- 1College of Plant Protection, Shenyang Agricultural University, Shenyang, China
- 2Liaoning Key Laboratory for Biological Invasions and Global Changes, Shenyang Agricultural University, Shenyang, China
- 3College of Bioscience and Biotechnology, Shenyang Agricultural University, Shenyang, China
Increasing evidence from low-latitude ranges has demonstrated that native parasitic plants are promising biocontrol agents for some major invasive weeds. However, related mechanisms and the effect of environments on the control effect of the parasite are still unclear. In addition, few related studies have been conducted in high latitude (>40°), where the exotic plant richness is the highest in the globe, but natural enemies are relatively scarce. During field surveys, a Cuscuta species was found on the cosmopolitan invasive weed Ambrosia trifida L. in Shenyang, northeast China. Here, we first studied the impacts of the parasite on the invader at three sites with different light regimes and related mechanisms, then the haustorial connections between the parasite and the invader using anatomy and measurement of carbon (C) and nitrogen (N) stable isotope compositions (δ13C, δ15N), and finally identified the parasite using two molecular marks. The parasite was identified as C. japonica Choisy. This native holoparasitic vine posed serious C rather than N limitation to the invader, explaining its greatly inhibitory effects on the invader. Its negative effects were stronger on reproductive relative to vegetative growth, and at high relative to low light habitats, which indicated that the higher the vigor of the host is, the higher the impact of the parasite pose. The parasite could establish haustorial connections with phloem, xylem, and pith of the invader and thus obtain resources from both leaves and roots, which was confirmed by difference of δ13C and δ15N between the two species. The parasite had significantly higher leaf C concentrations and δ13C than its invasive host, being a strong C sink of the parasitic association. Our results indicate that C. japonica may be a promising biological control agent for the noxious invader in China.
Introduction
Currently, biological invasion is widely considered as one of the major drivers of global biodiversity loss (Díaz et al., 2019). As a major group of invasive alien species (IAS) worldwide (Pyšek et al., 2008), range-expanding exotic plants can override, displace local species, and dominate invaded communities (Hejda et al., 2009; Zheng et al., 2015; Iqbal et al., 2021). The enemy release hypothesis (ERH) and biotic resistance hypothesis (BRH) are two long-standing hypotheses explaining the success of some alien plants (Qin et al., 2013; Zheng et al., 2015; Zhao et al., 2020). The former posits that introduced plants experience fewer natural enemies in their new relative to old ranges and can therefore proliferate more vigorously (Keane and Crawley, 2002). The seeds and leaves of Ambrosia trifida L. (target plant in current study) suffer much less attack from aboveground enemies in its invasive range China than in the USA, its native range (Zhao et al., 2020). In addition, belowground enemies also strongly inhibit seed germination and seedling growth of the invader in the USA. BRH holds that interactions with native species limit invaders’ impacts. Native parasitic plants restrict invasive hosts and seem more environment-benign than introduced enemies, which may pose non-target effects in their new ranges besides host shift (Van Driesche et al., 2010). These native parasitic plants, which keep their invasive hosts in check, present a sustainable element of IAS management schemes (Yu et al., 2011; Cirocco et al., 2016; Wang et al., 2020; Těšitel et al., 2021). However, the relevant evidence is still limited.
Parasitic plants tend to forage whichever species is fast-growing and abundant in habitats, two of the major features of invasive plants. High growth rates can increase the competitive ability of plants at the expense of defense, showing a ‘growth-defense trade-off’ (Herms and Mattson, 1992; Feng et al., 2009). The invasive plants with high vigor were hypothesized to be the preferred hosts of local parasites (Alpert, 2006; Callaway and Maron, 2006). Studies have demonstrated that phototropic Cuscuta spp. prefer foraging hosts with high resources (Kelly, 1992; Li et al., 2007), plant height, and growth rates. These traits generally exist in many cosmopolitan invasive plant species, particularly the first (Liu et al., 2022). In China, a higher leaf nitrogen (N) concentration and less structural defense (toughness) have been found in 47 invasive plants compared with their co-occurring natives (Huang et al., 2020), including two notorious invasive plants, A. trifida and A. artemisiifolia L. These susceptible invaders with high abundance have more chances of being infected. Host preference has been found on many invasive plant species in their dominated communities (Pennings and Callaway, 1996; Prider et al., 2009; Yu et al., 2011).
Evidence is growing that native parasitic plants effectively constrain invasive hosts and favor community restoration. For instance, the native root hemiparasitic Rhinanthus has been demonstrated to extensively suppress the growth of native problematic Calamagrostis epigejos (L.) Roth and used as an effective tool for restoring the grasslands invaded by C. epigejos in Czech Republic (Těšitel et al., 2017; Těšitel et al., 2018). In South Australia, another native hemiparasitic vine (Cassytha pubescens R. Br. (Lauraceae)) decreased 40%–60% of biomass of the invasive shrub Ulex europaeus L., while it had no effects on two native hosts in a series of greenhouse experiments, regardless of light or N conditions (Cirocco et al., 2016; Cirocco et al., 2017). In addition, Cirocco et al. (2018) found that C. pubescens efficiently impaired the physiological performance of U. eurolaeus across all three sites in the fields. In South China, the native Cuscuta australis (R. Br.) greatly decreased the plant cover (by 50%–60%) and rooted node number (by 29%–64%) of three clonal perennial invaders and promoted the restoration of invaded communities (Yu et al., 2011). To date, however, related studies chiefly focused on the parasite impacts on perennial invaders, and little progress has been made in the novel biocontrol scheme for annual invasive hosts (especially in high latitudes), particularly in their reproduction, which determines their future spreads.
The isotopic signature of carbon (C) and N provided much detailed information on nutrient retention and theft in host–parasite associations in a long-term scale. Fractionation of isotopes indicated that most reactions discriminate against heavy isotopes and result in a relatively lower isotopic signature than the original source. The isotopic composition of leaf N (δ15N) provided a time-integrated measure of the external source, biological sink, and allocation of N over the period when the leaf material is metabolized (Kalcsits et al., 2014), and leaf C isotopic signature (δ13C) represented long-term leaf-level intrinsic water-use efficiency and degree of stomatal control over photosynthesis (Farquhar et al., 1989). A growing number of studies take advantage of the isotopic fractionation of C to reveal the C sources and effects of hemiparasites on their native or alien perennial hosts (Scalon and Wright, 2015; Cirocco et al., 2016; Cirocco et al., 2018). However, no study has addressed the specific N ‘trade agreement’ between parasites and their alien hosts.
Ambrosia trifida L. (giant ragweed; Asteraceae) is a summer annual herb native to temperate North America (Bassett and Terasmae, 1962), including Canada, the United States, and northern Mexico. This plant is currently a cosmopolitan invasive weed in temperate Eurasia where it easily overgrows most of the native vegetation and dominates communities. In both its native and introduced ranges, A. trifida is widely known as a noxious weed (Allard, 1943; Zhao et al., 2020). The tall height of the invader magnifies dispersal of its anemochorous pollens, which are productive and allergic to people who suffer from hay fever, and confers competitive advantage over resident vegetation by forming greater cover, as reported in other invaders (Feng et al., 2007). In China, it was first recorded in Tieling in the 1930s, and then in Shenyang, Liaoning, northeast China, in the early 1950s (Guan et al., 1983; Feng, 2020). It can grow up to 4.3 m in height in moist, fertile, and disturbed habitats, such as the banks of agricultural drainage ditches and roadsides. It has spread into more than 17 provinces, ranging from northeast Heilongjiang to southwest Sichuan and been listed in the first batch of national priority for IAS management (MAG, 2013). Thus, novel and efficient biocontrols are strongly needed for A. trifida.
In the summer of 2018, a previously unreported Cuscuta species was first observed on an A. trifida population growing along the north bank of Hunhe River in Shenyang, Liaoning. This novel host–parasite association was also found along Shenshui East Road, Shenyang, in 2019 and 2020. In this study, we first determined the negative effects of the parasite on the invader at three sites with different light intensities and the underlying mechanism then tested their haustorial connections using the anatomy and measurement of C and N isotopic signatures and finally identified the parasite using two molecular marks. We hypothesized that this parasite may efficiently restrict the growth and reproduction, by causing strong resource limitation in A. trifida. To test the hypothesis, we measured a suite of plant traits from leaf morphology, stem growth, to reproduction, such as leaf size, stem height, and achene production. We also evaluated and interpreted the resource budget of the association in terms of N and C. Our study may contribute to understanding the mechanism underlying the impacts of holoparasites on invasive plants, providing a basis for controlling invasive weeds using holoparasites in high-latitude areas.
Materials and methods
Study sites
Our study was carried out in Shenhe District, Shenyang City, Liaoning Province, northeast China. Shenyang is a semi-humid continental monsoon climate. Here, the annual mean temperature is 8.525°C, and the annual mean precipitation is 698.5 mm from 1981 to 2010. The accumulative rainfall and mean temperature in July, August, and September of 2020, during which our study was conducted, were 55.7, 333.5, and 82.8 mm, and 25.2, 24.5, and 18.0°C, respectively (data from National Meteorological Information Center of China).
