- 1Centre of the Region Haná for Biotechnological and Agricultural Research, Institute of Experimental Botany of the Czech Academy of Sciences, Olomouc, Czechia
- 2Department of Cell Biology and Genetics, Faculty of Science, Palacký University Olomouc, Olomouc, Czechia
- 3Faculty of Natural Sciences, Comenius University in Bratislava, Bratislava, Slovakia
- 4Institute of Plant Genetics, Polish Academy of Sciences, Poznań, Poland
- 5Institute of Plant Physiology and Genetics, Bulgarian Academy of Sciences, Sofia, Bulgaria
- 6Department of Computer Science, Faculty of Science, Palacký University Olomouc, Olomouc, Czechia
Powdery mildew is one of the most devastating diseases of wheat which significantly decreases yield and quality. Identification of new sources of resistance and their implementation in breeding programs is the most effective way of disease control. Two major powdery mildew resistance loci conferring resistance to all races in seedling and adult plant stages were identified in the emmer wheat landrace GZ1. Their positions, effects, and transferability were verified using two linkage maps (1,510 codominant SNP markers) constructed from two mapping populations (276 lines in total) based on the resistant GZ1 line. The dominant resistance locus QPm.GZ1-7A was located in a 90 cM interval of chromosome 7AL and explains up to 20% of the trait variation. The recessive locus QPm.GZ1-2A, which provides total resistance, explains up to 40% of the trait variation and was located in the distal part of chromosome 2AL. The locus was saturated with 14 PCR-based markers and delimited to a 0.99 cM region which corresponds to 4.3 Mb of the cv. Zavitan reference genome and comprises 55 predicted genes with no apparent candidate for the QPm.GZ1-2A resistance gene. No recessive resistance gene or allele was located at the locus before, suggesting the presence of a new powdery mildew resistance gene in the GZ1. The mapping data and markers could be used for the implementation of the locus in breeding. Moreover, they are an ideal base for cloning and study of host–pathogen interaction pathways determined by the resistance genes.
Introduction
Hexaploid bread wheat (Triticum aestivum subsp. aestivum, 2n = 6× = 42, AABBDD) and tetraploid durum (pasta) wheat (Triticum turgidum subsp. durum, 2n = 4× = 28, AABB) are significant commercial grain crops worldwide. Their high and stable yield is the most important aspect of food security. Nevertheless, wheat yields could be threatened by various fungal diseases such as powdery mildew, rust, or Fusarium head blight (Chrpová et al., 2013; Bansal et al., 2020; Dreiseitl, 2021). Powdery mildew, caused by Blumeria graminis (DC.) E.O. Speer f. sp. tritici is one of the most devastating fungal diseases which decreases the yield and quality of susceptible varieties by up to 40% (Dreiseitl, 2011). Growing resistant cultivars is an effective way to control powdery mildew infections, as it represents the most economical and environmentally safe approach to eliminate the use of fungicides. Unfortunately, intensive breeding narrowed down the bread wheat gene pool (Feuillet et al., 2008). Therefore, the introduction of resistance genes derived from wheat landraces and related species into agriculturally used cultivars is an attractive way of wheat gene pool enrichment.
To date, more than 70 Pm genes and their alleles were identified in 60 loci across different species of wheat (Li et al., 2019; McIntosh et al., 2019) and most of them are major resistance genes (R-genes). In most cases, the R-genes code protein receptors with leucine-rich repeats (LRR), which recognize proteins secreted by the pathogen. They are usually dominant and short-living following the gene-for-gene concept (Flor, 1971). On the other hand, more durable resistance that is effective against a broader range of races, but often incomplete (called slow mildewing), could be provided by the involvement of a single locus with several resistance genes (Janáková et al., 2019) or by multiple minor resistance genes (Van Der Plank, 1963; Kou and Wang, 2010). The coding of this type of resistance is often dispersed into multiple discrete quantitative trait loci (QTLs). Nevertheless, loci with long-lasting resistance resembling the non-host resistance and effective against all tested isolates were observed. One of them is the Lr34 (Yr18/Pm38) locus carrying a gene with pleiotropic effect (ABC transporter) conferring resistance to various fungal pathogens, such as powdery mildew, leaf rust, and stripe rust (Krattinger et al., 2009). Another one, the Mlo locus identified in barley, is effective against all races throughout the season and encodes a plant membrane protein (Büschges et al., 1997). The Mlo resistance is determined as homozygous-recessive and has been successfully employed in agriculture for more than 40 years. So far, only one such gene has been identified and is used exclusively in spring cultivars to minimize the probability of its breakage.
However, the use of the R-genes in plant breeding forces strong selection pressure on pathogen populations. Therefore, this type of resistance is generally short-term and, in the case of cultivated crops, tends to be effective for about 3–5 years before it loses efficiency (Wolfe and McDermott, 1994; Dreiseitl, 2003). Moreover, the frequently observed quantitative nature of non-specific resistance makes it difficult to handle in breeding programs (Kou and Wang, 2010). The main reason is the problematic co-inheritance of all minor genes after crossbreeding, which can result in lower efficiency of the resistance. The scarcity of durable and race-independent resistance genes requires a continuous search for new sources of resistance to powdery mildew. Recently, a total resistance against a wide range of powdery mildew races at all growth stages was identified in tetraploid emmer wheat landrace GZ1 (Triticum turgidum subsp. dicoccum). Here, we present the mapping and characterization of the major loci involved in the GZ1 resistance against powdery mildew.
Materials and Methods
Plant Material
The powdery mildew-resistant spring emmer wheat line GZ1 (T. turgidum subsp. dicoccum) was collected during the expedition in Sobotište na Myjave in Slovakia and provided by Ing. Štefan Masár (Research Institute of Plant Production, Piešt’any, Slovakia). The dicoccum lines Eichenbarlebener (winter, EBL) and DIM25 (spring, PI 94633) were obtained from the Plant Breeding and Acclimatization Institute (IHAR)—National Research Institute (Radzików, Poland) and the United States Department of Agriculture (Beltsville, MD, United States), respectively. F1 hybrids were created from a cross of the GZ1 line with the EBL and DIM25 lines, where GZ1 was used as pollen donor (to eliminate possible effects of GZ1 cytoplasm). F2 mapping populations were derived from progenies of the F1 hybrids.
Powdery Mildew Resistance Assessment
Leaf segments of the lines GZ1, EBL, and DIM25 were inoculated individually with 30 isolates derived from the powdery mildew population captured by stationary collection at Bratislava, Slovakia, in May 2004. From 2005 to 2020, the GZ1, EBL, and DIM25 lines were sown annually in the autumn in field conditions at Bratislava-Prievoz, Slovakia.
Isolates A17, A24, and A3ab of Blumeria graminis (DC) E.O. Speer f. sp. tritici (Bgt) were used for phenotyping of the parental lines and mapping populations. The isolates were selected based on their aggressiveness (the shortest time from spore inoculation of plant material to the formation of conidiophores). All isolates originate from a collection of Bgt at the Research Institute of Plant Production in Piešt’any. In the preliminary testing on the parental and F1 lines (data not shown), all isolates performed without significant difference and indicated a single homozygous-recessive gene conferring broad-spectrum resistance. To diminish the influence of increased phenotype variability due to single isolate fitness and virulence genes composition, a mixture (1:1:1) of all three isolates was used for phenotyping.
Mapping reliability was enhanced by phenotyping F2:3 progeny according to which the original phenotype of each F2 line was reconstructed as a codominant marker. About 20 seedlings of each F2:3 line were grown in plastic pots filled with peat. After 10 days, the primary leaves were cut into 2.5 cm long segments and deposited on Petri dishes filled with 0.5% agar medium containing 862 mg/L of benzimidazole (Sigma-Aldrich, St. Louis, MO, United States). The inoculated leaf segments were incubated for 13 days in a growth chamber under continuous light (800 lux) at 18°C. Subsequently, the response to powdery mildew was visually evaluated as the presence of colonies on all F2:3 lines (susceptibility, score 0), the absence of colonies on all segments (resistance, score 1), or colonies only on several lines (heterozygous, score 0.5). Deviation from Mendelian inheritance was evaluated using the chi-square goodness of fit test (Pearson, 1900).1
Genotyping and Linkage Map Construction
DNA was extracted from dried (37°C for at least 24 h) 2 cm long young leaf segments. The dry leaf segments were homogenized in the presence of two glass balls (5 mm) at 27 Hz for 3 min using a MM301 mill (Retsch, Haan, Germany). DNA was extracted and purified using a NucleoSpin® Plant II kit (Macherey-Nagel, Düren, Germany) and quantified with a NanoDrop 2000 (Thermo Fisher Scientific, Waltham, MA, United States). DNA was genotyped using the DArTseq approach at Diversity Arrays Technology Pty., Ltd. (Canberra, Australia)2 and the obtained sequencing data were analyzed together for both mapping populations. Markers were subjected to quality testing followed by the removal of markers with more than 10 missing data points or significant segregation distortion (over 30%). Only codominant polymorphic DArTseq markers were used for map construction.
