- 1Division of Crop Biotechnics, Department of Biosystems, KU Leuven, Leuven, Belgium
- 2Division of Mechatronics, Biostatistics and Sensors, Department of Biosystems, KU Leuven, Leuven, Belgium
- 3KU Leuven Plant Institute, Heverlee, Belgium
- 4Laboratory of Molecular Plant Biology, Department of Biology, KU Leuven, Leuven, Belgium
- 5Flanders Centre of Postharvest Technology, Leuven, Belgium
The necrotrophic fungus Botrytis cinerea is a major threat to strawberry cultivation worldwide. By screening different Fragaria vesca genotypes for susceptibility to B. cinerea, we identified two genotypes with different resistance levels, a susceptible genotype F. vesca ssp. vesca Tenno 3 (T3) and a moderately resistant genotype F. vesca ssp. vesca Kreuzkogel 1 (K1). These two genotypes were used to identify the molecular basis for the increased resistance of K1 compared to T3. Fungal DNA quantification and microscopic observation of fungal growth in woodland strawberry leaves confirmed that the growth of B. cinerea was restricted during early stages of infection in K1 compared to T3. Gene expression analysis in both genotypes upon B. cinerea inoculation suggested that the restricted growth of B. cinerea was rather due to the constitutive resistance mechanisms of K1 instead of the induction of defense responses. Furthermore, we observed that the amount of total phenolics, total flavonoids, glucose, galactose, citric acid and ascorbic acid correlated positively with higher resistance, while H2O2 and sucrose correlated negatively. Therefore, we propose that K1 leaves are more resistant against B. cinerea compared to T3 leaves, prior to B. cinerea inoculation, due to a lower amount of innate H2O2, which is attributed to a higher level of antioxidants and antioxidant enzymes in K1. To conclude, this study provides important insights into the resistance mechanisms against B. cinerea, which highly depend on the innate antioxidative profile and specialized metabolites of woodland strawberry leaves.
Introduction
Strawberry (Fragaria × ananassa) is one of the most important berry crops worldwide with a global production of approximately 8.9 million metric tons in 2019 (FAOSTAT, 2021). The fruit are highly appreciated for their flavor and nutritional values (Ulrich et al., 1997; Wang and Lin, 2000; Giampieri et al., 2015). However, strawberry cultivation is often hampered by the occurrence of numerous pests and diseases, including the necrotrophic fungus Botrytis cinerea, resulting in gray mold of fruit and leaves and, as such, causing huge production and economic losses (Petrasch et al., 2019). B. cinerea is mainly controlled by fungicides. However, the adverse effects of fungicide use on the environment and the development of fungicide-resistant strains urge the development of alternative methods to limit B. cinerea infection of strawberry. Breeding for more resistant varieties is a promising process, but it is not straightforward as resistance in strawberry to B. cinerea is quantitative, genetically complex and depends on a wide variety of defense mechanisms. To achieve this goal, a detailed comparative study of B. cinerea-susceptible and resistant cultivars is needed to better understand the underlying mechanisms of increased resistance against B. cinerea.
B. cinerea produces cell wall degrading enzymes, reactive oxygen species (ROS), and toxins to infect and colonize plants (Blanco-Ulate et al., 2013; Nakajima and Akutsu, 2014). Likewise, plants also react via different mechanisms to restrict B. cinerea infection, for example the induction of hormonal signaling pathways regulating the production of defense-related proteins and specialized metabolites, and the production of reactive oxygen species (ROS). Also constitutive levels of defense-related molecules prior to pathogen attack can be critical for the outcome of the interaction. Upon B. cinerea inoculation of strawberry fruit, genes involved in ethylene (ET) and jasmonic acid (JA) biosynthesis and signaling are upregulated including 1-AMINOCYCLOPROPANE-1-CARBOXYLATE SYNTHASE 2 (ACS2), 1-AMINOCYCLOPROPANE-1-CARBOXYLATE OXYGENASE (ACO), LIPOXYGENASE (LOX) and ALLENE OXIDE SYNTHASE (AOS; Xiong et al., 2018; Haile et al., 2019). Additionally, the activity and expression of pathogenesis-related proteins, such as β-1,3-glucanases (βGLU) and chitinases, pathogenesis related protein family 10 (PR10) and polygalacturonase inhibitor proteins (PGIPs), enzymes inhibiting polygalacturonases produced by pathogens, are upregulated in strawberry fruit after B. cinerea inoculation (Mehli et al., 2004, 2005; Nagpala et al., 2016; Wang et al., 2016; Haile et al., 2019). Interestingly, the constitutive level of PGIPs, prior to B. cinerea inoculation, also contributes to strawberry fruit resistance. For example, a study on five F. × ananassa cultivars demonstrated that the cultivar with the highest constitutive expression level of PGIP was the least susceptible to B. cinerea (Mehli et al., 2004). Moreover, overexpression of FaPGIP in strawberry plants resulted in increased resistance against B. cinerea (Schaart, 2004).
Second, the production of ROS by plant cells is an important strategy against biotic stresses (Jajic et al., 2015). Hydrogen peroxide (H2O2), one of the most important ROS compounds, can either directly inhibit pathogen growth or act as a signaling molecule leading to reinforcement of cell walls, accumulation of phytoalexins, and programmed cell death (PCD), specifically restricting growth of biotrophic pathogens (Kärkönen and Kuchitsu, 2015; Huang et al., 2019). However, the production of ROS, leading to host cell death, facilitates infection by necrotrophic pathogens such as B. cinerea (Govrin and Levine, 2000; Khanam et al., 2005). Interestingly, constitutively higher levels of H2O2 and O2− correlated both negatively as well as positively with plant resistance against several biotic stresses (Asselbergh et al., 2007; Meng et al., 2019; Shao et al., 2019; Rahman et al., 2020). In both grapevine and strawberry, it has been reported that increased resistance against B. cinerea is linked with low basal levels of ROS (H2O2 and O2−; Meng et al., 2019; Rahman et al., 2020). The delicate balance between ROS production and scavenging is also important for plant survival under adverse conditions. Plants contain enzymatic and non-enzymatic antioxidant systems essential for ROS homeostasis (Gill and Tuteja, 2010a). Enzymatic systems include superoxide dismutase (SOD), catalase (CAT), ascorbate peroxidase (APX), glutathione peroxidase (GPX), glutathione reductase (GR) and glutathione S-transferase (GST) while non-enzymatic compounds include ascorbic acid (AsA), phenolics, flavonoids, glutathione and sugars (Gill and Tuteja, 2010b; Das and Roychoudhury, 2014; Matros et al., 2015). The activity of antioxidant enzymes has also been suggested to correlate with plant resistance. For example, grapevine genotypes with increased resistance against B. cinerea showed higher constitutive peroxidase activity in leaves and fruit than susceptible genotypes, prior to inoculation (Rahman et al., 2020). A similar observation was made in tomato leaves, where SOD activity was higher in more resistant genotypes after Alternaria solani inoculation (Ray et al., 2015). AsA, one of the important non-enzymatic antioxidants known for its ability to scavenge H2O2 in plants either spontaneously or via APX enzymes, can improve stress tolerance in plants (Akram et al., 2017). AsA can affect plant resistance by the orchestration of different mechanisms. For example, in Arabidopsis thaliana, AsA-deficient mutants show increased PCD, an increased amount of salicylic acid and PR proteins leading to enhanced constitutive resistance against the hemibiotrophic pathogen Pseudomonas syringae (Pavet et al., 2005; Mukherjee et al., 2010). On the other hand, AsA-deficient mutants are less resistant to the necrotrophic pathogen Alternaria brassicicola (Botanga et al., 2012). Additionally, treatment of strawberry fruit with AsA inhibited B. cinerea growth, potentially by increasing the total phenolics content of strawberry (Elkorany and Mohamed, 2008). Similar to H2O2, AsA potentially influence plant disease resistance levels depending on the pathogen’s lifestyle.
Finally, both primary and specialized metabolites contribute to restricting pathogen infection either by having a role in plant resistance or by directly inhibiting the pathogen (Rojas et al., 2014). For example, spermine treatment resulted in higher levels of glucose, fructose and sucrose in Arabidopsis, as well as higher resistance to B. cinerea. Moreover, Colletotrichum gloeosporioides showed enhanced colonization in tomato fruit with low sugar content (Ziv et al., 2020). Acetic acid suppresses the growth of C. gloeosporioides directly, and lowers the rate of infection in strawberries (Kang et al., 2003; Weber et al., 2016). Strawberry fruit containing high levels of specialized metabolites are shown to have increased resistance against B. cinerea. Unripe strawberry fruit contain more flavonoids than mature strawberry fruit, including catechin, agrimoniin, ellagic acid conjugates, proanthocyanidins, and flavan-3-ols (Di Venere et al., 1998; Puhl and Treutter, 2008; Aaby et al., 2012), which could be one of the reasons why immature strawberry fruit is less sensitive to B. cinerea. Moreover, the concentration of proanthocyanidin has been used as a biochemical marker of strawberry fruit resistance against B. cinerea (Jersch et al., 1988; Di Venere et al., 1998; Hébert et al., 2002). Treatment of strawberry fruit with terpinen-4-ol improved plant resistance against B. cinerea by activating the phenylpropanoid metabolic pathway (Li et al., 2020). Similarly, treatment of strawberry leaves with red light or chitosan resulted in increased concentrations of total phenolics and flavonoids and higher resistance against B. cinerea (Meng et al., 2019; Peian et al., 2021).
The induced defense response of strawberry leaves and fruit against B. cinerea has been well-documented (Jersch et al., 1988; Nagpala et al., 2016; Bui et al., 2019; Haile et al., 2019; Hu et al., 2019; Petrasch et al., 2019). However, while it is clear that constitutive resistance mechanisms also play a role in the resistance of strawberry against B. cinerea, a comprehensive view on the underlying mechanisms is lacking. In the present study, we identified two woodland strawberry genotypes with altered resistance against B. cinerea and investigated the mechanisms behind the increased resistance of K1 compared to T3 by examining expression of defense-related genes, the production of H2O2, AsA, specialized and primary metabolites and the expression and activity of ROS scavenging enzymes, prior to inoculation.
Materials and Methods
Plant Material and Pathogen Inoculum
Fragaria vesca ssp. vesca Kreuzkogel 1 (K1) and F. vesca ssp. vesca Tenno 3 (T3; provided by “Professor Staudt Collection”) were grown in a greenhouse at 21–23°C under light (on average 14 h light/10 h dark, 250 W/m2) and a relative humidity of 65%, except for 1 h before sunset, the humidity was increased to 90%.
Botrytis cinerea strain B05.10 was cultivated on potato dextrose broth agar (PDA) medium for 5 d in the dark at 25°C, then exposed to UV-A (315 nm-400 nm) for 12 h and allowed to sporulate for 5–9 d in the dark. The spores were collected and the concentration was adjusted to 108 spores/mL using a hemocytometer counting chamber and stored in 25% glycerol at −80°C. For leaf inoculations, B. cinerea spores were diluted to 106 spores/mL with ½ potato dextrose broth (PDB).
Leaf Inoculations and Disease Assays
During strawberry growth, leaves were labeled using their date of emergence. For all experiments, trifoliate strawberry leaves were collected, cut into three leaflets, disinfected with 5% bleach (Loda Bleach 10°) for 5 min and cleansed with sterile distilled water. To evaluate the susceptibility against B. cinerea of the two genotypes, 3 weeks old leaves were used. After disinfection, dried detached leaflets were immediately transferred to 0.8% agar plates and drop-inoculated with 5 μl of 106 spores/mL on the adaxial leaf surface avoiding inoculation on the main vein. The plates were kept at room environment. For disease assays, the lesion area was measured 5 d post inoculation (dpi) and 14 or 15 detached leaflets from each genotype were evaluated. Wilcox test was used to evaluate statistically significant differences between the genotypes.
The resistance level of K1 and T3 genotypes was assessed based on the disease severity index (DSI) according to Curvers et al. (2010) and Rahman et al. (2018) with minor modifications. DSI was calculated using five different classes (0: no symptoms; 1: 0 < lesion area percentage < 25%; 2: 25% < lesion area percentage < 50%; 3: 50% < lesion area percentage < 75%; 4: 75% < lesion area percentage < 100%) using the formula [(0 × a) + (1 × b) + (2 × c) + (3 × d)/(a + b + c + d)] × 100/3 where a, b, c, and d are the number of leaves within the scores of 0, 1, 2, 3 or 4, respectively. Next, the DSI was used to classify the different resistance levels: 0: highly resistant; 0–25%: resistant; 25–50%: moderately resistant; 50–75%: susceptible; 75–100% highly susceptible.
To follow up B. cinerea development, three inoculated leaflets per genotype were collected 24 h post inoculation (hpi). Leaflets were visualized, after staining the pathogen with trypan blue as described by Fernández-Bautista et al. (2016), using a stereomicroscope (Olympus SZX9/Highlight 3,100). The area of stained hyphae and spores was measured with ImageJ by converting the pictures to a binary version (Fahrentrapp et al., 2019). Each treatment was performed with one leaflet and three replicates. Wilcox test was used to evaluate statistically significant differences between the genotypes.
Quantification of Botrytis cinerea DNA on Fragaria vesca Leaves
For detection and quantification of B. cinerea, 3 weeks old strawberry leaflets were inoculated with three droplets of 5 μl of 106 spores/mL B. cinerea and samples were collected by punching 1 cm circle discs around the infection site at 0, 6, 24, 48, 72 and 96 hpi. DNA was extracted according to the cetyltrimethylammonium bromide (CTAB) method (Tamari et al., 2013) with minor modifications (Supplementary Text 1). Each treatment was performed with two leaflets (six discs) and three replicates. The leaf samples were frozen immediately in liquid nitrogen and stored at −80°C.
Using genomic DNA of B. cinerea as template, quantitative polymerase chain reaction (qPCR) was performed with primers targeting the intergenic spacer (IGS) to specifically detect and quantify B. cinerea (Suarez et al., 2005). The qPCR reaction was performed in 20 μl total volume containing 2 μl of genomic DNA (gDNA) template, 2 μl of 5 μM forward and reverse primers (IGS), 4 μl of RNase-free-Milli-Q water and 10 μl of SYBR green (PowerUp™ SYBR™ Green Master Mix, Fisher Scientific, BIO-RAD) according to the manufacturer’s instruction. DNA from mycelium was used to generate calibration curves to quantify the amount of fungal DNA and Wilcox test was used to evaluate statistically significant differences between the genotypes. The sequences of the IGS primers can be found in Supplementary Table 1.
Metabolite and Enzyme Activity Measurements
For the AsA, DHA, and the activity of CAT, APX, GPX and GR measurements, 3 weeks old leaves were used. For AsA and DHA, each treatment was performed with three leaflets and seven replicates. For the activity of CAT, APX, GPX and GR, each analysis was performed with three leaflets and three replicates. For H2O2, total phenolics, total flavonoids and primary metabolites measurements, trifoliate strawberry leaves were collected and the leaflets were analyzed. First, we showed that the three leaflets had similar resistance level to B. cinerea (Supplementary Figure 1). Then the left leaflets were used to evaluate their resistance against B. cinerea by the method described before (leaf inoculations and disease assays), while the middle and right leaflets were frozen immediately in liquid nitrogen and stored at −80°C for metabolite measurements. The samples were ground into a fine powder in liquid nitrogen before being measured.
Measurement of H2O2 Levels
H2O2 levels were measured as described by Junglee (Junglee et al., 2014) with minor modifications. Homogenized leaf material (50 mg) was extracted in 1 ml of solution containing 0.5 ml of 1 M KI, 0.25 ml of 1% trichloroacetic acid (TCA), and 0.25 ml of 10 mM potassium phosphate buffer (pH = 5.8) for 15 min at 4°C, centrifuged at 12,000 × g for 15 min at 4°C. Next, 200 μl of supernatant was placed in UV-microplate wells and incubated at room temperature for 20 min before measuring the absorbance at 350 nm via a spectrophotometer (SpectraMax® Plus). The measurement was performed using three technical replicates and 18 biological replicates. Based on a standard curve, the H2O2 concentration was calculated as μmol/gFW. For tissue coloration background, a control was created using water instead of KI. T-test was used to evaluate statistically significant differences between the genotypes.