Three sites were selected along Shenshui East Road (41°48′57.33″N, 123°33′06.19″E; 57 m a.s.l). The parasite on A. trifida in this area was first found in 2019. It may come from the nearby bank of Hunhe River, where the parasite on the invader was first found in 2018. Site 1 was on the slope of the road, with full sunshine; site 2 on the shoulder of the road, under the canopy of tall trees (Ulmus pumila L.); and site 3 on the road margin, under the canopy gap. The impacts of this parasite on A. trifida were determined in late August, 2020, when the invader was in the vigorous reproductive growth stage.
Samplings and measurements
At each site, six pairs of A. trifida plants infected and -uninfected by the parasite were selected, and the two plants in each pair were spaced within 1 m apart in order to decrease the potential effects of habitat heterogeneity. The main stem of each sampled plant had 16–17 nodes to ensure that the plants were at a similar growth stage. For each A. trifida plant sampled, height was measured using a stainless steel rule, and the ratio of infected main stem length to plant height as a measure of parasite infection load; the stem diameter at 40-cm height (base of stem usually had an irregular circle or oval shape) was measured using a reading Vernier caliper, and the number of branches (≥5 cm), male super-inflorescences, and all young achenes were counted, respectively.
Relative chlorophyll content was measured on the fourth leaf (from tip) of each plant using a SPAD 502 Plus Chlorophyll Meter (Minolta, Osaka, Japan). Then, the leaf was collected and placed in a Ziplock bag and immediately taken back in an ice box to our laboratory to measure the leaf area with a LI-3000C portable leaf area meter (LICOR, Lincoln, NE, USA). The leaves were oven-dried separately to a constant weight at 60°C and weighted using an analytical balance. Leaf mass per area (LMA) was calculated as the ratio of leaf biomass to area. To decrease the potential effects of the possible difference in leaf thickness on the relative chlorophyll content, the SPAD value measured for each leaf in the field was converted to mass-based SPAD value: the product of the measured SPAD value and LMA.
The dried leaves were ground using a ball mill (GT200, Grinder, Beijing, China) and stored separately in Ziplock bags for measuring C and N concentrations and their stable isotope composition (δ13C and δ15N). The measurements were performed using an elemental analyzer (Elementar Vario Micro Cube; Elementar, Langenselbold, Germany) connected to an isotope ratio mass spectrometer (IsoPrime100; IsoPrime, Ltd., Cheadle, UK) at Shenyang Institute of Applied Ecology, Chinese Academy of Science, Shenyang, China.
Stems of the parasite on the third internode of each sampled A. trifida plant were carefully collected for measurement of C and N concentrations and their stable isotope compositions. The methods were the same as those for leaf materials.
For testing the haustorial connection between the invader and the parasite, i.e., the parasitism between the two species, ≈ 2-cm-long stem segments of the host penetrated by the Cuscuta stems were collected, rinsed with distilled water, and fixed with the formalin-aceto-alcohol (FAA) fixing fluid. Anatomy of the haustorium was examined using a paraffin section (Fischer et al., 2008), and micrographs were taken on a light microscope (Nikon Eclipse Ci-L, Tokyo, Japan) with a digital camera (Nikon DS-U3).
Identification of the parasite
The stems of the parasite on A. trifida were collected at four sites (see Supplementary Table 1), specimen of the parasite–host association (SYAUB24418) was collected from the north bank of Hunhe River, and deposited in the Herbarium of Shenyang Agricultural University. Total genomic DNA of these stems was extracted using the Plant Genomic DNA Kit (Tiangen Biotech, Beijing, China). The sequences for the nuclear rDNA internal transcribed spacer 2 (ITS2) and chloroplast trnL intron were obtained using the novel primers S2F (5′-ATGCGATACTTGGTGTGAAT-3′)/S3R (5′-GACGCTTCTCCAGACTACAAT-3′) (Chen et al., 2010), and universal primers cB49317F (5′-CGAAATCGGTAGACGCTACG-3′)/dA49855R (5′-GGGGATAGAGGGACTTGAAC-3′) (Taberlet et al., 1991), respectively. The parasite DNA template (570 ng, 2 μl) and 0.5 μl of each primer (10 μM) were added to 12.5 μl 2× San Taq® Fast PCR mix buffer (Sangon Biotech, Shanghai, China), and sterile ddH2O was added to a final volume of 25 μl. The PCRs of ITS2 and trnL ran the same program as follows: initial denaturation at 94°C for 4 min, followed by 35 cycles of denaturation at 94°C for 10 s, annealing at 55°C for 20 s, extension at 72°C for 66 s, and a final extension for 5 min. These amplifications were performed in a T100 thermocycler (Bio-Rad, Hercules, CA, USA). The amplicons were cleaned with a Sanprep Column PCR Product Purification Kit (Sangon Biotech), cloned into the T1 vector with a p-Easy®-T1 Simple Cloning Kit (Transgene Biotech, Beijing China), and sequenced at Sangon Biotech.
The GenBank accession numbers for these sequences are as follows: for ITS2 (435 bp): MK070163, MW486637, MW486627, and MW486639; for trnL (386 bp): MK879389, MW618675, MW618665, and MW618676. Phylogenetic trees of these sequences of ITS2 and trnL were generated using the Bayesian inference method in MrBayes 3.2.7a (Ronquist et al., 2012). The best-fit model was determined by jModelTest 2.1.10. Reference sequences that are highly similar to the ones from our study (13 rITSs >99.1%, 7 trnLs >99.48%) were retrieved from GenBank (Supplementary Table 2).
Statistical analyses
Two-way ANOVA was used to test the effects of the parasite treatments or the groups of species and parasitic treatments (parasite, and A. trifida with and without infection; only for N and C concentrations, C:N ratio, δ13C and δ15N), study sites, and their interaction on all variables measured in this study; one-way ANOVA was used to test the difference in each variables among sites for A. trifida with the same parasitic treatments or the parasite. For N- and C-related variables, the difference among the parasite and A. trifida with and without infection at each site as well as the mean values of three sites was tested using one-way ANOVA; for the remaining variables, independent sample t-test was used to analyze the difference between A. trifida with and without infection at each site as well as the mean values of three sites. The numbers of branch and male super-inflorescences were log3-transformed; LMA and achene number were square-root-transformed in order to meet the conditions of variance analysis. All analyses were done using SPSS 13.0 (Chicago, IL, USA).
Results
Inhibitory effects of the parasite on the invader
Similar levels of parasite infection load on A. trifida at different sites, i.e., 45.76 ± 5.72% (site 1), 41.14 ± 8.94% (site 2), and 51.84 ± 7.17% (site 3) (P = 0.431), of the main stem length were coiled by the stems of C. japonica. The parasite significantly decreased the height, stem diameter, branch number, male super-inflorescence number, achene number, leaf area, and relative chlorophyll content of A. trifida, and its effects were significantly different at different sites (Supplementary Table 3, Figures 1–3). On average, the parasite decreased the height, stem diameter, branch number, male super-inflorescence number, achene number, leaf area, and relatively chlorophyll content of the invader by 28.33%, 25.93%, 50.98%, 45.51%, 76.06%, 55.17%, and 17.88%, respectively. Among the seven variables, achene number was the most susceptible to the parasitism, followed by leaf area and branch number, while relative chlorophyll content was the least susceptible followed by stem diameter and plant height.
