Plant Physiol. 169, 391–402. doi: 10.1104/pp.15.00943
Chern, M. S., Bobb, A. J., and Bustos, M. M. (1996). The regulator of MAT2 (ROM2) protein binds to early maturation promoters and represses PvALF-activated transcription. Plant Cell. 8, 305–321. doi: 10.1105/tpc.8.2.305
Debeaujon, I., Léon-Kloosterziel, K. M., and Koornneef, M. (2000). Influence of the testa on seed dormancy, germination, and longevity in Arabidopsis. Plant Physiol. 122, 403–414. doi: 10.1104/pp.122.2.403
Dekkers, B. J., He, H., Hanson, J., Willems, L. A., Jamar, D. C., Cueff, G., et al. (2016). The Arabidopsis DELAY OF GERMINATION 1 gene affects ABSCISIC ACID INSENSITIVE 5 (ABI5) expression and genetically interacts with ABI3 during Arabidopsis seed development. Plant J. 85, 451–465. doi: 10.1111/tpj.13118
Ezcurra, I., Wycliffe, P., Nehlin, L., Ellerström, M., and Rask, L. (2000). Transactivation of the Brassica napus napin promoter by ABI3 requires interaction of the conserved B2 and B3 domains of ABI3 with different cis-elements: B2 mediates activation through an ABRE, whereas B3 interacts with an RY/G-box. Plant J. 24, 57–66. doi: 10.1046/j.1365-313x.2000.00857.x
Gaudinier, A., Rodriguez-Medina, J., Zhang, L., Olson, A., and Liseron-Monfils, C. (2018). Transcriptional regulation of nitrogen-associated metabolism and growth. Nature 563, 259–264. doi: 10.1038/s41586-018-0656-3
Gonzalez, A., Mendenhall, J., Huo, Y., and Lloyd, A. (2009). TTG1 complex MYBs. MYB5 and TT2, control outer seed coat differentiation. Dev. Biol. 325, 412–421. doi: 10.1016/j.ydbio.2008.10.005
Groot, S. P., Surki, A. A., de Vos, R. C., and Kodde, J. (2012). Seed storage at elevated partial pressure of oxygen, a fast method for analysing seed ageing under dry conditions. Ann. Bot. 110, 1149–1159. doi: 10.1093/aob/mcs198
Gualberti, G., Papi, M., Bellucci, L., Ricci, I., Bouchez, D., Camilleri, C., et al. (2002). Mutations in the Dof zinc finger genes DAG2 and DAG1 influence with opposite effects the germination of Arabidopsis seeds. Plant Cell 14, 1253–1263. doi: 10.1105/tpc.010491
Gupta, S., Malviya, N., Kushwaha, H., Nasim, J., Bisht, N. C., Singh, V. K., et al. (2015). Insights into structural and functional diversity of Dof (DNA binding with one finger) transcription factor. Planta 241, 549–562. doi: 10.1007/s00425-014-2239-3
Gutierrez, L., Van Wuytswinkel, O., Castelain, M., and Bellini, C. (2007). Combined networks regulating seed maturation. Trends Plant Sci. 12, 294–300. doi: 10.1016/j.tplants.2007.06.003
Hagan, N. D., Upadhyaya, N., Tabe, L. M., and Higgins, T. J. V. (2003). The redistribution of protein sulfur in transgenic rice expressing a gene for a foreign, sulfur-rich protein. Plant J. 34, 1–11.
Han, X., Kumar, D., Chen, H., Wu, S., and Kim, J. Y. (2014). Transcription factor-mediated cell-to-cell signalling in plants. J. Exp. Bot. 65, 1737–1749. doi: 10.1093/jxb/ert422
Havé, M., Marmagne, A., Chardon, F., and Masclaux-Daubresse, C. (2017). Nitrogen remobilization during leaf senescence: lessons from Arabidopsis to crops. J. Exp. Bot. 68, 2513–2529. doi: 10.1093/jxb/erw365
Holdsworth, M. J., Bentsink, L., and Soppe, W. J. J. (2008). Molecular networks regulating Arabidopsis seed maturation, after-ripening, dormancy and germination. New Phytol. 179, 33–54. doi: 10.1111/j.1469-8137.2008.02437.x
Hundertmark, M., Buitink, J., Leprince, O., and Hincha, D. (2011). The reduction of seed-specific dehydrins reduces seed longevity in Arabidopsis thaliana. Seed Sci. Res. 21, 165–173.