Measurement of Antioxidant Enzyme Activities
The extraction of CAT, APX, and GPX enzymes was performed as previously described (Van Rensburg et al., 2021) with minor modifications. Three weeks old strawberry trifoliate leaves were powdered in liquid nitrogen and 200 mg was extracted with 600 μl extraction buffer (100 mM phosphate buffer (pH 7.0), 0.1% Triton X-100, 15% glycerol, 1 mM phenylmethylsulfonyl fluoride (PMSF), 1 mM ascorbic acid, and 0.35 mM β-mercaptoethanol) by grinding with plastic micro pestles inside 2 ml Eppendorf tubes for 60 s and then incubated on ice for 1 h. Next, samples were centrifuged at 4°C for 10 min at 15,000 × g. Finally, the supernatants were kept at −80°C until further analysis, except for APX, which was measured immediately.
APX activity was measured as described previously (Van Rensburg et al., 2021). Reactions were carried out in 200 μl solution containing 185 μl of 100 mM phosphate buffer (pH 7.0),10 μl0.5 mM ascorbic acid and 5 μl enzyme extract. The absorbance of the reaction at 290 nm was measured using a spectrophotometer (SpectraMax® Plus) at 10 s intervals for 5 min.
CAT activity was measured as previously described with minor modifications (Van Rensburg et al., 2021). The enzyme extract was diluted 10 times before being measured in a quartz cuvette with 1 ml 100 mM phosphate buffer (pH 7.0) and 10 μl diluted enzyme extract. Before starting the reaction, the background was monitored for 30 s. The decrease in absorbance at 240 nm was measured using a spectrophotometer (SpectraMax® Plus) at 10 s intervals for 5 min. GR activity was measured as previously described with minor modifications (El-Shabrawi et al., 2010). The reagents used were 200 μl potassium phosphate buffer (100 mM, pH 7.6), 10 μl 1 mM ethylenediaminetetraacetic acid (EDTA), 10 μl 6 mM 5,5′-dithio-bis-(2-nitrobenzoic acid; DTNB), 20 μl 0.2 mM oxidized glutathione (GSSG) and 15 μl enzyme extract. Reaction was initiated by adding 10 μL 5 mM of NADPH. The increase in absorbance at 412 nm was measured using a spectrophotometer (SpectraMax® Plus) at 10 s intervals for 5 min.
The activity of these three enzymatic reactions was determined using the reaction’s linear range, with enzyme activity reported in units U/mg protein, where 1 U is equal to the change in OD of 0.01 per min. The protein was measured using Coomassie Brilliant Blue G-250 (Sedmak and Grossberg, 1977).
The extraction of GPX was performed as described previously (Van Rensburg et al., 2021). After the extraction, the GPX activity was measured by the “glutathione peroxidase assay kit” according to the manufacturer’s protocol (Abbexa).
For the activity of CAT, APX, GPX and GR, T-test was used to evaluate statistically significant differences between the genotypes.
Measurement of Ascorbic Acid and Dehydroascorbate
Total AsA, AsA and oxidized dehydroascorbate (DHA) levels (total AsA-AsA) were measured as described by Stevens with minor modifications (Stevens et al., 2006). Briefly, homogenized leaf material (500 mg) was extracted in 1.3 ml of pre-cooled 6% TCA, incubated on ice for 15 min and centrifuged at 20,000 × g for 20 min at 4°C. Next, 90 μl of supernatant was used for measuring the absorbance at 550 nm using a spectrophotometer (SpectraMax® Plus). The concentration of AsA and DHA was expressed in μg/g FW. T-test was used to evaluate statistically significant differences between the genotypes.
Measurement of Total Phenolics and Flavonoids
Total phenolic and flavonoid levels were determined following the method of Meng (Meng et al., 2019) with minor modifications. In short, homogenized leaf material (50 mg) was mixed with 1 ml of 80% methanol and sonicated for 20 min for 2 times with a 10 min stop in between. After centrifugation, the supernatant was collected for the measurement.
To determine total phenolics, 200 μl of supernatant was mixed thoroughly with 50 μl of the Folin–Ciocalteu reagent (BIPP Merck Life Science) for 3 min, followed by adding 50 μl of 10% Na2CO3 solution. Next, 700 μl of distilled water was added, the samples were left at room temperature for 2 h in dark, and then 200 μl of reaction solution was used to measure the absorbance at 765 nm. The total phenolic content was calculated as mg/g FW based on a standard curve established with gallic acid equivalent. To determine total flavonoids, 200 μl of supernatant was mixed with 60 μl of 5% NaNO2 in 800 μl distilled water for 5 min, then 120 μl of 10% AI(NO3)3 solution was added to react for 6 min. Finally, 400 μl of 1 M NaOH and 420 μl of distilled water was added and 200 μl of reaction solution from each sample was used to measure the absorbance at 510 nm. The total flavonoid content was calculated as mg/g FW based on a standard curve established with rutin equivalent (RE). Both measurements were performed using three technical replicates and 27 biological replicates for K1 and 44 biological replicates for T3. T-test was used to evaluate statistically significant differences between the genotypes.
Gas Chromatography–Mass Spectrometry Analysis
The metabolites were extracted from the leaf samples and derivatized using a previously described method (Hu et al., 2019) with minor modification. The homogenized samples (50 ± 2 mg) were extracted with 1.8 ml of pre-cooled methanol:water (4:1, v/v) by vortexing for 1 min and then sonicated at room temperature for 20 min. After centrifugation, 600 μL of the supernatant was transferred into two 2 ml Eppendorf tubes to measure sugars and acids separately.
For derivatization of sugars, shikimic acid and quininic acid, the same method as described by Terzoudis (Terzoudis et al., 2022) was used. For acids analysis, only 20 μl of 0.1 g/l 3-(4-hydroxyphenyl)-propionic acid was added to the samples as the internal standard and after drying, the derivatization was performed by adding 120 μL of methoxylamin-hydrochloride (20 g/l in pyridine, Sigma Aldrich) for 90 min at 37°C, 700 rpm. Next, 120 μl of BSTFA (Sigma Aldrich) was added and then incubated for 30 min at 60°C, 700 rpm. After centrifugation, 100 μL of supernatant was used for detection. In total, 18 detached leaves from each genotype were evaluated.
For separation and detection of analytes, GCMS was used in the same way as described by Terzoudis (Terzoudis et al., 2022). The concentration of primary metabolites was determined based on calibration curves and was calculated as mg/g FW. These results were first tested for normal distribution by the Shapiro–Wilk test, and then Wilcox and t-tests were used to assess statistically significant differences between the genotypes.
qRT-PCR for Expression Analysis
Three weeks old leaves were collected to detect the expression of defense-related, ROS-related and specialized metabolism biosynthesis genes. Each analysis was performed with three trifoliate leaves and three biological replicates. Generalized linear models (glm) were used to evaluate statistically significant differences between the genotypes for defense related gene expression. Wilcox test was used to evaluate statistically significant differences between the genotypes for ROS-related and specialized metabolism biosynthesis-related gene expression. The leaf samples were frozen immediately in liquid nitrogen and stored at −80°C.
Total RNA was extracted using the CTAB method (Yu et al., 2012) combined with the RNeasy plant mini kit (Qiagen, Germany) with minor modifications (Supplementary Text 2). DNase treatment and cDNA synthesis were performed using DNAse I (New England Biolabs® Inc) and SuperScript IV Reverse Transcriptase kit (ThermoFisher Scientific), respectively, according to the manufacturer’s instructions. Primers used for defense-related marker genes are shown in Supplementary Table 1. Quantitative Reverse Transcription PCR (qRT-PCR) reactions were performed in 20 μl containing 6 μl of cDNA template, 2 μl of 5 μM forward and reverse primers, and 10 μl of SYBR green. The parameters for performing qRT-PCR are similar to the qPCR described earlier, except for the number of amplification cycles (40 instead of 35). The relative quantification of gene products was based on the ∆Ct method (Schmittgen and Livak, 2008). Transcript levels were normalized to the reference genes HISTONE H4 (H4) and UBIQUITIN-PROTEIN LIGASE (UBC9; Zhang et al., 2018; Jose et al., 2020). All primers can be found in Supplementary Table 1.
Data Analysis
For GC–MS, the data was analyzed by means of Principal Component Analysis (PCA) and Partial Least Squares Regression (PLSR) both on the correlation matrices using The Unscrambler® X (10.5.1) according to Hu (Hu et al., 2019).
For the other experiments, statistical analysis was conducted using R.1 Different statistical tests were used for the different datasets and indicated in previous method and the figure legends. For the boxplots, the box limits are the 25th and 75th percentile, the middle line in the individual boxes represents the median, the white point in the individual boxes marks the mean, whiskers extend to 1.5-fold the interquartile range of the 25th and 75th percentiles. For bar plots, values are represented as means of replicates with standard errors shown by vertical bars.
Results
Genotype K1 Is More Resistant to Botrytis cinerea Than T3
In an initial screen for altered leaf susceptibility of different F. vesca genotypes against Botrytis cinerea (Supplementary Figure 2), we identified two genotypes for further research, i.e., Fragaria vesca ssp. vesca Kreuzkogel 1 (K1) and F. vesca ssp. vesca Tenno 3 (T3). We confirmed in an independent experiment that at 5 dpi, B. cinerea inoculation results in significant larger lesions in T3 compared to K1 (624 mm2 vs. 227 mm2; Figure 1). Based on the DSI for K1 (42%) and T3 (69%), we defined the genotypes as moderately resistant and susceptible, respectively.
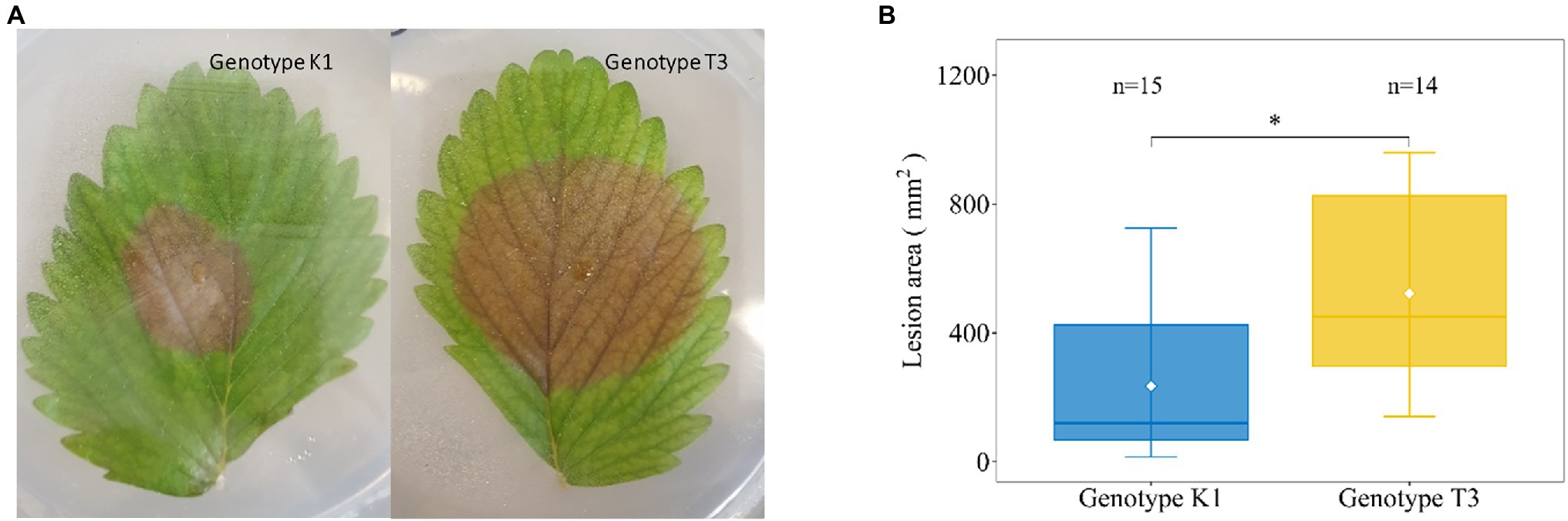
Figure 1. Representative picture of disease symptoms (A) and lesion area (B) on strawberry leaves of Kreuzkogel 1 (K1) and Tenno 3 (T3) at 5 d post Botrytis cinerea inoculation. The asterisk ‘*’ indicates significant differences among two genotypes by Wilcox test at p < 0.05.
qPCR was performed to analyze the amount of B. cinerea DNA in both strawberry genotypes at different time points post inoculation (Figure 2). A significantly higher amount of B. cinerea DNA of 48 and 115.2% was observed at 48 and 96 hpi, respectively, in genotype T3 compared to K1.
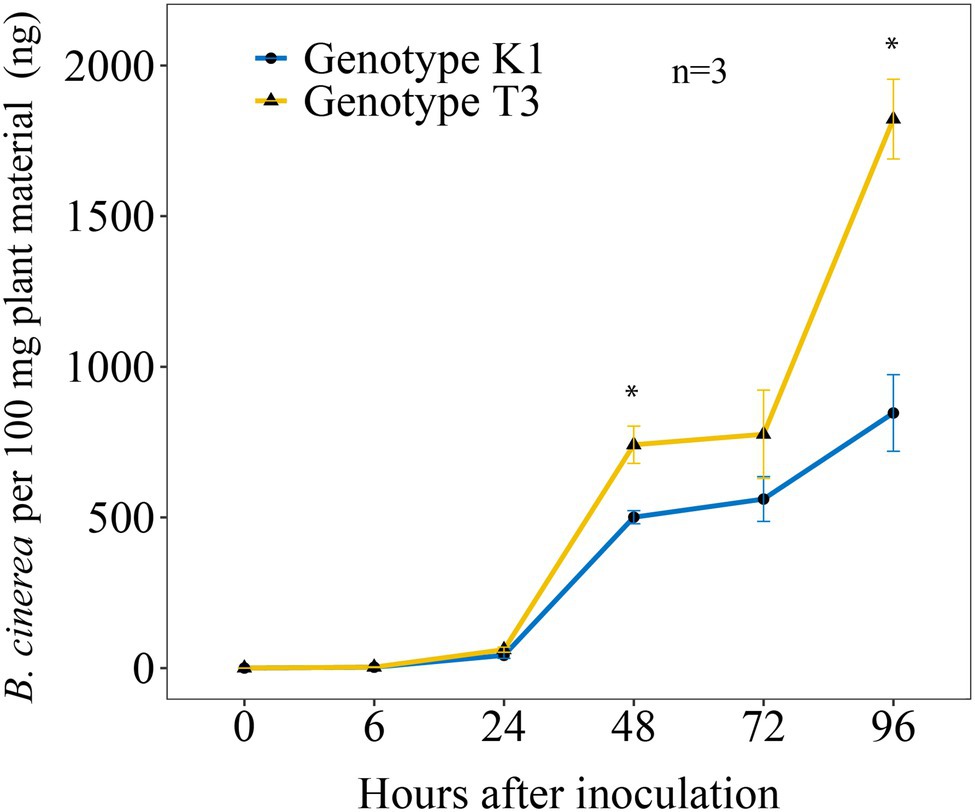
Figure 2. Amount of B. cinerea B05.10 DNA per 100 mg leaves at different time points post inoculation (0, 6, 24, 48, 72 and 96 h post B. cinerea inoculation) in Kreuzkogel 1 (K1) and Tenno 3 (T3). The asterisk ‘*’ indicates significant differences among two genotypes by Wilcox test at p < 0.05. Values are represented as means of three replicates with standard error shown by vertical bars.
Next, fungal growth was microscopically observed at 24 hpi (Figure 3). More hyphal growth was observed in T3 compared to K1 (Figure 3A). The hyphal growth area was calculated using ImageJ software confirming that hyphal growth on T3 leaves was significantly higher (2.08-fold) compared to K1 at 24 hpi (Figure 3B). Altogether, these data show that K1 is more resistant against B. cinerea than T3.