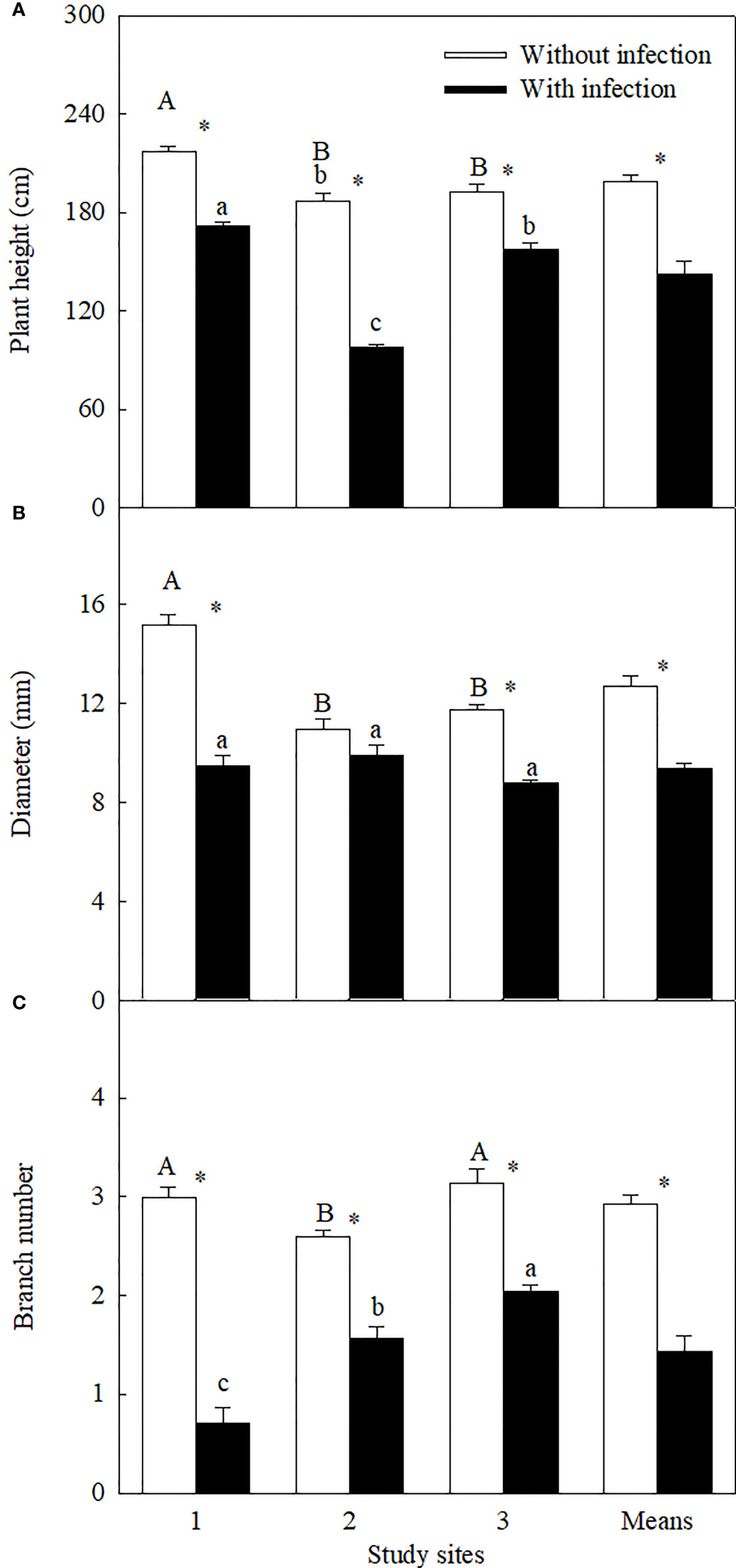
Figure 1 Plant height (A), stem diameter (B), and branch number (log3-transformed;) (C) of Ambrosia trifida uninfected (open bars) and infected (closed bars) by Cuscuta japonica at different sites. Means + 1 SE (n = 6). Different upper- and lowercase letters indicate significant differences among sites for uninfected and infected plants, respectively (P < 0.05; one-way ANOVA); * indicates significant difference between uninfected and infected plants at the same site (P < 0.05; independent sample t-test).
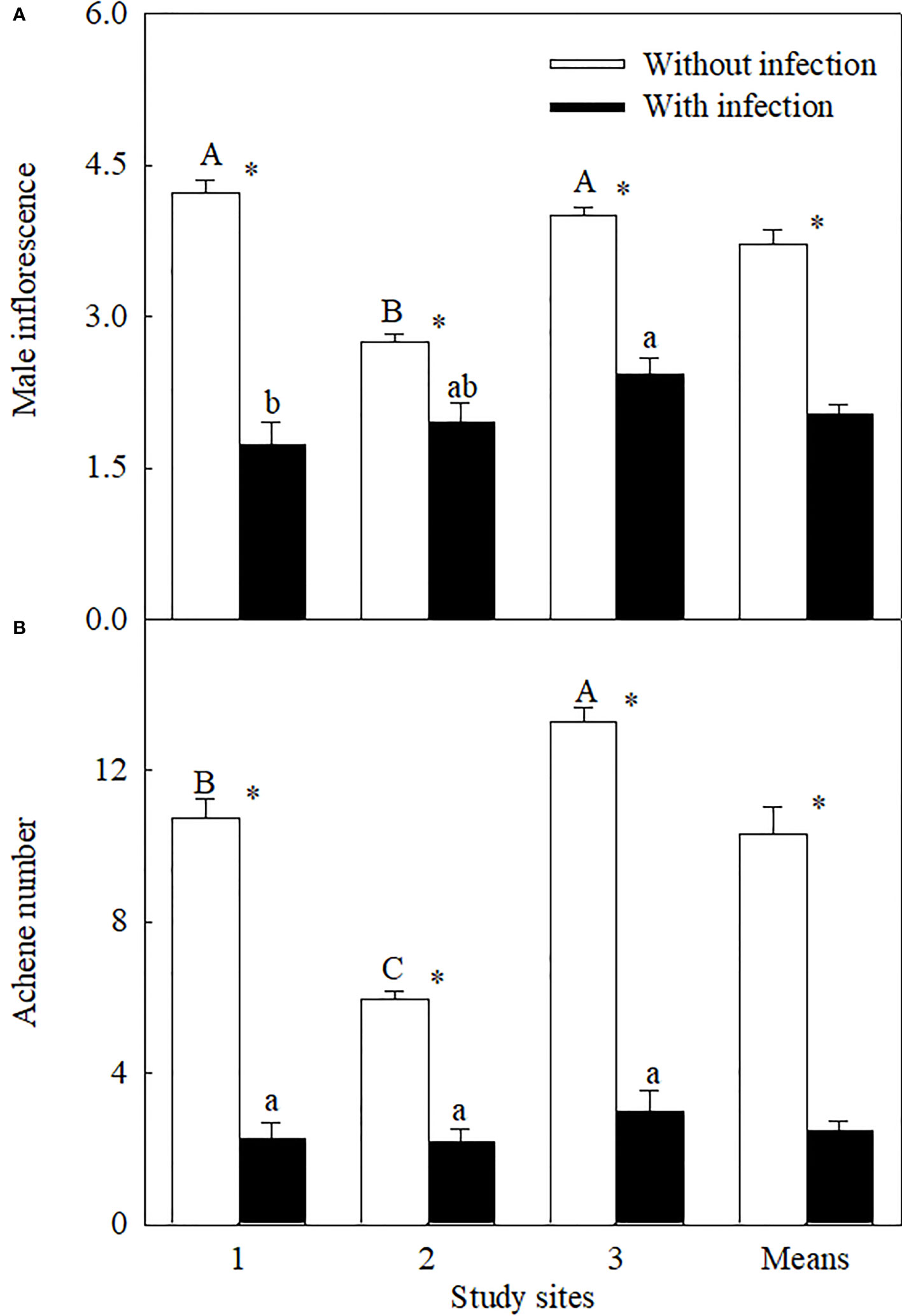
Figure 2 The number of male super-inflorescence (log3-transformed;) (A) and achene (square-root-transformed; (B) of Ambrosia trifida uninfected (open bars) and infected (closed bars) by Cuscuta japonica at different sites. Means + 1 SE (n = 6). Different upper- and lowercase letters indicate significant differences among sites for uninfected and infected plants, respectively (P < 0.05; one-way ANOVA); * indicates significant difference between uninfected and infected plants at the same site (P < 0.05; independent sample t-test).
