- Coastal Salinity Tolerant Grass Engineering and Technology Research Center, Ludong University, Yantai, China
Nitrogen (N) application is one of the most effective methods to alleviate salt-induced damage on plants. Forage bermudagrass has higher utilization potential on saline soil, but whether its N requirement changed under high salt stress has not been studied. Through examining plant growth-related traits, salt-stress-responsive physiological traits, photosynthesis, N metabolism, and forage quality supplied with different N concentrations under high salt stress (200 mM NaCl), we noticed that the optimum N requirement of forage bermudagrass reduced. When supplied with 10 mM N under higher salt stress, plants had a similar biomass, turf color, and chlorophyll content with plants supplied with 15 mM N, accompanied by a lower firing rate and Na+ content of leaves. The N content, crude protein, crude fat content, the expression of AMTs (ammonium transporters), NR (nitrate reductase), GS (glutamine synthetase), and GOGAT (glutamate synthetase), the chlorophyll fluorescence curve, and parameters of leaves (e.g., PIABS; PICS; ABS/RC; TRo/RC; ETo/RC) all peaked under 10 mM N under high salt stress instead of 15 mM N. Through exploring the proper N application under higher salt stress and its alleviation mechanisms, our results indicated that moderate reduction in N application under high salt level had a maximum promotion effect on the salt tolerance of forage bermudagrass without growth or forage quality inhibition. These response mechanisms obtained can provide a useful reference for N application in moderation rather than in excess on forage bermudagrass, especially in higher salinity areas.
Introduction
Bermudagrass [Cynodon dactylon (L.) Pers.] is one of the most widely used grass species as forage and turfgrass in warm climatic regions. Bermudagrass was also considered to be a salt-tolerant grass species (Maas and Hoffman, 1977; Chen and Liu, 2012). However, the growth, forage quality, and turf quality of bermudagrass could be seriously inhibited by salt stress (Marcum and Pessarakli, 2006), greatly limiting its wide application in salinity areas. Salt stress generally disrupts the osmotic and ionic equilibrium in bermudagrass cells, which would lead to ionic toxicity, osmotic stress, and secondary stresses (Ahanger et al., 2019; Maksup et al., 2020; Amin et al., 2021), including nutritional imbalance, inhibition of photosynthesis, and membrane disorganization (Munns and Tester, 2008). Like other plants, bermudagrass has established corresponding response mechanism in acclimation to external salt stress to reduce their damage (Deinlein et al., 2014; Zhao et al., 2020), such as the induction of antioxidant enzymes (Baby and Jini, 2010; Ahmad et al., 2019), ion transport and compartmentation, compatible solutes synthesis, and accumulation (Zhao et al., 2020).
Nitrogen (N) fertilizer plays a critical role in alleviating salt-induced damage in plants (Mansour, 2000; Villa et al., 2003; Esmaili et al., 2005; Jia et al., 2017). As the most important macro-nutrient of plants, N is an important constituent of the nucleic acids, proteins, chlorophyll, and many other N metabolites (Kishorekumar et al., 2020). N also mediates the utilization of potassium, phosphorus, and other elements and serves as an important component of proteins involved in a series of metabolic processes to coordinate plant growth and development (Zubillaga et al., 2002). Two primary inorganic N sources ammonium (NH4+) and nitrate (NO3–) from soil can be directly absorbed by roots and are transported to plants by their respective transporters ammonium transporters (AMTs) and nitrate transporters (NRT) (Giagnoni et al., 2016; Dechorgnat et al., 2019). The NH4+ absorbed must be transformed into NO3– under the catalyst of nitrate reductase (NR). Then, NO3– can be further assimilated and converted into amino acid through glutamine synthetase (GS) and glutamate synthase (GOGAT) metabolic pathway, which was considered to be the main pathway for N assimilation in plants (Xu et al., 2012).
However, reduced N uptake of plants always occurs due to the high Na+ and Cl– content in the saline soil (Akram et al., 2011). Salinity results in significantly decreased activities of NR, GS, and GOGAT in many plants species, such as maize and rice (Khan and Srivastava, 1998; Wang et al., 2012), and further inhibits the N use efficiency in plants (Murtaza et al., 2013; Singh et al., 2016; Teh et al., 2016). Moreover, several N-containing compounds could be induced by salt stress and contributed to salt tolerance of plants (Hoai et al., 2003; Dluzniewska et al., 2007; Sudmalis et al., 2018) through participating in osmotic adjustment, the promotion of photosynthetic capacity, and mitigation of oxidative stress caused by excessive ROS (Homaee et al., 2002; Song et al., 2006). Therefore, N application is considered to be one of the most effective methods of improving plant growth in salinity regions where N content is lower than that in non-salinity land.
Related research also showed that, rather than N alone, plant growth was significantly affected by the interaction between soil salinity and N. Some reports showed that the alleviation effects of N fertilizer supplied might associate with its concentrations supplied. For instance, low-to-moderate N application could mitigate the adverse effects from salt stress, while excessive N could aggravate the negative effects of salt stress on cotton (Chen et al., 2010). Moreover, excessive application or inefficient use of N fertilizer will lead to secondary salinization, which in turn adversely inhibits plant growth (Soussi et al., 1998; Chen et al., 2010; Lacerda et al., 2016). In ryegrass, moderately reducing N application has the maximum alleviation effect, especially under mild salt stress (Shao et al., 2020). To sum up, the N requirement of plants might be changed (demand goes up or down) under a certain level of salt stress (higher and lower). However, there were no consistent regular changes in the N requirement under different salt concentrations or among different species.
Although bermudagrass has higher utilization potential on saline soil, excessive N application on higher levels of saline soil that cannot be fully utilized by bermudagrass might contribute to N leaching and lead to groundwater pollution. To this end, we attempt to reveal the physiological response mechanism of bermudagrass to salt stress after moderate N application by synthetically analyzing the growth rate, physiological indicators, quality-related traits, and the metabolism of N under control and salt stress treatment with different N supplied levels. The response mechanism obtained will be important for the agricultural practice to guide rational N fertilization application on forage bermudagrass cultures to further improve their salt stress resistance, maintain their growth and quality, and promote their utilization, especially under severe salt stress.