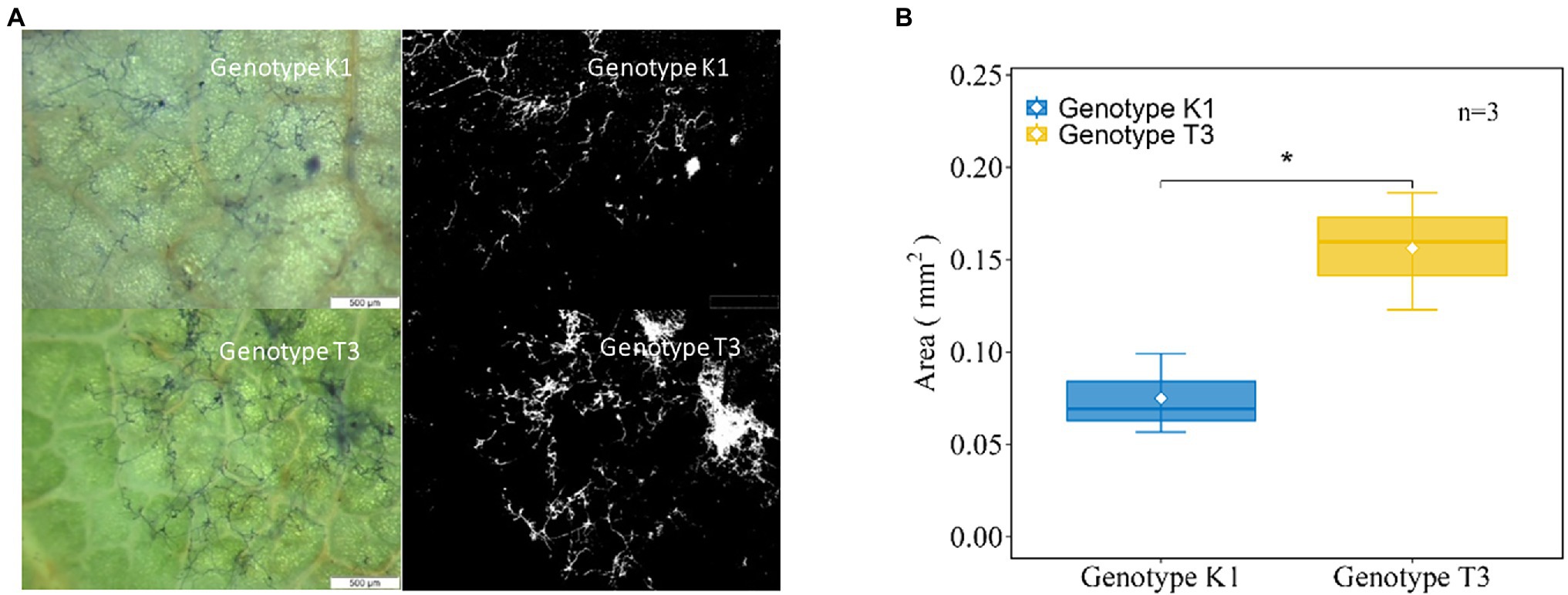
Figure 3. (A) Microscopic (left) and binary image (right) of the hyphal growth at 24 h post inoculation (hpi) for Kreuzkogel 1 (K1) and Tenno 3 (T3). The microscopic evaluation was done by trypan blue staining of fungal growth and the binary image was converted and analyzed by the ImageJ software. (B) Total area (mm2) of hyphae on leaves of K1 and T3 at 24 hpi. The asterisk ‘*’ indicates significant difference among two genotypes by Wilcox test at p < 0.05.
Induced Defense Responses Have a Minor Role in Botrytis cinerea Resistance for Both Genotypes
To evaluate the expression of AOS, PAL, JASMONIC ACID CARBOXYL METHYLTRANSFERASE (JMT), LOX, PGIP, PECTINESTERASE INHIBITOR (PEI), PATHOGENESIS RELATED PROTEIN FAMILY 1 (PR1) and PR10 upon B. cinerea inoculation, qRT-PCR analysis on both genotypes was performed at 24 and 48 hpi (Supplementary Figure 3). No clear indication for a stronger defense response was found for both genotypes. However, before B. cinerea inoculation, a significantly higher expression of AOS, βGLU and JMT of 1.98-fold, 10.46-fold and 7.65-fold, respectively, was detected in K1 compared to T3 (Figure 4). This result suggests that the increased resistance of K1 against B. cinerea, compared to T3 is due to the basal resistance mechanisms rather than induced defense responses.
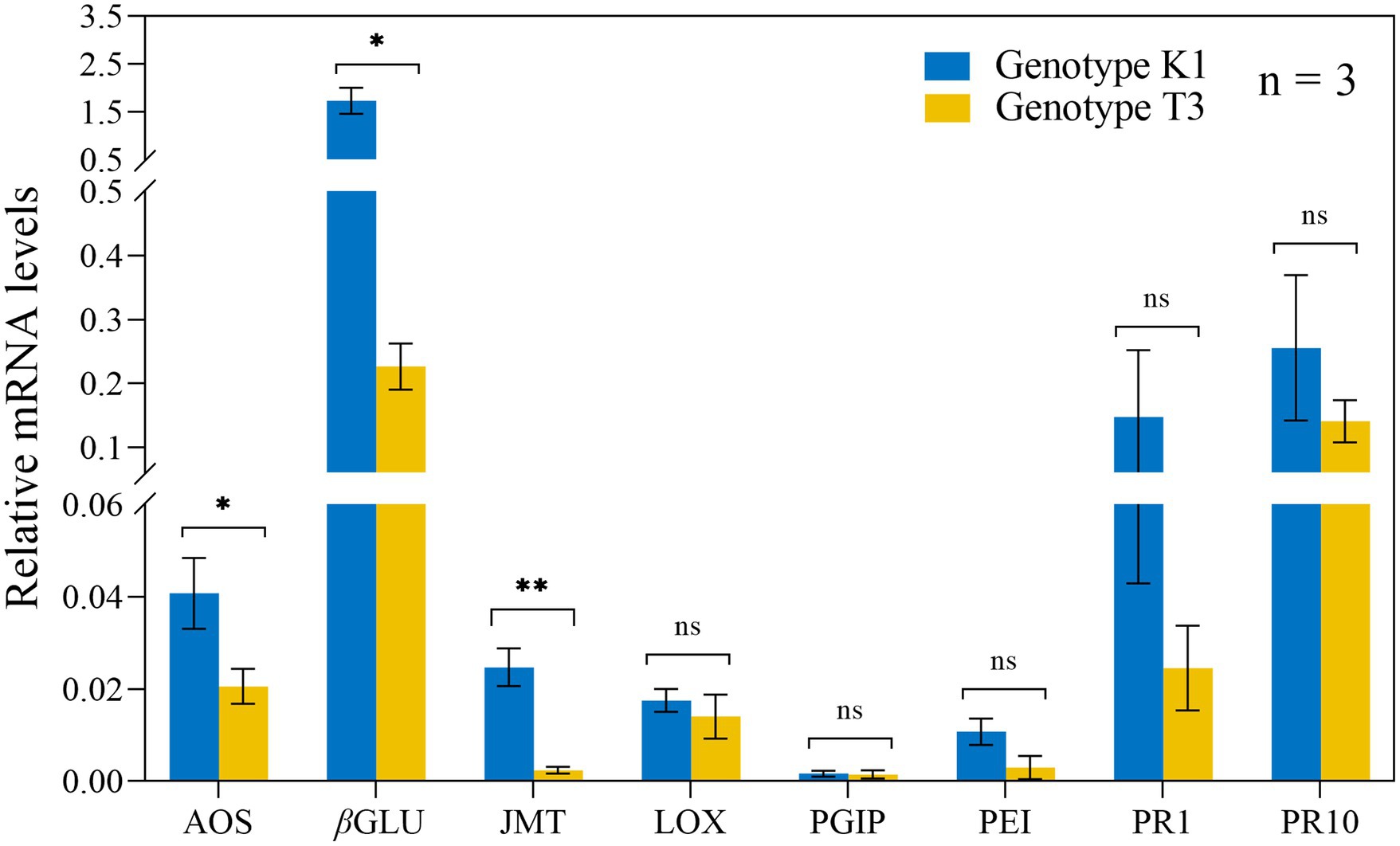
Figure 4. Relative expression levels of eight defense-related genes in leaves of Kreuzkogel 1 (K1) and Tenno 3 (T3), prior to B. cinerea inoculation, were measured via qRT-PCR. All values were normalized to the expression level of the H4 and UBC9 housekeeping genes. Significant differences between two genotypes, based on the generalized linear models (glm), are represented by ns p > 0.05, *p < 0.05, **p < 0.01. AOS, ALLENE OXIDE SYNTHASE; βGLU, β-1,3-GLUCANASE; JMT, JASMONIC ACID CARBOXYL METHYLTRANSFERASE; LOX, LIPOXYGENASE; PGIP, POLYGALACTURONASE INHIBITOR PROTEIN; PEI, PECTINESTERASE INHIBITOR; PR1, PATHOGENESIS-RELATED PROTEIN FAMILY 1; PR10, PATHOGENESIS-RELATED PROTEIN FAMILY 10.
Lower Levels of H2O2 in K1 Correlate With Higher Resistance Against Botrytis cinerea
It has been previously reported that in one to five week old leaves of F. × ananassa strawberry plants, H2O2 levels correlated positively with gray mold disease severity (Meng et al., 2019). Therefore, we aimed at investigating the correlation between constitutive H2O2 levels in strawberry leaves and B. cinerea resistance for both genotypes. We first determined the amount of H2O2 in the two strawberry genotypes (Figure 5). The amount of H2O2 (μmoL/g FW) was significantly lower in K1 (0.93 ± 0.10) compared to T3 (2.14 ± 0.18; Figure 5A). Next, the H2O2 levels were correlated with B. cinerea resistance for both genotypes. Lesion area (mm2) showed a high positive correlation with H2O2 levels for K1 (R = 0.77, p = 0) and T3 (R = 0.83, p = 0). Accordingly, T3 leaves showed a larger lesion area and higher H2O2 content compared to K1 (Figure 5B), suggesting that lower levels of H2O2 are important for the increased resistance against B. cinerea, which is in line with previous observations (Govrin and Levine, 2000; Khanam et al., 2005; Meng et al., 2019).
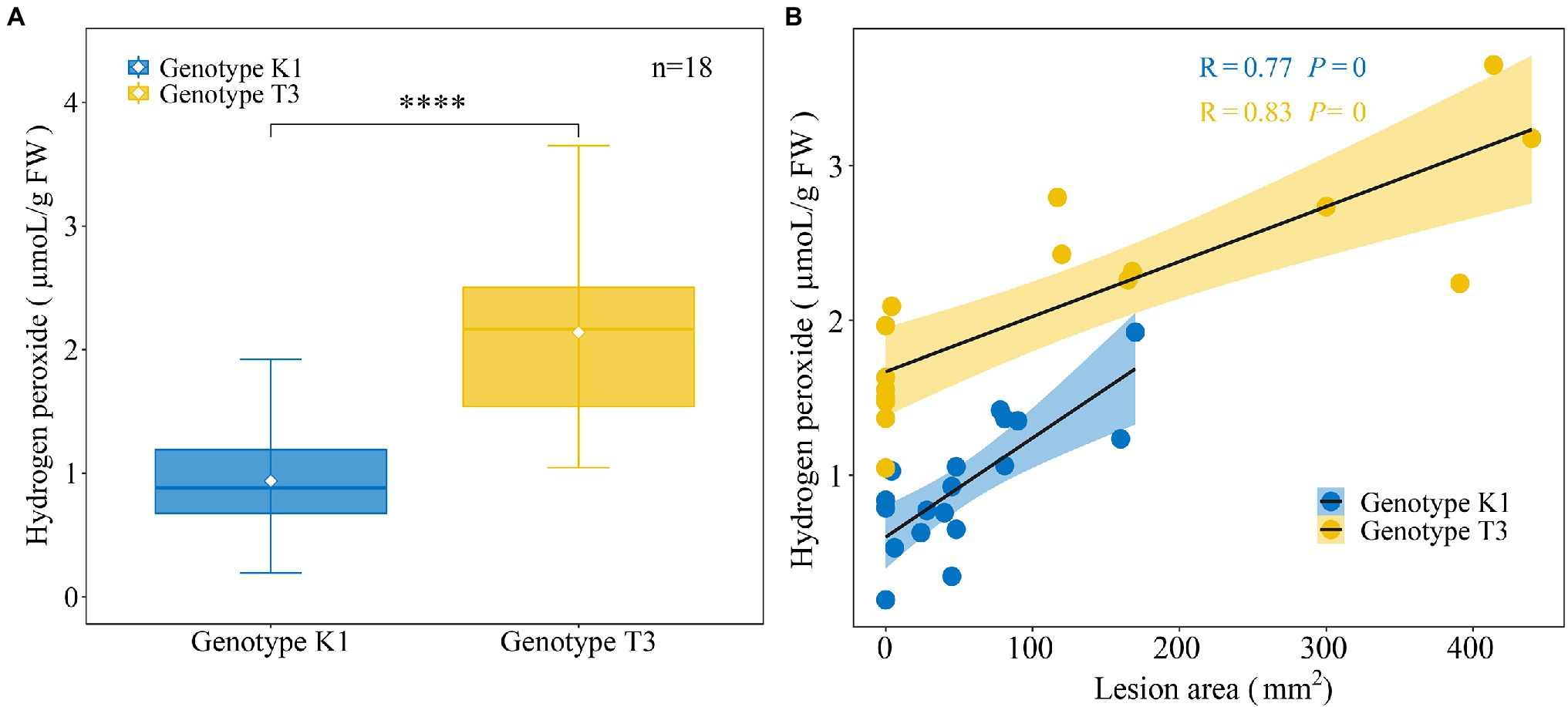
Figure 5. Analysis of H2O2 production of leaves of Kreuzkogel 1 (K1) and Tenno 3 (T3). (A) The amount of H2O2 in strawberry middle and right leaflets for K1 and T3 without B. cinerea inoculation. The asterisk ‘*’ indicates significant differences among two genotypes by t-test at p < 0.0001. (B) The correlation between lesion area, measured on strawberry left leaflets at 5 dpi H2O2 in strawberry middle and right leaflets for K1 and T3, respectively, without B. cinerea inoculation.
Higher Expression Levels and Activity of ROS Scavenging Enzymes Were Observed in K1
The level of H2O2 is regulated by the expression and activity of ROS production and ROS scavenging enzymes (Foyer and Noctor, 2016). Here, we analyzed the enzymatic and non-enzymatic systems involved in controlling H2O2 levels to investigate the mechanisms underlying the difference in H2O2 levels between the two genotypes.
No significant differences in the transcript levels of NADPH OXIDASE (NOX), RESPIRATORY BURST OXIDASE HOMOLOGS (RBOHA), and SUPEROXIDE DISMUTASE (SOD) isoforms were observed between the genotypes (Figure 6). Also, the expression of CATALASE (CAT) and PEROXIDASE (POD), which catalyze the conversion of H2O2 to H2O and O2 did not differ significantly between the two genotypes (Figure 6). Glutathione reductase (GR) and ascorbate peroxidase (APX) as well as glutathione peroxidase (GPX) are important enzymes of the AsA-GSH cycle and GPX cycle, respectively, and the gene expression of different isoforms was tested in both genotypes. Transcript levels of GR, two isoforms of APX gene (APX1 and APX3) and one of the five GPX genes (GPX6_1) were significantly higher expressed in K1 compared to T3 (Figure 6). In addition, the activity of the antioxidant enzymes GPX, APX, GR and CAT were analyzed in both genotypes. GPX and APX activities were significantly higher in K1 compared to T3, while CAT activity did not differ between two genotypes (Figure 7) and these results correlate nicely with their corresponding gene expression. GR activity was not different between the two genotypes (Figure 7). Overall, we can propose that the lower H2O2 in K1 compared to T3 is due to the higher expression level and activity of ROS scavenging enzymes.