Kagaya, Y., Toyoshima, R., Okuda, R., Usui, H., Yamamoto, A., and Hattori, T. (2005). LEAFY COTYLEDON1 controls seed storage protein genes through its regulation of FUSCA3 and ABSCISIC ACID INSENSITIVE3. Plant Cell Physiol. 46, 399–406. doi: 10.1093/pcp/pci048
Kim, D., Langmead, B., and Salzberg, S. L. (2015). HISAT: a fast spliced aligner with low memory requirements. Nat. Methods 12, 357–360. doi: 10.1038/nmeth.3317
Kitagawa, M., and Jackson, D. (2017). Plasmodesmata-Mediated cell-to-cell communication in the shoot apical meristem: how stem cells talk. Plants 6:12. doi: 10.3390/plants6010012
Kranner, I., Birtiæ, S., Anderson, K. M., and Pritchard, H. W. (2006). Glutathione half-cell reduction potential: a universal stress marker and modulator of programmed cell death? Free Radic Biol. Med. 40, 2155–2165. doi: 10.1016/j.freeradbiomed.2006.02.013
Krebbers, E., Herdies, L., De Clercq, A., Seurinck, J., Leemans, J., Van Damme, J., et al. (1988). Determination of the processing sites of an Arabidopsis 2S albumin and characterization of the complete gene family. Plant Physiol. 87, 859–866. doi: 10.1104/pp.87.4.859
Le Hir, R., and Bellini, C. (2013). The plant-specific dof transcription factors family: new players involved in vascular system development and functioning in Arabidopsis. Front. Plant Sci. 4:164. doi: 10.3389/fpls.2013.00164
Le, B. H., Cheng, C., Bui, A. Q., Wagmaister, J. A., Henry, K. F., Pelletier, J., et al. (2010). Global analysis of gene activity during Arabidopsis seed development and identification of seed-specific transcription factors. Proc. Natl. Acad. Sci. U.S.A. 107, 8063–8070. doi: 10.1073/pnas.1003530107
Lee, J. Y., and Zhou, J. (2012). “Function and identification of mobile transcription factors,” in Advance in Plant Biology; Short and Long Distance Signaling, eds F. Kragler and M. Hulskamp (New York, NY: Springer), 61–86.
Lee, J. Y., Colinas, J., Wang, J. Y., Mace, D., Ohler, U., and Benfey, P. N. (2006). Transcriptional and posttranscriptional regulation of transcription factor expression in Arabidopsis roots. Proc. Natl. Acad. Sci. U.S.A. 103, 6055–6060. doi: 10.1073/pnas.0510607103
Lee, Y. P., Baek, K. H., Lee, H. S., Kwak, S. S., Bang, J. W., and Kwon, S. Y. (2010). Tobacco seeds simultaneously over-expressing Cu/Zn-superoxide dismutase and ascorbate peroxidase display enhanced seed longevity and germination rates under stress conditions. J. Exp. Bot. 61, 2499–2506. doi: 10.1093/jxb/erq085
Leon-Kloosterziel, K. M., Keijzer, C. J., and Koornneef, M. (1994). A seed shape mutant of arabidopsis that is affected in integument development. Plant Cell 6, 385–392. doi: 10.1105/tpc.6.3.385
Leprince, O., Pellizzaro, A., Berriri, S., and Buitink, J. (2017). Late seed maturation: drying without dying. J. Exp. Bot. 68, 827–841. doi: 10.1093/jxb/erw363
Lhuillier-Sounde’le’, A., Munier-Jolain, N., and Ney, B. (1999). Influence of nitrogen availability on seed nitrogen accumulation in pea. Crop Sci. 39, 1741–1748.
