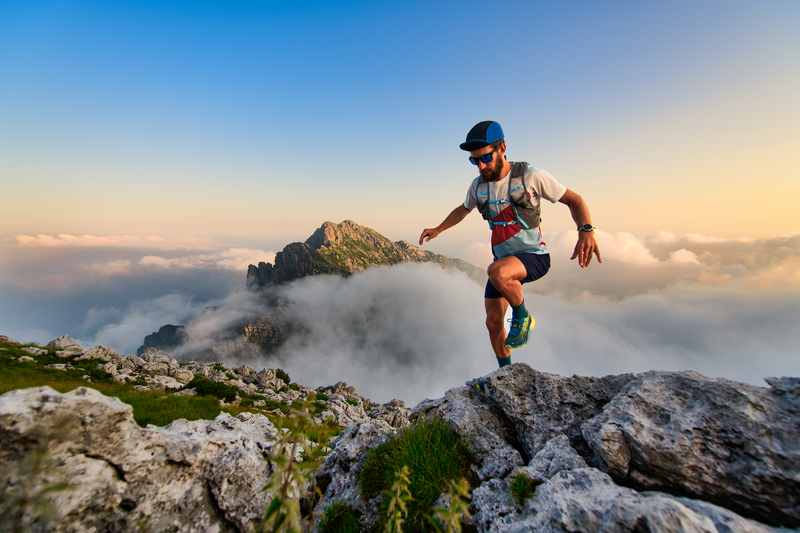
94% of researchers rate our articles as excellent or good
Learn more about the work of our research integrity team to safeguard the quality of each article we publish.
Find out more
ORIGINAL RESEARCH article
Front. Plant Sci. , 27 July 2022
Sec. Plant Abiotic Stress
Volume 13 - 2022 | https://doi.org/10.3389/fpls.2022.905100
This article is part of the Research Topic Chlorophyll Fluorescence Measurements and Plant Stress Responses, Volume II View all 13 articles
Jerusalem artichoke (Helianthus tuberosus L.), a vegetable with medical applications, has a strong adaptability to marginal barren land, but the suitability as planting material in saline land remains to be evaluated. This study was envisaged to examine salt tolerance in Jerusalem artichoke from the angle of photosynthetic apparatus stability by dissecting the photosynthetic electron transport process. Potted plants were exposed to salt stress by watering with a nutrient solution supplemented with NaCl. Photosystem I (PSI) and photosystem II (PSII) photoinhibition appeared under salt stress, according to the significant decrease in the maximal photochemical efficiency of PSI (△MR/MR0) and PSII. Consistently, leaf hydrogen peroxide (H2O2) concentration and lipid peroxidation were remarkably elevated after 8 days of salt stress, confirming salt-induced oxidative stress. Besides photoinhibition of the PSII reaction center, the PSII donor side was also impaired under salt stress, as a K step emerged in the prompt chlorophyll transient, but the PSII acceptor side was more vulnerable, considering the decreased probability of an electron movement beyond the primary quinone (ETo/TRo) upon depressed upstream electron donation. The declined performance of entire PSII components inhibited electron inflow to PSI, but severe PSI photoinhibition was not averted. Notably, PSI photoinhibition elevated the excitation pressure of PSII (1-qP) by inhibiting the PSII acceptor side due to the negative and positive correlation of △MR/MR0 with 1-qP and ETo/TRo, respectively. Furthermore, excessive reduction of PSII acceptors side due to PSI photoinhibition was simulated by applying a specific inhibitor blocking electron transport beyond primary quinone, demonstrating that PSII photoinhibition was actually accelerated by PSI photoinhibition under salt stress. In conclusion, PSII and PSI vulnerabilities were proven in Jerusalem artichoke under salt stress, and PSII inactivation, which was a passive consequence of PSI photoinhibition, hardly helped protect PSI. As a salt-sensitive species, Jerusalem artichoke was recommended to be planted in non-saline marginal land or mild saline land with soil desalination measures.
As a major abiotic stress endangering agricultural production, soil salinity usually lies in farmland with irrational irrigation and saline land in inland arid and coastal regions (Nikalje et al., 2017). Under salt stress, plants are first confronted with osmotic stress and then have to endure ionic toxicity; however, the damages to biological macromolecules often resulted from the salt-induced excess generation of reactive oxygen species (ROS) in plant cells (Gill and Tuteja, 2010; Hossain and Dietz, 2016; Chen et al., 2018). In photosynthetic organisms, ROS can be considered a by-product of photosynthetic electron transport in the chloroplast (Asada, 2006; Gill and Tuteja, 2010; Foyer, 2018).
