- Institute of Plant and Microbial Biology, Academia Sinica, Taipei, Taiwan
Cytochrome (Cyt) b559 is a key component of the photosystem II (PSII) complex for its assembly and proper function. Previous studies have suggested that Cytb559 has functional roles in early assembly of PSII and in secondary electron transfer pathways that protect PSII against photoinhibition. In addition, the Cytb559 in various PSII preparations exhibited multiple different redox potential forms. However, the precise functional roles of Cytb559 in PSII remain unclear. Recent site-directed mutagenesis studies combined with functional genomics and biochemical analysis, as well as high-resolution x-ray crystallography and cryo-electron microscopy studies on native, inactive, and assembly intermediates of PSII have provided important new structural and mechanistic insights into the functional roles of Cytb559. This mini-review gives an overview of new exciting results and their significance for understanding the structural and functional roles of Cytb559 in PSII.
Introduction
Cytochrome (Cyt) b559 is an essential component of the photosystem II (PSII) complex for proper functioning and assembly (reviews by Whitmarsh and Pakrasi, 1996; Stewart and Brudvig, 1998; Shinopoulos and Brudvig, 2012; Müh and Zouni, 2015; Chu and Chiu, 2016). Cytb559 is present in the PSII of all oxygenic photosynthetic organisms but is not found in anoxygenic type II reaction centers of photosynthetic bacteria (Majumder and Blankenship, 2015). Thus, Cytb559 likely co-evolved with the oxygen-evolving function of cyanobacteria. Cytb559 is a heme-bridged heterodimer protein that is comprised of 1 α and 1 β subunit (subunits PsbE and PsbF encoded by psbE and psbF, respectively) (Umena et al., 2011; review by Müh and Zouni, 2015). Each subunit provides a histidine ligand for the non-covalently bound heme, which is located near the cytoplasmic side of PSII (Babcock et al., 1985). In contrast, most mono-heme cytochromes are made of a single polypeptide (Majumder and Blankenship, 2015). In addition, the Cytb559 in different PSII preparations features multiple distinct redox potential forms: high potential (HP) with Em + 370–400 mV, intermediate potential (IP) with Em of about 200 mV, and low potential (LP) with Em of about 0–80 mV (Ortega et al., 1988; Thompson et al., 1989; Kaminskaya et al., 1999; Roncel et al., 2001). The redox potential of the HP form in Cytb559 is unusually high for b-type cytochromes. The redox midpoint potentials of most b-type cytochromes were in the range of −225 to +168 mV (Liu et al., 2014). The HP form is typically predominant in native PSII preparations, whereas the IP and LP forms are predominant in less intact or inactive PSII preparations such as Tris-washing treatment, which removes manganese and extrinsic proteins of PSII (Ghanotakis et al., 1986; Thompson et al., 1989; Kaminskaya et al., 1999; Roncel et al., 2003).
Many studies have suggested that Cytb559 may participate in secondary electron transfer pathways that protect PSII against photoinhibition (Heber et al., 1979; Thompson and Brudvig, 1988; Barber and De Las Rivas, 1993; Poulson et al., 1995; Faller et al., 2001; Tracewell and Brudvig, 2008; review by Shinopoulos and Brudvig, 2012). Cytb559 in the HP form may donate its electron via a β-carotene molecule (CarD2) to reduce the highly oxidizing chlorophyll (P680+) in PSII reaction centers under donor-side photoinhibitory conditions. In addition, Cytb559 may accept an electron from the acceptor side of PSII [e.g., or reduced plastoquinones (PQs)] to prevent the formation of reactive oxygen species under acceptor-side photoinhibitory conditions (Nedbal et al., 1992; Barber and De Las Rivas, 1993; Bondarava et al., 2003, 2010). Moreover, previous studies showed that the Cytb559 in tris-treated PSII has superoxide oxidase and reductase activities (Tiwari and Pospíšil, 2009; Pospisil, 2011). However, the precise functional roles of Cytb559 in PSII are still not clear.
Previous mutagenesis studies on the model cyanobacterium Synechocystis sp. PCC 6803 (hereafter Synechocystis), the green alga Chlamydomonas reinhardtii, and tobacco (Nicotiana tabacum) all showed that the assembly of PSII reaction centers requires the presence of both the α and β subunits of Cytb559 (Pakrasi et al., 1988; Morais et al., 1998; Swiatek et al., 2003). In addition, several studies demonstrated that Cytb559 subunits interacted with D2 to form the essential intermediate complex D2 module during the early steps of PSII assembly (Komenda et al., 2004; Kiss et al., 2019). To study the structural and redox roles of the heme coordination of Cytb559 in PSII, a series of site-directed mutants with mutations on histidine heme ligands of Cytb559 was constructed and characterized in the model cyanobacterium Synechocystis and green alga Chlamydomonas (Pakrasi et al., 1991; Morais et al., 2001; Hung et al., 2007; Hamilton et al., 2014). Most of these Cytb559 mutants accumulated only a little active PSII and, therefore, were unable to grow photoautotrophically. These previous findings suggest that proper coordination of the heme cofactor in Cytb559 is important for the assembly or stability of PSII in Synechocystis (Pakrasi et al., 1991; Hung et al., 2007, 2010).
Tandem Gene Amplification Restored PSII Accumulation of Cytb559 Mutant Cyanobacteria
A recent study developed a novel antenna attenuation method that restored photoautotrophic growth and PSII accumulation in several Cytb559 mutant strains of Synechocystis with mutations in His-22 residues (heme ligands) of PsbE and PsbF (Figure 1A; Chiu et al., 2022). Whole-genome sequencing revealed that both types of autotrophic transformants (spontaneously generated in the early study or generated from the new antenna attenuation method in this recent study) carried 5–15 copies of tandem amplifications of chromosomal segments containing the mutated psbEFLJ operon (Figure 1B). Multiple copies of the psbEFLJ operon in these transformants were maintained only during autotrophic growth, whereas the number of copies gradually decreased under photoheterotrophic conditions (Figure 1C). This situation led to a 10- to 20-fold increase in transcript level of the mutated Cytb559 gene (Figure 1D). The resulting overproduction of mutation-destabilized Cytb559 subunits allowed for sufficient PSII accumulation and restored the photoautotrophic growth of the strains. This study demonstrated how tandem gene amplification restored PSII accumulation and photoautotrophic growth in Cytb559 mutants of cyanobacteria, which may be an important adaptive mechanism of cyanobacteria for survival.
