- 1Tianjin Genovo Biotechnology Co., Ltd., Tianjin, China
- 2Institute of Crop Sciences, Chinese Academy of Agricultural Sciences, Beijing, China
Soybean is one of the important food, feed, and biofuel crops in the world. Soybean genome modification by genetic transformation has been carried out for trait improvement for more than 4 decades. However, compared to other major crops such as rice, soybean is still recalcitrant to genetic transformation, and transgenic soybean production has been hampered by limitations such as low transformation efficiency and genotype specificity, and prolonged and tedious protocols. The primary goal in soybean transformation over the last decade is to achieve high efficiency and genotype flexibility. Soybean transformation has been improved by modifying tissue culture conditions such as selection of explant types, adjustment of culture medium components and choice of selection reagents, as well as better understanding the transformation mechanisms of specific approaches such as Agrobacterium infection. Transgenesis-based breeding of soybean varieties with new traits is now possible by development of improved protocols. In this review, we summarize the developments in soybean genetic transformation to date, especially focusing on the progress made using Agrobacterium-mediated methods and biolistic methods over the past decade. We also discuss current challenges and future directions.
Introduction
Soybean [Glycine max (L.) Merrill] is a legume crop belonging to the family of Leguminosae, a subfamily of Papilionoideae. Soybean is grown worldwide and is one of the most important crop plants for its high seed oil and protein content, and for its capability to fix atmospheric nitrogen by symbioses with soil-borne microorganisms. Recent studies on high-quality reference genome sequencing of a United States variety, Williams82 (Schmutz et al., 2010), a Japanese variety, Enrei (Shimomura et al., 2015), a Chinese cultivar, Zhonghuang13, and a wild soybean, W05 (Shen et al., 2018; Xie et al., 2019) have estimated that there exist a total of 46,430 protein-coding genes in soybean, 70% more than that in Arabidopsis. Soybean is an ancient polyploidy (palaeopolyploid) plant with a highly duplicated genome. Nearly 75% of the genes are present in multiple copies, representing a threefold redundancy due to its long evolutionary history (Schmutz et al., 2010). Some repetitive sequence families may be species-specific (Morgante et al., 1997). Several other databases have been developed, including an expressed sequence tag (EST) database, full-length cDNAs and cDNA microarrays (Stacey et al., 2004; Umezawa et al., 2008), and a haplotype map (GmHapMap) (Torkamaneh et al., 2019). These resources provide a wide range of opportunities for soybean improvement by marker-assisted breeding and with transgenic and genome editing approaches, and for understanding gene function through various forward and reverse genetic approaches. Most of these approaches are reliant on high-throughput transformation systems.
Genetic transformations allow for various genes of interest to be introduced and expressed in cells of living organisms, which can also overcome barriers of sexual incompatibility. Soybean genetic transformation was originally developed in late 1980s. The first fertile transgenic soybeans were produced by either regeneration of cotyledonary nodes infected with Agrobacterium tumefaciens (Hinchee et al., 1988) or by particle bombardment using meristems of immature soybean seeds (Mccabe et al., 1988). The development of soybean transgenic methods before 2013 has previously been extensively reviewed (Homrich et al., 2012; Yamada et al., 2012; Lee et al., 2013; Mariashibu et al., 2013). Soybean improvements using these transformation methods have been continued over the last 30 years. Since the first transgenic herbicide-resistant soybean product was commercialized in the mid 1990s, soybean has become one of the most important crops improved using modern biotechnology and one of the major commercially grown transgenic plants around the world. Genetically modified (GM) soybean, especially the GM Roundup Ready soybean resistant to glyphosate herbicides, has been grown in many countries including the United States, Argentina, and Brazil (Pagano and Miransari, 2016), which has made it a leading biotech crop. This soybean variety allows for growers to spray herbicides to kill any weeds in the field while not killing the soybean crop1. It was reported that about 105 million hectares of GM soybean was grown in 2017, and that about 272 million metric tons of seeds were produced, which accounted for 80% of all soybean production in the world (Voora et al., 2020). Genetic engineering has been conducted to enhance the protein quality of soybean by altering biosynthetic feedback pathways that increase lysine and sulfur-containing amino acids (Falco et al., 1995). Many types of GM soybeans have improved traits such as increased oleic acid content, decreased linolenic acid content, delayed flowering time, modified plant architecture and increased yield (Yamada et al., 2012). With increasing soybean demands around the world, especially from China, developing GM soybean varieties with high quality and yield is the main task for soybean researchers and breeders. Recently, genome editing (GE) technologies, especially the clustered regularly interspaced short palindromic repeats (CRISPR)/CRISPR-associated (Cas) technology, have been used for studying soybean genetics and commercial trait development (reviewed in Xu et al., 2020). However, using genome editing technologies on plants has been heavily dependent on efficient transformation systems and regeneration of plants containing edited events (Ran et al., 2017; Gao, 2021). Therefore, an efficient and genotype-flexible transformation system is key to realizing soybean improvement using these new technologies. Unfortunately, soybean remains recalcitrant to routine transformations compared to other major cultivated crops such as rice (Chen et al., 2018b). Low transformation frequency and genotype inflexibility are major hurdles that limit soybean transgenesis and breeding. In this review, we will summarize the major achievements that have been made in this field since 2013, and describe current best methods used for achieving stable and transient transformations in soybean. We also describe the remaining challenges that need to be addressed.
Current Transformation Methods Developed for Soybean
Various transformation methods have been developed for soybean. Here, we will summarize each transformation method and its ability to produce either stable transgenic plants or transient events used for soybean research (Figure 1).
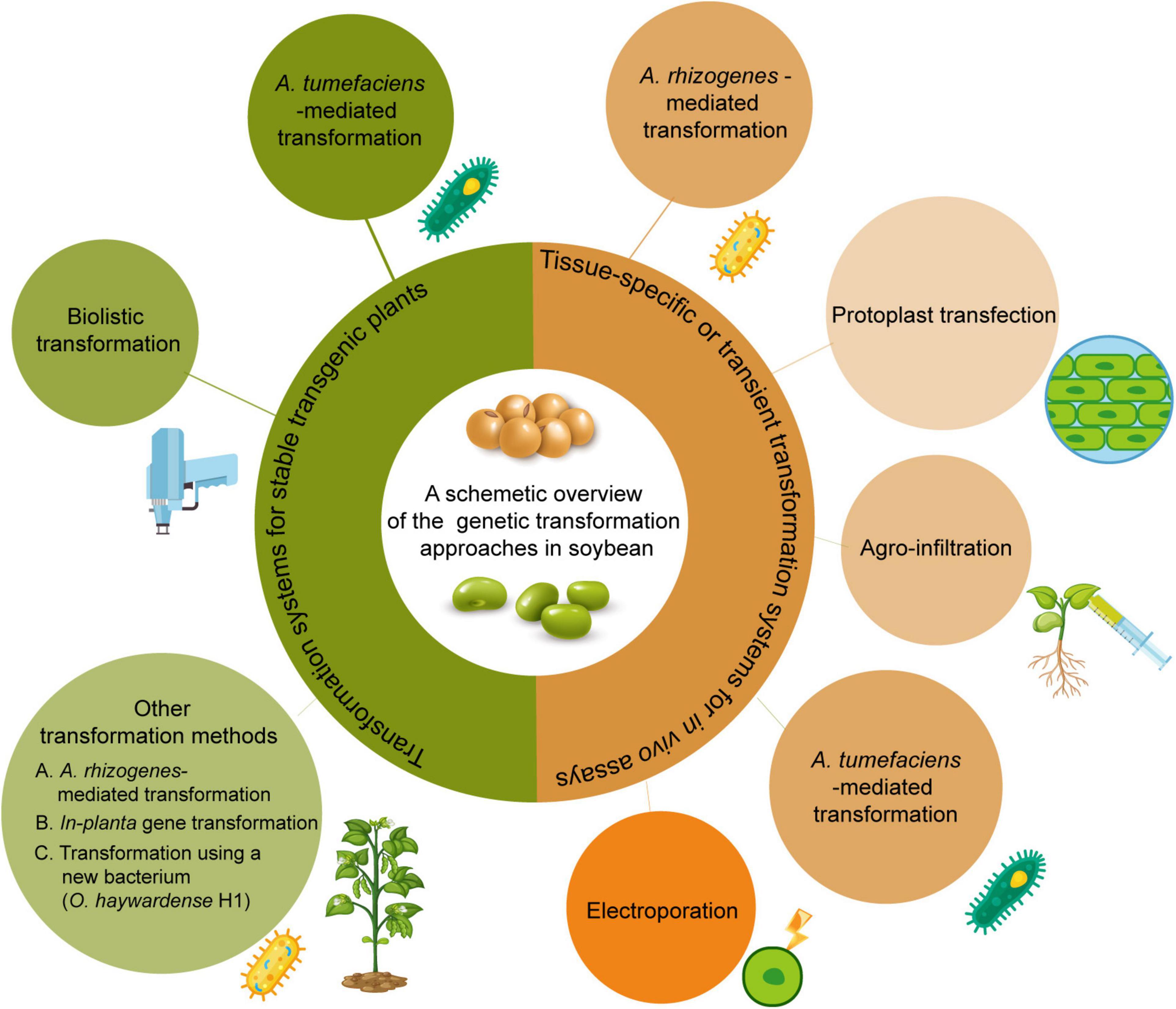
Figure 1. Current available genetic transformation methods for soybean. The left part with greenish color shows transformation methods to produce stable whole plants. The right part with yellowish color shows transformation methods for transient assay.
Tissue-Specific or Transient Transformation Systems for in vivo Assays
Transient assays are used for a variety of studies including the functional genomics of in vivo gene expression and subcellular gene localization, and determination of genome editing efficiency. For soybean, Agrobacterium rhizogenes-mediated transformation, protoplast transfection, Agro-infiltration, and electroporation have been developed. Agrobacterium transformation and protoplast transfection are frequently performed for transient assays.
Agrobacterium rhizogenes-Mediated Transformation
Agrobacterium rhizogenes-mediated transformation leads to development of a hairy-root phenotype. This method relies on co-transfer of T-DNAs from the Ri plasmid and a binary vector containing a gene of interest into the plant genome (Christey, 2001; Broothaerts et al., 2005). Large numbers of transgenic hairy-roots can be obtained in the absence of exogenous plant growth regulators (Collier et al., 2005), and each represents an independent transformation event (Kereszt et al., 2007). The relatively short timeframe (approximately 6–8 weeks) for recovering transformants is a major advantage for screening genes and promoters or expressing foreign genes in a stably transformed plant as a bioreactor (Cho et al., 2000; Bahramnejad et al., 2019). This method is also used for studying functional genomics in soybean roots. This approach has been used to characterize promoters (Hernandez-Garcia et al., 2009; Li et al., 2014), propagation of nematodes (Cho et al., 2000), symbiotic interactions (Hayashi et al., 2012), pathogenic interactions (Li et al., 2010), gene silencing by RNA interference (RNAi) (Subramanian et al., 2004), and recently for measuring genome editing activity (Du et al., 2016; Cheng et al., 2021). Recently, a reporter gene AtMyb75, encoding an R2R3 type MYB transcription factor, was ectopically expressed in hairy roots-mediated by A. rhizogenes and induced purple/red colored anthocyanin accumulation in soybean hairy roots. This is a convenient, non-destructive, low cost, directly visual selection of transgenic hairy roots (Fan et al., 2020). Several efficient transformation protocols have been developed for studying functional genomics and root biology (Kereszt et al., 2007; Kuma et al., 2015; Chen et al., 2018a,c; Fan et al., 2020; Song et al., 2021).
Protoplast Transfection
The first genetic transformation of soybean protoplasts was achieved by electroporation by Lin et al. (1987). Dhir et al. (1992) was the first to report the transformation of immature cotyledon-derived protoplasts and regeneration of transgenic plants from calli derived from electroporation-transfected protoplasts. Protoplasts could be a good explant for transformation if an efficient regeneration system is established, especially since a large number of protoplasts can be transfected at a time and many forms of genetic materials such as DNA, RNA, and protein can be delivered. Unfortunately, protoplast transfection has not yet been conducted for soybean transgenic plant production. The main challenge is achieving protoplast regeneration, which has yet to be reported in soybean. Protoplast-based transfection has been mainly conducted to evaluate gene functions (Yi et al., 2010; Faria et al., 2011; Kidokoro et al., 2015; Xiong et al., 2019), screen promoters (Sultana et al., 2019), and validate vectors for GE (Sun et al., 2015; Demorest et al., 2016; Do et al., 2019; Patil et al., 2022). Recently, Wu and Hanzawa (2018) developed a method to isolate protoplasts from leaves of soybean seedlings and established a PEG-mediated transfection method that can achieve high transfection efficiency compared to other transient assays.
Agro-Infiltration
Agrobacteria can be infiltrated into the intercellular space of plant tissues to permit the delivery of genes from different organisms into plant genomes (Grimsley et al., 1986). Ever since this method was successfully established for soybean (King et al., 2015), it has been used for virus-induced gene silencing (VIGS) (Kim et al., 2016) and expression of hairpin RNA for RNAi against two-spot spider mites (Dubey et al., 2017).
Agrobacterium tumefaciens-Mediated Transformation
Except for stable transformation, A. tumefaciens is used to carry out transformation for soybean transient assay. Kun et al. (2017) established an Agrobacterium-mediated transient system using calli induced from hypocotyl explants. It has been successfully used in many specific assays including Western blot and Co-IP assay for protein analysis. The system is genotype-flexible and cost-saving. However, it takes a couple of months to complete the assay.
Electroporation
Electroporation is a technique that utilizes a high intensity electric pulse to create transient pores in the cell membrane, thereby facilitating the uptake of macromolecules such as DNA. Christou et al. (1988) conducted electroporation to deliver constructs into soybean calli and showed stable integration of genes but did not succeed in regenerating plants. Later, Chowrira et al. (1995) reported on electroporation of intact nodal meristems which avoided the soybean tissue culture process completely, but no transgenic plants have been recovered.
Transformation Systems for Stable Transgenic Plants
Agrobacterium-mediated transformation and biolistic methods, and in planta transformation and protoplast transfection methods have been applied for generation of transgenic soybean plants. Among these methods, the A. tumefaciens-mediated and biolistic methods are the two major platforms for stable soybean transformation. The general transformation procedure of both methods is shown in Figure 2. The other methods mentioned above are used less because of relatively low efficiency and the specific technique and equipment required in these methods.
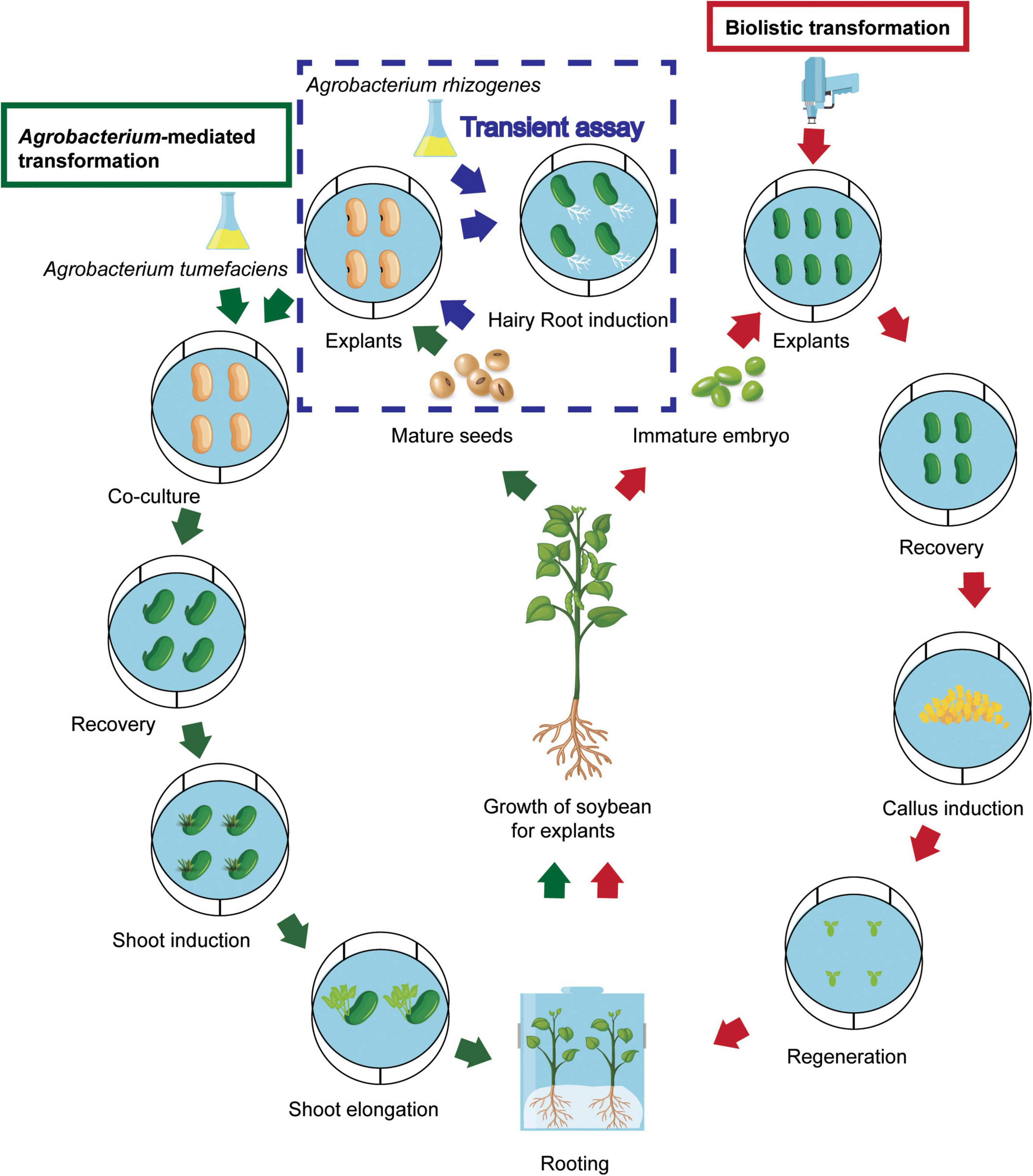
Figure 2. General procedure of Agrobacterium-mediated and biolistic soybean transformation. The route following the green arrows is the Agrobacterium tumefaciens-mediated transformation procedure. The route following the red arrows is the biolistic transformation procedure. The route following the blue arrows in the frame with broken blue line shows Agrobacterium rhizogenes-mediated transformation for transient assay.
A. tumefaciens-Mediated Transformation
A. tumefaciens-mediated transformation of soybean was first initiated using cotyledonary nodes by Hinchee et al. (1988). Since the system was established based on regeneration of mature or immature seed explants, the simplicity and relatively high TF of the method have made it a favorite method for soybean. Relatively high efficient Agrobacterium-mediated transformation protocol has been gradually developed through improving factors such as using an appropriate Agrobacterium strain, a good explant, culture media with adequate antioxidant chemicals and combinations of appropriate plant growth regulators for a specific soybean genotype (reviewed in Yamada et al., 2012; Lee et al., 2013; Li et al., 2017; Table 1). Key elements of the progress are summarized in a later section. Main advantages of Agrobacterium transformation include relatively high ratio of single-copy gene insertion, relative simplicity of the transformation procedure, and low cost (Hwang et al., 2017). However, there is a limitation in delivery of genetic material. It delivers DNA plasmids but cannot deliver DNA fragments, RNAs, or proteins.
Biolistic Transformation
Biolistic transformation, known as gene gun or particle bombardment, delivers small tungsten or gold particles coated with desired genes to target plant cells (Christou et al., 1988). Since an electrical-discharge gene gun was first used in soybean to regenerate a fertile transgenic plant (Mccabe et al., 1988), gene delivery to meristematic soybean cells by particle bombardment has been considered to be more genotype-flexible for transfer of foreign DNA into soybean (Homrich et al., 2012). Recently, embryogenic callus based biolistic method becomes more popular due to its relatively higher efficiency compared to other explants and its directly delivering way which meets the need for genome editing using RNA and RNPs editing reagents for recovery of DNA-free edited events. In comparison to the A. tumefaciens-mediated method, the biolistic method offers benefits with their capacity to transform organelles and deliver RNA, proteins, nanoparticles, dyes, and complexes to cells (Klein et al., 1987; Liang et al., 2017). The drawback is mainly high transgene copy and relatively high cost, and its application has been restricted in limited soybean genotypes because of unavailable meristematic explants. Compared to plasmid bombardment, utilization of specific constructs including linear minimal expression cassettes (MECs) in biolistic transformation enables the production of plants carrying much simpler patterns of transgene integration, which has been confirmed in plants such as wheat (Ismagul et al., 2018). The major progress in soybean biolistic transformation is presented in a later section and summarized in Table 1.
Other Stable Transformation Methods
A. rhizogenes-Mediated Transformation
Transgenic plants can also be produced by regeneration of hairy roots transformed with A. rhizogenes. Success of stable transformation has been reported in many plant species (Christey, 2001). In soybean, stable soybean transgenic plants were produced from hairy roots using primary-node explants infected by a disarmed A. rhizogenes strain SHA17 (Olhoft et al., 2007) and the several reports of targeted mutation events using genome editing also have been obtained from hairy roots through A. rhizogenes-mediated transformation (Curtin et al., 2011; Haun et al., 2014; Demorest et al., 2016). However, genotype inflexibility has been the main hurdle for using the method in soybean.
In-Planta Gene Transformation
This is an alternative method in which Agrobacterium is used to infect explants, but it does not involve in vitro culture and regeneration of plant cells or tissues (Kalbande and Patil, 2016), thereby reducing time and labor cost, and, most importantly, avoiding somaclonal variation occurrence during in vitro culture-mediated genetic transformation and regeneration. In soybean, an Agrobacterium suspension is directly injected into the ovary (Liu et al., 2009), axillary meristematic region of germinated seedling (Chee et al., 1989), or stigma in which exogenous DNA was introduced into cells via the “pollen-tube-pathway” (Hu and Wang, 1999). Transgenic events could be obtained from progeny seeds. Liu et al. (2009) reported the transfer of a minimal linear marker-free and vector-free smGFP cassette into soybean by pollen tube-mediated gene transfer. Mangena (2019) summarized the progress made in in planta transformation and formulated a simple protocol using in planta Agrobacterium injection of seedlings. Although this could be a tissue culture bypass method and attempts for new ways are made from time to time, its efficiency has been very low and it is often not repeatable. This method has not been widely used.