Love, M. I., Huber, W., and Anders, S. (2014). Moderated estimation of fold change and dispersion for RNA-seq data with DESeq2. Genome Biol. 15:550. doi: 10.1186/s13059-014-0550-8
Mae, T. (2004). “Leaf senescence and nitrogen metabolism,” in Plant Cell Death Processes, ed. L. D. Nooden (San Diego, CA: Academic Press), 157–168.
Martin, M. (2011). Cutadapt removes adapter sequences from high-throughput sequencing reads. EMBnet. J. 17, 10–12. doi: 10.1089/cmb.2017.0096
Mi, H., Ebert, D., Muruganujan, A., Mills, C., Albou, L. P., Mushayamaha, T., et al. (2021). PANTHER version 16: a revised family classification, tree-based classification tool, enhancer regions and extensive API. Nucleic Acids Res. 49, D394–D403. doi: 10.1093/nar/gkaa1106
Miranda, M., Borisjuk, L., Tewes, A., Heim, U., Sauer, N., Wobus, U., et al. (2001). Amino acid permeases in developing seeds of Vicia faba L.: expression precedes storage protein synthesis and is regulated by amino acid supply. Plant J. 28, 61–72. doi: 10.1046/j.1365-313x.2001.01129.x
Molina, I., Ohlrogge, J. B., and Pollard, M. (2008). Deposition and localization of lipid polyester in developing seeds of Brassica napus and Arabidopsis thaliana. Plant J. 53, 437–449. doi: 10.1111/j.1365-313X.2007.03348.x
Mönke, G., Seifert, M., Keilwagen, J., Mohr, M., Grosse, I., Hähnel, U., et al. (2012). Toward the identification and regulation of the Arabidopsis thaliana ABI3 regulon. Nucleic Acids Res. 40, 8240–8254. doi: 10.1093/nar/gks594
Moreno-Risueno, M. A., Martínez, M., Vicente-Carbajosa, J., and Carbonero, P. (2007). The family of DOF transcription factors: from green unicellular algae to vascular plants. Mol. Genet Genomics 277, 379–390. doi: 10.1007/s00438-006-0186-9
Nguyen, T. P., Cueff, G., Hegedus, D. D., Rajjou, L., and Bentsink, L. (2015). A role for seed storage proteins in Arabidopsis seed longevity. J. Exp. Bot. 66, 6399–6413. doi: 10.1093/jxb/erv348
Nguyen, T. P., Keizer, P., van Eeuwijk, F., Smeekens, S., and Bentsink, L. (2012). Natural variation for seed longevity and seed dormancy are negatively correlated in Arabidopsis. Plant Physiol. 160, 2083–2092. doi: 10.1104/pp.112.206649
Noguero, M., Atif, R. M., Ochatt, S., and Thompson, R. D. (2013). The role of the DNA-binding One Zinc Finger (DOF) transcription factor family in plants. Plant Sci. Int. J. Exp. Plant Biol. 209, 32–45. doi: 10.1016/j.plantsci.2013.03.016
North, H., Baud, S., Debeaujon, I., Dubos, C., Dubreucq, B., Grappin, P., et al. (2010). Arabidopsis seed secrets unravelled after a decade of genetic and omics-driven research. Plant J. 61, 971–981. doi: 10.1111/j.1365-313X.2009.04095.x
Ogas, J., Kaufmann, S., Henderson, J., and Somerville, C. (1999). PICKLE is a CHD3 chromatin-remodeling factor that regulates the transition from embryonic to vegetative development in Arabidopsis. Proc. Natl. Acad. Sci. U.S.A. 96, 13839–13844. doi: 10.1073/pnas.96.24.13839
Ohto, M. A., Floyd, S. K., Fischer, R. L., Goldberg, R. B., and Harada, J. J. (2009). Effects of APETALA2 on embryo, endosperm, and seed coat development determine seed size in Arabidopsis. Sex Plant Reprod. 22, 277–289. doi: 10.1007/s00497-009-0116-1