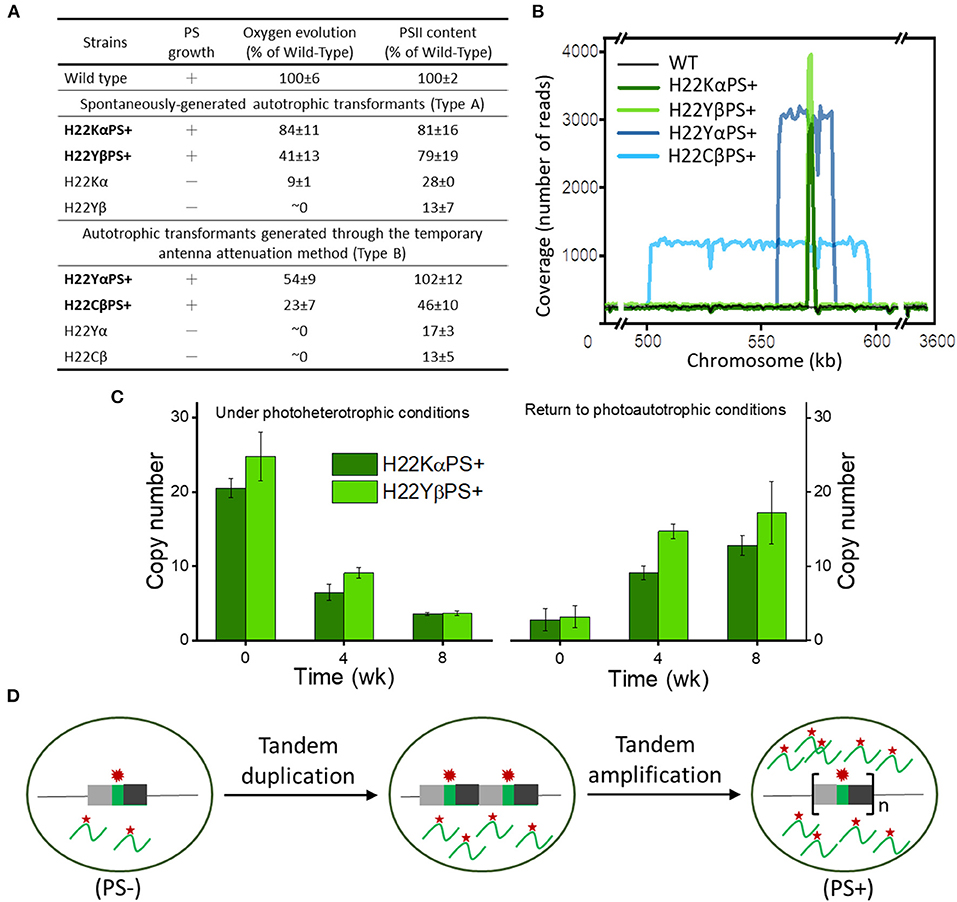
Figure 1. Photosynthetic growth and genetic properties of cytochrome b559 (Cytb559) autotrophic transformants. (A) General properties of 2 distinct types of Cytb559 autotrophic transformants. (B) Copy number, size, and location of repeat elements in autotrophic transformant cells. (C) Quantitative droplet digital polymerase chain reaction (PCR) analysis of the time course of copy number variation of psbEFLJ in autotrophic transformants grown under photoheterotrophic conditions and then returned to photoautotrophic conditions. (D) Model for tandem gene amplification of chromosome segments containing the psbEFLJ operon (asterisk) in autotrophic transformants recovering their photosynthetic growth [Reprinted with permission from Chiu et al. (2022), open access article by the New Phytologist Foundation].
In contrast, in Thermosynechococcus elongatus, the heme coordination of Cytb559 is not required for the assembly of PSII variants with psbA3 as the D1 subunit (Sugiura et al., 2015; Nakamura et al., 2019). Although the H23Aα and H23Mα Cytb559 mutants of T. elongatus assembled only apo-Cytb559 as unambiguously shown by electron paramagnetic resonance (EPR) analysis, they grew photoautotrophically and accumulated active PSII at the wild-type level (Sugiura et al., 2015). The greater structural stability of the thermophilic PSII complex is an important factor why heme ligand mutations do not significantly impair the PSII assembly in T. elongatus.
Structural Determinants of Redox Potentials of Cytb559
One of the distinct features of Cytb559 in PSII is the presence of different redox potential forms. The HP form of Cytb559 predominates in native PSII preparations of plants and Thermosynechococcus. In addition, for some unknown reason, intact PSII preparations from Synechocystis contained primarily the IP form of Cytb559 but lacked the HP form (Ortega et al., 1994; Chiu et al., 2009). Under Tris-washing treatments, inactive PSII preparations from plants and Synechocystis usually contained predominantly the LP form (Thompson et al., 1989; Berthomieu et al., 1992; Mamedov et al., 2007; Chiu et al., 2009), whereas inactive PSII preparations from Thermosynechococcus contained primarily the IP form and lacked the LP form (Roncel et al., 2003). Therefore, the redox properties of Cytb559 in PSII significantly differ in different species.