Transformation Using a New Bacterium
Recently a novel bacterium, Ochrobactrum haywardense H1 (Oh H1), was discovered and it is capable of efficient plant transformation (Cho et al., 2022). Ochrobactrum is able to host for Agrobacterium-derived vir and T-DNA and helps to deliver transgenes in soybean. Oh H1-8 generated high-quality transgenic events by single-copy, plasmid backbone-free insertion at frequencies higher than those of Agrobacterium strains. It achieved high transformation efficiency in several soybean genotypes, which can be up to 35%. The application of the new bacterium-mediated transformation in soybean needs to be evaluated further.
Progress Made to Improve Soybean Transformation Over the Last Decade
Since 2010, increasing the transformation frequency (TF) has been the main focus for soybean transformation improvement. Several major factors affecting soybean TF based on Agrobacterium-mediated transformation have been identified, and progress has been made in establishing a high-throughput transformation system (Zhang et al., 2014; Arun et al., 2015, 2016; Yang X. F. et al., 2016; Li et al., 2017; Chen et al., 2018b; Karthik et al., 2020; Pareddy et al., 2020). Some confirmed positive elements in Agrobacterium-mediated transformation protocols have also been applied for enhancing soybean biolistic transformation (Table 1).
Agrobacterium-Mediated Transformation
Soybean transgenic plant production still relies on Agrobacterium-mediated transformation (Figure 2 and Table 1). Recently, high TFs of over 10% have been obtained in more and more soybean genotypes using improved protocols (Zhang et al., 2014; Arun et al., 2015, 2016; Yang X. F. et al., 2016; Li et al., 2017; Chen et al., 2018b; Karthik et al., 2020; Pareddy et al., 2020). The enhancement of TF is based on changes in several factors, including explant, selectable marker, and culture medium composition such as antioxidants, of these protocols (Table 1).
Adjustment of Infection Method and Improving Regeneration
Reducing the explant tissue browning and necrosis caused by Agrobacterium enhances construct delivery and regeneration of transformed cells. Changing the ways for preparation of Agrobacterium infection solutions and co-cultivation media, and modifying infection methods can achieve this goal and eventually increase transformation efficiency. Addition of antioxidants such as dithiothreitol (DTT) in infection solutions and extending co-cultivation time to 5 days achieved an infection efficiency of more than 96% and, hence, increased TF (Li et al., 2017). Infection solutions prepared with a two-round overnight culture of Agrobacterium using AB minimal media in second round culture significantly increased transformation frequency in comparison with the culture using normal YEP medium (Pareddy et al., 2020). It was also found to be beneficial to A. tumefaciens infection when the co-cultivation temperature for soybean transformation was set to 23°C under dim light (Yang X. F. et al., 2016). The same group also demonstrated to alleviate explant necrosis and significantly improve the transformation efficiency when antioxidants alone such as α-lipoic acid (α-LA, 0.12 mM) and silver nitrate (AgNO3, 20 μM), or combinations of antioxidants such as L-cysteine (1 mM) + DTT (3.3 mM) + AgNO3 (20 μM), and L-cysteine (1 mM) + DTT (3.3 mM), were added in the solid co-cultivation medium. For improving regeneration, it was found that adding 6-benzylaminopurine (BAP) in a germinating medium could significantly increase regeneration efficiency, which led to enhancement of TF; the optimal BAP concentration for shoot formation was 0.5 mg/L (Zhang et al., 2014). More examples are presented in Table 1.
Genotype Effect and Explant Choice
In the tissue culture-based transformation process, the composition of culture media and susceptibility of selected explants to Agrobacterium influence soybean transgenic frequency. A highly efficient in vitro culturing system and regeneration of cells susceptible to Agrobacterium are prerequisites for a reliable transformation protocol. Until now, the TF for most tested genotypes of soybean has remained quite low at a level mostly below 5% when conducting Agrobacterium-mediated transformation [summarized in Yamada et al. (2012); Jia et al. (2015), and Li et al. (2017); Table 1]. Since 2000, many research groups have used model soybean varieties such as Jack, Bert, and Williams serials and other specific genotypes because of their amenability to transformation (Olhoft and Somers, 2001; Olhoft et al., 2001, 2003; Paz et al., 2004, 2006; Zeng et al., 2004; Luth et al., 2015). Recently, soybean transformations with high TFs have been reported using specific genotypes. For example, it was claimed 23.1% with Zhonghuang13 (Zhang et al., 2014) and an average of 14% TF for a local Indian genotype, DS-9712 (Hada et al., 2018). Improvement based on Agrobacterium-mediated soybean transformation has been made to expand target genotypes from conventional model varieties to many elite varieties (Ko et al., 2003; Yi and Yu, 2006; Sato et al., 2007; Song et al., 2013; Arun et al., 2015; Pareddy et al., 2020). For example, over 5% TF for more than 10 varieties was achieved with a robust protocol (Pareddy et al., 2020).
Since Hinchee et al. (1988) obtained transgenic events, the cotyledonary node of mature seeds has been the most favorite explant used for Agrobacterium-mediated soybean transformation using many other explants such as embryonic tips and calli (Figure 3). Cotyledonary node regions have axillary meristems at the junction between cotyledon and hypocotyl, which can proliferate and regenerate by the formation of multiple adventitious shoots on a culture medium containing cytokinin. Successful transformation has been achieved using similar organogenesis from various explants, which include germination seeds (Chee et al., 1989), embryonic shoot tips (Martinell et al., 2002; Liu et al., 2004), cotyledonary nodes from immature seeds (Parrott et al., 1989; Yan et al., 2000; Ko et al., 2003), cotyledonary nodes from mature seeds (Meurer et al., 1998; Zhang et al., 1999; Donaldson and Simmonds, 2000; Olhoft and Somers, 2001; Olhoft et al., 2001; Paz et al., 2004; Zeng et al., 2004; Liu et al., 2008), half-seeds (Paz et al., 2006; Pareddy et al., 2020), whole cotyledonary nodes (Zhang et al., 2014) and hypocotyls (Dan and Reichert, 1998; Liu et al., 2004; Wang and Xu, 2008), and other explants with different regeneration procedures such as calli induced from geminated seedlings (Hong et al., 2007) and embryogenic suspension cultures (Trick and Finer, 1998). However, successful and repeatable production of transgenic soybean via Agrobacterium-mediated transformation has mainly been based on protocols with explants containing cotyledonary nodes from young seedlings and imbibed mature seeds (Zhang et al., 1999; Olhoft et al., 2003; Paz et al., 2006). Recently, half-seeds have gradually become the trend for explants since (Paz et al., 2006) their first use, because half-seed explants possess advantages to have more nutrition supply for shoot regeneration compared to cotyledonary nodes and to be prepared within a short time (less than 1 day) due to using imbibed seeds, which reduces the period of total regeneration and labor cost. Based on descriptions of explants in several reports (Paz et al., 2006; Pareddy et al., 2020), half-seed, whole cotyledon, and split seed explants can now be put under the same category of half-seed explants. Obtaining TFs of over 10% for soybean with half-seed explants have been demonstrated in many reports (Zhang et al., 2014; Arun et al., 2016; Li et al., 2017; Chen et al., 2018b; Hada et al., 2018) (Table 1). The highest TF of 34.6% has been obtained using these explants together with nitric oxide treatment in a co-cultivation medium in the protocol made by Karthik et al. (2020). Some specific explant treatments such as sonication in combination with vacuum infiltration, sonication in combination with surfactant, or just sonication (Mariashibu et al., 2013; Arun et al., 2015; Guo et al., 2015; Zhang et al., 2016; Hada et al., 2018), and pre-wounding with a multi-needle consisting of 30 thin fibers (Xue et al., 2006) or a micro-brush (Yamada et al., 2010) were also used before Agrobacterium infection to increase infection rate and TFs, because these treatments facilitate the penetration of Agrobacterium into plant tissues and increase the contact between plant cells and the bacterium, and stimulate the infection ability of the bacterium, which leads to T-DNA transfer into plant cells.
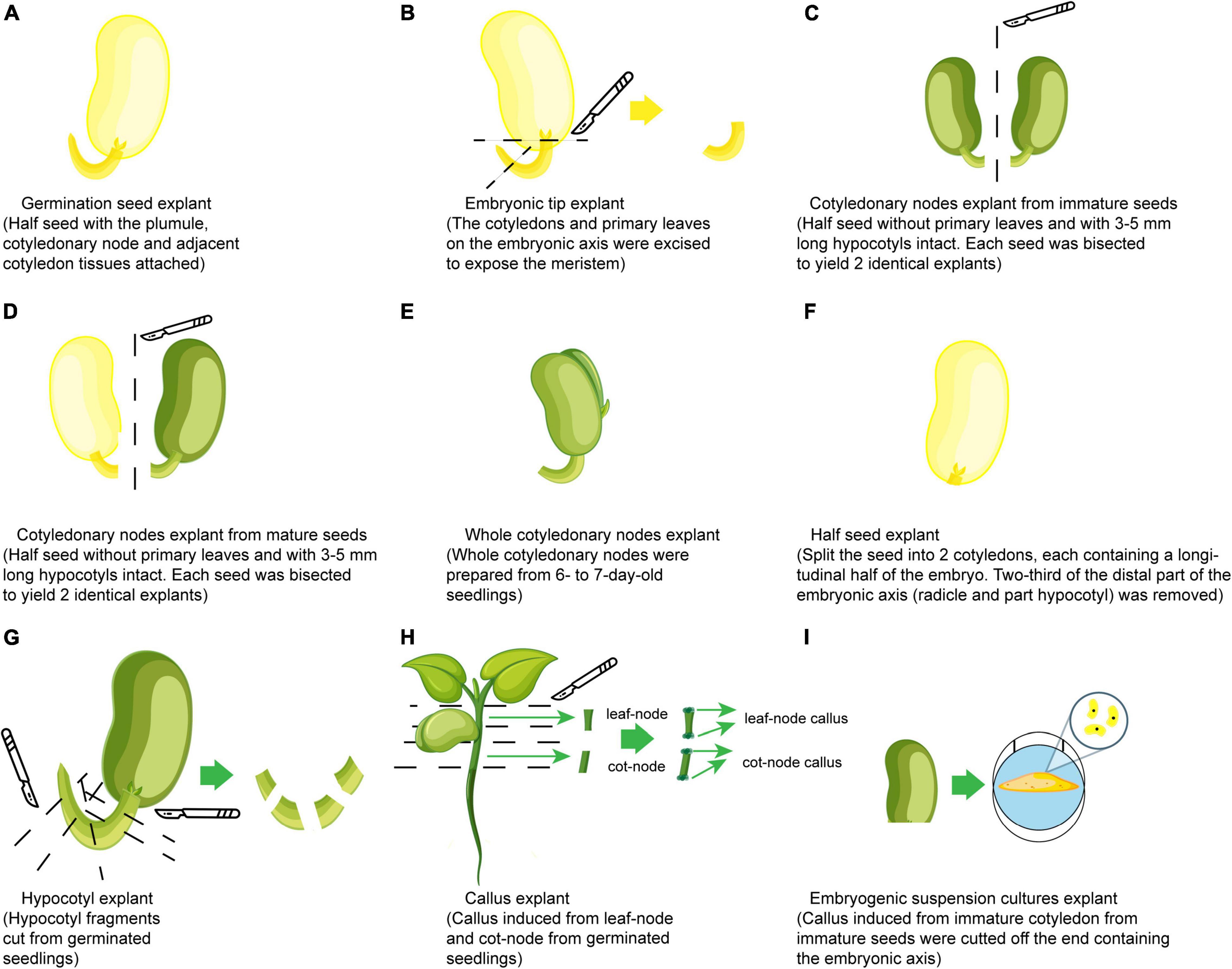
Figure 3. Types of explants used for soybean transformation. (A) Germination seed. (B) Embryonic shoot tips. (C) Cotyledonary nodes from immature seeds. (D) Cotyledonary nodes from mature seeds (yellow cotyledon means use cotyledon directly from mature seed; the green one means use mature cotyledon after germination under light). (E) Whole cotyledonary node. (F) Half-seed. (G) Hypocotyl. (H) Callus induced from geminated seedlings. (I) Embryogenic suspension cultures.
Addition of Antioxidants in Medium
Antioxidants, in general, are known to reduce pathogen-induced programed cell death (Mittler et al., 1999). These include inhibitors of polyphenol oxidases (PPOs) and peroxidases (PODs) through the action of their thiol group, such as compounds L-cysteine, DTT, and sodium thiosulfate. They are commonly used to reduce enzymatic browning in food processing caused by deposition of tannins (Nicolas et al., 1994; Ghidelli et al., 2014). Polyvinylpyrrolidone (PVP), DTT, L-cysteine, glutathione, α-LA, L-ascorbic acid, and citric acid have been confirmed to decrease tissue necrosis of explants used for Agrobacterium-mediated transformation (Barampuram and Zhang, 2011). Either one or more than 2 of the chemicals have been used in soybean transformation (Olhoft and Somers, 2001; Olhoft et al., 2003; Paz et al., 2004; Yi and Yu, 2006; Liu et al., 2008). L-cysteine and DTT have been frequently used in soybean transformation since its first use by Olhoft et al. (2001). Reports clearly showed that there was less browning on the cut and damaged surfaces of the hypocotyl, cotyledon node region, and on the cotyledon of explants, which increased the TF of stable transformations (Olhoft et al., 2001, 2003; Paz et al., 2004; Li et al., 2017). A high average TF of 12.7% resulted from the combination of L-cysteine and DTT, which was significantly greater than that of either L-cysteine or DTT alone (Olhoft et al., 2003). The positive effect has been continuously confirmed in recent reports (Table 1). Another type of antioxidants is a group of sulfur-containing compounds involved in several multienzyme complexes such as α-LA. These include pyruvate dehydrogenase, α-ketoglutarte dehydrogenase, branched-chain ketoacid dehydrogenase, and glycine decarboxylase (Dan et al., 2009). Adding the antioxidant α-LA in a co-cultivation medium could increase transient GUS expression and increased the percentage of shoot induction (Yang X. F. et al., 2016). In this report, 0.12 mM α-LA was found to be the most useful for alleviating browning and necrosis. Other antioxidants conventionally used in plant tissue culture, such as ascorbic acid, PVP, and citric acid, may promote soybean transformation efficiency, but their roles have not yet been made clear (Li et al., 2017). Plant hormone-like antioxidants such as sodium nitroprusside (SNP), a nitric oxide (NO) donor, play varied roles in growth and development of plants. Nitric oxide is involved in cell metabolism and morphogenesis and acts as a signaling molecule in response to various biotic and abiotic stresses (Verma et al., 2020), and can alleviate abiotic stress threat in plants reacting quickly with ROS. SNP significantly enhanced regeneration and development rate of soybean plants (Karthik et al., 2019); addition of SNP also significantly increased soybean TF by up to 34.6% with the half-seed method (Karthik et al., 2020).
Addition of Other Chemicals in Culture Medium
Except for antibiotics, chemicals related to host defense response, ethylene inhibitors, surfactants, demethylating reagents, polyamines, and antagonist α-aminooxyacetic acid (AOA) are proved to have a positive effect on improving TF. L-glutamine and L-asparagine are types of chemicals that weaken host defense responses. It has been reported that the addition of L-glutamine into a culture medium alone or in combination with a cold shock pretreatment could enhance Agrobacterium transformation efficiency (Zhang et al., 2013). Although the mechanism is still not clear, L-glutamine could play a role in lessening host defense responses by attenuating the expression of certain pathogenesis-related genes (PRs), and potentially improve the efficiency of Agrobacterium-mediated plant transformation (Zhang et al., 2013, 2014). It was demonstrated that TF was significantly increased in soybean when additional L-glutamine or L-asparagine alone, or both of them were added in all culture media (Chen et al., 2018b). The TF was 8.8 ± 1.5 (L-glutamine), 5.9 ± 2.1% (L-asparagine), 11 ± 0 (both), and 3.5 ± 2.4% (without any one of them). Ethylene inhibitors such as AgNO3 have a positive effect on transformation. It has been reported that Ag+ interferes with the binding of ethylene receptor sites and helps reduce ethylene production by promotion of polyamine biosynthesis (Roustan et al., 1990). The main function of AgNO3 is to eliminate the potential danger to plant cells and tissues in liverwort caused by ethylene (Beyer, 1979). It has already been confirmed to promote somatic embryo production and shoot regeneration in wheat and maize (Carvalho et al., 1997; Fernandez et al., 1999). This effect has been proved to improve soybean TF (Olhoft et al., 2004; Li et al., 2017). A nearly 10% TF with genotype Heilong44 was reported when BAP and AgNO3 were added into a culture medium (Wang and Xu, 2008). Surfactants such as SilwetL-77 and pluronic acid F68 also increase TF, which initially showed to enhance T-DNA delivery in wheat Agrobacterium-mediated transformation when added into an inoculation medium (Cheng et al., 1997). This was also confirmed in soybean transformation. It was reported that adding SilwetL-77 to an infection medium coupled with hygromycin-based selection strategies led to transformation efficiencies ranging from 3.8 to 11.7% in Chinese soybean varieties (Liu et al., 2008). SilwetL-77 has been frequently used to increase soybean TF (Yamada et al., 2010; Guo et al., 2015). Surfactants may enhance T-DNA delivery by aiding A. tumefaciens attachment and/or by elimination of certain substances that inhibit A. tumefaciens attachment (Opabode, 2006). Polyamines enhance plant cell differentiation, induce totipotency, and increase cell division (Rakesh et al., 2021). Addition of polyamines in the plant transformation process leads to vir gene induction and T-DNA transfer, and increases transformation efficiency (Kumar and Rajam, 2005). As high as 29.3% TF in soybean has been achieved by addition of spermidine, spermine, and putrescine in a culture medium compared with its counterparts (14.6%) and with respective plant growth regulator (PGR) alone (Arun et al., 2016). Demethylating reagents commonly applied in epigenetic research such as 5-azacytidine (5-Azac), significantly improve the transient transfection efficiency and transgene expression level in low-efficiency genotypes. Treatment with 5-Azac improved the shoot regeneration efficiency in low-efficiency genotypes during the process of Agrobacterium-mediated soybean transformation. This indicates that lower methylation level in transgenes contributed to enhance shoot regeneration in Agrobacterium-mediated soybean transformation (Zhao et al., 2019b). Antagonist AOA relieves the structural membrane barriers of Agrobacterium entering cells, hinders the perception of intercellular signal transmission, and thus effectively alleviates defense responses and increases the susceptibility of cells to Agrobacterium infection. Combined use of AOA and sonication treatments (novel method) greatly improved T-DNA delivery efficiency in soybean (Zhang et al., 2015, 2016).
Refining Selection Agents
The most frequently used selectable markers in both the somatic embryogenesis- and organogenesis-based soybean transformation methods are genes conferring resistance to herbicides or antibiotics so as to reduce escape rate significantly. The selectable markers include bar and pat genes conferring resistance to phosphinothricin, the active ingredient in BASTA and bialaphos herbicides (Zhang et al., 1999; Olhoft and Somers, 2001; Olhoft et al., 2001; Paz et al., 2004; Testroet et al., 2017; Pareddy et al., 2020), EPSPS (5-enolpyruvylshikimate-3-phosphate synthase) genes conferring resistance to the herbicide glyphosate (Martinell et al., 1999; Clemente et al., 2000; Yao, 2001; Guo et al., 2015; Xiao et al., 2019), and the nptII gene conferring resistance to the antibiotics kanamycin (Homrich et al., 2012) and hph or hpt II (hygromycin phosphotransferase) genes conferring resistance to hygromycin B (Yan et al., 2000; Ko et al., 2003; Olhoft et al., 2003; Liu et al., 2008). Recently, Hph and Bar or Pat have been proven to be the most favorite selectable markers (Table 1). An average transformation frequency as high as 29.3% was achieved with the half-seed (Arun et al., 2016) and 13.3–18.6% with cotyledonary node explant (Arun et al., 2015) employing hygromycin B selection, which is better than or comparable with that by Olhoft et al. (2003). Glufosinate has also been used as a selection agent based on the bar or the pat gene and initially had less than 10% TF in soybean transformations involving the half-seed (Paz et al., 2006) and embryo tip (Dang and Wei, 2007) explants. Recently TFs of over 10% have been obtained with cotyledonary nodes (Hada et al., 2014; Yang X. F. et al., 2016) and half-seeds (Li et al., 2017; Chen et al., 2018b; Pareddy et al., 2020). A 34.6% TF was reported using a protocol with addition of sodium nitroprusside (SNP) when Basta was sprayed for selection (Karthik et al., 2020). Since an epsps/glyphosate selection based protocol is established (Martinell et al., 1999), glyphosate has gradually been incorporated into transformation as a selectable agent and has shown its beneficial side for high stringency. In order to quickly and efficiently screen glyphosate-tolerant events, a rapid and convenient spotting method was established for screening regenerated glyphosate-tolerant T0 plantlets (Guo et al., 2020a). In this report, an optimized Agrobacterium-mediated soybean transformation system with rapid and effective selection of transformed cells was developed, with TFs ranging from 2.9 to 5.6%. Especially, 96% regenerated T0 plantlets showed clear tolerance to glyphosate and their transgenic nature were confirmed by molecular analysis. Spectinomycin was also used as a selective agent to obtain transgenic soybean when the aminoglycoside-3′′-adenylyltransferase gene (aadA) was used as a selectable marker (Martinell et al., 2002). The spectinomycin selection protocol demonstrated higher frequency of transformation, a shorter period of time needed to complete each protocol, and lower cost compared with the glyphosate selective protocol. Soybean transformation using a GFP as a detection marker was also reported, and transgenic plants could be identified at an early stage, although the frequency was not high (2.5%) (Yang S. et al., 2019). Combined with a normal selectable marker and a selection agent, GmFAST (fluorescence-accumulating seed technology) has recently been developed to identify homologous transgenic seeds. It is a marker composed of a soybean seed-specific promoter coupled to the OLE1-GFP gene, which encodes a GFP fusion of the oil-body membrane protein OLEOSIN1 of Arabidopsis thaliana and is a time-saving and efficient method to produce homologous transgenic events (Iwabuchi et al., 2020).