Oñate-Sánchez, L., and Vicente-Carbajosa, J. (2008). DNA-free RNA isolation protocols for Arabidopsis thaliana, including seeds and siliques. BMC Res. Notes 1:93. doi: 10.1186/1756-0500-1-93
Parcy, F., Valon, C., Kohara, A., Miséra, S., and Giraudat, J. (1997). The abscisic acid-insensitive3, fusca3, and leafy cotyledon1 loci act in concert to control multiple aspects of Arabidopsis seed development. Plant Cell 9, 1265–1277. doi: 10.1105/tpc.9.8.1265
Pelletier, J. M., Kwong, R. W., Park, S., Le, B. H., Baden, R., Cagliari, A., et al. (2017). LEC1 sequentially regulates the transcription of genes involved in diverse developmental processes during seed development. Proc. Natl. Acad. Sci. U.S.A. 114, E6710–E6719. doi: 10.1073/pnas.1707957114
Popescu, S. C., Popescu, G. V., Bachan, S., Zhang, Z., Gerstein, M., Snyder, M., et al. (2009). MAPK target networks in Arabidopsis thaliana revealed using functional protein microarrays. Genes Dev. 23, 80–92. doi: 10.1101/gad.1740009
Prieto-Dapena, P., Castaño, R., Almoguera, C., and Jordano, J. (2006). Improved resistance to controlled deterioration in transgenic seeds. Plant Physiol. 142, 1102–1112. doi: 10.1104/pp.106.087817
Rajjou, L., and Debeaujon, I. (2008). Seed longevity: survival and maintenance of high germination ability of dry seeds. C R Biol. 331, 796–805. doi: 10.1016/j.crvi.2008.07.021
Rajjou, L., Lovigny, Y., Groot, S. P., Belghazi, M., Job, C., and Job, D. (2008). Proteome-wide characterization of seed aging in Arabidopsis: a comparison between artificial and natural aging protocols. Plant Physiol. 148, 620–641. doi: 10.1104/pp.108.123141
Ramachandran, V., Tobimatsu, Y., Masaomi, Y., Sano, R., Umezawa, T., Demura, T., et al. (2020). Plant-specific Dof transcription factors VASCULAR-RELATED DOF1 and VASCULAR-RELATED DOF2 regulate vascular cell differentiation and lignin biosynthesis in Arabidopsis. Plant Mol Biol. 104, 263–281. doi: 10.1007/s11103-020-01040-9
Reidt, W., Wohlfarth, T., Ellerström, M., Czihal, A., Tewes, A., Ezcurra, I., et al. (2000). Gene regulation during late embryogenesis: the RY motif of maturation-specific gene promoters is a direct target of the FUS3 gene product. Plant J. 21, 401–408. doi: 10.1046/j.1365-313x.2000.00686.x
Renard, J., Martínez-Almonacid, I., Queralta Castillo, I., Sonntag, A., and Hashim, A. (2021). Apoplastic lipid barriers regulated by conserved homeobox transcription factors extend seed longevity in multiple plant species. New Phytol. 231, 679–694. doi: 10.1111/nph.17399
Renard, J., Martínez-Almonacid, I., Sonntag, A., Molina, I., Moya-Cuevas, J., Bissoli, G., et al. (2020a). PRX2 and PRX25, peroxidases regulated by COG1, are involved in seed longevity in Arabidopsis. Plant Cell Environ. 43, 315–326. doi: 10.1111/pce.13656
Renard, J., Niñoles, R., Martínez-Almonacid, I., Gayubas, B., Mateos-Fernández, R., Bissoli, G., et al. (2020b). Identification of novel seed longevity genes related to oxidative stress and seed coat by genome-wide association studies and reverse genetics. Plant Cell Environ. 43, 2523–2539. doi: 10.1111/pce.13822