Photosynthetic electron transport from water to NADP+ is powered by photosystem II (PSII) and photosystem I (PSI), and this electron transport chain also involves other electron carriers such as oxygen-evolving complex, primary and secondary quinone (QA and QB), and plastoquinone (PQ). In contrast to the equilibrium state of ROS in plants under normal growth condition, depressed CO2 assimilation will inhibit photosynthetic electron transport in a feedback way and then elevate excitation pressure in the chloroplast under abiotic stress (Murata et al., 2007; Takahashi and Murata, 2008; Zhang et al., 2014; Yan et al., 2015, 2018b). As a consequence, a great number of photosynthetic electrons tend to be transferred to O2 rather than NADP+ to generate superoxide anion (O2–). Hydrogen peroxide (H2O2) is generated from O2– through dismutation reaction, and then hydroxyl radical, the most dangerous ROS, may be synthesized by the Fenton reaction finally (Gill and Tuteja, 2010; Foyer, 2018). In addition, the elevated excitation pressure can also cause greater production of singlet oxygen in PSII reaction centers (Foyer, 2018). The excess generation of these ROS may bring about PSII and PSI photoinhibition by impairing photosynthetic membrane proteins or lipids. In particular, Oukarroum et al. (2015) illustrated that PSI and PSII photochemical capacities were negatively correlated with ROS production. At present, salt-induced PSII photoinhibition has been extensively documented. Traditionally, PSII is considered more vulnerable than PSI under abiotic stresses, and rapid PSII photoinhibition can protect PSI by reducing ROS production at its acceptor side by restricting electron flow to PSI under light stress or high temperature (Yan et al., 2013a,b; Zivcak et al., 2014; Zhang et al., 2016). In contrast, limited restriction on PSII electron donation is liable to induce PSI photoinhibition under chilling stress (Zhang et al., 2011, 2014; Yang et al., 2014). PSI was also demonstrated to be a possible photoinhibition site under salt stress in our recent study, as PSII photoinhibition hardly prevented PSI photoinhibition in a salt-sensitive honeysuckle cultivar (Yan et al., 2015). Compared with PSII photoinhibition, PSI photoinhibition is more harmful in light of its difficult recovery (Sonoike, 2011), and particularly, PSI vulnerability poses a big threat to PSII by aggravating feedback inhibition at the PSII acceptor side. Therefore, PSII and PSI interaction is very important for plants to adapt to abiotic stress. To date, less attention has been paid to the salt tolerance of PSI than PSII, and moreover, PSII and PSI interaction remain largely unknown under salt stress. In other words, the characterization of photosynthetic electron transport has not been thoroughly dissected in plants under salt-induced oxidative stress, since PSII and PSI interaction relies on this electron transport process.
The Jerusalem artichoke (Helianthus tuberosus L.) is a vegetable native to North America. The Jerusalem artichoke can be used for medical applications and ethanol production because the tubers contain quantities of fructose and inulin (Baldini et al., 2004; Saengthongpinit and Saijaanantakul, 2005). In recent years, we have analyzed photosynthetic characteristics at various leaf expansion stages, verified PSII susceptibility to high temperature, and particularly demonstrated the sensitivity to waterlogging from aspects of PSI vulnerability and photosynthesis in Jerusalem artichoke (Yan et al., 2012, 2013b, 2018b). Notably, Jerusalem artichoke has been selected for an attempt to utilize marginal land in the coastal zone in China, considering its high capacity to acclimate to barren soil (Long et al., 2016). It has been reported that salt stress can decrease CO2 assimilation and induce oxidative injury with chlorophyll loss in Jerusalem artichoke (Long et al., 2009; Huang et al., 2012; Li et al., 2017). However, the stability of photosystems has not been paid enough attention in Jerusalem artichoke under salt-induced oxidative stress, let alone the characterization of photosynthetic electron transport.
A new technique has been recently developed to simultaneously detect prompt chlorophyll fluorescence (PF), modulated 820 nm reflection (MR), and delayed chlorophyll fluorescence (DF) (Goltsev et al., 2009; Strasser et al., 2010; Gao et al., 2014; Yan et al., 2018a). In this study, we aimed to investigate photosystems performance and interaction by analyzing the photosynthetic electron transport process in Jerusalem artichoke under salt-induced oxidative stress using this technique. This study can deeply unveil the mechanism of plant resistance to salt stress and may aid in the exploitation of marginal abandoned land.
In Laizhou Bay, China, Jerusalem artichoke tubers were gathered and cultivated in the room as in the previous study (Yan et al., 2018b). The tubers were planted in plastic pots (20 cm in diameter and 25 cm high) filled with vermiculite and cultured in an artificial climatic room (Qiushi, China). There was one tuber in each pot, and the vermiculite was kept wet by watering. The photon flux density, day/night temperature, and humidity were controlled at 400 μmol m–2 s–1 (12 h/day from 07:00 to 19:00), 25/18°C, and 70% in the room, respectively. After 1 month, the germinated seedlings appeared, and their growth was ensured by daily watering with Hoagland nutrient solution (pH 5.7). After 1 month, 45 uniform seedling plants were chosen and divided into three groups. In the first group, the control plants were not subjected to NaCl stress. In the second group, plants were subjected to 100 mM NaCl stress. In the third group, plants were subjected to 200 mM NaCl stress. NaCl was added to the nutrient solution gradually by 50 mM step every day to the final treatment concentrations (100 and 200 mM) on the same day, and thereafter, the salt stress persisted for 8 days. During the salt treatment experiment, the solution was refreshed every 2 days, and before refreshing the solution, the culture substrate was thoroughly leached using the nutrient solution to avoid ion accumulation. The newest fully expanded leaves were sampled to measure physiological and biochemical parameters.
