- 1Key Laboratory of Southwest China Wildlife Resources Conservation (Ministry of Education), College of Life Science, China West Normal University, Nanchong, China
- 2College of Environmental Science and Engineering, China West Normal University, Nanchong, China
- 3School of Agricultural Science, Xichang College, Xichang, China
Poaceae is the most prominent monocot family that contains the primary cereal crops wheat, rice, and maize. These cereal species exhibit physiological diversity, such as different photosynthetic systems and environmental stress tolerance. Phosphoenolpyruvate carboxylase (PEPC) in Poaceae is encoded by a small multigene family and plays a central role in C4-photosynthesis and dicarboxylic acid metabolism. Here, to better understand the molecular basis of the cereal species diversity, we analyzed the PEPC gene family in wheat together with other grass species. We could designate seven plant-type and one bacterial-type grass PEPC groups, ppc1a, ppc1b, ppc2a, ppc2b, ppc3, ppc4, ppcC4, and ppc-b, respectively, among which ppc1b is an uncharacterized type of PEPC. Evolutionary inference revealed that these PEPCs were derived from five types of ancient PEPCs (ppc1, ppc2, ppc3, ppc4, and ppc-b) in three chromosomal blocks of the ancestral Poaceae genome. C4-photosynthetic PEPC (ppcC4) had evolved from ppc1b, which seemed to be arisen by a chromosomal duplication event. We observed that ppc1b was lost in many Oryza species but preserved in Pooideae after natural selection. In silico analysis of cereal RNA-Seq data highlighted the preferential expression of ppc1b in upper ground organs, selective up-regulation of ppc1b under osmotic stress conditions, and nitrogen response of ppc1b. Characterization of wheat ppc1b showed high levels of gene expression in young leaves, transcriptional responses under nitrogen and abiotic stress, and the presence of a Dof1 binding site, similar to ppcC4 in maize. Our results indicate the evolving status of Poaceae PEPCs and suggest the functional association of ppc1-derivatives with adaptation to environmental changes.
Introduction
Poaceae (the grass family) is the largest group for monocot plant species and contains several primary cereal crops, such as wheat, rice, maize, and sorghum. These cereals are categorized into three subfamilies, Pooideae, Ehrhartoideae, and Panicoideae. Pooideae includes important cereals, wheat, barley, and oats and is represented by a model grass Brachypodium distachyon (The International Brachypodium Initiative, 2010). Ehrhartoideae contains rice, and Panicoideae does maize and sorghum (Prasad et al., 2011). These three taxonomic groups were established with complex genomic events (Salse et al., 2008; Jiao et al., 2014). Grass species exhibit various physiological properties in photosynthetic systems and abiotic stress tolerance (Pardo and VanBuren, 2021). Understanding of the molecular basis of the diversity of grass species is necessary to develop useful cultivated cereal plants further.
One of the carbon-fixation enzymes, phosphoenolpyruvate carboxylase (PEPC), is indispensable to plant individuals. PEPC catalyzes the irreversible β-carboxylation of phosphoenolpyruvate (PEP) by incorporating HCO3− to yield oxaloacetate (O’Leary et al., 2011). This enzyme is well recognized as the central photosynthetic enzyme in C4 and Crassulacean acid metabolism (CAM) plants while it works on the anaplerotic provision of carbon skeletons to the citrate acid cycle in bacteria (Kai et al., 2003; von Caemmerer and Furbank, 2016). Plant PEPCs play roles in nitrogen metabolism, fatty acid biosynthesis, and respiration (O’Leary et al., 2011; Shi et al., 2015; Yamamoto et al., 2015, 2020). In addition, PEPC might act on re-fixing CO2 released by respiration and is likely to act on abiotic stress adaptation (Sánchez et al., 2006; O’Leary et al., 2011; Kandoi et al., 2016).
The plant genomes maintain four to 10 PEPC isogenes (Sánchez et al., 2006; Masumoto et al., 2010; Wang et al., 2016; Waseem and Ahmad, 2019; Zhao et al., 2019). In plants, two primary PEPC classes are defined: plant-type and bacterial-type PEPC (O’Leary et al., 2011). Plant-type PEPCs are categorized into photosynthetic types comprising C4-photosynthetic PEPC, CAM-type PEPC, and non-photosynthetic type ones. Most non-photosynthetic PEPCs are cytosolic isoforms, but a chloroplast-targeted isoform was found in grass species rice (Masumoto et al., 2010). Cytosolic PEPCs are predominant in plant species with multiple isoforms, from which gene expression patterns differ (Caburatan and Park, 2021). In rice, four cytosolic PEPCs, one chloroplast PEPC, and one bacterial-type PEPC are known, but biological roles of these PEPCs seem to be not identical (Masumoto et al., 2010; Yamamoto et al., 2014a, 2015). The chloroplast-targeted PEPC Osppc4 supports ammonium assimilation in rice (Masumoto et al., 2010). Previous phylogenetic studies approached the evolutionary history of photosynthetic PEPCs in monocot species (Christin et al., 2007; Christin and Besnard, 2009; Deng et al., 2016). However, the origin and the evolutionary processes of grass PEPCs remain obscure. This fact is due to the complexity of the grass genome evolution, technical issues in the phylogenetic analyses, and unknown PEPC isogene compositions in Pooideae and Panicoideae.
In the present study, we conducted a cross-species genome-wide analysis of PEPCs in wheat and other grass species (the analytical scheme is shown in Figure 1). Our analyses identified wheat PEPCs, clarified the evolutionary history of grass PEPCs driven by chromosomal-level duplications, and revealed gene expression patterns of model grass PEPCs associated with environmental changes. We discovered a PEPC isoform group “ppc1b,” which is the natural origin of C4-photosynthetic PEPCs and originated from one of the ancient grass PEPC ppc1. RNA-Seq data analyses indicated abiotic stress responses of ppc1b in wheat, T. turgidum, Brachypodium, and barley. Verification of nitrogen-dependent response of PEPC in wheat revealed the selective response of ppc1b. We found evolutionary conservation of a Dof1 transcription factor binding site in the ppc1b promoter region. Overall, we represent the evolutionary history of grass PEPCs and designate the molecular groups of grass PEPCs for framing grass PEPC research. Finally, we discuss the biological significance of the molecular plasticity of ppc1b.
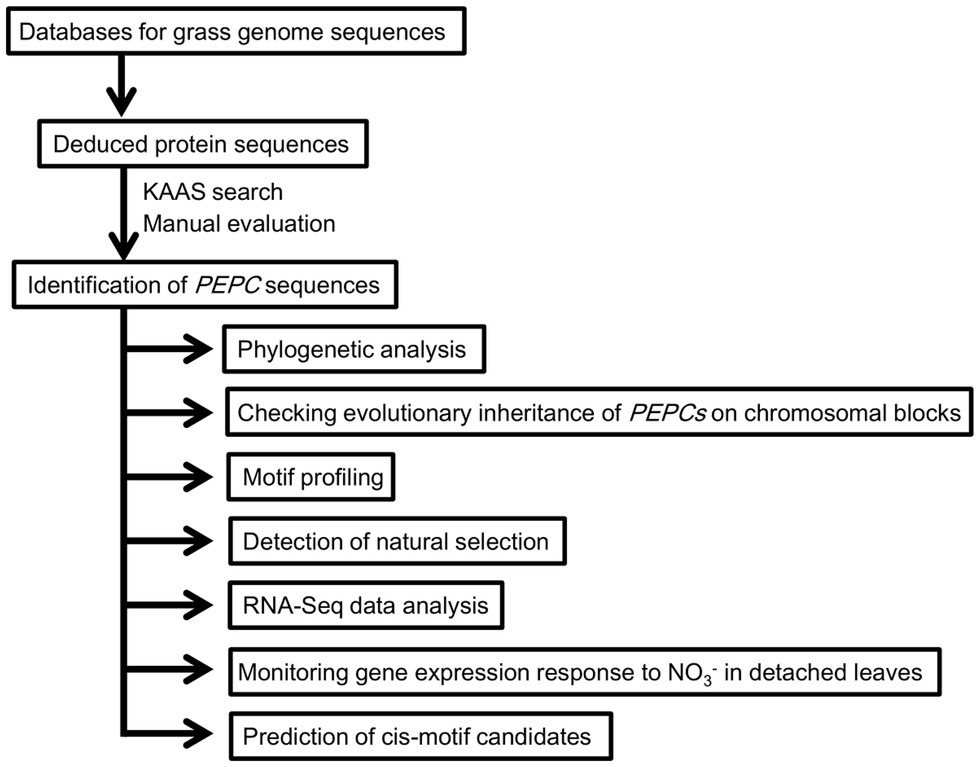
Figure 1. Schematic representation of cross-species genome-wide analysis of grass Phosphoenolpyruvate carboxylases (PEPCs).
Materials and Methods
Mining of PEPC Protein Sequences
The predicted protein sequences in Triticum aestivum L. (cultivar Chinese Spring) were collected from Ensembl Plant (Bolser et al., 2017, release 46) and queried against the KEGG metabolic pathway database1 by the assignment method of KAAS searches as a bi-directional best hit (Moriya et al., 2007). Proteins with the KEGG Orthology identifier K01595 (phosphoenolpyruvate carboxylase) were tentatively designated as PEPC and subjected to manual check to retain only reliable PEPC sequences. The wheat PEPC loci were named according to the homologies to the potential counterpart isoforms in rice. Predicted protein sequences in other 24 monocot species, Aegilops tauschii, B. distachyon, Eragrostis tef, Hordeum vulgare cultivar Morex and Golden Promise, Leesa perrieri, Musa acuminata, Oryza barthii, O. brachyantha, O. glaberrima, O. glumaepatula, O. longistaminata, O. rufipogon, O. meridionalis, O. nivara, O. punctata, and O. sativa indica varieties 93–11 and R498, O. sativa japonica variety kitaake, Panicum hallii, Setaria italica, S. viridis, Sorghum bicolor, T. dicoccoides, T. spelta, T. turgidum, and Zea mays, were also collected from Ensembl Plant. Predicted sequences for O. meyeriana var.granulata were from GenBank (accession: SPHZ00000000.2). Predicted protein sequences for Phyllostachys edulis were from BambooGBD (Zhao et al., 2018). All the protein sequences of O. officinalis (Shenton et al., 2020) were also collected. PEPC proteins for these protein data sets were also searched as mentioned above (Supplementary Table S1).
Analysis of mRNA Sequencing Data
Public RNA sequencing (RNA-Seq) profiles in wheat were downloaded from the U.S. National Center for Biotechnology Information Sequence Read Archive2 for monitoring the organ-specific gene expression patterns of PEPC isogenes in the reference cultivar Chinese Spring. The analyzed profiles for spatial gene expression patterns included 16 organs, including root, leaf, and stem (Supplementary Table S2). RNA-Seq profiles in grass species under abiotic stress conditions were also analyzed (Supplementary Table S2). These sequencing reads were trimmed by Trimmomatic version 0.39 (Bolger et al., 2014), and obtained high-quality reads were mapped on the reference genome of wheat using DART version 1.3.6 (Lin and Hsu, 2018) with the default condition. Generated bam files were sorted by SAMtools version 1.9 (Li et al., 2009) and processed by featureCounts version 2.0.1 (Liao et al., 2014) to obtain read counts per gene. The obtained count data were normalized by TCC version 1.30.0 with the iDEGES/edgeR method (Sun et al., 2013) and converted into transcript-level or gene-level (TPM) values. Averaged TPM values were applied in case replicated samples were available. HeatMapper (Verhaak et al., 2006) was used to visualize of the spatial gene expression patterns of wheat PEPC isogenes. Principal component analysis (PCA) of the gene expression levels for PEPC was conducted using the R statistical software3 with the multivariate exploratory data analysis package FactoMineR version 2.4 (Lê et al., 2008).
Plant Materials
Seeds of the common wheat variety Chinese spring were soaked with 0.01% KMnO4 solution for 30 min to sterilize their surface. After washing with purified water several times, the seeds were incubated in water at 4°C for 3 days. Then, the seeds were placed on wet filter papers under a dark condition. Germinating seeds were transplanted into a mixture of vermiculite and perlite (ratio 2:1) on plastic plant growth trays and placed in a laboratory space under natural light with a Murashige and Skoog-based medium, which lacks nitrogen nutrition (Supplementary Table S3). After 2 weeks, the bases of the main leaf blades were cut using a razor, and the detached leaves were incubated with 40 mM KNO3 solution or a mock solution of 20 mM K2SO4 (added SO42− instead of NO3− with the same strength of K+ ion) under a fluorescent light (approx. 15,000 lux) at 22°C with humidity of 60–70% (Supplementary Figure S1). The detached leaves at 0, 3, 6, 12, and 24 h after the treatment were harvested and quickly frozen in liquid nitrogen to be stored at −80°C until use.