Righetti, K., Vu, J. L., Pelletier, S., Vu, B. L., Glaab, E., Lalanne, D., et al. (2015). Inference of longevity-related genes from a robust coexpression network of seed maturation identifies regulators linking seed storability to biotic defense-related pathways. Plant Cell 27, 2692–2708. doi: 10.1105/tpc.15.00632
Robinson-Beers, K., Pruitt, R. E., and Gasser, C. S. (1992). Ovule development in Wild-Type Arabidopsis and two female-sterile mutants. Plant Cell 4, 1237–1249. doi: 10.1105/tpc.4.10.1237
Rolletschek, H., Hosein, F., Miranda, M., Heim, U., Götz, K. P., Schlereth, A., et al. (2005). Ectopic expression of an amino acid transporter (VfAAP1) in seeds of Vicia narbonensis and pea increases storage proteins. Plant Physiol. 137, 1236–1249. doi: 10.1104/pp.104.056523
Ruta, V., Longo, C., Lepri, A., De Angelis, V., Occhigrossi, S., Costantino, P., et al. (2020). The DOF transcription factors in seed and seedling development. Plants 9:218. doi: 10.3390/plants9020218
Saez-Aguayo, S., Ralet, M. C., Berger, A., Botran, L., Ropartz, D., Marion-Poll, A., et al. (2013). PECTIN METHYLESTERASE INHIBITOR6 promotes Arabidopsis mucilage release by limiting methylesterification of homogalacturonan in seed coat epidermal cells. Plant Cell 25, 308–323. doi: 10.1105/tpc.112.106575
Santos-Mendoza, M., Dubreucq, B., Baud, S., Parcy, F., Caboche, M., and Lepiniec, L. (2008). Deciphering gene regulatory networks that control seed development and maturation in Arabidopsis. Plant J. 54, 608–620. doi: 10.1111/j.1365-313X.2008.03461.x
Sarrion-Perdigones, A., Palaci, J., Granell, A., and Orzaez, D. (2014). Design and construction of multigenic constructs for plant biotechnology using the GoldenBraid cloning strategy. Methods Mol. Biol. 1116, 133–151. doi: 10.1007/978-1-62703-764-8_10
Sattler, S. E., Gilliland, L. U., Magallanes-Lundback, M., Pollard, M., and DellaPenna, D. (2004). Vitamin E is essential for seed longevity and for preventing lipid peroxidation during germination. Plant Cell 16, 1419–1432.
Schmid, M., Davison, T. S., Henz, S. R., Pape, U. J., Demar, M., Vingron, M., et al. (2005). A gene expression map of Arabidopsis thaliana development. Nat. Genet. 37, 501–516.
Scossa, F., Laudencia-Chingcuanco, D., Anderson, O. D., Vensel, W. H., and Lafiandra, D. (2008). Comparative proteomic and transcriptional profiling of a bread wheat cultivar and its derived transgenic line overexpressing a low molecular weight glutenin subunit gene in the endosperm. Proteomics 8, 2948–2966. doi: 10.1002/pmic.200700861
Skopelitis, D. S., Paranychianakis, N. V., Paschalidis, K. A., Pliakonis, E. D., Delis, I. D., and Yakoumakis, D. I. (2006). Abiotic stress generates ROS that signal expression of anionic glutamate dehydrogenases to form glutamate for proline synthesis in tobacco and grapevine. Plant Cell 18, 2767–2781. doi: 10.1105/tpc.105.038323
Sreenivasulu, N., and Wobus, U. (2013). Seed-development programs: a systems biology-based comparison between dicots and monocots. Annu. Rev. Plant Biol. 64, 189–217. doi: 10.1146/annurev-arplant-050312-120215
Stadler, R., Lauterbach, C., and Sauer, N. (2005). Cell-to-cell movement of green fluorescent protein reveals post-phloem transport in the outer integument and identifies symplastic domains in Arabidopsis seeds and embryos. Plant Physiol. 139, 701–712. doi: 10.1104/pp.105.065607