Structural determinants of the different redox-potential forms of Cytb559 are still not clear. Previous studies suggested that the different redox-potential forms may be due to changes in hydrophobicity of the heme ligation environment (Krishtalik et al., 1993; Roncel et al., 2003), mutual orientation of the planes of histidine heme ligands (Babcock et al., 1985), or protonation or H-bonding pattern of the heme ligation environment (Ortega et al., 1988; Berthomieu et al., 1992; Roncel et al., 2001). A recent cryo-electron microscopy (cryo-EM) study (Kato et al., 2021) presented a 1.95-Å resolution structural model of the native PSII preparation (PSII-D) from Thermosynechococcus, expected to predominantly feature the HP form of Cytb559. The bonding distances for the His–Fe heme ligation of Cytb559 are about 2.1Å (Figure 2A, Supplementary Table 1). The 1.93-Å resolution cryo-EM structural model of intact PSII preparations from Synechocystis (Gisriel et al., 2022), which may predominantly feature the IP form of Cytb559, show an apparent elongation in bonding distances (about 2.4Å) for the His–Fe heme ligation of Cytb559 (Figure 2B, Supplementary Table 1). In addition, the His–Fe bond of the His 22 residue on the β subunit to the heme is slightly tilted from the heme normal (Supplementary Figure 1). The 2.53-Å resolution cryo-EM structural models of inactive PSII preparations (Apo-PSII-M) of Synechocystis (Gisriel et al., 2020), which may predominantly feature the LP form of Cytb559 (Ortega et al., 1994; Chiu et al., 2009), show a further increase in bonding distances (about 2.5–2.6 Å) of His–Fe ligation to the heme, tilting of His–Fe bonds, as well as an apparent alteration in the orientations and electrostatic interactions of heme propionate groups of Cytb559 (Figure 2C, Supplementary Table 1, Supplementary Figure 1B). These structural changes in His–Fe bonds and heme ligation environments of the Cytb559 in inactive PSII are likely induced by conformational changes in the Cytb559 α and β subunits associated with loss of extrinsic polypeptides and psbJ (Gisriel et al., 2020).
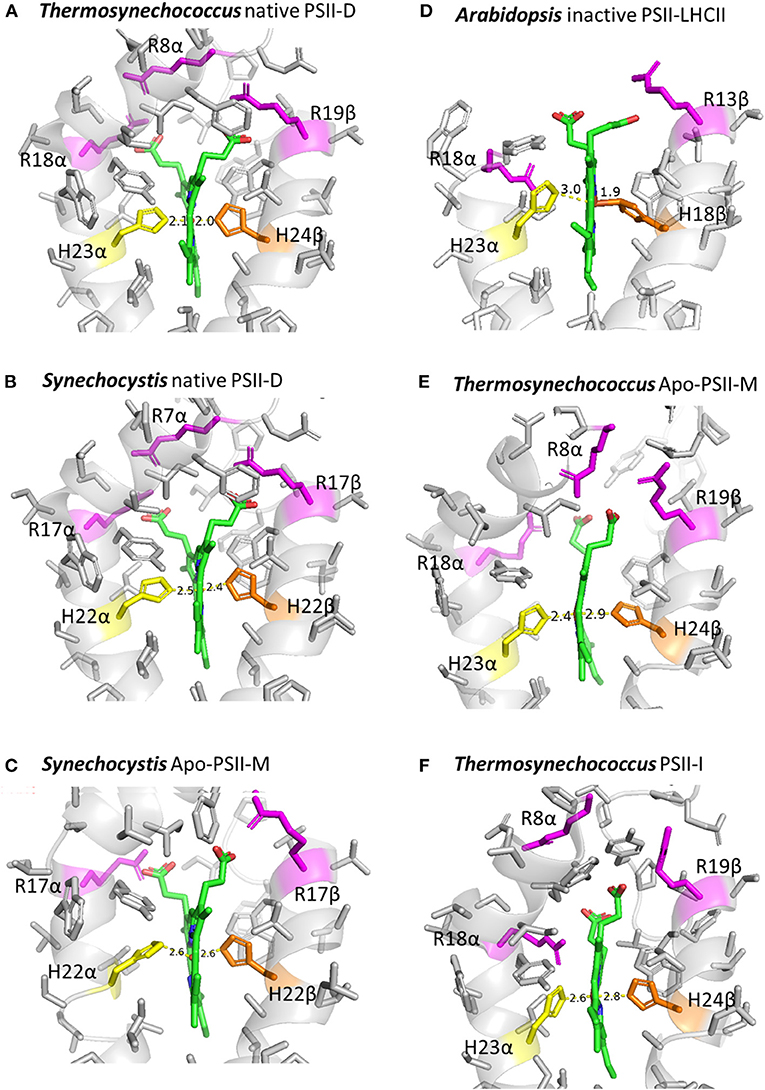
Figure 2. Heme coordination environments of Cytb559 in cryo-EM structural models of different types of PSII preparations. (A) Native PSII dimer (PSII-D) of Thermosynechococcus (PDB 7D1U); (B) native PSII-D of Synechocystis (PDB 7RCV); (C) inactive PSII monomer (Apo-PSII-M) of Synechocystis (PDB 6WJ6); (D) inactive PSII-LHCII supercomplex from Arabidopsis (PDB 7OUI); (E) Apo-PSII-M of Thermosynechococcus (PDB 7NHO); and (F) PSII assembly intermediate (PSII-I) of Thermosynechococcus (PDB 7NHP). The figures were created using PyMol.
In addition, striking changes in the His–Fe ligation as well as orientation and interacting environments of the heme propionates of Cytb559 were observed in the 2.7-Å resolution cryo-EM structural model of inactive LHCII-PSII supercomplex of Arabidopsis (Graça et al., 2021; Figure 2D, Supplementary Table 1). The bonding distances of His-Fe ligations of the heme in Cytb559 of inactive PSII of Arabidopsis were 3 and 1.9 Å. In addition, a recent Cryo-EM study using the ΔpsbJ mutant of Thermosynechococcus reported a structure for the Apo-PSII monomer (Apo-PSII-M) without psbJ (Zabret et al., 2021). The heme-coordination structure of Cytb559 in Apo-PSII-M was distorted. The bonding distances of His-Fe ligations of the heme in Cytb559 of T. elongatus were unevenly elongated from 2.1 Å in the native PSII (Figure 2A) to 2.4 and 2.8Å in Apo-PSII-M (Figure 2E). In addition, a conserved electrostatic interaction between the arginine 18 residue on the beta subunit of Cytb559 and a heme propionate was replaced by the arginine 8 residue on the α subunit of Cytb559 in the Apo-PSII-M of Thermosynechococcus. In contrast, the heme coordination structures of Cytb559 in the active PSII-LHCII supercomplex of pea (Su et al., 2017) and the native PSII monomer of Thermosynechococcus (Yu et al., 2021) were very similar to that in the native PSII dimer of Thermosynechococcus (Supplementary Table 1).
