- Tianjin Key Laboratory of Animal and Plant Resistance, Tianjin Normal University, Tianjin, China
Metacaspases (MCAs), a family of caspase-like proteins, are important regulators of programmed cell death (PCD) in plant defense response. Autophagy is an important regulator of PCD. This study explored the underlying mechanism of the interaction among PCD, MCAs, and autophagy and their impact on wheat response to salt stress. In this study, the wheat salt-responsive gene TaMCA-Id was identified. The open reading frame (ORF) of TaMCA-Id was 1,071 bp, coding 356 amino acids. The predicted molecular weight and isoelectric point were 38,337.03 Da and 8.45, respectively. TaMCA-Id had classic characteristics of type I MCAs domains, a typical N-terminal pro-domain rich in proline. TaMCA-Id was mainly localized in the chloroplast and exhibited nucleocytoplasmictrafficking under NaCl treatment. Increased expression of TaMCA-Id in wheat seedling roots and leaves was triggered by 150 mM NaCl treatment. Silencing of TaMCA-Id enhanced sensitivity of wheat seedlings to NaCl stress. Under NaCl stress, TaMCA-Id-silenced seedlings exhibited a reduction in activities of superoxide dismutase (SOD), peroxidase (POD), and catalase (CAT), higher accumulation of H2O2 and , more serious injury to photosystem II (PSII), increase in PCD level, and autophagy activity in leaves of wheat seedlings. These results indicated that TaMCA-Id functioned in PCD through interacting with autophagy under NaCl stress, which could be used to improve the salt tolerance of crop plants.
Introduction
As major environmental stress, salt stress impaired plant growth, especially at the seedling stage, limited crop yield, and became a danger to food security (Moukhtari et al., 2020). Salt stress harms plant growth in multiple ways, such as ion toxicity, alteration of biochemical, physiological, and metabolic processes, causing osmotic stress, water stress, nutritional disorders, genotoxicity, membrane disorganization, and inhibition of cell division (Wang et al., 2011; Yang et al., 2018; Yu et al., 2020). The plant salt-stress responses were associated with excess accumulation of reactive oxygen species (ROS) that contribute to the interrelationship among the salt-sensitive syndromes (He et al., 2020). ROS have dual functions, i.e., they can produce oxidative damage and initiate cell signaling (Mittler, 2017). Superoxide anion () and hydrogen peroxide (H2O2) are the two main ROS (Beltrán González et al., 2020). Plants have developed complicated defense mechanisms against salt stresses, containing osmotic adjustment, ion homeostasis, and ROS scavenging (Yu et al., 2020). It has reported that both programmed cell death (PCD) and autophagy regulate plant tolerance to stress conditions, which are associated with ROS accumulation (Zhou et al., 2018a; Yue et al., 2021a).
The PCD is an orderly cell suicide to eliminate unwanted or damaged cells during plant development and against multiple stresses, which is regulated by multifactors (Locato and De Gara, 2018; Yue et al., 2021a; Zhou et al., 2021). Caspases, a family of cysteine-dependent aspartate-directed proteases, are important regulators of PCD (Ojha et al., 2015). Many caspases have an active site and need aspartate residues in the protein substrates for cleavage (Julien and Wells, 2017). Although the caspases have not yet been found in plants, it has been reported that caspase-like enzymatic activity functions in many cell death pathways in plants (Luan et al., 2021). Nevertheless, metacaspase (MCA), a class of cysteine proteases present in plants, is structurally related to caspase and has caspase-like catalytic domain containing 20 kDa (p20) and 10 kDa (p10) subunits that are crucial for mediating PCD (Fagundes et al., 2015; Bansal et al., 2020). MCA includes type I and type II, according to its protein structure (Yao et al., 2020). Type I MCAs have a typical N-terminal pro-domain rich in proline, and Type II MCAs contain a linker region of 160–180 amino acids between the p20 and p10 subunits, but lack the typical N-terminal pro-domain (Fagundes et al., 2015). In the last decade, the biological functions of MCAs have been well-characterized. There were nine MCA genes in Arabidopsis (AtMCA1 to AtMCA9), which were categorized into Type I and Type II. Type I contained AtMCA1 to AtMCA3, and Type II contained AtMCA4 to AtMCA9 (Coll et al., 2010). Besides, the MCs family has been systematically characterized, and the structural and functional features of MCAs were elucidated in rice (Wang and Zhang, 2014), Viridiplantae (Fagundes et al., 2015), tomato (Liu et al., 2016b), cucumber (Zhou et al., 2018b), Rosaceae (Cao et al., 2019), Gossypium species (Fan et al., 2019), and maize (Luan et al., 2021).
Growing evidence has confirmed that MCAs functioned in signaling, stress acclimation, and PCD pathways in plants, which acted positively or negatively to mediate PCD in plant response to stress. For example, Liu et al. (2016a) indicated that many HbMCAs involved in the regulation of the drought, cold, and salt stress caused cell death. Hao et al. (2016) found that silencing TaMCA1 promoted disease resistance of wheat seedlings to Pst using virus-induced gene silencing (VIGS). Yao et al. (2020) showed that AhMCA1 positively regulated Al-induced PCD in root tips of peanut plants through inhibiting the activities of antioxidant enzymes and increasing ROS accumulation. Fernandez et al. (2021) found that both MoMCA1 and MoMCA2 played a vital role in oxidative stress-induced apoptosis and had an important role in sporulation and subsequently pathogenicity during rice blast disease progression.
Autophagy, a catabolic intracellular nutrient-scavenging pathway, has a vital role in regulating plant responses to multiple stresses (Wang et al., 2021). It has been verified that there is an intrinsic relation between autophagy and PCD in plant stress response (Yue et al., 2021a; Zhou et al., 2021). When autophagy is triggered by stress, intracellular remnants are sequestered into the double-membrane autophagosomes and subsequently delivered to the vacuoles for degradation in plants (Üstün et al., 2017). Both autophagosome formation and autophagosome-vacuole fusion required induction of a set of autophagy-related genes (ATGs) and activation of specific proteases, such as MCAs (Coll et al., 2014). Minina et al. (2013) indicated that activation of autophagy requires type II MCA mcII-Pa, and silencing of mcaII-Pa resulted in a similar result with the symptoms observed in autophagy-inhibited plants of Norway spruce, implicating that mcII-Pa was an upstream regulator of autophagy in the embryogenesis. Berenguer et al. (2020) demonstrated that both MCA and autophagy were activated and eventually dictated cell death during microspore embryogenesis under heat stress. However, the interplay among MCAs, PCD, and autophagy in wheat seedlings' response to salt stress has not been clarified. Therefore, we focused on the function of TaMCA-Id-4D in response of wheat seedlings to salt stress, which was cloned from wheat seedlings. Barley stripe mosaic virus-induced silencing (BSMV-VIGS) method was employed to acquire TaMCA-Id-silenced seedlings, which were used to explore the mechanism of interaction between autophagy and TaMCA-Id in the process of NaCl stress-triggered PCD. The results may help to clarify the molecular components involved in plant PCD and autophagy, especially under salt stress conditions.
Materials and Methods
Wheat Seedling Growth and Stress Conditions
Jimai22, a salt-tolerant variety, was acquired from the Tianjin Academy of Agricultural Sciences (China) (Dou et al., 2021). Jimai 22 is also a high-yield, multi-resistant, and high-quality wheat variety with medium gluten. Wheat seedlings were cultured by hydroponics as previously described (Yue et al., 2018, 2021a). At the two-leaf stage, healthy seedlings at the same growth rate were chosen and exposed to the same one-fourth strength Hoagland's solution added with 150 mM NaCl, and the seedlings grown in the one-fourth strength Hoagland's solution without NaCl were set as the controls. After 48 h exposure to 150 mM NaCl, the leaves and about 2 cm root tips of the control and treated group were separately collected from at least five seedlings and placed into liquid nitrogen for the extraction of RNA, which used for the following gene cloning and quantitative real-time PCR (qPCR) assay.