Materials and Methods
Plant Materials and Growth Conditions
A common tetraploid bermudagrass cultivar “Wrangle” was used in this experiment. The experimental materials used for treatment were generated by asexual propagation using the cuttings at the top of each branch from the original bermudagrass plants cultured in the greenhouse. The cuttings were planted in a solid medium (sand applied with Hoagland solution) for 1 week, and then, the same number of uniform stolons (about 20 stolons per pot) in each pot was retained in the solid medium. After 1 month, the plants of each pot were then removed from the solid medium and washed clean with water. Before the treatments were initiated, the plants were cultured in Hoagland solution to acclimate for 1 week. Subsequently, the plants were cut to the same height and transferred into different treatments in a hydroponic culture using a modified Hoagland solution. Each treatment contains four replicates. The treatments used NH4NO3 as an N source. The other components included 0.2 mM KH2PO4, 1 mM MgSO4, 1.5 mM KCl, 2.5 mM CaCl2, 1 × 10–3 mM H3BO3, 5 × 10–5 mM (NH4)6Mo7O24, 5 × 10–4 mM CuSO4, 1 × 10–3 mM ZnSO4, 1 × 10–3 mM MnSO4, and 0.1 mM Fe(III)-EDTA. The hydroponic culture media were processed in a growth chamber under the following conditions: 22/18°C (day/night), 60% relative humidity, 450 μmol m–2 s–1 photons, and a 16-h day/8-h night cycle. The culture solution was refreshed every 2 days.
Experimental Design
The salt treatment condition of bermudagrass was determined based on previous studies (Dudeck et al., 1983; Adavi et al., 2006) through a simple and fast short-term salt treatment experiment (Marcum and Pessarakli, 2006; Chen et al., 2008). A pre-experiment (experiment 1) was conducted to observe the salt-induced damage of bermudagrass supplied with different N levels (2, 5, 10, and 15 mM) grown under different NaCl levels (a single step—up to 0, 50, 100, and 200 mM, respectively). After 1 month of treatment, the plant growth rate and leaf Na+ content were detected. According to the results of pre-experiment, 2 mM (deficiency), 10 mM (moderate), and 15 mM (excessive) N levels were chosen to explore the underlying alleviation mechanism of the reduction in N demand under high salt stress (a single step—up to 200 mM NaCl) in the subsequent experiment (experiment 2). After 1-month treatment of experiment 2, the plant growth-related trait (plant height, biomass, turf color, and leaf firing rate), salt-stress-responsive physiological traits (ion content and antioxidant enzyme activities), nitrogen content, chlorophyll content, chlorophyll a fluorescence transient, and quality-related traits of samples were measured and the gene expression involved in N metabolism was analyzed.
Measurements
Plant Growth-Related Trait
Before treatment of experiments 1 and 2, the plant height and biomass of each sample were recorded. After treatment for 1 month, the plant height and the relative increment of biomass were determined according to the method described by Hu et al. (2013). The leaf color (based on a scale of 1–9, with 9 being best) and leaf firing rate were valued based on visual inspection (Fu and Huang, 2001).
Ion Content
After treatment, the plant samples were dried at 130°C for half an hour and then placed at 75°C for 3 days to further determine ion contents. 0.1 g dried sample was finely ground and then digested with 10 mL sulfuric acid using graphite digestion apparatus (SH220N; Hanon, Jinan, Shandong, China) with a temperature of 420°C for 2 h. After digestion, the supernatant was diluted 5–10 times. Na+ and K+ contents of each sample were measured by a flame spectrophotometer (F-500; Shanghai, China) based on the method as previously described (Fu and Huang, 2001).
Antioxidant Enzyme Activity
Fully expanded fresh leaves (0.3 g) were ground into powder with liquid nitrogen. Ice-cold phosphate buffer (4 mL; 50 mM, pH 7.8) was added to the powder. All the samples were centrifuged at 12,000 rpm for 20 min at 4°C. The supernatant was collected to measure the activity of antioxidant enzyme based on the method as previously described (Fu and Huang, 2001).
Nitrogen Content and Quality-Related Traits
After the samples were dried, green leaves, old leaves, stems, and roots were separated and ground into powder using a grinder (DFT40; Jiuping, Wuxi, Jiangsu, China). Then, the samples were digested with 10 mL sulfuric acid using graphite digestion apparatus under the temperature of 420°C for 2 h. The nitrogen content and crude protein content of samples were determined by the Kjeldahl apparatus. The Soxhlet extraction method was used to determine the crude fat content. The crude fiber content was measured according to a protocol by the International Standard Organization (2000) intermediate filtration method. The weighed samples were boiled by adding H2SO4 followed by NaOH. The extracted residue was dried at 130°C for 2 h (KSL-1200X; KeJing, Hefei, Anhui, China). Then, the dried sample was weighed and put in a furnace (500°C for 3 h). Finally, the crude fiber content of each sample was weighed. The crude ash content was determined by the high-temperature burning method. Samples were weighed and put into the muffle furnace. The temperature of the muffle furnace was set to 580°C for 5 h, and then, the crude ash of each sample was weighed. The quality-related traits were determined according to the AOAC method.
Chlorophyll Content and Chlorophyll a Fluorescence Transient
After treatment, leaves (fresh weight: 0.1 g) were chopped and placed into centrifuge tubes containing 4 mL dimethyl sulfoxide for 3 days. One milliliter of extract and 2 mL of dimethyl sulfoxide were mixed. The UV absorbance of each mixture was measured at 663 and 645 nm wavelengths by a UV spectrophotometer (UV-1700; Meixi, Shanghai, China). Chlorophyll fluorescence transient (OJIP curve) was determined using a pulse-amplitude modulation fluorimeter (PAM 2500, Heinz Walz GmbH). Before measurement, the plants were pre-processed in the dark for half an hour and then exposed to 3,000 μmol photons m–2 s–1 red light condition. Each treatment was repeated three times. The chlorophyll fluorescence parameters were further determined according to the calculation method described previously (Yusuf et al., 2010).