Measurement of PEPC Activity and Protein Expression
Soluble proteins including PEPC were extracted from powdered leaf tissues in a buffer [100 mM Tris–HCl (pH 7.8); 1 mM EDTA; 1 mM 2-mercaptoethanol; 10% (w/v) glycerol] with Complete Protease Inhibitor Cocktail (F. Hoffmann-La Roche Ltd., Basel, Switzerland) at 4°C by using a motor and pestle. The homogenate was centrifuged at 13,000 g for 20 min, and the resultant supernatant was used for measuring PEPC activity by coupling with the malate dehydrogenase reaction according to the procedures of Yamamoto et al. (2014a). This PEPC assay was carried out in 2 mL of a solution containing 50 mM Tricine-KOH (pH 8.3), 5 mM MgSO4, 0.15 mM NADH, 5 mM KHCO3, 5 mM PEP (cyclohexylammonium salt), 4 mM DTT, and 3 U of pig heart malate dehydrogenase at 25°C. Three biological replicates were used for PEPC assays with two technical replicates. According to the manufacturer’s protocol, we determined soluble protein content by using Bradford Protein Assay Kit (TIANGEN BIOTECH CO., LTD, Beijing, China). Chromogenic Western blot analysis of PEPCs was carried out using a polyclonal antibody for maize leaf PEPC (Abcam plc, Cambridge, United Kingdom). The NBT/BCIP reaction scheme detected PEPC proteins.
Wheat RNA Preparation and cDNA Synthesis
Frozen leaves were powdered using a motor and pestle with liquid nitrogen. Approximately, 50 mg of the powdered sample was used for total RNA extraction with LABGENE plant RNA Isolation Kit (LABGENE Biotechnology Co., Ltd., Chengdu, China) according to the manufacturer’s protocol. The extracted total RNAs were analyzed by NanoDrop2000c Spectrophotometer (Thermo Fisher Scientific, Waltham, MA, United States) and gel electrophoresis to verify the quantity and the integrity. Approximately, 1 μg of total RNA fraction with no less than 1.9 of A260/A280 and 1.8 of A260/A230 was applied for first-strand cDNA synthesis using PrimeScript RT Reagent Kit with gDNA Eraser (Takara Bio Inc., Kusatsu, Japan).
cDNA Cloning and Prokaryotic Expression of Wheat PEPC
Phosphoenolpyruvate carboxylase (PEPC) cDNAs were amplified from first-stranded cDNAs synthesized from wheat leaf RNAs using gene-specific primers using KOD plus (Toyobo Co. LTD., Osaka, Japan). The amplified fragments were cloned into pMD19-T, and the insert sequences were determined by primer walking. The cDNA sequences of full-length wheat PEPC cDNAs were deposited to Genbank [accession number: ON055387-ON055389]. these will be released after acceptance of this manuscript.
The 678 bp cDNA fragment of Tappc1bD, which corresponded to its consensus N-terminal region of Tappc1b, was subcloned into the EcoRI and HindIII site of pET28a(+), and the constructed recombinant expression construct was transformed into Escherichia coli BL21 (DE3). Three independent Escherichia coli transformants were applied to the functional expression of the recombinant PEPC fragment assays. In the assays, transformed E. coli colonies were inoculated on LB medium and shaken at 180 rpm for 12 h at 30°C. Then 1 mL of the bacterial culture was added to 30 mL of fresh LB medium and shaken at 180 rpm at 37°C until OD = 600 reached 0.5. After adding IPTG at the final concentration of 0.4 mM, the bacterial cultures were shaken at 180 rpm at 25°C for 5 h. E. coli cells were collected by centrifugation and frozen at –80°C until soluble protein extraction. PEPC activities were measured using “Phosphoenolpyruvate Carboxylase Activity Assay Kit, Ultraviolet Colorimetric Method” (Sangon Biotech Co., Ltd., Shanghai, China).
Quantitative RT-PCR
The valid concentrations of cDNAs were estimated based on Ct value differences in a preliminary qRT-PCR assay for an actin gene (GenBank ID: AB1811991; Wei et al., 2015) and determined dilution factors of the original first-strand cDNA fractions to adjust the internal actin concentrations among samples. Then, 0.5 μL of the diluted cDNA fractions were assayed in qPCR using TB Green Premix Ex Taq II (Takara Bio Inc.) and gene-specific primers (Supplementary Table S4). Biologically triplicate assays were carried out with at least two technical replicates. Relative gene expression levels to actin were calculated by the 2-ΔΔCT method (Livak and Schmittgen, 2001).
Comparison of Promoter Sequences of PEPC
Three-kilo base upstream regions of the transcribed regions for PEPC isogenes were retrieved. Potential cis-regulatory motifs were searched using Multiple Em for Motif Elicitation (MEME; Bailey et al., 2015). The search was performed by the discriminative mode, which allowed for mining motifs seen in Tappc1b, ppcC4 isogenes, and Tappc4 but not found in the other eight PEPC isogenes in wheat.
Construction and Analyses of Phylogenetic Trees
Phosphoenolpyruvate carboxylase protein sequences of the grass species with Oryza sativa cultivar Nipponbare were aligned by MUSCLE (Edgar, 2004) implemented in MEGA 7 with gap open penalties of −2.9, hydrophobicity multiplier of 1.2, eight-time iterations of the UPGMA method (Tamura et al., 2013). The aligned sequences were used for reconstructing a phylogenetic tree by the maximum likelihood (ML) method with “WAG with Freqs. (+F) model” and the conditions of partial deletion of gaps at 95%. We added several PEPC sequences of non-plant species, including algae (Chlamydomonas reinhardtii, Ostreococcus tauri, and Anabaena sp.), bacterial species (Synechocystis sp., Marchantia polymorpha, and Escherichia coli), and an Archaea species, Ferroglobus placidus to be out grouped. Five hundred bootstrap trials tested the reliability of branches in the tree.
Using TreeSAAP version 3.2 (Woolley et al., 2003), potential positive selection sites were searched with the default setting parameters. Branch-level and branch-site level natural selection was detected using EasyCodeML version 1.0 (Gao et al., 2019) with the default condition using an ML phylogeny of plant-type PEPCs in A. tauschii, B. distachyon, O. nivara, O. sativa, O. rufipogon, S. italica, S. bicolor, Z. mays, and wheat.
Statistical Analysis
Student’s t-test was applied for numerical data by using Microsoft Excel. Statistical significance was determined with value of p < 0.05 or 0.01.
Results
Grass PEPCs Belong to Eight Molecular Groups
Our initial question was which molecular types of PEPCs are encoded in the wheat genome. The automated prediction allowed identifying 16 PEPC isogenes, comprising 14 plant-type PEPC and two bacterial-type PEPC: these were categorized into six isogene types: Tappc1a, Tappc1b, Tappc2, Tappc3, Tappc4, and Tappc-b (Table 1). These PEPCs were distributed in 11 wheat chromosomes: 3A, 3B, 3D, 5A, 5B, 5D, 6A, 6B, 6D, 7A, and 7D. Except for the bacterial-type PEPC Tappc-b and one of the plant-type PEPC isogene groups Tappc1b, of which orthologue in rice was not found, PEPC isogenes were conserved across the A, B, and D genomes. We found additional PEPC isogenes Tappc1bB on 7B chromosome and Tappc-bA on 3A chromosome by TBLASTN searches, although the predicted protein sequences were not assigned to PEPC with our above-mentioning criteria. The presence of Tappc1b in the A, B, and D genomes were validated by RT-PCR using gene-specific UTR primers. The amplified cDNA fragments were cloned to be sequenced by primer walking. The transcribed sequences were perfectly matched with the reference genome sequences with confirmation of the exon-intron structure of Tappc1b in the GT-AG rule. Prokaryotic expression of a Tappc1b N-terminal polypeptide indicated that Tappc1b isogenes encode PEPC proteins (Supplementary Figure S2).
To be consistent with our knowledge, we found typical conserved PEPC motifs such as the phosphorylation site of plant-type PEPC, catalytic bases, glucose-6-phosphate binding site, hydrophobic pockets, PEP binding site, tetramer formation, Mg2+ binding site, HCO3− binding site, and PEP and Asp binding site (Supplementary Figure S3). Tappc4 in A, B, and D genomes contained plastid transit peptides on the N termini, found in the rice ortholog Osppc4 (Masumoto et al., 2010). Although the total branch lengths of plant-type PEPCs exhibited no obvious difference, it is clear that ppc2 and ppc3 are likely to be more evolved from the common ancestor of the PEPCs analyzed (Supplementary Figure S4).
To assess the evolutionary relationship of the wheat PEPC isogenes and other grass PEPCs, we performed a phylogenetic analysis of PEPC protein sequences of 28 monocot species. The phylogenetic tree with the maximum likelihood (ML) method dissected the PEPC groups in grass species (Figure 2); in addition to C4-photosynthetic PEPC group “ppcC4,” seven lineages (groups) of “ppc-b,” “ppc4,” “ppc1a,” “ppc1b,” “ppc3,” “ppc2a,” and “ppc2b” were designated. The single lineage ppc-b is for the bacterial-type PEPC. The chloroplast-type PEPC group ppc4 is located in the first branch among plant-type PEPCs. The second branch among plant-type PEPCs comprised ppc1a, ppc1b, and ppcC4, which must have a common ancestral PEPC. The ppc1b group exhibited faster evolving than ppc1a after diversifying these two close lineages. Notably, ppc1b was possibly the origin of ppcC4, of which evolutionary speed seemed accelerated after the diversification from ppc1b. The same phylogenic relationship of ppc1b and ppcC4 was observed by the ML method with an alternative condition and neighbor-joining phylogenetic trees under eight different parameter settings (Supplementary Figure S5). The third branch among plant-type PEPCs is ppc3, which showed evolutionary conservation between wheat and Oryza species. The last branch is ppc2, which comprises ppc2a and ppc2b, where different gene duplication between Pooideae and Oryza species was observed; ppc2a was uniquely present in the Oryza genomes.
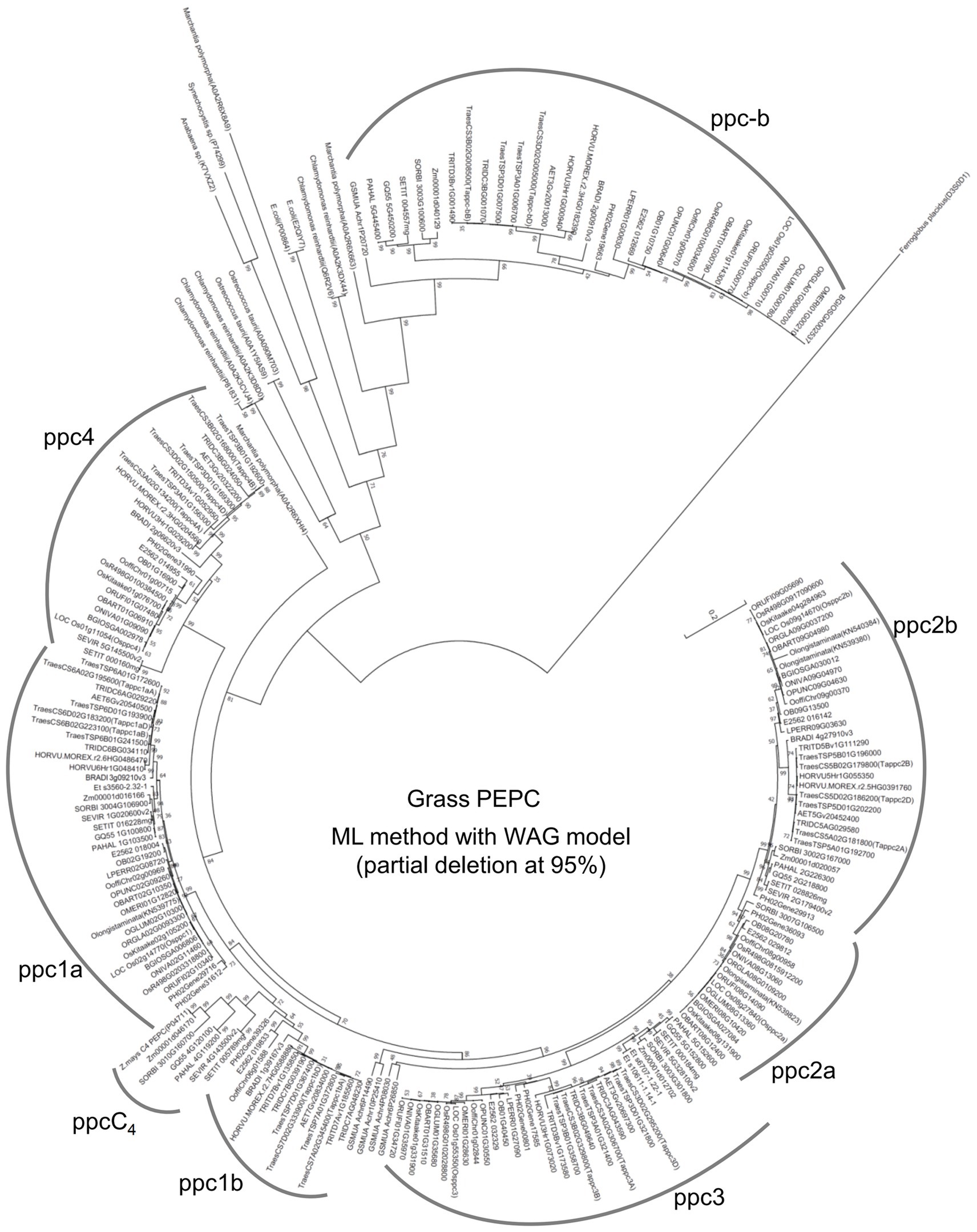
Figure 2. An maximum likelihood (ML) phylogenetic tree dissects grass PEPC proteins. Out-grouped PEPCs were shown as follows; Chlamydomonas reinhardtii (P81831, A0A2K3CVJ4, A0A2K3D8D0, Q6R2V6, and A0A2K3DX44), Ostreococcus tauri (A0A1Y5IAS9, A0A090M703), Anabaena sp. (K7VXZ2), Synechocystis sp. (P74299), Marchantia polymorpha (A0A2R6X663), Escherichia coli (P00864, E2QIY7), and Ferroglobus placidus (D3S0D1). The log likelihood for this tree was “−37030.92.”