Generally, the efficiency of Agrobacterium-mediated transformation in soybean has been enhanced by improving both Agrobacterium infection and explant regeneration. Addition of antioxidants such as DDT, L-cysteine, and NO in a co-cultivation medium and some infection-assisting specific chemicals including surfactants and AgNO3, and some regeneration-promoting elements such as polyamines (Table 1), plays an important role in the improvement. All of the measures have facilitated Agrobacterium to transform the meristematic region of soybean explants. Recently, a specific Agrobacterium-mediated protocol was reported, which conducted bombardment to make wounding and reduce the lab work time to only 2 days in the transformation process and kept the rest time to grow T0 plants in a glasshouse (Paes de Melo et al., 2020). Transgenic events were screened using a swab to spread glufosinate solution on leaves of putative events and the TF reached nearly 10%. This method avoided many tissue culture steps and may be a cost-saving protocol.
Biolistic Transformation
Since Mccabe et al. (1988) reported the first transgenic soybean plant using the biolistic method, many reports of soybean biolistic transformation have been published and the development of this method in the first 25 years has been reviewed by Homrich et al. (2012); Lee et al. (2013), Mariashibu et al. (2013), and Mangena et al. (2017). Initially, meristems of soybean tissues as the target tissue were used for bombardment such as embryonic axes of immature and mature seeds (Sato et al., 1993; Aragão et al., 2000; Rech et al., 2008; Soto et al., 2017). In later studies, somatic embryos (Finer and Mcmullen, 1991; Finer and Larkin, 2008; Finer, 2016) were the most frequently used explants for biolistic transformation. However, chimeric transgenic plants were produced because of multiple cell layers (L1, L2, and L3) in the original apical meristem of soybean (Christou, 1990; Christou and Mccabe, 1992). Fortunately, using secondary somatic embryos and new selective markers such as EPSPS has eliminated transgenic chimeras (Sato et al., 1993; Martinell et al., 1999). Somatic embryo regeneration and proliferation were initiated either on semi-solid media (Parrott et al., 1989) or liquid suspension culture media (Finer and Nagasawa, 1988). Co-transformation of multiple plasmids or multiple gene inserts in same constructs with selectable markers has been achieved (Hadi et al., 1996; Li et al., 2015). Since 2010, factors such as explant type, abiotic stress treatment, selectable marker, and tissue culture method have been the main focus to improve biolistic transformation TF, and reliable protocols for the biolistic method with embryogenesis-based explant have been developed to produce a reasonable number of transgenic plants (Table 1). For example, a TF of up to 6% was achieved with cp4epsps as selectable marker when embryonic axes of mature seeds of the INCASoy-36 Cuban cultivar were bombarded (Soto et al., 2017). Chennareddy et al. (2018) combined an immature half-seed explant with an intact embryonic axis, cold and plasmolysis pre-treatment, and a specific somatic embryogenic callus regeneration medium in their protocol. They achieved 5% TF with HPH/hygromycin selection and 2.7% with DSM2/glufosinate selection. A selection system using NPTII/G418 was developed for a biolistic-transformed embryogenic callus rather than the most used HPH/hygromycin system and similar TF in comparison with the HPH system was obtained (Itaya et al., 2018). The current status is that soybean biolistic transformation still relies on an embryogenic callus, since it is the prerequisite for establishing a robust transformation system for a specific genotype. Selection for the amenability of an embryogenic callus induced from local elite varieties (genotypes) is the main focus (Joyner et al., 2010; Abbasi et al., 2016; Islam et al., 2017; Raza et al., 2020). An improved biolistic soybean transformation protocol was published using an embryogenic callus induced from an immature cotyledon explant (Finer, 2016), which is a robust one and can produce quite a lot of transgenic plants within 6–9 months.
Recent Applications of Soybean Transformation for Trait Improvement
Transgenic technology has been used to improve soybean agronomic traits, which include yield component, grain quality, and biotic and abiotic stress tolerance, and economic traits such as oil and biofuel quality, and specific chemical content in seed for human health, and other traits. Trait improvements through forward and reverse genetic approaches in the last 5 years are summarized in Table 2; i.e., downregulation of the pyruvate dehydrogenase kinase gene GmPDHK through RNAi made an average of 42.2% protein content in seeds of transgenic plants, which is significantly increased compared with the non-transgenic control (Jones et al., 2020). Soybean seeds with linolenic acid content in excess of 50% of the total oil have been generated by increasing the expression of the FAD3 gene, which encodes the enzyme that converts linoleic acid to linolenic acid (Yeom et al., 2020). Overexpressing the GmmiR156b (Squamosa promoter-binding protein-like, SPL) gene in soybean and transgenic plants produced significantly increased numbers of long branches, nodes, and pods that exhibited increased 100-seed weight, resulting in a 46–63% increase in yield per plant and no significant impact on plant height in a growth room or under field conditions (Sun et al., 2019). Stable GmMYB14-overexpressing (GmMYB14-OE) transgenic soybean plants demonstrate semi-dwarfism and a compact plant architecture associated with decreased cell size, causing decreased plant height, internode length, leaf area, leaf petiole length, and leaf petiole angle, and improved yield in high density and drought tolerance under field conditions (Chen et al., 2021b). Salt-tolerant transgenic soybean and its applications in field are summarized in a review (Cao et al., 2018). Resistance to soybean cyst nematode (SCN; Heterodera glycines) in stably transformed soybean plants is enhanced by downregulation of the HgY25 and HgPrp17 genes, which are related to reproduction and fitness (Tian et al., 2019). Overexpression of PAC1 and GmKR3, a TIR–NBS–LRR-type R gene, can increase multiple virus resistance in transgenic soybean and, thus, provide an efficient control strategy against RNA viruses such as SMV, BCMV, WMV, and BPMV (Xun et al., 2019). Overexpression of GmDR1 [Glycine max disease resistance 1 (Glyma.10G094800)] led to enhanced resistance not only against F. virguliforme but also against spider mites (Tetranychus urticae, Koch), soybean aphids (Aphis glycines, Matsumura), and SCN (Ngaki et al., 2021). Many types of herbicide-resistant transgenic soybean, such as glyphosate-resistant, dicamba-, and 2,4-D-resistant, are grown widely in the United States (Nandula, 2019). Transgenic soybean plays an important role in soybean production worldwide now, and transgenic soybean covers 50% of the global transgenic crop area, occupying 94.1 million ha (Nandula, 2019). Therefore, a better soybean transformation system is the base for soybean improvement through transgenic technology.
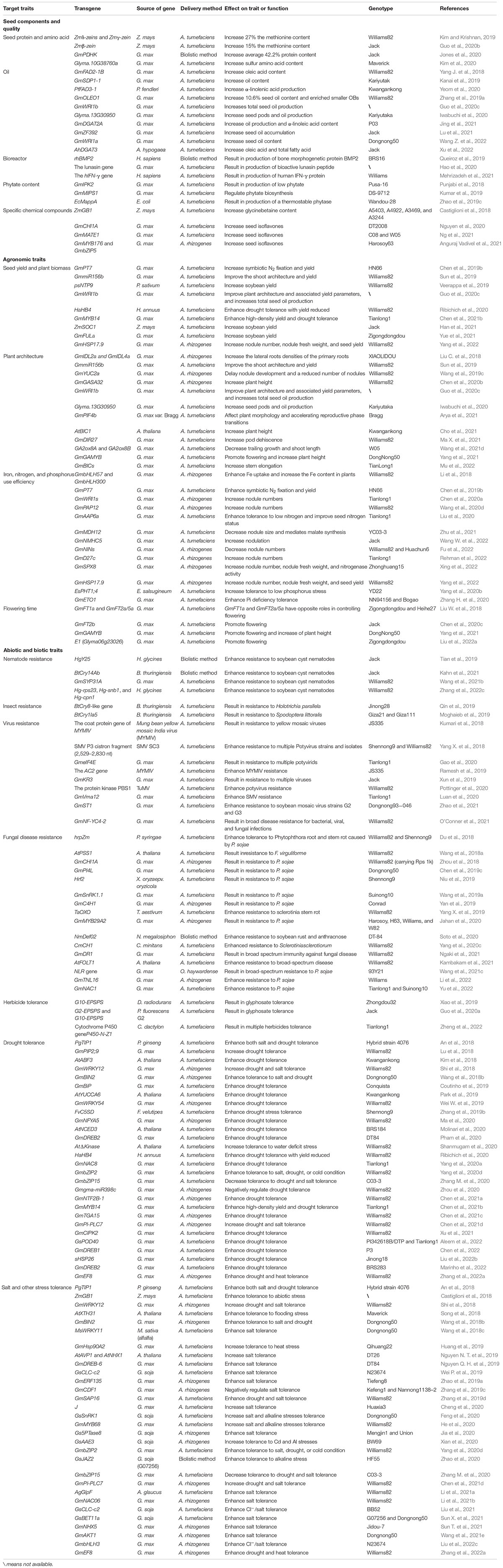
Table 2. Summary of transgenic approaches for soybean trait improvement and functional genomics in the last 5 years.
Challenges and Future Directions in Soybean Transformation
Although much effort has been made to improve the transformation systems for soybean, there are some challenges such as genotype flexibility, low transformation frequency, time to time chimerism in T0 transgenic plants, and availability of a system for new breeding technologies such as genome editing.
Genotype Flexibility
Like in other recalcitrant plant species, genotype inflexibility has been an obstacle that restricted the scope of soybean transformation. The ideal soybean transformation target material for trait improvement would be any elite variety with excellent agronomic characteristics. However, most reliable transformations are still based on specific genotypes although genotypes amenable to transformation have expanded to some preferred genotypes. For example, in the early stage, successful Agrobacterium-mediated transformation occurred in several genotypes and their derivatives such as Williams, Williams79, and Williams82 (Paz et al., 2004, 2006). High-efficiency Agrobacterium-mediated transformation is only achieved in a limited number of elite lines (Zhang et al., 2014; Arun et al., 2015, 2016; Yang J. et al., 2016; Li et al., 2017; Chen et al., 2018b). High-efficiency genotypes possess greater susceptibility to Agrobacterium infection, which has been confirmed in many reports (Jia et al., 2015; Yang J. et al., 2016; Yang X. F. et al., 2016; Zhao et al., 2019b). The competency of cotyledons of seeds to Agrobacterium infection and the ability to regenerate plants are key factors. These may be determined by cell defense response, including attachment of A. tumefaciens to plant cells, plant signals sensed by A. tumefaciens, regulating vir gene expression, T-DNA/virulence protein transport or initial contact of A. tumefaciens to plants and cytoplasmic trafficking, and nuclear import of T-DNA and effector proteins (Hwang et al., 2017). An important step to enhance the transformation efficiency of recalcitrant genotypes is to improve the genotypes’ susceptibility to Agrobacterium infection. Many commonly used treatments to increase transformation efficiency such as heat shock, cold shock, antioxidants, and hypoxia may act by suppression of cellular response to Agrobacterium infection (Zhang et al., 2013). Combinations of various positive factors discovered or developed recently have promoted Agrobacterium-mediated soybean transformation to extend genotype scope (Table 1). For example, transgenic events have been obtained from 19 out of 20 genotypes based on an improved protocol (Pareddy et al., 2020) and 7 out of 8 genotypes (Zhao et al., 2019b). One important progress in these reports is that over 5% of TFs were obtained in nearly half of these genotypes. The second factor that affects genotype flexibility is the regeneration ability of donor genotypes, which restricts TFs for both Agrobacterium-mediated and biolistic transformations. Increasing the amenability of many soybean genotypes to regenerate may be conducted by either adding some specific chemicals in the culture medium described above or using plant regeneration factors or regeneration booster genes. Significant progress has been made to improve transformations from various tissue types using plant regeneration factors such as maize (Zea mays) morphogenic genes, Baby boom (BBM) and Wuschel2 (WUS2) genes in maize plant (Lowe et al., 2016), and plant growth regulators such as GROWTH-REGULATING FACTORS (GRF) genes used in monocot and dicot species including soybean (Gordon-Kamm et al., 2019; Debernardi et al., 2020; Kong et al., 2020). Use of these genes significantly increased transformation frequency and reduced genotype obstacle for transformation, providing a good solution for genotype-inflexibility bottleneck in transformation of crops including soybean. For example, introducing AtGRF5 and GRF5 orthologs into soybean cells could improve regeneration and, hence, increase transformation TFs significantly (Kong et al., 2020). GRFs can also enhance shoot organogenesis and callus regeneration, which has been confirmed in dicots including sugar beet, canola, and sunflower. Meanwhile, somatic embryogenesis can be promoted using some genes introduced into explants in soybean, such as soybean orthologs of the Arabidopsis (A. thaliana) MADS box genes AGAMOUS-Like15 (GmAGL15) and GmAGL18, which can also expand soybean genotypes suitable for transformation, especially for biolistic transformation (Zheng and Perry, 2014). Transformation bypass tissue culture such as in planta transformation is an alternative way to overcome genotype inflexibility in soybean (Liu et al., 2009; Mangena, 2019). Nanotechnology-based transformation can also be employed to overcome host range limitation including genotype inflexibility, and can simplify delivery way using pollen channel, and highly increase efficiency (Wang and Zhao, 2019). By integration of multiple-omics technologies, genes related to transformation efficiency should be discovered for increasing transformation efficiency. Use of the novel bacterium O. haywardense H1 may also increase the genotype scope for transformation, since it was claimed to be less genotype sensitive when it was used for soybean transformation (Cho et al., 2022).
Low Transformation Frequency
The average TF for varieties (genotypes) reported is lower than 5%, although improvements have been made by modifying the main factors described above (Table 1). Since the biolistic method tends to use an embryogenic callus as explant because of less chimerism compare to an embryo axis, TFs for biolistic transformation are dependent on the success of embryogenic callus induction for a specific genotype. Therefore, the main focus to improve TFs is to select genotypes that are amenable to embryogenic callus induction, or to stimulate a genotype to produce an embryogenic callus. As described above, the regeneration booster provides a new way to induce an embryogenic callus without genotype limitation, which has been confirmed in monocot plants (Lowe et al., 2018; Gordon-Kamm et al., 2019; Debernardi et al., 2020). Enhancement of TFs for soybean Agrobacterium-mediated transformation is mainly achieved by improving regeneration rates of explants and increasing the susceptibility of explants to Agrobacterium. Half-seed explants have been the major choice, because these explants could provide more nutrition and less damage than cotyledonary nodes (Table 1). Continuously modifying MS-based culture medium composition (Murashige and Skoog, 1962), especially by addition of chemicals discovered through the study of omics, has played a big role in TF improvement, and has been summarized in the section above. Combinations of many factors have promoted the TFs of soybean transformation (Table 1). More efforts should be made to increase the average TFs close to that of other major crops. Again, the morphogenic genes including GRFs described above may play an important role in enhancing soybean transformation frequency.
Chimerism in T0 Transgenic Plant
Chimerism in legume transformation is fairly common, which causes non-transmission of transgenes to subsequent generations either completely or at a lower ratio expected by Mendelian genetics. Therefore, minimizing chimerism in transgenic plants is required to obtain transmission of transgenes to the T1 generation. In soybean, Agrobacterium-mediated transformation of cotyledonary nodes by organogenesis has been extensively conducted for transgenic production in research and commercial product development (Barwale et al., 1986; Homrich et al., 2012; Yamada et al., 2012; Lee et al., 2013; Mariashibu et al., 2013; Mangena et al., 2017). Plant regeneration by organogenesis with an explant containing an embryo axis may be the main cause, since shoots regenerated from soybean shoot tips were derived from 3 superimposed cellular layers (L1, L2, and L3) in the original apical meristem (Christou, 1990; Christou and Mccabe, 1992). Transformed cells existed primarily in the L1 and L2 layers but not in the L3 layer of the apical meristems of regenerated shoots, indicating possible escape in the regenerated shoots during transformation, and this chimerism has been confirmed (Mccabe et al., 1988; Sato et al., 1993). Currently, the chimerism in transgenic soybean is still a major concern in the research community, and inheritance study has been always an important part in transformation protocol development (Pareddy et al., 2020). Improvement for reducing escapes or chimeric rate has been made when strict select stringency was used, especially some new selectable markers/reagents such as AHAS/imazapyr (Aragão et al., 2000; Rech et al., 2008), EPSPS/glyphosate (Martinell et al., 1999; Guo et al., 2015, 2020a; Soto et al., 2017), and AADA/spectinomycin (Martinell et al., 2002). Meanwhile, the modified protocols made use of specific explants, such as somatic embryogenic calli, to reduce the chance of infection with cells at the late development stage, and combined proper selection of chemical agents with high stringency to decrease escape rate dramatically, which led to more than 90% T0 transgenic plants transmitting their transgenes into T1 generation (Soto et al., 2017; Chennareddy et al., 2018; Guo et al., 2020a). Therefore, transgenic soybean with chimeric issues may due to insufficient selection that existed in various protocols.
Development of Transformation Method for New Breeding Technology
Genome editing is the recent advancement in genome engineering, which has revolutionized crop research and plant breeding. GE, through site-specific nucleases (SSNs), can precisely make changes in targeted genome sequence sites by disruption including insertion and deletion, base changes, sequence replacement, and sequence insertion. SSNs include zinc-finger nucleases (ZFNs), transcription activator-like effector nucleases (TALENs), and CRISPR/CAS. GE is a fast-developing technology that will potentially play an important role in genomics study and will create opportunities for rapid development of elite cultivars with desired traits. The development of soybean GE has been reviewed in Xu et al. (2020). Recent GE applications in soybean for trait improvement have been summarized in Table 3. For example, function analysis of photo period-related genes such as LHY homologs, J and E1, and tof 16 (Time of Flowering 16) using GE technology showed that more than 80% accessions in low latitude harbor the mutations of tof16 and J, which suggests that loss of functions of Tof16 and J is the major genetic basis of soybean adaptation into tropics. Therefore, maturity and yield traits can be quantitatively improved by modulating the genetic complexity of various alleles of LHY homologs, J, and E1 (Bu et al., 2021; Dong et al., 2021). The findings uncover the adaptation trajectory of soybean from its temperate origin to the tropics. Knockout of GmJAG1, which controls the number of seeds per pod (NSPP), increases by over 8% the yield of a Chinese variety, Huachun 6 (Cai et al., 2021). GmMs1 KO events in soybean were created, which showed male sterility phenotype (Jiang et al., 2021; Nadeem et al., 2021). SCN-resistant mechanisms such as t-SNAREs binding Rhg1 α-SNAP (Dong et al., 2020) and WI12Rhg1 interacting with DELLAs (Dong and Hudson, 2022) were found using GE as a tool. Targeted chromosome cleavage by CRISPR/Cas9 can conceivably induce rearrangements and, thus, emergence of new resistance gene paralogs. CRISPR/Cas9-mediated chromosome rearrangements in nucleotide-binding-site-leucine-rich-repeat (NBS-LRR) gene families of soybean produced a new disease-resistant gene (Nagy et al., 2021). Raffinose family oligosaccharides (RFOs) are major soluble carbohydrates in soybean seeds that cannot be digested by humans and other monogastric animals. Double mutation events, knockouts in two soybean galactinol synthase (GOLS) genes, GmGOLS1A and its homeolog GmGOLS1B, showed a reduction in the total RFO content of soybean seeds from 64.7 to 41.95 mg/g dry weight, a 35.2% decrease (Le et al., 2020). This product improved the soybean nutrition quality. Two transcription systems were also tested in soybean including the single transcriptional unit (STU), SpCas9 and sgRNA are driven by only one promoter, and in the conventional system, the two-component transcriptional unit (TCTU), SpCas9, is under the control of a pol II promoter, and sgRNAs are under the control of a pol III promoter. The results showed that the STU is more efficient (Carrijo et al., 2021). Cpf1 (Cas12a) systems have also been established in soybean for GE (Duan et al., 2021; Kim and Choi, 2021). Meanwhile, different GE systems for soybean have been established using specific editing reagent delivery methods developed for soybean transformation, which produce transgene-free GE events either with the biolistic method (Adachi et al., 2021) and selectable marker-free GT systems by O. haywardense H1-8-mediated delivery (Kumar et al., 2022), or by organ-specific editing using an egg cell-specific promoter (Zheng et al., 2020). All these GE studies on soybean demonstrate that the ability to conduct genome editing directly depends on plant transformation technologies, since recovery of stable events with the target gene edited is normally based on available transformation systems including editing reagent delivery and edited event regeneration. GE has the potential to avoid many regulatory issues regarding transgenics if specific editing reagents are used. Based on the CRISPR/CAS system, gRNA in the form of in vitro-synthesized RNA molecule, together with Cas9 as DNA construct, can be stably integrated into the host genome and constitutively expressed, which might lead to a transgenic event for a GE event. This issue can be resolved by introduction of editing tools without genomic integration or transient expression. Transgene-free or DNA-free edited events in many crops can now be obtained either by delivering the RNA form of sgRNA and Cas9 or Cas9 protein (RNP) using the biolistic method, or by protoplast transfection (Chen et al., 2019a; Xu et al., 2020; Gao, 2021; Kim and Choi, 2021). Transgene-free events can also be recovered with the Agrobacterium-mediated method without selection (Liang et al., 2017). However, genotype flexibility limitation is a major issue for soybean GE in the biolistic method, and low TF for some elite varieties is the main hurdle in the Agrobacterium-mediated method.