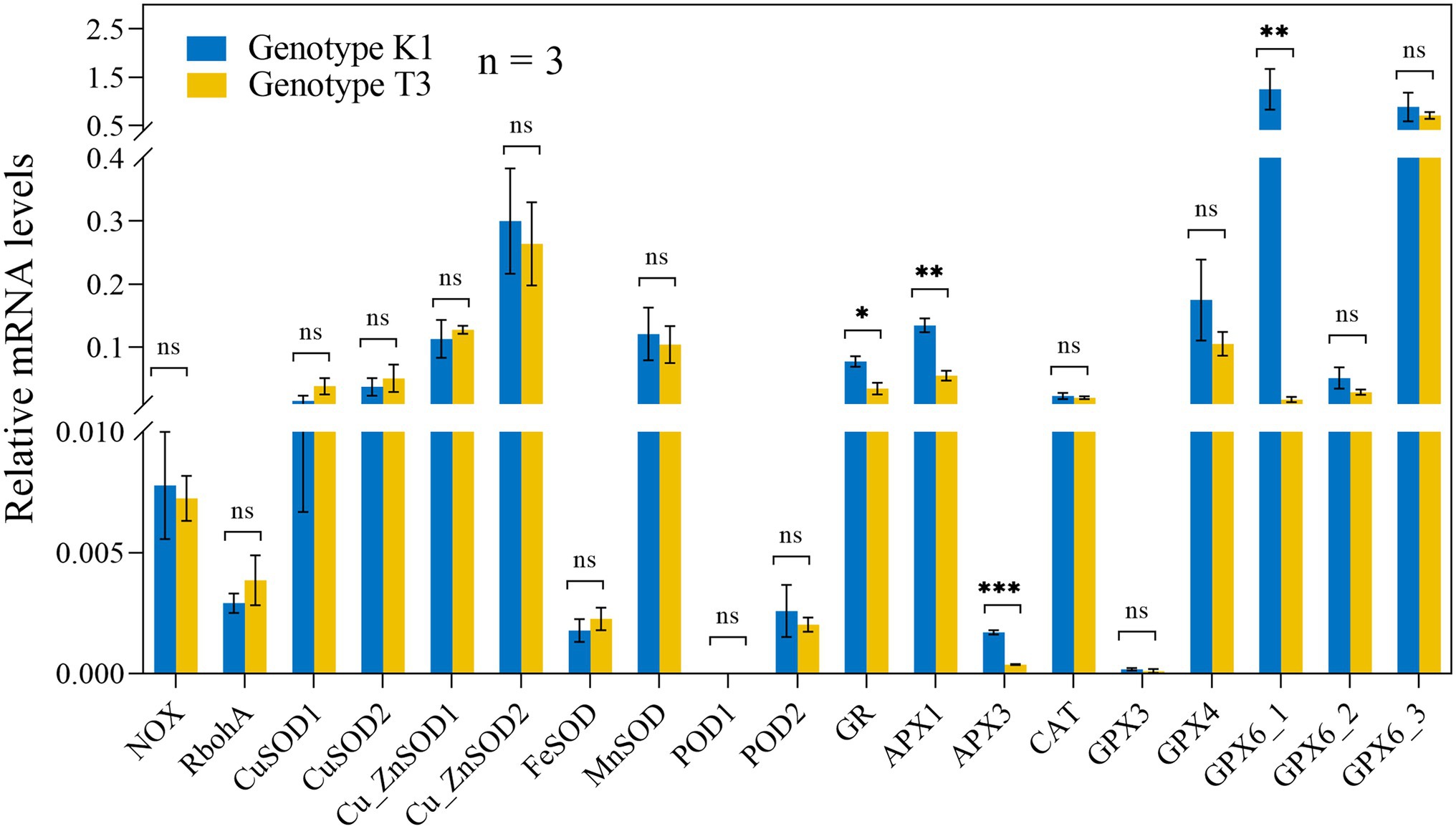
Figure 6. Relative expression levels of genes involved in H2O2 production and scavenging in leaves of Kreuzkogel 1 (K1) and Tenno 3 (T3) without B. cinerea inoculation were measured via qRT-PCR. All values were normalized to the expression level of the H4 and UBC9 housekeeping genes. Significant differences between two genotypes, based on the Wilcox test, are represented by ns p > 0.05, *p < 0.05, **p < 0.01 and ***p < 0.001. NOX, NADPH OXIDASE; RBOHA, RESPIRATORY BURST OXIDASE HOMOLOGS; SOD, SUPEROXIDE DISMUTASE; CAT, CATALASE; POD, PEROXIDASE; GR, GLUTATHIONE REDUCTASE; APX: ASCORBATE PEROXIDASE, GPX, GLUTATHIONE PEROXIDASE.
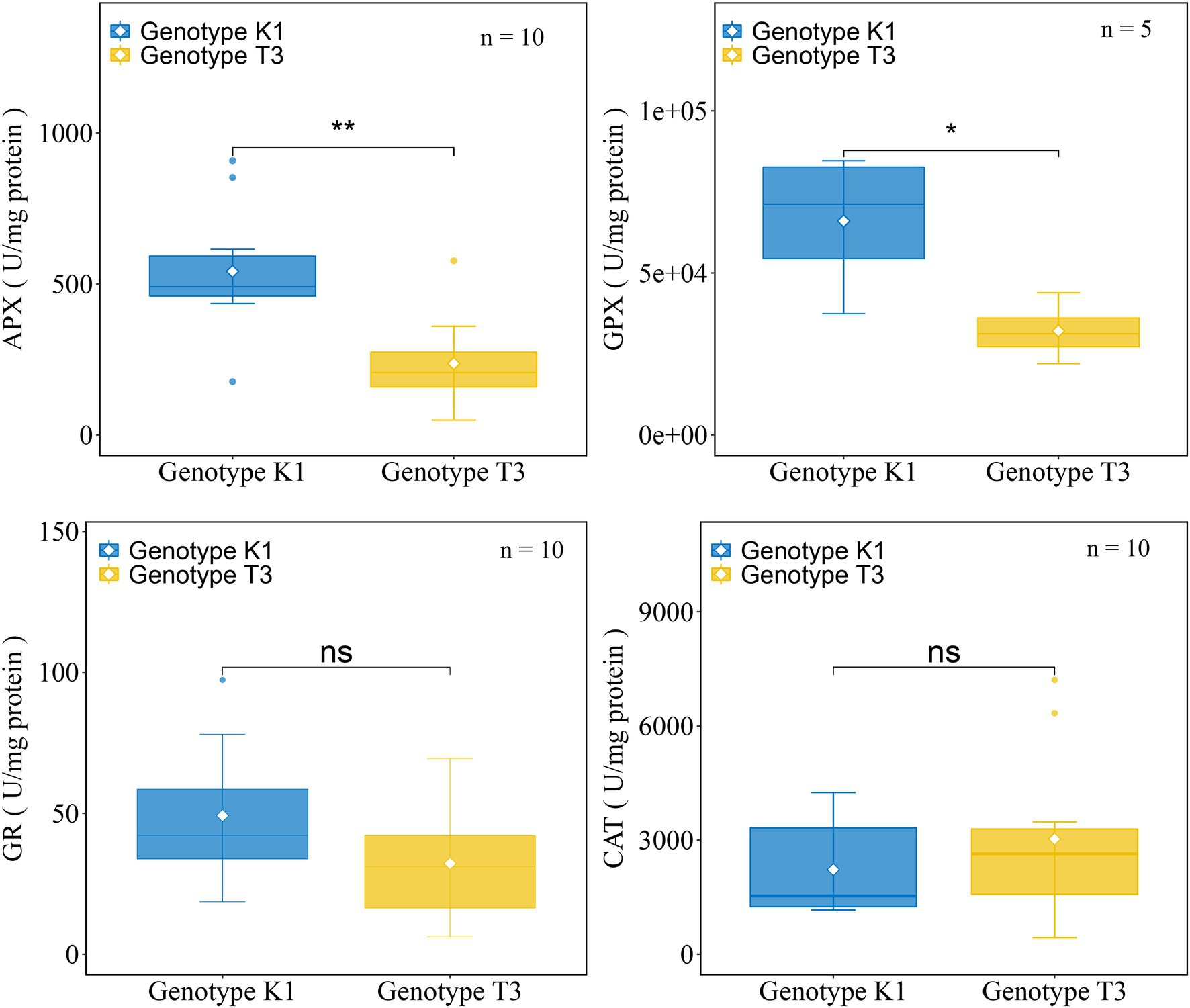
Figure 7. The activity of ROS scavenging enzymes in leaves of Kreuzkogel 1 (K1) and Tenno 3 (T3) without B. cinerea inoculation. Significant differences between two genotypes, based on a t-test, are represented by ns p > 0.05, *p < 0.05 and **p < 0.01. The individual points represent the outliers. APX, ascorbate peroxidase; GPX, glutathione peroxidase; GR, glutathione reductase; CAT, catalase.
Higher Levels of Ascorbic Acid and Phenolic Compounds Were Observed in K1
In addition to antioxidant enzymes, there are also non-enzymatic systems to scavenge H2O2 in plants, among which AsA has an important H2O2 scavenging function in the plant cell (Takahama and Oniki, 1997). In addition, APX, which metabolizes H2O2 to H2O using AsA as a specific electron donor, showed significant differences in gene expression and enzyme activity between the two genotypes (Figures 6, 7). Therefore, the levels of the major antioxidant AsA and its oxidized form dehydroascorbic acid (DHA) were measured. The amount of AsA was significantly higher in K1 (302.07 ± 43.62 μg/g FW) compared to T3 (241.40 ± 56.45 μg/g FW; Figure 8A). Similarly, the amount of DHA was significantly higher in K1 (70.18 ± 12.56 μg/g FW) compared to T3 (53.58 ± 14.94 μg/g FW; Figure 8B).
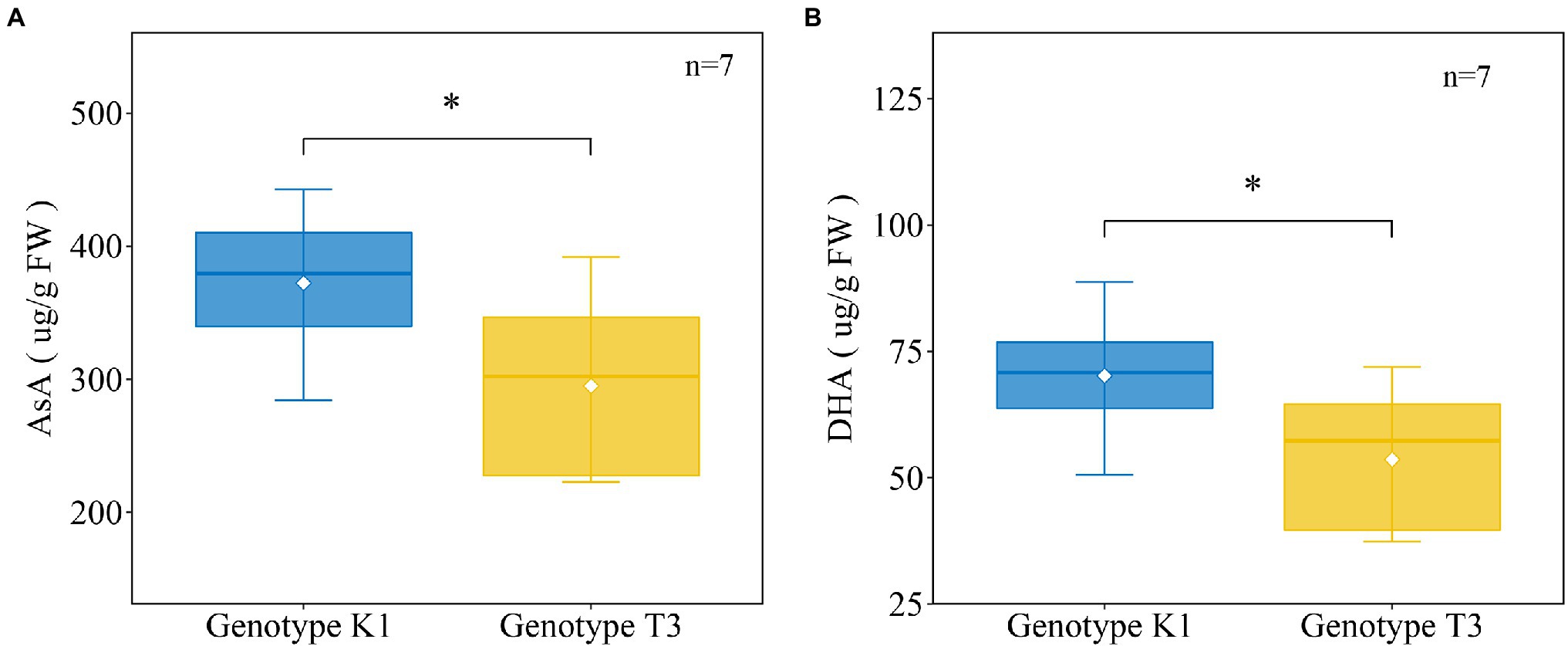
Figure 8. The amount of ascorbic acid (AsA) (A), dehydroascorbate (DHA) (B) in strawberry leaves of Kreuzkogel 1 (K1) and Tenno 3 (T3) without B. cinerea inoculation. The asterisk ‘*’ indicates significant differences among the two genotypes determined by a t-test at p < 0.05.
Also phenolics can act as ROS scavengers and are important metabolites against pathogens (Pourreza, 2013). High amounts of flavonoids and phenolics have previously been shown to be associated with increased resistance against B. cinerea (Ray et al., 2015). Here, except for 4-COUMAROYL-COA LIGASE (4CL) and FLAVONOL SYNTHASE (FLS), the expression of biosynthesis genes of the phenylpropanoid pathway such as PHENYLALANINE AMMONIA LYASE (PAL), CINNAMIC ACID 4-HYDROXYLASE (C4H), CINNAMOYL-COA REDUCTASE (CCR), CHALCONE SYNTHASE (CHS), CHALCONE ISOMERASE (CHI), FLAVANONE 3-HYDROXYLASE (F3H), DIHYDROFLAVONOL REDUCTASE (DFR), ANTHOCYANIDIN SYNTHASE (ANS), LEUCOANTHOCYANIDIN REDUCTASE (LAR) AND ANTHOCYANIDINN REDUCTASE (ANR) was significantly higher in K1 compared to T3 (Figure 9). Accordingly, also the total flavonoid and total phenolic contents were higher in K1 compared to T3 (Figures 10A,C). In addition, consistent with previous observations, total flavonoid and total phenolic contents of both genotypes correlated negatively with lesion area (Figures 10B,D; Ray et al., 2015).