Genomic Evidence for the Evolutionary Retention and Changes of Grass PEPCs
To seek genome-level evidence for the molecular evolution of grass PEPCs, we referred to the structural genome evolution model of Salse et al. (2008). It allowed inference of all the evolutionary origins and processes of PEPCs in rice, a wild rice O. officinalis, wheat, sorghum, and maize (Figure 3). Originally there were five ancestral genome blocks A4, A5, A7, A8, and A11, and A4 encodes ppc1, A5 encodes ppc3, ppc4, and ppc-b, and A8 encodes ppc2. Theoretically, the whole-genome duplication at around ~90 million years ago (MYA) increased the copies of each PEPC isogene group, resulting in a paralogous pair of ppc1 (ppc1a and ppc1b on A4 and A6, respectively) and ppc2 (ppc2a and ppc2b on A8 and A9, respectively). Following chromosomal breakages and fusions established a set of 12 ancestral grass chromosomes: A1–A12, among which A1 retains ppc3, ppc4, and ppc-b, A8 and A9 retain ppc2a and ppc2b, respectively, and A2 and A6 retain ppc1a and ppc1b, respectively. In this step, we assumed that the duplicated isogenes for ppc3, ppc4, and ppc-b were lost due to the redundancy. The 12 ancestral grass chromosomes with the chromosomal localizations of these PEPC isogenes are identical to O. officinalis. During the evolution of Oryza species, ppc1b was lost, and the PEPC gene composition of the cultivated rice was established; it was supported by the formation of ppc1b pseudogene on the corresponding locus in the BB genome-type wild rice O. punctata (Supplementary Figure S6). All the PEPC isogenes on the ancestral grass chromosome were inherited into the wheat A, B, and D genomes after multiple chromosomal recombination events. The genomic localization of these PEPC isogenes was typical among the wheat species (Supplementary Figure S7). These PEPC isogenes were retained on the genome of the Pooid grass model B. distachyon on the expected chromosomal segments even though its genome was nested (The International Brachypodium Initiative, 2010; Supplementary Figure S8). Regarding the C4-photosynthetic grass species sorghum and maize, the PEPC isogene composition of the common ancestral chromosomes might differ from the C3-photosynthetic grass species; namely, ppc4 was absent from A1, A4 maintains a copy of ppc1a or a pseudogene of ppc1a, and ppc1b on A6 evolved into ppcC4. These differences may have occurred in the recent 45–60 million years.
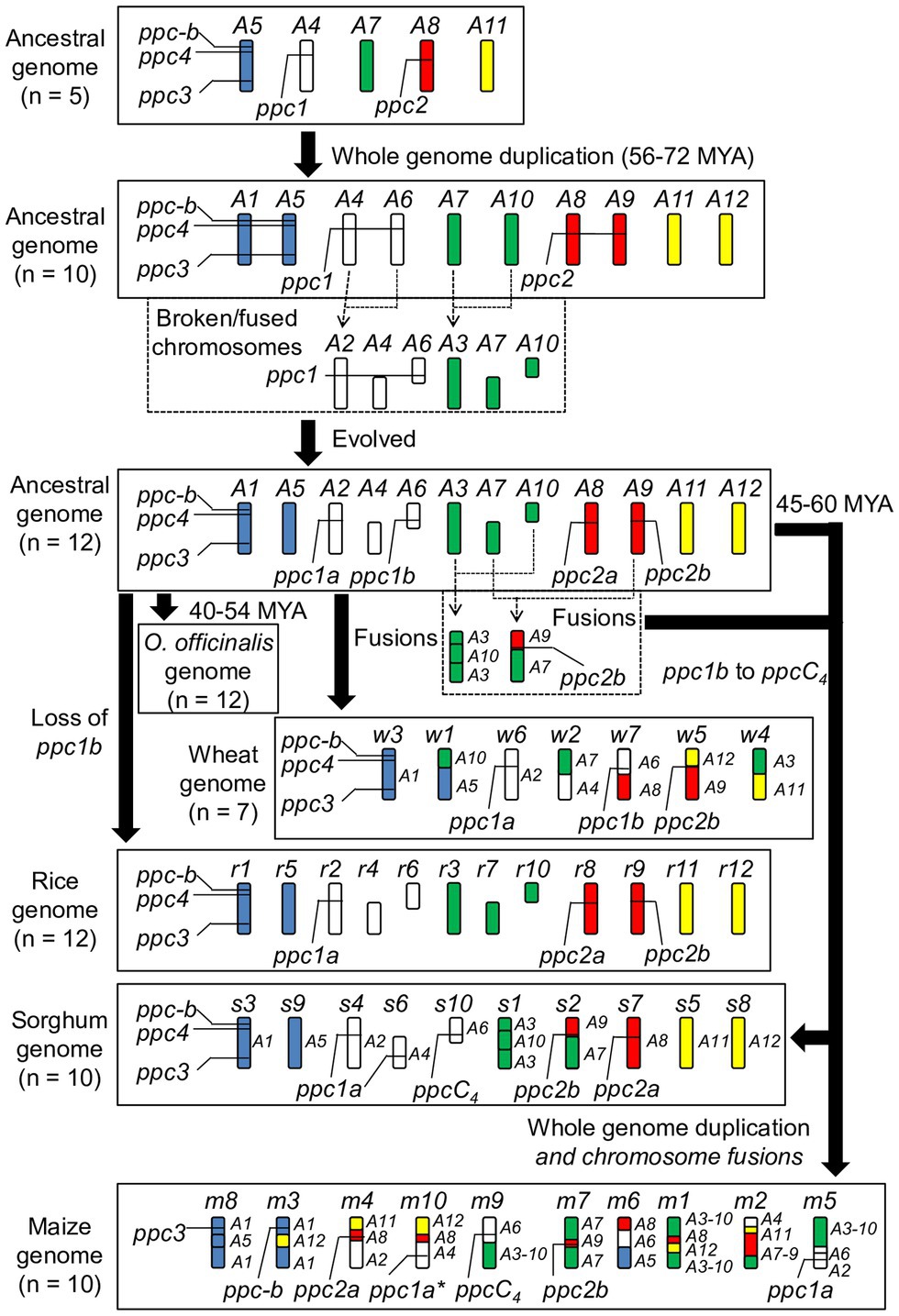
Figure 3. Inferred molecular inheritance of PEPC isogenes in the ancestral grass genome model. The molecular clock information was referred from a study by The International Brachypodium Initiative (2010). The present status of the rice genome, the O. officinalis genome, the wheat genome, the sorghum genome, and the maize genome were also shown. The asterisk represents “pseudogene.”
We noted that an alternative wild rice species O. meyeriana maintains ppc1b. To check the molecular evolution of ppc1b, we searched it for 15 Oryza species and the outgroup L. perrieri at the genome level using TBLASTN. It appeared that ppc1b sequence was absent from the genome assemblies of all the AA genome species analyzed (Supplementary Figure S9). The genomes having ppc1b were CC-type, GG-type, and KKLL-type ones. These results indicate that ppc1b was maintained until the recent evolution of Oryza genomes but lost in multiple sub-lineages independently. According to the example in O. punctata (Supplementary Figure S6), gene loss of ppc1b is likely to occur not due to chromosomal-level events but to locus-level events. We also searched ppc1b from other related species in Panicoideae to observe the absence of ppc1b (Supplementary Table S5).
To evaluate the phylogenetic relationship and molecular evolutionary status of ppc1b and ppcC4, we constructed a phylogenetic tree using additional genome data sets (Supplementary Table S5). Same with the phylogeny mentioned above, it represented that ppcC4 formed only one lineage and looked derived from the common ancestor with ppc1b (Figure 4). Here, we found additional evidence that ppc1b is the molecular origin of ppcC4. Namely, the C4 cereal species Eleusine coracana (finger millet), which is considered to be abiotic stress tolerant (Gupta et al., 2017), has both ppc1b and ppcC4 on the two types of allotetraploid genomes. Notably, the ppc1b in E. coracana is located next to the ppcC4 isogene on each type of sub-genome. In addition, the A-genome donor E. indica maintained both ppcC4 and ppc1b in the corresponding locus. These results indicate that these two PEPC isogenes were duplicated in the ancestral locus of a C3-photosynthetic species, and either of the gene copies evolved into ppcC4 (Figure 5). More importantly, we observed three types of recent duplication of ppcC4 in panicoid C4 grasses. Gene duplication of ppcC4 by multiplication looked occurred in Panicum virgatum and Echinochloa crus-galli. Gene duplication of ppcC4 at the locus level was seen in Paspalum vaginatum and Urochloa fusca. Miscanthus sinensis caused recent duplication of ppcC4 in a different chromosome. These recent duplications of ppcC4 suggest the physiological benefits of C4-photosynthetic PEPC.
Predicted Origins of the Ancient Grass PEPCs
The origins of the ancient grass PEPCs were predicted based on a phylogenetic tree of monocot PEPCs (Figure 6). The ppc-b group in non-grass monocot species formed one lineage with grass species. Hence ppc-b in grass species would have come from the common ancestral monocot species. The ppc1 group and ppc4 group seemed to originate from a common ancestral lineage, which corresponded to a cytosolic Acorus americanus PEPC (Aco010025), implying that the chloroplast-targeting of ppc4 was given after the duplication of ppc1 and ppc4. Since Joinvillea ascendens has the orthologues of ppc1 (Joasc.07G069900) and ppc4 (Joasc.06G112900), these two PEPC lineages were formed in the early stage of grass evolution perhaps. The ppc3 group formed one lineage, suggesting that ppc3 originated from one ancestral lineage corresponding to A. americanus PEPC (Aco018093). The origin of the ppc2a and ppc2b group was unclear because no orthologue in non-grass monocot species was found in our analysis. The marine monocot species Zostera marina has three PEPC isoforms (Zosma04g04800, Zosma05g25910, and Zosma06g00180), which are located on the upstream branch of the five plant-type PEPCs in land monocot plants, implying that the formation of plant-type grass PEPCs was dependent on the atmospheric environment during evolution.