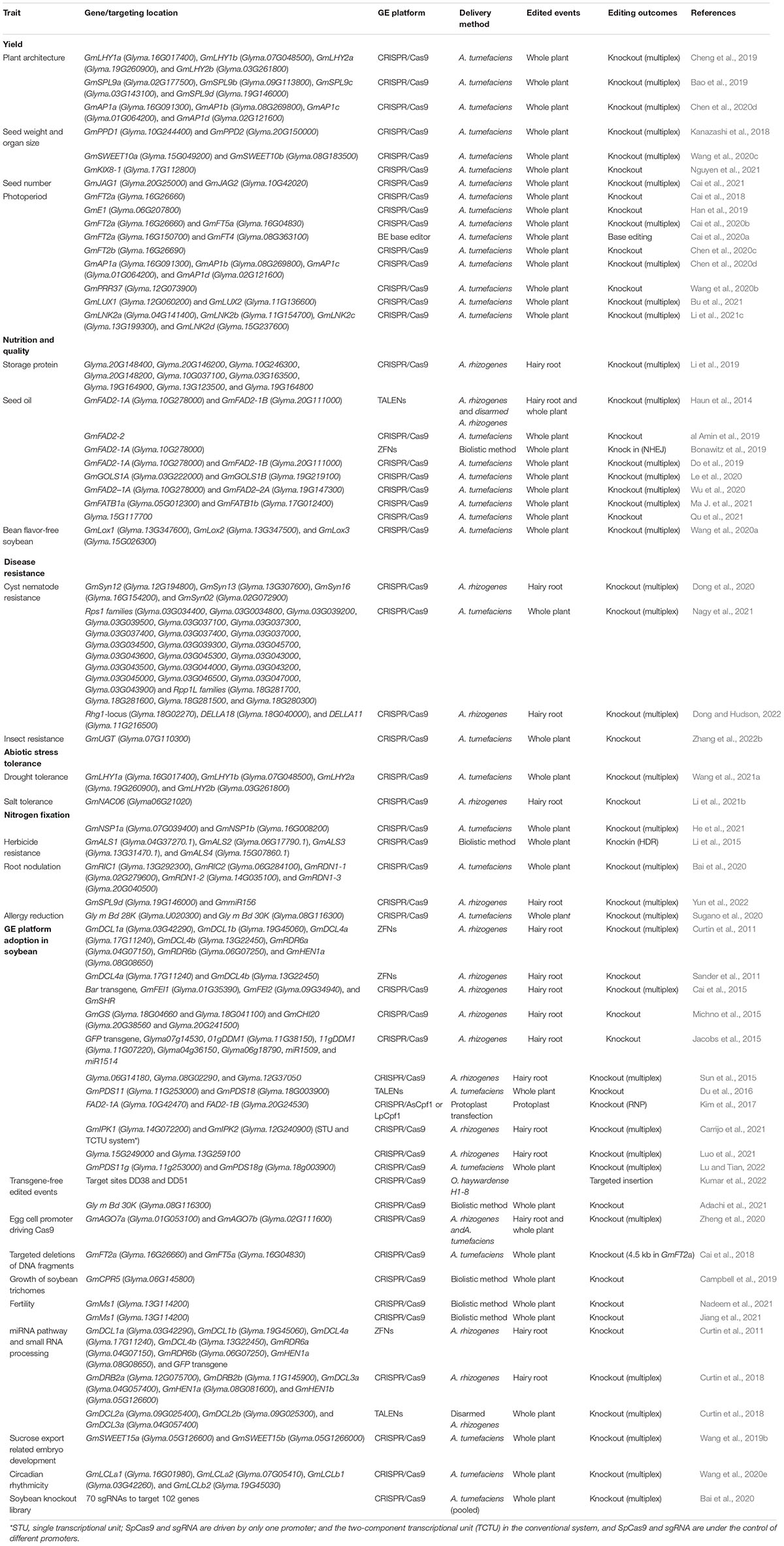
Table 3. List of soybean genes edited for functional genetics study and trait improvement using genome editing technology.
Conclusion
As summarized above, development of soybean transformation protocols, which pose genotype-flexibility and relatively high efficiency and can easily be adapted in any laboratory, is still a main task for researchers. Reducing biological restrictions such as genotype dependence or tissue-specific and method restrictions will eventually lead to transformation automation and versatile and high throughput, which will facilitate the application of next-generation breeding technologies such as genome editing for soybean improvement. These goals may be achieved with fast progress in fundamental research to unravel basic biological process and genetic background, especially when more regeneration regulators such as morphogenic genes are identified. Transgenic soybean in which various genes can be manipulated will accelerate the validation of gene function in the context of complex gene networks at different plant developmental stages, which will accelerate the understanding of the mechanism of soybean cell regeneration, and it is beneficial for us to modify transformation protocols. New technologies like nanoparticle delivery also bring us hope to break through these barriers as well as the transformation bypass method.
Author Contributions
HX and YG collected the materials. HX drew the figures and tables. YR wrote the manuscript. LQ and YR designed the article structure. All authors contributed to the article and approved the submitted version.
Acknowledgments
This study was supported by the National Transgenic Major Program of China (2016ZX08004001) and the Agricultural Science and Technology Innovation Program (ASTIP) of Chinese Academy of Agricultural Sciences.
Conflict of Interest
HX and YR were employed by Tianjin Genovo Biotechnology Co., Ltd.
The remaining authors declare that the research was conducted in the absence of any commercial or financial relationships that could be construed as a potential conflict of interest.
Publisher’s Note
All claims expressed in this article are solely those of the authors and do not necessarily represent those of their affiliated organizations, or those of the publisher, the editors and the reviewers. Any product that may be evaluated in this article, or claim that may be made by its manufacturer, is not guaranteed or endorsed by the publisher.
Footnotes
References
Abbasi, Z., Hooshyar, S., and Bagherieh-Najjar, M. B. (2016). Improvement of callus production and shoot regeneration using various organs of soybean (Glycine max L. Merr) by response surface methodology. Vitro Cell. Dev. Pl. 52, 537–545. doi: 10.1007/s11627-016-9778-1
Adachi, K., Hirose, A., Kanazashi, Y., Hibara, M., Hirata, T., Mikami, M., et al. (2021). Site-directed mutagenesis by biolistic transformation efficiently generates inheritable mutations in a targeted locus in soybean somatic embryos and transgene-free descendants in the T1 generation. Transgenic Res. 30, 77–89. doi: 10.1007/s11248-020-00229-4
al Amin, N., Ahmad, N., Wu, N., Pu, X., Ma, T., Du, Y., et al. (2019). CRISPR-Cas9 mediated targeted disruption of FAD2–2 microsomal omega-6 desaturase in soybean (Glycine max.L). BMC Biotechnol. 19:9. doi: 10.1186/s12896-019-0501-2
Aleem, M., Riaz, A., Raza, Q., Aleem, M., Aslam, M., Kong, K., et al. (2022). Genome-wide characterization and functional analysis of class III peroxidase gene family in soybean reveal regulatory roles of GsPOD40 in drought tolerance. Genomics 114, 45–60. doi: 10.1016/j.ygeno.2021.11.016
An, J., Cheng, C., Hu, Z., Chen, H., Cai, W., and Yu, B. (2018). The Panax ginseng PgTIP1 gene confers enhanced salt and drought tolerance to transgenic soybean plants by maintaining homeostasis of water, salt ions and ROS. Environ. Exp. Bot. 155, 45–55. doi: 10.1016/j.envexpbot.2018.06.025
Anguraj Vadivel, A. K., McDowell, T., Renaud, J. B., and Dhaubhadel, S. (2021). A combinatorial action of GmMYB176 and GmbZIP5 controls isoflavonoid biosynthesis in soybean (Glycine max). Commun. Biol. 4:356. doi: 10.1038/s42003-021-01889-6
Aragão, F. J. L., Sarokin, L., Vianna, G. R., and Rech, E. L. (2000). Selection of transgenic meristematic cells utilizing a herbicidal molecule results in the recovery of fertile transgenic soybean [Glycine max (L.) Merril] plants at a high frequency. Theor. Appl. Genet. 101, 1–6. doi: 10.1007/s001220051441
Arun, M., Chinnathambi, A., Subramanyam, K., Karthik, S., Sivanandhan, G., Theboral, J., et al. (2016). Involvement of exogenous polyamines enhances regeneration and Agrobacterium-mediated genetic transformation in half-seeds of soybean. 3 Biotech 6:148. doi: 10.1007/s13205-016-0448-0
Arun, M., Subramanyam, K., Mariashibu, T. S., Theboral, J., Shivanandhan, G., Manickavasagam, M., et al. (2015). Application of sonication in combination with vacuum infiltration enhances the Agrobacterium-mediated genetic transformation in Indian soybean cultivars. Appl. Biochem. Biotechnol. 175, 2266–2287. doi: 10.1007/s12010-014-1360-x
Arya, H., Singh, M. B., and Bhalla, P. L. (2021). Overexpression of PIF4 affects plant morphology and accelerates reproductive phase transitions in soybean. Food Energy Secur. 10:e291. doi: 10.1002/fes3.291
Bahramnejad, B., Naji, M., Bose, R., and Jha, S. (2019). A critical review on use of Agrobacterium rhizogenes and their associated binary vectors for plant transformation. Biotech. Adv. 37:107405. doi: 10.1016/j.biotechadv.2019.06.004
Bai, M., Yuan, J., Kuang, H., Gong, P., Li, S., Zhang, Z., et al. (2020). Generation of a multiplex mutagenesis population via pooled CRISPR-Cas9 in soya bean. Plant Biotechnol. J. 18, 721–731. doi: 10.1111/pbi.13239
Bao, A., Chen, H., Chen, L., Chen, S., Hao, Q., Guo, W., et al. (2019). CRISPR/Cas9-mediated targeted mutagenesis of GmSPL9 genes alters plant architecture in soybean. BMC Plant Biol. 19:131. doi: 10.1186/s12870-019-1746-6
Barampuram, S., and Zhang, Z. J. (2011). “Recent advances in plant transformation,” in Plant Chromosome Engineering. Methods in Molecular Biology (Methods and Protocols, ed. J. A. Birchler (Totowa, NJ: Humana Press), 1–35.
Barwale, U. B., Kerns, H. R., and Widholm Jack, M. (1986). Plant regeneration from callus cultures of several soybean genotypes via embryogenesis and organogenesis. Planta 167, 473–481. doi: 10.1007/BF00391223
Beyer, E. (1979). Effect of silver ion, carbon dioxide, and oxygen on ethylene action and metabolism. Plant Physiol. 63, 169–173. doi: 10.1104/pp.63.1.169
Bonawitz, N. D., Ainley, W. M., Itaya, A., Chennareddy, S. R., Cicak, T., Effinger, K., et al. (2019). Zinc finger nuclease-mediated targeting of multiple transgenes to an endogenous soybean genomic locus via non-homologous end joining. Plant Biotechnol. J. 17, 750–761. doi: 10.1111/pbi.13012
Broothaerts, W., Mitchell, H. J., Weir, B., Kaines, S., Smith, L. M., Yang, W., et al. (2005). Gene transfer to plants by diverse species of bacteria. Nature 433, 629–633. doi: 10.1038/nature03309
Bu, T., Lu, S., Wang, K., Dong, L., Li, S., Xie, Q., et al. (2021). A critical role of the soybean evening complex in the control of photoperiod sensitivity and adaptation. Proc. Natl. Acad. Sci. U. S. A. 118:e2010241118. doi: 10.1073/pnas.2010241118
Cai, Y., Chen, L., Liu, X., Guo, C., Sun, S., Wu, C., et al. (2018). CRISPR/Cas9-mediated targeted mutagenesis of GmFT2a delays flowering time in soya bean. Plant Biotechnol. J. 16, 176–185. doi: 10.1111/pbi.12758
Cai, Y., Chen, L., Liu, X., Sun, S., Wu, C., Jiang, B., et al. (2015). CRISPR/Cas9-mediated genome editing in soybean hairy roots. PLoS One 10:e0136064. doi: 10.1371/journal.pone.0136064
Cai, Y., Wang, L., Chen, L., Wu, T., Liu, L., Sun, S., et al. (2020b). Mutagenesis of GmFT2a and GmFT5a mediated by CRISPR/Cas9 contributes for expanding the regional adaptability of soybean. Plant Biotechnol. J. 18, 298–309. doi: 10.1111/pbi.13199
Cai, Y., Chen, L., Zhang, Y., Yuan, S., Su, Q., Sun, S., et al. (2020a). Target base editing in soybean using a modified CRISPR/Cas9 system. Plant Biotechnol. J. 18, 1996–1998. doi: 10.1111/pbi.13386
Cai, Z., Xian, P., Cheng, Y., Ma, Q., Lian, T., Nian, H., et al. (2021). CRISPR/Cas9-mediated gene editing of GmJAGGED1 increased yield in the low latitude soybean variety Huachun 6. Plant Biotechnol. J. 19, 1898–1900. doi: 10.1111/pbi.13673
Campbell, B. W., Hoyle, J. W., Bucciarelli, B., Stec, A. O., Samac, D. A., Parrott, W. A., et al. (2019). Functional analysis and development of a CRISPR/Cas9 allelic series for a CPR5 ortholog necessary for proper growth of soybean trichomes. Sci. Rep. 9:14757. doi: 10.1038/s41598-019-51240-7
Cao, D., Li, Y., Liu, B., Kong, F., and Tran, L. P. (2018). Adaptive mechanisms of soybean grown on salt−affected soils. Land Degrad. Dev. 29, 1054–1064. doi: 10.1002/ldr.2754
Carrijo, J., Illa-Berenguer, E., LaFayette, P., Torres, N., Aragao, F. J. L., Parrott, W., et al. (2021). Two efficient CRISPR/Cas9 systems for gene editing in soybean. Transgenic Res. 30, 239–249. doi: 10.1007/s11248-021-00246-x
Carvalho, C. H. S., Bohorova, N., Bordallo, P. N., Abreu, L. L., Valicente, F. H., Bressan, W., et al. (1997). Type II callus production and plant regeneration in tropical maize genotypes. Plant Cell Rep. 17, 73–76. doi: 10.1007/s002990050355
Castiglioni, P., Bell, E., Lund, A., Rosenberg, A. F., Galligan, M., Hinchey, B. S., et al. (2018). Identification of GB1, a gene whose constitutive overexpression increases glycinebetaine content in maize and soybean. Plant Direct 2:e00040. doi: 10.1002/pld3.40
Chee, P. P., Fober, K. A., and Slightom, J. L. (1989). Transformation of soybean (Glycine max) by infecting germinating seeds with Agrobacterium tumefaciens. Plant Physiol. 91, 1212–1218. doi: 10.1104/pp.91.3.1212
Chen, K., Liu, W., Li, X., and Li, H. (2020b). Overexpression of GmGASA32 promoted soybean height by interacting with GmCDC25. Plant Signal. Behav. 16:1855017. doi: 10.1080/15592324.2020.1855017
Chen, B., Zhang, G., Li, P., Yang, J., Guo, L., Benning, C., et al. (2020a). Multiple GmWRI1s are redundantly involved in seed filling and nodulation by regulating plastidic glycolysis, lipid biosynthesis and hormone signalling in soybean (Glycine max). Plant Biotechnol. J. 18, 155–171. doi: 10.1111/pbi.13183
Chen, L., Cai, Y., Qu, M., Wang, L., Sun, H., Jiang, B., et al. (2020c). Soybean adaption to high-latitude regions is associated with natural variations of GmFT2b, an ortholog of FLOWERING LOCUS T. Plant Cell Environ. 43, 934–944. doi: 10.1111/pce.13695
Chen, L., Nan, H., Kong, L., Yue, L., Yang, H., Zhao, Q., et al. (2020d). Soybean AP1 homologs control flowering time and plant height. J. Integr. Agr. 62, 1868–1879. doi: 10.1111/jipb.12988
Chen, L., Yang, H., Fang, Y., Guo, W., Chen, H., Zhang, X., et al. (2021b). Overexpression of GmMYB14 improves high-density yield and drought tolerance of soybean through regulating plant architecture mediated by the brassinosteroid pathway. Plant Biotechnol. J. 19, 702–716. doi: 10.1111/pbi.13496
Chen, K., Su, C., Tang, W., Zhou, Y., Xu, Z., Chen, J., et al. (2021a). Nuclear transport factor GmNTF2B-1 enhances soybean drought tolerance by interacting with oxidoreductase GmOXR17 to reduce reactive oxygen species content. Plant J. 107, 740–759. doi: 10.1111/tpj.15319
Chen, Z., Fang, X., Yuan, X., Zhang, Y., Li, H., Zhou, Y., et al. (2021c). Overexpression of transcription factor GmTGA15 enhances drought tolerance in transgenic soybean hairy roots and Arabidopsis plants. Agronomy 11, 170. doi: 10.3390/agronomy11010170
Chen, Z. F., Ru, J. N., Sun, G. Z., Du, Y., Chen, J., Zhou, Y. B., et al. (2021d). Genomic-wide analysis of the PLC family and detection of GmPI-PLC7 responses to drought and salt stresses in soybean. Front. Plant Sci. 12:631470. doi: 10.3389/fpls.2021.631470
Chen, K., Tang, W., Zhou, Y., Chen, J., Xu, Z., Ma, R., et al. (2022). AP2/ERF transcription factor GmDREB1 confers drought tolerance in transgenic soybean by interacting with GmERFs. Plant Physiol. Biochem. 170, 287–295. doi: 10.1016/j.plaphy.2021.12.014
Chen, K., Wang, Y., Zhang, R., Zhang, H., and Gao, C. (2019a). CRISPR/Cas genome editing and precision plant breeding in agriculture. Ann. Rev. Plant Biol. 70, 667–697. doi: 10.1146/annurev-arplant-050718-100049
Chen, L., Qin, L., Zhou, L., Li, X., Chen, Z., Sun, L., et al. (2019b). A nodule-localized phosphate transporter GmPT7 plays an important role in enhancing symbiotic N2 fixation and yield in soybean. New Phytol. 221, 2013–2025. doi: 10.1111/nph.15541
Chen, X., Fang, X., Zhang, Y., Wang, X., Zhang, C., Yan, X., et al. (2019c). Overexpression of a soybean 4-coumaric acid: coenzyme A ligase (GmPI4L) enhances resistance to Phytophthora sojae in soybean. Front. Plant Sci. 46:304–313. doi: 10.1071/FP18111
Chen, L., Cai, Y., Liu, X., Yao, W., Guo, C., Sun, S., et al. (2018b). Improvement of soybean Agrobacterium-mediated transformation efficiency by adding glutamine and asparagine into the culture media. Int. J. Mol. Sci. 19:3039. doi: 10.3390/ijms19103039
Chen, L., Cai, Y., Liu, X., Guo, C., Sun, S., Wu, C., et al. (2018a). Soybean hairy roots produced in vitro by Agrobacterium rhizogenes-mediated transformation. Crop J. 6, 162–171. doi: 10.1016/j.cj.2017.08.006
Chen, L., Chunyu, Z., Mingxia, F., Wenjuan, M., Meiming, C., Fengchun, C., et al. (2018c). GmIDL2a and GmIDL4a, encoding the inflorescence deficient in abscission-like protein, are involved in soybean cell wall degradation during lateral root emergence. Int. J. Mol. Sci. 19:2262.