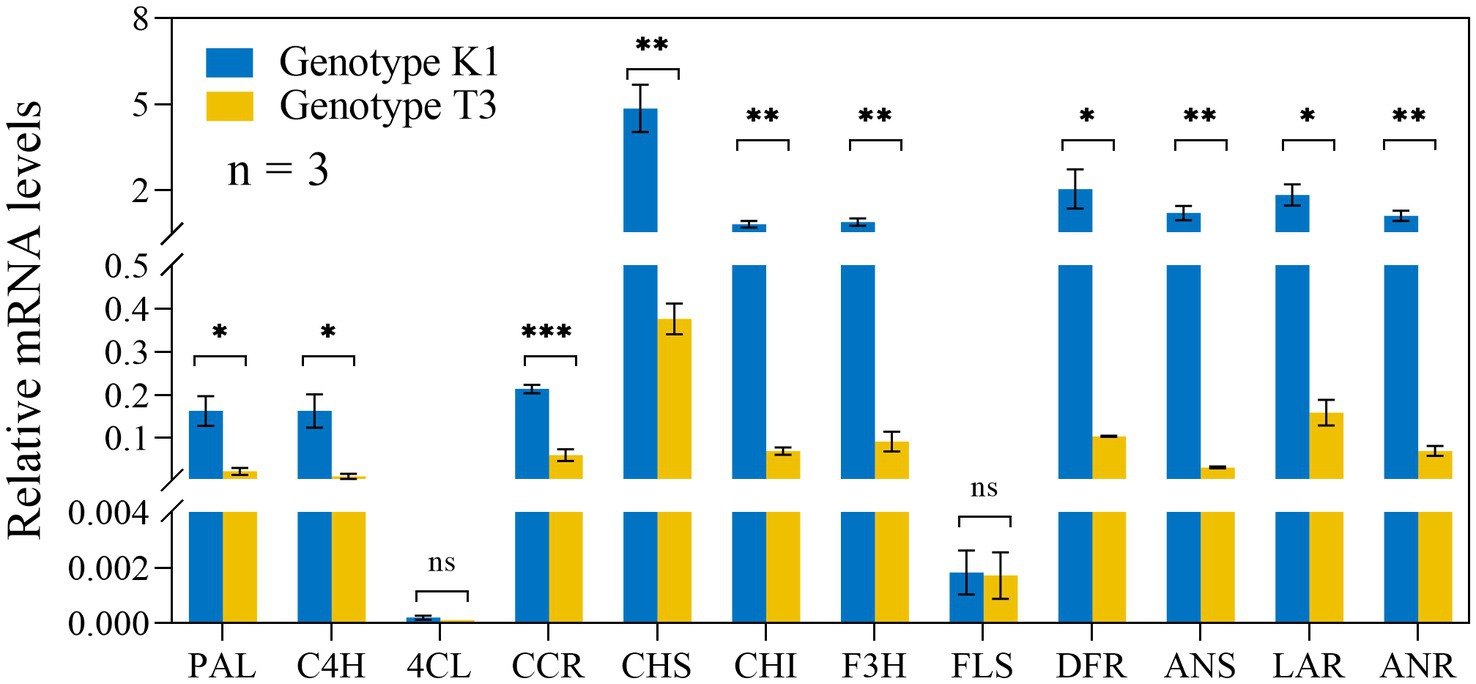
Figure 9. Relative expression levels of genes involved in the phenylpropanoid pathway in leaves of Kreuzkogel 1 (K1) and Tenno 3 (T3) without B. cinerea inoculation were measured via qRT-PCR. All values were normalized to the expression level of the H4 and UBC9 housekeeping genes. Significant differences between two genotypes, based on the Wilcox test, are represented by ns p > 0.05, *p < 0.05, **p < 0.01 and ***p < 0.001. PAL, PHENYLALANINE AMMONIA LYASE; C4H, CINNAMIC ACID 4-HYDROXYLASE; 4CL, 4-COUMAROYL-COA LIGASE; CCR: CINNAMOYL-COA REDUCTASE; CHS, CHALCONE SYNTHASE; CHI, CHALCONE ISOMERASE; F3H, FLAVANONE 3-HYDROXYLASE; FLS, FLAVONOL SYNTHASE; DFR, DIHYDROFLAVONOL REDUCTASE; ANS, ANTHOCYANIDIN SYNTHASE; LAR, LEUCOANTHOCYANIDIN REDUCTASE; ANR, ANTHOCYANIDIN REDUCTASE.
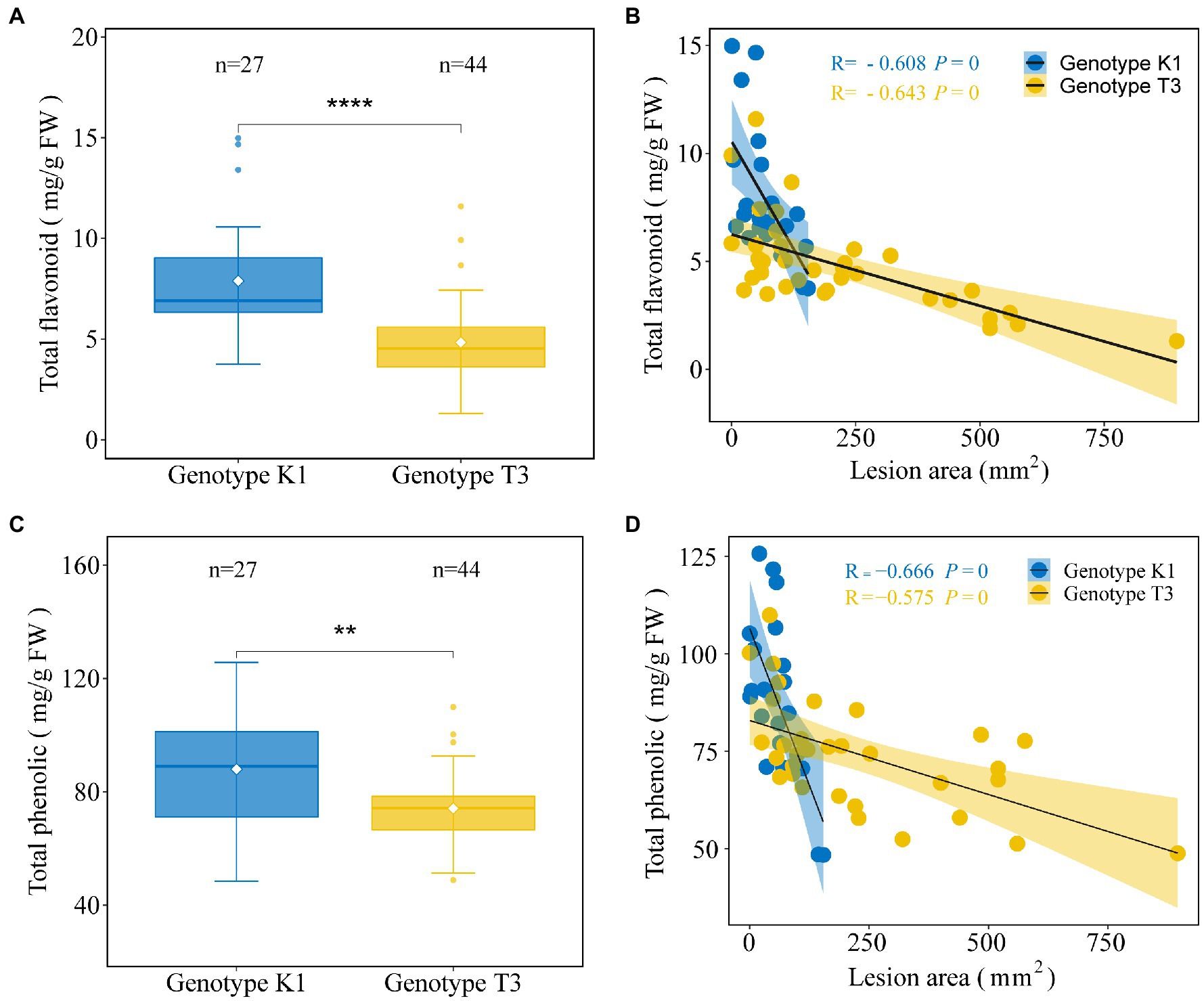
Figure 10. Analysis of total flavonoid and total phenolic contents of leaves of Kreuzkogel 1 (K1) and Tenno 3 (T3). The amount of total flavonoid (A) and total phenolic (C) in strawberry middle and right leaflets for K1 and T3 without B. cinerea inoculation. Significant differences between two genotypes, based on a t-test, are represented by **p < 0.01 and ****p < 0.0001. The individual points represent the outliers. The correlation between lesion area, measured on strawberry left leaflets, at 5 days after B. cinerea inoculation and total flavonoid (B) and total phenolic contents (D) in strawberry middle and right leaflets without B. cinerea inoculation for K1 and T3, respectively.
Several Primary Metabolites Contribute to the Higher Resistance of K1
Primary metabolites are precursors of specialized metabolites and several metabolites (sugars and amino acids) contribute to the physiological or morphological adaptations of strawberry plants to restrict pathogen invasion (Hu et al., 2019). Principal component analysis (PCA) and partial least squares regression (PLSR) were performed to investigate the effect of primary metabolites on plant resistance in leaves of T3 and K1. PCA analysis showed variance between the two genotypes (Figure 11A). Most variation (28.7%) was captured by PC 1 which was related to differences between the two genotypes, while PC 2 (18.1%) described the variation within the genotypes. In the PLSR correlation loading plot (Figure 11B), the metabolites, selected based on a jack-knifing test, that contributed most to the separation of the two genotypes were fructose, glucose, galactose, sucrose, trehalose, maltose, shikimic acid, citric acid, glyceric acid, phenylalanine, isoleucine, valine, proline, serine, lysine and leucine. The concentration of these metabolites and the correlation with lesion area for two genotypes are shown in Table 1.
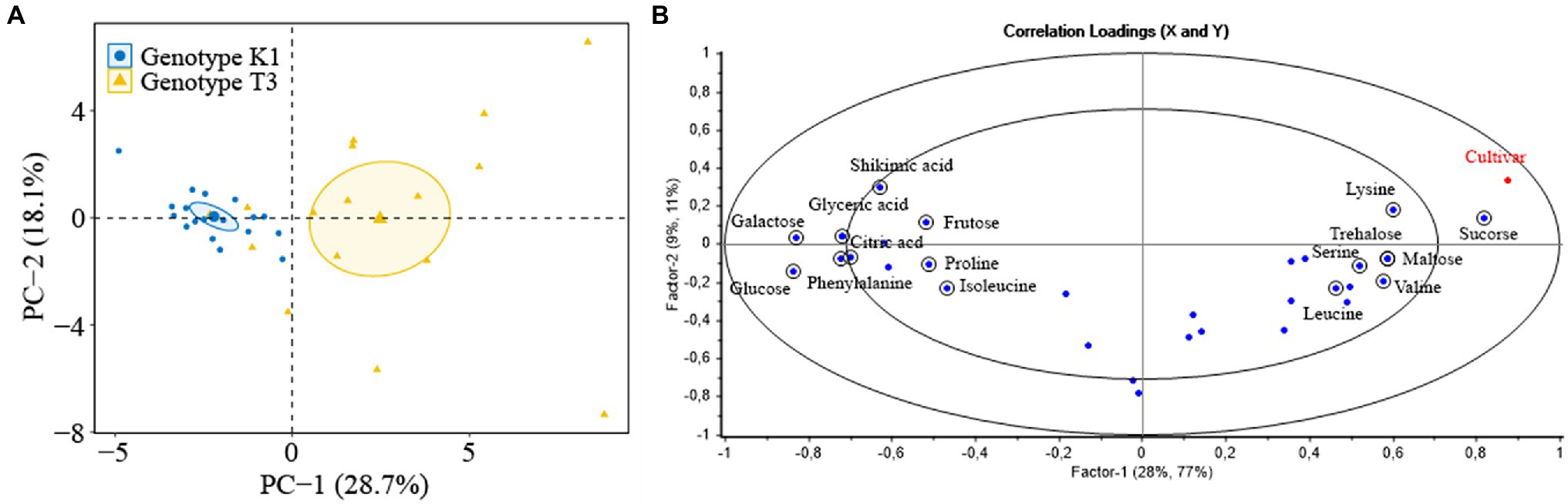
Figure 11. (A) PCA score plot of primary metabolites of strawberry leaves of Kreuzkogel 1 (K1) and Tenno 3 (T3). One dot represents one strawberry leaf, and PC-1 and PC-2 refer to the first and the second factor used to explain the variance. (B) Correlation loading plots of PLSR analysis with the primary metabolite profile of strawberry leaves of K1 and T3 as variables and cultivar (category) as response. The outer ellipse indicates 100% of explained variance, while the inner ellipse indicates 50% of explained variance. One blue dot represents one specific metabolite (X-factor), and encircled metabolites contributed to the separation of two genotypes which selected by jack-knifing test. The red dot represent the category cultivar (Y-factor).
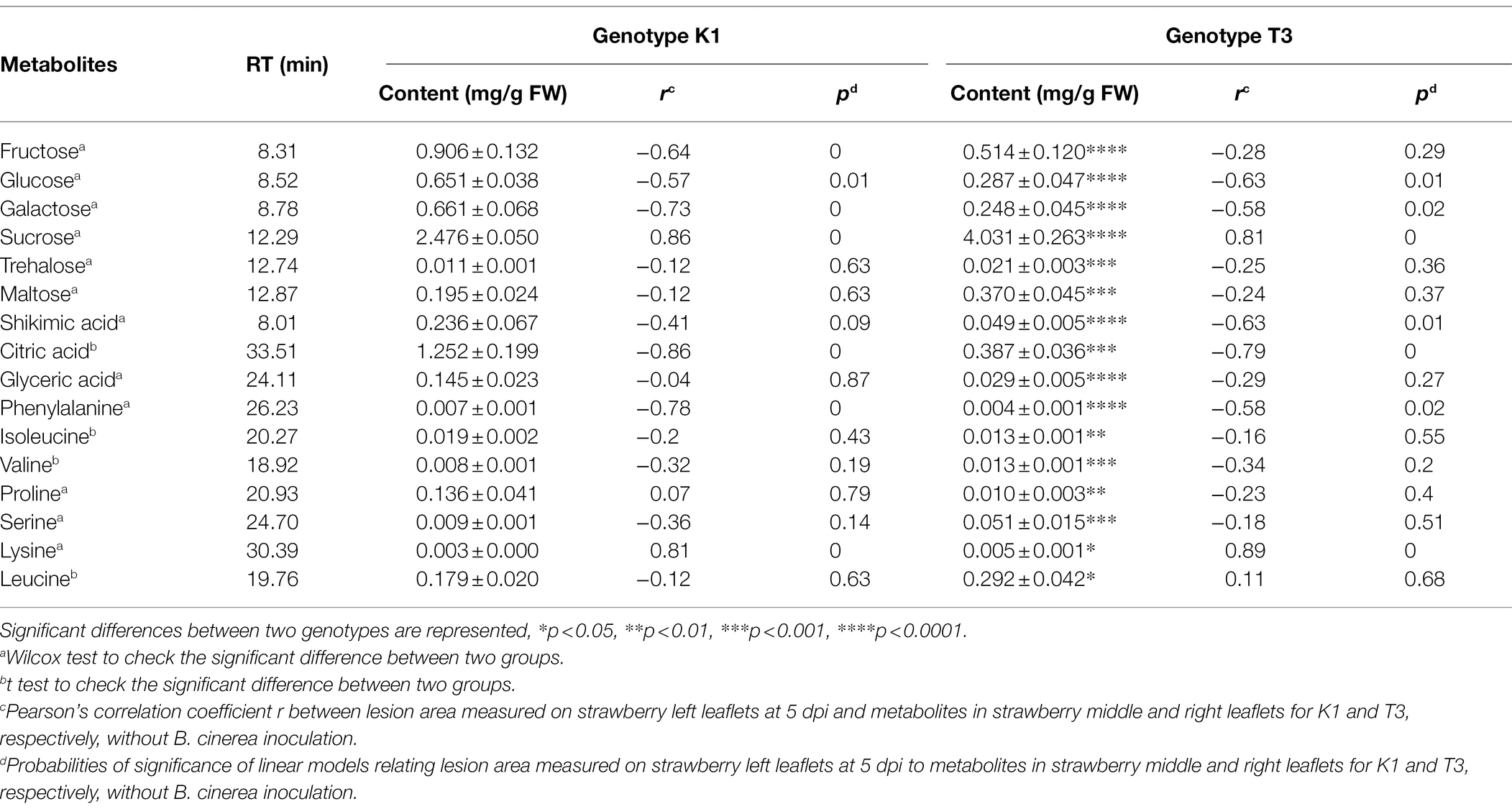
Table 1. Important primary metabolites based on the PLSR contributed to the separation of genotype Kreuzkogel 1 (K1) and Tenno 3 (T3).
As reported in Table 1, significant differences were observed for 16 metabolites selected from the PLSR approach between the two genotypes. In addition, three other metabolites that were not selected by PLSR analyses, fucose, quininic acid, and fumaric acid, also showed significant differences between the two genotypes (Supplementary Table 2). Furthermore, the amount of 11 metabolites is significant higher in K1 compared to T3 while for 9 metabolites the amount is lower in K1 compared to T3 (Table 1; Supplementary Table 2). Among the measured metabolites, glucose, galactose, citric acid and phenylalanine correlated negatively with LA, while sucrose and lysine correlated positively (p < 0.05). The amount of trehalose, maltose, glyceric acid, isoleucine, valine, proline, serine and leucine did not significantly correlate with lesion area in any of the two genotypes, while the amount of fructose and shikimic acid correlated with lesion area in genotype K1 and genotype T3, respectively.