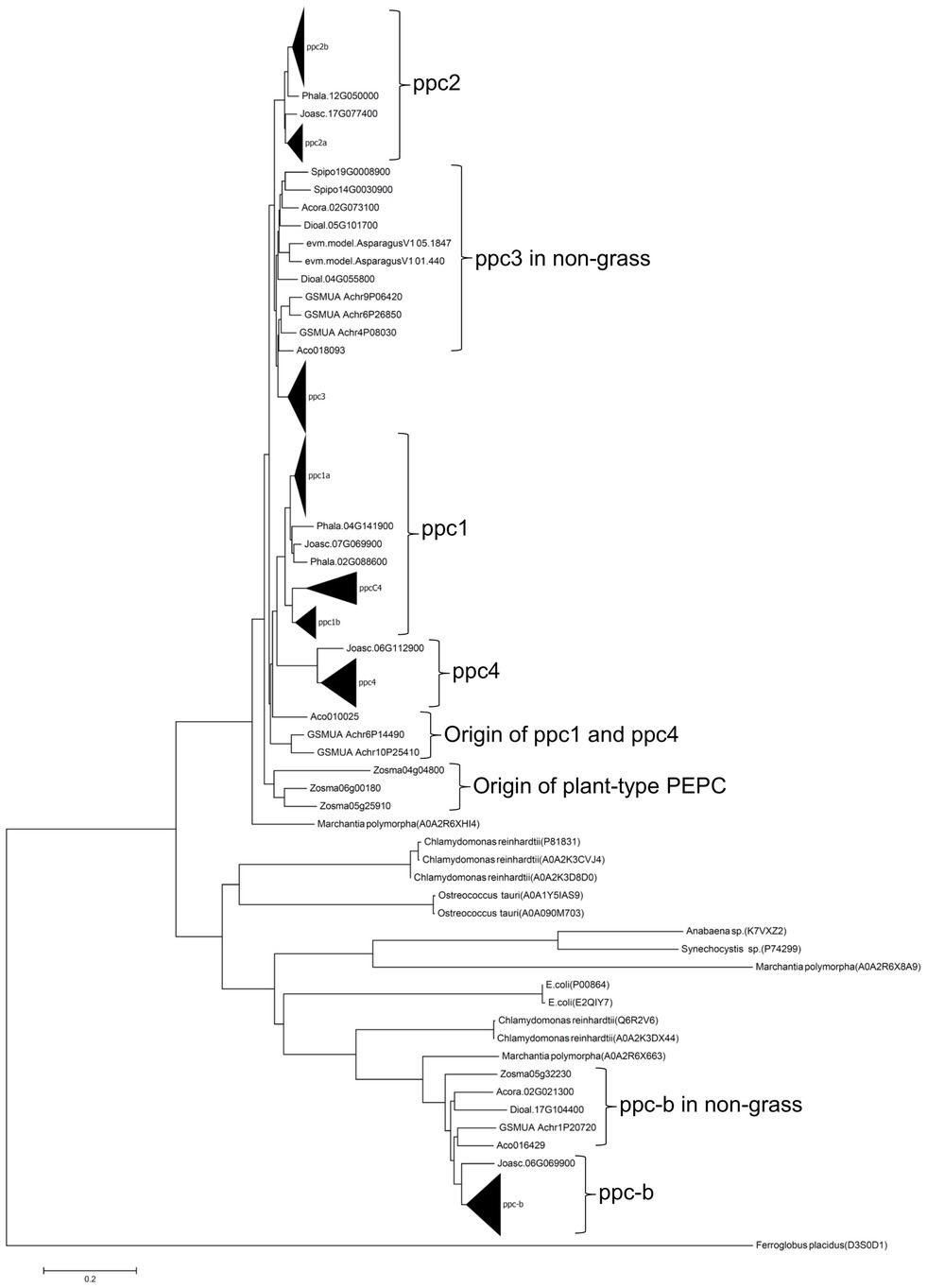
Figure 6. A NJ phylogenetic tree with JTT model for monocot PEPCs. Key PEPC protein identifiers are follows: Joasc.17G077400, Joasc.09G001100, Joasc.06G112900, and Joasc.07G069900 (Joinvillea ascendens), Phala.12G050000, Phala.04G0141900, and Phala.02G088600 (Pharus latifolius), Spipo19G0008900 and Spipo14G0030900 (Spirodela polyrhiza), Acora.02G073100 and Acora.02G021300 (Acorus americanus), Aco018093, Aco010025, and Aco016429 (Ananas comosus), Dioal.05G101700, Dioal.04G055800, and Dioal.17G104400 (Dioscorea alata), evm.model.AsparagusV1 05.1847 and evm.model.AsparagusV1 01.440 (Asparagus officinalis), GSMUA Achr9P06420, GSMUA Achr6P26850, GSMUA Achr4P08030, GSMUA Achr6P14490, GSMUA Achr10P25410, and GSMUA Achr1P20720 (Musa acuminata), Zosma04g04800, Zosma06g00180, Zosma05g25910, and Zosma05g32230 (Zostera marina). Black triangles represent sub-lineages of PEPC types. The bar shows the branch length of 0.2.
Structural Differences of Grass PEPC Groups
Fixed amino acid substitutions would play essential roles in the biochemical properties of PEPCs. Muramatsu et al. (2015) reported that biochemical properties of plant-type PEPCs in rice differed from each other. We searched conserved amino acid sequences in each PEPC group but differed from any other PEPC group across the grass PEPCs. A total of 45 fixed amino acid substitutions were found in either ppc4, ppcC4, or ppc-b (Supplementary Figure S10). The bacterial PEPCs have 41 distinct amino acid positions, and ppc4 and ppcC4 have three and two unique amino acid substitutions, respectively. The unique substitutions for ppc4 are R122K, E185Q, and Q222L, whose biochemical functions are unknown (Supplementary Figure S11). The distinct amino acid substitutions for ppcC4 were A531P and A780S (Supplementary Figure S11). The former amino acid substitution was reported as a positive selection site in C4-photosynthetic PEPCs (Christin et al., 2007), and the latter amino acid substitution in ppcC4 is well-known as the leading cause of the advanced biochemical property of C4-photosynthetic PEPCs (Bläsing et al., 2000). For other plant-type PEPCs, we found no unique amino acid substitutions for each group. These results implicate that unfixed amino acid substitution sites in particular PEPC groups involve the diverged biochemical properties of non-photosynthetic plant-type PEPCs. We could find 34 fixed substitutions between ppc2b and ppc4. Regarding ppc1a and ppc2b, 18 fixed substitution sites were found. Seven amino acid substitutions (V42L, S100K, G155K, Q364K, R495N, M717L, and S753L) largely dissected two evolutionary lineages between ppc4/ppc1a/ppc1b and ppc3/ppc2a/ppc2b (Supplementary Figure S11). S100K, Q364K, and R495N, which need more than one nucleotide substitution and bring changes of amino acid characteristics, may be a hint of the evolution of wheat PEPCs in biochemical properties. The functionality of S100K in allosteric regulation of maize C4-photosynthetic PEPC was reported by González-Segura et al. (2018), while other determinants of the C4-photosynthetic PEPC characteristics in the range of the amino acids 296–437, which corresponds to 302–443 in maize C4-photosynthetic PEPC (Engelmann et al., 2002) remain to be unknown. The substitution Q364K, located on the plant-specific sequence, is a potential candidate site.
Positive Selection of ppc1b
The accelerated molecular evolution of ppc1b after diversifying from the ppc1a lineage implicates the occurrence of natural selection. We could mine 11 potential positive selection sites in plant-type wheat PEPCs (Supplementary Table S6). These substitutions were associated with seven classes of physicochemical amino acid properties such as “Polarity” (p), “Equilibrium constant (ionization of COOH)” (pK’), and “Power to be at the C-terminal, α-helix” (αc), used by the tool TreeSAAP. Then, using the methods implemented in CodeML, we detected positive selection in the ppc1b branch after divergence from ppcC4 (Figure 7A). Branch-site model search revealed one positive selection site (Glu480Ser) for the ppc1b branch with statistical significance (5% level; Figure 7B). This substitution corresponded to the physicochemical property of αc, implicating biochemical relevance. Branch-site model search for ppcC4 detected three positive selection sites (A243Y), (R560P), and (H679F) after the divergence from the ppc1b lineage with statistical significance, being consistent with our knowledge that the present forms of C4-photosynthetic PEPC were made by adaptive evolution. Two of these sites (A243Y) and (H679F) were not found by Christin et al. (2007).
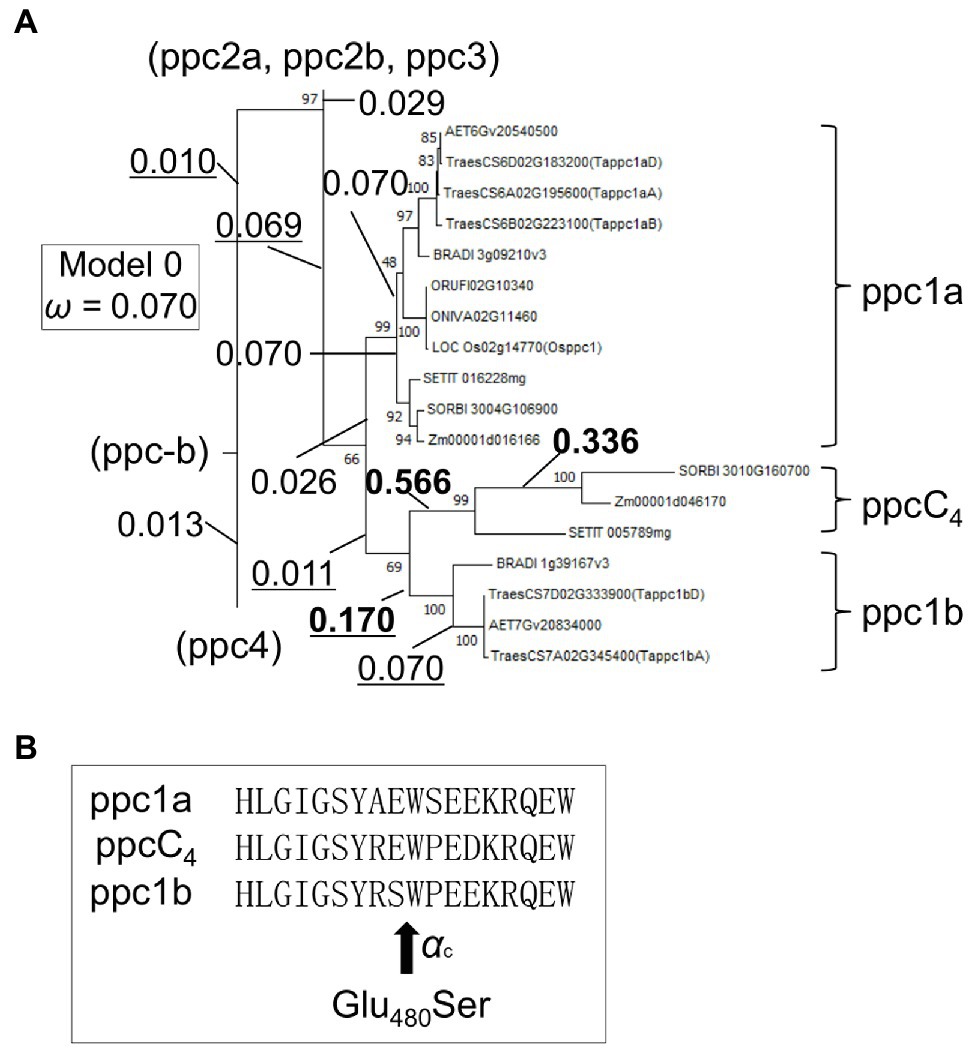
Figure 7. Detected positive selection in the ppc1b and ppcC4 lineages. (A) A partial phylogenetic tree showing ω values of branches. The ω values of the ppc1b lineage were underlined. Bold ω values represent statistical significance. (B) The positive selection site in the ppc1b branch. The amino acid positions are maize C4 PEPC coordinate’s. αc represents the physicochemical amino acid property related to the substitution.
Gene Expression Characteristics of PEPC Isogenes in Wheat and Other Grass Models
The expression levels for the wheat PEPC isogenes in public RNA-Seq profiles showed divergent spatial expression patterns (Supplementary Figures S12, S13). Tappc1a isogenes exhibited preferential expressions in roots and reproductive organs. Tappc1b isogenes represented high-level expressions in the leaf sample at a seedling stage. Tappc2 isogenes showed ubiquitous expression patterns, suggesting their functions are housekeeping. Tappc3 isogenes exhibited preferential expressions in reproductive organs, including ovaries. Tappc4 showed preferential expressions in leaf samples. Gene expressions of Tappc-b isogenes were undetectable in many samples, while low-level expressions were observed in reproductive organs such as anther, ovary, and spike. Overall, the orthologous PEPC isogenes were generally expressed in the same manner (Supplementary Figures S12, S13), suggesting that the gene regulation of the orthologous PEPC isogenes in the three types of genomes were conserved among each other.