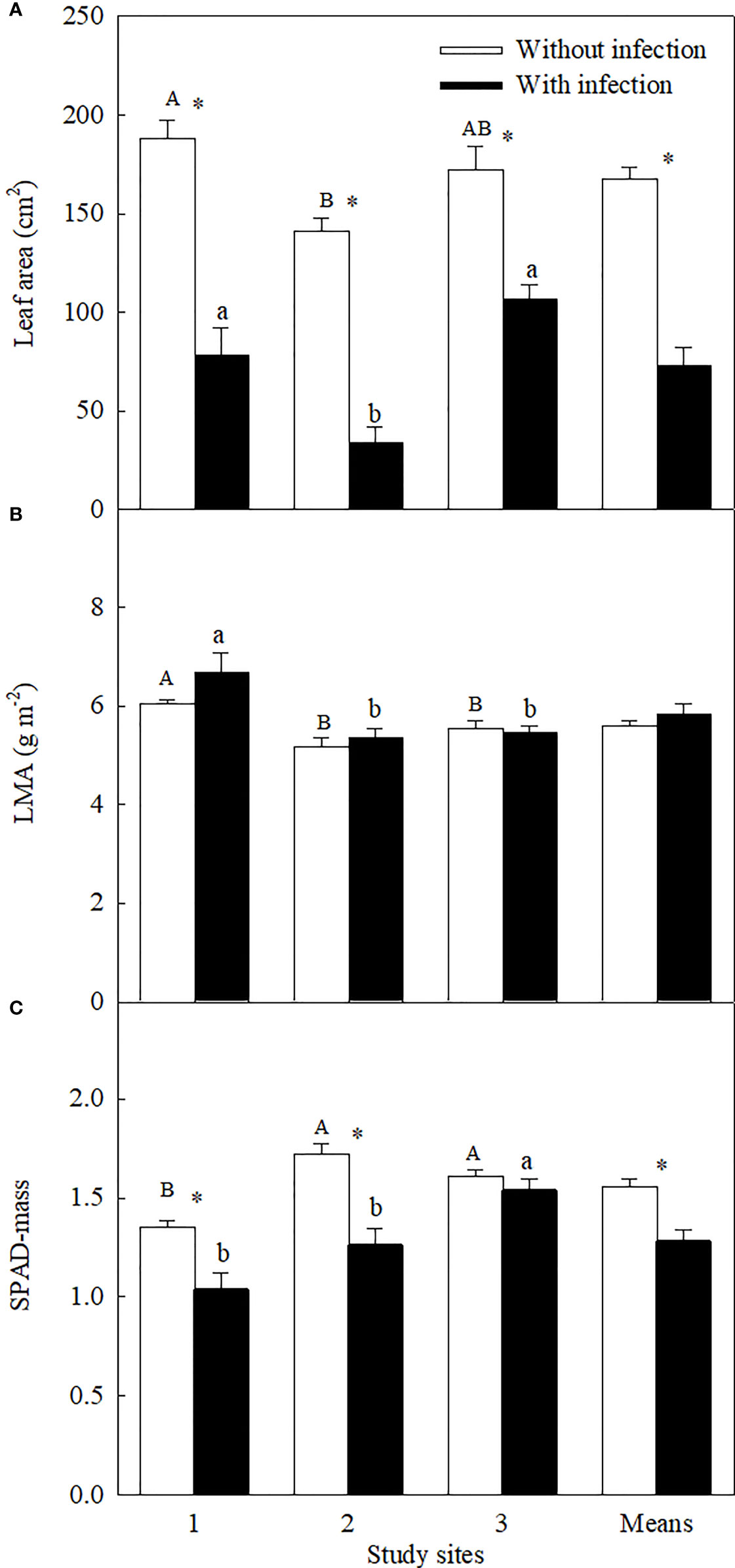
Figure 3 Leaf area (A), leaf dry mass per unit area (LMA, square-root-transformed; (B), and leaf relative chlorophyll content (mass-based SPAD; (C) of Ambrosia trifida uninfected (open bars) and infected (closed bars) by Cuscuta japonica at different sites. Means + 1 SE (n = 6). Different upper- and lowercase letters indicate significant differences among sites for uninfected and infected plants, respectively (P < 0.05; one-way ANOVA); * indicates significant difference between uninfected and infected plants at the same site (P < 0.05; independent sample t-test).
Among the three sites, stem diameter, branch number, male super-inflorescence number, and achene number were decreased the most by the parasite at site 1, i.e., by 37.32%, 76.22%, 58.99%, and 79.08%, respectively. The decreases in these four variables (except the branch number) were the least at site 2. Plant height (by 47.71%), leaf area (by 76.07%), and relative chlorophyll content (by 26.76%) were decreased the most at site 2.
The LMA of the invader was increased by attack of the parasite at site 1, but not at sites 2 and 3 (Figure 3B). The LMA of the invader was bigger at site 1 than at sites 2 and 3, no matter the plants without and with infection.
C and N concentrations and isotope compositions
Group of species and C. japonica treatments and its interaction with study sites (except δ13C) significantly influenced all five C- and N-related variables, and sites significantly affected isotope compositions only (Supplementary Table 4). On average, the parasite significantly decreased the leaf C concentration by 2.72%, while it did not significantly influence N concentration and C:N ratio (Figure 4). Specifically for each site, the parasite decreased leaf N concentration significantly at site 2 but not at sites 1 and 3 and decreased leaf C concentration at sites 1 and 2 but not at site 3, while it did not influence the C:N ratio at any site. The parasite showed a significantly lower stem N concentration but higher C concentration and C:N ratio than its host at any site.
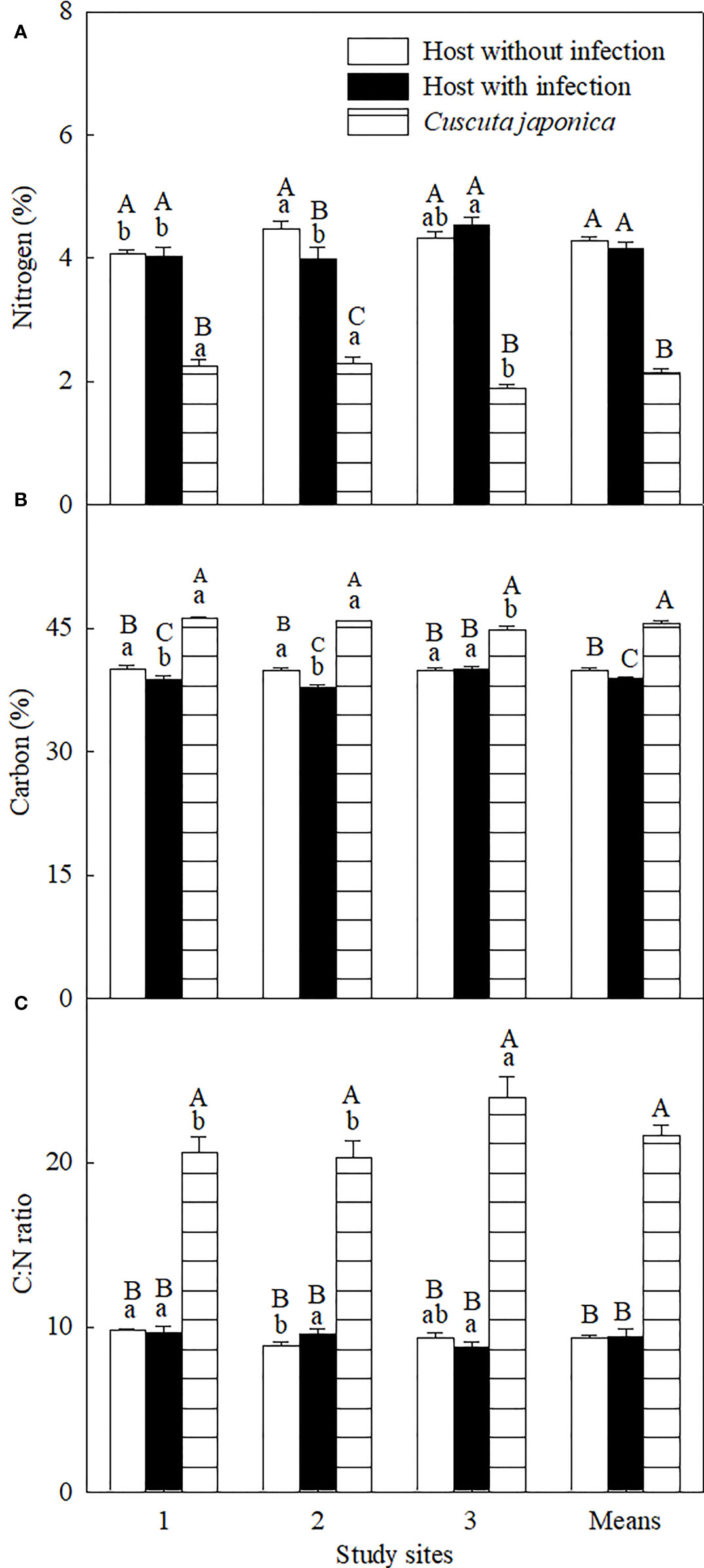
Figure 4 Concentrations of leaf nitrogen (A) and carbon (B), and C:N ratio (C) of Ambrosia trifida uninfected (open bars) and infected (closed bars) by Cuscuta japonica, and the stems of C. japonica (horizontal-striped bars) at different sites. Means + 1 SE (n = 6). Different lowercase letters indicate significant differences among sites for uninfected and infected host plants and the parasite, respectively; different uppercase letters indicate significant differences among uninfected and infected host plants and the parasite at the same site (P < 0.05; one-way ANOVA).
The parasite significantly decreased leaf δ15N at all three sites and increased leaf δ13C at site 1 but not at sites 2 and 3 (Figure 5). On average, the parasite significantly decreased leaf δ15N by 16.57%, while it did not influence leaf δ13C. Compared with its host, the parasite showed significantly higher δ15N at sites 1 and 3 but not at site 2, and higher leaf δ13C at all study sites. On average, δ15N and δ13C of the parasite were 8.02% and 18.60% higher than those of its host, respectively.