Linkage maps were constructed using MultiPoint UltraDense v4.1 (MultiQTL Ltd., Haifa, Israel3; Ronin et al., 2017). DArTseq single nucleotide polymorphism (SNP) markers were preliminarily filtered and processed as an F2 population with default settings. Markers with more than four missing data points and χ2-values greater than 10 (p = 0.05) were removed. Markers were clustered into multiple linkage groups (LGs) of ordered co-segregating markers using a guided evolutionary strategy (GES) algorithm (Ronin et al., 2010) with 10 jackknife re-sampling runs. After the first clustering, the 14 longest LGs were selected for subsequent analysis due to the tetraploid character of T. turgidum. Markers that disrupted the monotony and caused unstable neighborhoods were checked for segregation ratios, linkage distances, missing data, and associations with other markers, and were eventually removed to stabilize the LGs until the global variation value decreased below 1.1. Subsequently, the LGs were saturated with additional markers from the Heap group using the “Extending linkage group” function with the coefficient of enlargement increased stepwise from 1.0 to 1.2. The order of markers was rechecked for monotony distortion and map size enlargement, and those causing disruption were eliminated. Only the “skeleton” maps were used for subsequent analyses. The resulting LGs were exported to Microsoft Excel with recombination frequencies converted to centiMorgans (cM) using the Kosambi mapping function (Kosambi, 1943).
Individual linkage groups were assigned to a particular chromosome based on the wheat DArTseq consensus map (Diversity Arrays Technology Pty., Ltd., Canberra, Australia, see text footnote 2). Exported LGs were visualized in MapChart v2.32 (Voorrips, 2002).
Quantitative Trait Loci Analysis
Mapping of quantitative trait loci (QTLs) was performed independently for both mapping populations using MultiQTL v2.6 (MultiQTL Ltd., Haifa, Israel, see text footnote 3, Korol et al., 2001). The skeleton maps, which contain only the most informative skeleton markers, were used. QTL mapping was carried out by multiple interval mapping (MIM, Kao et al., 1999) using single-QTL per chromosome sub-models. QTL LOD threshold values, standard deviations, and 95% confidence intervals (CI) of QTL positions were estimated with bootstrap analysis (10,000 iterations). QTLs were declared significant when their LOD scores exceeded the respective p < 0.01 critical LOD thresholds. QTL effects were determined as the percentage of explained variance (PEV) of the trait relative to its phenotypic variation.
Marker Development, Candidate Gene Identification, and 2A Map Saturation
The 2A chromosomes from all three parental lines were flow-sorted from liquid suspensions of mitotic chromosomes prepared from the root tips of seedlings as described by Vrána et al. (2000). The chromosome samples were fluorescently labeled by FISHIS using (GAA)7 oligonucleotides labeled by Alexa488 on both termini (Integrated DNA Technologies, Coralville, IA, United States) and counterstained by DAPI (4′,6-diamidino-2-phenylindole) as described by Giorgi et al. (2013). Bivariate flow karyotyping and chromosome sorting were performed on a FACSAria II SORP flow cytometer and sorter (Becton Dickinson Immunocytometry Systems, San Jose, CA, United States). Contamination of sorted fractions by other chromosomes was determined using fluorescence in situ hybridization (FISH) using probes for telomeric repeats, Afa repeat, and (GAA)n, according to Kubaláková et al. (2003). Before DNA sequencing, 100,000 flow-sorted chromosomes were treated with 60 ng/ml proteinase K (Roche, Basel, Switzerland) for 40 h at 50°C, and DNA was purified using a Microcon YM-100 column (MilliporeSigma, Burlington, MA, United States) as described by Šimková et al. (2008). About 20 ng of purified DNA was fragmented in 20 μl using a Bioruptor Plus (Diagenode, Liège, Belgium) five times for 30 s at the HIGH setting. The sequencing libraries were prepared from sheared DNA using a NEBNext Ultra II DNA Library Prep Kit for Illumina (New England Biolabs, Ipswich, MA, United States) with the following modification: (i) size selection was directed for a larger final library size (∼1,000 bp), (ii) PCR enrichment was done in nine cycles, (iii) library was size-selected using a BluePippin (Sage Science, Beverly, MA, United States) in pre-cast 1.5% agarose gel cassettes. The library was sequenced on a NovaSeq 6000 (Illumina, San Diego, CA, United States) and 2 × 250 bp paired-end reads were produced. The raw data were trimmed for low-quality bases using Trimmomatic (Bolger et al., 2014) and assembled to scaffolds with Meraculous v2.0.5 (Chapman et al., 2011) using 111-bp k-mers. Scaffolds shorter than 1 kb were discarded.
Candidate genes for GZ1 powdery mildew resistance loci were identified by anchoring the mapped QTLs to the cv. Zavitan reference genome sequence (Avni et al., 2017). Sequences of markers from identified QTLs were BLASTN (Altschul et al., 1990) aligned using default parameters. Only the best blast hits were considered. Annotated genes from regions delimited by the alignments were extracted from a list of high-confidence annotated genes (Avni et al., 2017).
PCR markers for map saturation were designed according to Janáková et al. (2019) with minor modifications. Sequences of selected genes were BLASTN aligned with the assemblies of all three chromosomes 2A and identified scaffolds were compared. Only low-copy sequences with nucleotide identity greater than 95% were considered for marker development, and those containing short indels were preferred. If no indel was identified, markers were designed based on SNPs. PCR primers flanking the polymorphism were designed using Primer3web v4.1.0 (Untergasser et al., 2012). The Xgwm294 marker was used for genotyping according to Mohler et al. (2013). All primer pairs were tested on all parental lines.
The PCR reaction mix (15 μl) contained 0.01% (w/v) o-cresolsulphonephtalein, 1.5% (w/v) sucrose, 0.2 mM of each dNTP, 0.6 U of Taq DNA polymerase, 1 μM of each primer, 10 mM Tris-HCl, 50 mM KCl, 1.5 mM MgCl2, 0.1% (v/v) Triton X-100, and 10–20 ng of genomic DNA. The reaction conditions consisted of an initial denaturation (95°C/5 min), followed by 40 cycles of 95°C/30 s, an optimized annealing temperature (Table 1) for 30 s, and 72°C for 30 s. The PCR reactions were completed with an elongation step of 72°C/10 min. The amplicons were electrophoretically separated on 4% non-denaturing polyacrylamide gels using a Mega-Gel Apparatus C-DASG-400-50 (C.B.S. SCIENTIFIC, CA, United States) and were stained with ethidium bromide and visualized with an InGenius LHR2 Gel Imaging System (Synoptics, Cambridge, United Kingdom).
Results
GZ1, DIM25, and EBL Reaction to Powdery Mildew
After screening with the 30 isolates, only GZ1 showed complete resistance to all isolates used in the screening test. The EBL and DIM25 showed susceptibility to all isolates. Annual sowing of the three lines at the locality Bratislava, Slovakia, resulted in frequent infections of the EBL and DIM25 lines. In all 15 seasons, on the GZ1 line, no symptoms of the powdery mildew were observed.
Mapping Populations and Powdery Mildew Resistance Analysis
Two F2 mapping populations were derived from crosses of the resistant GZ1 line with two susceptible cultivars, EBL and DIM25, comprising 125 and 151 F2 lines, respectively. These two populations were used for QTL mapping and verification, and also, testing of the trait’s transferability to different genetic backgrounds. To increase the reliability of phenotyping for mapping purposes and enhance the survival of genotyped F2 lines, about 20 seedlings from subsequent F2:3 families were phenotyped and F2 phenotypes were deduced as codominant markers.
The GZ1 × EBL F2 population comprised 16 susceptible, 34 resistant, and 63 heterozygous individuals. In the remaining 12 lines, significant contamination with Aspergillus fungi was observed and were considered unreliable. According to χ2 of 7.09 (p = 0.0289), the segregation ratio does not fit the expected Mendelian segregation ratio for a single gene inheritance (1:2:1). The verification GZ1 × DIM25 F2 population comprised 15 susceptible, 59 resistant, and 73 heterozygous lines. Four lines altered by Aspergillus contamination were ignored; χ2 was 26.35 (p = 0.0000019) confirming the distortion from the 1:2:1 ratio; therefore, QTL analysis was performed with these phenotype data.
In contrast to preliminary phenotyping, the F2:3 phenotype analysis revealed two types of susceptibility reactions. Besides resistance reaction with no colonies, small and large colonies were also observed (Figure 1).