Discussion
Strawberry is an important fruit crop grown in more than 70 countries (Van Lammerts Bueren et al., 2011). Gray mold caused by B. cinerea leads to substantial economic losses worldwide (Williamson et al., 2007). B. cinerea can infect multiple parts of the strawberry plant including fruit, flowers and leaves (Petrasch et al., 2019). Currently, fungicides are used to control B. cinerea on strawberries, which has resulted in the increased development of fungicide-resistant strains (Leroch et al., 2011, 2013; Veloukas et al., 2011; Bestfleisch et al., 2013). To solve this problem, breeding for B. cinerea-resistant genotypes could be an alternative management strategy (Roudeillac, 2003). In this study, a moderately resistant and susceptible F. vesca genotype were analyzed for H2O2 production, ASA, primary and specialized metabolites production, antioxidant enzyme activities and the expression of defense-related genes to investigate the constitutive resistance mechanisms present in both genotypes.
The Moderately Resistant Genotype K1 Limits Botrytis cinerea Growth Compared to the Susceptible Genotype T3
Our results on symptom development, qPCR and microscopy after B. cinerea inoculation demonstrate that K1 is more resistant to B. cinerea than T3 (Figures 1–3). A previous study, investigating the rate of adult Drosophila suzukii emergence on K1 and T3 fruit exposed to egg-laying fly females, found that less fly emergences occurred in K1 compared to T3 (Gong et al., 2016). Consequently, K1 may represent a valuable germplasm for breeding new varieties with resistance to biotic stress (Bestfleisch et al., 2015).
We found a significant higher development of hyphae on T3 leaves compared to K1 at 24 hpi (Figure 3), but for B. cinerea DNA, significant differences were observed only after 48 hpi (Figure 2). This suggests that the sensitivity of the qPCR assay is not high enough to distinguish the differences before 24 hpi. Overall, K1 can repress the B. cinerea hyphal growth at the early infection stage and possibly inhibit spore germination. A previous study on grapes reported a similar result where B. cinerea spores germinated at a lower rate on a resistant cultivar than on a susceptible cultivar (Wan et al., 2015). Several antioxidants can inhibit B. cinerea growth, for example, catechin and quercetin-3-galactoside inhibit germ tube elongation while gallic acid inhibits spore germination (Tao et al., 2010). In this case, antioxidants, including AsA, phenolics, flavonoids and primary metabolites, contributed to the growth restriction of B. cinerea in K1 compared to T3 (Figure 10; Table 1).
Summary of Potential Mechanisms for Higher Resistance of K1 to Botrytis cinerea Than T3
Based on the results of this study, a conceptual model is proposed showing how constitutive levels of primary and specialized metabolites, H2O2, AsA, antioxidant enzymes, and defense-related genes prior to B. cinerea inoculation generate a series of reactions in strawberry leaves that led to the increased resistance of K1 leaves to B. cinerea (Figure 12). Summarized, first the defense-related genes βGLU, JMT and AOS showed a higher expression in K1 compared to T3 which could result in the increased resistance of K1. Second, the H2O2 content was lower in K1 compared to T3 and a negative correlation between the H2O2 content and plant resistance to B. cinerea was observed. Moreover, the activity of both antioxidant enzymes APX and GPX was higher in K1 and could contribute to the lower H2O2 content. Third, the precursors glucose, shikimic acid and phenylalanine of the phenylpropanoid pathway were more abundant in K1 compared to T3. Moreover, a higher expression of genes involved in the phenylpropanoid biosynthesis pathway was observed, which correlated with the higher levels of total phenolics and total flavonoids observed. As such, these compounds could contribute to plant defense directly or indirectly through H2O2 scavenging. Fourth, H2O2 can also be scavenged by high levels of ascorbic acid observed in K1 and generated from glucose using galactose as intermediate. Additionally, the higher citric acid content in K1 potentially inhibits H2O2 by inducing gene expression and enzyme activity of antioxidative enzymes (APX, GPX). Finally, the higher amount of sucrose in T3 may serve as a nutrient to promote B. cinerea growth, thus decreasing plant resistance. Further details of this model are discussed in detail below based on available literature.
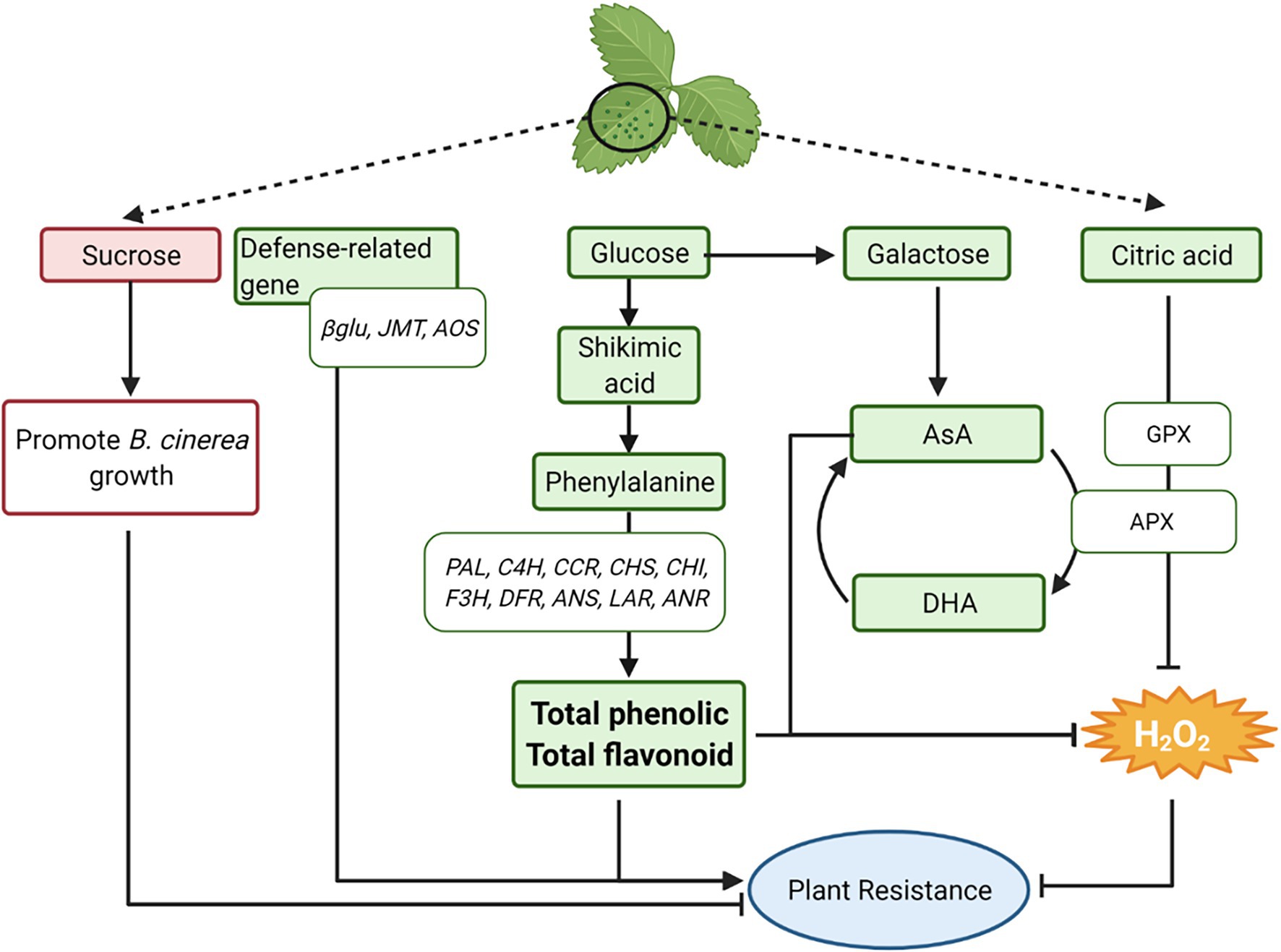
Figure 12. A conceptual model illustrating potential resistance mechanisms to B. cinerea in the more tolerant woodland strawberry F. vesca ssp. vesca Kreuzkogel 1 (K1) including primary metabolites, H2O2, phenolic compounds, ascorbic acid and defense-related genes. A detailed description of the figures can be found in the “Summary of Potential Mechanisms for Higher Resistance of K1 to B. cinerea Than T3”. The green boxes represent a higher content of the specific metabolites/genes in genotype K1 compared to T3, while the red boxes represent a lower content. βGLU: β-1,3-GLUCANASE, JMT: JASMONIC ACID CARBOXYL METHYLTRANSFERASE, AOS: ALLENE OXIDE SYNTHASE; PAL, PHENYLALANINE AMMONIA LYASE; C4H, CINNAMIC ACID 4-HYDROXYLASE; 4CL, 4-COUMAROYL-COA LIGASE; CCR: CINNAMOYL-COA REDUCTASE; CHS, CHALCONE SYNTHASE; CHI, CHALCONE ISOMERASE; F3H, FLAVANONE 3-HYDROXYLASE; FLS, FLAVONOL SYNTHASE; DFR, DIHYDROFLAVONOL REDUCTASE; ANS, ANTHOCYANIDIN SYNTHASE; LAR, LEUCOANTHOCYANIDIN REDUCTASE; ANR, ANTHOCYANIDIN REDUCTASE; APX: ASCORBATE PEROXIDASE, GPX, GLUTATHIONE PEROXIDASE. This figure has been created with BioRender.com.
Defense-Related Genes Involved in Strawberry Resistance to Botrytis cinerea
After B. cinerea inoculation, almost no induction of defense-related genes could be observed for both genotypes (Supplementary Figure 3). However, constitutive expression of the defense-related genes βGLU, AOS, and JMT, was higher in K1 compared to T3. βGLU can reduce pathogen virulence by degrading pathogen cell wall β-1,3-glucan. A higher expression of βGLU was observed in strawberries after treatment with β-aminobutyric acid treatment and resulted in increased resistance to B. cinerea. Moreover, after B. cinerea inoculation, βGLU was upregulated in strawberry fruit (Wang et al., 2016). AOS enzymes are involved in jasmonic acid biosynthesis (Farmer and Goossens, 2019), whereas JMT is responsible for converting JA to MeJA (Seo et al., 2001). The expression of JMT and AOS was higher in K1 compared to T3, suggesting that more JA and/or MeJA is present in K1, leading a stronger resistance to B. cinerea (Figure 4). The result is consistent with previous data reporting higher JA levels in a more resistant grape genotype compared to a susceptible genotype (Rahman et al., 2020). Moreover, a similar result was also found in Arabidopsis where overexpression of JMT resulted in increased resistance against B. cinerea (Seo et al., 2001). Overall, the higher expression of βGLU, JMT and AOS can contribute to the higher resistance of K1 to B. cinerea.
H2O2, Enzymatic Antioxidants and AsA Impact the Strawberry Resistance Against Botrytis cinerea
A hypersensitive response, leading to programmed cell death caused by an oxidative burst, is considered to be very important in restricting growth of biotrophic pathogens (Liu et al., 2019). Nevertheless, defense reactions effective against biotrophic pathogens are believed to increase susceptibility to necrotrophic pathogens since these micro-organisms can derive nutrients from dead cells (Lyon et al., 2007). For example, H2O2 levels correlated positively with B. cinerea growth and high H2O2 levels facilitate B. cinerea symptom development on Arabidopsis and strawberry leaves (Govrin and Levine, 2000; Meng et al., 2019). Moreover, a higher H2O2 content found in broad bean leaves after salicylic acid treatment under red light was associated with a higher susceptibility to B. cinerea (Khanam et al., 2005). In addition, B. cinerea infection can be suppressed by spraying antioxidants on plants (Elad, 1992). In grapes, low constitutive ROS production was also associated with a high level of resistance to B. cinerea (Rahman et al., 2020). Similar results were found in cucumber, where a lower accumulation of H2O2, O2− was found in a resistant genotype compared to a susceptible genotype after B. cinerea inoculation (Yang et al., 2020). In this study, we observed that T3 leaves have a higher level of H2O2 compared to K1 and that there was a significant positive correlation between H2O2 content and symptom development (i.e., Lesion area) for both genotypes (Figure 5). We can, therefore, hypothesize that the higher H2O2 content promotes B. cinerea infection.
The low constitutive level of H2O2 observed in K1 compared to T3 could be attributed to several mechanisms, such as a higher activity of antioxidant enzymes, a higher content of phenolic and flavonoid compounds and AsA (Figures 5–10, 12). In this study, a significantly higher expression of genes encoding GR, APX1, APX3 and GPX6_1 was observed in K1 compared to T3, while transcript levels of all other genes encoding antioxidant enzymes did not differ between the genotypes (Figure 6). Moreover, APX and GPX enzyme activities were also significantly higher in K1 compared to T3 (Figure 7). No significant differences were observed in CAT transcript levels and activity between the two genotypes, probably resulting from the higher affinity of APX for H2O2 than CAT (Pandey et al., 2017). Overall, these results suggest that K1 has more active H2O2 scavenging systems based on a higher GPX6_3, GR, APX1 and APX3 expression and higher GPX and APX activity compared to T3. Interestingly, treatment of plants, such as Brassica juncea, castor beans and sunflower, with citric acid increased the activity of SOD, CAT, APX, GPX and POD to reduce ROS content (Farid et al., 2017; Al Mahmud et al., 2018; Mallhi et al., 2019). Thus, the higher activity of APX and GPX in our study could be due to the higher citric acid content in K1 compared to T3 (Table 1; Figure 7). The importance of citric acid in increased plant resistance was further demonstrated by the negative correlation between citric acid content and lesion area (Table 1).
AsA is a well-known antioxidant that efficiently scavenges ROS (Horemans et al., 2000). A higher amount of AsA, APX activity and transcript levels and lower amounts of H2O2 were detected in K1 compared to T3 (Figures 5–8) which is in line with a previous report that AsA is participating in ROS scavenging via the action of APX (Das and Roychoudhury, 2014). The most important pathway to produce AsA is the L-galactose pathway which is generated from D-glucose via several intermediates, including L-galactose and fructose (Wheeler et al., 1998), higher concentrations of all these sugars were detected in K1 compared to T3 (Table 1; Supplementary Table 2; Figure 8). After AsA synthesis, APX oxidizes AsA to MDHA which spontaneously disproportionated into DHA. In turn, DHA can be reduced back to AsA by DHAR (Dell’Aglio and Mhamdi, 2020). Furthermore, previous research found a positive correlation between constitutive AsA levels and fruit resistance to B. cinerea in apple (Davey et al., 2007). Similarly, higher AsA levels were found on sun-exposed, less susceptible side of apple than the shaded side (Bui et al., 2019). In general, the higher levels of AsA and APX activities in K1 contribute to the decreased H2O2 content and increased tolerance to B. cinerea.
Phenolic Compounds and Their Biosynthesis Precursors and Intermediates Contribute to the Strawberry Resistance Against Botrytis cinerea
Phenolic compounds can have ROS scavenging activity and play an important role in plant defense (Pollastri and Tattini, 2011; Agati et al., 2012; Bose et al., 2014; Yang et al., 2018). Phenylpropanoids are synthesized via phenylalanine, derived from the shikimate pathway with shikimic acid as a central metabolite. In our study, K1 showed a higher level of phenolic compounds (shikimic acid, phenylalanine, total phenolics and flavonoids) compared to T3, and all compounds content showed a highly positive correlation with plant defense (Figures 9, 10; Table 1). The increased level of the total phenolics and flavonoids observed in K1 could be connected with the higher level of shikimic acid and phenylalanine and the higher expression of the genes involved in the phenylpropanoid pathway including PAL, C4H, CCR, CHS, CHI, F3H, DFR, ANS, LAR, and ANR. This result is in line with previous studies that unripe strawberry fruit with higher amounts of flavonoids and phenolics are less susceptible to B. cinerea (Di Venere et al., 1998; Puhl and Treutter, 2008; Aaby et al., 2012). Moreover, the higher levels of total phenolics after red light treatment also contributed to the increase in strawberry leaf tolerance to B. cinerea (Meng et al., 2019). Consequently, phenolic and flavonoid compounds in strawberry leaves play an important role as constitutive resistance mechanism against B. cinerea either directly or via scavenging H2O2.