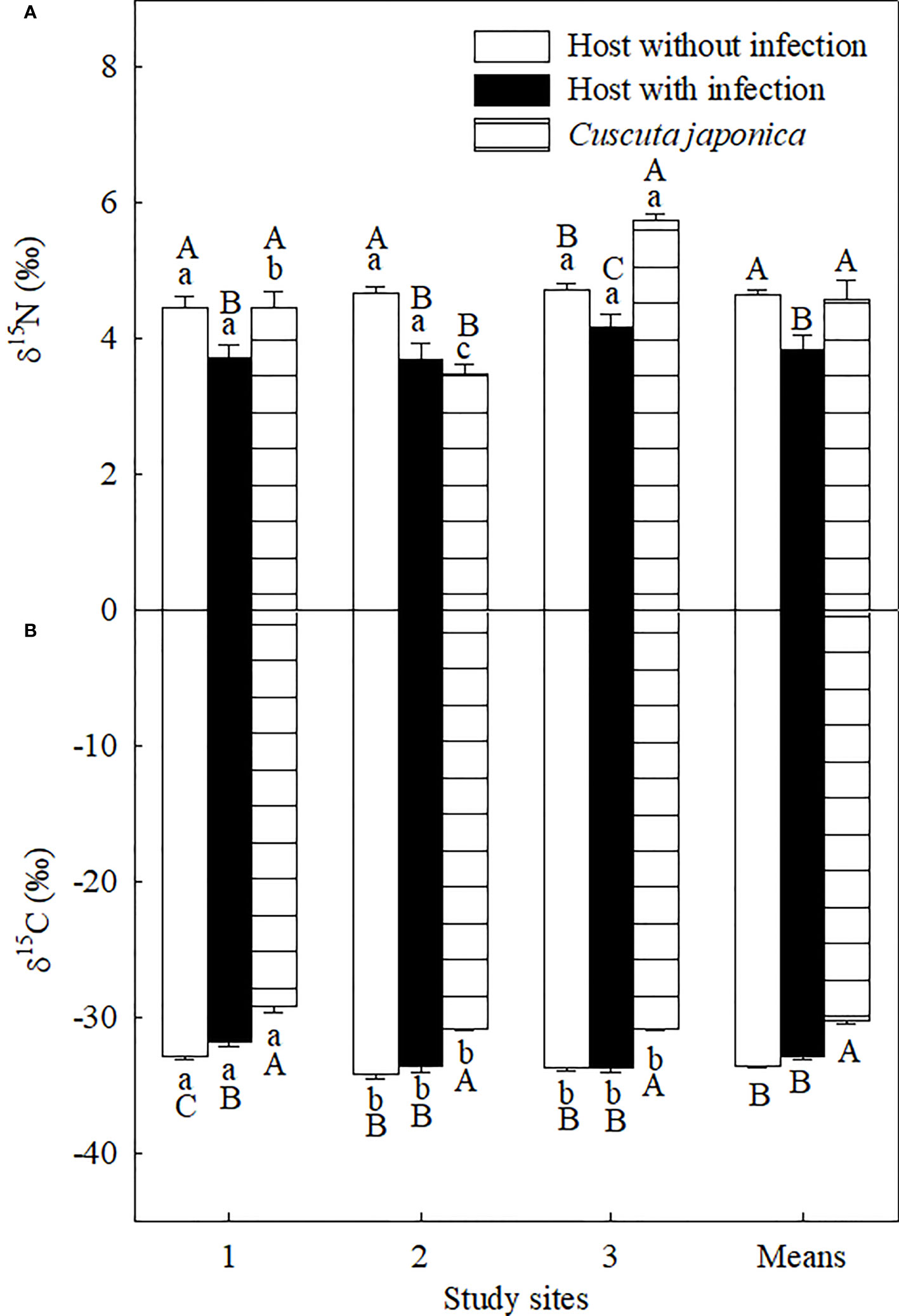
Figure 5 Stable N (δ15N; (A) and C (δ13C; (B) isotope compositions of Ambrosia trifida uninfected (open bars) and infected (closed bars) by Cuscuta japonica, and the stems of C. japonica (horizontal-striped bars) at different sites. Means + 1 SE (n = 6). Different lowercase letters indicate significant differences among sites for uninfected and infected host plants and the parasite, respectively; different uppercase letters indicate significant differences among uninfected and infected host plants and the parasite at the same site (P < 0.05; one-way ANOVA).
Anatomy of the parasitic connection
The haustorium of the parasite penetrated into the stem of A. trifida (Figure 6). The endophyte of the haustorium grew among the parenchyma and vascular bundles of the stem of the invader, including the bundle cap, phloem, xylem, and pith. The apical cells of the haustorium hyphal had conspicuous large nuclei with enlarged nucleoli and dense cytoplasm, showing their active metabolisms.
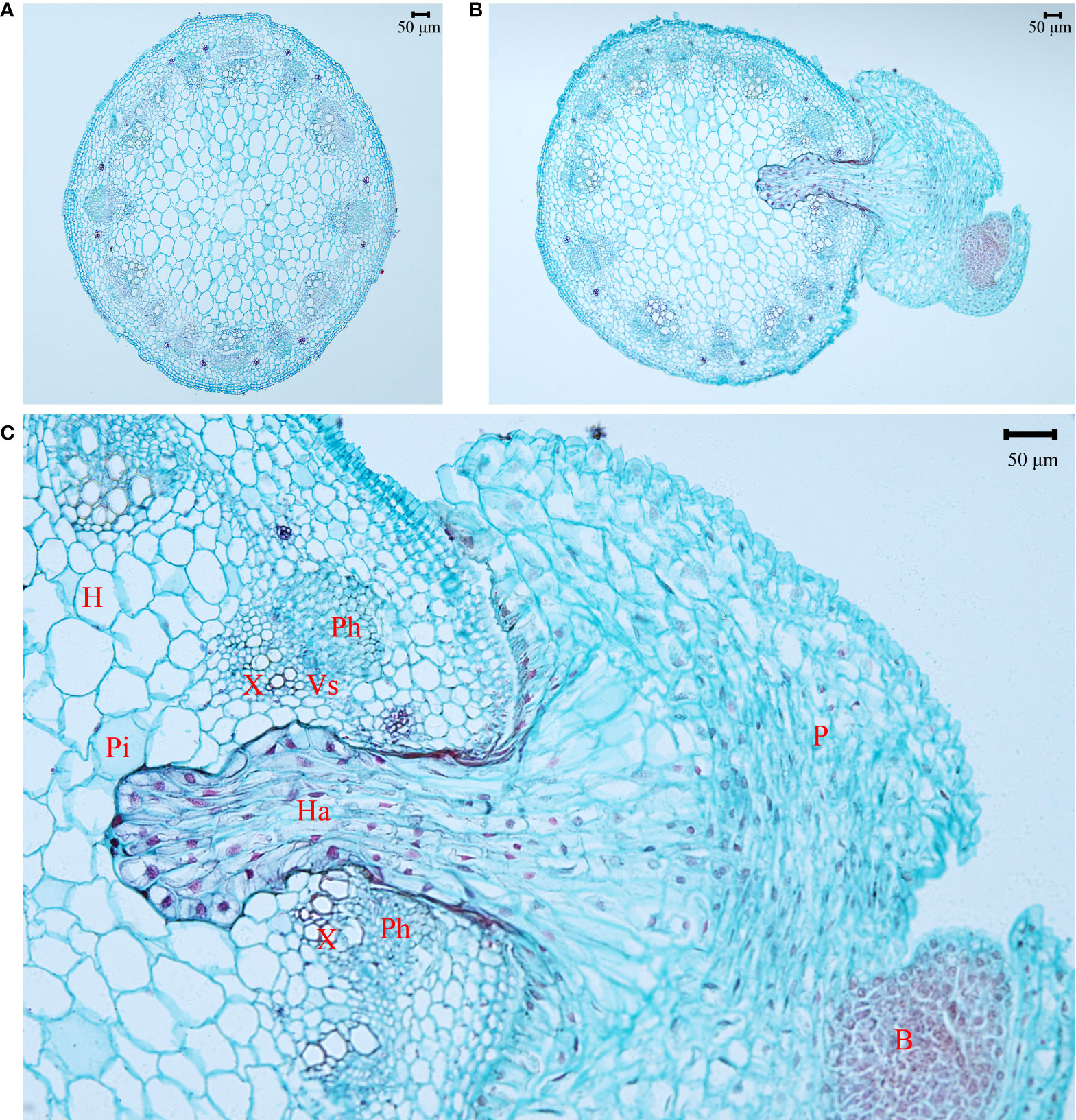
Figure 6 Anatomical structure of Ambrosia trifida stems uninfected (A) and infected (B) by Cuscuta japonica, and the magnified area of haustorial endophyte (C) in the host stem. Haustorium (Ha) of the parasite (P) penetrated into and reached pith (Pi) of the host stem (H). Ph, phloem; X, xylem; Vs, vascular stripe; B, bud. Bar = 50 μm.
Identification of the parasite
The leafless stem of the parasite (1.36 ± 0.16 mm in diameter; n = 4 per site, 12 in total) on A. trifida was pale yellow at sites 1 and 3 and green at site 2 (with relatively low light), with purplish striations. Its capsules (4.19 ± 0.19 mm long; n = 24) were ovoid and had one elongated style with two-lobed stigmas, which reached the middle of the tube. Based on these characteristics, the parasite was preliminarily identified as Cuscuta japonica Choisy (Fang et al., 1995), an indigenous parasitic plant in China.
All four rITS2 sequences of the parasite were clustered within a C. japonica-dominated clade, including one sequence of C. europaea L. (Supplementary Figure 1A). All four trnLs sequences of the parasite were clustered within the clade comprising five trnLs sequences C. japonica (Supplementary Figure 1B). All this evidence proves that the parasite is C. japonica.