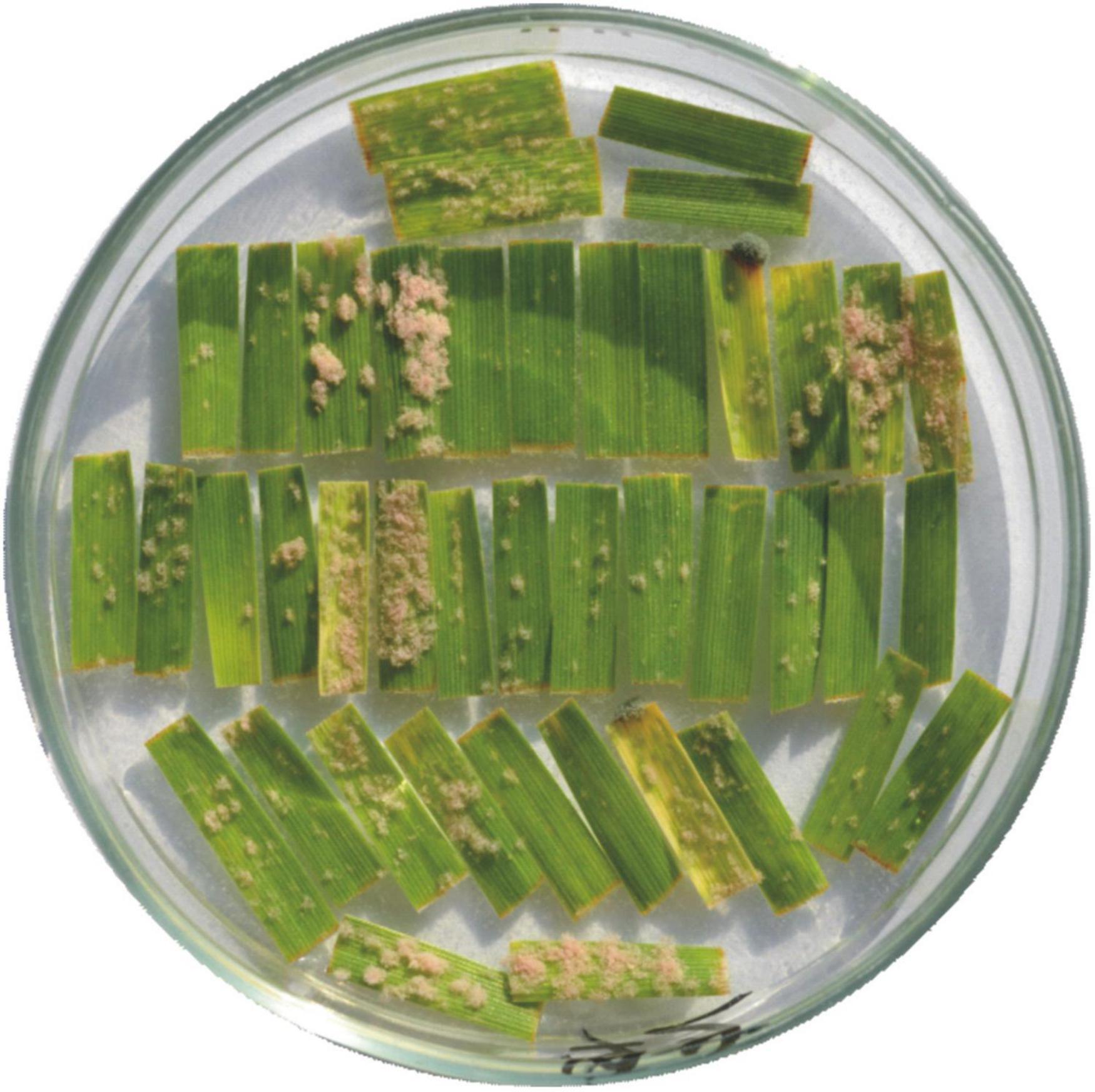
Figure 1. Observed reaction to powdery mildew infection. The first line (horizontal) represents two segments of susceptible line EBL (left) and two segments of resistant line GZ1 (right). The remaining leaf segments (vertical) represent tested F2:3 families, always two primary leaf segments of a different plant of the same family (counted from the left upper corner). Plant reaction to powdery mildew: resistant (no colonies, e.g., segments 5–8, and 25–26) or susceptible (with colonies). Among the susceptible plants, there is a difference in the reaction. Some segments were completely infected and covered with large colonies (e.g., segments 3 and 4), while on others, there were only small colonies (e.g., segments 1 and 2).
Genetic Map Construction
A total of 23,012 SNP markers were obtained from the DArTseq analysis for both mapping populations. Data filtering was done individually for each mapping population. After quality and normal segregation (χ2) filtering, a set of 7,985 and 6,218 SNP markers (2,291 shared markers) was used for the construction of GZ1 × EBL and GZ1 × DIM25 linkage maps.
The skeleton map of GZ1 × EBL comprises 14 linkage groups with 862 skeleton markers (Supplementary Table 1) and a genetic length of 3,102.54 cM. An average marker density is 3.69 cM. The length of the linkage groups ranged from 186.99 cM (chromosome 4B) to 262.11 cM (chromosome 7A) with an average of 221.61 cM. The individual linkage groups comprised 62 skeleton markers on average, with the highest number of markers (88) on chromosome 7A and the lowest (38) on chromosome 4B. The lowest and highest marker densities were observed for chromosomes 4B (4.92 cM) and 2B (2.64 cM), respectively (Table 2).
GZ1 × DIM25 skeleton map was constructed using 648 markers (Supplementary Table 2) and spanned 2,377.86 cM with an average marker density of 3.72 cM. The length of the linkage groups ranged from 81.12 cM (chromosome 4B) to 222.11 cM (chromosome 3B) with an average of 169.85 cM. The linkage groups comprised 46 markers on average. Similarly, to the previous map, the highest number of markers (64) was assigned to chromosome 7A, while chromosome 4B contained the lowest number of markers (21). Chromosomes 5A and 7B had the lowest (4.74 cM) and the highest (3.04 cM) marker density, respectively (Table 2).
Quantitative Trait Loci Analysis
Quantitative trait loci mapping revealed four genomic regions associated with the resistance on chromosomes 2A, 3A, 3B, and 7A. QTLs located on chromosomes 3A and 3B had low LOD scores and were not detected in both mapping populations. Therefore, they were not verified and were abandoned. However, QTLs located on chromosomes 2A (denominated QPm.GZ1-2A) and 7A (denominated QPm.GZ1-7A) were significant in both mapping populations. QPm.GZ1-2A had a LOD score of 15.65 (GZ1 × EBL) and 20.8 (GZ1 × DIM25) and explained 31.7 and 39.6% of phenotypic variance (PEV) for this trait, respectively. QPm.GZ1-7A reached the LOD score of 9.7 (GZ1 × EBL, PEV 20.9%) and 7.3 (GZ1 × DIM25, PEV 11%; Table 3).
The QTL analysis of the GZ1 × EBL population placed the QPm.GZ1-2A locus in the 151.6–167.7 cM interval of chromosome 2A (16.1 cM) with a peak at 159.65 cM (Figure 2 and Table 3). The region comprises 7 markers and is flanked by markers 996555-38 and 2276132-44 (Supplementary Table 1 and Figure 2). The syntenic region in the reference genome of cv. Zavitan (Avni et al., 2017) delimited by the flanking markers (Supplementary Table 3 and Figure 2) covers 22.7 Mb (696,152,174–718,833,310 bp) on chromosome 2AL. In the GZ1 × DIM25 verification mapping population, the QPm.GZ1-2A locus was placed in a 153.9–156.9 cM region with a peak at 155.44 cM (Figure 2 and Table 3). It comprises 3 markers and is flanked by markers 1022408-13 and 7353838-21 (Supplementary Table 2 and Figure 2). This region corresponds to 10.9 Mb (700,992,011–711,927,608 bp) of the cv. Zavitan chromosome 2A and completely overlaps with the GZ1 × EBL locus (Supplementary Table 4 and Figure 2).
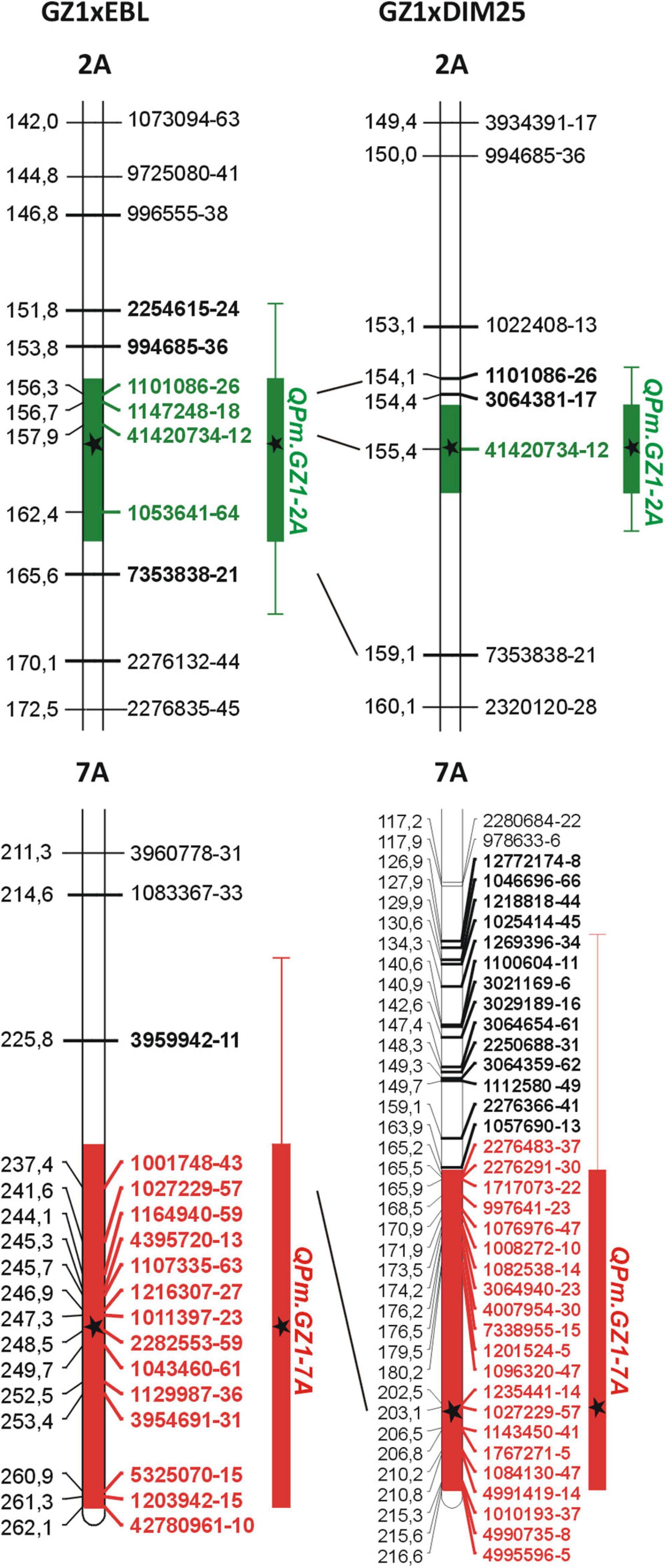
Figure 2. Highly significant QTLs for powdery mildew resistance mapped in the GZ1 × EBL and GZ1 × DIM25 mapping populations. Only relevant parts of chromosomes with mapped QTLs are shown. The genetic distances (left side) are in cM (Kosambi). In the GZ1 × EBL, the QPm.GZ1-2A was in the region from 155.55 to 163.75 cM (8.2 cM; green bar) of chromosome 2AL with the QTL peak at 159.65 cM (black star). This region comprises 4 markers (bold green). The confidence interval extended the area from 151.6 to 167.7 cM (16.1 cM; thin green line). This 16.1 cM long region comprises 7 markers (bold). In the GZ1 × DIM25, QPm.GZ1-2A was in a 1.54 cM long region (154.67–156.21 cM; green bar) with one marker (bold green). The QTL peak is at 155.44 cM (black star) of chromosome 2AL. The confidence interval extended the area of this QTL to 3 cM (153.9–156.9 cM; thin green line and 3 markers in bold). In the GZ1 × EBL, QPm.GZ1-7A was in a 28.96 cM long region (red bar) of the chromosome 7AL and comprises 14 markers (bold red). Confidence interval extends the area to 42.3 cM (thin red line) with 15 markers (bold). The QTL peak is at 248.12 cM (black star). In the GZ1 × DIM25, the QPm.GZ1-7A was in a 52.34 cM long region (red bar, 21 markers) of the chromosome 7AL, with the confidence interval of 90.8 cM (red thin line) comprising 35 markers (bold) with the QTL peak at 204.25 cM (black star). The shared markers are indicated by lines connecting the maps.