Fresh leaf tissues were sampled for measuring MDA, H2O2, Na+, and relative water contents using colorimetric methods, and the detailed procedure was reported in our previous studies (Yan et al., 2015, 2018b).
An open photosynthetic system (LI-6400XTR, Li-Cor, Lincoln, NE, United States) equipped with a fluorescence leaf chamber (6400-40 LCF, Li-Cor) was utilized, and the same measuring procedure in our previous study was adopted for measuring the photosynthetic rate (Pn) and stomatal conductance (gs) (Yan et al., 2018b). The actual photochemical efficiency of PSII (ΦPSII) and photochemical quenching coefficient were also recorded, and then PSII excitation pressure (1-qP) was calculated.
The detection of PF, DF, and MR transients was simultaneously conducted using a multifunctional plant efficiency analyzer (MPEA, Hansatech, Norfolk, United Kingdom) with the same illumination procedure as in our previous study (Yan et al., 2018a). According to Schansker et al. (2003) and Strasser et al. (2010), the maximal photochemical efficiencies of PSII (Fv/Fm) and PSI (ΔMR/MR0), QA reducing reaction centers per PSII antenna chlorophyll (RC/ABS), variable fluorescence intensity at K step (Vk), the probability with which an electron moves beyond QA (ETo/TRo), and from the intersystem electron carriers to reduce PSI end electron acceptors (REo/ETo) were calculated.
One-way ANOVA was performed using SPSS 16.0 (SPSS Inc., Chicago, IL, United States) for all data, which are the average value from five replicate plants. The average value was compared through the LSD test. Regression analysis of △MR/MR0 with 1-qP and ETo/TRo was also performed using SPSS 16.0.
The level of lipid peroxidation in plant tissues can be reflected by MDA content. After 8 days of 100 mM NaCl stress, H2O2, and MDA contents were significantly elevated by 48.89 and 14.86% in the leaves of Jerusalem artichoke, and the increase was up to 152.46 and 46.42% under 200 mM NaCl stress (Figures 1A,B). Leaf Na+ was significantly increased by 2.65– and 5.92-fold after 8 days of 100 and 200 mM NaCl stress, respectively (Figure 1C). Leaf relative water content remarkably decreased on day 8 under 100 and 200 mM NaCl stress, and there was no significant difference in leaf relative water content between the two salt treatments (Figure 1D).
Figure 1. Changes in leaf H2O2 (A), malondialdehyde (MDA) (B), Na+ (C), and relative water (D) contents in Jerusalem artichoke after 8 days of 100 and 200 mM NaCl stress. Data in the figure are the average value of five replicates (±SD), and the different letters on error bars indicate remarkable differences among salt treatments at P < 0.05. CP, T1, and T2 indicate control plants, plants exposed to 100 and 200 mM NaCl, respectively, and these symbols are also used in the following figures.
After 2 days of 100 mM NaCl stress, Pn, gs, and ΦPSII were significantly reduced, and the reduction was up to 52.51, 68.09, and 37.66% on day 8 (Figures 2A–C). In comparison, the reduction of Pn, gs, and ΦPSII was far greater under 200 mM NaCl stress (Figures 2A–C). Under 100 mM NaCl stress, 1-qP was significantly elevated on day 2, and the elevation reached 27.67% on day 8, whereas the elevation of 1-qP was greater under 200 mM NaCl stress (Figure 2D).
Figure 2. Changes in photosynthetic rate (Pn) (A), stomatal conductance (gs) (B), actual photochemical efficiency of photosystem II (PSII) (ΦPSII) (C), and PSII excitation pressure (1-qP) (D) in Jerusalem artichoke under 100 and 200 mM NaCl stress. Data in the figure are the average value of five replicates (±SD), and the different letters on error bars indicate remarkable differences among salt treatments at P < 0.05.
J and I steps indicate the accumulation of reduced QA and PQ (Schansker et al., 2003, 2005; Yan et al., 2013b). J and I steps obviously rose under salt stress on day 8, suggesting that PQ and QA re-oxidation were inhibited (Figure 3A). The occurrence of K step around 300 μs suggests the injury on OEC at the PSII donor side (Oukarroum et al., 2013, 2016). After 8 days of 200 mM NaCl stress, the PSII donor side was damaged, as indicated by the occurrence of the K step (Figure 3A). In contrast, J and I steps were less elevated, and the K step did not appear under 100 mM NaCl stress (Figure 3A).
Figure 3. Transients of prompt chlorophyll fluorescence (A), delayed chlorophyll fluorescence (B), modulated 820 nm reflection (C), and photosystem I (PSI) oxidation and re-reduction amplitude (D) in Jerusalem artichoke after 8 days of 100 and 200 mM NaCl stress. The specific steps in chlorophyll fluorescence transient are O, K, J, I, and P. The value of modulated 820 nm at the onset of red light illumination [0.7 ms, the first reliable modulated reflection (MR) measurement] is MR0. PSI oxidation and re-reduction amplitude were represented by MR0–MRmin and MRmax–MRmin, respectively. Data of MR0–MRmin and MRmax–MRmin indicate the average value of five replicates (±SD), and the different letters on error bars indicate significant differences at P < 0.05. In delayed chlorophyll fluorescence curves, D0, I1, I2, and D2 are the initial point, the first (7 ms) and second (50 ms) maximal peaks, and the minimum point. The initial microsecond delayed fluorescence signal at 0.3 ms is indicated by DF0.3 ms. The signals were plotted on a logarithmic timescale, and each curve is the mean of five replicate plants.