Sucrose May Promote Botrytis cinerea Development in Strawberry
The increased accumulation of sucrose and total sugar content in T3 may promote B. cinerea development (Table 1; Supplementary Table 2). For example, sucrose was reported to promote B. cinerea growth and invasion in tomato leaves both in vitro and in vivo. Accordingly a tomato mutant with a lower total sugar content is less susceptible to B. cinerea (Asai et al., 2016; Courbier et al., 2020).
Conclusion
In this study, we show that K1 is more resistant to B. cinerea than T3 and that the increased resistance is due to a combination of constitutive resistance mechanisms mainly linked to the antioxidative profile of K1. We observed a lower amount of H2O2 in leaves of K1 correlating with a higher activity of the antioxidant enzymes APX and GPX and a higher amount of non-enzymatic antioxidants (phenolic compounds, citric acid, and ascorbic acid). The increased level of phenolic compounds, caused by the higher expression of genes involved in the phenylpropanoid pathway and a higher level of the shikimic acid and phenylalanine, inhibited the build-up of high H2O2 levels could also directly contribute to plant resistance. Citric acid potentially reduced H2O2 levels by upregulating the activity of APX and GPX; similarly, high levels of AsA produced by increased levels of glucose and galactose might have resulted in reduced H2O2 content. In conclusion, our study reveals that the innate antioxidative profile of strawberry leaves plays a major role in the resistance of woodland strawberry leaves against B. cinerea.
Data Availability Statement
The original contributions presented in the study are included in the article/Supplementary Material, further inquiries can be directed to the corresponding author.
Author Contributions
YZ, BC, and MH designed the experiments. YZ performed all experiments, whereas KT was involved in the GC–MS experiments, and LV was involved in the disease assays, the quantification of fungal DNA, and the microscopic analysis. YZ analyzed the data and created all figures. YZ and BC wrote the manuscript with input from all co-authors. All authors read and approved the final manuscript.
Funding
YZ has been supported by the China Scholarship Council at the KU Leuven (No. 201706990031). BC acknowledges financial support from the BelOrta chair (ITP-LSBEL1-O2010).
Conflict of Interest
The authors declare that the research was conducted in the absence of any commercial or financial relationships that could be construed as a potential conflict of interest.
Publisher’s Note
All claims expressed in this article are solely those of the authors and do not necessarily represent those of their affiliated organizations, or those of the publisher, the editors and the reviewers. Any product that may be evaluated in this article, or claim that may be made by its manufacturer, is not guaranteed or endorsed by the publisher.
Acknowledgments
The authors thank the “Professor Staudt Collection” for providing plant material. The authors also thank Tom Struyf for his guidance with the measurement of the antioxidant enzymes.
Supplementary Material
The Supplementary Material for this article can be found online at: https://www.frontiersin.org/articles/10.3389/fpls.2022.912667/full#supplementary-material
Footnotes
References
Aaby, K., Mazur, S., Nes, A., and Skrede, G. (2012). Phenolic compounds in strawberry (Fragaria x ananassa Duch.) fruits: composition in 27 cultivars and changes during ripening. Food Chem. 132, 86–97. doi: 10.1016/j.foodchem.2011.10.037
Agati, G., Azzarello, E., Pollastri, S., and Tattini, M. (2012). Flavonoids as antioxidants in plants: location and functional significance. Plant Sci. 196, 67–76. doi: 10.1016/j.plantsci.2012.07.014
Akram, N. A., Shafiq, F., and Ashraf, M. (2017). Ascorbic acid-A potential oxidant scavenger and its role in plant development and abiotic stress tolerance. Front. Plant Sci. 8:613. doi: 10.3389/fpls.2017.00613
Al Mahmud, J., Hasanuzzaman, M., Nahar, K., Bhuyan, M. H. M. B., and Fujita, M. (2018). Insights into citric acid-induced cadmium tolerance and phytoremediation in Brassica juncea L.: coordinated functions of metal chelation, antioxidant defense and glyoxalase systems. Ecotoxicol. Environ. Saf. 147, 990–1001. doi: 10.1016/j.ecoenv.2017.09.045
Asai, Y., Kobayashi, Y., and Kobayashi, I. (2016). Increased expression of the tomato SISWEET15 gene During Grey Mold infection and the possible involvement of the sugar efflux to Apoplasm in the disease susceptibility. J Plant Pathol Microbiol 7:1. doi: 10.4172/2157-7471.1000329
Asselbergh, B., Curvers, K., França, S. C., Audenaert, K., Vuylsteke, M., Van Breusegem, F., et al. (2007). Resistance to Botrytis cinerea in sitiens, an abscisic acid-deficient tomato mutant, involves timely production of hydrogen peroxide and cell wall modifications in the epidermis. Plant Physiol. 144, 1863–1877. doi: 10.1104/pp.107.099226
Bestfleisch, M., Höfer, M., Hanke, M. V., Flachowsky, H., Richter, K., and Schulte, E. (2013). Breeding of resistant strawberry cultivars for organic fruit production - preliminary results with Botrytis cinerea. Acta Hortic. 976, 87–90. doi: 10.17660/ACTAHORTIC.2013.976.8
Bestfleisch, M., Luderer-Pflimpfl, M., Höfer, M., Schulte, E., Wünsche, J. N., Hanke, M.-V., et al. (2015). Evaluation of strawberry (Fragaria L.) genetic resources for resistance to Botrytis cinerea. Plant Pathol. 64, 396–405. doi: 10.1111/PPA.12278
Blanco-Ulate, B., Vincenti, E., Powell, A. L. T., and Cantu, D. (2013). Tomato transcriptome and mutant analyses suggest a role for plant stress hormones in the interaction between fruit and Botrytis cinerea. Front. Plant Sci. 4:142. doi: 10.3389/fpls.2013.00142
Bose, J., Rodrigo-Moreno, A., and Shabala, S. (2014). ROS homeostasis in halophytes in the context of salinity stress tolerance. J. Exp. Bot. 65, 1241–1257. doi: 10.1093/jxb/ert430
Botanga, C. J., Bethke, G., Chen, Z., Gallie, D. R., Fiehn, O., and Glazebrook, J. (2012). Metabolite profiling of Arabidopsis inoculated with Alternaria brassicicola reveals that ascorbate reduces disease severity. Mol. Plant-Microbe Interact. 25, 1628–1638. doi: 10.1094/MPMI-07-12-0179-R
Bui, T. T. A., Wright, S. A. I., Falk, A. B., Vanwalleghem, T., Van Hemelrijck, W., Hertog, M. L. A. T. M., et al. (2019). Botrytis cinerea differentially induces postharvest antioxidant responses in ‘Braeburn’ and ‘Golden delicious’ apple fruit. J. Sci. Food Agric. 99, 5662–5670. doi: 10.1002/JSFA.9827
Courbier, S., Grevink, S., Sluijs, E., Bonhomme, P., Kajala, K., Van Wees, S. C. M., et al. (2020). Far-red light promotes Botrytis cinerea disease development in tomato leaves via jasmonate-dependent modulation of soluble sugars. Plant Cell Environ. 43, 2769–2781. doi: 10.1111/pce.13870
Curvers, K., Seifi, H., Mouille, G., de Rycke, R., Asselbergh, B., Van Hecke, A., et al. (2010). Abscisic acid deficiency causes changes in cuticle permeability and pectin composition That influence tomato resistance to Botrytis cinerea. Plant Physiol. 154, 847–860. doi: 10.1104/PP.110.158972
Das, K., and Roychoudhury, A. (2014). Reactive oxygen species (ROS) and response of antioxidants as ROS-scavengers during environmental stress in plants. Front. Environ. Sci. 2:53. doi: 10.3389/fenvs.2014.00053
Davey, M. W., Auwerkerken, A., and Keulemans, J. (2007). Relationship of apple vitamin C and antioxidant contents to harvest date and postharvest pathogen infection. J. Sci. Food Agric. 87, 802–813. doi: 10.1002/JSFA.2777
Dell’Aglio, E., and Mhamdi, A. (2020). What are the roles for dehydroascorbate reductases and glutathione in sustaining ascorbate accumulation? Plant Physiol. 183, 11–12. doi: 10.1104/pp.20.00388
Di Venere, D., Linsalata, V., Ippolito, A., Nigro, F., Arcuti, P., and Lattanzio, V. (1998). “Endogenous phenolics, ripening and susceptibility of strawberry fruits (Fragaria x ananassa Duch.) to post-harvest diseases.” in XIXth International Conference on Polyphenols (FRA); September 1–4, 1998.
Elad, Y. (1992). The use of antioxidants (free radical scavengers) to control grey mould (Botrytis cinerea) and white mould (sclerotinia sclerotiomm) in various crops. Plant Pathol. 41, 417–426. doi: 10.1111/j.1365-3059.1992.tb02436.x
Elkorany, A., and Mohamed, R. (2008). The use of antioxidants to control grey mould and enhance yield and quality of strawberry. J. Agric. Env. Sci. Alex. Univ. 7, 1–30.
El-Shabrawi, H., Kumar, B., Kaul, T., Reddy, M. K., Singla-Pareek, S. L., and Sopory, S. K. (2010). Redox homeostasis, antioxidant defense, and methylglyoxal detoxification as markers for salt tolerance in Pokkali rice. Protoplasma 245, 85–96. doi: 10.1007/s00709-010-0144-6
Fahrentrapp, J., Ria, F., Geilhausen, M., and Panassiti, B. (2019). Detection of Gray Mold leaf infections prior to visual symptom appearance using a five-band multispectral sensor. Front. Plant Sci. 10:628. doi: 10.3389/fpls.2019.00628
FAOSTAT (2021). Available at: https://www.fao.org/faostat/en/#home (Accessed November 4, 2021).
Farid, M., Ali, S., Rizwan, M., Ali, Q., Abbas, F., Bukhari, S. A. H., et al. (2017). Citric acid assisted phytoextraction of chromium by sunflower; morpho-physiological and biochemical alterations in plants. Ecotoxicol. Environ. Saf. 145, 90–102. doi: 10.1016/j.ecoenv.2017.07.016
Farmer, E. E., and Goossens, A. (2019). Jasmonates: what ALLENE OXIDE SYNTHASE does for plants. J. Exp. Bot. 70, 3373–3378. doi: 10.1093/jxb/erz254
Fernández-Bautista, N., Domínguez-Núñez, J., Moreno, M. M., and Berrocal-Lobo, M. (2016). Plant tissue trypan blue staining During phytopathogen infection. Bio-Protocol 6, 2019–2021. doi: 10.21769/bioprotoc.2078
Foyer, C. H., and Noctor, G. (2016). Stress-triggered redox signalling: what’s in pROSpect? Plant Cell Environ. 39, 951–964. doi: 10.1111/pce.12621
Giampieri, F., Forbes-Hernandez, T. Y., Gasparrini, M., Alvarez-Suarez, J. M., Afrin, S., Bompadre, S., et al. (2015). Strawberry as a health promoter: An evidence based review. Food Funct. 6, 1386–1398. doi: 10.1039/c5fo00147a
Gill, S. S., and Tuteja, N. (2010a). Reactive oxygen species and antioxidant machinery in abiotic stress tolerance in crop plants. Plant Physiol. Biochem. 48, 909–930. doi: 10.1016/j.plaphy.2010.08.016
Gill, S. S., and Tuteja, N. (2010b). Reactive oxygen species and antioxidant machinery in abiotic stress tolerance in crop plants. Plant Physiol. Biochem. 48, 909–930. doi: 10.1016/J.PLAPHY.2010.08.016
Gong, X., Bräcker, L., Bölke, N., Plata, C., Zeitlmayr, S., Metzler, D., et al. (2016). Strawberry accessions with reduced Drosophila suzukii emergence From fruits. Front. Plant Sci. 7:1880. doi: 10.3389/fpls.2016.01880
Govrin, E. M., and Levine, A. (2000). The hypersensitive response facilitates plant infection by the necrotrophic pathogen Botrytis cinerea. Curr. Biol. 10, 751–757. doi: 10.1016/S0960-9822(00)00560-1
Haile, Z. M., Nagpala-De Guzman, E. G., Moretto, M., Sonego, P., Engelen, K., Zoli, L., et al. (2019). Transcriptome profiles of strawberry (Fragaria vesca) fruit interacting With Botrytis cinerea at different ripening stages. Front. Plant Sci. 10:1131. doi: 10.3389/fpls.2019.01131
Hébert, C., Charles, M. T., Gauthier, L., Willemot, C., Khanizadeh, S., and Cousineau, J. (2002). Strawberry proanthocyanidins: biochemical markers for Botrytis cinerea resistance and shelf-life predictability. Acta. Hortic. 567, 659–662. doi: 10.17660/ActaHortic.2002.567.143
Horemans, N., Foyer, H., Potters, G., and Asard, H. (2000). Ascorbate function and associated transport systems in plants. Plant Physiol. Biochem. 38, 531–540. doi: 10.1016/S0981-9428(00)00782-8
Hu, Z., Chang, X., Dai, T., Li, L., Liu, P., Wang, G., et al. (2019). Metabolic profiling to identify the latent infection of strawberry by Botrytis cinerea. Evol. Bioinforma. 15:1176934319838518. doi: 10.1177/1176934319838518
Huang, H., Ullah, F., Zhou, D. X., Yi, M., and Zhao, Y. (2019). Mechanisms of ROS regulation of plant development and stress responses. Front. Plant Sci. 10:800. doi: 10.3389/fpls.2019.00800
Jajic, I., Sarna, T., and Strzalka, K. (2015). Senescence, stress, and reactive oxygen species. Plan. Theory 4, 393–411. doi: 10.3390/plants4030393
Jersch, S., Scherer, C., Huth, G., and Schlösser, E. (1988). Proanthocyanidins as basis for quiescence of Botrytis cinerea in immature strawberry fruits/Proanthocyanidine als Ursache der Quieszenz von Botrytis cinerea in unreifen Erdbeerfrüchten on JSTOR. J. Plant Dis. Prot. 96, 365–378.
Jose, S., Abbey, J., Jaakola, L., and Percival, D. (2020). Selection and validation of reliable reference genes for gene expression studies from Monilinia vaccinii-corymbosi infected wild blueberry phenotypes. Sci. Rep. 10, 1–10. doi: 10.1038/S41598-020-68597-9
Junglee, S., Urban, L., Sallanon, H., and Lopez-Lauri, F. (2014). Optimized assay for hydrogen peroxide determination in plant tissue using potassium iodide. Am. J. Anal. Chem. 5, 730–736. doi: 10.4236/ajac.2014.511081
Kang, H.-C., Park, Y.-H., and Go, S.-J. (2003). Growth inhibition of a phytopathogenic fungus, Colletotrichum species by acetic acid. Microbiol. Res. 158, 321–326. doi: 10.1078/0944-5013-00211
Kärkönen, A., and Kuchitsu, K. (2015). Reactive oxygen species in cell wall metabolism and development in plants. Phytochemistry 112, 22–32. doi: 10.1016/j.phytochem.2014.09.016
Khanam, N. N., Ueno, M., Kihara, J., Honda, Y., and Arase, S. (2005). Suppression of red light-induced resistance in broad beans to Botrytis cinerea by salicylic acid. Physiol. Mol. Plant Pathol. 66, 20–29. doi: 10.1016/j.pmpp.2005.03.006
Leroch, M., Kretschmer, M., and Hahn, M. (2011). Fungicide resistance phenotypes of Botrytis cinerea isolates from commercial vineyards in south West Germany. J. Phytopathol. 159, 63–65. doi: 10.1111/J.1439-0434.2010.01719.X
Leroch, M., Plesken, C., Weber, R. W. S., Kauff, F., Scalliet, G., and Hahn, M. (2013). Gray Mold populations in German strawberry fields are resistant to multiple fungicides and dominated by a novel clade closely related to Botrytis cinerea. Appl. Environ. Microbiol. 79, 159–167. doi: 10.1128/AEM.02655-12
Li, Z., Wang, N., Wei, Y., Zou, X., Jiang, S., Xu, F., et al. (2020). Terpinen-4-ol enhances disease resistance of postharvest strawberry fruit more effectively than tea tree oil by activating the phenylpropanoid metabolism pathway. J. Agric. Food Chem. 68, 6739–6747. doi: 10.1021/acs.jafc.0c01840
Liu, C., Chen, L., Zhao, R., Li, R., Zhang, S., Yu, W., et al. (2019). Melatonin induces disease resistance to Botrytis cinerea in tomato fruit by activating Jasmonic acid Signaling pathway. J. Agric. Food Chem. 67, 6116–6124. doi: 10.1021/acs.jafc.9b00058
Lyon, G. D., Goodman, B. A., and Williamson, B. (2007). “Botrytis cinerea perturbs redox processes as an attack strategy in plants,” in Botrytis: Biology, Pathology and Control. eds. Y. Elad, B. Williamson, P. Tudzynski, and N. Delen (Netherlands: Springer), 119–141.