Taken together, the differences in the bonding of His–Fe ligation and the electrostatic environment of the two heme propionate groups of Cytb559 may serve as important structural determinants for different redox forms of Cytb559 in various PSII preparations. These striking structural changes in the heme ligation environment for inactive PSII is expected to change the hydrophobicity of the heme ligation environment (Krishtalik et al., 1993; Roncel et al., 2003) and may also facilitate the binding of exogenous ligands for superoxide oxidase and reductase activities (Tiwari and Pospíšil, 2009; Pospisil, 2011). Of note, a previous FTIR study reported significant structural changes in the environment of a histidine ligand and a propionic group of the heme between the LP and HP forms of Cytb559 (Berthomieu et al., 1992). In addition, previous site-directed mutant results also demonstrated that changes in bonding of His–Fe heme ligation and the electrostatic environment of the heme propionates of Cytb559 strongly influenced the ratio of different redox forms of Cytb559 in mutant PSII (Hung et al., 2010; Chiu et al., 2013; Guerrero et al., 2014). However, because the peripheral location of Cytb559 in the PSII complex may result in uncertainty on heme iron coordination structure (refer to the B values in Supplementary Table 1), structural models at higher resolution may be required to validate whether the variations in the structure of His–Fe ligation are significant in current lower-resolution models of inactive and assembly intermediates of PSII (Figure 2, Supplementary Table 1).
PsbY Protein is Required for the High Redox Potential Form of Cytb559 in Arabidopsis
A recent study on ΔpsbY Arabidopsis mutants showed that Cytb559 was present in only its oxidized LP form in the absence of the PsbY protein (von Sydow et al., 2016). No HP form of Cytb559 was found in ΔpsbY Arabidopsis mutants. In contrast, wild-type and complement mutant plants contained about 50% of the HP form of Cytb559. PsbY was proposed to protect the heme of Cytb559 against reducing agents or affect the coordination environment of Cytb559, thus leading to changes in its redox properties (von Sydow et al., 2016). The steady-state oxygen evolution activities in mutant plants were comparable to that in wild-type plants under normal light conditions but mutant plants were more susceptible to photoinhibition than the wild type under high light conditions (von Sydow et al., 2016).
A Thylakoid Membrane-Bound Rubredoxin May Act Together With Cytb559 in De Novo Assembly and Repair of PSII
A conserved thylakoid membrane-bound rubredoxin (RBD1 in photosynthetic eukaryotes and RubA in cyanobacteria) is required for PSII biogenesis in diverse oxygenic photoautotrophs (Calderon et al., 2013; García-Cerdán et al., 2019; Kiss et al., 2019; Che et al., 2022). RubA-deficient mutant strains of Synechocystis were unable to maintain photoautotrophic growth under fluctuating light and showed severe defects in assembly of the heterodimeric D1/D2 reaction center complex (Kiss et al., 2019). A recent study on RBD1 mutants using C. reinhardtii indicated that the transmembrane domain of RBD1 is essential for de novo PSII assembly, and that its rubredoxin domain is involved in PSII repair (García-Cerdán et al., 2019). In addition, the rubredoxin domain of RBD1 (and RubA) is exposed to the cytoplasm and exhibits a redox midpoint potential of +114 mV. Reduction of RBD1 content can be mediated by ferredoxin-NADP+ reductase in vitro (García-Cerdán et al., 2019). These results suggest that RBD1 (and RubA) may act together with Cytb559 to protect the intermediates of PSII reaction center complexes against photooxidative damage during de novo assembly and repair (García-Cerdán et al., 2019; Kiss et al., 2019).
Psb28 Protein Binds to Cytb559 in the RC47 Complex During the Assembly of PSII
A recent study that conducted chemical cross-linking combined with mass spectrometry predicted the location of Psb28 to be in close proximity to the N-terminal domain of the Cytb559 protein (Weisz et al., 2017). In addition, this study proposed a protective role for Psb28, whereby it blocks electron transport in the acceptor side of PSII to protect the RC47 complex against excess photodamage during the assembly process. Another recent study, which conducted cryo-EM, solved the structure of the PSII assembly intermediate from a deletion strain of the psbJ of T. elongatus (Zabret et al., 2021). The deletion of PsbJ stalled PSII assembly at a specific transition and induced the accumulation of assembly factors Psb27 and Psb28. The cryo-EM map (2.94Å) of PSII-I (for the PSII intermediate) provided a snapshot of the attachment of the CP43 module to the pre-assembled RC47 complex (Zabret et al., 2021). This PSII-I contains 3 assembly factors (Psb27, Psb28, and Psb34). In the structure of PSII-I, Psb28 binds on cytosolic faces of the D1 and D2 subunits, directly above the QB binding site. The binding of Psb28 induced large conformational changes at the PSII acceptor sideof the RC47 complex, which distorted the QBbinding pocket and replaced the bicarbonate ligand of non-heme iron with glutamate. This distinct structural motif is also present in reaction centers of non-oxygenic photosynthetic bacteria (Zabret et al., 2021). These results reveal the structural and functional roles of psb28 in protecting the RC47 complex against damage during PSII assembly. Furthermore, the structure of the heme coordination of Cytb559 in PSII-I was significantly distorted. The bonding distances for axial heme ligations of Cytb559 were elongated (2.6 and 2.8Å), and the electrostatic interactions between two propionate groups of the heme and 2 conserved arginine residues were weakened or broken (Figure 2F, Supplementary Table 1).
The QC Site May Be Involved in Modulating Short-Term Light Responses in PSII of Cyanobacteria
A previous study on the 2.9-Å resolution PSII crystal structure revealed the binding of a PQ molecule, QC, in a hydrophobic cavity near Cytb559 (Guskov et al., 2009). Previous studies proposed that the QC site may be involved in exchange of PQ/plastoquinol (PQH2) on the QB site from the pool (Guskov et al., 2009; Müh et al., 2012) or in modulating the redox potential and reactivity of Cytb559 (Kruk and Strzałka, 1999; Kruk and Strzalka, 2001; Kaminskaya et al., 2007a,b; Bondarava et al., 2010). However, the binding of PQ to the QC site appeared to be weak or transient (Koji and Takumi, 2014; Van Eerden et al., 2017), and the QC molecule was not detected in recent high-resolution crystal structure models of native PSII from T. vulcanus (Umena et al., 2011; Suga et al., 2015).