Gene Expression Analysis
Fresh leaves of each sample were quickly ground into powder with liquid nitrogen. RNA was extracted using RNA pure Plant Kit (Tiangen Biotech, Beijing, China) and then reverse-transcribed using ReverTra Ace qPCR RT Kit (Applied Biosystems, Foster City, CA). Quantitative real-time RT-PCR analysis was performed using SYBR Green real-time PCR master mix (YeSen, Shanghai, China) and ABI real-time PCR system (Applied Biosystems, Foster City, CA) as described before (Hu et al., 2013). The relative expression level of each gene was determined according to the 2–ΔCt method (Vandesompele et al., 2002). The technical requirement of RT-qPCR fitted MIQE Guidelines (Bustin et al., 2009). Specific primers of selected genes are listed in Supplementary Table 6.
Statistical Analysis
Two-way analysis of variance (ANOVA) was used with “N” and “Salt” as two main factors and “N*Salt” as one interaction term. The significance level was set at P < 0.05 as a threshold to analyze the significant affection of individual factors and their interactions with detected variables in this study. Histogram results were expressed as mean ± SD of four replicates. Tukey’s test was used to evaluate the effects of N and Salt application.
Results
Plant Growth and Na+ Content Under Different Nitrogen and Salt Levels
We first made a pre-experiment to observe the salt-induced damage of bermudagrass supplied with different N levels (2, 5, 10, and 15 mM) grown under different NaCl levels (0, 50, 100, and 200 mM) (Supplementary Figure 1). Under control (0 mM NaCl) and low salt conditions (50 and 100 mM NaCl), the plant height peaked at 15 mM N (Supplementary Figure 1A). After a higher NaCl exposure (200 mM NaCl), the plant height showed no significant difference among different N levels (Supplementary Figures 1A,C). Moreover, plants grown under 10 mM N had the lowest leaf Na+ content under 200 mM NaCl treatment (Figure 1B). We then chose 2 mM N (deficiency), 10 mM N (moderate), and 15 mM N (excessive) and did a separate experiment to focus on the alleviation mechanism of moderate N application at high salt levels (200 mM NaCl). The results showed a similar tendency to the pre-experiment. Without NaCl treatment, the plant height increased with the increase in N concentration and peaked under 15 mM N, while the biomass peaked under 10 mM N and then declined under 15 mM N. When exposed to 200 mM NaCl, the biomass of plants had no significant alteration among different N levels (Figure 1B). Besides, both N and salt significantly affected plant height (Supplementary Table 1 and Figures 1A,B). Plant biomass was significantly affected by salt, but not by N (Supplementary Table 1 and Figure 1B). The interactive effect of N by salt was significant for both plant height and biomass (Supplementary Table 1). Moreover, the plants supplied with 10 mM N under salt stress had a similar turf color (Figure 1C) and a lower leaf firing rate (Figure 1D) compared with the plants supplied with 15 mM N.
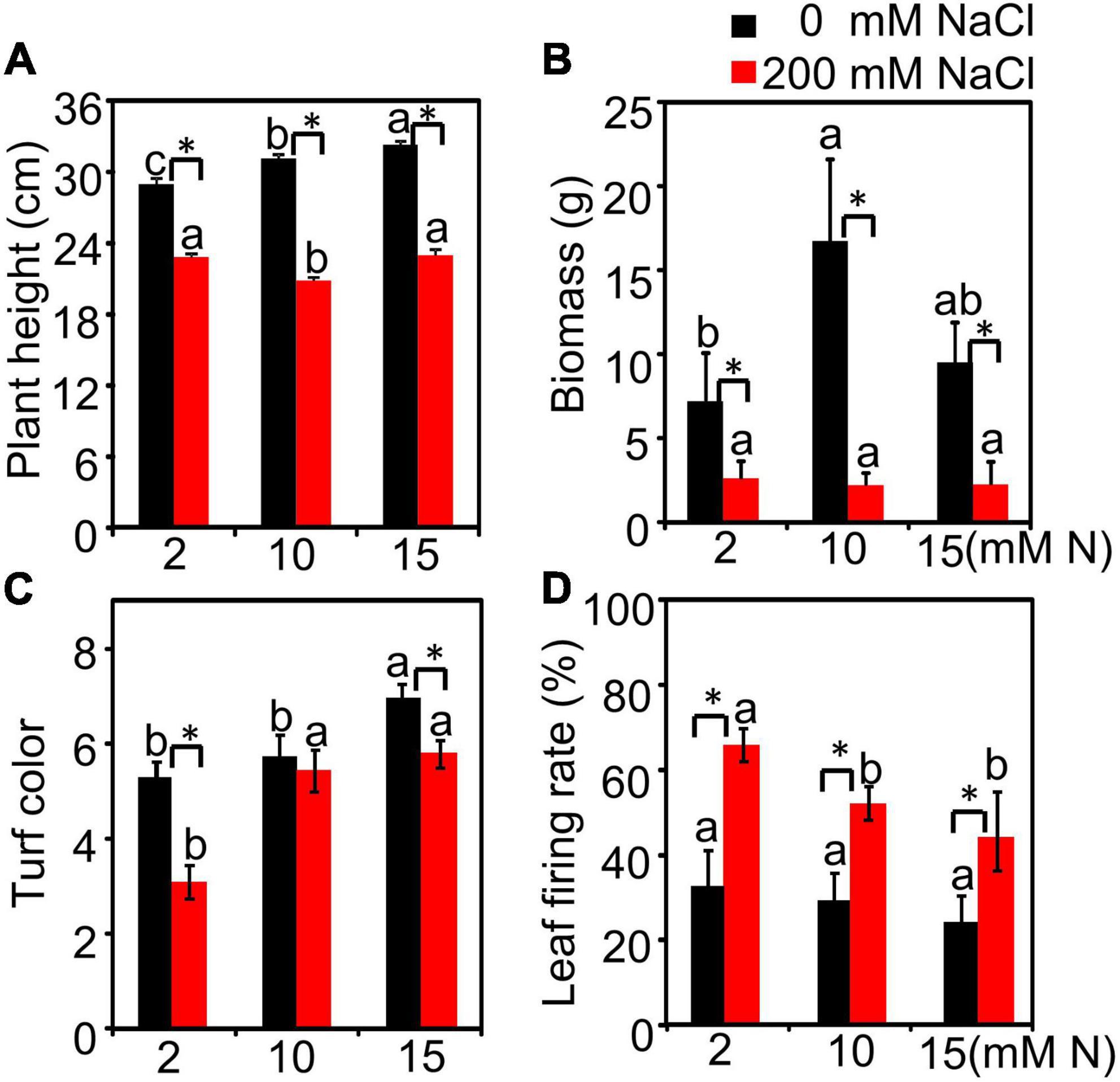
Figure 1. Morphological parameters of bermudagrass grown under different treatments. The plants were cut to the same height and then transferred into different nitrogen levels (2, 10, and 15 mM) under NaCl (0 and 200 mM) treatment in hydroponic culture. After treatment, the plant height (A), biomass (B), leaf color (C), and firing rate (D) were measured. Different letters above the columns indicate statistically significant differences at P < 0.05 under different N levels with the same NaCl level by Tukey’s test. * Shows significant differences (P < 0.05) under 200 mM NaCl treatment and 0 mM NaCl condition with the same N level by Tukey’s test.