By compilation of public RNA sequencing profiles under three types of abiotic stress and nitrogen stress, we observed varied responses of wheat PEPC isogenes. Under drought stress conditions, Tappc1b and Tappc4 isogenes showed upregulation in flag leaves, while Tappc2 isogenes showed down-regulation (Figure 8; Supplementary Table S7). Other PEPC isogenes showed no noticeable transcriptional changes under the conditions. Under a salt stress condition, Tappc1a isogenes and Tappc1b isogenes exhibited upregulation in roots but downregulation in leaves. The salt stress decreased the gene expression levels of Tappc2 isogenes and Tappc4 isogenes. These results suggest that Tappc1b isogenes is responsible for osmotic stress adaptation. By contrast, under a heat stress condition, transcriptions of Tappc1a, Tappc1b, Tappc2, and Tappc4 isogenes were downregulated. Since the gene expression levels of Tappc3 and Tappc-b isogenes were low, we could not monitor the effects of the abiotic stress. Regarding nitrogen stress, we observed that nitrogen availability differently affected gene expression levels of PEPC isogenes in wheat organs (Supplementary Tables S8, S9). Gene expression levels of Tappc1b isogenes showed apparent increases in shoots under sufficient nitrogen conditions while decreases in roots. These results indicate that Tappc1b is under organ type-dependent different gene regulations.
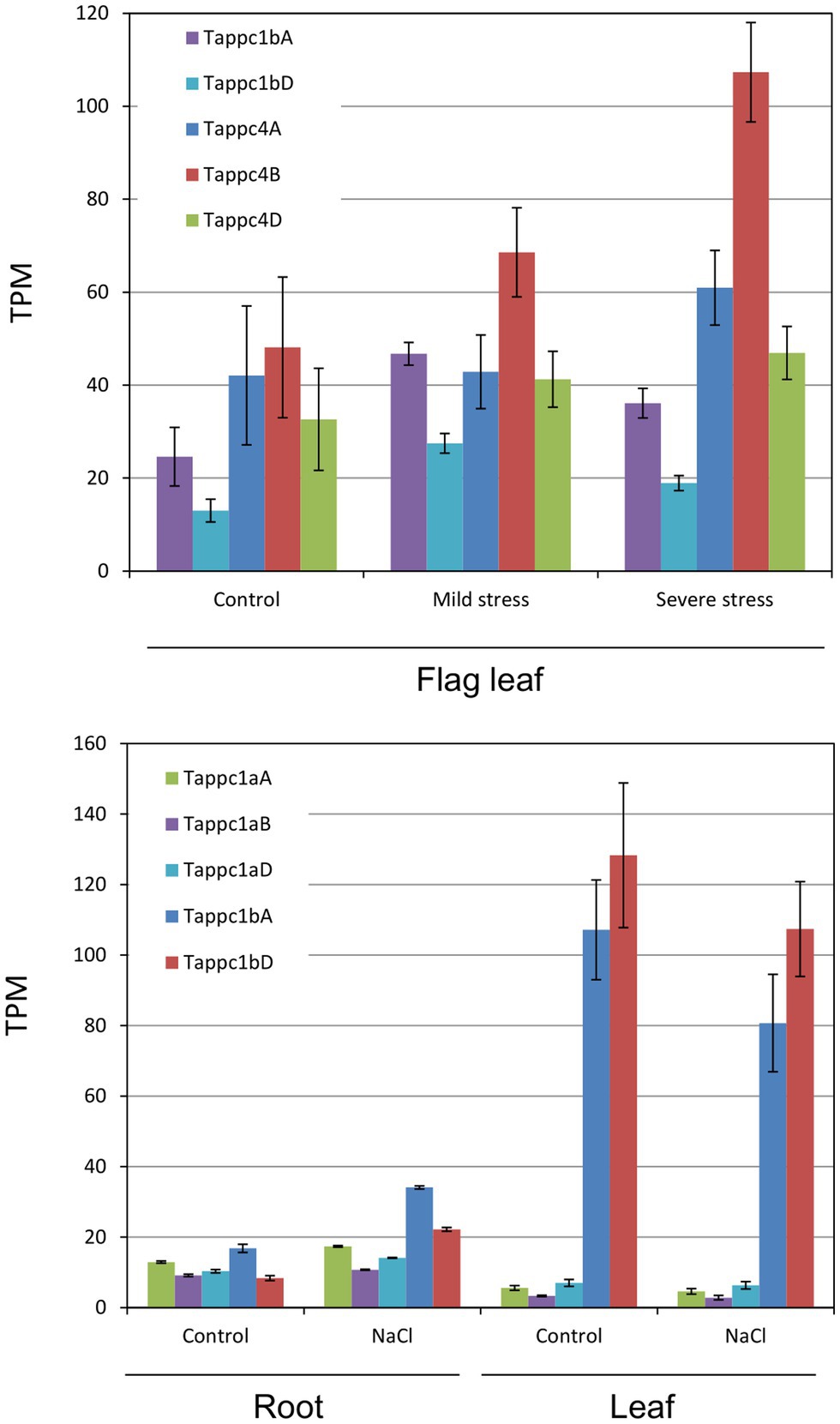
Figure 8. Abiotic stress responses of Tappc1a, Tappc1b, and Tappc4 isogenes. Asterisks represent statistical significance at 5% level in Student’s t-test. (A) Drought stress responses in flag leaves, (B) salt stress (100 mM NaCl) responses in roots and leaves.
To know details of the transcriptional regulation of PEPC isogenes, public RNA-Seq profiles in other grass model plants were analyzed. Brachypodium data indicated light-dependent and salt stress-induced gene regulation of ppc1b (Supplementary Tables S10, S11). Barley data in the aerial part represented potential nitrate-dependent transcriptional enhancement of ppc1b dependent on nitrate supplement (Supplementary Table S12). RNA-Seq profiles in T. turgidum indicated selective transcriptional enhancement of ppc1b isogenes in leaf-stem samples and spikelets (Supplementary Table S13). For the C4-photosynthetic cereals, sorghum and maize, no clear expression changes of PEPC isogenes were observed under nitrogen stress conditions (Supplementary Tables S12–S15). The leaf RNA-Seq profiles in E. coracana, of which genome maintains ppcC4 and ppc1b both, represented distinct differences at the gene expression level between ppcC4 and ppc1b (Supplementary Table S16), confirming their molecular types and physiological roles are different. Overall, the gene expression patterns of ppc1b differed from other types of PEPC isogenes.
Selective Transcriptional Regulation of Tappc1b, Tappc2, and Tappc4 in Response to NO3−
To identify the biologically significant response of PEPC to nitrogen supply, we prepared 2-week old wheat seedlings under a nitrogen-deficient condition and supplied 40 mM NO3− to detached leaves from these plants. As expected, the supplement of NO3− increased PEPC activity according to the incubation time. PEPC activity showed significant upregulation at 24 h after incubation compared to the mock condition (at 5% level in Student’s t-test; Figures 9A,B). The patterns of PEPC activity were very similar in two ways of measurements: fresh weight-basis and soluble protein amount-basis. The increases of PEPC activity after the treatment in control and NO3−-treated samples were observed due to higher strength of light provision after the detachment physical stress of leaf detachment. Thus, the up-regulation of PEPC activity in leaves under the supplement of NO3− was not acute but not long-periodical in our condition. The concomitant increase of PEPC proteins at 24 h after the NO3− treatment was confirmed by Western blotting (Supplementary Figure S14).
To check the transcriptional status of all the PEPC isogenic groups, we conducted qRT-PCR using a consensus primer set for each isogene group. The results indicated up-regulation of Tappc1b, Tappc2, and Tappc4 groups by NO3− (Figures 8C–G). Gene expression of the Tappc-b group was undetectable in our experimental condition. To verify NO3− response at isogene level, we performed additional qRT-PCR assays using gene-specific primers designed on 3′UTR regions for Tappc1b, Tappc2, and Tappc4 groups (Figures 8H–N). The most obvious response was observed for Tappc1b at 6 h after incubation, but significant upregulation at 3, 12, and 24 h after incubation was also observed. Tappc4 exhibited upregulation with a lesser fold change than that of Tappc1b. In addition, Tappc2 exhibited upregulation clearly after 24 h of induction, indicating the presence of an alternative mechanism of transcriptional upregulation of PEPC. The downregulation of Tappc4 in the mock condition might be due to the effect of SO42− (Supplementary Figure S15).
Candidate cis-Motifs for the Transcriptional Regulation of Tappc1b and Tappc4 by NO3−
The NO3− responses of Tappc1b and Tappc4 in the exact timing imply that the same regulatory mechanism regulates these two PEPCs. To approach the regulatory mechanism of the transcriptional upregulation of Tappc1b and Tappc4, we conducted comparative motif searches in the promoter regions of these wheat PEPC isogenes and the orthologous isogenes with Tappc1b, including ppcC4. We could predict a cis-motif (GCCTTTCCAACCGCCAAGRG), which are from Tappc1b and ppcC4 (Figure 10). Notably, the motif contained a similar sequence with the transcription factor Dof1 binding core motif “AAAGG.” This result was consistent with that Dof1 possibly regulates plant PEPCs (Yanagisawa, 2000). In addition, it contained a Dof1 core region bound to Dof1 protein in a gel-shift assay (Yanagisawa and Sheen, 1998), suggesting Dof1-mediated transcriptional regulation of wheat PEPC isogenes. In addition, seven cis-motif candidates were predicted (Supplementary Figure S16). The motif (SGGCTGKGGCCWGTGVTGRGSG) includes a candidate nitrate-response element found by Pathak et al. (2009).
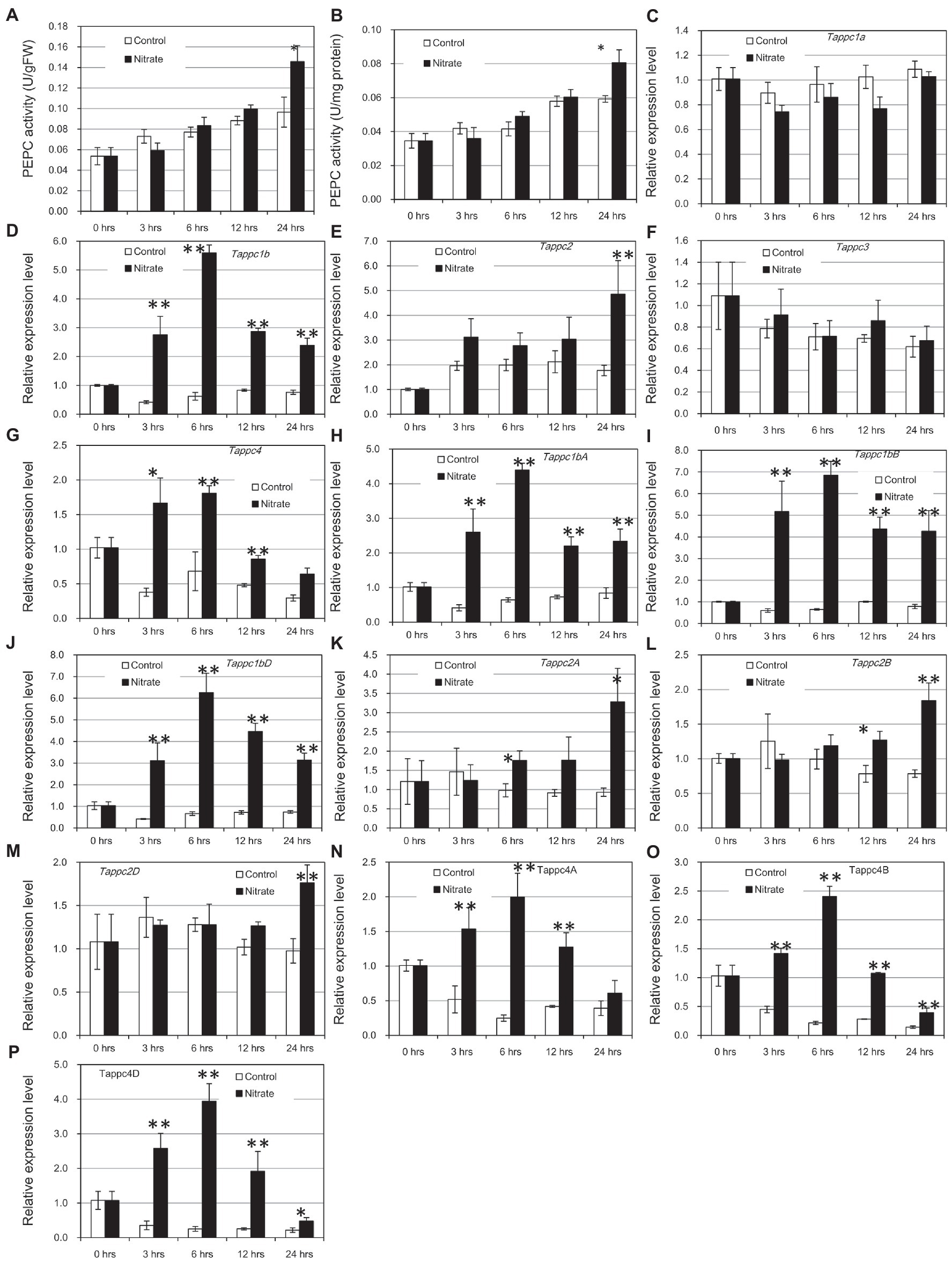
Figure 9. Enzyme activity and gene expressions of PEPCs in response to nitrate. Error bars indicate SEs. Double and single asterisks indicate statistical significance at 5 and 10% level, respectively. (A) PEPC activity per g fresh weight, (B) PEPC activity per mg protein, (C–G) Relative expression levels of wheat PEPC isogene groups, and (H–P) Relative expression levels of Tappc1b, Tappc2, and Tappc4 isogenes in the A, B, and D genomes.