Cloning, Sequencing, and Phylogenetic Analysis of TaMCA-Id
Total RNA in the wheat root or leaf samples was extracted with TRIzol reagent (Invitrogen, USA). Then, reverse transcription kits (Promega, USA) were employed to reverse transcribe RNA into first-strand cDNA. The cDNA was used as a template in the following PCR assay. A unigene (accession no. TraesCS4D02G358100) released from EnsemblPlants was chosen and cloned, which was conferred to wheat seedlings' tolerance to salt stress in an RNA-Seq assay (Yue et al., 2021b). Its complete coding sequence (CDS) was amplified from the first-strand cDNA using TransStart Fast Pfu DNA polymerase (TransGen, China), with a specific pair of primers presented in Supplementary Table 1. The procedure of PCR followed the manufacturer's instructions. After poly(A) tailing, the PCR products were cloned into the vector of pGEM®-T Easy (Promega, USA) and then sequenced by Sangon Biotech (Shanghai, China). The molecular weight (Mw) and theoretical isoelectric point (pI) of the putative TaMCA-Id were assessed using ExPASy software (http://www.expasy.org/). The homology search proteins using the deduced amino acid sequence of TaMCA-Id were carried out according to BLASTp program of the National Center for Biotechnology Information (NCBI) (http://www.ncbi.nlm.nih.gov/). The multiple sequence alignment was conducted with Bioedit software. The functional and conserved domains were predicted with Smart software (http://smart.embl-heidelberg.de/smart/set_mode.cgi). The maximum likelihood phylogenetic tree was built with MEGA-X software.
Subcellular Localization of TaMCA-Id
The open reading frame of TaMCA-Id without termination codon was amplified from the first-strand cDNA or vector of pGEM®-T Easy-TaMC1. Then, the amplification products were digested with XbaI and BamHI and were subcloned into the pAN583:mCherry vector with pEASY®-Basic Seamless Cloning and Assembly Kit (TransGen Biotech, China), generating the TaMC1-mCherry fusion construct pAN583:TaMC1-mCherry. Then, the pAN583:TaMC1-mCherry fusion construct and pAN583:mCherry (control) were transferred into wheat protoplasts, respectively, with PEG-mediated transformation, as described by Li et al. (2018). After incubated for 20 h at 25°C, the mCherry signals in the transformed wheat protoplast were detected with a confocal laser scanning microscope (Nikon Ti2 Eclipse A1). The primers were presented in Supplementary Table 1.
BSMV-VIGS Assay
A cDNA fragment of 170 bp (+512 bp to +680 bp) was employed to acquire TaMCA-Id silenced vector. After this fragment was inserted in BSMVγ plasmid (BSMVγ:TaMCA-Id), the plasmids of BSMVα, BSMVγ:GFP, and BSMVγ:TaMCA-Id were linearized with Mlu I (Takala, Dalian, China). BSMVβ was linearized with Spe I (Takala, Dalian, China). Then, RiboMAX largeScale RNA Production-T7 kit (Promega, USA) was used to in vitro transcribe these linearized plasmids. Ribom 7G Cap Analog (Promega, USA) was employed to produce the 5′-capped BSMV RNA molecules for following BSMV-VIGS inoculated experiments. These experiments (containing vector construction, in vitro transcription, BSMV-VIGS inoculation, TaMCA-Id-silenced seedlings identification, and silence efficiency assessment of TaMCA-Id were conducted as previously described (Yue et al., 2021a). For salt stress, part of the BSMV-VIGS-incubated seedlings with three expanded leaves were exposed to 150 mM NaCl treatment for 6 days. All experiments were repeated at least three times, and 20 seedlings were treated for each repeat. The used primes are present in Supplementary Table 1.
Physiological Measurements and Histochemical Staining
After 150 mM NaCl was stressed for 6 days, the control and TaMCA-Id-silenced seedlings were used to carry out the following experiment. The in situ generation of H2O2 and in wheat leaves was detected using 3,3-diaminobenzidine (DAB) and nitro blue tetrazolium (NBT), respectively. The staining procedures were carried out as previously described (Yue et al., 2021a). The quantitative determination of H2O2 and was performed following a previous study (Yue et al., 2021b). Evans blue staining was carried out to visualize the degree of cell damage in the wheat leaves under NaCl treatment. The leaves samples were immersed in 0.25% Evans blue staining solution and stained for 24 h in darkness. Then, the tissues were rinsed with decolorization buffer (absolute ethanol and glycerol, 9:1, v/v) until they became colorless. Then, the leaves were photographed by stereoscopic microscope (Nikon C-fled2). Monodansylcadaverine (MDC) was used to label autophagosomes (Khalid et al., 2019). The observation of autophagosomes in wheat leaves was carried out according to our previous research (Yue et al., 2021a).
The activities of peroxidase (POD), superoxide dismutase (SOD), and catalase (CAT) were analyzed as described by Yue et al. (2021a). For determination of the caspase-3 activity, the leaf tissues (0.1 g) were ground with liquid nitrogen and then were immersed in the lysis buffer (150–200 μl) according to the Caspase-3 Colorimetric Assay Kit manufacturer's guidance (Cat#: KGA202, KeyGEN BioTECH, China). Then, homogenate collection and detection of the supernatant protein concentration and determination of caspase-3-like activity were performed following the manufacturer's instructions.
qPCR Assay
Total RNA extraction of the leaf samples, first-strand cDNA transcription, and qPCR assay were conducted as previously described (Yue et al., 2018, 2021a). The involved primers are present in Supplementary Table 1.
Determination of Chlorophyll Fluorescence Parameters
The representative chlorophyll fluorescence parameters, containing PSII photochemistry (Fv/Fm), quantum yield of PSII (Y(II)), quenching coefficient (qP), electron transfer rate (ETR), and non-photochemical quenching coefficient (NPQ), were examined according to our previous study (Yue et al., 2021a).
Statistical Analyses
Every experiment was performed at least three repetitions. The data were present as mean ± SD. All statistical analyses were carried out according to Duncan's multiple range tests with SPSS software. P < 0.05 indicated significant differences.
Results
Cloning and Characterization of the Wheat MC Gene TaMCA-Id
After amplification, the complete coding sequence (CDS) of the salt-responsive unigene (accession no. TraesCS4D02G358100) was acquired. Sequence analysis showed that the open reading frame TaMCA-Id was 1,071 bp in length, which encoded 356 amino acid residues with molecular weight and isoelectric point of 38,337.03 Da and 8.45, respectively. It was located on chromosome 4D. According to Chen et al. (2020), TaMCA-Id was used as a probe to search for homologs in the Triticeae-GeneTribe database. TraesCS5A02G548200, located on chromosome 5A, TraesCS4B02G381800, located on the chromosome 4B, and TraesCS4D02G358800, located on the chromosome 4D, were identified as TaMCA-Id homologs in wheat. They encode peptide sequences of 299, 352, and 346 amino acids, respectively. The amino acid residues of the above three homologs and TaMCA-Id share identities of 85–94% (Supplementary Figure 1). TaMCA-Id protein had a putative conserved Peptidase_C14 domain with caspase-like catalytic activity (Supplementary Figure 2). Multi-sequence alignments of TaMCA-Id sequence and nine MCs of Arabidopsis thaliana indicated that TaMCA-Id contained a typical N-terminal pro-domain that was a specific characteristic of type I MCs, and there was also a linker region between the p20 and p10 subunits that was a specific property of Type II MCs (Figure 1A). However, the phylogenetic tree revealed that the phylogenetic relationship of TaMCA-Id was related to AtMCA1, which belonged to type I MCAs (Figure 1B). Based on the above bioinformatics analysis and Minina et al. (2020) study, the amplified gene was eventually named TaMCA-Id.