Several QC-site Synechocystis mutant strains (e.g., S28Aβ, V32Fβ, and A16FJ) showed significantly higher photosynthesis growth rate and biomass accumulation than wild-type strains (Huang et al., 2016, 2018). In addition, the ratios of redox potential forms of Cytb559 for QC-site mutant PSII core complexes were similar to those for the wild type. Furthermore, QC-site mutant cells had distinct effects on short-term light responses (state transition and blue light-inducing non-photochemical quenching) (Huang et al., 2016, 2018). Taken together, the results suggest that the mutations on the QC site of PSII may modulate short-term light adaptations of the photosynthetic apparatus in Synechocystis.
Conclusions and Perspectives
Recent mutagenesis studies combined with high-resolution protein crystallography and cryo-EM structural analysis as well as functional genomics and biochemical analysis have greatly advanced our understanding of the structural and functional roles of Cytb559 in the assembly, proper function, and photoprotection of PSII. Studies have revealed possible structural determinants for different redox forms of Cytb559 in various PSII preparations. In addition, several assembly factors and protein subunits may act together with Cytb559 to protect the intermediates of PSII reaction center complexes during de novo assembly and repair. These integrated approaches may lead to the final proof of the molecular mechanisms of Cytb559 in PSII.
Author Contributions
Y-FC and H-AC wrote the article. H-AC acquired the funding and supervised the project. Both authors read and approved the manuscript.
Funding
This study was supported by Academia Sinica for H-AC.
Conflict of Interest
The authors declare that the research was conducted in the absence of any commercial or financial relationships that could be construed as a potential conflict of interest.
Publisher's Note
All claims expressed in this article are solely those of the authors and do not necessarily represent those of their affiliated organizations, or those of the publisher, the editors and the reviewers. Any product that may be evaluated in this article, or claim that may be made by its manufacturer, is not guaranteed or endorsed by the publisher.
Acknowledgments
We thank Dr. Tzu-ping Ko of Academia Sinica Protein Clinic for the assistance with interpretations of Cryo-EM structural models.
Supplementary Material
The Supplementary Material for this article can be found online at: https://www.frontiersin.org/articles/10.3389/fpls.2022.914922/full#supplementary-material
Abbreviations
Car, β-carotene; Cryo-EM, cryo-electron microscopy; Cytb559, cytochrome b559; EPR, electron paramagnetic resonance; LHCII, light-harvesting complex II; HP, high potential; IP, intermediate potential; LP, low potential; PQ, plastoquinone; PQH2, plastoquinol; PSII, photosystem II; QB, the secondary quinone electron acceptor in PSII; QC, the third plastoquinone-binding site in PSII.
References
Babcock, G. T., Widger, W. R., Cramer, W. A., Oertling, W. A., and Metz, J. G. (1985). Axial ligands of chloroplast cytochrome-b-559: identification and requirement for a heme-cross-linked polypeptide structure. Biochemistry 24, 3638–3645. doi: 10.1021/bi00335a036
Barber, J., and De Las Rivas, J. (1993). A functional model for the role of cytochrome b559 in the protection against donor and acceptor side photoinhibition. Proc. Nat. Acad. Sci. U.S.A. 90, 10942–10946. doi: 10.1073/pnas.90.23.10942
Berthomieu, C., Boussac, A., Maentele, W., Breton, J., and Nabedryk, E. (1992). Molecular changes following oxidoreduction of cytochrome b559 characterized by Fourier transform infrared difference spectroscopy and electron paramagnetic resonance: photooxidation in photosystem II and electrochemistry of isolated cytochrome b559 and iron protoporphyrin IX-bisimidazole model compounds. Biochemistry 31, 11460–11471. doi: 10.1021/bi00161a026
Bondarava, N., De Pascalis, L., Al-Babili, S., Goussias, C., Golecki, J. R., Beyer, P., et al. (2003). Evidence that cytochrome b559 mediates the oxidation of reduced plastoquinone in the dark. J. Biol. Chem. 278, 13554–13560. doi: 10.1074/jbc.M212842200
Bondarava, N., Gross, C. M., Mubarakshina, M., Golecki, J. R., Johnson, G. N., and Krieger-Liszkay, A. (2010). Putative function of cytochrome b559 as a plastoquinol oxidase. Physiol. Plant. 138, 463–473. doi: 10.1111/j.1399-3054.2009.01312.x
Calderon, R. H., García-Cerdán, J. G., Malnoë, A., Cook, R., Russell, J. J., Gaw, C., et al. (2013). A conserved rubredoxin is necessary for photosystem II accumulation in diverse oxygenic photoautotrophs. J. Biol. Chem. 288, 26688–26696. doi: 10.1074/jbc.M113.487629
Che, L., Meng, H., Ruan, J., Peng, L., and Zhang, L. (2022). Rubredoxin 1 is required for formation of the functional photosystem II core complex in Arabidopsis thaliana. Front. Plant Sci. 13,824358–824358. doi: 10.3389/fpls.2022.824358
Chiu, Y.-F., Chen, Y.-H., Roncel, M., Dilbeck, P. L., Huang, J.-Y., Ke, S.-C., et al. (2013). Spectroscopic and functional characterization of cyanobacterium Synechocystis PCC 6803 mutants on the cytoplasmic-side of cytochrome b559 in photosystem II. Biochim. Biophys. Acta Bioenerg. 1827, 507–519. doi: 10.1016/j.bbabio.2013.01.016