During PSI oxidation, MR0 decreased to the minimal value (MRmin). Subsequently, PSI re-reduction was initiated, and MR/MR0 increased to the maximal level (MRmax). MR transient was remarkably changed by salt stress, as MR0–MRmin and MRmax–MRmin significantly decreased (Figures 3C,D), suggesting the negative effect on both PSI oxidation and re-reduction, and the variations were greater in plants under 200 mM NaCl stress than 100 mM NaCl stress (Figures 3C,D). Under salt stress, DF transient was prominently suppressed in line with lowered I1 and I2 peaks, and obviously, the influence was less in plants under 100 mM NaCl stress than 200 mM NaCl stress (Figure 3B).
After 200 mM NaCl stress for 5 days, △MR/MR0 and Fv/Fm were significantly reduced, and the reduction was up to 54.31 and 9.06% on day 8 (Figures 4A,B). After 8 days of 100 mM NaCl stress, the obvious decrease of 32.15 and 2.94% appeared in △MR/MR0 and Fv/Fm, respectively (Figures 4A,B). The greater decrease in △MR/MR0 than Fv/Fm implied that PSI photoinhibition was more severe than PSII photoinhibition under NaCl stress. After 5 days of 200 mM NaCl stress, ETo/Tro, and REo/ETo significantly declined, while the marked decrease in them was not found until 8 days of 100 mM NaCl stress (Figures 4E,F). No obvious effect on Vk was noted under 100 mM NaCl stress, but it was significantly increased after 200 mM NaCl stress for 5 days (Figure 4C). Under salt stress, only a mild decrease was observed in RC/ABS (Figure 4D).
Figure 4. Changes in the maximal photochemical efficiency of photosystem II (PSII) (Fv/Fm) (A), photosystem I (PSI) (△MR/MR0) (B), variable fluorescence intensity at K step (Vk) (C), primary quinone reducing reaction centers per PSII antenna chlorophyll (RC/ABS) (D), probability that an electron moves beyond primary quinone (ETo/TRo) (E), and probability with which an electron from the intersystem electron carriers is transferred to reduce end electron acceptors at the PSI acceptor side (REo/ETo) (F) in Jerusalem artichoke under 100 and 200 mM NaCl stress. Data in the figure are the average value of five replicates (±SD), and the different letters on error bars indicate remarkable differences among salt treatments at P < 0.05.
According to the regression analysis, △MR/MR0 had a significant positive correlation with ETo/TRo, whereas, the correlation between 1-qP and △MR/MR0 was markedly negative (Figures 5A,C). DCMU functioned as a specific inhibitor for intervening electron transport from QA– to QB–, and Fv/Fm and ETo/TRo were significantly decreased in plants applied with DCMU than those without DCMU application after 8 days of salt stress (Figures 5B,D).
Figure 5. Regression of the maximal photochemical efficiency of photosystem II (PSI) (△MR/MR0) with PSII excitation pressure (1-qP) (A) and probability that an electron moves beyond primary quinone (ETo/TRo) (C) in Jerusalem artichoke. The significant correlation at P < 0.05 was indicated by #. Effects of applying DCMU on the maximal photochemical efficiency of PSII (Fv/Fm) (B) and ETo/TRo (D) in Jerusalem artichoke after 8 days of 100 and 200 mM NaCl stress. For reagent treatment, the leaves after 5 days of 100 and 200 mM NaCl stress were immersed in 0 or 70 μM DCMU for 3 h in the dark. Data of Fv/Fm and ETo/TRo indicate the average value of five replicates (±SD), and the different letters on error bars indicate remarkable differences between the leaves with and without DCMU treatment at P < 0.05.
As an ordinary finding, photosynthesis was depressed by salt stress in line with stomatal closure in Jerusalem artichoke (Figures 2A,B). The inhibited CO2 fixation can cause feedback inhibition on photosynthetic electron transport and accelerate ROS production as more photosynthetic electrons are diverged to oxygen (Gill and Tuteja, 2010; Foyer, 2018). Exactly, the elevated leaf lipid peroxidation and H2O2 concentration proved salt-induced oxidative stress on Jerusalem artichoke (Figures 1A,B). Elevated ROS generation in photosynthetic organisms is usually associated with the inhibited photosynthetic electron transport and can cause photosystems photoinibition with oxidative damage to photosynthetic membranes lipids and proteins (Murata et al., 2007; Sonoike, 2011; Oukarroum et al., 2015). Therefore, photosystem photoinhibition seems to be a feasible proxy for the oxidative threat to the plant (Zhang et al., 2012, 2014; Yan et al., 2015, 2018b). Under salt stress, Na+ toxicity may induce more severe oxidative stress on photosystems than osmotic pressure (Muranaka et al., 2002; Allakhverdiev and Murata, 2008; Cha-um and Kirdmanee, 2010; Hossain et al., 2017). In this study, leaf oxidative damage also resulted from Na+ toxicity rather than osmotic pressure to a greater extent, as severe lipid peroxidation appeared with greater leaf Na+ accumulation rather than leaf water deficit under salt stress with 200 mM NaCl than 100 mM NaCl (Figures 1C,D).