Sugliani, M., Rajjou, L., Clerkx, E. J., Koornneef, M., and Soppe, W. J. (2009). Natural modifiers of seed longevity in the Arabidopsis mutants abscisic acid insensitive3-5 (abi3-5) and leafy cotyledon1-3 (lec1-3). New Phytol. 184, 898–908. doi: 10.1111/j.1469-8137.2009.03023.x
Supek, F., Bošnjak, M., Škunca, N., and Šmuc, T. (2011). REVIGO summarizes and visualizes long lists of gene ontology terms. PLoS One 6:e21800. doi: 10.1371/journal.pone.0021800
Suzuki, M., Wang, H. H., and McCarty, D. R. (2007). Repression of the LEAFY COTYLEDON 1/B3 regulatory network in plant embryo development by VP1/ABSCISIC ACID INSENSITIVE 3-LIKE B3 genes. Plant Physiol. 143, 902–911. doi: 10.1104/pp.106.092320
Tejedor-Cano, J., Prieto-Dapena, P., Almoguera, C., Carranco, R., Hiratsu, K., Ohme-Takagi, M., et al. (2010). Loss of function of the HSFA9 seed longevity program. Plant Cell Environ. 33, 1408–1417. doi: 10.1111/j.1365-3040.2010.02159.x
Tian, R., Wang, F., Zheng, Q., Niza, E., Downie, A. B., and Perry, S. E. (2020). Direct and indirect targets of the arabidopsis seed transcription factor ABSCISIC ACID INSENSITIVE3. Plant J. 103, 1679–1694. doi: 10.1111/tpj.14854
Uauy, C., Distelfeld, A., Fahima, T., Blechl, A., and Dubcovsky, J. (2006). A NAC Gene regulating senescence improves grain protein, zinc, and iron content in wheat. Science 314, 1298–1301. doi: 10.1126/science.1133649
van der Klei, H., Van Damme, J., Casteels, P., and Krebbers, E. (1993). A fifth 2S albumin isoform is present in Arabidopsis thaliana. Plant Physiol. 101, 1415–1416. doi: 10.1104/pp.101.4.1415
Verma, S., Attuluri, V. P. S., and Robert, H. S. (2022). Transcriptional control of Arabidopsis seed development. Planta 255:90.
Vicente-Carbajosa, J., Moose, S. P., Parsons, R. L., and Schmidt, R. J. (1997). A maize zinc-finger protein binds the prolamin box in zein gene promoters and interacts with the basic leucine zipper transcriptional activator Opaque2. Proc. Natl. Acad. Sci. U.S.A. 94, 7685–7690. doi: 10.1073/pnas.94.14.7685
Wan, L., Ross, A. R., Yang, J., Hegedus, D. D., and Kermode, A. R. (2007). Phosphorylation of the 12 S globulin cruciferin in wild-type and abi1-1 mutant Arabidopsis thaliana (thale cress) seeds. Biochem. J. 404, 247–256. doi: 10.1042/BJ20061569
Wang, F., and Perry, S. E. (2013). Identification of direct targets of FUSCA3, a key regulator of Arabidopsis seed development. Plant Physiol. 161, 1251–1264. doi: 10.1104/pp.112.212282
Wang, H. W., Zhang, B., Hao, Y. J., Huang, J., Tian, A. G., Liao, Y., et al. (2007). The soybean Dof-type transcription factor genes. GmDof4 and GmDof11, enhance lipid content in the seeds of transgenic Arabidopsis plants. Plant J. 52, 716–729. doi: 10.1111/j.1365-313X.2007.03268.x
Winter, D., Vinegar, B., Nahal, H., Ammar, R., Wilson, G. V., and Provart, N. J. (2007). An “Electronic Fluorescent Pictograph” browser for exploring and analyzing large-scale biological data sets. PLoS One 2:e718. doi: 10.1371/journal.pone.0000718