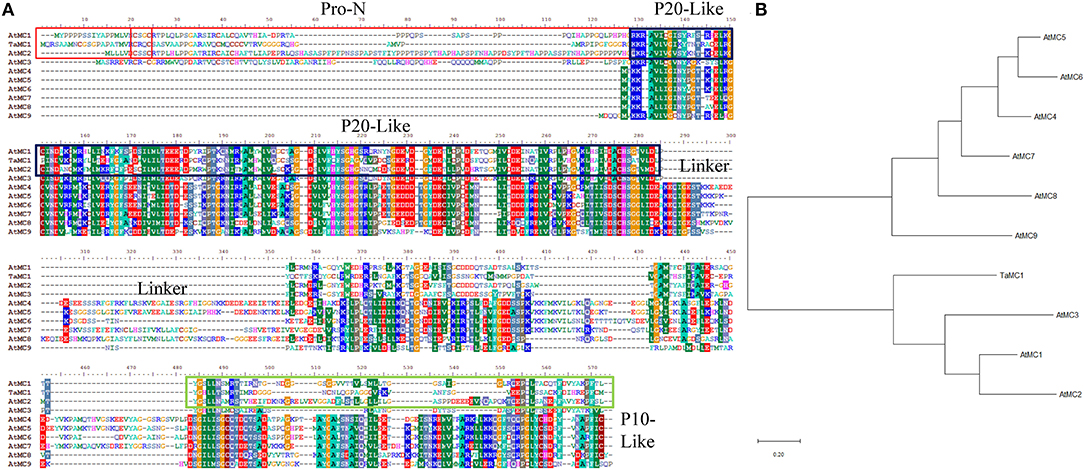
Figure 1. Sequence analysis of TaMCA-Id. (A) Multi-sequence alignment of TaMCA-Id with other metacaspase proteins from Arabidopsis. Amino acid sequences from 10 metacaspase share similar structures, containing Pro-N domain, P20-like domain, Linker, and P10-like domain. Ta, Triticum aestivum L.; At, Arabidopsis thaliana. (B) Phylogenetic analysis of TaMCA-Id. TaMCA-Id and function-known metacaspase in Arabidopsis thaliana (At) are used to construct their phylogenetic tree using MEGA-X software. TaMC1 in the figure indicates TaMCA-Id. AtMC1 to AtMC9 in the figure indicate AtMCA1 to AtMCA9, respectively.
TaMCA-Id Responds to NaCl Treatment
qPCR assays were conducted to investigate the expression response of TaMCA-Id to a time-series NaCl treatment in wheat seedlings. The result indicated that TaMCA-Id expression in the roots and leaves was significantly upregulated under NaCl treatment (Figure 2). The results proved that TaMCA-Id was involved in the NaCl stress response of wheat seedlings.
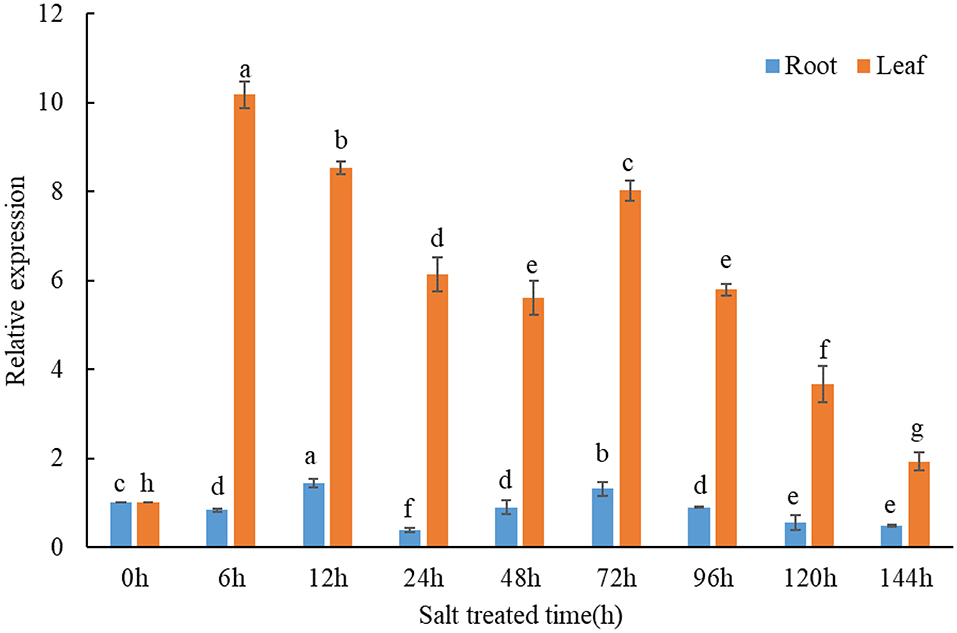
Figure 2. Transcript levels of TaMCA-Id in roots and leaves of wheat seedlings under NaCl treatment using qPCR assay. Data are presented as mean ± SD from at least three independent experiments. Bars with different letters are significantly different at P < 0.05.
Subcellular Localization of TaMCA-Id
mCherry and TaMCA-Id-mCherry were each expressed in wheat mesophyll protoplasts to investigate the localization of TaMCA-Id. As shown in Figure 3, under normal conditions for the maintenance of transfected protoplasts, the fluorescence signals of mCherry spread in the whole cell, including the nucleus, while those of TaMCA-Id-mCherry mainly spread in the chloroplast. Under NaCl treatment, some of this TaMCA-Id-mCherry fusion protein was tracked in the nucleus or in the cytoplasm (Figure 3). These features may ensure that TaMCA-Id functions effectively in signal transduction under NaCl stress in wheat seedling leaves.
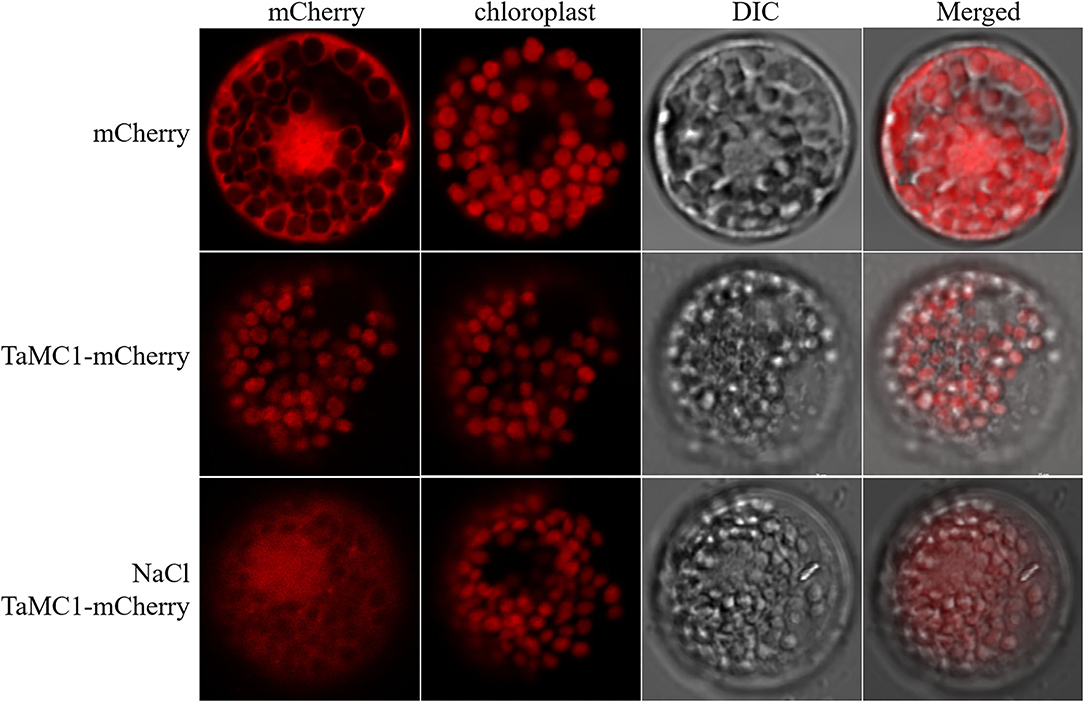
Figure 3. Subcellular localization of TaMCA-Id. Wheat protoplasts were transformed with pAN583:mCherry or GFP:mCherry via the polyethylene glycol (PEG)-mediated method. TaMCA-Id was mainly localized to the chloroplast in wheat protoplasts, and exhibited nucleocytoplasmictrafficking under NaCl treatment. TaMC1 in the figure indicates TaMCA-Id.
Silencing of TaMCA-Id Increases Salt Sensitivity of Wheat Seedlings
To better investigate the function of TaMCA-Id in wheat response to NaCl stress, we studied TaMCA-Id loss of function in wheat seedlings by conducting BSMV-VIGS-inoculated experiment. After the third and newly grown leaves of the BSMV-VIGS-inoculated seedlings showed virus infection-induced chlorosis, at least three seedlings were randomly selected to examine the silencing efficiency of TaMCA-Id. Compared with BSMV-VIGS-GFP-inoculated seedlings, the expression of TaMCA-Id was significantly suppressed in the leaves of TaMCA-Id-silenced seedlings (Supplementary Figure 3).