Consistent with leaf ROS burst, salt stress actually caused PSI and PSII photoinhibition according to the significantly lowered Fv/Fm and △MR/MR0 in Jerusalem artichoke (Figures 4A,B). The classic proxy for the photochemical capability of the PSII reaction center, Fv/Fm rarely reflects PSII whole performance (Li et al., 2009). Under 200 mM NaCl stress, the elevated J step and declined ETo/Tro suggested the inhibited electron transport beyond QA with accumulated QA–, while electron donation from the oxygen-evolving complex was also constrained due to the increased Vk (Figures 3A, 4C,E). I1 peak indicating the accumulation of S3Z+P680QA– can comprehensively reflect the state of the whole PSII, including active reaction centers and electron transporters at both donor and acceptor sides (Goltsev et al., 2009; Gao et al., 2014). Depressed I1 corroborated salt-induced damage on PSII (Figure 3B). The value of ETo/TRo is dependent not only on electrons transferred beyond QA but also on electrons donation from upstream electron carriers. Thus, the PSII acceptor side exhibited greater salt susceptibility than the reaction center and donor side in view of the significant reduction in ETo/TRo on the premise of lowered electron donation from the upstream under 200 mM NaCl stress. Consistently, the similar variations of ETo/TRo, Fv/Fm, and Vk under 100 mM NaCl stress also verified the greater susceptibility of the PSI acceptor side (Figures 4A,C,E). In addition, unchanged Vk and K step with lowered Fv/Fm under 100 mM NaCl stress suggested that salt sensitivity of the PSII donor side was lower than the PSII reaction center (Figures 4A,C). In summary, the salt sensitivity of PSII components gradually rose along with the direction of photosynthetic electron transport. The responses of whole PSII components also implied PSII vulnerability in Jerusalem artichoke under salt stress.
The declined PSII performance was consistent with the elevated PSII excitation pressure upon declined CO2 assimilation and restricted electron flow to PSI when photosynthesis reached a steady-state (Figures 2A,C,D). In MR transients, the lowered PSI re-reduction amplitude also suggested the restricted electron donation from PSII (Figures 3C,D). The restricted electron donation from PSII can help protect PSI against photoinhibition by decreasing the probability of ROS generation at the PSI acceptor side. However, PSI photoinhibition was never prevented under salt stress and was even more severe than PSII photoinhibition, considering the greater decreased amplitude of △MR/MR0 than Fv/Fm (Figures 4A,B). Limited electron inflow should improve PSI oxidation by blocking its re-reduction in MR transients; however, PSI oxidation was curtailed with decreased PSI oxidative amplitude, confirming that PSI encounters greater damage than PSII (Figures 3C,D). Because the reopening of PSII reaction centers is prolonged by electron transfer from reduced quinone to plastoquinone before the plastoquinone pool is fully reduced, an I2 phase appears in DF transient (Goltsev et al., 2009). Salt-induced decrease in I2 coincided with decreased REo/ETo and elevated I step, and all these changes pointed to that salt-induced PSI damage led to inhibition of PQ re-oxidation (Figures 3A,B, 4F). To summarize, PSI was more vulnerable to salt stress than PSII in Jerusalem artichoke, but in disagreement with the traditional viewpoint, PSII inactivation offered scarce protection to PSI.
In accordance with the negative correlation of △MR/MR0 with 1-qP and the positive correlation of △MR/MR0 with ETo/TRo (Figures 5A,C), PSI photoinhibition led to feedback inhibition on PSII electron outflow at the acceptor side and then elevated exciting pressure of PSII in Jerusalem artichoke upon salt stress. In addition, over-reduction of PSII acceptor side due to PSI photoinhibition was simulated by the experiment of DCMU application, and the result demonstrated that PSII photoinhibition was actually accelerated by PSI photoinhibition in Jerusalem artichoke under salt stress (Figures 5B,D). Thus, salt-induced depression on PSII performance should be interpreted as a result of PSI photoinhibition, and the passive PSII inactivation was rarely capable of defending PSI oxidative injury. Invalid PSII and PSI interaction has been found with PSI vulnerability in sensitive plants under abiotic stress and can bring about detrimental effects on the entire photosynthetic apparatus (Zhang et al., 2014; Yan et al., 2018b). Accordingly, Jerusalem artichoke should be classified as a salt-sensitive plant.
Photosystem II and PSI vulnerability to salt stress were illustrated in Jerusalem artichoke, and PSII inactivation, which was a passive consequence of PSI photoinhibition, hardly helped defend PSI. Given the salt sensitivity of Jerusalem artichoke, it is better to select non-saline marginal land for planting in agricultural practice, or the mild saline land in the coastal zone can also be used in combination with some desalination measures such as freshwater leaching and applying salt separation layer.