The QPm.GZ1-7A in the GZ1 × EBL map was located on chromosome 7AL within a 42.3 cM interval (219.7–262.0 cM) with a peak at 248.12 cM (Figure 2 and Table 3). The region comprises 15 markers and is flanked by markers 1083367-33 and 42780961-10 (Supplementary Table 1 and Figure 2). The syntenic locus in the cv. Zavitan genome delimited by the flanking markers covers 47 Mb (678,080,238–725,069,898 bp; Supplementary Table 5). In the GZ1 × DIM25 map, the QPm.GZ1-7A was in a 90.8 cM interval (125.8–216.6 cM) with a peak at 204.25 cM and is flanked by markers 12772174-8 and 4995596-5 (Supplementary Table 2 and Figure 2). The region comprises 35 markers (Figure 2 and Supplementary Table 6) and corresponds to 143.8 Mb (564,777,524–708,600,178 bp) of the cv. Zavitan chromosome 7AL and overlays the GZ1 × EBL QPm.GZ1-7A locus (Supplementary Tables 5, 6).
QPm.GZ1-2A and QPm.GZ1-7A Interactions
Interactions between these two QTLs were examined by comparing phenotypic data of lines with different genomic compositions at the resistance loci. The homozygous-recessive constitution of the GZ1 genotype at the QPm.GZ1-2A locus was in all cases associated with total resistance to powdery mildew with no respect to genotype constitution at QPm.GZ1-7A (Figure 3A). Progeny testing of individual F2 lines confirmed that QPm.GZ1-2A is recessively inherited because the heterozygous constitution at the QPm.GZ1-2A locus with homozygous EBL or DIM25 genotype at QPm.GZ1-7A resulted in plants with large colonies or without colonies (Figure 3B) to the advantage of susceptible ones. The homozygous constitution (EBL or DIM25) at the QPm.GZ1-2A locus with the heterozygous constitution at the QPm.GZ1-7A locus resulted in segregation for large colonies, small colonies, and no colonies to the advantage of resistant ones, suggesting that the QPm.GZ1-7A is a dominant gene (Figure 3C). The homozygous constitution of EBL or DIM25 at the QPm.GZ1-2A locus with homozygous GZ1 constitution at QPm.GZ1-7A was associated with the presence of a few small colonies or no colonies (Figure 3D), suggesting that the phenotype is mediated by dosage-dependent gene effects.
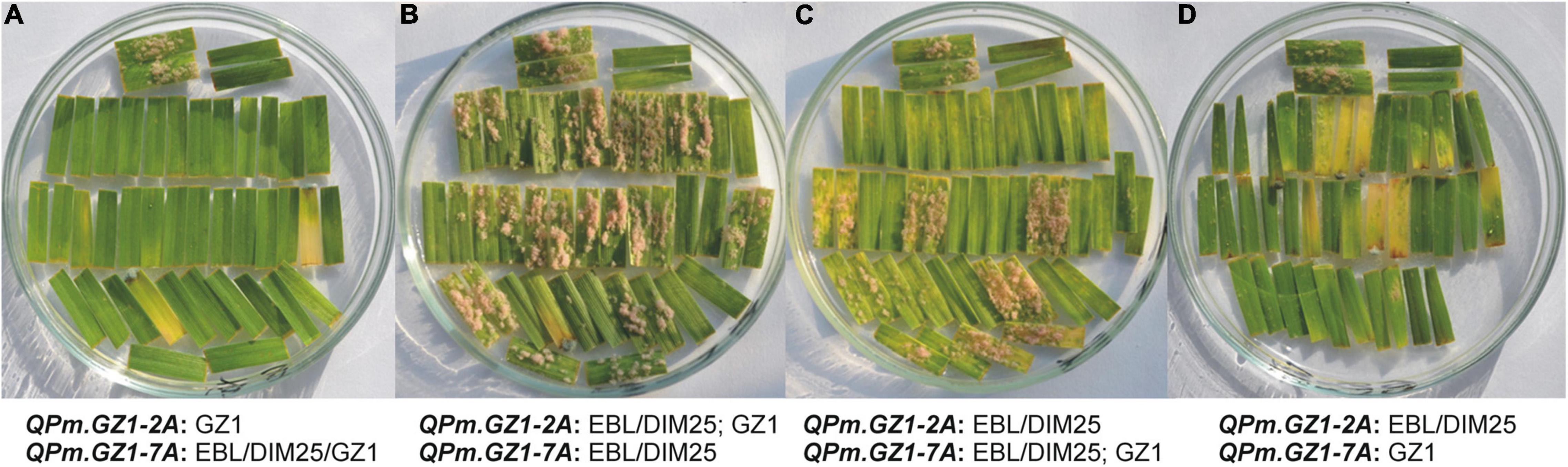
Figure 3. Phenotypic reaction of F2:3 families to a mixture of powdery mildew isolates A17, A23, and A3ab focusing on the interaction between QPm.GZ1-2A and QPm.GZ1-7A. Examples of phenotypic reactions of individual F2 lines with allelic states of the resistance loci as indicated under the pictures (GZ1 = homozygous and resistant, EBL/DIM25/GZ1 = any allele, EBL/DIM25 = homozygous and susceptible, EBL/DIM25; GZ1 = heterozygous). In all pictures, the top two pairs of horizontally placed segments are susceptible control EBL (left) and resistant control GZ1 (right). For all F2 lines, about 20 F2:3 progenies were tested and each of them is represented by two leaf segments. (A) F2 lines homozygous for the GZ1 allele at the QPm.GZ1-2A irrespective of the QPm.GZ1-7A showed complete resistance. (B) Lines that were heterozygous at QPm.GZ1-2A and carry the homozygous susceptible allele at QPm.GZ1-7A segregated in a ratio of 1 (resistant): 3 (susceptible). (C) Lines heterozygous at QPm.GZ1-7A and homozygous susceptible at QPm.GZ1-2A segregated in a ratio of approximately 3 (resistant): 1 (susceptible). Of 21 F2:3 progeny lines, leaf segments of seven plants (7, 9, 12, 15, 18, 20, 21) were covered with large colonies, while segments of eight plants (1, 5, 6, 10, 11, 13, 17, 19) had very small colonies The remaining six plants (2, 3, 4, 8, 14, 16) had almost no visible colonies. (D) Lines that were homozygous for the GZ1 allele at the QPm.GZ1-7A but homozygous for the susceptible parent at the QPm.GZ1-2A inhibited infection of powdery mildew almost completely.
Map Saturation at the QPm.GZ1-2A Resistance Locus
The major resistance locus QPm.GZ1-2A was selected for saturation (Supplementary Tables 2, 3). As stated above, the QPm.GZ1-2A locus was delimited to the corresponding 22.7 Mb region of the cv. Zavitan reference genome sequence, which comprises 316 predicted genes (Supplementary Table 3). The locus was saturated with 14 new markers and the marker Xgwm294 associated with the Pm50 gene (Supplementary Table 7). Marker development was facilitated by sequencing the 2A chromosomes of the parental lines. The assemblies of the GZ1, DIM25, and EBL chromosomes 2A comprise 365, 366, and 364 Mb with N50 of 26.1, 24.3, and 23.3 kb, respectively. The saturation allowed to delimit the QPm.GZ1-2A resistance locus to a region between markers owm2016 and 1101086-26. This region is 0.99 cM long and corresponds to 4.3 Mb of the cv. Zavitan genome (Supplementary Table 7) with 55 annotated (Supplementary Table 3) high-confidence genes (excluding transposon related genes). Of these, three genes have a known relation to resistance (Supplementary Table 3).