Na+ Content Affected by Nitrogen Levels Under High Salt Stress
The Na+ and Na+/K+ of different tissues detected from different treatments were further measured. The results showed that salt had a significant effect on Na+ and Na+/K+ of all detected tissues, while N had no significant effect on roots Na+ and Na+/K+ of green leaves and roots. The interactive effect of N by salt was significant for Na+ of green leaves, stems, old leaves, and roots and the Na+/K+ of green leaves, old leaves, and roots (Supplementary Table 2). Under the high salt condition, the Na+ contents of green leaves and stems had the lowest values when plants were grown with 10 mM N (Figures 2A,B). Moreover, the Na+ content of old leaves grown under 10 mM N had a significantly lower value compared with that grown under 15 mM N (Figure 2C). However, the content of Na+ of roots showed no significant difference among different N levels (Figure 2D). When grown with 10 or 15 mM N, the Na+/K+ of green leaves significantly declined compared with 2 mM N under salt stress. However, the Na+/K+ of plants grown with 10 or 15 mM N showed no significant difference (Figure 2E). Moreover, in the stems, the value of Na+/K+ showed no significant difference among different N levels under high salt stress (Figure 2F). When grown under 10 mM N, the Na+/K+ of old leaves had a significantly lower value compared with that grown under 15 mM N (Figure 2G), while the Na+/K+ of roots had no significant difference among N levels (Figure 2H).
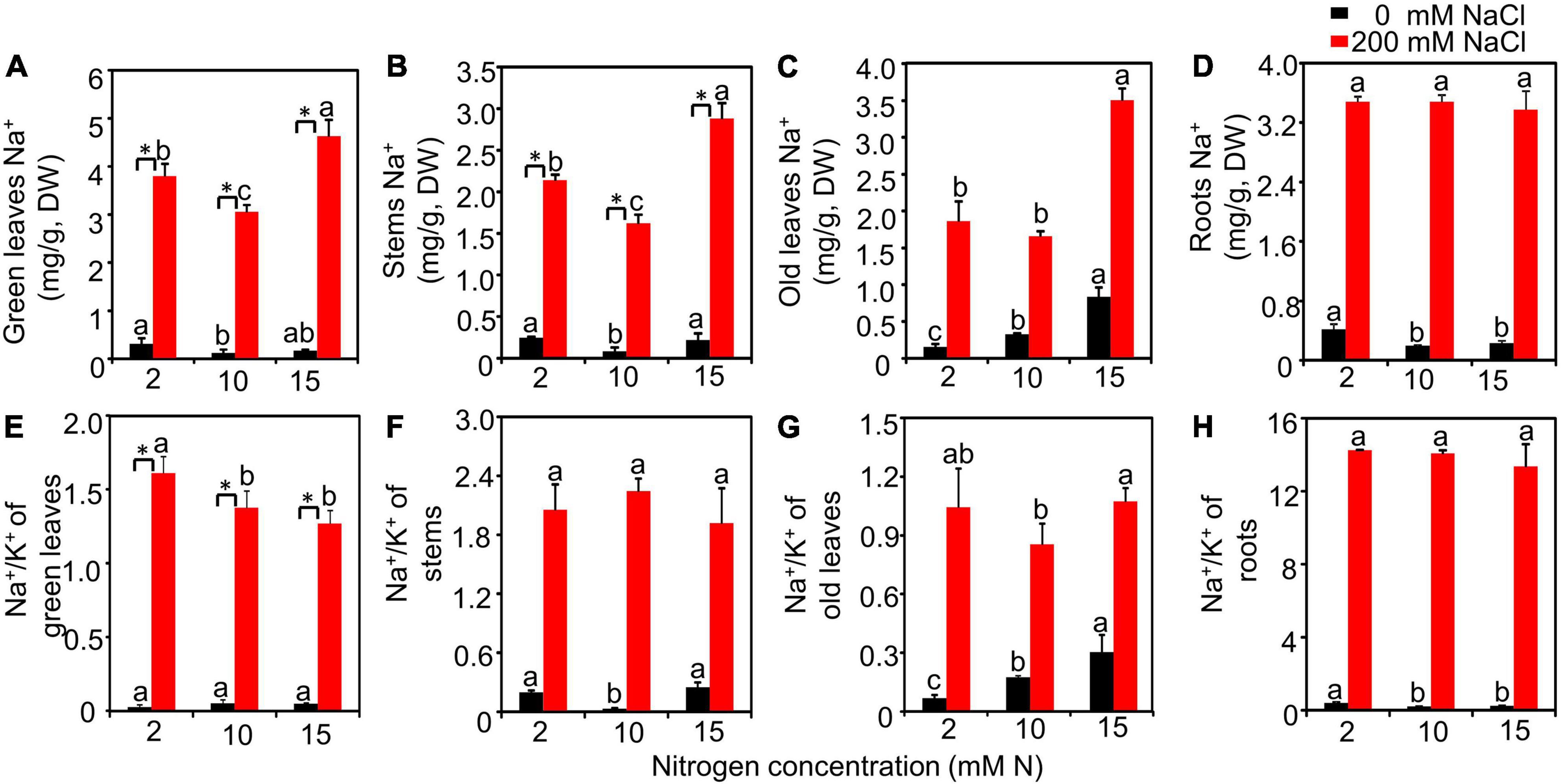
Figure 2. The Na+ content and Na+/K+ of the bermudagrass under different treatments. Na+ content of green leaves (A), stems (B), old leaves (C), and roots (D) and Na+/K+ of green leaves (E), stems (F), old leaves (G), and roots (H) of plants grown with different nitrogen concentrations exposed to different NaCl levels, respectively. Different letters above the columns indicate statistically significant differences at P < 0.05 under different N levels with the same NaCl level by Tukey’s test. * Shows significant differences (P < 0.05) under 200 mM NaCl treatment and 0 mM NaCl condition with the same N level by Tukey’s test.