Mallhi, Z. I., Rizwan, M., Mansha, A., Ali, Q., Asim, S., Ali, S., et al. (2019). Citric acid enhances plant growth, photosynthesis, and phytoextraction of Lead by alleviating the oxidative stress in Castor beans. Plan. Theory 8:525. doi: 10.3390/plants8110525
Matros, A., Peshev, D., Peukert, M., Mock, H. P., and Van Den Ende, W. (2015). Sugars as hydroxyl radical scavengers: proof-of-concept by studying the fate of sucralose in Arabidopsis. Plant J. 82, 822–839. doi: 10.1111/TPJ.12853
Mehli, L., Kjellsen, T. D., Dewey, F. M., and Hietala, A. M. (2005). A case study from the interaction of strawberry and Botrytis cinerea highlights the benefits of comonitoring both partners at genomic and mRNA level. New Phytol. 168, 465–474. doi: 10.1111/j.1469-8137.2005.01526.x
Mehli, L., Schaart, J. G., Kjellsen, T. D., Tran, D. H., Salentijn, E. M. J., Schouten, H. J., et al. (2004). A gene encoding a polygalacturonase-inhibiting protein (PGIP) shows developmental regulation and pathogen-induced expression in strawberry. New Phytol. 163, 99–110. doi: 10.1111/j.1469-8137.2004.01088.x
Meng, L., Höfte, M., and Van Labeke, M. C. (2019). Leaf age and light quality influence the basal resistance against Botrytis cinerea in strawberry leaves. Environ. Exp. Bot. 157, 35–45. doi: 10.1016/j.envexpbot.2018.09.025
Mukherjee, M., Larrimore, K. E., Ahmed, N. J., Bedick, T. S., Barghouthi, N., Traw, M. B., et al. (2010). Ascorbic acid deficiency in arabidopsis induces constitutive priming that is dependent on hydrogen peroxide, salicylic acid, and the NPR1 gene. Mol. Plant-Microbe Interact. 23, 340–351. doi: 10.1094/MPMI-23-3-0340
Nagpala, E. G., Guidarelli, M., Gasperotti, M., Masuero, D., Bertolini, P., Vrhovsek, U., et al. (2016). Polyphenols variation in fruits of the susceptible strawberry cultivar Alba during ripening and upon fungal pathogen interaction and possible involvement in unripe fruit tolerance. J. Agric. Food Chem. 64, 1869–1878. doi: 10.1021/acs.jafc.5b06005
Nakajima, M., and Akutsu, K. (2014). Virulence factors of Botrytis cinerea. J. Gen. Plant Pathol. 80, 15–23. doi: 10.1007/s10327-013-0492-0
Pandey, S., Fartyal, D., Agarwal, A., Shukla, T., James, D., Kaul, T., et al. (2017). Abiotic stress tolerance in plants: myriad roles of ascorbate peroxidase. Front. Plant Sci. 8:581. doi: 10.3389/FPLS.2017.00581/FULL
Pavet, V., Olmos, E., Kiddle, G., Mowla, S., Kumar, S., Antoniw, J., et al. (2005). Ascorbic acid deficiency activates cell death and disease resistance responses in Arabidopsis. Plant Physiol. 139, 1291–1303. doi: 10.1104/pp.105.067686
Peian, Z., Haifeng, J., Peijie, G., Sadeghnezhad, E., Qianqian, P., Tianyu, D., et al. (2021). Chitosan induces jasmonic acid production leading to resistance of ripened fruit against Botrytis cinerea infection. Food Chem. 337:127772. doi: 10.1016/j.foodchem.2020.127772
Petrasch, S., Knapp, S. J., van Kan, J. A. L., and Blanco-Ulate, B. (2019). Grey mould of strawberry, a devastating disease caused by the ubiquitous necrotrophic fungal pathogen Botrytis cinerea. Mol. Plant Pathol. 20, 877–892. doi: 10.1111/mpp.12794
Pollastri, S., and Tattini, M. (2011). Flavonols: old compounds for old roles. Ann. Bot. 108, 1225–1233. doi: 10.1093/aob/mcr234
Pourreza, N. (2013). Phenolic compounds as potential antioxidant. Jundishapur J. Nat. Pharm. Prod. 8, 149–150. doi: 10.17795/jjnpp-15380
Puhl, I., and Treutter, D. (2008). Ontogenetic variation of catechin biosynthesis as basis for infection and quiescence of Botrytis cinerea in developing strawberry fruits. J. Plant Dis. Prot. 115, 247–251. doi: 10.1007/BF03356272
Rahman, M. U., Hanif, M., Wan, R., Hou, X., Ahmad, B., and Wang, X. (2018). Screening Vitis genotypes for responses to Botrytis cinerea and evaluation of antioxidant enzymes, reactive oxygen species and Jasmonic acid in resistant and susceptible hosts. Molecules 24, 5–24. doi: 10.3390/MOLECULES24010005
Rahman, M. U., Ma, Q., Ahmad, B., Hanif, M., and Zhang, Y. (2020). Histochemical and microscopic studies predict that grapevine genotype “ju mei gui” is highly resistant against Botrytis cinerea. Pathogens 9:253. doi: 10.3390/pathogens9040253
Ray, S., Mondal, S., Chowdhury, S., and Kundu, S. (2015). Differential responses of resistant and susceptible tomato varieties to inoculation with Alternaria solani. Physiol. Mol. Plant Pathol. 90, 78–88. doi: 10.1016/j.pmpp.2015.04.002
Rojas, C. M., Senthil-Kumar, M., Tzin, V., and Mysore, K. (2014). Regulation of primary plant metabolism during plant-pathogen interactions and its contribution to plant defense. Front. Plant Sci. 5:17. doi: 10.3389/FPLS.2014.00017
Roudeillac, P. (2003). The strawberry of the future: A necessary alliance between the discoveries of the breeders and the know-how of the growers to address food safety and consumer satisfaction. Acta. Hortic. :626, 75–83. doi: 10.17660/actahortic.2003.626.9
Schaart, J. G. (2004). Towards Consumer-Friendly Cisgenic Strawberries which Are Less Susceptible to Botrytis cinerea. Netherlands: Wageningen University and Research.
Schmittgen, T. D., and Livak, K. J. (2008). Analyzing real-time PCR data by the comparative CT method. Nat. Protoc. 36, 1101–1108. doi: 10.1038/NPROT.2008.73
Sedmak, J. J., and Grossberg, S. E. (1977). A rapid, sensitive, and versatile assay for protein using Coomassie brilliant blue G250. Anal. Biochem. 79, 544–552. doi: 10.1016/0003-2697(77)90428-6
Seo, H. S., Song, J. T., Cheong, J. J., Lee, Y. H., Lee, Y. W., Hwang, I., et al. (2001). Jasmonic acid carboxyl methyltransferase: A key enzyme for jasmonate-regulated plant responses. Proc. Natl. Acad. Sci. U. S. A. 98, 4788–4793. doi: 10.1073/pnas.081557298
Shao, Y., Guo, M., He, X., Fan, Q., Wang, Z., Jia, J., et al. (2019). Constitutive H2O2 is involved in sorghum defense against aphids. Rev. Bras. Bot. 42, 271–281. doi: 10.1007/s40415-019-00525-2
Stevens, R., Buret, M., Garchery, C., Carretero, Y., and Causse, M. (2006). Technique for rapid, small-scale analysis of vitamin C levels in fruit and application to a tomato mutant collection. J. Agric. Food Chem. 54, 6159–6165. doi: 10.1021/jf061241e
Suarez, M. B., Walsh, K., Boonham, N., O’Neill, T., Pearson, S., and Barker, I. (2005). Development of real-time PCR (TaqMan®) assays for the detection and quantification of Botrytis cinerea in planta. Plant Physiol. Biochem. 43, 890–899. doi: 10.1016/j.plaphy.2005.07.003
Takahama, U., and Oniki, T. (1997). A peroxidase/phenolics/ascorbate system can scavenge hydrogen peroxide in plant cells. Physiol. Plant. 101, 845–852. doi: 10.1111/J.1399-3054.1997.TB01072.X
Tamari, F., Hinkley, C. S., and Ramprashad, N. (2013). A comparison of DNA extraction methods using Petunia hybrida tissues. J. Biomol. Tech. 24, 113–118. doi: 10.7171/jbt.13-2403-001
Tao, S., Zhang, S., Tsao, R., Charles, M. T., Yang, R., and Khanizadeh, S. (2010). In vitro antifungal activity and mode of action of selected polyphenolic antioxidants on Botrytis cinerea. Arch. Phytopathol. Plant Prot. 43, 1564–1578. doi: 10.1080/03235400802583834
Terzoudis, K., Hertog, M. L. A. T., and Nicolaï, B. M. (2022). Dynamic labelling reveals central carbon metabolism responses to stepwise decreasing hypoxia and reoxygenation during postharvest in pear fruit. Postharvest Biol. Technol. 186:111816. doi: 10.1016/J.POSTHARVBIO.2021.111816
Ulrich, D., Hoberg, E., Rapp, A., and Kecke, S. (1997). Analysis of strawberry flavour - discrimination of aroma types by quantification of volatile compounds. Eur. Food Res. Technol. 205, 218–223. doi: 10.1007/s002170050154
Van Lammerts Bueren, E. T., Wilbois, K. P., Baker, B., and Raaijmakers, M. (2011). “Values and principles in organic farming and consequences for breeding approaches and techniques,” in Organic Crop Breeding E. T. Van Bueren and J. R. Myers (United States: Wiley-Blackwell), 125–138.
Van Rensburg, H. C. J., Limami, A. M., and Van den Ende, W. (2021). Spermine and spermidine priming against Botrytis cinerea modulates ros dynamics and metabolism in arabidopsis. Biomol. Ther. 11, 1–25. doi: 10.3390/BIOM11020223
Veloukas, T., Leroch, M., Hahn, M., and Karaoglanidis, G. S. (2011). Detection and molecular characterization of boscalid-resistant Botrytis cinerea isolates from strawberry. Plant Dis. 95, 1302–1307. doi: 10.1094/PDIS-04-11-0317
Wan, R., Hou, X., Wang, X., Qu, J., Singer, S. D., Wang, Y., et al. (2015). Resistance evaluation of Chinese wild Vitis genotypes against Botrytis cinerea and different responses of resistant and susceptible hosts to the infection. Front. Plant Sci. 6:854. doi: 10.3389/fpls.2015.00854
Wang, K., Liao, Y., Xiong, Q., Kan, J., Cao, S., and Zheng, Y. (2016). Induction of direct or priming resistance against Botrytis cinerea in strawberries by β-aminobutyric acid and their effects on sucrose metabolism. J. Agric. Food Chem. 64, 5855–5865. doi: 10.1021/acs.jafc.6b00947
Wang, S. Y., and Lin, H. S. (2000). Antioxidant activity in fruits and leaves of blackberry, raspberry, and strawberry varies with cultivar and developmental stage. J. Agric. Food Chem. 48, 140–146. doi: 10.1021/jf9908345
Weber, N., Veberic, R., Rescic, J., Mikulic-Petkovsek, M., Stampar, F., Koron, D., et al. (2016). Alternative products against anthracnose affect selected primary and secondary metabolites in strawberry fruit. Fruits 71, 363–371. doi: 10.1051/fruits/2016028
Wheeler, G. L., Jones, M. A., and Smirnoff, N. (1998). The biosynthetic pathway of vitamin C in higher plants. Nature 393, 365–369. doi: 10.1038/30728
Williamson, B., Tudzynski, B., Tudzynski, P., and Van Kan, J. A. L. (2007). Botrytis cinerea: The cause of grey mould disease. Mol. Plant Pathol. 8, 561–580. doi: 10.1111/j.1364-3703.2007.00417.x
Xiong, J. S., Zhu, H. Y., Bai, Y. B., Liu, H., and Cheng, Z. M. (2018). RNA sequencing-based transcriptome analysis of mature strawberry fruit infected by necrotrophic fungal pathogen Botrytis cinerea. Physiol. Mol. Plant Pathol. 104, 77–85. doi: 10.1016/j.pmpp.2018.08.005
Yang, C., Liang, Y., Qiu, D., Zeng, H., Yuan, J., and Yang, X. (2018). Lignin metabolism involves Botrytis cinerea BcGs1-induced defense response in tomato. BMC Plant Biol. 18:103. doi: 10.1186/s12870-018-1319-0
Yang, Y., Wang, X., Chen, P., Zhou, K., Xue, W., Abid, K., et al. (2020). Redox status, JA and ET Signaling pathway regulating responses to Botrytis cinerea infection Between the resistant cucumber genotype and its susceptible mutant. Front. Plant Sci. 11:1467. doi: 10.3389/FPLS.2020.559070/BIBTEX
Yu, D., Tang, H., Zhang, Y., Du, Z., Yu, H., and Chen, Q. (2012). Comparison and improvement of different methods of RNA isolation from strawberry (Fragria * ananassa). J. Agric. Sci. 4:p51. doi: 10.5539/jas.v4n7p51
Zhang, Y., Peng, X., Liu, Y., Li, Y., Luo, Y., Wang, X., et al. (2018). Evaluation of suitable reference genes for qRT-PCR normalization in strawberry (Fragaria × ananassa) under different experimental conditions. BMC Mol. Biol. 19, 1–10. doi: 10.1186/S12867-018-0109-4/FIGURES/4
Ziv, C., Kumar, D., Sela, N., Itkin, M., Malitsky, S., Schaffer, A. A., et al. (2020). Sugar-regulated susceptibility of tomato fruit to Colletotrichum and penicillium requires differential mechanisms of pathogenicity and fruit responses. Environ. Microbiol. 22, 2870–2891. doi: 10.1111/1462-2920.15031
Keywords: woodland strawberry, Botrytis cinerea, defense-related genes, hydrogen peroxide, antioxidant enzymes, ascorbic acid, specialized metabolites, primary metabolites
Citation: Zhao Y, Vlasselaer L, Ribeiro B, Terzoudis K, Van den Ende W, Hertog M, Nicolaï B and De Coninck B (2022) Constitutive Defense Mechanisms Have a Major Role in the Resistance of Woodland Strawberry Leaves Against Botrytis cinerea. Front. Plant Sci. 13:912667. doi: 10.3389/fpls.2022.912667
Edited by:
Kanniah Rajasekaran, Agricultural Research Service, United States Department of Agriculture, United StatesReviewed by:
Satyabrata Nanda, Centurion University of Technology and Management, IndiaJuan Carlos Díaz Ricci, Universidad Nacional de Tucumán, Argentina
Copyright © 2022 Zhao, Vlasselaer, Ribeiro, Terzoudis, Van den Ende, Hertog, Nicolaï and De Coninck. This is an open-access article distributed under the terms of the Creative Commons Attribution License (CC BY). The use, distribution or reproduction in other forums is permitted, provided the original author(s) and the copyright owner(s) are credited and that the original publication in this journal is cited, in accordance with accepted academic practice. No use, distribution or reproduction is permitted which does not comply with these terms.
*Correspondence: Barbara De Coninck, YmFyYmFyYS5kZWNvbmluY2tAa3VsZXV2ZW4uYmU=