Cheng, M., Fry, J. E., Pang, S., Zhou, H., Hironaka, C. M., Duncan, D. R., et al. (1997). Genetic transformation of wheat mediated by Agrobacterium tumefaciens. Plant Physiol. 115, 971–980. doi: 10.1104/pp.115.3.971
Cheng, Q., Dong, L., Su, T., Li, T., Gan, Z., Nan, H., et al. (2019). CRISPR/Cas9-mediated targeted mutagenesis of GmLHY genes alters plant height and internode length in soybean. BMC Plant Biol. 19:562. doi: 10.1186/s12870-019-2145-8
Cheng, Q., Gan, Z., Wang, Y., Lu, S., Hou, Z., Li, H., et al. (2020). The soybean gene J contributes to salt stress tolerance by up-regulating salt-responsive genes. Front. Plant Sci. 11:272. doi: 10.3389/fpls.2020.00272
Cheng, Y., Wang, X., Cao, L., Ji, J., Liu, T., and Duan, K. (2021). Highly efficient Agrobacterium rhizogenes-mediated hairy root transformation for gene functional and gene editing analysis in soybean. Plant Methods 17:73. doi: 10.1186/s13007-021-00778-7
Chennareddy, S., Cicak, T., Mall, T., Effinger, K., Sardesai, N., Pareddy, D., et al. (2018). Improved direct transformation via particle bombardment of split-immature embryo explants in soybean (Glycine max). Plant Cell Tiss. Org. 135, 23–35. doi: 10.1007/s11240-018-1440-7
Cho, H. J., Farrand, S. K., Noel, G. R., and Widholm, J. M. (2000). High-efficiency induction of soybean hairy roots and propagation of the soybean cyst nematode. Planta 210, 195–204. doi: 10.1007/PL00008126
Cho, H. J., Moy, Y., Rudnick, N. A., Klein, T. M., Yin, J., Bolar, J., et al. (2022). Development of an efficient marker-free soybean transformation method using the novel bacterium Ochrobactrum haywardense H1. Plant Biotechnol. J. 20, 977–990. doi: 10.1111/pbi.13777
Cho, H. S., Lee, Y. J., Kim, H. J., Park, M.-Y., Yeom, W. W., Song, J. H., et al. (2021). Overexpression of Arabidopsis thaliana blue-light inhibitor of cryptochromes 1 gene alters plant architecture in soybean. Plant Biotechnol. Rep. 15, 459–469. doi: 10.1007/s11816-021-00693-2
Chowrira, G. M., Akella, V., and Lurquin, P. F. (1995). Electroporation-mediated gene transfer into intact nodal meristemsin planta. Mol. Biotech. 3, 17–23. doi: 10.1007/BF02821331
Christey, M. C. (2001). Use of ri-mediated transformation for production of transgenic plants. In Vitro Cell. Dev. Pl. 37, 687–700. doi: 10.1007/s11627-001-0120-0
Christou, P. (1990). Morphological description of transgenic soybean chimeras created by the delivery, integration and expression of foreign DNA using electric discharge particle acceleration. Ann. Bot. 66, 379–386. doi: 10.1093/oxfordjournals.aob.a088039
Christou, P., McCabe, D., and Swain, W. (1988). Stable transformation of soybean callus by DNA-coated gold particles. Plant Physiol. 87, 671–674. doi: 10.1104/pp.87.3.671
Christou, P., and Mccabe, D. E. (1992). Prediction of germ-line transformation events in chimeric Ro transgenic soybean plantlets using tissue-specific expression patterns. Plant J. 2, 283–290. doi: 10.1111/j.1365-313X.1992.00283.x
Clemente, T. E., LaVallee, B. J., Howe, A. R., Conner-Ward, D., Rozman, R. J., Hunter, P. E., et al. (2000). Progeny analysis of glyphosate selected transgenic soybeans derived from Agrobacterium-Mediated transformation. Crop Sci. 40, 797–803. doi: 10.2135/cropsci2000.403797x
Collier, R., Fuchs, B., Walter, N., Kevin Lutke, W., and Taylor, C. G. (2005). Ex vitro composite plants: an inexpensive, rapid method for root biology. Plant J. 43, 449–457. doi: 10.1111/j.1365-313X.2005.02454.x
Coutinho, F. S., Dos Santos, D. S., Lima, L. L., Vital, C. E., Santos, L. A., Pimenta, M. R., et al. (2019). Mechanism of the drought tolerance of a transgenic soybean overexpressing the molecular chaperone BiP. Physiol. Mol. Biol. Plants 25, 457–472. doi: 10.1007/s12298-019-00643-x
Curtin, S. J., Xiong, Y., Michno, J. M., Campbell, B. W., Stec, A. O., Cermak, T., et al. (2018). CRISPR/Cas9 and TALENs generate heritable mutations for genes involved in small RNA processing of Glycine max and Medicago truncatula. Plant Biotechnol. J. 16, 1125–1137. doi: 10.1111/pbi.12857
Curtin, S. J., Zhang, F., Sander, J. D., Haun, W. J., Starker, C., Baltes, N. J., et al. (2011). Targeted mutagenesis of duplicated genes in soybean with zinc-finger nucleases. Plant Physiol. 156, 466–473. doi: 10.1104/pp.111.172981
Dan, Y., Armstrong, C. L., Dong, J., Feng, X., Fry, J. E., Keithly, G. E., et al. (2009). Lipoic acid—an unique plant transformation enhancer. In Vitro Cell. Dev. Biol. Plant 45, 630–638. doi: 10.1007/s11627-009-9227-5
Dan, Y., and Reichert, N. A. (1998). Organogenic regeneration of soybean from hypocotyl explants. In Vitro Cell. Dev. Pl. 34, 14–21. doi: 10.1007/BF02823117
Dang, W., and Wei, Z. M. (2007). An optimized Agrobacterium-mediated transformation for soybean for expression of binary insect resistance genes. Plant Sci. 173, 381–389. doi: 10.1016/j.plantsci.2007.06.010
Debernardi, J. M., Tricoli, D. M., Ercoli, M. F., Hayta, S., Ronald, P., Palatnik, J. F., et al. (2020). A GRF-GIF chimeric protein improves the regeneration efficiency of transgenic plants. Nat. Biotech. 38, 1274–1279. doi: 10.1038/s41587-020-0703-0
Demorest, Z. L., Coffman, A., Baltes, N. J., Stoddard, T. J., Clasen, B. M., Luo, S., et al. (2016). Direct stacking of sequence-specific nuclease-induced mutations to produce high oleic and low linolenic soybean oil. BMC Plant Biol. 16:225. doi: 10.1186/s12870-016-0906-1
Dhir, S. K., Dhir, S., Savka, M. A., Belanger, F., Kriz, A. L., Farrand, S. K., et al. (1992). Regeneration of transgenic soybean (Glycine max) plants from electroporated protoplasts. Plant Physiol. 99, 81–88. doi: 10.1104/pp.99.1.81
Do, P. T., Nguyen, C. X., Bui, H. T., Tran, L. T. N., Stacey, G., Gillman, J. D., et al. (2019). Demonstration of highly efficient dual gRNA CRISPR/Cas9 editing of the homeologous GmFAD2-1A and GmFAD2-1B genes to yield a high oleic, low linoleic and alpha-linolenic acid phenotype in soybean. BMC Plant Biol. 19:311. doi: 10.1186/s12870-019-1906-8
Donaldson, P. A., and Simmonds, D. H. (2000). Susceptibility to Agrobacterium tumefaciens and cotyledonary node transformation in short-season soybean. Plant Cell Rep. 19, 478–484. doi: 10.1007/s002990050759
Dong, J., and Hudson, M. E. (2022). WI12Rhg1 interacts with DELLAs and mediates soybean cyst nematode resistance through hormone pathways. Plant Biotechnol. J. 20, 283–296. doi: 10.1111/pbi.13709
Dong, J., Zielinski, R. E., and Hudson, M. E. (2020). t-SNAREs bind the Rhg1 α-SNAP and mediate soybean cyst nematode resistance. Plant J. 104, 318–331. doi: 10.1111/tpj.14923
Dong, L., Fang, C., Cheng, Q., Su, T., Kou, K., Kong, L., et al. (2021). Genetic basis and adaptation trajectory of soybean from its temperate origin to tropics. Nat. Commun. 12:5445. doi: 10.1038/s41467-021-25800-3
Du, H., Zeng, X., Zhao, M., Cui, X., Wang, Q., Yang, H., et al. (2016). Efficient targeted mutagenesis in soybean by TALENs and CRISPR/Cas9. J. Biotech. 217, 90–97. doi: 10.1016/j.jbiotec.2015.11.005
Du, Q., Yang, X., Zhang, J., Zhong, X., Kim, K. S., Yang, J., et al. (2018). Over-expression of the Pseudomonas syringae harpin-encoding gene hrpZm confers enhanced tolerance to Phytophthora root and stem rot in transgenic soybean. Transgenic Res. 27, 277–288. doi: 10.1007/s11248-018-0071-4
Duan, K., Cheng, Y., Ji, J., Wang, C., Wei, Y., and Wang, Y. (2021). Large chromosomal segment deletions by CRISPR/LbCpf1-mediated multiplex gene editing in soybean. J. Integr. Plant Biol. 63, 1620–1631. doi: 10.1111/jipb.13158
Dubey, V. K., Lee, U. G., Kwon, D. H., and Lee, S. H. (2017). Agroinfiltration-based expression of hairpin RNA in soybean plants for RNA interference against Tetranychus urticae. Pestic. Biochem. Physiol. 142, 53–58. doi: 10.1016/j.pestbp.2017.01.004
Falco, S. C., Guida, T., Locke, M., Mauvais, J., Sanders, C., Ward, R. T., et al. (1995). Transgenic canola and soybean seeds with increased lysine. Biotechnology 13, 577–582. doi: 10.1038/nbt0695-577
Fan, Y., Wang, X., Li, H., Liu, S., Jin, L., Lyu, Y., et al. (2020). Anthocyanin, a novel and user-friendly reporter for convenient, non-destructive, low cost, directly visual selection of transgenic hairy roots in the study of rhizobia-legume symbiosis. Plant Methods 16:94. doi: 10.1186/s13007-020-00638-w
Faria, J. A. Q. A., Reis, P. A. B., Reis, M. T. B., Rosado, G. L., Pinheiro, G. L., Mendes, G. C., et al. (2011). The NAC domain-containing protein, GmNAC6, is a downstream component of the ER stress- and osmotic stress-induced NRP-mediated cell-death signaling pathway. BMC Plant Biol. 11:129. doi: 10.1186/1471-2229-11-129
Feng, X., Feng, P., Yu, H., Yu, X., Sun, Q., Liu, S., et al. (2020). GsSnRK1 interplays with transcription factor GsERF7 from wild soybean to regulate soybean stress resistance. Plant Cell Environ. 43, 1192–1211. doi: 10.1111/pce.13726
Fernandez, S., Michaux-Ferrière, N., and Coumans, M. F. (1999). The embryogenic response of immature embryo cultures of durum wheat (Triticum durum Desf.): histology and improvement by AgNO3. Plant Growth Regul. 28, 147–155. doi: 10.1023/A:1006142504577
Finer, J. J. (2016). Generation of transgenic soybean (Glycine max) via particle bombardment of embryogenic cultures. Curr. Protoc. Plant Bio. 1, 592–603. doi: 10.1002/cppb.20039
Finer, J. J., and Larkin, K. M. (2008). “Genetic transformation of soybean using particle bombardment and SAAT approaches,” in Handbook of New Technologies For Genetic Improvement of Legumes, ed. K. PB (Boca Raton, USA: CRC Press/Taylor and Francis Group), 103–123.
Finer, J. J., and Mcmullen, M. D. (1991). Transformation of soybean via particle bombardment of embryogenic suspension culture tissue. In Vitro Cell. Dev. Pl. 27, 175–182. doi: 10.2307/4292952
Finer, J. J., and Nagasawa, A. (1988). Development of an embryogenic suspension culture of soybean (Glycine max Merrill.). Plant Cell Tiss. Org. 15, 125–136. doi: 10.1007/BF00035754
Fu, M., Sun, J., Li, X., Guan, Y., and Xie, F. (2022). Asymmetric redundancy of soybean Nodule Inception (NIN) genes in root nodule symbiosis. Plant Physiol. 188, 477–489. doi: 10.1093/plphys/kiab473
Gao, C. (2021). Genome engineering for crop improvement and future agriculture. Cell 184, 1621–1635. doi: 10.1016/j.cell.2021.01.005
Gao, L., Luo, J., Ding, X., Wang, T., Hu, T., Song, P., et al. (2020). Soybean RNA interference lines silenced for eIF4E show broad potyvirus resistance. Mol. Plant Pathol. 21, 303–317. doi: 10.1111/mpp.12897
Ghidelli, C., Mateos, M., Rojas-Argudo, C., and Pérez-Gago, M. B. (2014). Effect of antioxidants on enzymatic browning of eggplant extract and fresh-cut tissue. J. Food Process. Pres. 38, 1501–1510. doi: 10.1111/jfpp.12109
Gordon-Kamm, W., Sardesai, N., Arling, M., Lowe, K., Hoerster, G., Betts, S., et al. (2019). Using morphogenic genes to improve recovery and regeneration of transgenic plants. Plants 8, 38. doi: 10.3390/plants8020038
Grimsley, N., Hohn, B., Hohn, T., and Walden, R. (1986). “Agroinfection,” an alternative route for viral infection of plants by using the Ti plasmid. Proc. Natl. Acad. Sci. U. S. A. 83, 3282–3286. doi: 10.1073/pnas.83.10.3282
Guo, B., Guo, Y., Hong, H., Jin, L., Zhang, L., Chang, R. Z., et al. (2015). Co-expression of G2-EPSPS and glyphosate acetyltransferase GAT genes conferring high tolerance to glyphosate in soybean. Front. Plant Sci. 6:847. doi: 10.3389/fpls.2015.00847
Guo, B. F., Hong, H. L., Han, J. N., Zhang, L. J., and Qiu, L. J. (2020a). Development and identification of glyphosate-tolerant transgenic soybean via direct selection with glyphosate. J. Integr. Agr. 19, 1186–1196. doi: 10.1016/S2095-3119(19)62747-4
Guo, C., Liu, X., Chen, L., Cai, Y., Yao, W., Yuan, S., et al. (2020b). Elevated methionine content in soybean seed by overexpressing maize β-zein protein. Oil Crop Sci. 5, 11–16. doi: 10.1016/j.ocsci.2020.03.004
Guo, W., Chen, L., Chen, H., Yang, H., You, Q., Bao, A., et al. (2020c). Overexpression of GmWRI1b in soybean stably improves plant architecture and associated yield parameters, and increases total seed oil production under field conditions. Plant Biotechnol. J. 18, 1639–1641. doi: 10.1111/pbi.13324
Hada, A., Gupta, A. K., Jeevaraj, T., Manickavasagam, M., Ganapathi, A., Jolly, M., et al. (2014). Developing rapid and reliable regeneration system using cotyledonary-node method in Indian soybean genotypes (Glycine max L.). Int. J. Inno.Res. Sci. 3, 12678–12686.
Hada, A., Krishnan, V., Mohamed Jaabir, M. S., Kumari, A., Jolly, M., Praveen, S., et al. (2018). Improved Agrobacterium tumefaciens-mediated transformation of soybean [Glycine max (L.) Merr.] following optimization of culture conditions and mechanical techniques. In Vitro Cell. Dev. Pl. 54, 672–688. doi: 10.1007/s11627-018-9944-8
Hadi, M. Z., McMullen, M. D., and Finer, J. J. (1996). Transformation of 12 different plasmids into soybean via particle bombardment. Plant Cell Rep. 15, 500–505. doi: 10.1007/BF00232982
Han, J., Guo, B., Guo, Y., Zhang, B., Wang, X., and Qiu, L. J. (2019). Creation of early flowering germplasm of soybean by CRISPR/Cas9 technology. Front. Plant Sci. 10:1446. doi: 10.3389/fpls.2019.01446
Han, X., Wang, D., and Song, G. Q. (2021). Expression of a maize SOC1 gene enhances soybean yield potential through modulating plant growth and flowering. Sci. Rep. 11:12758. doi: 10.1038/s41598-021-92215-x
Hao, Y., Fan, X., Guo, H., Yao, Y., Ren, G., Lv, X., et al. (2020). Overexpression of the bioactive lunasin peptide in soybean and evaluation of its anti-inflammatory and anti-cancer activities invitro. J. Biosci. Bioeng. 129, 395–404. doi: 10.1016/j.jbiosc.2019.11.001
Haun, W., Coffman, A., Clasen, B. M., Demorest, Z. L., Lowy, A., Ray, E., et al. (2014). Improved soybean oil quality by targeted mutagenesis of the fatty acid desaturase 2 gene family. Plant Biotechnol. J. 12, 934–940. doi: 10.1111/pbi.12201
Hayashi, M., Saeki, Y., Haga, M., Harada, K., Kouchi, H., and Umehara, Y. (2012). Rj (rj) genes involved in nitrogen-fixing root nodule formation in soybean. Breeding Sci. 61, 544–553. doi: 10.1270/jsbbs.61.544
He, C., Gao, H., Wang, H., Guo, Y., He, M., Peng, Y., et al. (2021). GSK3-mediated stress signaling inhibits legume–rhizobium symbiosis by phosphorylating GmNSP1 in soybean. Mol. Plant 14, 488–502. doi: 10.1016/j.molp.2020.12.015
He, Y., Dong, Y., Yang, X., Guo, D., Qian, X., Yan, F., et al. (2020). Functional activation of a novel R2R3-MYB protein gene, GmMYB68, confers salt-alkali resistance in soybean (Glycine max L.). Genome 63, 13–26. doi: 10.1139/gen-2018-0132
Hernandez-Garcia, C. M., Martinelli, A. P., Bouchard, R. A., and Finer, J. J. (2009). A soybean (Glycine max) polyubiquitin promoter gives strong constitutive expression in transgenic soybean. Plant Cell Rep. 28, 837–849. doi: 10.1007/s00299-009-0681-7
Hinchee, M. A. W., Connor-Ward, D. V., Newell, C. A., Mcdonnell, R. E., Sato, S. J., Gasser, C. S., et al. (1988). Production of transgenic soybean plants using Agrobacterium-mediated DNA transfer. Biotechnology 6, 915–922. doi: 10.1038/nbt0888-915
Homrich, M. S., Wiebke-Strohm, B., Weber, R. L., and Bodanese-Zanettini, M. H. (2012). Soybean genetic transformation: a valuable tool for the functional study of genes and the production of agronomically improved plants. Genet. Mol. Biol. 35, 998–1010. doi: 10.1590/s1415-47572012000600015
Hong, H., Zhang, H., Olhoft, P., Hill, S., Wiley, H., Toren, E., et al. (2007). Organogenic callus as the target for plant regeneration and transformation via Agrobacterium in soybean (Glycine max (L.) Merr.). In Vitro Cell. Dev. Pl. 43, 558–568. doi: 10.1007/s11627-007-9066-1
Hu, C. Y., and Wang, L. (1999). In planta soybean transformation technologies developed in China: procedure, confirmation and field performance. In Vitro Cell. Dev. Pl. 35, 417–420. doi: 10.2307/4293277
Huang, Y., Xuan, H., Yang, C., Guo, N., Wang, H., Zhao, J., et al. (2019). GmHsp90A2 is involved in soybean heat stress as a positive regulator. Plant Sci. 285, 26–33. doi: 10.1016/j.plantsci.2019.04.016
Hwang, H. H., Yu, M., and Lai, E. M. (2017). Agrobacterium-mediated plant transformation: biology and applications. Arabidopsis Book 15:e0186. doi: 10.1199/tab.0186
Islam, N., Islam, T., Hossain, M., Bhattacharjee, B., Hossain, M., and Islam, S. M. S. (2017). Embryogenic callus induction and efficient plant regeneration in three varieties of soybean (Glycine max). Plant Tiss. Cul. Biotechnol. 27, 41–50. doi: 10.3329/ptcb.v27i1.35011
Ismagul, A., Yang, N., Maltseva, E., Iskakova, G., Mazonka, I., Skiba, Y., et al. (2018). A biolistic method for high-throughput production of transgenic wheat plants with single gene insertions. BMC Plant Biol. 18:135. doi: 10.1186/s12870-018-1326-1
Itaya, A., Zheng, S., and Simmonds, D. (2018). Establishment of neomycin phosphotransferase II (nptII) selection for transformation of soybean somatic embryogenic cultures. In Vitro Cell. Dev. Pl. 54, 184–194. doi: 10.1007/s11627-017-9875-9
Iwabuchi, K., Shimada, T. L., Yamada, T., and Hara-Nishimura, I. (2020). A space-saving visual screening method. Glycine max FAST, for generating transgenic soybean. Plant Signal. Behav. 15:1722911. doi: 10.1080/15592324.2020.1722911
Jacobs, T. B., LaFayette, P. R., Schmitz, R. J., and Parrott, W. A. (2015). Targeted genome modifications in soybean with CRISPR/Cas9. BMC Biotechnol. 15:16. doi: 10.1186/s12896-015-0131-2
Jahan, M. A., Harris, B., Lowery, M., Infante, A. M., Percifield, R. J., and Kovinich, N. (2020). Glyceollin transcription factor GmMYB29A2 regulates soybean resistance to Phytophthora sojae. Plant Physiol. 183, 530–546. doi: 10.1104/pp.19.01293
Jia, Q., Sun, S., Kong, D., Song, J., Wu, L., Yan, Z., et al. (2020). Ectopic expression of Gs5PTase8, a soybean inositol polyphosphate 5-phosphatase, enhances salt tolerance in plants. Int. J. Mol. Sci. 21, 1023. doi: 10.3390/ijms21031023
Jia, Y., Yao, X., Zhao, M., Zhao, Q., Du, Y., Yu, C., et al. (2015). Comparison of soybean transformation efficiency and plant factors affecting transformation during the Agrobacterium infection process. Int. J. Mol. Sci. 16, 18522–18543. doi: 10.3390/ijms160818522
Jiang, B., Chen, L., Yang, C., Wu, T., Yuan, S., Wu, C., et al. (2021). The cloning and CRISPR/Cas9-mediated mutagenesis of a male sterility gene MS1 of soybean. Plant Biotechnol. J. 19, 1098–1100. doi: 10.1111/pbi.13601
Jing, G., Tang, D., Yao, Y., Su, Y., Shen, Y., Bai, Y., et al. (2021). Seed specifically over-expressing DGAT2A enhances oil and linoleic acid contents in soybean seeds. Biochem. Bioph. Res. Co. 568, 143–150. doi: 10.1016/j.bbrc.2021.06.087
Jones, S. I., Hunt, M. R., and Vodkin, L. O. (2020). An embryo lethal transgenic line manifests global expression changes and elevated protein/oil ratios in heterozygous soybean plants. PLoS One 15:e0233721. doi: 10.1371/journal.pone.0233721
Joyner, E. Y., Boykin, L. S., and Lodhi, M. A. (2010). Callus induction and organogenesis in soybean [Glycine max (L.) Merr.] cv. pyramid from mature cotyledons and embryos. Open Plant Sci. J. 4, 18–21. doi: 10.2174/1874294701004010018
Kahn, T. W., Duck, N. B., McCarville, M. T., Schouten, L. C., Schweri, K., Zaitseva, J., et al. (2021). A Bacillus thuringiensis Cry protein controls soybean cyst nematode in transgenic soybean plants. Nat. Commun. 12:3380. doi: 10.1038/s41467-021-23743-3
Kalbande, B. B., and Patil, A. S. (2016). Plant tissue culture independent Agrobacterium tumefaciens mediated In-planta transformation strategy for upland cotton (Gossypium hirsutum). J. Genet. Eng. Biotechnol. 14, 9–18. doi: 10.1016/j.jgeb.2016.05.003
Kambakam, S., Ngaki, M. N., Sahu, B. B., Kandel, D. R., Singh, P., Sumit, R., et al. (2021). Arabidopsis non-host resistance PSS30 gene enhances broad-spectrum disease resistance in the soybean cultivar Williams 82. Plant J. 107, 1432–1446. doi: 10.1111/tpj.15392
Kanai, M., Yamada, T., Hayashi, M., Mano, S., and Nishimura, M. (2019). Soybean (Glycine max L.) triacylglycerol lipase GmSDP1 regulates the quality and quantity of seed oil. Sci. Rep. 9:8924. doi: 10.1038/s41598-019-45331-8
Kanazashi, Y., Hirose, A., Takahashi, I., Mikami, M., Endo, M., Hirose, S., et al. (2018). Simultaneous site-directed mutagenesis of duplicated loci in soybean using a single guide RNA. Plant Cell Rep. 37, 553–563. doi: 10.1007/s00299-018-2251-3
Karthik, S., Pavan, G., and Manickavasagam, M. (2020). Nitric oxide donor regulates Agrobacterium-mediated genetic transformation efficiency in soybean [Glycine max (L.) Merrill]. Plant Cell Tiss. Org. 141, 655–660. doi: 10.1007/s11240-020-01808-3
Karthik, S., Tuteja, N., Ganapathi, A., and Manickavasagam, M. (2019). Pea p68, a DEAD-box helicase, enhances salt tolerance in marker-free transgenic plants of soybean [Glycine max (L.) Merrill]. 3 Biotech 9:10. doi: 10.1007/s13205-018-1553-z
Kereszt, A., Li, D., Indrasumunar, A., Nguyen, C. D., Nontachaiyapoom, S., Kinkema, M., et al. (2007). Agrobacterium rhizogenes-mediated transformation of soybean to study root biology. Nat. Protoc. 2, 948–952. doi: 10.1038/nprot.2007.141
Kidokoro, S., Watanabe, K., Ohori, T., Moriwaki, T., Maruyama, K., Mizoi, J., et al. (2015). Soybean DREB1/CBF-type transcription factors function in heat and drought as well as cold stress-responsive gene expression. Plant J. 81, 505–518. doi: 10.1111/tpj.12746
Kim, H., and Choi, J. (2021). A robust and practical CRISPR/crRNA screening system for soybean cultivar editing using LbCpf1 ribonucleoproteins. Plant Cell Rep. 40, 1059–1070. doi: 10.1007/s00299-020-02597-x
Kim, H., Kim, S. T., Ryu, J., Kang, B. C., Kim, J. S., and Kim, S. G. (2017). CRISPR/Cpf1-mediated DNA-free plant genome editing. Nat. Commun. 8:14406. doi: 10.1038/ncomms14406
Kim, H. J., Cho, H. S., Pak, J. H., Kwon, T., Lee, J. H., Kim, D. H., et al. (2018). Confirmation of drought tolerance of ectopically expressed AtABF3 gene in soybean. Mol. Cells 41, 413–422. doi: 10.14348/molcells.2018.2254
Kim, K. H., Lim, S., Kang, Y. J., Yoon, M. Y., Nam, M., Jun, T. H., et al. (2016). Optimization of a virus-induced gene silencing system with soybean yellow common mosaic virus for gene function studies in soybeans. Plant Pathol. J. 32, 112–122. doi: 10.5423/PPJ.OA.04.2015.0063
Kim, W.-S., and Krishnan, H. B. (2019). Impact of co-expression of maize 11 and 18 kDa δ-zeins and 27 kDa γ-zein in transgenic soybeans on protein body structure and sulfur amino acid content. Plant Sci. 280, 340–347. doi: 10.1016/j.plantsci.2018.12.016
Kim, W.-S., Sun-Hyung, J., Oehrle, N. W., Jez, J. M., and Krishnan, H. B. (2020). Overexpression of ATP sulfurylase improves the sulfur amino acid content, enhances the accumulation of Bowman–Birk protease inhibitor and suppresses the accumulation of the β-subunit of β-conglycinin in soybean seeds. Sci. Rep. 10:14989. doi: 10.1038/s41598-020-72134-z
King, J. L., Finer, J. J., and McHale, L. K. (2015). Development and optimization of agroinfiltration for soybean. Plant Cell Rep. 34, 133–140. doi: 10.1007/s00299-014-1694-4
Klein, T. M., Wolf, E. D., Wu, R., and Sanford, J. C. (1987). High-velocity microprojectiles for delivering nucleic acids into living cells. Nature 327, 70–73. doi: 10.1038/327070a0
Ko, T. S., Lee, S., Krasnyanski, S., and Korban, S. S. (2003). Two critical factors are required for efficient transformation of multiple soybean cultivars: agrobacterium strain and orientation of immature cotyledonary explant. Theor. Appl. Genet. 107, 439–447. doi: 10.1007/s00122-003-1264-6
Kong, J., Martin-Ortigosa, S., Finer, J., Orchard, N., Gunadi, A., Batts, L. A., et al. (2020). Overexpression of the transcription factor GROWTH-REGULATING FACTOR5 improves transformation of dicot and monocot Species. Front. Plant Sci. 11:572319. doi: 10.3389/fpls.2020.572319
Kuma, K. M., Lopes-Caitar, V. S., Romero, C. C. T., Silva, S. M. H., Kuwahara, M. K., Carvalho, M. C. C. G., et al. (2015). A high efficient protocol for soybean root transformation by Agrobacterium rhizogenes and most stable reference genes for RT-qPCR analysis. Plant Cell Rep. 34, 1987–2000. doi: 10.1007/s00299-015-1845-2
Kumar, A., Kumar, V., Krishnan, V., Hada, A., and Marathe, A. C. P. (2019). Seed targeted RNAi-mediated silencing of GmMIPS1 limits phytate accumulation and improves mineral bioavailability in soybean. Sci. Rep. 9:7744. doi: 10.1038/s41598-019-44255-7
Kumar, S., Liu, Z.-B., Sanyour-Doyel, N., Lenderts, B., Worden, A., Anand, A., et al. (2022). Efficient gene targeting in soybean using Ochrobactrum haywardense-mediated delivery of a marker-free donor template. Plant Physiol. 00, 1–10. doi: 10.1093/plphys/kiac075
Kumar, S. V., and Rajam, M. (2005). Polyamines enhance Agrobacterium tumefaciens vir gene induction and T-DNA transfer. Plant Sci. 168, 475–480. doi: 10.1016/j.plantsci.2004.09.018
Kumari, A., Hada, A., Subramanyam, K., Theboral, J., Misra, S., Ganapathi, A., et al. (2018). RNAi-mediated resistance to yellow mosaic viruses in soybean targeting coat protein gene. Acta Physiol. Plant 40:32. doi: 10.1007/s11738-018-2608-9
Kun, X., Zhang, X.-M., Fan, C.-M., Chen, F.-L., Zhu, J.-L., Zhang, S.-L., et al. (2017). A callus transformation system for gene functional studies in soybean. J. Integr. Agr. 16, 1913–1922. doi: 10.1016/S2095-3119(16)61621-0
Le, H., Nguyen, N. H., Ta, D. T., Le, T. N. T., Bui, T. P., Le, N. T., et al. (2020). CRISPR/Cas9-mediated knockout of galactinol synthase-encoding genes reduces raffinose family oligosaccharide levels in soybean seeds. Front. Plant Sci. 11:612942. doi: 10.3389/fpls.2020.612942
Lee, H., Park, S.-Y., and Zhang, Z. J. (2013). “An overview of genetic transformation of soybean,” in A Comprehensive Survey of International Soybean Research - Genetics, Physiology, Agronomy and Nitrogen Relationships, ed. J. E. Board (London, UK: IntechOpen).