Discussion
Tetraploid wheat Triticum turgidum subsp. dicoccum GZ1 was studied for its broad-range race non-specific resistance to Blumeria graminis (DC) E.O. Speer f. sp. tritici. Analysis of F1 hybrids indicated that the powdery mildew resistance might be controlled by a single recessive gene due to the susceptible reaction of all individuals (data not shown). Race non-specific recessive resistance is very rare because most of the resistance genes are usually coded by dominant R-genes following the gene-for-gene concept (Flor, 1971). Major R-genes provide resistance to only one or a few races (race-specific resistance). Race non-specific resistance, effective against a wide range of races, is usually determined by several genes (Van Der Plank, 1963). However, in some cases, race non-specific resistance could also be controlled by major genes, as it is in the case of barley Mildew resistance locus o (Mlo). The loss of function of both alleles of a single Mlo gene in barley was found to confer recessively inherited broad-spectrum resistance at all growth stages (Jørgensen, 1992). A similar reaction to powdery mildew was observed due to a recessive mutation of a single gene in the tetraploid GZ1-based hybrids (Figure 1). However, in hexaploid bread wheat, all Mlo-like genes in all three homoeologous genomes must be silenced to achieve resistance, as was shown by Wang et al. (2014) using knockout of the gene family by TALENs. This is a strong difference from the GZ1 gene. Additionally, the Mlo resistance is characterized by cell wall appositions, papillae formation at the encounter sites, early chlorophyll decay, and spontaneous mesophyll cell death leading to chlorosis and leaf necrosis negatively affecting yield (Bayles et al., 1990; Peterhansel et al., 1997; Piffanelli et al., 2002; Makepeace et al., 2007). In the case of the GZ1 resistance, leaf necrosis was not observed supporting the hypothesis of different resistance mechanisms, which makes the gene highly attractive for mapping and cloning.
The susceptible winter and spring cvs. EBL and DIM25, respectively, were used for the construction of mapping populations and confirmed transferability of the GZ1 resistance to different genetic backgrounds. In contrast to original assumptions of the presence of a single resistance gene, a reproducible shift from 1:2:1 segregation ratio was observed. Moreover, employing the F2:3 lines allowed the identification of three reaction types (Figure 1). Both observations surprisingly indicated the presence of more than one resistance gene. The accumulation of several resistance genes in a single line is quite common and the accumulation of several major resistance genes is highly desirable or even created by breeders using the gene pyramiding approach (Koller et al., 2018).
The DArTseq genotyping of the GZ1-derived F2 mapping populations provided sufficient marker density for subsequent QTL analysis (Table 2). Both maps have size and marker density (Table 2) comparable with published tetraploid wheat maps (e.g., Peleg et al., 2008). Due to an unexpected deviation of phenotypic data from the Mendelian segregation ratio, what suggests polygenic inheritance of the trait, the QTL analysis was applied. The QTL analysis identified two highly significant loci contributing to the resistance variation. Their LOD scores significantly exceeded their respective LOD threshold values (Table 3). Powdery mildew resistance of GZ1 was found to be controlled by the QPm.GZ1-2A and QPm.GZ1-7A loci on the chromosome 2AL and 7AL, respectively. Reliability of mapping of both resistance loci was confirmed by their detection in both populations in overlapping positions (Figure 2 and Supplementary Tables 3, 4). This high-quality mapping could be attributed to categorical phenotype assessment of the F2 lines using the F2:3 progenies. Even though the conversion of phenotype to a codominant marker provides reliable mapping which eliminates the residual variability (environmental influences), it is suitable only for mapping of a limited number of loci with a large contribution to phenotype variance. This approach cannot match the resolution achieved by scaling (e.g., Dreiseitl, 2022); therefore, polygenic resistance determined by a higher number of loci with a small contribution to phenotype may not be detected and verified due to the influence of the residual variability.
In this study, a homozygous-recessive resistance conferred by a single gene was expected, so the less sensitive but more robust phenotyping approach was employed. The suitability of this phenotyping approach was confirmed by reliable and verified mapping of two major resistance loci as stated above. Each mapped locus showed distinct phenotypic manifestation. Homozygous-recessive QPm.GZ1-2A confers total resistance with no respect to QPm.GZ1-7A (Figure 3A). The progeny of plants dominant for the 2A and heterozygous for the 7A locus shows segments with large colonies, small colonies, or no visible colonies (Figure 3C). In the case of the homozygous-dominant constitution of loci on chromosomes 2A and 7A, symptoms manifest as small colonies or no visible colonies, suggesting an additive effect of the alleles at the 7A locus (Figure 3D). However, further work is required to verify this effect.
To date, eight powdery mildew resistance genes have been identified on chromosome 2AL. These include formally named multi-allelic Pm4 (Briggle, 1966), and tentatively designated genes for powdery mildew such as PmLK906 (Niu et al., 2008), PmHNK54 (Xu et al., 2011), PmPS5A (Zhu et al., 2005), PmYm66 (Hu, 2008), Pm50 (Mohler et al., 2013), PmHo (Komáromi et al., 2016), and pmX (Fu et al., 2013). Pm4 consists of four resistance alleles Pm4a, Pm4b, Pm4c, and Pm4d (The et al., 1979; Hao et al., 2008; Schmolke et al., 2011). The homology of the QPm.GZ1-2A locus with loci of all these genes was determined by examining their genomic positions according to their co-segregating markers. The direct comparison revealed that only PmHNK54, which was located together with QPm.GZ1-2A between the Xbarc5 and Xgwm312 markers maps to the same region (Figure 4). However, this gene was found to be dominant (Xu et al., 2011), whereas GZ1 resistance was so far characterized as recessive. Therefore, QPm.GZ1-2A is distinguishable from PmHNK54.
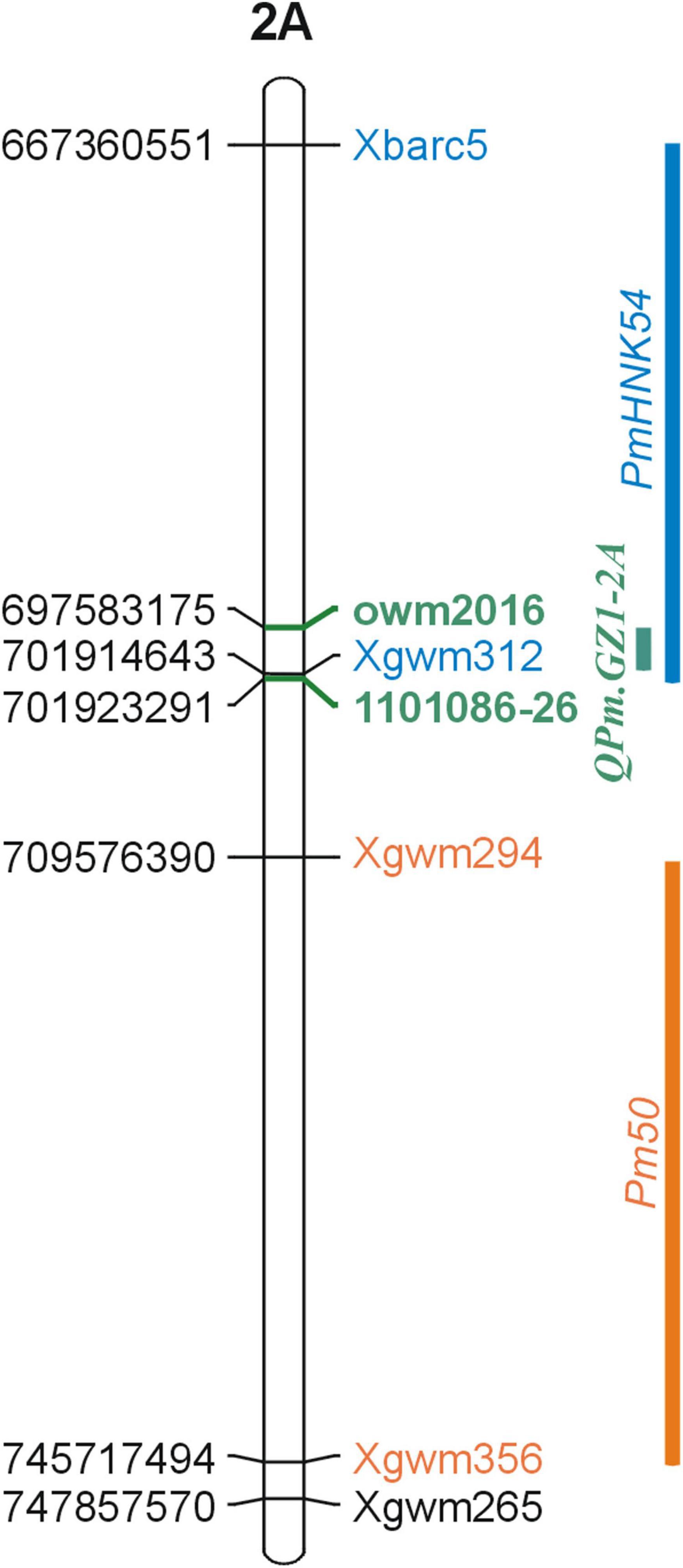
Figure 4. Genomic comparison of known powdery mildew resistance loci adjacent to the QPm.GZ1-2A locus. The reference genome sequence of cv. Zavitan was used to analyze the QPm.GZ1-2A locus position on the genome level. The QPm.GZ1-2A locus (green bar) is flanked by the owm2016 and 1101086-26 markers (green) overlapping the PmHNK54 locus (Xu et al., 2011, blue) and neighboring the Pm50 locus. Mohler et al. (2013) located the Pm50 3.8 cM distally from the Xgwm294 marker and it does not overlap with the QPm.GZ1-2A locus. PmHNK54 was mapped 6 cM proximally to the Xgwm312 marker. The QPm.GZ1-2A and PmHNK54 loci share about 4.3 Mb of the 31 Mb PmHNK54 region between the Xgwm312 and Xbarc5 markers; however, PmHNK54 was found to be dominant and is different from the recessive QPm.GZ1-2A locus.