Antioxidant Enzyme Activities Affected by Nitrogen Levels Under High Salt Stress
The antioxidant enzyme activities in the leaves of plants grown under different treatments were also determined. The results showed that, with the increase in N application level, the peroxidase (POD) enzyme activity showed an upward trend and peaked at 10 mM N level followed by a downward trend under both control and salt conditions (Figure 3A). Under control conditions, the catalase (CAT) enzyme activity had a higher value when the plants were supplied with 15 mM N compared with 10 mM N (Figure 3B). After 200 mM NaCl exposure, the CAT and superoxide dismutase (SOD) enzyme activity of leaves increased slightly when the plants were grown under 10 mM N condition compared with 2 and 15 mM N conditions, but the difference was not significant (Figures 3B,C).
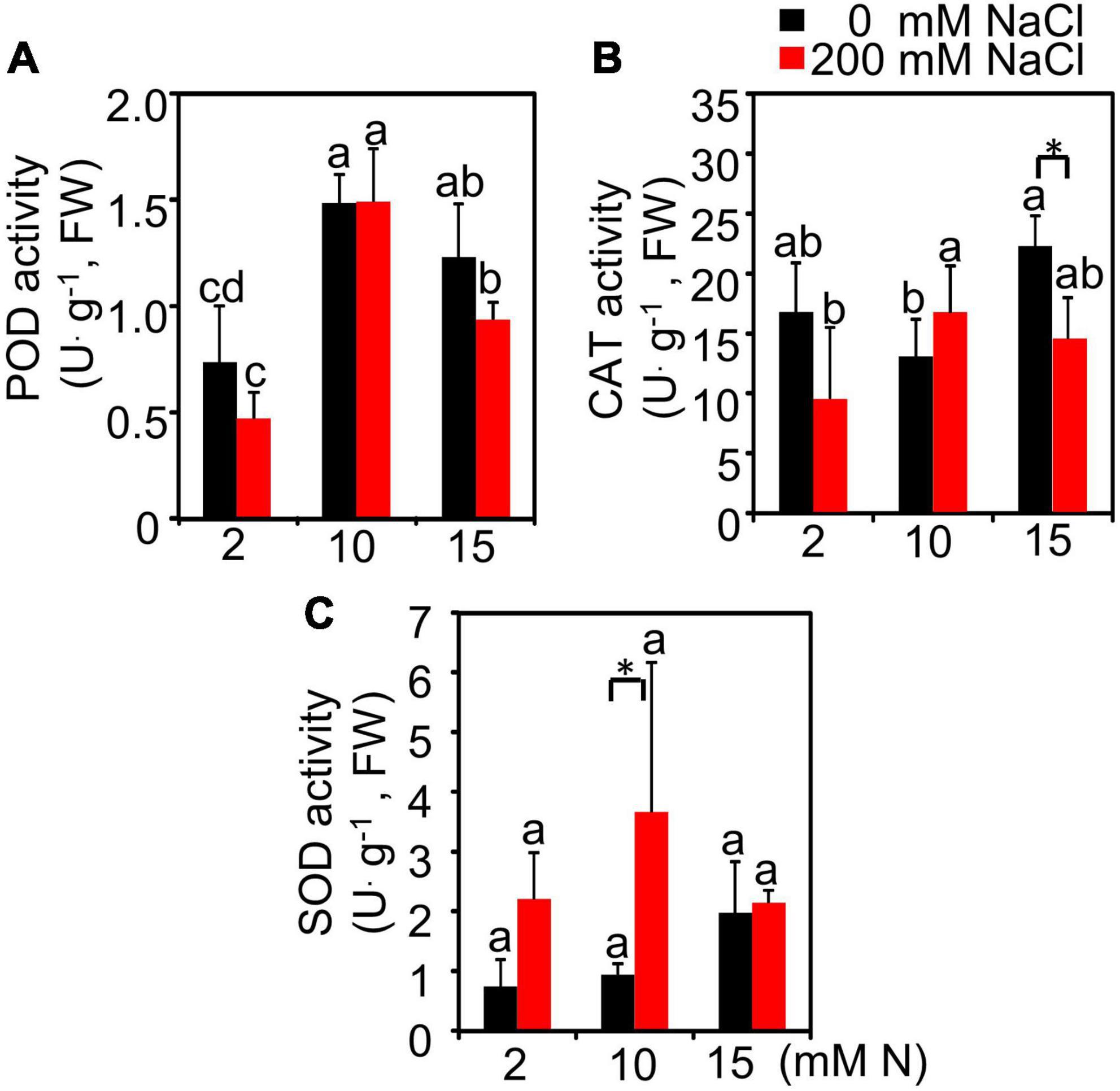
Figure 3. Effect of nitrogen application on the antioxidant enzyme activities of bermudagrass under control and salt treatment. POD activity (A), CAT activity (B), and SOD activity (C) in the leaves of plants grown with different nitrogen concentrations (2, 10, and 15 mM N) exposed to control (0 mM NaCl) and salt treatment (200 mM NaCl), respectively. Different letters above the columns indicate statistically significant differences at P < 0.05 under different N levels with the same NaCl level by Tukey’s test. * Shows significant differences (P < 0.05) under 200 mM NaCl treatment and 0 mM NaCl condition with the same N level by Tukey’s test.