Li, C., Nguyen, V., Liu, J., Fu, W., Chen, C., Yu, K., et al. (2019). Mutagenesis of seed storage protein genes in soybean using CRISPR/Cas9. BMC Res. Notes 12:176. doi: 10.1186/s13104-019-4207-2
Li, C., Zhang, H., Wang, X., and Liao, H. (2014). A comparison study of Agrobacterium-mediated transformation methods for root-specific promoter analysis in soybean. Plant Cell Rep. 33, 1921–1932. doi: 10.1007/s00299-014-1669-5
Li, F., Ni, H., Yan, W., Xie, Y., Liu, X., Tan, X., et al. (2021a). Overexpression of an aquaporin protein from Aspergillus glaucus confers salt tolerance in transgenic soybean. Transgenic Res. 30, 727–737. doi: 10.1007/s11248-021-00280-9
Li, M., Chen, R., Jiang, Q., Sun, X., Zhang, H., and Hu, Z. (2021b). GmNAC06, a NAC domain transcription factor enhances salt stress tolerance in soybean. Plant Mol. Biol. 105, 333–345. doi: 10.1007/s11103-020-01091-y
Li, Z., Cheng, Q., Gan, Z., Hou, Z., Zhang, Y., Li, Y., et al. (2021c). Multiplex CRISPR/Cas9-mediated knockout of soybean LNK2 advances flowering time. Crop J. 9, 767–776. doi: 10.1016/j.cj.2020.09.005
Li, J., Todd, T. C., Oakley, T. R., Lee, J., and Trick, H. N. (2010). Host-derived suppression of nematode reproductive and fitness genes decreases fecundity of Heterodera glycines Ichinohe. Planta 232, 775–785. doi: 10.1007/s00425-010-1209-7
Li, L., Gao, W., Peng, Q., Zhou, B., Kong, Q., Ying, Y., et al. (2018). Two soybean bHLH factors regulate response to iron deficiency. J. Integr. Plant Biol. 60, 608–622. doi: 10.1111/jipb.12651
Li, S., Cong, Y., Liu, Y., Wang, T., Shuai, Q., Chen, N., et al. (2017). Optimization of Agrobacterium-mediated transformation in soybean. Front. Plant Sci. 8:246. doi: 10.3389/fpls.2017.00246
Li, Z., Liu, Z. B., Xing, A., Moon, B. P., Koellhoffer, J. P., Huang, L., et al. (2015). Cas9-guide RNA directed genome editing in soybean. Plant Physiol. 169, 960–970. doi: 10.1104/pp.15.00783
Li, Z., Sushuang, D., Huidong, X., Xingxing, F., Ruidong, S., Jinming, Z., et al. (2022). A novel TIR-NBS-LRR gene regulates immune response to Phytophthora root rot in soybean. Crop J. doi: 10.1016/j.cj.2022.03.003
Liang, Z., Chen, K., Li, T., Zhang, Y., Wang, Y., Zhao, Q., et al. (2017). Efficient DNA-free genome editing of bread wheat using CRISPR/Cas9 ribonucleoprotein complexes. Nat. Commun. 8:14261. doi: 10.1038/ncomms14261
Lin, W., Odell, J. T., and Schreiner, R. M. (1987). Soybean protoplast culture and direct gene uptake and expression by cultured soybean protoplasts. Plant Physiol. 84, 856–861. doi: 10.1104/pp.84.3.856
Liu, C., Zhang, C., Fan, M., Ma, W., Chen, M., Cai, F., et al. (2018). GmIDL2a and GmIDL4a, encoding the inflorescence deficient in abscission-like protein, are involved in soybean cell wall degradation during lateral root emergence. Int. J. Mol. Sci. 19:2262. doi: 10.3390/ijms19082262
Liu, W., Jiang, B., Ma, L., Zhang, S., Zhai, H., Xu, X., et al. (2018). Functional diversification of Flowering Locus T homologs in soybean: GmFT1a and GmFT2a/5a have opposite roles in controlling flowering and maturation. New Phytol. 217, 1335–1345. doi: 10.1111/nph.14884
Liu, H. K., Yang, C., and Wei, Z. M. (2004). Efficient Agrobacterium tumefaciens-mediated transformation of soybeans using an embryonic tip regeneration system. Planta 219, 1042–1049. doi: 10.1007/s00425-004-1310-x
Liu, J., Su, Q., An, L., and Yang, A. (2009). Transfer of a minimal linear marker-free and vector-free smGFP cassette into soybean via ovary-drip transformation. Biotechnol. Lett. 31, 295–303. doi: 10.1007/s10529-008-9851-x
Liu, L. F., Le, G., Zhang, L. X., Cai, Y. P., Song, W. W., and Han, T. F. (2022a). Co-silencing E1 and its homologs in an extremely late-maturing soybean cultivar confers super-early maturity and adaptation to high-latitude short-season regions. J. Integr. Agr. 21, 326–335. doi: 10.1016/S2095-3119(20)63391-3
Liu, S. Y., Liu, J. F., Zhang, Y. Z., Jiang, Y. S., Hu, S. W., Qu, J., et al. (2022b). Cloning of the soybean sHSP26 gene and analysis of its drought resistance. Phyton 97, 1465–1482. doi: 10.32604/phyton.2022.018836
Liu, X., Pi, B., Du, Z., Yang, T., Gu, M., Sun, S., et al. (2022c). The transcription factor GmbHLH3 confers Cl–/salt tolerance to soybean by upregulating GmCLC1 expression for maintenance of anion homeostasis. Environ. Exp. Bot. 194:104755. doi: 10.1016/j.envexpbot.2021.104755
Liu, S., Wang, D., Mei, Y., Xia, T., Xu, W., Zhang, Y., et al. (2020). Overexpression of GmAAP6a enhances tolerance to low nitrogen and improves seed nitrogen status by optimizing amino acid partitioning in soybean. Plant Biotechnol. 18, 1749–1762. doi: 10.1111/pbi.13338
Liu, S. J., Wei, Z. M., and Huang, J. Q. (2008). The effect of co-cultivation and selection parameters on Agrobacterium-mediated transformation of Chinese soybean varieties. Plant Cell Rep. 27, 489–498. doi: 10.1007/s00299-007-0475-8
Liu, X., Liu, F., Zhang, L., Cheng, C., Wei, P., and Yu, B. (2021). GsCLC-c2 from wild soybean confers chloride/salt tolerance to transgenic Arabidopsis and soybean composite plants by regulating anion homeostasis. Physiol. Plantarum 172, 1867–1879. doi: 10.1111/ppl.13396
Lowe, K., La Rota, M., Hoerster, G., Hastings, C., Wang, N., Chamberlin, M., et al. (2018). Rapid genotype “independent” Zea mays L. (maize) transformation via direct somatic embryogenesis. In Vitro Cell. Dev. Pl. 54, 240–252. doi: 10.1007/s11627-018-9905-2
Lowe, K., Wu, E., Wang, N., Hoerster, G., Hastings, C., Cho, M. J., et al. (2016). Morphogenic regulators Baby boom and Wuschel improve monocot transformation. Plant Cell 28, 1998–2015. doi: 10.1105/tpc.16.00124
Lu, L., Dong, C., Liu, R., Zhou, B., Wang, C., and Shou, H. (2018). Roles of soybean plasma membrane intrinsic protein GmPIP2;9 in drought tolerance and seed development. Front. Plant Sci. 9:530. doi: 10.3389/fpls.2018.00530
Lu, L., Wei, W., Li, Q.-T., Bian, X.-H., Lu, X., Hu, Y., et al. (2021). A transcriptional regulatory module controls lipid accumulation in soybean. New Phytol. 231, 661–678. doi: 10.1111/nph.17401
Lu, Q. S. M., and Tian, L. (2022). An efficient and specific CRISPR-Cas9 genome editing system targeting soybean phytoene desaturase genes. BMC Biotechnol. 22:7. doi: 10.1186/s12896-022-00737-7
Luan, H., Liao, W., Song, Y., Niu, H., and Zhi, H. (2020). Transgenic plant generated by RNAi-mediated knocking down of soybean Vma12 and soybean mosaic virus resistance evaluation. AMB Express 10, 1–10. doi: 10.1186/s13568-020-00997-6
Luo, Y., Na, R., Nowak, J. S., Qiu, Y., Lu, Q. S., Yang, C., et al. (2021). Development of a Csy4-processed guide RNA delivery system with soybean-infecting virus ALSV for genome editing. BMC Plant Biol. 21:419. doi: 10.1186/s12870-021-03138-8
Luth, D., Warnberg, K., and Wang, K. (2015). “Soybean [Glycine max (L.) Merr.],” in Agrobacterium Protocols. Methods in Molecular Biology, ed. K. Wang (New York, NY: Springer), 275–284.
Ma, J., Sun, S., Whelan, J., and Shou, H. (2021). CRISPR/Cas9-mediated knockout of GmFATB1 significantly reduced the amount of saturated fatty acids in soybean seeds. Int. J. Mol. Sci. 22:3877. doi: 10.3390/ijms22083877
Ma, X., Xu, W., Liu, T., Chen, R., Zhu, H., Zhang, H., et al. (2021). Functional characterization of soybean (Glycine max) DIRIGENT genes reveals an important role of GmDIR27 in the regulation of pod dehiscence. Genomics 113, 979–990. doi: 10.1016/j.ygeno.2020.10.033
Ma, X. J., Yu, T. F., Li, X. H., Cao, X. Y., Ma, J., Chen, J., et al. (2020). Overexpression of GmNFYA5 confers drought tolerance to transgenic Arabidopsis and soybean plants. BMC Plant Biol. 20:123. doi: 10.1186/s12870-020-02337-z
Mangena, P. (2019). A simplified in-planta genetic transformation in soybean. Res. J. Biotech. 14, 117–125.
Mangena, P., Mokwala, P., and Nikolova, R. V. (2017). “Challenges of in vitro and in vivo Agrobacterium-mediated genetic transformation in soybean,” in Soybean - The Basis of Yield, Biomass and Productivity, ed. M. Kasai (London: IntechOpen), 75–94.
Mariashibu, T. S., Subramanyam, K., Arun, M., Mayavan, S., Rajesh, M., Theboral, J., et al. (2013). Vacuum infiltration enhances the Agrobacterium-mediated genetic transformation in Indian soybean cultivars. Acta Physiol. Plant 35, 41–54. doi: 10.1007/s11738-012-1046-3
Marinho, J. P., Pagliarini, R. F., Molinari, M. D. C., Marcolino-Gomes, J., Caranhoto, A. L. H., Marin, S. R. R., et al. (2022). Overexpression of full-length and partial DREB2A enhances soybean drought tolerance. Agronomy Sci. Biotech. 8, 1–21. doi: 10.33158/ASB.r141.v8.2022
Martinell, B. J., Julson, L. A., Hinchee, M. A. W., Connor-Ward, D., McCabe, D., and Emler, C. (1999). Efficiency Soybean Transformation Protocol. Patent Version No.: US5914451. St. Louis, Mo: U.S. Patent and Trademark Office, Monsanto Company.
Martinell, B. J., Julson, L. S., Emler, C. A., Huang, Y., Mccabe, D. E., and Williams, E. J. (2002). Soybean Agrobacterium Transformation Method. Patent Version No.: US6384301. St. Louis, MO: U.S. Patent and Trademark Office, Monsanto Company.
Mccabe, D. E., Swain, W. F., Martinell, B. J., and Christou, P. (1988). Stable transformation of soybean (Glycine Max) by particle acceleration. Nat. Biotech. 6, 923–926. doi: 10.1038/nbt0888-923
Mehrizadeh, V., Dorani, E., Mohammadi, S. A., and Ghareyazie, B. (2021). Expression of recombinant human IFN-γ protein in soybean (Glycine max L.). Plant Cell Tiss. Org. 146, 127–136. doi: 10.1007/s11240-021-02052-z
Meurer, C. A., Dinkins, R. D., and Collins, G. B. (1998). Factors affecting soybean cotyledonary node transformation. Plant Cell Rep. 18, 180–186. doi: 10.1007/s002990050553
Michno, J. M., Wang, X., Liu, J., Curtin, S. J., Kono, T. J., and Stupar, R. M. (2015). CRISPR/Cas mutagenesis of soybean and Medicago truncatula using a new web-tool and a modified Cas9 enzyme. GM Crops Food 6, 243–252. doi: 10.1080/21645698.2015.1106063
Mittler, R., Herr, E. H., Orvar, B. L., van Camp, W., Willekens, H., Inzé, D., et al. (1999). Transgenic tobacco plants with reduced capability to detoxify reactive oxygen intermediates are hyperresponsive to pathogen infection. Proc. Natl. Acad. Sci. U. S. A. 96, 14165–14170. doi: 10.1073/pnas.96.24.14165
Moghaieb, R. E. A., Khashaba, E. H., and Abdel Elazim, A. M. (2019). The toxicity of Cry1Ia5 transgenic soybean plants against Spodoptera littoralis. J. Plant Prot. Res. 59, 185–191. doi: 10.24425/jppr.2019.129286
Molinari, M. D. C., Fuganti-Pagliarini, R., Marin, S. R. R., Ferreira, L. C., Barbosa, D. A., Marcolino-Gomes, J., et al. (2020). Overexpression of AtNCED3 gene improved drought tolerance in soybean in greenhouse and field conditions. Genet. Mol. Biol. 43:e20190292. doi: 10.1590/1678-4685-gmb-2019-0292
Morgante, M., Jurman, I., Shi, L., Zhu, T., Keim, P., and Rafalski, J. A. (1997). The STR120 satellite DNA of soybean: organization, evolution and chromosomal specificity. Chromosome Res. 5, 363–373. doi: 10.1023/a:1018492208247
Mu, R., Lyu, X., Ji, R., Liu, J., Zhao, T., Li, H., et al. (2022). GmBICs modulate low blue light-induced stem elongation in soybean. Front. Plant Sci. 13:803122. doi: 10.3389/fpls.2022.803122
Murashige, T., and Skoog, F. (1962). A Revised Medium for Rapid Growth and Bio Assays with Tobacco Tissue Cultures. Physiol. Plant. 15, 473–497. doi: 10.1111/j.1399-3054.1962.tb08052.x
Nadeem, M., Chen, A., Hong, H., Li, D., Li, J., Zhao, D., et al. (2021). GmMs1 encodes a kinesin-like protein essential for male fertility in soybean (Glycine max L.). J. Integr. Plant Biol. 63, 1054–1064. doi: 10.1111/jipb.13110
Nagy, E. D., Stevens, J. L., Yu, N., Hubmeier, C. S., LaFaver, N., Gillespie, M., et al. (2021). Novel disease resistance gene paralogs created by CRISPR/Cas9 in soy. Plant Cell Rep. 40, 1047–1058. doi: 10.1007/s00299-021-02678-5
Nandula, V. K. (2019). Herbicide resistance traits in maize and soybean: current status and future outlook. Plants 8:377. doi: 10.3390/plants8090337
Ng, M.-S., Ku, Y.-S., Yung, W.-S., Cheng, S.-S., Man, C.-K., Yang, L., et al. (2021). MATE-type proteins are responsible for isoflavone transportation and accumulation in soybean seeds. Int. J. Mol. Sci. 22:12017. doi: 10.3390/ijms222112017
Ngaki, M. N., Sahoo, D. K., Wang, B., and Bhattacharyya, M. K. (2021). Overexpression of a plasma membrane protein generated broad-spectrum immunity in soybean. Plant Biotechnol. J. 19, 502–516. doi: 10.1111/pbi.13479
Nguyen, C., Paddock, K., Zhang, Z., and Stacey, M. (2021). GmKIX8-1 regulates organ size in soybean and is the causative gene for the major seed weight QTL qSw17-1. New Phytol. 229, 920–934. doi: 10.1111/nph.16928
Nguyen, H. Q., Le, T. H. T., Nguyen, T. N. L., Nguyen, T. G., Sy, D. T., Tu, Q. T., et al. (2020). Overexpressing GmCHI1A increases the isoflavone content of transgenic soybean (Glycine max (L.) Merr.) seeds. In Vitro Cell. Dev. Pl. 56, 842–850. doi: 10.1007/s11627-020-10076-x
Nguyen, N. T., Vu, H. T., Nguyen, T. T., Nguyen, L.-A. T., Nguyen, M.-C. D., Hoang, K. L., et al. (2019). Co-expression of Arabidopsis AtAVP1 and AtNHX1 to improve salt tolerance in soybean. Crop Sci. 59, 1133–1143. doi: 10.2135/cropsci2018.10.0640
Nguyen, Q. H., Vu, L. T. K., Nguyen, L. T. N., Pham, N. T. T., Nguyen, Y. T. H., Le, S. V., et al. (2019). Overexpression of the GmDREB6 gene enhances proline accumulation and salt tolerance in genetically modified soybean plants. Sci. Rep. 9:19663. doi: 10.1038/s41598-019-55895-0
Nicolas, J. J., Richard-Forget, F. C., Goupy, P. M., Amiot, M. J., and Aubert, S. Y. (1994). Enzymatic browning reactions in apple and apple products. Criti. Rev. Food Sci. 34, 109–157. doi: 10.1080/10408399409527653
Niu, L., Yang, J., Zhang, J., He, H., Xing, G., Zhao, Q., et al. (2019). Introduction of the harpinXooc-encoding gene hrf2 in soybean enhances resistance against the oomycete pathogen Phytophthora sojae. Transgenic Res. 28, 257–266. doi: 10.1007/s11248-019-00119-4
O’Conner, S., Zheng, W., Qi, M., Kandel, Y., Fuller, R., Whitham, S. A., et al. (2021). GmNF-YC4-2 increases protein, exhibits broad disease resistance and expedites maturity in soybean. Int. J. Mol. Sci. 22:3586. doi: 10.3390/ijms22073586
Olhoft, P. M., Bernal, L. M., Grist, L. B., Hill, D. S., Mankin, S. L., Shen, Y., et al. (2007). A novel Agrobacterium rhizogenes-mediated transformation method of soybean [Glycine max (L.) Merrill] using primary-node explants from seedlings. In Vitro Cell. Dev. Pl. 43, 536–549. doi: 10.1007/s11627-007-9050-9
Olhoft, P. M., Flagel, L. E., Donovan, C. M., and Somers, D. A. (2003). Efficient soybean transformation using hygromycin B selection in the cotyledonary-node method. Planta 216, 723–735. doi: 10.1007/s00425-002-0922-2
Olhoft, P. M., Flagel, L. E., and Somers, D. A. (2004). T-DNA locus structure in a large population of soybean plants transformed using the Agrobacterium-mediated cotyledonary-node method. Plant Biotechnol. J. 2, 289–300. doi: 10.1111/j.1467-7652.2004.00070.x
Olhoft, P. O., Lin, K. L., Galbraith, J. G., Nielsen, N. N., and Somers, D. S. (2001). The role of thiol compounds in increasing Agrobacterium-mediated transformation of soybean cotyledonary-node cells. Plant Cell Rep. 20, 731–737. doi: 10.1007/s002990100388
Olhoft, P. O., and Somers, D. S. (2001). L-Cysteine increases Agrobacterium-mediated T-DNA delivery into soybean cotyledonary-node cells. Plant Cell Rep. 20, 706–711. doi: 10.1007/s002990100379
Opabode, J. T. (2006). Agrobacterium-mediated transformation of plants: emerging factors that influence efficiency. Biotech. Mol. Bio. Rev. 1, 12–20.