Discussion
Our study showed that the parasite significantly suppressed the growth of the invasive plant A. trifida in the fields, and its inhibitory effects were greater on reproductive relative to vegetative growth and at high relative to low light habitats. Carbon rather than N constraint may be the mechanism underlying the inhibitory effects of the parasite on the invader. The parasite was identified as Cuscuta japonica based on its morphological characteristics and DNA sequences (ITS2 and trnL). The phloem-feeding C. japonica could also build a haustorial connection with xylem vessels especially at low light habitats and thus directly obtain N from the root, which was confirmed by our stable isotope composition measurements. Many studies found the negative effects of Cuscuta species on invasive plants (Qiu et al., 2010; Yu et al., 2011; Yang and Li, 2012; Vrbnicanin et al., 2013; Wang et al., 2019; Wang et al., 2020), but few studies compared their effects on vegetative versus reproductive growth and at different habitats with different resources. Thus, our understanding of the effects of parasitic plants on invasive plants is still limited, preventing the use of the novel agent in control of invasive plants.
Effect of the parasite on the invader and related mechanisms
Consistent with our hypothesis, both vegetative and reproductive growths of A. trifida were severely inhibited by C. japonica, which was at least partially associated with its negative effects on leaf area and chlorophyll content. All A. trifida plants infected by the parasite were less than 1.72 m tall (Figure 1A). For the invader, the decreased height may contribute to a decrease in its growth and ecological impacts. Hejda et al. (2009) found that the invasive plants taller than 1.9 m usually have larger hazard to diversity and evenness of co-occurring native species by forming populations with greater cover and shading the native species. Ferenc and Sheppard (2020) found that plant height is associated with growth, reproduction, and competition by comparing 20 alien annual species. In addition, the decreasing height of A. trifida may also affect its pollen dispersal, decreasing its pollination and the risk of causing people’s allergies. The parasite C. campestris was also found to significantly decrease the height of A. trifida in Serbia (Vrbnicanin et al., 2013) and Ageratina adenophora [Spreng.] R.M. King et H. Rob. in China (Qiu et al., 2010). Similarly, C. australis can inhibit the growth of invasive plant Erigeron annuus [L.] Pers. (Yang and Li, 2012) and Solidago canadensis L. (Yang et al., 2015).
Compared with vegetative growth, reproduction of A. trifida (numbers of male super-inflorescences and achenes) was more severely inhibited by C. japonica. These results were consistent with our previous findings that bur biomass decreased more greatly than aboveground biomass (87.6% to 97.2% vs. 68.7% to 80.7%) for the invasive plant Xanthium strumarium L. when infected by Cuscuta in the fields (Wang et al., 2019). Strong inhibitory effects of other parasites on reproduction were also found in other invasive plants. For example, the number of burs decreased for Solanum rostratum at different sites in northeast China when infected by C. campestris (Wang et al., 2020). Prider et al. (2011) found that the native stem hemiparasitic C. pubescens is more effective in constraining the reproduction of invasive woody shrub Cytisus scoparius than an introduced specialized seed predator Bruchidius villosus in South Australia. However, few studies to date have compared the impacts of native parasites on vegetative and reproductive growth of invasive plants.
Competition for C may be one of the reasons that C. japonica more strongly inhibited the reproductive relative to vegetative growth of A. trifida. Production of super-inflorescence and growth of pollens and fruits are the main C sink for plants at the reproductive growth stage. The phloem-feeding holoparasitic C. japonica obtains all carbohydrates exclusively from its host plants. Compared with its host, higher δ13C and C concentrations indicated that the parasite is a strong C sink for A. trifida. Based on the higher leaf δ13C, A. trifida plants infected relative to uninfected by C. japonica had a more remarkable C constraint. Host plants often initiate defense response to biotic stress when infected by parasites, such as enhancing accumulation of abscisic acid (ABA), reducing stomatal conductance (gs), and encouraging resource conservation (Ton et al., 2009). A high ABA concentration inhibited leaf expansion (Van Volkenburgh and Davies, 1983) and internode length. Under the stress of parasitic plants, many sensitive invasive hosts increased leaf ABA content (Chen et al., 2011) and reduced gs and thus photosynthesis (Frost et al., 1997; Prider et al., 2009; Cirocco et al., 2016), leaf size and stem growth (Shen et al., 2007).
In contrast to the C constraint, infection of C. japonica increased the relative availability of N for A. trifida, which was shown by the significant decrease in leaf δ15N and similar N concentration for the infected and uninfected plants (Figures 4A, 5A). The leaf retention time of N may be increased by the infection, increasing accumulative leaf N-use efficiency. The lower δ15N for A. trifida plants infected relative to uninfected by C. japonica was associated with the higher δ15N of the parasite, i.e., more 15N was used by the parasite compared with its host. The higher δ15N of C. japonica was due to the fractionation of 15N by roots and leaves of its host.
Stem holoparasitic Cuscuta spp. can establish haustorial connections with the phloem and even the xylem vessels of their hosts (Figure 6; Hegenauer et al., 2017) and obtain organic N from leaves and/or roots. In most plants, inorganic N assimilation primarily occurred in roots and leaves, and organic N was transported via xylem (the major supply route) and phloem, respectively (Kalcsits et al., 2014). Scalon and Wright (2015) found that N is not the limiting nutrient for xylem feeder such as mistletoes.
Influence of environments on the effects of the parasite
Consistent with the light environments of the study sites, LMA and δ13C were higher, while leaf relative chlorophyll content was lower for A. trifida at site 1 (with full sunshine) than at sites 2 (shade) and 3 (partial shade). These changes are a typical response to light regimes and could help plants to increase photosynthesis and water use efficiency (Feng et al., 2007; Feng, 2008). For the invader at site 1, the higher decrease in vegetative and reproductive growth caused by C. japonica indicates that the inhibitory effects of the parasite are stronger at high relative to low light environments. The higher N and C concentrations of the stems of C. japonica at high light environment revealed its strong growth vitality, which may pose severer limitation to the vigor of its host. Similarly, Cirocco et al. (2016) found that the negative effects of C. pubescens on growth are stronger for both invasive and native hosts when water availability is high relative to low. These results indicate that the parasite may impose stronger impacts on hosts (particularly reproduction) at more favorable environments.
For the morphological and chemical traits such as height, leaf area, and leaf N and C concentrations, however, the negative impact of C. japonica on the invader were stronger at site 2 (shade) than at sites 1 and 3. Intriguingly, the stronger decrease in these traits did not lead to a more significant decrease in vegetative and reproductive growths, which decreased more greatly at sites 1 and 3. Performance of the host depends not only on its production capacity of photosynthate (associated with leaf area and N content) but also on photosynthate allocation between it and the parasite. We hypothesize that more proportion of photosynthate is allocated to the parasite at high light (site 1 and 3) relative to low (site 2) light habitats, posing stronger impacts on the host, which provides a mechanism for the hypothesis that the higher the vigor of the host is, the higher the impact of the parasite pose, as mentioned above. Cuscuta spp. may be a stronger C sink than any host organ (Pennings and Callaway, 2002).
The stronger impacts of C. japonica on height and leaf area of the invader at site 2 may be associated with its strong water competition with its host. It has been demonstrated that these traits are sensitive to increasing leaf water stress (Koch et al., 2004). Leaf photosynthesis, LMA, root-to-shoot ratio, and mycorrhizal infection rate are generally lower at low light relative to high light habitats (Figure 3; Feng et al., 2007; Feng, 2008; Johnson et al., 2015). The impacts of C. japonica may further decrease the root-to-shoot ratio and mycorrhizal infection rate (by decreasing photosynthesis and consuming photosynthate), decreasing water as well as nutrient uptake. More importantly, the haustoria of C. japonica may more easily penetrate the stems and establish a connection with the xylem of the invader at site 2 compared with other sites (Figure 6), where the host is more delicate. This connection made C. japonica to intercept the water transported upward by xylem, greatly decreasing water supple for leaf and apical growth points. The same also applies to N transported upward by the xylem, consistent with the lower N concentration in the A. trifida infected relative to uninfected by the parasite. This inference was confirmed by our anatomical observation of the haustorial connection and the similar δ15N for the parasite and its host at site 2. The leaves of A. trifida and the stems of the xylem-feeding C. japonica all obtain N from xylem and thus had similar δ15N (Figure 5A).
Conclusion
The native Cuscuta japonica poses severe C but not N limitation to the invasive plant Ambrosia trifida and greatly suppresses its vegetative growth and reproduction performance in the fields. The negative impacts of the parasite are stronger at high relative to low light habitat, supporting the hypothesis that the higher the vigor of the host is, the higher the impact of the parasite pose. The significant decrease in plant height and branch number and the approximate failure in achene production indicate that the native Cuscuta may greatly decrease the ecological impacts of the invader on recipient ecosystems in the current and even the following growth season. These results indicate, for the first time, that the parasite is a promising biological agent for the noxious invader in China. A better understanding of the mechanisms underlying the impacts of native parasites on invasive plants in terms of physiology and ecology will shed light on the potential use of the parasites in controlling noxious invasive weeds, and thus the parasites’ impacts on invasive plants merit further study, through either controlled experiments or larger-scale field studies.