The raw data supporting the conclusions of this article will be made available by the authors, without undue reservation.
KY designed and performed the experiment and wrote the manuscript. HM, JC, and YS participated in the experiment. XD and SZ participated in the data analysis. All authors have read the manuscript and approved the final version of the manuscript.
This study was jointly financed by the National Key Research and Development Project in China (2019YFD1002702), the Joint Funds of the National Natural Science Foundation of China (U2106214), and the Yantai Science and Technology Innovation Development Plan (2020MSGY065).
The authors declare that the research was conducted in the absence of any commercial or financial relationships that could be construed as a potential conflict of interest.
All claims expressed in this article are solely those of the authors and do not necessarily represent those of their affiliated organizations, or those of the publisher, the editors and the reviewers. Any product that may be evaluated in this article, or claim that may be made by its manufacturer, is not guaranteed or endorsed by the publisher.
ETo/TRo, the probability for an electron movement beyond primary quinone; gs, stomatal conductance; MDA, malondialdehyde; Pn, photosynthetic rate; PSI, photosystem I; PSII, photosystem II; RC/ABS, primary quinone reducing reaction centers per PSII antenna chlorophyll; PQ, plastoquinone; REo/ETo, the probability with which an electron from the intersystem electron carriers is transferred to reduce end electron acceptors at the PSI acceptor side; QA, primary quinone; ROS, reactive oxygen species; Vk, variable fluorescence intensity at K step; △ MR/MR0, the maximal photochemical capacity of PSI; Φ PSII, actual photochemical efficiency of PSII; 1-qP, excitation pressure of PSII.
Allakhverdiev, S. I., and Murata, N. (2008). Salt stress inhibits photosystems II and I in cyanobacteria. Photosynth. Res. 98, 529–539. doi: 10.1007/s11120-008-9334-x
Asada, K. (2006). Production and scavenging of reactive oxygen species in chloroplasts and their functions. Plant Physiol. 141, 391–396. doi: 10.1104/pp.106.082040
Baldini, M., Danuso, F., Turi, M., and Vannozzi, P. (2004). Evaluation of new clones of Jerusalem artichoke (Helianthus tuberosus L.) for inulin and sugar yield from stalks and tubers. Ind. Crop. Prod. 19, 25–40. doi: 10.1016/S0926-6690(03)00078-5
Cha-um, S., and Kirdmanee, C. (2010). Effects of water stress induced by sodium chloride and mannitol on proline accumulation, photosynthetic abilities and growth characters of eucalyptus (Eucalyptus camaldulensis Dehnh.). New For. 40, 349–360. doi: 10.1007/s11056-010-9204-1
Chen, M., Yang, Z., Liu, J., Zhu, T., Wei, X., Fan, H., et al. (2018). Adaptation mechanism of salt excluders under saline conditions and its applications. Int. J. Mol. Sci. 19:3668. doi: 10.3390/ijms19113668
Foyer, C. H. (2018). Reactive oxygen species, oxidative signaling and the regulation of photosynthesis. Environ. Exp. Bot. 154, 134–142. doi: 10.1016/J.ENVEXPBOT.2018.05.003
Gao, J., Li, P., Ma, F., and Goltsev, V. (2014). Photosynthetic performance during leaf expansion in Malus micromalus probed by chlorophyll a fluorescence and modulated 820nm reflection. J. Photochem. Photobiol. B Biol. 137, 144–150. doi: 10.1016/J.JPHOTOBIOL.2013.12.005
Gill, S. S., and Tuteja, N. (2010). Reactive oxygen species and antioxidant machinery in abiotic stress tolerance in crop plants. Plant Physiol. Biochem. 48, 909–930. doi: 10.1016/J.PLAPHY.2010.08.016
Goltsev, V., Zaharieva, I., Chernev, P., and Strasser, R. J. (2009). Delayed fluorescence in photosynthesis. Photosynth. Res. 101, 217–232. doi: 10.1007/S11120-009-9451-1
Hossain, M. S., Alam, M. U., Rahman, A., Hasanuzzaman, M., Nahar, K., Al Mahmud, J., et al. (2017). Use of iso-osmotic solution to understand salt stress responses in lentil (Lens culinaris Medik.). S. Afr. J. Bot. 113, 346–354. doi: 10.1016/j.sajb.2017.09.007
Hossain, M. S., and Dietz, K. J. (2016). Tuning of redox regulatory mechanisms, reactive oxygen species and redox homeostasis under salinity stress. Front. Plant Sci. 7:548. doi: 10.3389/FPLS.2016.00548
Huang, Z., Long, X., Wang, L., Kang, J., Zhang, Z., Zed, R., et al. (2012). Growth, photosynthesis and H+-ATPase activity in two Jerusalem artichoke varieties under NaCl-induced stress. Process Biochem. 47, 591–596. doi: 10.1016/J.PROCBIO.2011.12.016