Paes de Melo, B., Lourenço-Tessutti, I., Morgante, C., Santos, N., Pinheiro, L., Lins, C., et al. (2020). Soybean embryonic axis transformation: combining biolistic and Agrobacterium-mediated protocols to overcome typical complications of in vitro plant regeneration. Front. Plant Sci. 11:1228. doi: 10.3389/fpls.2020.01228
Pagano, M. C., and Miransari, M. (2016). “The importance of soybean production worldwide,” in Abiotic and Biotic Stresses in Soybean Production, ed. M. Miransari (San Diego: Academic Press), 1–26.
Pareddy, D., Chennareddy, S., Anthony, G., Sardesai, N., Mall, T., Minnicks, T., et al. (2020). Improved soybean transformation for efficient and high throughput transgenic production. Transgenic Res. 29, 267–281. doi: 10.1007/s11248-020-00198-8
Park, J. S., Kim, H. J., Cho, H. S., Jung, H. W., Cha, J. Y., Yun, D. J., et al. (2019). Overexpression of AtYUCCA6 in soybean crop results in reduced ROS production and increased drought tolerance. Plant Biotechnol. Rep. 13, 161–168. doi: 10.1007/s11816-019-00527-2
Parrott, W. A., Williams, E. G., Hildebrand, D. F., and Collins, G. B. (1989). Effect of genotype on somatic embryogenesis from immature cotyledons of soybean. Plant Cell Tiss. Org. 16, 15–21. doi: 10.1007/BF00044068
Patil, G. B., Stupar, R. M., and Zhang, F. (2022). “Protoplast Isolation, transfection, and gene editing for soybean (Glycine max),” in Proc. Natl. Acad. Sci, eds K. Wang and F. Zhang (New York, NY: Springer US), 173–186. doi: 10.1007/978-1-0716-2164-6_13
Paz, M. M., Martinez, J. C., Kalvig, A. B., Fonger, T. M., and Wang, K. (2006). Improved cotyledonary node method using an alternative explant derived from mature seed for efficient Agrobacterium-mediated soybean transformation. Plant Cell Rep. 25, 206–213. doi: 10.1007/s00299-005-0048-7
Paz, M. M., Shou, H., Guo, Z., Zhang, Z., Banerjee, A. K., and Wang, K. (2004). Assessment of conditions affecting Agrobacterium-mediated soybean transformation using the cotyledonary node explant. Euphytica 136, 167–179. doi: 10.1023/B:EUPH.0000030669.75809.dc
Pham, T. T. N., Nguyen, H. Q., Nguyen, T. N. L., Dao, X. T., Sy, D. T., Le, V. S., et al. (2020). Overexpression of the GmDREB2 gene increases proline accumulation and tolerance to drought stress in soybean plant. Australian J. Crop Sci. 14, 495–503. doi: 10.21475/ajcs.20.14.03.p2173
Pottinger, S. E., Bak, A., Margets, A., Helm, M., Tang, L., Casteel, C., et al. (2020). Optimizing the PBS1 decoy system to confer resistance to potyvirus infection in Arabidopsis and soybean. Mol. Plant Microbe Interact. 33, 932–944. doi: 10.1094/MPMI-07-19-0190-R
Punjabi, M., Bharadvaja, N., Jolly, M., Dahuja, A., and Sachdev, A. (2018). Development and evaluation of low phytic acid soybean by siRNA triggered seed specific silencing of inositol polyphosphate 6-/3-/5-kinase gene. Front. Plant Sci. 9:804. doi: 10.3389/fpls.2018.00804
Qin, D., Liu, X. Y., Miceli, C., Zhang, Q., and Wang, P. W. (2019). Soybean plants expressing the Bacillus thuringiensis cry8-like gene show resistance to Holotrichia parallela. BMC Biotechnol. 19:66. doi: 10.1186/s12896-019-0563-1
Qu, S., Jiao, Y., Abraham, L., and Wang, P. (2021). Correlation analysis of new soybean [Glycine max (L.) Merr] gene Gm15G117700 with oleic acid. Phyton 90, 1177–1192. doi: 10.32604/phyton.2021.015206
Queiroz, L. N., Maldaner, F. R., Mendes, E. A., Sousa, A. R., D’Allastta, R. C., Mendonca, G., et al. (2019). Evaluation of lettuce chloroplast and soybean cotyledon as platforms for production of functional bone morphogenetic protein 2. Transgenic Res. 28, 213–224. doi: 10.1007/s11248-019-00116-7
Rakesh, B., Sudheer, W. N., and Nagella, P. (2021). Role of polyamines in plant tissue culture: an overview. Plant Cell Tiss. Org. 145, 487–506. doi: 10.1007/s11240-021-02029-y
Ramesh, S. V., Shivakumar, M., Praveen, S., Chouhan, B. S., and Chand, S. (2019). Expression of short hairpin RNA (shRNA) targeting AC2 gene of Mungbean yellow mosaic India virus (MYMIV) reduces the viral titre in soybean. 3 Biotech 9:334. doi: 10.1007/s13205-019-1865-7
Ran, Y., Liang, Z., and Gao, C. (2017). Current and future editing reagent delivery systems for plant genome editing. Sci. China Life Sci. 60, 490–495. doi: 10.1007/s11427-017-9022-1
Raza, G., Singh, M., and Bhalla, P. (2020). Somatic embryogenesis and plant regeneration from commercial soybean cultivars. Plants 9:38. doi: 10.3390/plants9010038
Rech, E. L., Vianna, G. R., and Aragao, F. J. (2008). High-efficiency transformation by biolistics of soybean, common bean and cotton transgenic plants. Nat. Protoc. 3, 410–418. doi: 10.1038/nprot.2008.9
Rehman, N. U., Abbas, F., Imran, M., Alam, I., Imran, M., Ullah, I., et al. (2022). Genome wide analysis of DWARF27 genes in soybean and functional characterization of GmD27c reveals eminent role of strigolactones in rhizobia interaction and nodulation in Glycine max. Mol. Bio. Rep. [Epub ahead of print]. doi: 10.1007/s11033-022-07127-4
Ribichich, K. F., Chiozza, M., Avalos-Britez, S., Cabello, J. V., Arce, A. L., Watson, G., et al. (2020). Successful field performance in warm and dry environments of soybean expressing the sunflower transcription factor HB4. J. Exp. Bot. 71, 3142–3156. doi: 10.1093/jxb/eraa064
Roustan, J. P., Latche, A., and Fallot, J. (1990). Inhibition of ethylene production and stimulation of carrot somatic embryogenesis by salicylic acid. Biol. Plant. 32, 273–276. doi: 10.1007/BF02886947
Sander, J. D., Dahlborg, E. J., Goodwin, M. J., Cade, L., Zhang, F., Cifuentes, D., et al. (2011). Selection-free zinc-finger-nuclease engineering by context-dependent assembly (CoDA). Nat. Methods 8, 67–69. doi: 10.1038/nmeth.1542
Sato, H., Yamada, T., Kita, Y., Ishimoto, M., and Kitamura, K. (2007). Production of transgenic plants and their early seed set in Japanese soybean variety, Kariyutaka. Plant Biotechnol. 24, 533–536. doi: 10.5511/plantbiotechnology.24.533
Sato, S., Newell, C., Kolacz, K., Tredo, L., Finer, J., and Hinchee, M. (1993). Stable transformation via particle bombardment in two different soybean regeneration systems. Plant Cell Rep. 12, 408–413. doi: 10.1007/BF00234702
Schmutz, J., Cannon, S. B., Schlueter, J., Ma, J., Mitros, T., Nelson, W., et al. (2010). Genome sequence of the palaeopolyploid soybean. Nature 463, 178–183. doi: 10.1038/nature08670
Shanmugam, S., Zhao, S., Nandy, S., Srivastava, V., and Khodakovskaya, M. (2020). Modification of soybean growth and abiotic stress tolerance by expression of truncated ERECTA protein from Arabidopsis thaliana. PLoS One 15:e0233383. doi: 10.1371/journal.pone.0233383
Shen, Y., Liu, J., Geng, H., Zhang, J., Liu, Y., Zhang, H., et al. (2018). De novo assembly of a Chinese soybean genome. Sci. China Life Sci. 61, 871–884. doi: 10.1007/s11427-018-9360-0
Shi, W. Y., Du, Y. T., Ma, J., Min, D. H., Jin, L. G., Chen, J., et al. (2018). The WRKY transcription factor GmWRKY12 confers drought and salt tolerance in soybean. Int. J. Mol. Sci. 19:4087. doi: 10.3390/ijms19124087
Shimomura, M., Kanamori, H., Komatsu, S., Namiki, N., Mukai, Y., Kurita, K., et al. (2015). The Glycine max cv. enrei genome for improvement of Japanese soybean cultivars. Int. J. Genomics 2015: 358127. doi: 10.1155/2015/358127
Song, J., Toth, K., Montes-Luz, B., and Stacey, G. (2021). Soybean hairy root transformation: a rapid and highly efficient method. Curr. Protoc. 1:e195. doi: 10.1002/cpz1.195
Song, L., Valliyodan, B., Prince, S., Wan, J., and Nguyen, H. T. (2018). Characterization of the XTH gene family: new insight to the roles in soybean flooding tolerance. Int. J. Mol. Sci. 19:2705. doi: 10.3390/ijms19092705
Song, Z. Y., Tian, J.l, Fu, W. Z., Li, L., Lu, L. H., Zhou, L., et al. (2013). Screening Chinese soybean genotypes for Agrobacterium-mediated genetic transformation suitability. J. Zhejiang Univ. Sci. B 14, 289–298. doi: 10.1631/jzus.B1200278
Soto, N., Delgado, C., Hernández, Y., Rosabal, Y., Ferreira, A., Pujol, M., et al. (2017). Efficient particle bombardment-mediated transformation of Cuban soybean (INCASoy-36) using glyphosate as a selective agent. Plant Cell Tiss. Org. 128, 187–196. doi: 10.1007/s11240-016-1099-x
Soto, N., Hernandez, Y., Delgado, C., Rosabal, Y., Ortiz, R., Valencia, L., et al. (2020). Field resistance to Phakopsora pachyrhizi and Colletotrichum truncatum of transgenic soybean expressing the NmDef02 plant defensin gene. Front. Plant Sci. 11:562. doi: 10.3389/fpls.2020.00562
Stacey, G., Vodkin, L., Parrott, W. A., and Shoemaker, R. C. (2004). National science foundation-sponsored workshop report. draft plan for soybean genomics. Plant Physiol. 135, 59–70. doi: 10.1104/pp.103.037903
Subramanian, S., Hu, X., Lu, G., Odelland, J. T., and Yu, O. (2004). The promoters of two isoflavone synthase genes respond differentially to nodulation and defense signals in transgenic soybean roots. Plant Mol. Biol. 54, 623–639. doi: 10.1023/B:PLAN.0000040814.28507.35
Sugano, S., Hirose, A., Kanazashi, Y., Adachi, K., Hibara, M., Itoh, T., et al. (2020). Simultaneous induction of mutant alleles of two allergenic genes in soybean by using site-directed mutagenesis. BMC Plant Biol. 20:513. doi: 10.1186/s12870-020-02708-6
Sultana, M. S., Frazier, T. P., Millwood, R. J., Lenaghan, S. C., and Stewart, C. N. (2019). Development and validation of a novel and robust cell culture system in soybean (Glycine max (L.) Merr.) for promoter screening. Plant Cell Rep. 38, 1329–1345. doi: 10.1007/s00299-019-02455-5
Sun, T., Ma, N., Wang, C., Fan, H., Wang, M., Zhang, J., et al. (2021). A golgi-localized sodium/hydrogen exchanger positively regulates salt tolerance by maintaining higher K+/Na+ ratio in soybean. Front. Plant Sci. 12:638340. doi: 10.3389/fpls.2021.638340
Sun, X., Cai, X., Yin, K., Gu, L., Shen, Y., Hu, B., et al. (2021). Wild soybean SNARE proteins BET1s mediate the subcellular localization of the cytoplasmic receptor-like kinases CRCK1s to modulate salt stress responses. Plant J. 105, 771–785. doi: 10.1111/tpj.15072
Sun, X., Hu, Z., Chen, R., Jiang, Q., Song, G., Zhang, H., et al. (2015). Targeted mutagenesis in soybean using the CRISPR-Cas9 system. Sci. Rep. 5:10342. doi: 10.1038/srep10342
Sun, Z., Su, C., Yun, J., Jiang, Q., Wang, L., Wang, Y., et al. (2019). Genetic improvement of the shoot architecture and yield in soya bean plants via the manipulation of GmmiR156b. Plant Biotechnol. J. 17, 50–62. doi: 10.1111/pbi.12946
Testroet, A., Lee, K., Luth, D., and Wang, K. (2017). Comparison of transformation frequency using the bar gene regulated by the CaMV 35S or NOS promoter in Agrobacterium-mediated soybean (Glycine max L.) transformation. In Vitro Cell. Dev. Pl. 53, 188–199. doi: 10.1007/s11627-017-9810-0
Tian, B., Li, J., Vodkin, L. O., Todd, T. C., Finer, J. J., and Trick, H. N. (2019). Host-derived gene silencing of parasite fitness genes improves resistance to soybean cyst nematodes in stable transgenic soybean. Theor. Appl. Genet. 132, 2651–2662. doi: 10.1007/s00122-019-03379-0
Torkamaneh, D., Laroche, J., Valliyodan, B., O’Donoughue, L., Cober, E., Rajcan, I., et al. (2019). Soybean haplotype map (GmHapMap): a universal resource for soybean translational and functional genomics. BioRxiv [preprint] doi: 10.1101/534578
Trick, H. N., and Finer, J. J. (1998). Sonication-assisted Agrobacterium-mediated transformation of soybean [Glycine max (L.) Merrill] embryogenic suspension culture tissue. Plant Cell Rep. 17, 482–488. doi: 10.1007/s002990050429
Umezawa, T., Sakurai, T., Totoki, Y., Toyoda, A., Seki, M., Ishiwata, A., et al. (2008). Sequencing and analysis of approximately 40,000 soybean cDNA clones from a full-length-enriched cDNA library. DNA Res. 15, 333–346. doi: 10.1093/dnares/dsn024
Veerappa, R., Slocum, R. D., Siegenthaler, A., Wang, J., Clark, G., and Roux, S. J. (2019). Ectopic expression of a pea apyrase enhances root system architecture and drought survival in Arabidopsis and soybean. Plant Cell Environ. 42, 337–353. doi: 10.1111/pce.13425
Verma, N., Tiwari, S., Singh, V. P., and Prasad, S. M. (2020). Nitric oxide in plants: an ancient molecule with new tasks. Plant Growth Regul. 90, 1–13. doi: 10.1007/s10725-019-00543-w
Vianna, G. R., Aragao, F. J., and Rech, E. L. (2011). A minimal DNA cassette as a vector for genetic transformation of soybean (Glycine max). Genet. Mol. Biol. 10, 382–390. doi: 10.4238/vol10-1gmr1058
Voora, V., Larrea, C., and Bermudez, S. (2020). “Global market report: soybeans,” in International Institute for Sustainable Development, ed. S. Baliño (Winnipeg, Manitoba: International Institute for Sustainable Development).