The QPm.GZ1-7A was mapped to a large region of chromosome 7AL (144 Mb). So far, about 20 Pm genes have been localized on chromosome 7AL: Pm1 consisting of five different alleles: Pm1a, Pm1b, Pm1c (Pm18), Pm1d (Hsam et al., 1998), and Pm1e (Pm22; Singrün et al., 2003), Pm9 (Schneider et al., 1991), mlRd30 (Singrün et al., 2004), Pm37 (Srnić et al., 2005; Perugini et al., 2007), NC96BGTA4 (Srnić et al., 2005), MlIW172 (Ouyang et al., 2014), PmU (Qiu et al., 2005), NCA6 (Miranda et al., 2007), Mlm2033 and Mlm80 (Yao et al., 2006; Liang et al., 2016), MlIw72 (Ji et al., 2007), MlAG12 (Maxwell et al., 2009), MlWE18 (Han et al., 2009), PmG16 (Ben-David et al., 2010), PmTb7A.1 and PmTb7A.2 (Chhuneja et al., 2011), HSM1 (Li et al., 2013), MlUM15 (Worthington et al., 2014), Pm59 (Tan et al., 2018), and QPm.gb-7A (Desiderio et al., 2021). According to Desiderio et al. (2021), most of these genes are likely to be closely linked and create clusters. Most known R-genes encode immune receptors from the nucleotide-binding site leucine-rich repeats (NBS-LRR) protein family. Resistance genes are abundant in plant genomes and clustering was observed (International Wheat Genome Sequencing Consortium (IWGSC), 2018). QPm.GZ1-7A also resides in such a resistance gene-enriched region. However, this could be influenced by the large confidence interval of QPm.GZ1-7A, and therefore, additional work is necessary to differentiate between the already designated powdery mildew resistance genes and the QPm.GZ1-7A locus. Almost all genes, except Pm9 (Schneider et al., 1991) and mlRD30 (Singrün et al., 2004), are major dominant (R-genes). The physical interval retrieved for QPm.GZ1-7A in cv. Zavitan (46.9 Mb, Supplementary Table 5) comprises 34 disease resistance-related genes including nine from the LRR family. As mentioned above, QPm.GZ1-7A is dominant, suggesting that it could be an NBS-LRR-like gene.
The recessive character of QPm.GZ1-2A and its strong resistance effect make it a more attractive source of resistance compared to the QPm.GZ1-7A, and therefore, only the QPm.GZ1-2A was selected for further map saturation. The flanking markers of the GZ1 × EBL region (Supplementary Tables 1, 3) were used as the starting points. The 22.7 Mb long QPm.GZ1-2A region of the GZ1 × EBL map was saturated with 14 new markers (Table 1). The final QPm.GZ1-2A region (about 4.3 Mb) in the cv. Zavitan reference genome sequence (Avni et al., 2017) is flanked by the owm2016 and 1101086-26 markers (Supplementary Table 7). The narrowed down QPm.GZ1-2A region does not overlap with the QTL peaks (associated with the 41420734-12 marker, Figure 2) predicted by the QTL analysis. The observed proximal shift (Supplementary Table 7) could be attributed to noise in the phenotype data caused by the presence of two resistance genes.
The QPm.GZ1-2A region comprises 55 annotated genes (Supplementary Table 3) and none of them have any relation to the Mlo gene family. Additionally, the Mlo gene was mapped on chromosome 4H (Simons et al., 1997) and is not orthologous to the QPm.GZ1-2A mapped on chromosome 2A supporting the previous assumptions that QPm.GZ1-2A is different from the Mlo gene. However, three of these genes have relation to the resistance genes involved in pathogen attack signaling. Since genes from the signaling pathways are mostly dominant R-genes (e.g., Peart et al., 2005; Sánchez-Martín et al., 2016), there is only a small probability that the recessive QPm.GZ1-2A is one of them. However, one of them could be the PmHNK54 gene (Xu et al., 2011). Nevertheless, confirmation of the assumptions would require further work.
The broad-range resistance in all stages of plant development mediated by the single major QPm.GZ1-2A locus makes it extremely attractive to breeders and the high-density genetic map of the locus offers molecular markers for its effective implementation in breeding programs. Moreover, the results provide an ideal base for cloning and study of the novel recessive gene determining the resistance.
Data Availability Statement
The datasets presented in this study can be found in online repositories. The names of the repository/repositories and accession number(s) can be found in the article/Supplementary Material.
Author Contributions
MV, ZK, and MŠ designed the study. ZK, AL, and MM were responsible for marker development, genotyping, map construction, and data analysis. MŠ and EJ were responsible for the construction of mapping populations. MŠ, GB, and ZK performed the phenotypic evaluation. AL performed the statistical analysis. PC sorted the chromosomes and extracted chromosome-specific DNA. KH was responsible for survey sequencing and sequence assembly. ZK, MV, and JJ conducted the bioinformatics analyses. ZK conducted the other experiments. ZK and MV drafted the manuscript. All authors contributed to its editing and proofreading.
Funding
This work was supported by the Czech Science Foundation (No. 18-11688S), the Czech Republic Ministry of Agriculture (Nos. QK22010293 and QK1710302), the ERDF project “Plants as a Tool for Sustainable Global Development” (No. CZ.02.1.01/0.0/0.0/16_019/0000827), Slovak Research and Development Agency (No. APVV-20-0246), and National Scholarship Programme of the Slovak Republic of SAIA Agency (Georgi Bonchev). The authors from the Institute of Experimental Botany have received funding from the European Union’s Horizon 2020 Research and Innovation Program (No. 101000847, CROPDIVA), which made the continuation of the collaborative work possible.
Conflict of Interest
The authors declare that the research was conducted in the absence of any commercial or financial relationships that could be construed as a potential conflict of interest.
Publisher’s Note
All claims expressed in this article are solely those of the authors and do not necessarily represent those of their affiliated organizations, or those of the publisher, the editors and the reviewers. Any product that may be evaluated in this article, or claim that may be made by its manufacturer, is not guaranteed or endorsed by the publisher.
Acknowledgments
We also express our gratitude to Ing. Marie Seifertová, Helena Tvardíková, Radka Tušková, Eva Jahnová, and Anna Michalíková for excellent technical assistance.
Supplementary Material
The Supplementary Material for this article can be found online at: https://www.frontiersin.org/articles/10.3389/fpls.2022.897697/full#supplementary-material
Footnotes
- ^ graphpad.com/quickcalcs/chisquared1.cfm
- ^ diversityarrays.com
- ^ https://www.graphpad.com/quickcalcs/chisquared1.cfm
References
Altschul, S. F., Gish, W., Miller, W., Myers, E. W., and Lipman, D. J. (1990). Basic local alignment search tool. J. Mole. Biol. 215, 403–410. doi: 10.1016/s0022-2836(05)80360-2
Avni, R., Nave, M., Barad, O., Baruch, K., Twardziok, S. O., Gundlach, H., et al. (2017). Wild emmer genome architecture and diversity elucidate wheat evolution and domestication. Science 357, 93–97. doi: 10.1126/science.aan0032
Bansal, M., Adamski, N. M., Toor, P. I., Kaur, S., Molnár, I., Holušová, K., et al. (2020). Aegilops umbellulata introgression carrying leaf rust and stripe rust resistance genes Lr76 and Yr70 located to 9.47-Mb region on 5DS telomeric end through a combination of chromosome sorting and sequencing. Theoret. Appl. Genet. 133, 903–915. doi: 10.1007/s00122-019-03514-x
Bayles, C. J., Ghemawat, M. S., and Aist, J. R. (1990). Inhibition by 2-deoxy-D-glucose of callose formation, papilla deposition, and resistance to powdery mildew in an ml-o barley mutant. Physiol. Mole. Plant Pathol. 36, 63–72. doi: 10.1016/0885-5765(90)90092-c
Ben-David, R., Xie, W., Peleg, Z., Saranga, Y., Dinoor, A., and Fahima, T. (2010). Identification and mapping of PmG16, a powdery mildew resistance gene derived from wild emmer wheat. Theoret. Appl. Genet. 121, 499–510. doi: 10.1007/s00122-010-1326-5
Bolger, A. M., Lohse, M., and Usadel, B. (2014). Trimmomatic: a flexible trimmer for Illumina sequence data. Bioinformatics 30, 2114–2120. doi: 10.1093/bioinformatics/btu170
Briggle, L. W. (1966). Transfer of Resistance to Erysiphe graminis f. sp. tritici from Khapli emmer and Yuma durum to hexaploid wheat. Crop Sci. 6, 459–461. doi: 10.2135/cropsci1966.0011183x000600050020x