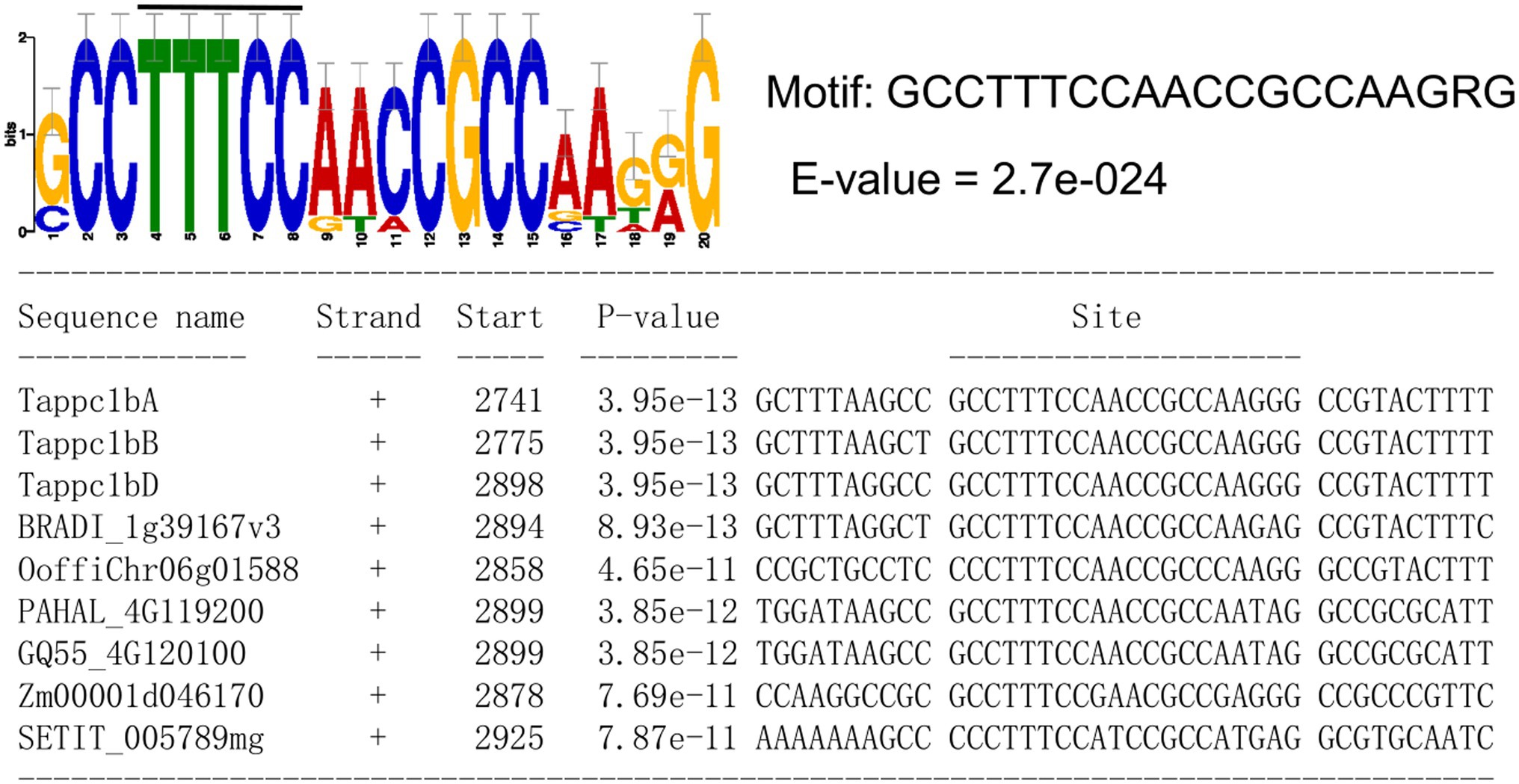
Figure 10. A predicted cis-motif associated with the nitrate responses of Tappc1b isogenes. The black bar above the motif logo represents the position of Dof1 binding core motif.
Discussion
To investigate the evolutionary processes of grass PEPCs, we analyzed PEPC isogene compositions in several grass species, including wheat, rice, maize, and sorghum. The plant-type PEPCs in grass species seem to be originated from one ancient lineage and formed five ancestral PEPCs, followed by dynamic molecular evolution with the whole-genome duplication in the early period of grass species divergence (around ~90 MYA) Huang et al. (2022). We discovered a new non-photosynthetic PEPC group ppc1b retained in particular grass genomes. It is likely to be the primary molecular origin of grass C4-photosynthetic PEPCs. The explicit designation of ppc1b allowed a clear illustration of the evolutionary processes of photosynthetic and non-photosynthetic PEPCs during evolution. Consistent with the phylogenetic analysis result using partial PEPC sequences by Christin et al. (2007), C3-photosynthetic Pooideae, including Triticum species, retain ppc1b. We detected ppc1b sequences in the genome assemblies of non-Triticum Pooids Avena eriantha, Dactylis glomerata, Lolium multiflorium, L. perenne, and Puccinellia tenuiflora in addition to B. distachyon (data not shown). By contrast, many species in the Oryza lineage have lost ppc1b during evolution. These facts support that PEPC is highly associated with grass species diversity.
Characterization of ppc1b revealed its molecular plasticity and similarity with C4-photosynthetic PEPCs. Namely, ppc1b was subjected to adaptive evolution after the divergence from ppcC4 and exhibited abiotic stress-relation in wheat and B. distachyon (Supplementary Figure S17). In addition, ppc1b in wheat maintains the regulatory characteristics of maize ppcC4: nitrogen-dependent transcription in photosynthetic organs (Sugiharto and Sugiyama, 1992; Suzuki et al., 1994) and abiotic stress responses (Supplementary Figures S17, S18). Our results indicated that wheat ppc1b is potentially regulated by Dof1, a nitrogen metabolism-associated transcription factor for ppcC4 in maize. Meanwhile, the protein sequences of Tappc1b isoforms are likely to be of plant-type PEPC in C3-photosynthetic plants (Supplementary Figure S5). We suppose that ppc1b and ppcC4 are representative genes that evolved with the functional diversification of grass species.
In the evolutionary story of grass C4-type PEPCs by Christin and Besnard (2009), the divergence of C4- photosynthetic PEPCs and non-photosynthetic PEPCs occurred before the divergence of C4 and C3 plants. Christin et al. (2007) indicated that C4- photosynthetic and non-photosynthetic PEPCs are distinguished based on the conserved motifs specific in C4-photosynthetic PEPC isoforms of a few distant polypeptide sites. Meanwhile, C4 plants had occurred in several evolutionary branches of monocot species. Hence, this story is hardly understood with ease unless convergent molecular evolution of PEPC or lateral transfer of C4-photosynthetic PEPC is accepted, even though their analysis suggests the PEPC class ppc-B2 is highly associated with the C4-photosynthetic PEPCs in grass species. Our integrative approach using grass genome data clarified that all the C4-photosynthetic PEPCs analyzed were derived from the common ancestor with ppc1b. The unique C4 grass species E. coracana, which has ppc1b and ppcC4 on the two types of allotetraploid genomes, represented the direct link between ppc1 and ppcC4. According to Christin et al. (2007), there seem to be several Chloridoideae species that retain ppc1b and ppcC4. Further genome-level analyses should reveal other evidence for the origin of C4-photosynthetic PEPCs.
One of the most crucial functions of plant PEPCs is an adaptation to the environment. C4-photosynthetic PEPCs were probably born to adapt to high-temperature conditions. Plant cells require substantial energy and biosynthesis of osmolytes such as proline (Pan et al., 2021). However, this stress represses photosynthetic activities, resulting in high energy demand. Here, PEPC could act on re-fixing carbon released by respiration to complement the limited carbon source. This fact might be one of the reasons why C4-photosynthetic species generally exhibit high abiotic stress tolerance (Pardo and VanBuren, 2021). Hence, we hypothesize that ppc1b and ppcC4 have evolved under substantial environmental stress. Retention of ppc1b in wheat species suggests its physiological benefits under osmotic stress. Typically, the Triticum-Aegilops complex is capable of growing well under semi-arid conditions. By contrast, cultivated rice prefers more wet conditions. For example, one of the Oryza species retaining ppc1b, O. coarctata, exhibits high salt tolerance (Chowrasia et al., 2018), while cultivated rice is well-known for its less tolerance to salt stress.
Gene loss of ppc1b during the evolution of Oryza species implicates the functional neutrality of ppc1b for these Oryza species. Otherwise, ppc1b was lost in cultivated rice due to the functional redundancy with other PEPC isogene. The former possibility can be explained by an ecologically different background between the Oryza species and Pooideae. Pooid cereals typically exhibit superior abiotic stress resistance, especially salt and drought stress. The latter possibility can be explained by an additional copy of ppc2 (ppc2a) in the Oryza species genomes. We observed selective drought stress response of ppc2b in rice public microarray data (Supplementary Figure S19). The presence of ppc2a in the Oryza genomes might have allowed the evolutionary adaptation of ppc2b to cope with the lack of ppc1b.
Response to nitrogen is one of the primary behaviors of plant PEPCs (Sugiharto et al., 1990; Zhang et al., 2012; Yamamoto et al., 2014b, 2017). Two proposed mechanisms explain the increased PEPC activity by nitrogen supply: transcriptional level and post-translational level regulations (Sugiharto et al., 1990; Sugiharto and Sugiyama, 1992; Wu and Wedding, 1992). Duff and Chollet (1995) applied a test system where the basal condition has a low concentration of NO3− and observed post-translational regulation of PEPC by in vivo phosphorylation. To identify NO3−-dependent response, we employed a detached leaf test system, where a high concentration of NO3− is supplied to a nitrogen-deficient condition. Since NO3− could be a signal of gene transcription (Sugiharto and Sugiyama, 1992; Dechorgnat et al., 2011; Medici and Krouk, 2014; Vidal et al., 2020), we assumed that our system would be more suitable than other systems applied in the previous studies. Under the limited availability of nitrogen, the gene expressions of Tappc1b and Tappc4 groups were maintained at a low level. Once NO3− was given, the expressions of these genes were induced quickly, i.e., within 3 h. We assume that the transcriptional induction of Tappc1b and Tappc4 orthologues in wheat would not be the indirect response to NO3−, while Tappc2 orthologues made a late transcriptional response in concordant with the increased PEPC activity. These three PEPC isogene groups could underlie the increased PEPC activity in the detached leaves, but the different expression manners might imply different roles in nitrate-related metabolism. We wonder if Tappc1b acts on balancing nitrogen-carbon metabolism in Pooideae.
Conclusion
We discovered a new PEPC isoform group ppc1b by the cross-species genome-wide analysis in Poaceae. PEPC isoforms belong to ppc1b would be the origin of grass C4-photosynthetic PEPCs. Those maintain similar characteristics to those of C4-photosynthetic PEPCs but are likely to play role in non-photosynthetic physiology, i.e., abiotic stress adaptation. Our results clearly indicated that C4-grass species genomes lack ppc1b due to the evolutionary change of ppc1b into C4-photosynthetic PEPC. The presence of ppc1b in the Eleucine genomes implicates tandem gene duplication of PEPC might affect physiological characters of cereal species. To see whether utilization of non-photosynthetic PEPC in plant biotechnology are useful for enhancing abiotic stress tolerance in cereal crops or not, experimental evaluation using transgenic techniques is required.