Under non-stressed conditions, TaMCA-Id silencing slightly affect root length and the third leaf length of wheat seedlings, while BSMV-VIGS inoculation produced a little decrease in the root length and the third leaf length compared with that in the wide-type (WT) seedlings (Supplementary Table 2). After exposure to NaCl treatment for 6 days, silencing of TaMCA-Id aggravated the NaCl stress-induced damage to wheat seedling growth (Supplementary Figure 4), with shorter lengths of both roots and the third leaves (Supplementary Table 2). The root length of TaMCA-Id-silenced seedlings and BMSV-VIGS-GFP-inoculated seedlings was decreased by 29% and 24% as compared with the controls, respectively. The leaf length of TaMCA-Id-silenced seedlings and BMSV-VIGS-GFP-inoculated seedlings was decreased by 28% and 26% as compared with the controls, respectively. The above results suggested that silencing of TaMCA-Id increased wheat seedling sensitivity to NaCl treatment.
Silencing of TaMCA-Id Enhances the Autophagy Activity in Wheat Leaves at the Seedling Stage Under NaCl Treatment
After exposure to NaCl treatment for 6 days, silencing of TaMCA-Id promoted autophagy in wheat seedling leaves, with upregulation of the key ATGs, including ATG2, ATG5, ATG7, ATG8, and ATG10 (Figure 4), and obvious increase of autophagosomes in wheat leaves (Supplementary Figure 5), as compared with those in the BSMV-VIGS-GFP-inoculated seedlings and the WT seedlings. These results implied that there was an interaction between autophagy and TaMCA-Id silencing in the regulating NaCl stress response of wheat seedlings.
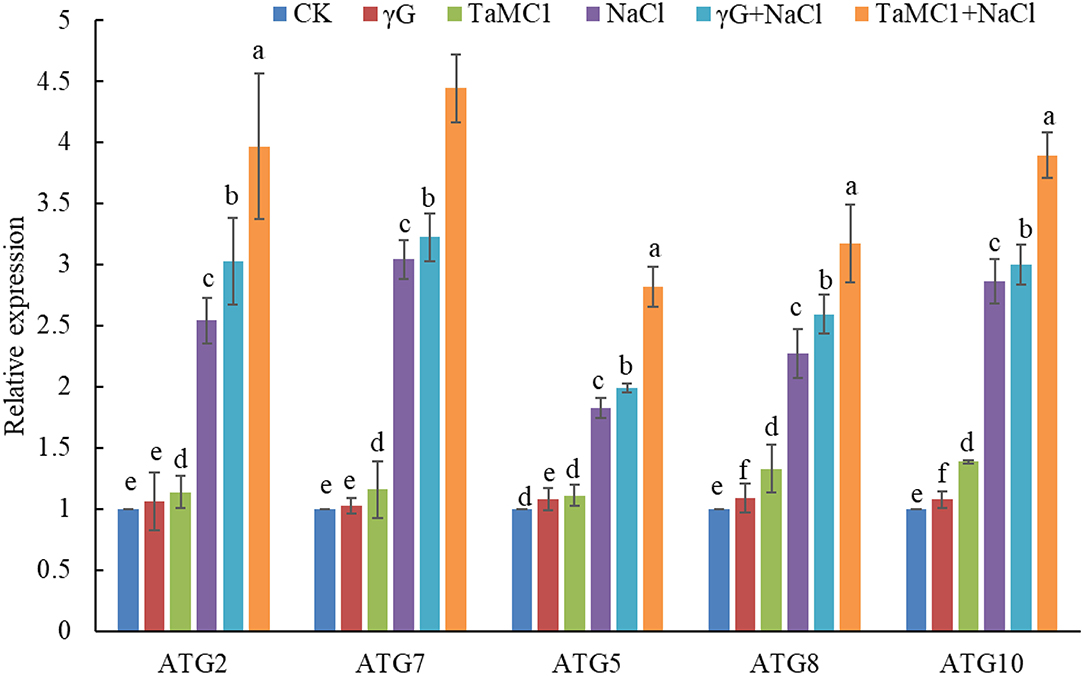
Figure 4. The effect of silencing of TaMCA-Id on the relative expression analysis of key ATGs in leaves of wheat seedlings under NaCl stress. CK was the wild-type wheat seedlings. γG was the BSMV-VIGS-GFP- inoculated wheat seedlings. TaMC1 was the BSMV-VIGS-TaMCA-Id- inoculated wheat seedlings. Data are shown as mean ± SD (n = 3) of three independent experiments. The different letters in each treatment show the significant difference (P < 0.05).
Silencing of TaMCA-Id Promotes NaCl Stress-Triggered PCD in Leaves of Wheat Seedlings
To further investigate whether TaMCA-Id silencing was involved in the cell death caused by NaCl treatment, Evans blue was used to in situ stain the wheat seedling leaves. The results showed that the cell death caused by NaCl treatment was obvious in wheat seedling leaves, and silencing TaMCA-Id aggravated NaCl stress-induced cell death in the leaves of wheat seedlings (Figure 5A). TUNEL assay was performed to determine whether PCD was involved in wheat response to NaCl stress. The results showed that NaCl treatment enhanced the ratio of TUNEL-positive PCD cells in wheat seedling leaves. Silencing of TaMCA-Id further elevated the NaCl stress-induced PCD ratio in wheat seedling leaves (Figure 5B). These results implied that TaMCA-Id had a significant regulatory effect on the PCD level in wheat seedling leaves under NaCl treatment.
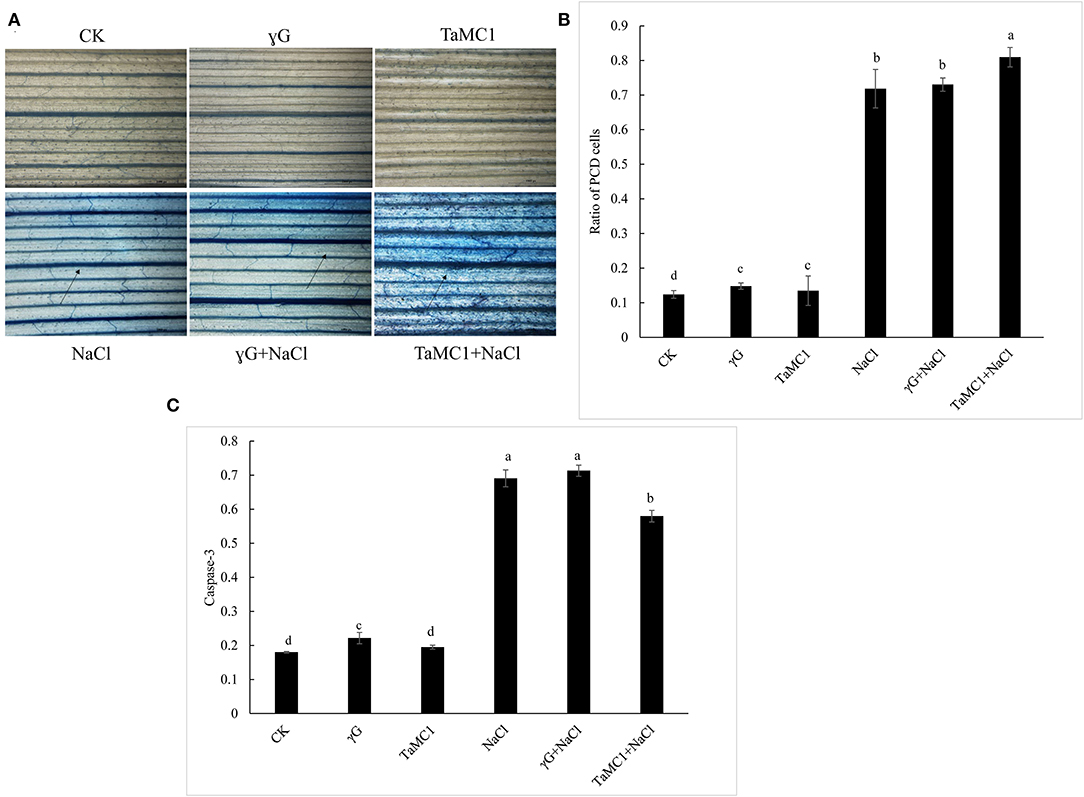
Figure 5. The effect of silencing of TaMCA-Id on cell death in leaves of wheat seedlings under NaCl stress. CK was the wild-type wheat seedlings. γG was the BSMV-VIGS-GFP- inoculated wheat seedlings. TaMC1 was the BSMV-VIGS-TaMCA-Id- inoculated wheat seedlings. (A) Evans blue staining results. (B) The ratio of PCD cells in wheat leaves determined with Tunel assays. Standard deviations are shown (n > 3, ±SD, P < 0.05). The different letters in each treatment show the significant difference (P < 0.05). (C) The effect of silencing of TaMCA-Id on the caspase-3-like activity in leaves of wheat seedlings under NaCl stress. Standard deviations are shown (n > 3, ±SD, P < 0.05). The different letters in each treatment show the significant difference (P < 0.05).