Büschges, R., Hollricher, K., Panstruga, R., Simons, G., Wolter, M., Frijters, A., et al. (1997). The barley Mlo gene: a novel control element of plant pathogen resistance. Cell 88, 695–705. doi: 10.1016/s0092-8674(00)81912-1
Chapman, J. A., Ho, I., Sunkara, S., Luo, S., Schroth, G. P., and Rokhsar, D. S. (2011). Meraculous: de novo genome assembly with short paired-end reads. PLoS One 6:e23501. doi: 10.1371/journal.pone.0023501
Chhuneja, P., Kumar, K., Stirnweis, D., Hurni, S., Keller, B., Dhaliwal, H. S., et al. (2011). Identification and mapping of two powdery mildew resistance genes in Triticum boeoticum L. Theoret. Appl. Genet. 124, 1051–1058. doi: 10.1007/s00122-011-1768-4
Chrpová, J., Šíp, V., Štoèková, L., Stehno, Z., and Capouchová, I. (2013). Evaluation of resistance to Fusarium head blight in spring wheat genotypes belonging to various Triticum species. Czech J. Genet. Plant Breed. 49, 149–156. doi: 10.17221/117/2013-cjgpb
Desiderio, F., Bourras, S., Mazzucotelli, E., Rubiales, D., Keller, B., Cattivelli, L., et al. (2021). Characterization of the resistance to powdery mildew and leaf rust carried by the bread wheat cultivar Victo. Internat. J. Mole. Sci. 22:3109. doi: 10.3390/ijms22063109
Dreiseitl, A. (2003). Adaptation of Blumeria graminis f.sp. hordei to barley resistance genes in the Czech Republic in 1971–2000. Plant, Soil Env. 49, 241–248. doi: 10.17221/4120-pse
Dreiseitl, A. (2011). Differences in powdery mildew epidemics in spring and winter barley based on 30-year variety trials. Ann. Appl. Biol. 159, 49–57. doi: 10.1111/j.1744-7348.2011.00474.x
Dreiseitl, A. (2021). Powdery mildew resistance phenotypes of wheat gene bank accessions. Biology 10:846. doi: 10.3390/biology10090846
Dreiseitl, A. (2022). Postulation of specific disease resistance genes in cereals: a widely used method and its detailed description. Pathogens 11:284. doi: 10.3390/pathogens11030284
Feuillet, C., Langridge, P., and Waugh, R. (2008). Cereal breeding takes a walk on the wild side. Trends Genet. 24, 24–32. doi: 10.1016/j.tig.2007.11.001
Flor, H. H. (1971). Current status of the gene-for-gene concept. Ann. Rev. Phytopathol. 9, 275–296. doi: 10.1146/annurev.py.09.090171.001423
Fu, B., Chen, Y., Li, N., Ma, H., Kong, Z., Zhang, L., et al. (2013). pmX: a recessive powdery mildew resistance gene at the Pm4 locus identified in wheat landrace Xiaohongpi. Theoret. Appl. Genet. 126, 913–921. doi: 10.1007/s00122-012-2025-1
Giorgi, D., Farina, A., Grosso, V., Gennaro, A., Ceoloni, C., and Lucretti, S. (2013). FISHIS: fluorescence in situ hybridization in suspension and chromosome flow sorting made easy. PLoS One 8:e57994. doi: 10.1371/journal.pone.0057994
Han, J., Zhang, L.-S., Li, G.-Q., Zhang, H.-T., Xie, C.-J., Yang, Z.-M., et al. (2009). Molecular mapping of powdery mildew resistance gene MlWE18 in wheat originated from wild emmer (Triticum turgidum var. dicoccoides). Acta Agronomi. Sin. 35, 1791–1797. doi: 10.3724/sp.j.1006.2009.01791
Hao, Y., Liu, A., Wang, Y., Feng, D., Gao, J., Li, X., et al. (2008). Pm23: a new allele of Pm4 located on chromosome 2AL in wheat. Theoret. Appl. Genet. 117, 1205–1212. doi: 10.1007/s00122-008-0827-y
Hsam, S. L. K., Huang, X. Q., Ernst, F., Hartl, L., and Zeller, F. J. (1998). Chromosomal location of genes for resistance to powdery mildew in common wheat (Triticum aestivum L. em Thell.). 5. Alleles at the Pm1 locus. Theoret. Appl. Genet. 96, 1129–1134. doi: 10.1007/s001220050848
Hu, T. (2008). Identification and molecular mapping of the powdery mildew resistance gene in wheat cultivar Yumai 66. Acta Agronom. Sin. 34, 545–550. doi: 10.3724/sp.j.1006.2008.00545
International Wheat Genome Sequencing Consortium (IWGSC) (2018). Shifting the limits in wheat research and breeding using a fully annotated reference genome. Science 361:eaar7191. doi: 10.1126/science.aar7191
Janáková, E., Jakobson, I., Peusha, H., Abrouk, M., Škopová, M., Šimková, H., et al. (2019). Divergence between bread wheat and Triticum militinae in the powdery mildew resistance QPm.tut-4A locus and its implications for cloning of the resistance gene. Theoret. Appl. Genet. 132, 1061–1072. doi: 10.1007/s00122-018-3259-3
Ji, X., Xie, C., Ni, Z., Yang, T., Nevo, E., Fahima, T., et al. (2007). Identification and genetic mapping of a powdery mildew resistance gene in wild emmer (Triticum dicoccoides) accession IW72 from Israel. Euphytica 159, 385–390. doi: 10.1007/s10681-007-9540-1
Jørgensen, I. H. (1992). Discovery, characterization and exploitation of Mlo powdery mildew resistance in barley. Euphytica 63, 141–152. doi: 10.1007/bf00023919
Kao, C.-H., Zeng, Z.-B., and Teasdale, R. D. (1999). Multiple interval mapping for quantitative trait loci. Genetics 152, 1203–1216. doi: 10.1093/genetics/152.3.1203
Koller, T., Brunner, S., Herren, G., Hurni, S., and Keller, B. (2018). Pyramiding of transgenic Pm3 alleles in wheat results in improved powdery mildew resistance in the field. Theoret. Appl. Genet. 131, 861–871. doi: 10.1007/s00122-017-3043-9
Komáromi, J., Jankovics, T., Fábián, A., Puskás, K., Zhang, Z., Zhang, M., et al. (2016). Powdery mildew resistance in wheat cultivar Mv Hombár is conferred by a new gene, PmHo. Phytopathology 106, 1326–1334. doi: 10.1094/phyto-03-16-0152-r
Korol, A. B., Ronin, Y. I., Itskovich, A. M., Peng, J., and Nevo, E. (2001). Enhanced efficiency of quantitative trait loci mapping analysis based on multivariate complexes of quantitative traits. Genetics 157, 1789–1803. doi: 10.1093/genetics/157.4.1789
Kosambi, D. D. (1943). The estimation of map distances from recombination values. Ann. Eugen. 12, 172–175. doi: 10.1111/j.1469-1809.1943.tb02321.x
Kou, Y., and Wang, S. (2010). Broad-spectrum and durability: understanding of quantitative disease resistance. Curr. Opin. Plant Biol. 13, 181–185. doi: 10.1016/j.pbi.2009.12.010
Krattinger, S. G., Lagudah, E. S., Spielmeyer, W., Singh, R. P., Huerta-Espino, J., McFadden, H., et al. (2009). A putative ABC transporter confers durable resistance to multiple fungal pathogens in wheat. Science 323, 1360–1363. doi: 10.1126/science.1166453
Kubaláková, M., Valárik, M., Bartoš, J., Vrána, J., Cíhalíková, J., Molnár-Láng, M., et al. (2003). Analysis and sorting of rye (Secale cereale L.) chromosomes using flow cytometry. Genome 46, 893–905. doi: 10.1139/g03-054
Li, G., Cowger, C., Wang, X., Carver, B. F., and Xu, X. (2019). Characterization of Pm65, a new powdery mildew resistance gene on chromosome 2AL of a facultative wheat cultivar. Theoret. Appl. Genet. 132, 2625–2632. doi: 10.1007/s00122-019-03377-2
Li, N., Wen, Z., Wang, J., Fu, B., Liu, J., Xu, H., et al. (2013). Transfer and mapping of a gene conferring later-growth-stage powdery mildew resistance in a tetraploid wheat accession. Mole. Breed. 33, 669–677. doi: 10.1007/s11032-013-9983-0
Liang, J., Fu, B., Tang, W., Khan, N. U., Li, N., and Ma, Z. (2016). Fine mapping of two wheat powdery mildew resistance genes located at the Pm1 cluster. Plant Gen. 9:0084. doi: 10.3835/plantgenome2015.09.0084
Makepeace, J. C., Oxley, S. J. P., Havis, N. D., Hackett, R., Burke, J. I., and Brown, J. K. M. (2007). Associations between fungal and abiotic leaf spotting and the presence of mlo alleles in barley. Plant Pathol. 56, 934–942. doi: 10.1111/j.1365-3059.2007.01680.x
Maxwell, J. J., Lyerly, J. H., Cowger, C., Marshall, D., Brown-Guedira, G., and Murphy, J. P. (2009). MlAG12: a Triticum timopheevii-derived powdery mildew resistance gene in common wheat on chromosome 7AL. Theoret. Appl. Genet. 119, 1489–1495. doi: 10.1007/s00122-009-1150-y
McIntosh, R. A., Dubcovsky, J., Rogers, W. J., Xia, X. C., and Raupp, W. J. (2019). Catalogue of gene symbols for wheat: 2019 supplement. Available online at: https://wheat.pw.usda.gov/GG3/sites/default/files/Catalogue%20of%20Gene%20Symbols%20for%20Wheat%20-%20supplement2019.pdf
Miranda, L. M., Perugini, L., Srnić, G., Brown-Guedira, G., Marshall, D., Leath, S., et al. (2007). Genetic mapping of a Triticum monococcum-derived powdery mildew resistance gene in common wheat. Crop Sci. 47, 2323–2329. doi: 10.2135/cropsci2007.01.0053
Mohler, V., Bauer, C., Schweizer, G., Kempf, H., and Hartl, L. (2013). Pm50: a new powdery mildew resistance gene in common wheat derived from cultivated emmer. J. Appl. Genet. 54, 259–263. doi: 10.1007/s13353-013-0158-9