Nitrogen and Protein Content Affected by Nitrogen Levels Under High Salt Stress
We then measured the N content and crude protein of green leaves and the residual N and crude protein content in old leaves and stems from different treatments. The results showed that N had a significant effect on N content or crude protein of green leaves and stems but had no significant effect on N content or crude protein content of old leaves. The effect of salt and the interactive effect of N by salt were significant for these variables measured (Supplementary Table 3). Without salt treatment, the N content of green leaves increased with the increase in N concentration and peaked under 15 mM N. After 200 mM NaCl exposure, the N content of green leaves peaked under 10 mM N and then declined under 15 mM N (Figure 4A). Without NaCl treatment, the residual N content in stems of plants grown with 2 mM N had a significantly lower value than those grown with 10 or 15 mM N. When 200 mM NaCl was added, the residual N content in stems of plants had the lowest value under 10 mM N (Figure 4B). Under high salt conditions, the residual N content in old leaves significantly increased under all three N levels. In addition, the residual N content in the stems of plants grown with 2 mM N was lower than those grown with 10 or 15 mM N (Figure 4C). The crude protein content of green leaves grown under control conditions peaked under 15 mM N, while it peaked under 10 mM N when the plants were grown under high salt conditions. This trend was consistent with that of the N content of green leaves (Figure 4D). The crude protein of stems and old leaves showed a significant increase under a high level of N application (15 or 10 mM) compared with a low level of N application (2 mM). After 200 mM NaCl exposure, although the crude protein of old leaves decreased when plants were grown under higher N levels (10 or 15 mM), the residual crude protein of stems has no significant difference among all three N levels (Figures 4E,F).
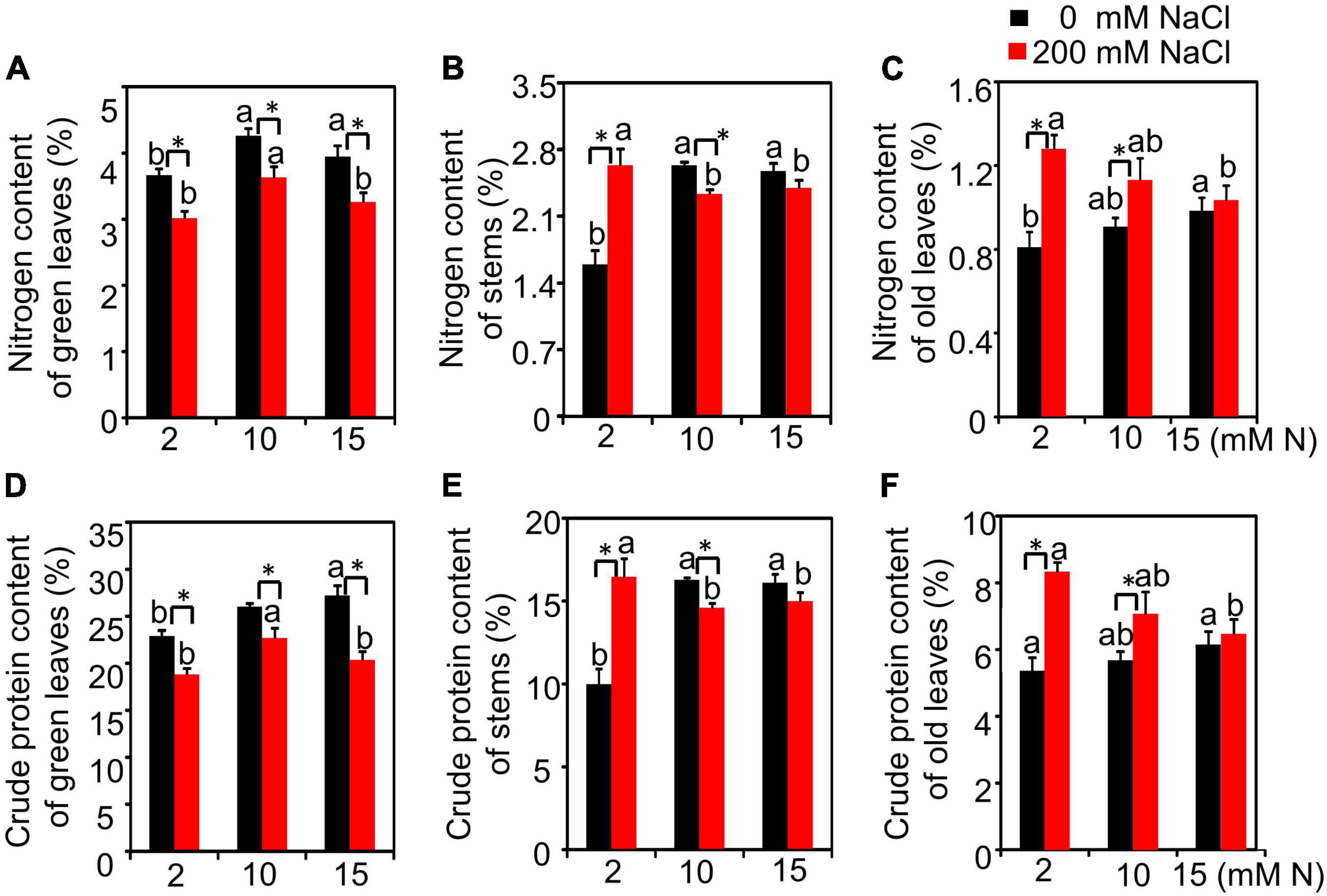
Figure 4. The nitrogen content and crude protein content of bermudagrass under different treatments. Nitrogen content of green leaves (A), stems (B), and old leaves (C). Crude protein of green leaves (D), stems (E), and old leaves (F). Different letters above the columns indicate statistically significant differences at P < 0.05 under different N levels with the same NaCl level by Tukey’s test. * Shows significant differences (P < 0.05) under 200 mM NaCl treatment and 0 mM NaCl condition with the same N level by Tukey’s test.
Nitrogen Metabolism-Related Gene Expression Affected by Nitrogen Levels Under High Salt Stress
The N content and crude protein of green leaves and the residual N and crude protein content in old leaves and stems from different treatments were further determined. N had a significant effect on NR, AMT, and GOGAT expression, while salt only had a significant effect on NR expression. The interactive effect of N by salt was significant for the expression of all genes detected (Supplementary Table 4). Without NaCl treatment, the expression of NR increased with the increase in N concentration and peaked at 15 mM N. After 200 mM NaCl exposure, although NR expression was inhibited by salt, there was no significant difference among different N concentrations (Figure 5A). AMT gene expression showed no significant difference among plants grown with different N concentrations under control conditions. Under high salt stress, the expression level of AMT showed an increased trend compared with the control condition when plants were grown with 10 mM N and the expression level was significantly higher than the other two N concentrations. On the contrary, the expression level of AMT decreased under 2 or 15 mM N (Figure 5B) after 200 mM NaCl exposure. Under control conditions, the expression of GS and GOGAT both peaked under 15 mM N. Salt stress significantly induced the expression of GS when supplied with 10 mM N, while the expression of GS was reduced significantly by salt when the plants were supplied with 15 mM N (Figure 5C). Moreover, under high salt stress conditions, the expression of GS and GOGAT both peaked under 10 mM N and then significantly declined under 15 mM N (Figures 5C,D).