Colorimetric experiment was employed to detect whether TaMCA-Id functioned in regulating caspase-3-like activity in wheat seedling leaves under NaCl treatment. After exposure to NaCl treatment for 6 days, silencing of TaMCA-Id triggered a higher NaCl stress-induced caspase 3-like activity in wheat seedlings leaves, indicating that TaMCA-Id probably acted as a regulator of caspase 3-like activity (Figure 5C). Taken together, these results suggested that TaMCA-Id functioned in promoting PCD in wheat leaves at the seedling stage under the treatment of NaCl.
Silencing of TaMCA-Id Reduces the Antioxidant Capacity of Wheat Seedling Leaves Under NaCl Treatment
To study the influence of TaMCA-Id on the antioxidant capacity of wheat leaves under NaCl treatment, the ROS levels and the activities of some antioxidant enzymes were monitored. Under non-stress conditions, there were no obvious changes in the accumulation of and H2O2 between BSMV-VIGS-GFP-inoculated and WT seedlings. NaCl stress significantly elevated the production of and H2O2 in the wheat leaves. Silencing of TaMCA-Id significantly kept the content of H2O2 and in wheat seedling leaves at higher levels under NaCl treatment (Figure 6A). The quantitative analysis results of H2O2 and concurred with the staining results (Figure 6B). After 6 days of NaCl treatment, the activities of POD, SOD, and CAT in wheat seedlings were elevated. Silencing of TaMCA-Id decreased the activities of POD, SOD, and CAT in the wheat leaves under NaCl treatment (Figure 6C). These results indicated that the TaMCA-Id silencing promoted and H2O2 generation in wheat seedling leaves by impairing antioxidant capacity under NaCl treatment.
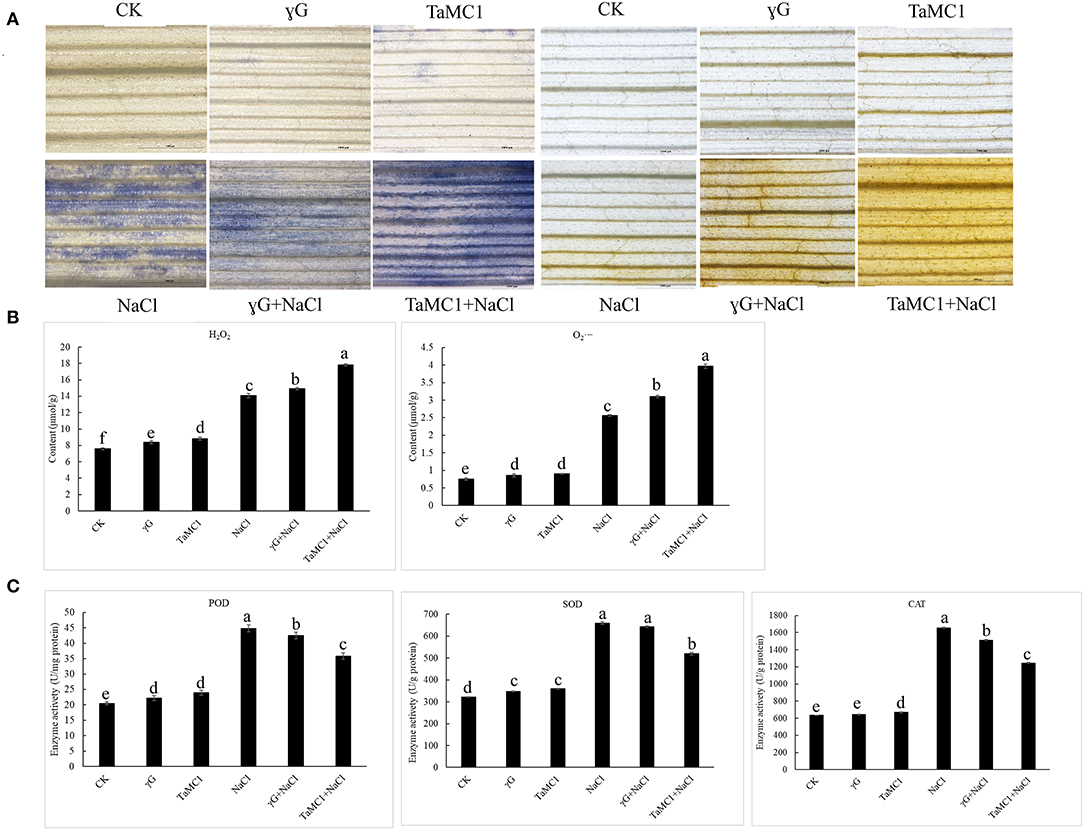
Figure 6. The effect of silencing of TaMCA-Id on the ROS accumulation in leaves of wheat seedlings under NaCl stress. CK was the wild-type wheat seedlings. γG was the BSMV-VIGS-GFP- inoculated wheat seedlings. TaMC1 was the BSMV-VIGS-TaMCA-Id- inoculated wheat seedlings. (A) NBT staining of the generation and accumulation of in leaves of wheat seedlings, and DAB staining of the generation and accumulation of H2O2 in leaves of wheat seedlings. (B) The content of and H2O2 in leaves of wheat seedlings. (C) The activities of POD, SOD, and CAT in leaves of wheat seedlings. Standard deviations are shown (n > 3, ± SD, P < 0.05). All the experiments presented in this study were performed at least three times, and similar results were obtained.
Silencing of TaMCA-Id Aggravates the NaCl Stress-Induced Injury of Photosystem II (PSII)
Compared with the control, NaCl stress significantly decreased Fv/Fm, Y(II), qP, and ETR and increased NPQ of wheat seedlings. After 6 days of NaCl treatment, silencing of TaMCA-Id further decreased Fv/Fm, Y(II), qP, and ETR and increased NPQ of wheat seedlings compared with those of the BSMV-VIGS-GFP-inoculated and WT seedlings (Table 1). These results indicated that the inhibition of TaMCA-Id expression aggravated NaCl treatment-induced PSII injury in wheat seedlings.
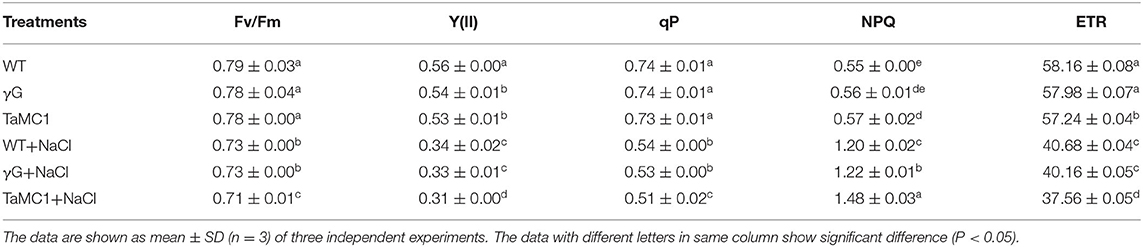
Table 1. The changes in chlorophyll fluorescence parameters in leaves of BSMV-VIGS-GFP- (γG), BSMV-VIGS-TaMCA-Id- (TaMC1)-inoculated wheat seedlings and the wild-type seedlings (WT) under NaCl stress.