Data availability statement
The datasets presented in this study can be found in online repositories. The names of the repository/repositories and accession number(s) can be found in the article/Supplementary Material.
Author contributions
W-BW and Y-LF conceived and designed the experiment. W-BW, F-FG, W-WF, and Q-YW performed the experiment and analyzed the data. W-BW and Y-LF interpreted the analyses and wrote the manuscript. All authors have read and approved the submitted manuscript.
Funding
This work is financially supported by the National Natural Science Foundation of China (32171667, 31971557 and 32171666) and the National Key Research and Development Program of China (2017YFC-1200101).
Acknowledgments
The authors are grateful to Dr. Ming-Chao Liu for assistance in sampling in the fields in 2018 and 2019, Jin-Yi Xu for assistance in measuring the concentrations and stable isotope compositions of C and N, and three reviewers for their helpful and constructive comments.
Conflict of interest
The authors declare that the research was conducted in the absence of any commercial or financial relationships that could be construed as a potential conflict of interest.
Publisher’s note
All claims expressed in this article are solely those of the authors and do not necessarily represent those of their affiliated organizations, or those of the publisher, the editors and the reviewers. Any product that may be evaluated in this article, or claim that may be made by its manufacturer, is not guaranteed or endorsed by the publisher.
Supplementary material
The Supplementary Material for this article can be found online at: https://www.frontiersin.org/articles/10.3389/fpls.2022.904326/full#supplementary-material
References
Allard, H. A. (1943). The north American ragweeds and their occurrence in other parts of the world. Science 98 (2544), 292–294. doi: 10.1126/science.98.2544.292
Alpert, P. (2006). The advantages and disadvantages of being introduced. Biol. Invasions 8, 1523–1534. doi: 10.1007/s10530-005-5844-z
Bassett, I. J., Terasmae, J. (1962). Ragweeds, ambrosia species, in Canada and their history in postglacial time. Can. J. Bot. 40 (1), 141–150. doi: 10.1139/b62-015
Callaway, R. M., Maron, J. L. (2006). What have exotic plant invasions taught us over the past 20 years? Trends Ecol. Evol. 21, 369–374. doi: 10.1016/j.tree.2006.04.008
Chen, H., Shen, H., Ye, W., Cao, H., Wang, Z. (2011). Involvement of ABA in reduced photosynthesis and stomatal conductance in Cuscuta campestris–Mikania micrantha association. Biol. Plantarum 55, 545–548. doi: 10.1007/s10535-011-0122-7
Chen, S., Yao, H., Han, J., Liu, C., Song, J., Shi, L., et al. (2010). Validation of the ITS2 region as a novel DNA barcode for identifying medicinal plant species. PloS One 5 (1), e8613. doi: 10.1371/journal.pone.0008613
Cirocco, R. M., Facelli, J. M., Watling, J. R. (2016). High water availability increases the negative impact of a native hemiparasite on its non-native host. J. Exp. Bot. 67, 1567–1575. doi: 10.1093/jxb/erv548
Cirocco, R. M., Facelli, J. M., Watling, J. R. (2017). Does nitrogen affect the interaction between a native hemiparasite and its native or introduced leguminous hosts? New Phytol. 213 (2), 812–821. doi: 10.1111/nph.14181
Cirocco, R. M., Facelli, J. M., Watling, J. R. (2018). A native parasitic plant affects the performance of an introduced host regardless of environmental variation across field sites. Funct. Plant Biol. 45 (11), 1128–1137. doi: 10.1071/FP17358
Díaz, S. M., Settele, J., Brondízio, E. S., Ngo, H. T., Guèze, M., Agard, J., et al (2019). Summary for policymakers of the global assessment report on biodiversity and ecosystem services of the intergovernmental science-policy platform on biodiversity and ecosystem services. Intergovernmental Science-Policy Platform Biodiversity Ecosystem Services. 1–37. Available at: http://hdl.handle.net/11336/116171.
Fang, R. C., Lytton, J. M., Uzi, P. (1995). Flora of China. 16 (Convolvulaceae. 20. Cuscuta.). St Louis, USA: Missouri botanical garden. 322–325. Available at: http://www.efloras.org/florataxon.aspx?flora_id=2&taxon_id=108730.
Farquhar, G. D., Ehleringer, I. J. R., Hubick, K. T., City, S. L., Farquhar, G. D., Ehleringer, I. J. R., et al. (1989). Carbon isotope discrimination and photosynthesis. Ann. Rev. Plant Physiol. Plant Mol. Biol. 40, 503–537. doi: 10.1146/annurev.pp.40.060189.002443
Feng, Y. L. (2008). Photosynthesis, nitrogen allocation and specific leaf area in invasive Eupatorium adenophorum and native Eupatorium japonicum grown at different irradiances. Physiol. Plantarum 133 (2), 318–326. doi: 10.1111/j.1399-3054.2008.01072.x
Feng, Y. L., Lei, Y. B., Wang, R. F., Callaway, R. M., Valiente-Banuet, A., Inderjit, et al. (2009). Evolutionary tradeoffs for nitrogen allocation to photosynthesis versus cell walls in an invasive plant. P. Natl. Acad. Sci. U.S.A. 106 (6), 1853–1856. doi: 10.1073/pnas.0808434106
Feng, Y. L., Wang, J. F., Sang, W. G. (2007). Biomass allocation, morphology and photosynthesis of invasive and noninvasive exotic species grown at four irradiance levels. Acta Oecol. 31 (1), 40–47. doi: 10.1016/j.actao.2006.03.009
Ferenc, V., Sheppard, C. S. (2020). The stronger, the better – trait hierarchy is driving alien species interaction. Oikos 129, 1455–1467. doi: 10.1111/oik.07338
Fischer, A. H., Jacobson, K. A., Rose, J., Zeller, R. (2008). Cutting sections of paraffin-embedded tissues. CSH Protoc. 2008, pdb–prot4987. doi: 10.1101/pdb.prot4987
Frost, D. L., Gurney, A. L., Press, M. C., Scholes, J. D. (1997). Striga hermonthica reduces photosynthesis in sorghum: The importance of stomatal limitations and a potential role for ABA? Plant Cell Environ. 20, 483–492. doi: 10.1046/j.1365-3040.1997.d01-87.x
Guan, G. Q., Gao, D. C., Cui, H. J., Guo, X. Z., Huang, B. H. (1983). Preliminary report of two species of Ambrosia in liaoning. Plant Quarant. 27, 16–18. doi: 10.19662/j.cnki.issn1005-2755.1983.06.004
Hegenauer, V., Körner, M., Albert, M. (2017). Plants under stress by parasitic plants. Curr. Opin. Plant Biol. 38, 34–41. doi: 10.1016/j.pbi.2017.04.006
Hejda, M., Pyšek, P., Jarošík, V. (2009). Impact of invasive plants on the species richness, diversity and composition of invaded communities. J. Ecol. 97 (3), 393–403. doi: 10.1111/j.1365-2745.2009.01480.x
Herms, D. A., Mattson, W. J. (1992). The dilemma of plants: To grow or defend. Q. Rev. Biol. 67 (3), 283–335. doi: 10.1086/417659
Huang, K., Kong, D. L., Lu, X. R., Feng, W. W., Liu, M. C., Feng, Y. L. (2020). Lesser leaf herbivore damage and structural defense and greater nutrient concentra-tions for invasive alien plants: Evidence from 47 pairs of invasive and non-invasive plants. Sci. Total Environ. 723, 137829. doi: 10.1016/j.scitotenv.2020.137829
Iqbal, M. F., Feng, Y. L., Feng, W. W., Liu, M. C., Lu, X. R. (2021). Ecological impacts of the invasive plant Xanthium strumarium and the impacts of three aboveground herbivores on the invader. Ecol. Indic. 131, 108140. doi: 10.1016/j.ecolind.2021.108140
Johnson, N. C., Wilson, G. W., Wilson, J. A., Miller, R. M., Bowker, M. A. (2015). Mycorrhizal phenotypes and the law of the minimum. New Phytol. 205 (4), 1473–1484. doi: 10.1111/nph.13172
Kalcsits, L. A., Buschhaus, H. A., Guy, R. D. (2014). Nitrogen isotope discrimination as an integrated measure of nitrogen fluxes, assimilation and allocation in plants. Physiol. Plantarum 151 (3), 293–304. doi: 10.1111/ppl.12167
Keane, R. M., Crawley, M. J. (2002). Exotic plant invasions and the enemy release hypothesis. Trends Ecol. Evol. 17, 164–170. doi: 10.1016/S0169-5347(02)02499-0
Kelly, C. K. (1992). Resource choice in Cuscuta europaea. P. Natl. Acad. Sci. U.S.A. 89 (24), 12194–12197. doi: https://doi.org/10.1073/pnas.89.24.12194
Koch, G. W., Sillett, S. C., Jennings, G. M., Davis, S. D. (2004). The limits to tree height. Nature 428 (6985), 851–854. doi: 10.1038/nature02417
Li, S.X., Hu, F., Kong, C.H., Tan, Z.W. (2007). Ecophysiological characteristics response of different soybean (Glycine max L.) cultivars to dodder (Cuscuta chinensis) parasitizing. Acta Ecol. Sinica. 27(7), 2748–2755. (Chinese with English Abstract): doi: 1000-0933 (2007)07-2748-08
Liu, M. C., Dong, T. F., Feng, W. W., Qu, B., Kong, D. L., van Kleunen, M., et al. (2022). Leaf trait differences between 97 pairs of invasive and native plants across China: Effects of identities of both the invasive and native species. NeoBiota 71, 1–22. doi: 10.3897/neobiota.71.71385
MAG (2013). Gazette of the ministry of agriculture of the people’s republic of China. Announcement No. 1897114 (3), 61–62. Available at: http://www.moa.gov.cn/nybgb/2013/dsanq/201712/t20171219_6119282.htm.