Chiu, Y.-F., Fu, H.-Y., Skotnicová, P., Lin, K.-M., Komenda, J., and Chu, H.-A. (2022). Tandem gene amplification restores photosystem II accumulation in cytochrome b559 mutants of cyanobacteria. New Phytol. 233, 766–780. doi: 10.1111/nph.17785
Chiu, Y.-F., Lin, W.-C., Wu, C.-M., Chen, Y.-H., Hung, C.-H., Ke, S.-C., et al. (2009). Identification and characterization of a cytochrome b559 Synechocystis 6803 mutant spontaneously generated from DCMU-inhibited photoheterotrophical growth conditions. Biochim. Biophys. Acta Bioenerg. 1787, 1179–1188. doi: 10.1016/j.bbabio.2009.05.007
Chu, H.-A., and Chiu, Y.-F. (2016). The roles of cytochrome b559 in assembly and photoprotection of photosystem II revealed by site-directed mutagenesis studies. Front. Plant Sci. 6, 1261–1267. doi: 10.3389/fpls.2015.01261
Faller, P., Pascal, A., and Rutherford, A. W. (2001). β-carotene redox reactions in photosystem II: electron transfer pathway. Biochemistry 40, 6431–6440. doi: 10.1021/bi0026021
García-Cerdán, J. G., Furst, A. L., McDonald, K. L., Schünemann, D., Francis, M. B., and Niyogi, K. K. (2019). A thylakoid membrane-bound and redox-active rubredoxin (RBD1) functions in de novo assembly and repair of photosystem II. Proc. Nat. Acad. Sci. U.S.A. 116, 16631–16640. doi: 10.1073/pnas.1903314116
Ghanotakis, D. E., Yocum, C. F., and Babcock, C. T. (1986). ESR spectroscopy demonstrates that cytochrome b559 remains low potential in Ca2+-reactivated, salt-washed PSII particles. Photosyn. Res. 9, 125–134. doi: 10.1007/BF00029738
Gisriel, C. J., Wang, J., Liu, J., Flesher, D. A., Reiss, K. M., Huang, H.-L., et al. (2022). High-resolution cryo-electron microscopy structure of photosystem II from the mesophilic cyanobacterium, Synechocystis sp. PCC 6803. Proc. Natl. Acad. Sci. U.S.A. 119, e2116765118. doi: 10.1073/pnas.2116765118
Gisriel, C. J., Zhou, K., Huang, H.-L., Debus, R. J., Xiong, Y., and Brudvig, G. W. (2020). Cryo-EM structure of monomeric photosystem II from Synechocystis sp. PCC 6803 lacking the water-oxidation complex. Joule 4, 2131–2148. doi: 10.1016/j.joule.2020.07.016
Graça, A. T., Hall, M., and Persson, K. (2021). High-resolution model of Arabidopsis Photosystem II reveals the structural consequences of digitonin-extraction. Sci Rep. 11, 15534. doi: 10.1038/s41598-021-94914-x
Guerrero, F., Zurita, J. L., Roncel, M., Kirilovsky, D., and Ortega, J. M. (2014). The role of the high potential form of the cytochrome b559: study of Thermosynechococcus elongatus mutants. Biochim. Biophys. Acta Bioenerg. 1837, 908–919. doi: 10.1016/j.bbabio.2014.02.024
Guskov, A., Kern, J., Gabdulkhakov, A., Broser, M., Zouni, A., and Saenger, W. (2009). Cyanobacterial photosystem II at 2.9-Å resolution and the role of quinones, lipids, channels and chloride. Nat. Struct. Mol. Biol. 16, 334–342. doi: 10.1038/nsmb.1559
Hamilton, M. L., Franco, E., Deák, Z., Schlodder, E., Vass, I., and Nixon, P. J. (2014). Investigating the photoprotective role of cytochrome b-559 in photosystem II in a mutant with altered ligation of the haem. Plant Cell Physiol. 55, 1276–1285. doi: 10.1093/pcp/pcu070
Heber, U., Kirk, M. R., and Boardman, N. K. (1979). Photoreactions of cytochrome b-559 and cyclic electron flow in photosystem II of intact chloroplasts. Biochim. Biophys. Acta Bioenerg. 546, 292–306. doi: 10.1016/0005-2728(79)90047-1
Huang, J.-Y., Chiu, Y.-F., Ortega, J. M., Wang, H.-T., Tseng, T.-S., Ke, S.-C., et al. (2016). Mutations of cytochrome b559 and PsbJ on and near the QC site in photosystem ii influence the regulation of short-term light response and photosynthetic growth of the cyanobacterium Synechocystis sp. PCC 6803. Biochemistry 55, 2214–2226. doi: 10.1021/acs.biochem.6b00133
Huang, J. Y., Hung, N. T., Lin, K. M., Chiu, Y. F., and Chu, H. A. (2018). Regulating photoprotection improves photosynthetic growth and biomass production in QC-site mutant cells of the cyanobacterium Synechocystis sp. PCC 6803. Photosynthetica 56, 192–199. doi: 10.1007/s11099-018-0765-0
Hung, C.-H., Huang, J.-Y., Chiu, Y.-F., and Chu, H.-A. (2007). Site-directed mutagenesis on the heme axial-ligands of cytochrome b559 in photosystem II by using cyanobacteria Synechocystis PCC 6803. Biochim. Biophys. Acta Bioenerg. 1767, 686–693. doi: 10.1016/j.bbabio.2007.02.016
Hung, C. H., Hwang, H. J., Chen, Y. H., Chiu, Y. F., Ke, S. C., Burnap, R. L., et al. (2010). Spectroscopic and functional characterizations of cyanobacterium Synechocystis PCC 6803 mutants on and near the heme axial ligand of cytochrome b559 in photosystem II. J. Biol. Chem. 285, 5653–5663. doi: 10.1074/jbc.M109.044719
Kaminskaya, O., Kurreck, J., Irrgang, K.-D., Renger, G., and Shuvalov, V. A. (1999). Redox and spectral properties of cytochrome b559 in different preparations of photosystem II. Biochemistry 38, 16223–16235. doi: 10.1021/bi991257g
Kaminskaya, O., Shuvalov, V. A., and Renger, G. (2007a). Evidence for a novel quinone-binding site in the photosystem II (PS II) complex that regulates the redox potential of cytochrome b559. Biochemistry 46, 1091–1105. doi: 10.1021/bi0613022
Kaminskaya, O., Shuvalov, V. A., and Renger, G. (2007b). Two reaction pathways for transformation of high potential cytochrome b559 of PS II into the intermediate potential form. Biochim. Biophys. Acta Bioenerg. 1767, 550–558. doi: 10.1016/j.bbabio.2007.02.005