Discussion
Salinity stress has a damaging impact on plant growth and threatens agronomical production (Signorelli et al., 2019). To counteract this, plants have developed many strategies for plant adaptability to salt stress. Among them, both autophagy and PCD are evolutionarily conserved strategies for plants to resist various environmental stresses (Chen et al., 2021; Ren et al., 2021). Crosstalk between PCD and autophagy as a whole at “process level” is very complex, which is regulated by cysteine proteases (animal caspases and plant MCAs; Minina et al., 2014). Under stress conditions, as a pro-survival mechanism, autophagy is always initiated to combat PCD via eliminating excess ROS-triggered oxidative damage and being conducive to cell survival (Yue et al., 2021a). For example, Zhou et al. (2018) indicated that tobacco mosaic virus (TMV) local inoculation activated PCD and autophagy in tomatoes, and ROS was the signaling molecule that promoted autophagy in root-tip cells of the tomato plant. Under waterlogging stress, autophagy can be induced to inhibit PCD, which maintained wheat seedlings' survival, with the increased activities of MCAs and caspase-like protease (Zhou et al., 2021). Our previous study confirmed that autophagy was induced by NaCl stress to improve the adaptability to NaCl treatment in wheat seedlings via negatively regulated salt-induced PCD. This process was accompanied with differential expression of TaMCA-Id in leaves of wheat seedlings, and silencing of ATG2 or ATG7 triggered higher or lower expression of different TaMCA-Id and significantly promoted PCD in wheat roots and leaves at the seedling stage under NaCl treatment (Yue et al., 2021a). These results suggest that there is an interplay between autophagy and PCD, which ensures the tight regulation of cellular homeostasis under stress conditions, and MCAs function in regulating the autophagy-PCD crosstalk induced by stress treatment. But the underlying regulating mechanisms are less clear.
The MCAs act to regulate plant growth, development, and multiple stress responses (Gong et al., 2020; Roohollah et al., 2020). This study acquired a novel TaMCA-Id that had typical characteristics of type I MCA family, which could contribute to the regulation of NaCl-caused PCD and activity of caspase 3-like proteases in wheat seedlings. The subcellular localization of TaMCA-Id was mainly in the chloroplast and was transferred from chloroplast to cytoplasm and nucleus induced by NaCl stress. The result was consistent with Castillo-Olamendi et al. (2008), which showed that an AtMCP1b was localized in the chloroplasts. Hao et al. (2016) suggested that Type I TaMCA1 was localized in the cytoplasm and mitochondria. Interestingly, Arabidopsis MCA9 was located in the apoplast, nucleus, and cytoplasm, and its subcellular localization was altered in the late autolysis process (Vercammen et al., 2004, 2006). In this study, the TaMCA-Id nucleocytoplasmictrafficking under NaCl stress might be important for PCD induction. The above results suggest that MCAs localize in different cellular compartments implying their functional diversification.
Consequently, we suggested that TaMCA-Id might play a vital role in the NaCl stress response of wheat seedlings, as a positive cell death regulator. We further carried out a knockdown of TaMCA-Id to confirm its function in the NaCl stress response of wheat seedlings. Chlorophyll fluorescence reflects PSII activity, which represents plant damage (He et al., 2018; Goussi et al., 2018). We found that silencing of TaMCA-Id damaged PSII and promoted NaCl-induced PCD in wheat seedling leaves under NaCl treatment. The result was consistent with a previous study that suggested TaMCA1 could suppress cell death caused by the mouse Bax gene in N. benthamiana and wheat leaves, and it could be a negative cell death regulator function in regulating wheat-Pst interaction (Hao et al., 2016). However, this result is different from the results that AhMCA1 positively regulated PCD in peanut root tips triggered by Al treatment (Yao et al., 2020). Interestingly, silencing of TaMCA-Id also promoted NaCl-induced autophagy in the leaves of wheat seedlings. A similar phenomenon was observed in Arabidopsis vascular xylem differentiation, and the vascular tracheary elements (TE) cell type presented even higher autophagic activity triggered by the inhibition of the TE-specific MCA9 using RNAi, which was detrimental to the surrounding cells (Escamez et al., 2016). These results implied that TaMCA-Id might function prior to cell death. Notably, our previous study suggested that ATG2- or ATG7-suppressed autophagy led to the upregulation of TaMCA-Id in wheat leaves at the seedling stage under NaCl treatment (Yue et al., 2021a). Taken together, these results showed that autophagy was activated by the inhibition of TaMCA-Id expression, which could initially maintain cellular homeostasis under NaCl treatment, through removing and recycling damaged proteins and organelles. There is a complex interaction between autophagy and PCD. They were activated together and had mutual regulation relationship under NaCl stress. During NaCl stress, autophagy to a certain extent could inhibit PCD, and the increase of PCD level with the treatment time extended also could lead to enhanced autophagy and eventually follow autophagic cell death, which is a non-apoptotic form of PCD, due to stress-induced nutrient deficiency.
Salt stress leads to the ROS burst in plants, which is a necessary event in plants' salt stress response (Zhang et al., 2019). A growing number of evidence indicated the function of ROS in the upstream of autophagy and PCD (Demidchik et al., 2018; Zhou et al., 2018a; Wang et al., 2020). Similarly, our study found that silencing of TaMCA-Id further enhanced ROS production induced by NaCl treatment in wheat leaves at the seedling stage. Eventually, the excess ROS might promote autophagy and PCD in leaves of wheat seedlings under NaCl treatment. Thus, we deduced that TaMCA-Id could contribute to the regulation of autophagy and PCD via ROS levels. Plants have invoked the antioxidant defense system, including SOD, CAT, POD, and other enzymes, to prevent oxidative damage (Buttar et al., 2020). Our results showed that silencing of TaMCA-Id suppressed the antioxidant defensive response to destroy the ROS scavenging system and thus resulted in excess ROS accumulation under NaCl treatment. The transcriptional levels of cell death- and defense-related genes that determine cell fate under NaCl treatment, including calcium-dependent protein kinases (CDPKs), containing CDPK21 and CDPK27, cysteine-rich receptor-like protein kinases (CRKs), containing CRK10 and CRK26, glyceraldehyde-3-phosphate dehydrogenase B (GAPD), MYB, NAC, cytochrome P450 94A2-like (CYP94A2), and respiratory burst oxidase homolog protein B-like (Rboh-like), were also changed in the leaves of TaMCA-Id-silenced seedlings compared with that in control seedlings (Supplementary Figure 6).
Conclusion
Autophagy and PCD are the salt tolerance strategies employed by wheat seedlings. They controlled the cellular homeostasis through eliminating damaged macromolecules and organelle, or unnecessary cells under NaCl stress. TaMCA-Id is mainly localized in the chloroplast and exhibits nucleocytoplasmictrafficking under NaCl stress. TaMCA-Id would be a regulator of stress-induced cell death through regulating ROS-induced autophagy. In a word, silencing of TaMCA-Id was able to reduce NaCl tolerance of wheat seedlings via promoting ROS generation which further took part in the regulation of autophagy and PCD triggered by NaCl treatment. This study suggests that there is an interplay between autophagy and PCD, which ensures the tight regulation of cellular homeostasis under stress conditions, and MCAs function in regulating the autophagy-PCD crosstalk induced by stress treatment.
Data Availability Statement
The raw data supporting the conclusions of this article will be made available by the authors, without undue reservation.
Author Contributions
J-yY: methodology, validation, formal analysis, writing (original draft), and funding acquisition. Y-jW: methodology, validation, and software. J-lJ and W-wW: visualization and software. H-zW: writing, reviewing, and editing the manuscript and supervision. All authors contributed to the article and approved the submitted version.
Funding
This study was supported by the National Science Foundation of China (Nos. 31501234 and 31971829) and the Youth Talent Support Program of Tianjin Normal University (043135202RC1702).
Conflict of Interest
The authors declare that the research was conducted in the absence of any commercial or financial relationships that could be construed as a potential conflict of interest.
Publisher's Note
All claims expressed in this article are solely those of the authors and do not necessarily represent those of their affiliated organizations, or those of the publisher, the editors and the reviewers. Any product that may be evaluated in this article, or claim that may be made by its manufacturer, is not guaranteed or endorsed by the publisher.
Acknowledgments
We thank the referees for their helpful comments and the other members of our laboratory for their help with the research and for their insightful remarks.
Supplementary Material
The Supplementary Material for this article can be found online at: https://www.frontiersin.org/articles/10.3389/fpls.2022.904933/full#supplementary-material
Abbreviations
MCAs, Metacaspases; PCD, programmed cell death; ATG, autophagy-related gene; BSMV-VIGS, barley stripe mosaic virus-induced silencing; VIGS, virus-induced gene silencing; ROS, reactive oxygen species; qPCR, quantitative real-time PCR; CDS, complete coding sequence; NCBI, National Center for Biotechnology Information; TMV, tobacco mosaic virus; TE, tracheary elements; O, superoxide anion; H2O2, hydrogen peroxide; DAB, 3, 3-diaminobenzidine; NBT, nitro blue tetrazolium; SOD, superoxide dismutase; POD, peroxidase; CAT, catalase; PSII, photosystem II; Fv/Fm, PSII photochemistry; Y(II), quantum yield of PSII; qP, quenching coefficient; ETR, electron transfer rate; NPQ, non-photochemical quenching coefficient; qRT-PCR, real-time quantitative PCR; CDPKs, calcium-dependent protein kinases; CRK, cysteine-rich receptor-like kinase; GAPD, glyceraldehyde-3-phosphate dehydrogenase B; CYP94A2, cytochrome P450 94A2-like; Rboh-like, respiratory burst oxidase homolog protein B-like.