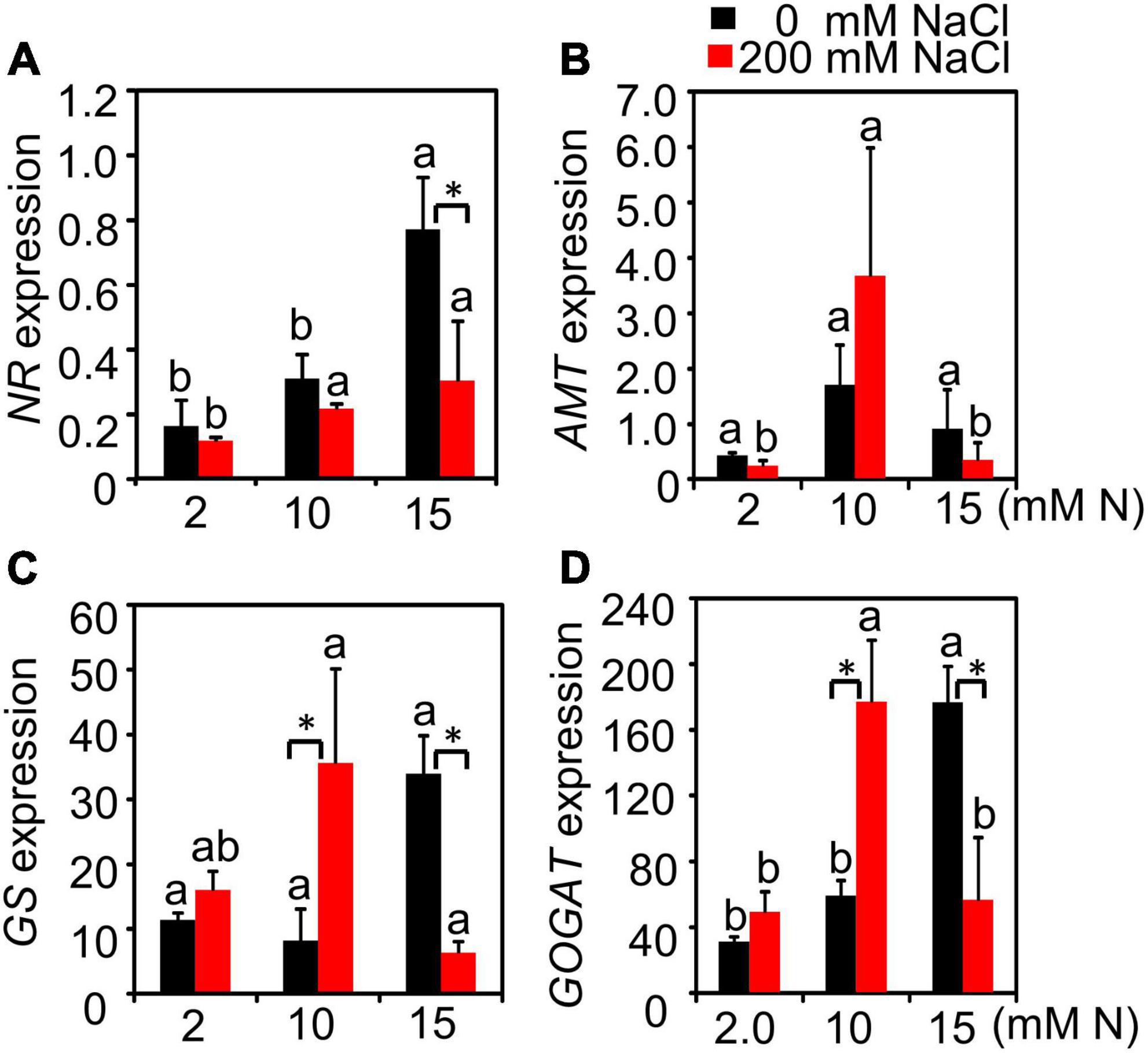
Figure 5. Relative expression level of N metabolism-related genes of bermudagrass under different treatments. The expression level of NR (A), AMT (B), GS (C), and GOGAT (D) in the leaves of bermudagrass grown under different nitrogen concentrations exposed to different NaCl levels (0 and 200 mM NaCl), respectively. Different letters above the columns indicate statistically significant differences at P < 0.05 under different N levels with the same NaCl level by Tukey’s test. * Shows significant differences (P < 0.05) under 200 mM NaCl treatment and 0 mM NaCl condition with the same N level by Tukey’s test.
Chlorophyll Fluorescence Affected by Nitrogen Levels Under High Salt Stress
The total chlorophyll content of leaves grown with different treatments was measured. Both N and salt significantly affected total chlorophyll content. The interactive effect of N by salt was not significant on total chlorophyll content (Supplementary Table 1). NaCl exposure did not significantly affect chlorophyll content as compared to the control condition (Figures 6A,B). However, the chlorophyll a and total chlorophyll content of leaves reached the highest level when the plants were grown under 10 mM N and then decreased slightly under 15 mM N with or without NaCl treatment (Figures 6A,B).