Wang, B., Sumit, R., Sahu, B. B., Ngaki, M. N., Srivastava, S. K., Yang, Y., et al. (2018a). Arabidopsis novel glycine-rich plasma membrane PSS1 protein enhances disease resistance in transgenic soybean plants. Plant Physiol. 176, 865–878. doi: 10.1104/pp.16.01982
Wang, L. S., Chen, Q. S., Xin, D. W., Qi, Z. M., Zhang, C., Li, S. N., et al. (2018b). Overexpression of GmBIN2, a soybean glycogen synthase kinase 3 gene, enhances tolerance to salt and drought in transgenic Arabidopsis and soybean hairy roots. J. Integr. Agr. 17, 1959–1971. doi: 10.1016/S2095-3119(17)61863-X
Wang, Y., Jiang, L., Chen, J., Tao, L., An, Y., Cai, H., et al. (2018c). Overexpression of the alfalfa WRKY11 gene enhances salt tolerance in soybean. PLoS One 13:e0192382. doi: 10.1371/journal.pone.0192382
Wang, G., and Xu, Y. (2008). Hypocotyl-based Agrobacterium-mediated transformation of soybean (Glycine max) and application for RNA interference. Plant Cell Rep. 27, 1177–1184. doi: 10.1007/s00299-008-0535-8
Wang, Y., Yang, Z., Kong, Y., Li, X., Li, W., Du, H., et al. (2020d). GmPAP12 is required for nodule development and nitrogen fixation under phosphorus starvation in soybean. Front. Plant Sci. 11:450. doi: 10.3389/fpls.2020.00450
Wang, S., Liu, S., Wang, J., Yokosho, K., Zhou, B., Yu, Y.-C., et al. (2020c). Simultaneous changes in seed size, oil content, and protein content driven by selection of SWEET homologues during soybean domestication. Nat. Sci. Rev. 7, 1776–1786. doi: 10.1093/nsr/nwaa110
Wang, L., Sun, S., Wu, T., Liu, L., Sun, X., Cai, Y., et al. (2020b). Natural variation and CRISPR/Cas9-mediated mutation in GmPRR37 affect photoperiodic flowering and contribute to regional adaptation of soybean. Plant Biotechnol. J. 18, 1869–1881. doi: 10.1111/pbi.13346
Wang, J., Kuang, H., Zhang, Z., Yang, Y., Yan, L., Zhang, M., et al. (2020a). Generation of seed lipoxygenase-free soybean using CRISPR-Cas9. Crop J. 8, 432–439. doi: 10.1016/j.cj.2019.08.008
Wang, Y., Yuan, L., Su, T., Wang, Q., Gao, Y., Zhang, S., et al. (2020e). Light- and temperature-entrainable circadian clock in soybean development. Plant Cell Environ. 43, 637–648. doi: 10.1111/pce.13678
Wang, X., Li, M.-W., Wong, F.-L., Luk, C.-Y., Chung, C. Y.-L., Yung, W.-S., et al. (2021d). Increased copy number of gibberellin 2-oxidase 8 genes reduced trailing growth and shoot length during soybean domestication. Plant J. 107, 1739–1755. doi: 10.1111/tpj.15414
Wang, R., Deng, M., Yang, C., Yu, Q., Zhang, L., Zhu, Q., et al. (2021b). A Qa-SNARE complex contributes to soybean cyst nematode resistance via regulation of mitochondria-mediated cell death. J. Exp. Bot. 72, 7145–7162. doi: 10.1093/jxb/erab301
Wang, W., Chen, L., Fengler, K., Bolar, J., Llaca, V., Wang, X., et al. (2021c). A giant NLR gene confers broad-spectrum resistance to Phytophthora sojae in soybean. Nat. Commun. 12:6263. doi: 10.1038/s41467-021-26554-8
Wang, X., Zhao, J., Fang, Q., Chang, X., Sun, M., Li, W., et al. (2021e). GmAKT1 is involved in K+ uptake and Na+/K+ homeostasis in Arabidopsis and soybean plants. Plant Sci. 304, 110736. doi: 10.1016/j.plantsci.2020.110736
Wang, K., Bu, T., Cheng, Q., Dong, L., Su, T., Chen, Z., et al. (2021a). Two homologous LHY pairs negatively control soybean drought tolerance by repressing the abscisic acid responses. New Phytol. 229, 2660–2675. doi: 10.1111/nph.17019
Wang, Y., Yang, W., Zuo, Y., Zhu, L., Hastwell, A. H., Chen, L., et al. (2019c). GmYUC2a mediates auxin biosynthesis during root development and nodulation in soybean. J. Exp. Bot. 70, 3165–3176. doi: 10.1093/jxb/erz144
Wang, L., Wang, H., He, S., Meng, F., Zhang, C., Fan, S., et al. (2019a). GmSnRK1.1, a sucrose non-fermenting-1(SNF1)-related protein kinase, promotes soybean resistance to Phytophthora sojae. Front. Plant Sci. 10:996. doi: 10.3389/fpls.2019.00996
Wang, S., Yokosho, K., Guo, R., Whelan, J., Ruan, Y. L., Ma, J. F., et al. (2019b). The soybean sugar transporter GmSWEET15 mediates sucrose export from endosperm to early embryo. Plant Physiol. 180, 2133–2141. doi: 10.1104/pp.19.00641
Wang, P., and Zhao, F.-J. (2019). Engineering crops without genome integration using nanotechnology. Trends Plant Sci. 24, 574–577. doi: 10.1016/j.tplants.2019.05.004
Wang, W., Wang, Z., Hou, W., Chen, L., Jiang, B., Ma, W., et al. (2022). GmNMHC5 may promote nodulation via interaction with GmGAI in soybean. Crop J. 10, 273–279. doi: 10.1016/j.cj.2021.03.019
Wang, Z., Wang, Y., Shang, P., Yang, C., Yang, M., Huang, J., et al. (2022). Overexpression of soybean GmWRI1a stably increases the seed oil content in soybean. Int. J. Mol. Sci. 23:5084. doi: 10.3390/ijms23095084
Wei, P., Che, B., Shen, L., Cui, Y., Wu, S., Cheng, C., et al. (2019). Identification and functional characterization of the chloride channel gene, GsCLC-c2 from wild soybean. BMC Plant Biol. 19:121. doi: 10.1186/s12870-019-1732-z
Wei, W., Liang, D. W., Bian, X. H., Shen, M., Xiao, J. H., Zhang, W. K., et al. (2019). GmWRKY54 improves drought tolerance through activating genes in abscisic acid and Ca2+ signaling pathways in transgenic soybean. Plant J. 100, 384–398. doi: 10.1111/tpj.14449
Wu, F., and Hanzawa, Y. (2018). A simple method for isolation of soybean protoplasts and application to transient gene expression analyses. J. Vis. Exp. 131:e57258. doi: 10.3791/57258
Wu, N., Lu, Q., Wang, P., Zhang, Q., Zhang, J., Qu, J., et al. (2020). Construction and analysis of GmFAD2-1A and GmFAD2-2A soybean fatty acid desaturase mutants based on CRISPR/Cas9 technology. Int. J. Mol. Sci. 21:1104. doi: 10.3390/ijms21031104
Xian, P., Cai, Z., Cheng, Y., Lin, R., Lian, T., Ma, Q., et al. (2020). Wild soybean oxalyl-CoA synthetase degrades oxalate and affects the tolerance to cadmium and aluminum stresses. Int. J. Mol. Sci. 21:8869. doi: 10.3390/ijms21228869
Xiao, P. Y., Liu, Y., and Cao, Y. P. (2019). Overexpression of G10-EPSPS in soybean provides high glyphosate tolerance. J. Integr. Agr. 18, 1851–1858. doi: 10.1016/S2095-3119(18)62124-0
Xie, M., Chung, C. Y., Li, M. W., Wong, F. L., Wang, X., Liu, A., et al. (2019). A reference-grade wild soybean genome. Nat. Commun. 10, 1–12. doi: 10.1038/s41467-019-09142-9
Xing, X., Du, H., Yang, Z., Li, X., Kong, Y., Li, W., et al. (2022). GmSPX8, a nodule-localized regulator confers nodule development and nitrogen fixation under phosphorus starvation in soybean. BMC Plant Biol. 22:161. doi: 10.1186/s12870-022-03556-2
Xiong, L., Li, C., Li, H., Lyu, X., Zhao, T., Liu, J., et al. (2019). A transient expression system in soybean mesophyll protoplasts reveals the formation of cytoplasmic GmCRY1 photobody-like structures. Sci. China Life Sci. 62, 1070–1077. doi: 10.1007/s11427-018-9496-5
Xu, H., Zhang, L., Zhang, K., and Ran, Y. (2020). Progresses, challenges, and prospects of genome editing in soybean (Glycine max). Front. Plant Sci. 11:571138. doi: 10.3389/fpls.2020.571138
Xu, M., Li, H., Liu, Z.-N., Wang, X.-H., Xu, P., Dai, S.-J., et al. (2021). The soybean CBL-interacting protein kinase, GmCIPK2, positively regulates drought tolerance and ABA signaling. Plant Physiol. Biochem. 167, 980–989. doi: 10.1016/j.plaphy.2021.09.026
Xu, Y., Yan, F., Zong, Y., Li, J., Gao, H., Liu, Y., et al. (2022). Proteomic and lipidomics analyses of high fatty acid AhDGAT3 transgenic soybean reveals the key lipase gene associated with the lipid internal mechanism. Genome 65, 153–164. doi: 10.1139/gen-2021-0043
Xue, R. G., Xie, H. F., and Zhang, B. (2006). A multi-needle-assisted transformation of soybean cotyledonary node cells. Biotechnol. Lett. 28, 1551–1557. doi: 10.1007/s10529-006-9123-6
Xun, H., Yang, X., He, H., Wang, M., Guo, P., Wang, Y., et al. (2019). Over-expression of GmKR3, a TIR-NBS-LRR type R gene, confers resistance to multiple viruses in soybean. Plant Mol. Biol. 99, 95–111. doi: 10.1007/s11103-018-0804-z
Yamada, T., Takagi, K., and Ishimoto, M. (2012). Recent advances in soybean transformation and their application to molecular breeding and genomic analysis. Breed. Sci. 61, 480–494. doi: 10.1270/jsbbs.61.480
Yamada, T., Watanabe, S., Arai, M., Harada, K., and Kitamura, K. (2010). Cotyledonary node pre-wounding with a micro-brush increased frequency of Agrobacterium-mediated transformation in soybean. Plant Biotechnol. 27, 217–220. doi: 10.5511/plantbiotechnology.27.217
Yan, B., Srinivasa Reddy, M. S., Collins, G. B., and Dinkins, R. D. (2000). Agrobacterium tumefaciens- mediated transformation of soybean [Glycine max (L.) Merrill.] using immature zygotic cotyledon explants. Plant Cell Rep. 19, 1090–1097. doi: 10.1007/s002990000236
Yan, Q., Si, J., Cui, X., Peng, H., and Dou, D. (2019). The soybean cinnamate 4-hydroxylase gene GmC4H1 contributes positively to plant defense via increasing lignin content. Plant Growth Regul. 88, 139–149. doi: 10.1007/s10725-019-00494-2
Yang, S., Feng, Y., Zhao, Y., Bai, J., and Wang, J. (2020b). Overexpression of a Eutrema salsugineum phosphate transporter gene EsPHT1;4 enhances tolerance to low phosphorus stress in soybean. Biotechnol. Lett. 42, 2425–2439. doi: 10.1007/s10529-020-02968-0
Yang, X., Yang, J., Li, H., Niu, L., Xing, G., Zhang, Y., et al. (2020c). Overexpression of the chitinase gene CmCH1 from Coniothyrium minitans renders enhanced resistance to Sclerotinia sclerotiorum in soybean. Transgenic Res. 29, 187–198. doi: 10.1007/s11248-020-00190-2
Yang, Y., Yu, T.-F., Ma, J., Chen, J., Zhou, Y.-B., Chen, M., et al. (2020d). The soybean bZIP transcription factor gene GmbZIP2 confers drought and salt resistance in transgenic plants. Int. J. Mol. Sci. 21:670. doi: 10.3390/ijms21020670
Yang, C., Huang, Y., Lv, W., Zhang, Y., Bhat, J. A., Kong, J., et al. (2020a). GmNAC8 acts as a positive regulator in soybean drought stress. Plant Sci. 293:110442. doi: 10.1016/j.plantsci.2020.110442
Yang, J., Xing, G., Niu, L., He, H., Guo, D., Du, Q., et al. (2018). Improved oil quality in transgenic soybean seeds by RNAi-mediated knockdown of GmFAD2-1B. Transgenic Res. 27, 155–166. doi: 10.1007/s11248-018-0063-4
Yang, X., Niu, L., Zhang, W., Yang, J., Xing, G., He, H., et al. (2018). RNAi-mediated SMV P3 cistron silencing confers significantly enhanced resistance to multiple Potyvirus strains and isolates in transgenic soybean. Plant Cell Rep. 37, 103–114. doi: 10.1007/s00299-017-2186-0
Yang, J., Xing, G., Qian, D. U., Sui, L., Guo, D., Niu, L., et al. (2016). Effects of different soybean genotypes on the transformation efficiency of soybean and analysis of the T-DNA insertions in the soybean genome. Soybean Sci. 35, 562–567. doi: 10.11861/j.issn.1000-9841.2016.04.0562
Yang, X. F., Yu, X. Q., Zhou, Z., Ma, W. J., and Tang, G. X. (2016). A high-efficiency Agrobacterium tumefaciens mediated transformation system using cotyledonary node as explants in soybean (Glycine max L.). Acta Physiol. Plant. 38:60. doi: 10.1007/s11738-016-2081-2
Yang, S., Yanfeng, H., Cheng, Z., Rice, J., Miao, L., Ma, J., et al. (2019). An efficient Agrobacterium-mediated soybean transformation method using green fluorescent protein as a selectable marker. Plant Signal. Behav. 14, 1–7. doi: 10.1080/15592324.2019.1612682
Yang, X., Yang, J., Wang, Y., He, H., Niu, L., Guo, D., et al. (2019). Enhanced resistance to sclerotinia stem rot in transgenic soybean that overexpresses a wheat oxalate oxidase. Transgenic Res. 28, 103–114. doi: 10.1007/s11248-018-0106-x
Yang, X., Li, X., Shan, J., Li, Y., Zhang, Y., Wang, Y., et al. (2021). Overexpression of GmGAMYB accelerate the transition to flowering and increases plant height in soybean. Front. Plant Sci. 12:667242. doi: 10.3389/fpls.2021.667242
Yang, Z., Du, H., Xing, X., Li, W., Kong, Y., Li, X., et al. (2022). A small heat shock protein, GmHSP17.9, from nodule confers symbiotic nitrogen fixation and seed yield in soybean. Plant Biotechnol. J. 20, 103–115. doi: 10.1111/pbi.13698
Yao, S. (2001). Optimization of Agrobacterium-mediated Genetic Transformation of Soybean Using Glufosinate as a Selective Agent. Ph.D. thesis. Baton Rouge, LA: Louisiana State University. https://digitalcommons.lsu.edu/gradschool_disstheses/329
Yeom, W., Kim, H., Lee, K.-R., Cho, H., Kim, J.-Y., Jung, H. W., et al. (2020). Increased production of α-linolenic acid in soybean seeds by overexpression of lesquerella FAD3-1. Front. Plant Sci. 10:1812. doi: 10.3389/fpls.2019.01812
Yi, J., Derynck, M. R., Li, X., Telmer, P., Marsolais, F., and Dhaubhadel, S. (2010). A single-repeat MYB transcription factor, GmMYB176, regulates CHS8 gene expression and affects isoflavonoid biosynthesis in soybean. Plant J. 62, 1019–1034. doi: 10.1111/j.1365-313X.2010.04214.x
Yi, X., and Yu, D. (2006). Transformation of multiple soybean cultivars by infecting cotyledonary-node with Agrobacterium tumefaciens. Afr. J. Biotech. 5, 1989–1993. doi: 10.5897/AJB2006.000-5100
Yu, G., Zou, J., Wang, J., Zhu, R., Qi, Z., Jiang, H., et al. (2022). A soybean NAC homolog contributes to resistance to Phytophthora sojae mediated by dirigent proteins. Crop J. 10, 332–341. doi: 10.1016/j.cj.2021.08.009
Yue, Y., Sun, S., Li, J., Yu, H., Wu, H., Sun, B., et al. (2021). GmFULa improves soybean yield by enhancing carbon assimilation without altering flowering time or maturity. Plant Cell Rep. 40, 1875–1888. doi: 10.1007/s00299-021-02752-y
Yun, J., Sun, Z., Jiang, Q., Wang, Y., Wang, C., Luo, Y., et al. (2022). The miR156b-GmSPL9d module modulates nodulation by targeting multiple core nodulation genes in soybean. New Phytol. 233, 1881–1899. doi: 10.1111/nph.17899
Zeng, P., Vadnais, D. A., Zhang, Z., and Polacco, J. C. (2004). Refined glufosinate selection in Agrobacterium-mediated transformation of soybean [Glycine max (L.) Merrill]. Plant Cell Rep. 22, 478–482. doi: 10.1007/s00299-003-0712-8
Zhang, B., and Xue, R. G. (2019). Refined factors in multi-needle-assisted transformation of soybean. Asian J. Biotechnol. Genet. Eng. 2, 1–7.
Zhang, D., Zhang, H., Hu, Z., Chu, S., Yu, K., Lv, L., et al. (2019a). Artificial selection on GmOLEO1 contributes to the increase in seed oil during soybean domestication. PLoS Genet. 15:e1008267. doi: 10.1371/journal.pgen.1008267
Zhang, L., Li, T., Wang, Y., Zhang, Y., and Dong, Y. S. (2019b). FvC5SD overexpression enhances drought tolerance in soybean by reactive oxygen species scavenging and modulating stress-responsive gene expression. Plant Cell Rep. 38, 1039–1051. doi: 10.1007/s00299-019-02424-y
Zhang, W., Liao, X., Cui, Y., Ma, W., Zhang, X., Du, H., et al. (2019c). A cation diffusion facilitator, GmCDF1, negatively regulates salt tolerance in soybean. PLoS Genet. 15:e1007798. doi: 10.1371/journal.pgen.1007798
Zhang, X. Z., Zheng, W. J., Cao, X. Y., Cui, X. Y., Zhao, S. P., Yu, T. F., et al. (2019d). Genomic analysis of stress associated proteins in soybean and the role of GmSAP16 in abiotic stress responses in Arabidopsis and soybean. Front. Plant Sci. 10:1453. doi: 10.3389/fpls.2019.01453
Zhang, F., Chen, C., Ge, H., Liu, J., Luo, Y., Liu, K., et al. (2014). Efficient soybean regeneration and Agrobacterium-mediated transformation using a whole cotyledonary node as an explant. Biotech. App. Biochem. 61, 620–625. doi: 10.1002/bab.1207
Zhang, H., Yang, Y., Sun, C., Liu, X., Lv, L., Hu, Z., et al. (2020). Up-regulating GmETO1 improves phosphorus uptake and use efficiency by promoting root growth in soybean. Plant Cell Environ. 43, 2080–2094. doi: 10.1111/pce.13816
Zhang, M., Liu, Y., Cai, H., Guo, M., Chai, M., She, Z., et al. (2020). The bZIP transcription factor GmbZIP15 negatively regulates salt- and drought-stress responses in soybean. Int. J. Mol. Sci. 21:7778. doi: 10.3390/ijms21207778
Zhang, Y., Zhao, Q., Zhang, J., Niu, L., Yang, J., Liu, X., et al. (2022c). Enhanced resistance to soybean cyst nematode in transgenic soybean via host-induced silencing of vital Heterodera glycines genes. Transgenic Res. 31, 239–248. doi: 10.1007/s11248-022-00298-7
Zhang, H.-Y., Hou, Z.-H., Zhang, Y., Li, Z.-Y., Chen, J., Zhou, Y.-B., et al. (2022a). A soybean EF-Tu family protein GmEF8, an interactor of GmCBL1, enhances drought and heat tolerance in transgenic Arabidopsis and soybean. Int. J. Biol. Macromol. 205, 462–472. doi: 10.1016/j.ijbiomac.2022.01.165
Zhang, Y., Guo, W., Chen, L., Shen, X., Yang, H., Fang, Y., et al. (2022b). CRISPR/Cas9-mediated targeted mutagenesis of GmUGT enhanced soybean resistance against leaf-chewing insects through flavonoids biosynthesis. Front. Plant Sci. 13:802716. doi: 10.3389/fpls.2022.802716
Zhang, W. J., Dewey, R. E., Boss, W., Phillippy, B. Q., and Qu, R. (2013). Enhanced Agrobacterium-mediated transformation efficiencies in monocot cells is associated with attenuated defense responses. Plant Mol. Biol. 81, 273–286. doi: 10.1007/s11103-012-9997-8
Zhang, Y. M., Liu, Z. H., Yang, R. J., Li, G. L., Guo, X. L., Zhang, H. N., et al. (2016). Improvement of soybean transformation via Agrobacterium tumefaciens methods involving α-aminooxyacetic acid and sonication treatments enlightened by gene expression profile analysis. Plant Cell Rep. 35, 1259–1271. doi: 10.1007/s00299-016-1958-2
Zhang, Y.-M., Zhang, H.-M., Liu, Z.-H., Guo, X.-L., Li, H.-C., Li, G.-L., et al. (2015). Inhibition of isoflavone biosynthesis enhanced T-DNA delivery in soybean by improving plant–Agrobacterium tumefaciens interaction. Plant Cell Tiss. Org. 121, 183–193. doi: 10.1007/s11240-014-0693-z
Zhang, Z., Xing, A., Staswick, P., and Clemente, T. E. (1999). The use of glufosinate as a selective agent in Agrobacterium-mediated transformation of soybean. Plant Cell Tiss. Org. 56, 37–46. doi: 10.1023/A:1006298622969
Zhao, C., Pan, X., Yu, Y., Zhu, Y., Kong, F., Sun, X., et al. (2020). Overexpression of a TIFY family gene, GsJAZ2, exhibits enhanced tolerance to alkaline stress in soybean. Mol. Breed. 40:33. doi: 10.1007/s11032-020-01113-z
Zhao, Q., Du, Y., Wang, H., Rogers, H. J., Yu, C., Liu, W., et al. (2019b). 5-Azacytidine promotes shoot regeneration during Agrobacterium-mediated soybean transformation. Plant Physiol. Biochem. 141, 40–50. doi: 10.1016/j.plaphy.2019.05.014
Zhao, M. J., Yin, L. J., Ma, J., Zheng, J. C., Wang, Y. X., Lan, J. H., et al. (2019a). The roles of GmERF135 in improving salt tolerance and decreasing ABA sensitivity in soybean. Front. Plant Sci. 10:940. doi: 10.3389/fpls.2019.00940
Zhao, Y., Zhu, L., Lin, C., Shen, Z., and Xu, C. (2019c). Transgenic soybean expressing a thermostable phytase as substitution for feed additive phytase. Sci. Rep. 9:14390. doi: 10.1038/s41598-019-51033-y
Zhao, X., Jing, Y., Luo, Z., Gao, S., Teng, W., Zhan, Y., et al. (2021). GmST1, which encodes a sulfotransferase, confers resistance to soybean mosaic virus strains G2 and G3. Plant Cell Environ. 44, 2777–2792. doi: 10.1111/pce.14066
Zheng, N., Li, T., Dittman, J., Su, J., Li, R., Gassmann, W., et al. (2020). CRISPR/Cas9-based gene editing using egg cell-specific promoters in Arabidopsis and soybean. Front. Plant Sci. 11:800. doi: 10.3389/fpls.2020.00800
Zheng, Q., and Perry, S. E. (2014). Alterations in the transcriptome of soybean in response to enhanced somatic embryogenesis promoted by orthologs of Agamous-like15 and Agamous-like18. Plant Physiol. 164, 1365–1377. doi: 10.1104/pp.113.234062
Zheng, T., Yu, X., Sun, Y., Zhang, Q., Zhang, X., Tang, M., et al. (2022). Expression of a cytochrome P450 gene from bermuda grass Cynodon dactylon in soybean confers tolerance to multiple herbicides. Plants 11:949. doi: 10.3390/plants11070949
Zhou, Y., Huang, J. L., Zhang, X. L., Zhu, L. M., Wang, X. F., Guo, N., et al. (2018). Overexpression of chalcone isomerase (CHI) increases resistance against Phytophthora sojae in soybean. J. Plant Biol. 61, 309–319. doi: 10.1007/s12374-018-0017-7
Zhou, Y., Liu, W., Li, X., Sun, D., Xu, K., Feng, C., et al. (2020). Integration of sRNA, degradome, transcriptome analysis and functional investigation reveals gma-miR398c negatively regulates drought tolerance via GmCSDs and GmCCS in transgenic Arabidopsis and soybean. BMC Plant Biol. 20:190. doi: 10.1186/s12870-020-02370-y
Keywords: soybean transformation, transformation efficiency, genotype, Agrobacterium, biolistic method, genome editing
Citation: Xu H, Guo Y, Qiu L and Ran Y (2022) Progress in Soybean Genetic Transformation Over the Last Decade. Front. Plant Sci. 13:900318. doi: 10.3389/fpls.2022.900318
Received: 20 March 2022; Accepted: 11 May 2022;
Published: 09 June 2022.
Edited by:
Madan K. Bhattacharyya, Iowa State University, United StatesReviewed by:
Shuo Li, Shandong University, ChinaMilind B. Ratnaparkhe, ICAR-Indian Institute of Soybean Research, India
Copyright © 2022 Xu, Guo, Qiu and Ran. This is an open-access article distributed under the terms of the Creative Commons Attribution License (CC BY). The use, distribution or reproduction in other forums is permitted, provided the original author(s) and the copyright owner(s) are credited and that the original publication in this journal is cited, in accordance with accepted academic practice. No use, distribution or reproduction is permitted which does not comply with these terms.
*Correspondence: Lijuan Qiu, cWl1bGlqdWFuQGNhYXMuY24=; Yidong Ran, eWlkb25ncmFuQGdlbm92by5vcmc=