Data Availability Statement
The cDNA sequence data that we obtained in this study can be found in the Genbank online repositories. The accession numbers are ON055387, ON055388, and ON055389.
Author Contributions
NY conducted computations, assisted experiments, analyzed the data, and wrote the manuscript. BL and WT performed experiments to analyze experimental data. In addition, ZY assisted experiments and data analyses to improve the manuscript. ZP and ZY financially supported this study. All authors contributed to the article and approved the submitted version.
Funding
This work is supported by the National Natural Science Foundation of China [Grant No. 32060456], the Natural Science Foundation of Sichuan Province [Grant No. 2022NSFSC0160], and the Science Foundation of China West Normal University (No. 19E060).
Conflict of Interest
The authors declare that the research was conducted in the absence of any commercial or financial relationships that could be construed as a potential conflict of interest.
Publisher’s Note
All claims expressed in this article are solely those of the authors and do not necessarily represent those of their affiliated organizations, or those of the publisher, the editors and the reviewers. Any product that may be evaluated in this article, or claim that may be made by its manufacturer, is not guaranteed or endorsed by the publisher.
Acknowledgments
We thank Dr. Wu and Chen in the College of Life Science, China West Normal University, for assisting in cultivating wheat seedlings and the RNA-Seq data analysis, respectively.
Supplementary Material
The Supplementary Material for this article can be found online at: https://www.frontiersin.org/articles/10.3389/fpls.2022.905894/full#supplementary-material
Supplementary Figure S1 | Photographs of wheat seedlings used in the nitrate induction experiment. (A) Two-week old wheat seedlings grown in a laboratory. (B) Detached wheat leaves under incubation with K2SO4 solution and KNO3 solution at 0 h.
Supplementary Figure S2 | Prokaryotic expression of a Tappc1b fragment in E. coli. (A) A partial sequence alignment of Tappc1b in the wheat genome (100% identical among each other). The expressed polypeptide fragment was shown. One of the conserved domain, which is important for PEPC enzyme catalysis, was underlined. (B) PEPC activity in E. coli BL21 (DE3) harboring pET28a and pET28a with the Tappc1bD fragment insert. Overnight culture of transformed E. coli were inoculated onto fresh LB medium, and IPTG was added when OD600 reached onto 0.5. Then, the bacterial cultures were incubated at 25°C for 5 h. E. coli cells were harvested to extract soluble proteins, and PEPC activities were measured. Asterisks indicate statistical significance between “Tappc1bD IPTG” and other samples at 5% level.
Supplementary Figure S3 | A multiple sequence alignment of wheat PEPC proteins. Known functional motifs were marked.
Supplementary Figure S4 | Distributions of branch lengths for wheat PEPCs. Rice PEPCs were analyzed as references.
Supplementary Figure S5 | Phylogenetic trees using the ML and neighbor-joining method with different parameters. (A,B) The ML method, (C–J) the neighbor-joining method.
Supplementary Figure S6 | Comparison of the ppc1b locus between Oryza officinalis and Oryza punctata. (A) Genomic structure of the ppc1b locus in Oryza officinalis and O. punctata. Genomic positions of these species were shown above genes, and identifiers of corresponding genes in O. sativa were shown under genes. (B) TBLASTN search of Tappc1bD against the O. punctata genome. The gene loss region in O. punctata was highlighted.
Supplementary Figure S7 | Chromosomal localization of ppc1b on the wheat, and other related genomes.
Supplementary Figure S8 | Chromosomal localization of ppc1b on the Brachypodium genome.
Supplementary Figure S9 | An evolutionary chart of wild rice and cultivated rice represents the presence and absence of ppc1b.
Supplementary Figure S10 | Amino acid sequence variation sites that are fixed in each PEPC group. The positions were referred to the maize C4-photosynthetic PEPC.
Supplementary Figure S11 | List of amino acid substitution sites that are related to the molecular evolution of plant-type PEPC.
Supplementary Figure S12 | Spatial gene expression patterns of wheat PEPC isogenes. (A) A bar chat represents estimated gene expression levels in various organs. (B) PCA biplot showing the interrelationship of Tappc isogenes at gene expression patterns. (C) Variance of principal components.
Supplementary Figure S13 | A bar chart showing the compositions of quantitative gene expression levels of PEPC isogenes in wheat. The horizontal axis represents TPM values.
Supplementary Figure S14 | Western blot analysis of PEPC in wheat detached leaves. The sample lane 1: Before detachment, lane 2: 24 h with K2SO4, lane 3: 24 h with KNO3. Polypeptides representing nearby apparent size of PEPCs were designated as PEPC proteins. Signal intensities of PEPC proteins were calculated using ImageJ. The asterisk indicates statistical significance between “Mock” and “KNO3“samples at 10% level.
Supplementary Figure S15 | The effect of K2SO4 to gene expression of Tappc4 in wheat detached leaves. Error bars indicate standard errors. The asterisk represents statistical significance at 5% level in Student’s t-test.
Supplementary Figure S16 | Other potential cis-motif candidates that are associated with nitrate response.
Supplementary Figure S17 | Gene expression patterns of maize C4-photosynthetic PEPC under abiotic stress conditions. The data were retrieved from qTeller (Woodhouse et al., 2021).
Supplementary Figure S18 | Selective up-regulation of ppc1b in leaves of three-week-old B. distachyon seedlings under a salt stress condition by 200 mM NaCl for 24 h (GenBank SRA BioProject accession: PRJNA636626). Error bars indicate standard errors. The asterisk and double asterisk represent statistical significance at 10 and 5%, respectively.
Supplementary Figure S19 | Rice microarray data represents selective transcriptional response of Osppc2b under drought stress conditions. The data were retrieved from OryzaExpress (Hamada et al., 2011). Error bars indicate standard errors.
Footnotes
References
Bailey, T. L., Johnson, J., Grant, C. E., and Noble, W. S. (2015). The MEME suite. Nucleic Acids Res. 43, W39–W49. doi: 10.1093/nar/gkv416
Bläsing, O. E., Westhoff, P., and Svensson, P. (2000). Evolution of C4 phosphoenolpyruvate carboxylase in Flaveria, a conserved serine residue in the carboxyl-terminal part of the enzyme is a major determinant for C4-specific characteristics. J. Biol. Chem. 275, 27917–27923. doi: 10.1074/jbc.M909832199
Bolger, A. M., Lohse, M., and Usadel, B. (2014). Trimmomatic: a flexible trimmer for Illumina sequence data. Bioinformatics 30, 2114–2120. doi: 10.1093/bioinformatics/btu170
Bolser, D. M., Staines, D. M., Perry, E., and Kersey, P. J. (2017). Ensembl plants: integrating tools for visualizing, mining, and analyzing plant genomic data. Methods Mol. Biol. 1533, 1–31. doi: 10.1007/978-1-4939-6658-5_1
Caburatan, L., and Park, J. (2021). Differential expression, tissue-specific distribution, and posttranslational controls of phosphoenolpyruvate carboxylase. Plan. Theory 10:1887. doi: 10.3390/plants10091887
Chowrasia, S., Rawal, H. C., Mazumder, A., Gaikwad, K., Sharma, T. R., Singh, N. K., et al. (2018). “Oryza coarctata Roxb” in The Wild Oryza Genomes. eds. T. K. Mondal and R. J. Henry (Cham: Springer), 87–104.
Christin, P. A., and Besnard, G. (2009). Two independent C4 origins in Aristidoideae (Poaceae) revealed by the recruitment of distinct phosphoenolpyruvate carboxylase genes. Am. J. Bot. 96, 2234–2239. doi: 10.3732/ajb.0900111
Christin, P. A., Salamin, N., Savolainen, V., Duvall, M. R., and Besnard, G. (2007). C4 photosynthesis evolved in grasses via parallel adaptive genetic changes. Curr. Biol. 17, 1241–1247. doi: 10.1016/j.cub.2007.06.036
Dechorgnat, J., Nguyen, C. T., Armengaud, P., Jossier, M., Diatloff, E., Filleur, S., et al. (2011). From the soil to the seeds: the long journey of nitrate in plants. J. Exp. Bot. 62, 1349–1359. doi: 10.1093/jxb/erq409
Deng, H., Zhang, L. S., Zhang, G. Q., Zheng, B. Q., Liu, Z. J., and Wang, Y. (2016). Evolutionary history of PEPC genes in green plants: implications for the evolution of CAM in orchids. Mol. Phylogenet. Evol. 94, 559–564. doi: 10.1016/j.ympev.2015.10.007
Duff, S., and Chollet, R. (1995). In vivo regulation of wheat-leaf phosphoenolpyruvate carboxylase by reversible phosphorylation. Plant Physiol. 107, 775–782. doi: 10.1104/pp.107.3.775
Edgar, R. C. (2004). MUSCLE: multiple sequence alignment with high accuracy and high throughput. Nucleic Acids Res. 32, 1792–1797. doi: 10.1093/nar/gkh340
Engelmann, S., Bläsing, O. E., Westhoff, P., and Svensson, P. (2002). Serine 774 and amino acids 296 to 437 comprise the major C4 determinants of the C4 phosphoenolpyruvate carboxylase of Flaveria trinervia. FEBS Lett. 524, 11–14. doi: 10.1016/S0014-5793(02)02975-7
Gao, F., Chen, C., Arab, D. A., Du, Z., He, Y., and Ho, S. Y. W. (2019). EasyCodeML: a visual tool for analysis of selection using CodeML. Ecol. Evol. 9, 3891–3898. doi: 10.1002/ece3.5015
González-Segura, L., Mújica-Jiménez, C., Juárez-Díaz, J. A., Güémez-Toro, R., Martinez-Castilla, L. P., and Muñoz-Clares, R. A. (2018). Identification of the allosteric site for neutral amino acids in the maize C4 isozyme of phosphoenolpyruvate carboxylase: The critical role of Ser-100. J. Biol. Chem. 293, 9945–9957. doi: 10.1074/jbc.RA118.002884
Gupta, S. M., Arora, S., Mirza, N., Pande, A., Lata, C., Puranik, S., et al. (2017). Finger millet: a “certain” crop for an “uncertain” future and a solution to food insecurity and hidden hunger under stressful environments. Front. Plant Sci. 8:643. doi: 10.3389/fpls.2017.00643
Hamada, K., Hongo, K., Suwabe, K., Shimizu, A., Nagayama, T., Abe, R., et al. (2011). OryzaExpress: an integrated database of gene expression networks and omics annotations in rice. Plant Cell Physiol. 52, 220–229. doi: 10.1093/pcp/pcq195
Huang, W., Zhang, L., Columbus, J. T., Hu, Y., Zhao, Y., Tang, L., et al. (2022). A well-supported nuclear phylogeny of Poaceae and implications for the evolution of C4 photosynthesis. Mol. Plant 15, 1–23. doi: 10.1016/j.molp.2022.01.015
Jiao, Y., Li, J., Tang, H., and Paterson, A. H. (2014). Integrated syntenic and phylogenomic analyses reveal an ancient genome duplication in monocots. Plant Cell 26, 2792–2802. doi: 10.1105/tpc.114.127597
Kai, Y., Matsumura, H., and Izui, K. (2003). Phosphoenolpyruvate carboxylase: three-dimensional structure and molecular mechanisms. Arch. Biochem. Biophys. 414, 170–179. doi: 10.1016/S0003-9861(03)00170-X
Kandoi, D., Mohanty, S., Govindjee,, and Tripathy, B. C. (2016). Towards efficient photosynthesis: overexpression of Zea mays phosphoenolpyruvate carboxylase in Arabidopsis thaliana. Photosynth. Res. 130, 47–72. doi: 10.1007/s11120-016-0224-3
Lê, S., Josse, J., and Husson, F. (2008). FactoMineR: an R package for multivariate analysis. J. Stat. Softw. 25, 1–18. doi: 10.18637/jss.v025.i01