Kato, K., Miyazaki, N., Hamaguchi, T., Nakajima, Y., Akita, F., Yonekura, K., et al. (2021). High-resolution cryo-EM structure of photosystem II reveals damage from high-dose electron beams. Commun. Biol. 4, 382–392. doi: 10.1038/s42003-021-01919-3
Kiss, É., Knoppová, J., Aznar, G. P., Piln,ý, J., Yu, J., Halada, P., et al. (2019). A Photosynthesis-specific rubredoxin-like protein is required for efficient association of the D1 and D2 proteins during the initial steps of photosystem II assembly. Plant Cell 31, 2241–2258. doi: 10.1105/tpc.19.00155
Koji, H., and Takumi, N. (2014). Molecular interactions of the quinone electron acceptors QA, QB, and QC in photosystem II as studied by the fragment molecular orbital method. Photosyn. Res. 120, 113–123. doi: 10.1007/s11120-012-9787-9
Komenda, J., Reisinger, V., Muller, B. C., Dobakova, M., Granvogl, B., and Eichacker, L. A. (2004). Accumulation of the D2 protein is a key regulatory step for assembly of the photosystem II reaction center complex in Synechocystis PCC 6803. J. Biol. Chem. 279, 48620–48629. doi: 10.1074/jbc.M405725200
Krishtalik, L. I., Tae, G. S., Cherepanov, D. A., and Cramer, W. A. (1993). The redox properties of cytochromes b imposed by the membrane electrostatic environment. Biophys. J. 65, 184–195. doi: 10.1016/S0006-3495(93)81050-6
Kruk, J., and Strzałka, K. (1999). Dark reoxidation of the plastoquinone-pool is mediated by the low-potential form of cytochrome b-559 in spinach thylakoids. Photosyn. Res. 62, 273–279. doi: 10.1023/A:1006374319191
Kruk, J., and Strzalka, K. (2001). Redox changes of cytochrome b(559) in the presence of plastoquinones. J. Biol. Chem. 276, 86–91. doi: 10.1074/jbc.M003602200
Liu, J., Chakraborty, S., Hosseinzadeh, P., Yu, Y., Tian, S., Petrik, I., et al. (2014). Metalloproteins containing cytochrome, iron-sulfur, or copper redox centers. Chem. Rev. 114, 4366–4469. doi: 10.1021/cr400479b
Majumder, E. L., and Blankenship, R. E. (2015). “The diversity of photosynthetic cytochromes,” in Cytochrome Complexes: Evolution, Structures, Energy Transduction, and Signaling, eds W. A. Cramer, and T. Kallas (Dordrecht: Springer). doi: 10.1007/978-94-017-7481-9_3
Mamedov, F., Gadjieva, R., and Styring, S. (2007). Oxygen-induced changes in the redox state of the cytochrome b559 in photosystem II depend on the integrity of the Mn cluster. Physiol. Plant 131, 41–49. doi: 10.1111/j.1399-3054.2007.00938.x
Morais, F., Barber, J., and Nixon, P. J. (1998). The chloroplast-encoded α subunit of cytochrome b-559 is required for assembly of the photosystem two complex in both the light and the dark in Chlamydomonas reinhardtii. J. Biol. Chem. 273, 29315–29320. doi: 10.1074/jbc.273.45.29315
Morais, F., Kuhn, K., Stewart, D. H., Barber, J., Brudvig, G. W., and Nixon, P. J. (2001). Photosynthetic water oxidation in cytochrome b(559) mutants containing a disrupted heme-binding pocket. J. Biol. Chem. 276, 31986–31993. doi: 10.1074/jbc.M103935200
Müh, F., Glöckner, C., Hellmich, J., and Zouni, A. (2012). Light-induced quinone reduction in photosystem II. Biochim. Biophys. Acta Bioenerg. 1817, 44–65. doi: 10.1016/j.bbabio.2011.05.021
Müh, F., and Zouni, A. (2015). “Cytochrome b559 in photosystem II,” in Cytochrome Complexes: Evolution, Structures, Energy Transduction, and Signaling, eds W. A. Cramer, and T. Kallas (Dordrecht: Springer). doi: 10.1007/978-94-017-7481-9_8
Nakamura, M., Boussac, A., and Sugiura, M. (2019). Consequences of structural modifications in cytochrome b559 on the electron acceptor side of photosystem II. Photosyn. Res. 139, 475–486. doi: 10.1007/s11120-018-0521-0
Nedbal, L., Samson, G., and Whitmarsh, J. (1992). Redox state of a one-electron component controls the rate of photoinhibition of photosystem II. Proc. Nat. Acad. Sci. U.S.A. 89, 7929–7933. doi: 10.1073/pnas.89.17.7929
Ortega, J. M., Hervás, M., De La Rosa, M. A., and Losada, M. (1994). Redox properties of cytochrome b559 in photosynthetic membranes from the cyanobacterium Synechocystis sp. PCC 6803. J. Plant Physiol. 144, 454–461. doi: 10.1016/S0176-1617(11)82122-X
Ortega, J. M., Hervás, M, and Losada, M. (1988). Redox and acid-base characterization of cytochrome b-559 in photosystem II particles. Euro. J. Biochem. 171, 449–455. doi: 10.1111/j.1432-1033.1988.tb13810.x
Pakrasi, H. B., Ciechi, P. D., and Whitmarsh, J. (1991). Site directed mutagenesis of the heme axial ligands of cytochrome b559 affects the stability of the photosystem II complex. EMBO J. 10, 1619–1627. doi: 10.1002/j.1460-2075.1991.tb07684.x
Pakrasi, H. B., Williams, J. G., and Arntzen, C. J. (1988). Targeted mutagenesis of the psbE and psbF genes blocks photosynthetic electron transport: evidence for a functional role of cytochrome b559 in photosystem II. EMBO J. 7, 325–332. doi: 10.1002/j.1460-2075.1988.tb02816.x
Pospisil, P. (2011). Enzymatic function of cytochrome b559 in photosystem II. J. Photochem. Photobiol. B Biol. 104, 341–347. doi: 10.1016/j.jphotobiol.2011.02.013
Poulson, M., Samson, G., and Whitmarsh, J. (1995). Evidence that cytochrome b559 protects photosystem II against photoinhibition. Biochemistry 34, 10932–10938. doi: 10.1021/bi00034a027