Withana-Gamage, T. S., Hegedus, D. D., Qiu, X., Yu, P. Q., May, T., Lydiate, D., et al. (2013). Characterization of Arabidopsis thaliana lines with altered seed storage protein profiles using synchrotron-powered FT-IR spectromicroscopy. J. Agric. Food Chem. 61, 901–912. doi: 10.1021/jf304328n
Wu, J., Chen, L., Chen, M., Zhou, W., Dong, Q., Jiang, H., et al. (2019). The DOF-Domain transcription factor ZmDOF36 positively regulates starch synthesis in transgenic maize. Front. Plant Sci. 2019:465. doi: 10.3389/fpls.2019.00465
Xi, X., Hu, Z., Nie, X., Meng, M., Xu, H., and Li, J. (2021). Cross Inhibition of MPK10 and WRKY10 participating in the growth of endosperm in Arabidopsis thaliana. Front. Plant Sci. 9:640346. doi: 10.3389/fpls.2021.640346
Yamamoto, A., Yoshii, M., Murase, S., and Fujita, M. (2014). Cell-by-cell developmental transition from embryo to post-germination phase revealed by heterochronic gene expression and ER-body formation in Arabidopsis leafy cotyledon mutants. Plant Cell Physiol. 55, 2112–2125. doi: 10.1093/pcp/pcu139
Yan, A., and Chen, Z. (2017). The pivotal role of abscisic acid signaling during transition from seed maturation to germination. Plant Cell Rep. 36, 689–703. doi: 10.1007/s00299-016-2082-z
Yanagisawa, S. (2016). “Structure, function, and evolution of the dof transcription factor family,” in Plant Transcription Factors, Vol. 12, ed. H. Daniel (Cambridge, MA: Academic Press), 183–197.
Yang, X., Tuskan, G. A., and Cheng, M. Z. (2006). Divergence of the Dof gene families in poplar. Arabidopsis, and rice suggests multiple modes of gene evolution after duplication. Plant Physiol. 142, 820–830. doi: 10.1104/pp.106.083642
Yazdanpanah, F., Maurino, V. G., Mettler-Altmann, T., Buijs, G., Bailly, M. N., Karimi Jashni, M., et al. (2019). NADP-MALIC ENZYME 1 affects germination after seed storage in Arabidopsis thaliana. Plant Cell Physiol. 60, 318–328.
Keywords: seed longevity, seed storage proteins, cruciferin, DOF4.1, transcription factor
Citation: Niñoles R, Ruiz-Pastor CM, Arjona-Mudarra P, Casañ J, Renard J, Bueso E, Mateos R, Serrano R and Gadea J (2022) Transcription Factor DOF4.1 Regulates Seed Longevity in Arabidopsis via Seed Permeability and Modulation of Seed Storage Protein Accumulation. Front. Plant Sci. 13:915184. doi: 10.3389/fpls.2022.915184
Received: 07 April 2022; Accepted: 23 May 2022;
Published: 01 July 2022.
Edited by:
Ewa M. Kalemba, Institute of Dendrology (PAN), PolandReviewed by:
Paola Vittorioso, Sapienza University of Rome, ItalyBarbara A. Halkier, University of Copenhagen, Denmark
Copyright © 2022 Niñoles, Ruiz-Pastor, Arjona-Mudarra, Casañ, Renard, Bueso, Mateos, Serrano and Gadea. This is an open-access article distributed under the terms of the Creative Commons Attribution License (CC BY). The use, distribution or reproduction in other forums is permitted, provided the original author(s) and the copyright owner(s) are credited and that the original publication in this journal is cited, in accordance with accepted academic practice. No use, distribution or reproduction is permitted which does not comply with these terms.
*Correspondence: Regina Niñoles, cmVuaW9yb0BpYm1jcC51cHYuZXM=; Jose Gadea, amdhZGVhdkBpYm1jcC51cHYuZXM=