Niu, J. S., Wang, B. Q., Wang, Y. H., Cao, A. Z., Qi, Z. J., and Shen, T. M. (2008). Chromosome location and microsatellite markers linked to a powdery mildew resistance gene in wheat line Lankao 90(6). Plant Breed. 127, 346–349. doi: 10.1111/j.1439-0523.2007.01480.x
Ouyang, S., Zhang, D., Han, J., Zhao, X., Cui, Y., Song, W., et al. (2014). Fine physical and genetic mapping of powdery mildew resistance gene MlIW172 originating from wild emmer (Triticum dicoccoides). PLoS One 9:e100160. doi: 10.1371/journal.pone.0100160
Pearson, K. (1900). X. On the criterion that a given system of deviations from the probable in the case of a correlated system of variables is such that it can be reasonably supposed to have arisen from random sampling. Lond. Edinb. Dublin Philosoph. Mag. J. Sci. 50, 157–175. doi: 10.1080/14786440009463897
Peart, J. R., Mestre, P., Lu, R., Malcuit, I., and Baulcombe, D. C. (2005). NRG1, a CC-NB-LRR Protein, together with N, a TIR-NB-LRR Protein, Mediates Resistance against Tobacco Mosaic Virus. Curr. Biol. 15, 968–973. doi: 10.1016/j.cub.2005.04.053
Peleg, Z., Saranga, Y., Suprunova, T., Ronin, Y., Röder, M. S., Kilian, A., et al. (2008). High-density genetic map of durum wheat × wild emmer wheat based on SSR and DArT markers. Theoret. Appl. Genet. 117, 103–115. doi: 10.1007/s00122-008-0756-9
Perugini, L. D., Murphy, J. P., Marshall, D., and Brown-Guedira, G. (2007). Pm37, a new broadly effective powdery mildew resistance gene from Triticum timopheevii. Theoret. Appl. Genet. 116, 417–425. doi: 10.1007/s00122-007-0679-x
Peterhansel, C., Freialdenhoven, A., Kurth, J., Kolsch, R., and Schulze-Lefert, P. (1997). Interaction analyses of genes required for resistance responses to powdery mildew in barley reveal distinct pathways leading to leaf cell death. Plant Cell 9, 1397–1409. doi: 10.1105/tpc.9.8.1397
Piffanelli, P., Zhou, F., Casais, C., Orme, J., Jarosch, B., Schaffrath, U., et al. (2002). The barley MLO modulator of defense and cell death is responsive to biotic and abiotic stress stimuli. Plant Physiol. 129, 1076–1085. doi: 10.1104/pp.010954
Qiu, Y. C., Zhou, R. H., Kong, X. Y., Zhang, S. S., and Jia, J. Z. (2005). Microsatellite mapping of a Triticum urartu Tum. derived powdery mildew resistance gene transferred to common wheat (Triticum aestivum L.). Theoret. Appl. Genet. 111, 1524–1531. doi: 10.1007/s00122-005-0081-5
Ronin, Y., Mester, D., Minkov, D., and Korol, A. (2010). Building reliable genetic maps: different mapping strategies may result in different maps. Nat. Sci. 02, 576–589. doi: 10.4236/ns.2010.26073
Ronin, Y. I., Mester, D. I., Minkov, D. G., Akhunov, E., and Korol, A. B. (2017). Building ultra-high-density linkage maps based on efficient filtering of trustable markers. Genetics 206, 1285–1295. doi: 10.1534/genetics.116.197491
Sánchez-Martín, J., Steuernagel, B., Ghosh, S., Herren, G., Hurni, S., Adamski, N., et al. (2016). Rapid gene isolation in barley and wheat by mutant chromosome sequencing. Gen. Biol. 17:1. doi: 10.1186/s13059-016-1082-1
Schmolke, M., Mohler, V., Hartl, L., Zeller, F. J., and Hsam, S. L. K. (2011). A new powdery mildew resistance allele at the Pm4 wheat locus transferred from einkorn (Triticum monococcum). Mole. Breed. 29, 449–456. doi: 10.1007/s11032-011-9561-2
Schneider, D. M., Heun, M., and Fischbeck, G. (1991). Inheritance of the powdery mildew resistance gene Pm9 in relation to Pm1 and Pm2 of wheat. Plant Breed. 107, 161–164. doi: 10.1111/j.1439-0523.1991.tb00545.x
Šimková, H., Svensson, J. T., Condamine, P., Høibová, E., Suchánková, P., Bhat, P. R., et al. (2008). Coupling amplified DNA from flow-sorted chromosomes to high-density SNP mapping in barley. BMC Gen. 9:1. doi: 10.1186/1471-2164-9-294
Simons, G., van der Lee, T., Diergaarde, P., van Daelen, R., Groenendijk, J., Frijters, A., et al. (1997). AFLP-based fine mapping of the Mlo gene to a 30-kb DNA segment of the barley genome. Genomics 44, 61–70. doi: 10.1006/geno.1997.4844
Singrün, C. H., Hsam, S. L. K., Hartl, L., Zeller, F. J., and Mohler, V. (2003). Powdery mildew resistance gene Pm22 in cultivar Virest is a member of the complex Pm1 locus in common wheat (Triticum aestivum L. em Thell.). Theoret. Appl. Gen. 106, 1420–1424. doi: 10.1007/s00122-002-1187-7
Singrün, C. H., Hsam, S. L. K., Zeller, F. J., Wenzel, G., and Mohler, V. (2004). Localization of a novel recessive powdery mildew resistance gene from common wheat line RD30 in the terminal region of chromosome 7AL. Theoret. Appl. Genet. 109, 210–214. doi: 10.1007/s00122-004-1619-7
Srnić, G., Murphy, J. P., Lyerly, J. H., Leath, S., and Marshall, D. S. (2005). Inheritance and chromosomal assignment of powdery mildew resistance genes in two winter wheat germplasm lines. Crop Sci. 45, 1578–1586. doi: 10.2135/cropsci2004.0530
Tan, C., Li, G., Cowger, C., Carver, B. F., and Xu, X. (2018). Characterization of Pm59, a novel powdery mildew resistance gene in Afghanistan wheat landrace PI 181356. Theoret. Appl. Genet. 131, 1145–1152. doi: 10.1007/s00122-018-3067-9
The, T., McIntosh, R., and Bennett, F. (1979). Cytogenetical studies in wheat. IX. Monosomic analyses, telocentric mapping and linkage relationships of genes Sr21, Pm4 and Mle. Austral. J. Biol. Sci. 32, 115–125. doi: 10.1071/bi9790115
Untergasser, A., Cutcutache, I., Koressaar, T., Ye, J., Faircloth, B. C., Remm, M., et al. (2012). Primer3—new capabilities and interfaces. Nucleic Acids Res. 40:e115. doi: 10.1093/nar/gks596
Voorrips, R. E. (2002). MapChart: software for the graphical presentation of linkage maps and QTLs. J. Hered. 93, 77–78. doi: 10.1093/jhered/93.1.77
Vrána, J., Kubaláková, M., Simková, H., Číhalíková, J., Lysák, M. A., and Dolezel, J. (2000). Flow sorting of mitotic chromosomes in common wheat (Triticum aestivum L.). Genetics 156, 2033–2041. doi: 10.1093/genetics/156.4.2033
Wang, Y., Cheng, X., Shan, Q., Zhang, Y., Liu, J., Gao, C., et al. (2014). Simultaneous editing of three homoeoalleles in hexaploid bread wheat confers heritable resistance to powdery mildew. Nat. Biotechnol. 32, 947–951. doi: 10.1038/nbt.2969
Wolfe, M. S., and McDermott, J. M. (1994). Population genetics of plant pathogen interactions: the example of the Erysiphe graminis-Hordeum vulgare pathosystem. Annu. Rev. Phytopathol. 32, 89–113. doi: 10.1146/annurev.py.32.090194.000513
Worthington, M., Lyerly, J., Petersen, S., Brown-Guedira, G., Marshall, D., Cowger, C., et al. (2014). MlUM15: an Aegilops neglecta-derived powdery mildew resistance gene in common wheat. Crop Sci. 54, 1397–1406. doi: 10.2135/cropsci2013.09.0634
Xu, W., Li, C., Hu, L., Wang, H., Dong, H., Zhang, J., et al. (2011). Identification and molecular mapping of PmHNK54: a novel powdery mildew resistance gene in common wheat. Plant Breed. 130, 603–607. doi: 10.1111/j.1439-0523.2011.01882.x
Yao, G., Zhang, J., Yang, L., Xu, H., Jiang, Y., Xiong, L., et al. (2006). Genetic mapping of two powdery mildew resistance genes in einkorn (Triticum monococcum L.) accessions. Theoret. Appl. Genet. 114, 351–358. doi: 10.1007/s00122-006-0438-4
Keywords: wheat, powdery mildew (Blumeria graminis D. C. f. sp. tritici), resistance, emmer, GZ1, QTL mapping
Citation: Korchanová Z, Švec M, Janáková E, Lampar A, Majka M, Holušová K, Bonchev G, Juračka J, Cápal P and Valárik M (2022) Identification, High-Density Mapping, and Characterization of New Major Powdery Mildew Resistance Loci From the Emmer Wheat Landrace GZ1. Front. Plant Sci. 13:897697. doi: 10.3389/fpls.2022.897697
Received: 16 March 2022; Accepted: 13 April 2022;
Published: 13 May 2022.
Edited by:
Thomas Miedaner, University of Hohenheim, GermanyReviewed by:
Volker Mohler, Bayerische Landesanstalt für Landwirtschaft (LfL), GermanyJingzhon Xie, Institute of Genetics and Developmental Biology (CAS), China
Copyright © 2022 Korchanová, Švec, Janáková, Lampar, Majka, Holušová, Bonchev, Juračka, Cápal and Valárik. This is an open-access article distributed under the terms of the Creative Commons Attribution License (CC BY). The use, distribution or reproduction in other forums is permitted, provided the original author(s) and the copyright owner(s) are credited and that the original publication in this journal is cited, in accordance with accepted academic practice. No use, distribution or reproduction is permitted which does not comply with these terms.
*Correspondence: Miroslav Valárik, dmFsYXJpa0B1ZWIuY2FzLmN6