Li, H., Handsaker, B., Wysoker, A., Fennell, T., Ruan, J., Homer, N., et al. (2009). The sequence alignment/map format and SAMtools. Bioinformatics 25, 2078–2079. doi: 10.1093/bioinformatics/btp352
Liao, Y., Smyth, G. K., and Shi, W. (2014). featureCounts: an efficient general purpose program for assigning sequence reads to genomic features. Bioinformatics 30, 923–930. doi: 10.1093/bioinformatics/btt656
Lin, H. N., and Hsu, W. L. (2018). DART: a fast and accurate RNA-seq mapper with a partitioning strategy. Bioinformatics 34, 190–197. doi: 10.1093/bioinformatics/btx558
Livak, K. J., and Schmittgen, T. D. (2001). Analysis of relative gene expression data using real-time quantitative PCR and the 2−ΔΔCT method. Methods 25, 402–408. doi: 10.1006/meth.2001.1262
Masumoto, C., Miyazawa, S., Ohkawa, H., Fukuda, T., Taniguchi, Y., Murayama, S., et al. (2010). Phosphoenolpyruvate carboxylase intrinsically located in the chloroplast of rice plays a crucial role in ammonium assimilation. Proc. Natl. Acad. Sci. U. S. A. 107, 5226–5231. doi: 10.1073/pnas.0913127107
Medici, A., and Krouk, G. (2014). The primary nitrate response: a multifaceted signaling pathway. J. Exp. Bot. 65, 5567–5576. doi: 10.1093/jxb/eru245
Moriya, Y., Itoh, M., Okuda, S., Yoshizawa, A. C., and Kanehisa, M. (2007). KAAS: an automatic genome annotation and pathway reconstruction server. Nucleic Acids Res. 35, W182–W185. doi: 10.1093/nar/gkm321
Muramatsu, M., Suzuki, R., Yamazaki, T., and Miyao, M. (2015). Comparison of plant-type phosphoenolpyruvate carboxylases from rice: identification of two plant-specific regulatory regions of the allosteric enzyme. Plant Cell Physiol. 56, 468–480. doi: 10.1093/pcp/pcu189
O’Leary, B., Park, J., and Plaxton, W. C. (2011). The remarkable diversity of plant PEPC (phosphoenolpyruvate carboxylase): recent insights into the physiological functions and post-translational controls of non-photosynthetic PEPCs. Biochem. J. 436, 15–34. doi: 10.1042/BJ20110078
Pan, J., Sharif, R., Xu, X., and Chen, X. (2021). Mechanisms of waterlogging tolerance in plants: research progress and prospects. Front. Plant Sci. 11:627331. doi: 10.3389/fpls.2020.627331
Pardo, J., and VanBuren, R. (2021). Evolutionary innovations driving abiotic stress tolerance in C4 grasses and cereals. Plant Cell 33, 3391–3401. doi: 10.1093/plcell/koab205
Pathak, R. R., Das, S. K., Choudhury, D., and Raghuram, N. (2009). Genomewide bioinformatic analysis negates any specific role for Dof, GATA and ag/cTCA motifs in nitrate responsive gene expression in Arabidopsis. Physiol. Mol. Biol. Plants 15, 145–150. doi: 10.1007/s12298-009-0016-8
Prasad, V., Strömberg, C. A., Leaché, A. D., Samant, B., Patnaik, R., Tang, L., et al. (2011). Late cretaceous origin of the rice tribe provides evidence for early diversification in Poaceae. Nat. Commun. 2:480. doi: 10.1038/ncomms1482
Salse, J., Bolot, S., Throude, M., Jouffe, V., Piegu, B., Quraishi, U. M., et al. (2008). Identification and characterization of shared duplications between rice and wheat provide new insight into grass genome evolution. Plant Cell 20, 11–24. doi: 10.1105/tpc.107.056309
Sánchez, R., Flores, A., and Cejudo, F. J. (2006). Arabidopsis phosphoenolpyruvate carboxylase genes encode immunologically unrelated polypeptides and are differentially expressed in response to drought and salt stress. Planta 223, 901–909. doi: 10.1007/s00425-005-0144-5
Shenton, M., Kobayashi, M., Terashima, S., Ohyanagi, H., Copetti, D., Hernández-Hernández, T., et al. (2020). Evolution and diversity of the wild rice Oryza officinalis complex, across continents, genome types, and ploidy levels. Genome Biol. Evol. 12, 413–428. doi: 10.1093/gbe/evaa037
Shi, J., Yi, K., Liu, Y., Xie, L., Zhou, Z., Chen, Y., et al. (2015). Phosphoenolpyruvate carboxylase in Arabidopsis leaves plays a crucial role in carbon and nitrogen metabolism. Plant Physiol. 167, 671–681. doi: 10.1104/pp.114.254474
Sugiharto, B., Miyata, K., Nakamoto, H., Sasakawa, H., and Sugiyama, T. (1990). Regulation of expression of carbon-assimilating enzymes by nitrogen in maize leaf. Plant Physiol. 92, 963–969. doi: 10.1104/pp.92.4.963
Sugiharto, B., and Sugiyama, T. (1992). Effects of nitrate and ammonium on gene expression of phosphoenolpyruvate carboxylase and nitrogen metabolism in maize leaf tissue during recovery from nitrogen stress. Plant Physiol. 98, 1403–1408. doi: 10.1104/pp.98.4.1403
Sun, J., Nishiyama, T., Shimizu, K., and Kadota, K. (2013). TCC: an R package for comparing tag count data with robust normalization strategies. BMC Bioinform. 14:219. doi: 10.1186/1471-2105-14-219
Suzuki, I., Cretin, C., Omata, T., and Sugiyama, T. (1994). Transcriptional and posttranscriptional regulation of nitrogen-responding expression of phosphoenolpyruvate carboxylase gene in maize. Plant Physiol. 105, 1223–1229. doi: 10.1104/pp.105.4.1223
Tamura, K., Stecher, G., Peterson, D., Filipski, A., and Kumar, S. (2013). MEGA6: molecular evolutionary genetics analysis version 6.0. Mol. Biol. Evol. 30, 2725–2729. doi: 10.1093/molbev/mst197
The International Brachypodium Initiative (2010). Genome sequencing and analysis of the model grass Brachypodium distachyon. Nature 463, 763–768. doi: 10.1038/nature08747
Verhaak, R. G., Sanders, M. A., Bijl, M. A., Delwel, R., Horsman, S., Moorhouse, M. J., et al. (2006). HeatMapper: powerful combined visualization of gene expression profile correlations, genotypes, phenotypes and sample characteristics. BMC Bioinform. 7:337. doi: 10.1186/1471-2105-7-337
Vidal, E. A., Alvarez, J. M., Araus, V., Riveras, E., Brooks, M. D., Krouk, G., et al. (2020). Nitrate in 2020: thirty years from transport to signaling networks. Plant Cell 32, 2094–2119. doi: 10.1105/tpc.19.00748
von Caemmerer, S., and Furbank, R. T. (2016). Strategies for improving C4 photosynthesis. Curr. Opin. Plant Biol. 31, 125–134. doi: 10.1016/j.pbi.2016.04.003
Wang, N., Zhong, X., Cong, Y., Wang, T., Yang, S., Li, Y., et al. (2016). Genome-wide analysis of Phosphoenolpyruvate carboxylase gene family and their response to abiotic stresses in soybean. Sci. Rep. 6:38448. doi: 10.1038/srep38448
Waseem, M., and Ahmad, F. (2019). The phosphoenolpyruvate carboxylase gene family identification and expression analysis under abiotic and phytohormone stresses in Solanum lycopersicum L. Gene 690, 11–20. doi: 10.1016/j.gene.2018.12.033
Wei, L., Wang, L., Yang, Y., Wang, P., Guo, T., and Kang, G. (2015). Abscisic acid enhances tolerance of wheat seedlings to drought and regulates transcript levels of genes encoding ascorbate-glutathione biosynthesis. Front. Plant Sci. 6:458. doi: 10.3389/fpls.2015.00458
Woodhouse, M. R., Sen, S., Schott, D., Portwood, J. L., Freeling, M., Walley, J. W., et al. (2021). qTeller: A tool for comparative multi-genomic gene expression analysis. Bioinformatics 38, 236–242. doi: 10.1093/bioinformatics/btab604
Woolley, S., Johnson, J., Smith, M. J., Crandall, K. A., and McClellan, D. A. (2003). TreeSAAP: selection on amino acid properties using phylogenetic trees. Bioinformatics 19, 671–672. doi: 10.1093/bioinformatics/btg043
Wu, M. X., and Wedding, R. T. (1992). Inactivation of maize leaf phosphoenolpyruvate carboxylase by the binding to chloroplast membranes. Plant Physiol. 100, 382–387. doi: 10.1104/pp.100.1.382
Yamamoto, N., Kinoshita, Y., Sugimoto, T., and Masumura, T. (2017). Role of nitrogen-responsive plant-type phosphoenolpyruvate carboxylase in the accumulation of seed storage protein in ancient wheat (spelt and kamut). Soil Sci. Plant Nutr. 63, 23–28. doi: 10.1080/00380768.2016.1275039
Yamamoto, N., Kubota, T., Masumura, T., Shiraishi, N., Tanaka, K., Sugimoto, T., et al. (2014a). Molecular cloning, gene expression and functional expression of a phosphoenolpyruvate carboxylase Osppc1 in developing rice seeds: implication of involvement in nitrogen accumulation. Seed Sci. Res. 24, 23–36. doi: 10.1017/S0960258513000354
Yamamoto, N., Sasou, A., Saito, Y., Sugimoto, T., and Masumura, T. (2015). Protein and gene expression characteristics of a rice phosphoenolpyruvate carboxylase Osppc3; its unique role for seed cell maturation. J. Cereal Sci. 64, 100–108. doi: 10.1016/j.jcs.2015.04.008
Yamamoto, N., Sugimoto, T., and Masumura, T. (2014b). Concomitant increases of the developing seed phosphoenolpyruvate carboxylase activity and the seed protein content of field-grown wheat with nitrogen supply. Agric. Sci. 5:1558. doi: 10.4236/as.2014.514167
Yamamoto, N., Sugimoto, T., Takano, T., Sasou, A., Morita, S., Yano, K., et al. (2020). The plant-type phosphoenolpyruvate carboxylase Gmppc2 is developmentally induced in immature soy seeds at the late maturation stage: a potential protein biomarker for seed chemical composition. Biosci. Biotechnol. Biochem. 84, 552–562. doi: 10.1080/09168451.2019.1696179
Yanagisawa, S. (2000). Dof1 and Dof2 transcription factors are associated with expression of multiple genes involved in carbon metabolisms in maize. Plant J. 21, 281–288. doi: 10.1046/j.1365-313x.2000.00685.x
Yanagisawa, S., and Sheen, J. (1998). Involvement of maize Dof zinc finger proteins in tissue-specific and light-regulated gene expression. Plant Cell 10, 75–89. doi: 10.1105/tpc.10.1.75
Zhang, Y. H., Zhou, S. L., Huang, Q., Leng, G. H., Xue, Q. W., Stewart, B. A., et al. (2012). Effects of sucrose and ammonium nitrate on phosphoenolpyruvate carboxylase and ribulose-1, 5-bisphosphate carboxylase activities in wheat ears. Aust. J. Crop. Sci. 6, 822–827.
Zhao, H., Gao, Z., Wang, L., Wang, J., Wang, S., Fei, B., et al. (2018). Chromosome-level reference genome and alternative splicing atlas of moso bamboo (Phyllostachys edulis). Gigascience 7:giy115. doi: 10.1093/gigascience/giy115
Keywords: abiotic stress, gene function and evolution, grass genome evolution, nitrate response, phosphoenolpyruvate carboxylase, positive selection, ppc1b, Pooideae
Citation: Yamamoto N, Tong W, Lv B, Peng Z and Yang Z (2022) The Original Form of C4-Photosynthetic Phosphoenolpyruvate Carboxylase Is Retained in Pooids but Lost in Rice. Front. Plant Sci. 13:905894. doi: 10.3389/fpls.2022.905894
Edited by:
olivier Panaud, Université de Perpignan Via Domitia, FranceReviewed by:
Prathima Perumal Thirugnanasambandam, Indian Council of Agricultural Research, Coimbatore, IndiaSubramanian Babu, VIT University, India
Pandiyan Muthuramalingam, Gyeongsang National University, South Korea
Copyright © 2022 Yamamoto, Tong, Lv, Peng and Yang. This is an open-access article distributed under the terms of the Creative Commons Attribution License (CC BY). The use, distribution or reproduction in other forums is permitted, provided the original author(s) and the copyright owner(s) are credited and that the original publication in this journal is cited, in accordance with accepted academic practice. No use, distribution or reproduction is permitted which does not comply with these terms.
*Correspondence: Zaijun Yang, eWFuZ3phaWp1bjFAMTI2LmNvbQ==
†These authors have contributed equally to this work