References
Bansal, R., Rana, N., Singh, A., Dhiman, P., Mandlik, R., Sonah, H., et al. (2020). Evolutionary understanding of metacaspase genes in cultivated and wild Oryza species and its role in disease resistance mechanism in rice. Genes 11:1412. doi: 10.3390/genes11121412
Beltrán González, A. N., López Pazos, M. I., and Calvo, D. J. (2020). Reactive oxygen species in the regulation of the GABA mediated inhibitory neurotransmission. Neuroscience 439, 137–145. doi: 10.1016/j.neuroscience.2019.05.064
Berenguer, E., Minina, E. A., Carneros, E., Bárány, I., Bozhkov, P. V., and Testillano, P. S. (2020). Suppression of metacaspase- and autophagy-dependent cell death improves stress-induced microspore embryogenesis in Brassica napus. Plant Cell Physiol. 61, 2097–2110. doi: 10.1093/pcp/pcaa128
Buttar, Z. A., Wu, S. N., Arnao, M. B., Wang, C., Ullah, I., and Wang, C. (2020). Melatonin suppressed the heat stress-induced damage in wheat seedlings by modulating the antioxidant machinery. Plants 9:809. doi: 10.3390/plants9070809
Cao, Y., Meng, D., Chen, T., Chen, Y., Zeng, W., Zhang, L., et al. (2019). Metacaspase gene family in Rosaceae genomes: comparative genomic analysis and their expression during pear pollen tube and fruit development. PLoS ONE 14:e0211635. doi: 10.1371/journal.pone.0211635
Castillo-Olamendi, L., Bravo-Garcìa, A., Morán, J., Rocha-Sosa, M., and Porta, H. (2008). AtMCP1b, a chloroplast-localised metacaspase, is induced in vascular tissue after wounding or pathogen infection. Funct. Plant Biol. 34, 1061–1071. doi: 10.1071/FP07153
Chen, H., Dong, J., and Wang, T. (2021). Autophagy in plant abiotic stress management. Int. J. Mol. Sci. 22:4075. doi: 10.3390/ijms22084075
Chen, Y., Song, W., Xie, X., Wang, Z., Guan, P., Peng, H., et al. (2020). A collinearity-incorporating homology inference strategy for connecting emerging assemblies in triticeae tribe as a pilot practice in the plant pangenomic era. Mol. Plant 13, 1694–1708. doi: 10.1016/j.molp.2020.09.019
Coll, N., Smidler, A., Puigvert, M., Popa, C., Valls, M., and Dangl, J. L. (2014). The plant metacaspase AtMC1 in pathogen-triggered programmed cell death and aging: functional linkage with autophagy. Cell Death Differ. 21, 1399–1408. doi: 10.1038/cdd.2014.50
Coll, N. S., Vercammen, D., Smidler, A., Clover, C., Van Breusegem, F., Dangl, J. L., et al. (2010). Arabidopsis type I metacaspases control cell death. Science 330, 1393–1397. doi: 10.1126/science.1194980
Demidchik, V., Tyutereva, E. V., and Voitsekhovskaja, O. V. (2018). The role of ion disequilibrium in induction of root cell death and autophagy by environmental stresses. Funct. Plant Biol. 45, 28–46. doi: 10.1071/FP16380
Dou, X. T., Wang, Y. J., Wang, H. Z., and Yue, J. Y. (2021). Physiological response and tolerance difference of two wheat varieties to NaCl stress. Acta Ecol. Sin. 41, 4976-4992. doi: 10.5846/stxb202005251330
Escamez, S., André, D., Zhang, B., Bollhöner, B., Pesquet, E., and Tuominen, H. (2016). METACASPASE9 modulates autophagy to confine cell death to the target cells during Arabidopsis vascular xylem differentiation. Biol. Open 5, 122–129. doi: 10.1242/bio.015529
Fagundes, D., Bohn, B., Cabreira, C., Leipelt, F., Dias, N., Bodanese-Zanettini, M. H., et al. (2015). Caspases in plants: metacaspase gene family in plant stress responses. Funct. Integr. Genom. 15, 639–649. doi: 10.1007/s10142-015-0459-7
Fan, S., Liu, A., Zhang, Z., Zou, X., Jiang, X., Huang, J., et al. (2019). Genome-wide identification and expression analysis of the metacaspase gene family in Gossypium Species. Genes 10:527. doi: 10.3390/genes10070527
Fernandez, J., Lopez, V., Kinch, L., Pfeifer, M. A., Gray, H., Garcia, N., et al. (2021). Role of two metacaspases in development and pathogenicity of the rice blast fungus Magnaporthe oryzae. mBio 12, e03471–e03420. doi: 10.1128/mBio.03471-20
Gong, X., Xie, Z., Qi, K., Zhao, L., Yuan, Y., Xu, J., et al. (2020). PbMC1a/1b regulates lignification during stone cell development in pear (Pyrus bretschneideri) fruit. Hortic Res 7:59. doi: 10.1038/s41438-020-0280-x
Goussi, R., Manaa, A., Derbali, W., Cantamessa, S., Abdelly, C., and Barbato, R. (2018). Comparative analysis of salt stress, duration and intensity, on the chloroplast ultrastructure and photosynthetic apparatus in Thellungiella salsuginea. J. Photochem. Photobiol. B 183, 275–287. doi: 10.1016/j.jphotobiol.2018.04.047
Hao, Y., Wang, X., Wang, K., Li, H., Duan, X., Tang, C., et al. (2016). TaMCA1, a regulator of cell death, is important for the interaction between wheat and Puccinia striiformis. Sci. Rep. 6:26946. doi: 10.1038/srep26946
He, F., Niu, M. X., Feng, C. H., Li, H. G., Su, Y., Su, W. L., et al. (2020). PeSTZ1 confers salt stress tolerance by scavenging the accumulation of ROS through regulating the expression of PeZAT12 and PeAPX2 in Populus. Tree Physiol. 40, 1292–1311. doi: 10.1093/treephys/tpaa050
He, L., Yu, L., Li, B., Du, N., and Guo, S. (2018). The effect of exogenous calcium on cucumber fruit quality, photosynthesis, chlorophyll fluorescence, and fast chlorophyll fluorescence during the fruiting period under hypoxic stress. BMC Plant Biol. 18:180. doi: 10.1186/s12870-018-1393-3
Julien, O., and Wells, J. (2017). Caspases and their substrates. Cell Death Differ. 24, 1380–1389. doi: 10.1038/cdd.2017.44
Khalid, A. R., Lv, X., Naeem, M., Mehmood, K., Shaheen, H., Dong, P., et al. (2019). Autophagy related gene (ATG3) is a key regulator for cell growth, development, and virulence of Fusarium oxysporum. Genes 10:658. doi: 10.3390/genes10090658
Li, K., Liu, Y., Yu, B., Yang, W., Yue, J., and Wang, H. (2018). Monitoring autophagy in wheat living cells by visualization of fluorescence protein-tagged ATG8. Plant Cell Tiss. Organ. Cult. 134, 481–489. doi: 10.1007/s11240-018-1437-2
Liu, H., Deng, Z., Chen, J., Wang, S., Hao, L., and Li, D. (2016a). Genome-wide identification and expression analysis of the metacaspase gene family in Hevea brasiliensis. Plant Physiol. Biochem. 105, 90–101. doi: 10.1016/j.plaphy.2016.04.011
Liu, H., Liu, J., and Wei, Y. (2016b). Identification and analysis of the metacaspase gene family in tomato. Biochem. Biophys. Res. Commun. 479, 523–529. doi: 10.1016/j.bbrc.2016.09.103
Locato, V., and De Gara, L. (2018). Programmed cell death in plants: an overview. Methods Mol. Biol. 1743, 1–8. doi: 10.1007/978-1-4939-7668-3_1
Luan, Q. L., Zhu, Y. X., Ma, S., Sun, Y., Liu, X. Y., Liu, M., et al. (2021). Maize metacaspases modulate the defense response mediated by the NLR protein Rp1-D21 likely by affecting its subcellular localization. Plant J. 105, 151–166. doi: 10.1111/tpj.15047