Pennings, S. C., Callaway, R. M. (1996). Impact of a parasitic plant on the structure and dynamics of salt marsh vegetation. Ecology 77, 1410–1419. doi: 10.2307/2265538
Pennings, S. C., Callaway, R. M. (2002). Parasitic plants: Parallels and contrasts with herbivores. Oecologia 131, 479–489. doi: 10.1007/s00442-002-0923-7
Prider, J. N., Facelli, J. M., Watling, J. R. (2011). Multispecies interactions among a plant parasite, a pollinator and a seed predator affect the reproductive output of an invasive plant, Cytisus scoparius. Austral Ecol. 36 (2), 167–175. doi: 10.1111/j.1442-9993.2010.02132.x
Prider, J. N., Watling, J. R., Facelli, J. M. (2009). Impacts of a native parasitic plant on an introduced and a native host species: Implications for the control of an invasive weed. Ann. Bot.-London. 103, 107–115. doi: 10.1093/aob/mcn214
Pyšek, P., Richardson, D. M., Pergl, J., Jarošík, V., Sixtová, Z., Weber, E. (2008). Geographical and taxonomic biases in invasion ecology. Trends. Ecol. Evol. 23, 237–244. doi: 10.1016/j.tree.2008.02.002
Qin, R. M., Zheng, Y. L., Valiente-Banuet, A., Callaway, R. M., Barclay, G. F., Pereyra, C. S., et al. (2013). The evolution of increased competitive ability, innate competitive advantages, and novel biochemical weapons act in concert for a tropical invader. New Phytol. 197 (3), 979–988. doi: 10.1111/nph.12071
Qiu, B., Sang, W. J., Wang, L. S., Zhang, X. W. (2010). A prevention effect of parasitic plant Cuscuta on Eupatorium - an invasive plant. J. Mount. Agric. Biol. 02, 185–188. doi: 10.15958/j.cnki.sdnyswxb.2010.02.009
Ronquist, F., Teslenko, M., van der Mark, P., Ayres, D. L., Darling, A., Höhna, S., et al. (2012). MrBayes 3.2: efficient Bayesian phylogenetic inference and model choice across a large model space. Syst. Boil. 61 (3), 539–542. doi: 10.1093/sysbio/sys029
Scalon, M. C., Wright, I. J. (2015). A global analysis of water and nitrogen relationships between mistletoes and their hosts: Broad-scale tests of old and enduring hypotheses. Funct. Ecol. 29, 1114–1124. doi: 10.1111/1365-2435.12418
Shen, H., Hong, L., Ye, W., Cao, H., Wang, Z. (2007). The influence of the holo-parasitic plant Cuscuta campestris on the growth and photosynthesis of its host Mikania micrantha. J. Exp. Bot. 58 (11), 2929–2937. doi: 10.1093/jxb/erm168
Taberlet, P., Gielly, L., Pautou, G., Bouvet, J. (1991). Universal primers for amplification of three non-coding regions of chloroplast DNA. Plant Mol. Boil. 17 (5), 1105–1109. doi: 10.1007/BF00037152
Těšitel, J., Li, A. R., Knotková, K., Mclellan, R., Bandaranayake, P. C. G., Watson, D. M. (2021). The bright side of parasitic plants: What are they good for? Plant Physiol. 185 (4), 1309–1324. doi: 10.1093/plphys/kiaa069
Těšitel, J., Mládek, J., Fajmon, K., Blažek, P., Mudrák, O. (2018). Reversing expansion of calamagrostis epigejos in a grassland biodiversity hotspot: Hemi-parasitic Rhinanthus major does a better job than increased mowing intensity. Appl. Veg. Sci. 21, 104–112. doi: 10.1111/avsc.12339
Těšitel, J., Mládek, J., Horník, J., Těšitelová, T., Adamec, V., Tichý, L. (2017). Suppressing competitive dominants and community restoration with native parasitic plants using the hemiparasitic Rhinanthus alectorolophus and the dominant grass Calamagrostis epigejos. J. Appl. Ecol. 54 (5), 1487–1495. doi: 10.1111/1365-2664.12889
Ton, J., Flors, V., Mauch-Mani, B. (2009). The multifaceted role of ABA in disease resistance. Trends Plant Sci. 14 (6), 310–317. doi: 10.1016/j.tplants.2009.03.006
Van Driesche, R. G., Carruthers, R. I., Center, T., Hoddle, M. S., Hough-Goldstein, J., Morin, L., et al. (2010). Classical biological control for the protection of natural ecosystems. Bio. Control. 54, S2–S33. doi: 10.1016/j.biocontrol.2010.03.003
Van Volkenburgh, E., Davies, W. J. (1983). Inhibition of light-stimulated leaf expansion by abscisic acid. J. Exp. Bot. 34 (7), 835–845. doi: 10.1093/jxb/34.7.835
Vrbnicanin, S., Saric-Krsmanovic, M., Bozic, D. (2013). The effect of field dodder (Cuscuta campestris yunck.) on morphological and fluorescence para-meters of giant ragweed (Ambrosia trifida l.) Pestic. phytomed. (Belgrade) 28 (1), 57–62. doi: 10.2298/PIF1301057V
Wang, W. B., An, M. N., Feng, Y. L., Qu, B. (2019). First report of dodder (Cuscuta australis) on the invasive weed Xanthium strumarium var. Canadense in China. Plant Dis. 103 (3), 591–591. doi: 10.1094/PDIS-07-18-1200-PDN
Wang, W. B., Gao, F. F., Shao, M. N., Liu, M. C., Zhai, H. F., Qu, B., et al. (2020). First record of field dodder (Cuscuta campestris) parasitizing invasive buffalo bur (Solanum rostratum). J. Plant Pathol. 102, 703–707. doi: 10.1007/s42161-020-00578-3
Yang, B. F., Du, L. S., Li, J. M. (2015). Effects of Cuscuta australis parasitism on the growth, reproduction and defense of Solidago canadensis. Chin. J. Appli. Ecol. 26 (11), 3309–3314. doi: 10.13287/j.1001-9332.20150915.005
Yang, B. F., Li, J. M. (2012). Effect of the parasitism of Cuscuta australis on the growth of three invasive plants. J. Zhejiang Univer. 02, 127–131. doi: 10.3785/j.issn.1008-9209.2012.02.002
Yu, H., Liu, J., He, W. M., Miao, S. L., Dong, M. (2011). Cuscuta australis restrains three exotic invasive plants and benefits native species. Bio. Invasions 13, 747–756. doi: 10.1007/s10530-010-9865-x
Zhao, Y. Z., Liu, M. C., Feng, Y. L., Wang, D., Feng, W. W., Clay, K., et al. (2020). Release from below- and aboveground natural enemies contributes to invasion success of a temperate invader. Plant Soil. 452, 19–28. doi: 10.1007/s11104-020-04520-5
Keywords: Ambrosia trifida, plant invasions, Cuscuta japonica, carbon, nitrogen, growth, reproduction, stable isotope composition
Citation: Wang W-B, Gao F-F, Feng W-W, Wu Q-Y and Feng Y-L (2022) The native stem holoparasitic Cuscuta japonica suppresses the invasive plant Ambrosia trifida and related mechanisms in different light conditions in northeast China. Front. Plant Sci. 13:904326. doi: 10.3389/fpls.2022.904326
Received: 25 March 2022; Accepted: 08 September 2022;
Published: 23 September 2022.
Edited by:
Ming Dong, Hangzhou Normal University, ChinaReviewed by:
Christiane M. Ritz, Senckenberg Museum of Natural History Görlitz, GermanyDaolin Du, Jiangsu University, China
Copyright © 2022 Wang, Gao, Feng, Wu and Feng. This is an open-access article distributed under the terms of the Creative Commons Attribution License (CC BY). The use, distribution or reproduction in other forums is permitted, provided the original author(s) and the copyright owner(s) are credited and that the original publication in this journal is cited, in accordance with accepted academic practice. No use, distribution or reproduction is permitted which does not comply with these terms.
*Correspondence: Yu-Long Feng, ZnlsQHN5YXUuZWR1LmNu; eWxfZmVuZ0B0b20uY29t