Li, L., Shao, T., Yang, H., Chen, M., Gao, X., Long, X., et al. (2017). The endogenous plant hormones and ratios regulate sugar and dry matter accumulation in Jerusalem artichoke in salt-soil. Sci. Total Environ. 578, 40–46. doi: 10.1016/J.SCITOTENV.2016.06.075
Li, P. M., Cheng, L. L., Gao, H. Y., Jiang, C. D., and Peng, T. (2009). Heterogeneous behavior of PSII in soybean (Glycine max) leaves with identical PSII photochemistry efficiency under different high temperature treatments. J. Plant Physiol. 166, 1607–1615. doi: 10.1016/j.jplph.2009.04.013
Long, X., Huang, Z., Zhang, Z., Li, Q., Zed, R., and Liu, Z. (2009). Seawater stress differentially affects germination, growth, photosynthesis, and ion concentration in genotypes of Jerusalem Artichoke (Helianthus tuberosus L.). J. Plant Growth Regul. 29, 223–231. doi: 10.1007/S00344-009-9125-4
Long, X. H., Liu, L. P., Shao, T. Y., Shao, H. B., and Liu, Z. P. (2016). Developing and sustainably utilize the coastal mudflat areas in China. Sci. Total Environ. 569-570, 1077–1086. doi: 10.1016/J.SCITOTENV.2016.06.170
Muranaka, S., Shimizu, K., and Kato, M. (2002). Ionic and osmotic effects of salinity on single-leaf photosynthesis in two wheat cultivars with different drought tolerance. Photosynthetica 40, 201–207. doi: 10.1023/A:1021337522431
Murata, N., Takahashi, S., Nishiyama, Y., and Allakhverdiev, S. I. (2007). Photoinhibition of photosystem II under environmental stress. Biochim. Biophys. Acta 1767, 414–421. doi: 10.1016/J.BBABIO.2006.11.019
Nikalje, G. C., Srivastava, A. K., Pandey, G. K., and Suprasanna, P. (2017). Halophytes in biosaline agriculture: mechanism, utilization, and value addition. Land Degrad. Dev. 29, 1081–1095. doi: 10.1002/LDR.2819
Oukarroum, A., Bussotti, F., Goltsev, V., and Kalaji, H. M. (2015). Correlation between reactive oxygen species production and photochemistry of photosystems I and II in Lemna gibba L. plants under salt stress. Environ. Exp. Bot. 109, 80–88. doi: 10.1016/J.ENVEXPBOT.2014.08.005
Oukarroum, A., El Madidi, S., and Strasser, R. J. (2016). Differential heat sensitivity index in barley cultivars (Hordeum vulgare L.) monitored by chlorophyll a fluorescence OKJIP. Plant Physiol. Biochem. 105, 102–108. doi: 10.1016/J.PLAPHY.2016.04.015
Oukarroum, A., Goltsev, V., and Strasser, R. J. (2013). Temperature effects on pea plants probed by simultaneous measurements of the kinetics of prompt fluorescence, delayed fluorescence and modulated 820 nm reflection. PLoS One 8:e59433. doi: 10.1371/JOURNAL.PONE.0059433
Saengthongpinit, W., and Saijaanantakul, T. (2005). Influence of harvest time and storage temperature on characteristics of inulin from Jerusalem artichoke (Helianthus tuberosus L.) tubers. Postharvest Biol. Technol. 37, 93–100. doi: 10.1016/J.POSTHARVBIO.2005.03.004
Schansker, G., Srivastava, A., Govindjee, and Strasser, R. J. (2003). Characterization of the 820-nm transmission signal paralleling the chlorophyll a fluorescence rise (OJIP) in pea leaves. Funct. Plant Biol. 30, 785–796. doi: 10.1071/FP03032
Schansker, G., Toth, S. Z., and Strasser, R. J. (2005). Methylviologen and dibromothymoquinone treatments of pea leaves reveal the role of photosystem I in the Chl a fluorescence rise OJIP. Biochim. Biophys. Acta 1706, 250–261. doi: 10.1016/J.BBABIO.2004.11.006
Sonoike, K. (2011). Photoinhibition of photosystem I. Physiol. Plant. 142, 56–64. doi: 10.1111/J.1399-3054.2010.01437.X
Strasser, R. J., Tsimilli-Michael, M., Qiang, S., and Goltsev, V. (2010). Simultaneous in vivo recording of prompt and delayed fluorescence and 820 nm reflection changes during drying and after rehydration of the resurrection plant Haberlea rhodopensis. Biochim. Biophys. Acta 1797, 1313–1326. doi: 10.1016/J.BBABIO.2010.03.008
Takahashi, S., and Murata, N. (2008). How do environmental stresses accelerate photoinhibition? Trends Plant Sci. 13, 178–182. doi: 10.1016/J.TPLANTS.2008.01.005
Yan, K., Chen, P., Shao, H., Zhao, S., Zhang, L., Zhang, L., et al. (2012). Photosynthetic characterization of Jerusalem artichoke during leaf expansion. Acta Physiol. Plant. 34, 353–360. doi: 10.1007/s11738-011-0834-5
Yan, K., Chen, P., Shao, H. B., Shao, C. Y., Zhao, S. J., and Brestic, M. (2013a). Dissection of photosynthetic electron transport process in sweet sorghum under heat stress. PLoS One 8:e62100. doi: 10.1371/JOURNAL.PONE.0062100