Minina, E. A., Filonova, L. H., Fukada, K., Savenkov, E. I., Gogvadze, V., Clapham, D., et al. (2013). Autophagy and metacaspase determine the mode of cell death in plants. J. Cell Biol. 203, 917–927. doi: 10.1083/jcb.201307082
Minina, E. A., Smertenko, A. P., and Bozhkov, P. V. (2014). Vacuolar cell death in plants: metacaspase releases the brakes on autophagy. Autophagy 10, 928–929. doi: 10.4161/auto.28236
Minina, E. A., Staal, J., Alvarez, V. E., Berges, J. A., Berman-Frank, I., Beyaert, R., et al. (2020). Classification and nomenclature of metacaspases and paracaspases: no more confusion with caspases. Mol. Cell 77, 927–929. doi: 10.1016/j.molcel.2019.12.020
Moukhtari, A. E., Cabassa-Hourton, C., Farissi, M., and Savouré, A. (2020). How does proline treatment promote salt stress tolerance during crop plant development? Front. Plant Sci. 11:1127. doi: 10.3389/fpls.2020.01127
Ojha, R., Ishaq, M., and Singh, S. K. (2015). Caspase-mediated crosstalk between autophagy and apoptosis: mutual adjustment or matter of dominance. J. Can. Res. Ther. 11, 514–524. doi: 10.4103/0973-1482.163695
Ren, H., Zhao, X., Li, W., Hussain, J., Qi, G., and Liu, S. (2021). Calcium signaling in plant programmed cell death. Cells 10:1089. doi: 10.3390/cells10051089
Roohollah, S. D., Angelica, L., Massume, A., and Hadi, P. A. (2020). Plausible association between drought stress tolerance of barley (Hordeum vulgare L.) and programmed cell death via MC1 and TSN1 genes. Physiol. Plant. 170, 46–59. doi: 10.1111/ppl.13102
Signorelli, S., Tarkowski, Ł. P., Van den Ende, W., and Bassham, D. C. (2019). Linking autophagy to abiotic and biotic stress responses. Trends Plant Sci. 24, 413–430. doi: 10.1016/j.tplants.2019.02.001
Üstün, S., and Hafrén, A., Hofius, D. (2017). Autophagy as a mediator of life and death in plants. Curr. Opin. Plant Biol. 40, 122–130. doi: 10.1016/j.pbi.2017.08.011
Vercammen, D., Belenghi, B., Cotte, B. V. D., Beunens, T., Gavigan, J. A., Rycke, R. D., et al. (2006). Serpin1 of Arabidopsis thaliana is a suicide inhibitor for metacaspase 9. J. Mol. Biol. 364, 625–636. doi: 10.1016/j.jmb.2006.09.010
Vercammen, D., van de Cotte, B., De Jaeger, G., Eeckhout, D., Casteels, P., Vandepoele, K., et al. (2004). Type II metacaspases Atmc4 and Atmc9 of Arabidopsis thaliana cleave substrates after arginine and lysine. J. Biol. Chem. 279, 45329–45336. doi: 10.1074/jbc.M406329200
Wang, C., Zhang, L. J., and Huang, R. D. (2011). Cytoskeleton and plant salt stress tolerance. Plant Signal Behav. 6, 29–31. doi: 10.4161/psb.6.1.14202
Wang, L., and Zhang, H. (2014). Genomewide survey and characterization of metacaspase gene family in rice (Oryza sativa). J. Genet. 93, 93–102. doi: 10.1007/s12041-014-0343-6
Wang, P., Nolan, T. M., Yin, Y., and Bassham, D. C. (2020). Identification of transcription factors that regulate ATG8 expression and autophagy in Arabidopsis. Autophagy 16, 123–139. doi: 10.1080/15548627.2019.1598753
Wang, P., Wang, T., Han, J., Li, M., Zhao, Y., Su, T., et al. (2021). Plant autophagy: an intricate process controlled by various signaling pathways. Front. Plant Sci. 12:754982. doi: 10.3389/fpls.2021.754982
Yang, Y., Guo, Z., Liu, Q., Tang, J., Huang, S., Dhankher, O., et al. (2018). Growth, physiological adaptation, and NHX gene expression analysis of Iris halophila under salt stress. Environ Sci Pollut Res 25, 25207–25216. doi: 10.1007/s11356-018-2593-y
Yao, S., Luo, S., Pan, C., Xiong, W., Xiao, D., Wang, A., et al. (2020). Metacaspase MC1 enhances aluminum-induced programmed cell death of root tip cells in Peanut. Plant Soil 448, 479–494. doi: 10.1007/s11104-020-04448-w
Yu, Z., Duan, X., Luo, L., Dai, S., Ding, Z., and Xia, G. (2020). How plant hormones mediate salt stress responses. Trends Plant Sci. 25, 1117–1130. doi: 10.1016/j.tplants.2020.06.008
Yue, J. Y., Wang, Y. J., Jiao, J. L., and Wang, H. Z. (2021a). Silencing of ATG2 and ATG7 promotes programmed cell death in wheat via inhibition of autophagy under salt stress. Ecotoxicol. Environ. Safety 225:112761. doi: 10.1016/j.ecoenv.2021.112761
Yue, J. Y., Wang, Y. J., Jiao, J. L., and Wang, H. Z. (2021b). Comparative transcriptomic and metabolic profiling provides insight into the mechanism by which the autophagy inhibitor 3-MA enhances salt stress sensitivity in wheat seedlings. BMC Plant Biol. 21:577. doi: 10.1186/s12870-021-03351-5
Yue, J. Y., Wei, X. J., and Wang, H. Z. (2018). Cadmium tolerant and sensitive wheat lines: their differences in pollutant accumulation, cell damage, and autophagy. Biol. Plant 62, 379–387. doi: 10.1007/s10535-018-0785-4
Zhang, H., Deng, C., Yao, J., Zhang, Y. L., Zhang, Y. N., Deng, S., et al. (2019). Populus euphratica JRL mediates ABA response, ionic and ROS homeostasis in Arabidopsis under salt stress. Int. J. Mol. Sci. 20:815. doi: 10.3390/ijms20040815
Zhou, L. L., Gao, K. Y., Cheng, L. S., Wang, Y. L., Cheng, Y. K., Xu, Q. T., et al. (2021). Short-term waterlogging-induced autophagy in root cells of wheat can inhibit programmed cell death. Protoplasma 258, 891–904. doi: 10.1007/s00709-021-01610-8
Zhou, S., Hong, Q., Li, Y., Li, Q., and Wang, M. (2018a). Autophagy contributes to regulate the ROS levels and PCD progress in TMV-infected tomatoes. Plant Sci. 269, 12–19. doi: 10.1016/j.plantsci.2017.11.002
Keywords: autophagy, metacaspase, NaCl stress, PCD, TaMCA1, wheat seedling
Citation: Yue J-y, Wang Y-j, Jiao J-l, Wang W-w and Wang H-z (2022) The Metacaspase TaMCA-Id Negatively Regulates Salt-Induced Programmed Cell Death and Functionally Links With Autophagy in Wheat. Front. Plant Sci. 13:904933. doi: 10.3389/fpls.2022.904933
Received: 26 March 2022; Accepted: 23 May 2022;
Published: 23 June 2022.
Edited by:
Baris Uzilday, Ege University, TurkeyReviewed by:
Simon Stael, Vlaams Instituut voor Biotechnologie, BelgiumSayed Mohammad Mohsin, Sher-e-Bangla Agricultural University, Bangladesh
Copyright © 2022 Yue, Wang, Jiao, Wang and Wang. This is an open-access article distributed under the terms of the Creative Commons Attribution License (CC BY). The use, distribution or reproduction in other forums is permitted, provided the original author(s) and the copyright owner(s) are credited and that the original publication in this journal is cited, in accordance with accepted academic practice. No use, distribution or reproduction is permitted which does not comply with these terms.
*Correspondence: Hua-zhong Wang, c2t5d2h6QHRqbnUuZWR1LmNu