Roncel, M., Boussac, A., Zurita, J., Bottin, H., Sugiura, M., Kirilovsky, D., et al. (2003). Redox properties of the photosystem II cytochromes b559 and c550 in the cyanobacterium Thermosynechococcus elongatus. J. Biol. Inorgan. Chem. 8, 206–216. doi: 10.1007/s00775-002-0406-7
Roncel, M., Ortega, J. M., and Losada, M. (2001). Factors determining the special redox properties of photosynthetic cytochrome b559. Euro. J. Biochem. 268, 4961–4968. doi: 10.1046/j.0014-2956.2001.02427.x
Shinopoulos, K. E., and Brudvig, G. W. (2012). Cytochrome b559 and cyclic electron transfer within photosystem II. Biochim. Biophys. Acta Bioenerg. 1817, 66–75. doi: 10.1016/j.bbabio.2011.08.002
Stewart, D. H., and Brudvig, G. W. (1998). Cytochrome b559 of photosystem II. Biochim. Biophys. Acta Bioenerg. 1367, 63–87. doi: 10.1016/S0005-2728(98)00139-X
Su, X., Ma, J., Wei, X., Cao, P., Zhu, D., Chang, W., et al. (2017). Structure and assembly mechanism of plant C2S2M2-type PSII-LHCII supercomplex. Science 357, 815–820. doi: 10.1126/science.aan0327
Suga, M., Akita, F., Hirata, K., Ueno, G., Murakami, H., Nakajima, Y., et al. (2015). Native structure of photosystem II at 1.95 Å resolution viewed by femtosecond X-ray pulses. Nature 517, 99–103. doi: 10.1038/nature13991
Sugiura, M., Nakamura, M., Koyama, K., and Boussac, A. (2015). Assembly of oxygen-evolving photosystem II efficiently occurs with the apo-Cytb559 but the holo-Cytb559 accelerates the recovery of a functional enzyme upon photoinhibition. Biochim. Biophys. Acta Bioenerg. 1847, 276–285. doi: 10.1016/j.bbabio.2014.11.009
Swiatek, M., Regel, R. E., Meurer, J., Wanner, G., Pakrasi, H. B., Ohad, I., et al. (2003). Effects of selective inactivation of individual genes for low-molecular-mass subunits on the assembly of photosystem II, as revealed by chloroplast transformation: the psbEFLJ operon in Nicotiana tabacum. Mol. Genet. Genom. 268, 699–710. doi: 10.1007/s00438-002-0791-1
Thompson, L. K., and Brudvig, G. W. (1988). Cytochrome b-559 may function to protect photosystem II from photoinhibition. Biochemistry 27, 6653–6658. doi: 10.1021/bi00418a002
Thompson, L. K., Miller, A. F., Buser, C. A., De Paula, J. C., and Brudvig, G. W. (1989). Characterization of the multiple forms of cytochrome b559 in photosystem II. Biochemistry 28, 8048–8056. doi: 10.1021/bi00446a012
Tiwari, A., and Pospíšil, P. (2009). Superoxide oxidase and reductase activity of cytochrome b559 in photosystem II. Biochim. Biophys. Acta Bioenerg. 1787, 985–994. doi: 10.1016/j.bbabio.2009.03.017
Tracewell, C. A., and Brudvig, G. W. (2008). Characterization of the secondary electron-transfer pathway intermediates of photosystem II containing low-potential cytochrome b559. Photosyn. Res. 98, 189–197. doi: 10.1007/s11120-008-9360-8
Umena, Y., Kawakami, K., Shen, J. R., and Kamiya, N. (2011). Crystal structure of oxygen-evolving photosystem II at a resolution of 1.9 Å. Nature 473, 55–60. doi: 10.1038/nature09913
Van Eerden, F. J., Melo, M. N., Frederix, P. W. J. M., Periole, X., and Marrink, S. J. (2017). Exchange pathways of plastoquinone and plastoquinol in the photosystem II complex. Nat. Commun. 8, 15214–15221. doi: 10.1038/ncomms15214
von Sydow, L., Schwenkert, S., Meurer, J., Funk, C., Mamedov, F., and Schröder, W. P. (2016). The PsbY protein of arabidopsis photosystem II is important for the redox control of cytochrome b559. Biochim. Biophys. Acta Bioenerg. 1857, 1524–1533. doi: 10.1016/j.bbabio.2016.05.004
Weisz, D. A., Liu, H., Zhang, H., Thangapandian, S., Tajkhorshid, E., Gross, M. L., et al. (2017). Mass spectrometry-based cross-linking study shows that the Psb28 protein binds to cytochrome b559 in photosystem II. Proc. Nat. Acad. Sci. U.S.A. 114, 2224–2229. doi: 10.1073/pnas.1620360114
Whitmarsh, J., and Pakrasi, H. (1996). “Formand function of cytochrome b559” in Oxygenic Photosynthesis: The Light Reactions, eds D. Ort, and C. Yocum (Dordrecht:Springer), 249–264. doi: 10.1007/0-306-48127-8_13
Yu, H., Hamaguchi, T., Nakajima, Y., Kato, K., Kawakami, K., Akita, F., et al. (2021). Cryo-EM structure of monomeric photosystem II at 2.78 Å resolution reveals factors important for the formation of dimer. Biochim. Biophys. Acta Bioenerg. 1862, 148471–148480. doi: 10.1016/j.bbabio.2021.148471
Keywords: photosynthesis, photosystem II, cytochrome b559, site-directed mutagenesis, photoprotection, photoinhibition
Citation: Chiu Y-F and Chu H-A (2022) New Structural and Mechanistic Insights Into Functional Roles of Cytochrome b559 in Photosystem II. Front. Plant Sci. 13:914922. doi: 10.3389/fpls.2022.914922
Received: 07 April 2022; Accepted: 27 April 2022;
Published: 08 June 2022.
Edited by:
Harvey J. M. Hou, Alabama State University, United StatesReviewed by:
Alain Boussac, UMR9198 Institut de Biologie Intégrative de la Cellule (I2BC), FranceGary Brudvig, Yale University, United States
Copyright © 2022 Chiu and Chu. This is an open-access article distributed under the terms of the Creative Commons Attribution License (CC BY). The use, distribution or reproduction in other forums is permitted, provided the original author(s) and the copyright owner(s) are credited and that the original publication in this journal is cited, in accordance with accepted academic practice. No use, distribution or reproduction is permitted which does not comply with these terms.
*Correspondence: Hsiu-An Chu, Q2h1aGFAZ2F0ZS5zaW5pY2EuZWR1LnR3