Yan, K., Chen, P., Shao, H. B., and Zhao, S. J. (2013b). Characterization of photosynthetic electron transport chain in bioenergy crop Jerusalem artichoke (Helianthus tuberosus L.) under heat stress for sustainable cultivation. Ind. Crop. Prod. 50, 809–815. doi: 10.1016/J.INDCROP.2013.08.012
Yan, K., Zhao, S., Cui, M., Han, G., and Wen, P. (2018b). Vulnerability of photosynthesis and photosystem I in Jerusalem artichoke (Helianthus tuberosus L.) exposed to waterlogging. Plant Physiol. Biochem. 125, 239–246. doi: 10.1016/J.PLAPHY.2018.02.017
Yan, K., Han, G., Ren, C., Zhao, S., Wu, X., and Bian, T. (2018a). Fusarium solani Infection depressed photosystem performance by inducing foliage wilting in apple seedlings. Front. Plant Sci. 9:479. doi: 10.3389/FPLS.2018.00479
Yan, K., Wu, C., Zhang, L., and Chen, X. (2015). Contrasting photosynthesis and photoinhibition in tetraploid and its autodiploid honeysuckle (Lonicera japonica Thunb.) under salt stress. Front. Plant Sci. 6:227. doi: 10.3389/FPLS.2015.00227
Yang, C., Zhang, Z. S., Gao, H. Y., Fan, X. L., Liu, M. J., and Li, X. D. (2014). The mechanism by which NaCl treatment alleviates PSI photoinhibition under chilling-light treatment. J. Photochem. Photobiol. B Biol. 140, 286–291. doi: 10.1016/J.JPHOTOBIOL.2014.08.012
Zhang, L. T., Zhang, Z. S., Gao, H. Y., Meng, X. L., Yang, C., Liu, J. G., et al. (2012). The mitochondrial alternative oxidase pathway protects the photosynthetic apparatus against photodamage in Rumex K-1 leaves. BMC Plant Biol. 12:40. doi: 10.1186/1471-2229-12-40
Zhang, Z. S., Jia, Y. J., Gao, H. Y., Zhang, L. T., Li, H. D., and Meng, Q. W. (2011). Characterization of PSI recovery after chilling-induced photoinhibition in cucumber (Cucumis sativus L.) leaves. Planta 234, 883–889. doi: 10.1007/S00425-011-1447-3
Zhang, Z. S., Jin, L. Q., Li, Y. T., Tikkanen, M., Li, Q. M., Ai, X. Z., et al. (2016). Ultraviolet-B radiation (UV-B) relieves chilling-light-induced PSI photoinhibition and accelerates the recovery of CO2 assimilation in cucumber (Cucumis sativus L.) leaves. Sci. Rep. 6:34455. doi: 10.1038/SREP34455
Zhang, Z. S., Yang, C., Gao, H. Y., Zhang, L. T., Fan, X. L., and Liu, M. J. (2014). The higher sensitivity of PSI to ROS results in lower chilling-light tolerance of photosystems in young leaves of cucumber. J. Photochem. Photobiol. B Biol. 137, 127–134. doi: 10.1016/J.JPHOTOBIOL.2013.12.012
Zivcak, M., Brestic, M., Kalaji, H. M., and Govindjee. (2014). Photosynthetic responses of sun- and shade-grown barley leaves to high light: Is the lower PSII connectivity in shade leaves associated with protection against excess of light? Photosynth. Res. 119, 339–354. doi: 10.1007/S11120-014-9969-8
Keywords: chlorophyll fluorescence, delayed chlorophyll fluorescence, malondialdehyde, modulated 820 nm reflection, photoinhibition
Citation: Yan K, Mei H, Dong X, Zhou S, Cui J and Sun Y (2022) Dissecting photosynthetic electron transport and photosystems performance in Jerusalem artichoke (Helianthus tuberosus L.) under salt stress. Front. Plant Sci. 13:905100. doi: 10.3389/fpls.2022.905100
Received: 26 March 2022; Accepted: 06 July 2022;
Published: 27 July 2022.
Edited by:
Vasilij Goltsev, Sofia University, BulgariaReviewed by:
Grażyna Mastalerczuk, Warsaw University of Life Sciences, PolandCopyright © 2022 Yan, Mei, Dong, Zhou, Cui and Sun. This is an open-access article distributed under the terms of the Creative Commons Attribution License (CC BY). The use, distribution or reproduction in other forums is permitted, provided the original author(s) and the copyright owner(s) are credited and that the original publication in this journal is cited, in accordance with accepted academic practice. No use, distribution or reproduction is permitted which does not comply with these terms.
*Correspondence: Kun Yan, a3lhbkBsZHUuZWR1LmNu; eWFua3VuYWNhZGVtaWNAMTYzLmNvbQ==
†These authors have contributed equally to this work and share first authorship
Disclaimer: All claims expressed in this article are solely those of the authors and do not necessarily represent those of their affiliated organizations, or those of the publisher, the editors and the reviewers. Any product that may be evaluated in this article or claim that may be made by its manufacturer is not guaranteed or endorsed by the publisher.
Research integrity at Frontiers
Learn more about the work of our research integrity team to safeguard the quality of each article we publish.