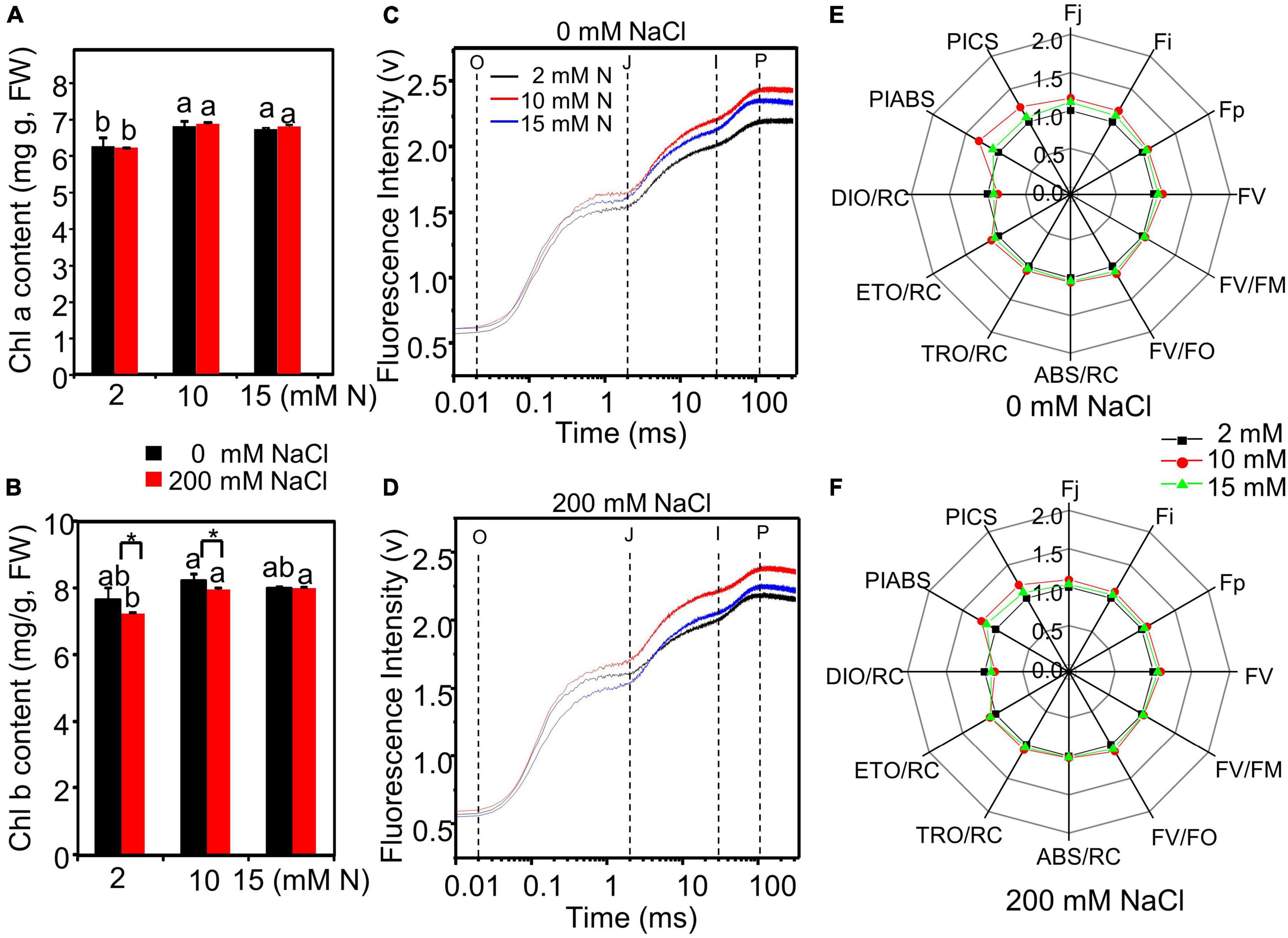
Figure 6. Chlorophyll fluorescence in the leaves of bermudagrass grown with different nitrogen levels under control and salt treatment. Chlorophyll a content (A) and total chlorophyll content (B) and OJIP curve in the leaves of bermudagrass grown with different nitrogen levels (2, 10, and 15 mM) under 0 mM (C) and 200 mM NaCl (D) concentrations, respectively (O: at 0.2 ms; J: at 2 ms; I: at 30 ms; P: at maximum fluorescence position). “Radar plots” of picked parameters characterizing the influence of nitrogen concentration on PS II of bermudagrass grown under 0 mM (E) and 200 mM NaCl (F), respectively. The parameters of plants grown under 2 mM N were set as control. Control = 1. * Shows significant differences (P < 0.05) under 200 mM NaCl treatment and 0 mM NaCl condition with the same N level by Tukey’s test.
Then, the impact of N levels on the photochemistry of photosystem II (PS II) of NaCl-treated bermudagrass was determined through chlorophyll a fluorescence transient–JIP test. Under control or salt condition, the fluorescence of the I and P phases of leaves grown with 10 mM N was stronger than those grown with 15 mM N, respectively (Figure 6). In particular, the OJIP curve was much higher when plants were exposed to salt under 10 mM N compared with plants grown with 15 mM N under control conditions (Figures 6C,D). The chlorophyll fluorescence parameters were further determined. Under control and salt conditions, the PIABS and PICS values, representing the overall activity of PSII, peaked under 10 mM N (Figures 6E,F and Supplementary Figures 2A,B). The absorption flux per RC (QA-reducing PSII reaction center) (ABS/RC) (Figure 6 and Supplementary Figure 2C), trapped energy flux per RC (TRo/RC) (Figure 6 and Supplementary Figure 2D), and electron transport flux per RC (ETo/RC) (Figure 6 and Supplementary Figure 2E) of plants grown with 10 mM N had a higher level or were similar to the levels of those grown with 2 or 15 mM N under control and salt conditions. On the contrary, dissipated energy flux per RC (DIo/RC) had a lower level under 10 mM N compared with other N levels under control and salt conditions (Figures 6E,F and Supplementary Figure 2F).
Quality-Related Traits Affected by Nitrogen Levels Under High Salt Stress
Salt had a significant effect on crude fat, crude fiber, and crude ash content, while N had a significant effect on crude fiber and crude ash content, but not crude fat. The interactive effect of N by salt was significant for all quality-related traits detected (Supplementary Table 5). Without salt treatment, the increased N application significantly decreased the crude fat content. The crude fat content had the highest value when plants were grown under 2 mM N compared with 10 or 15 mM N. After 200 mM NaCl exposure, the crude fat content significantly declined under 2 mM N, while the crude fat content was not obviously affected under 10 or 15 mM N (Figure 7A). Under 0 mM NaCl condition, the crude fiber content had the lowest value under 10 mM N. When exposed to NaCl, the crude fiber content of plants grown under 10 mM N was higher than those grown under 2 mM N. However, the value showed no significant difference with plants grown under 15 mM N (Figure 7B). Moreover, the ash content had the lowest value when plants were grown with 10 mM N under both control and salt treatment conditions (Figure 7C).
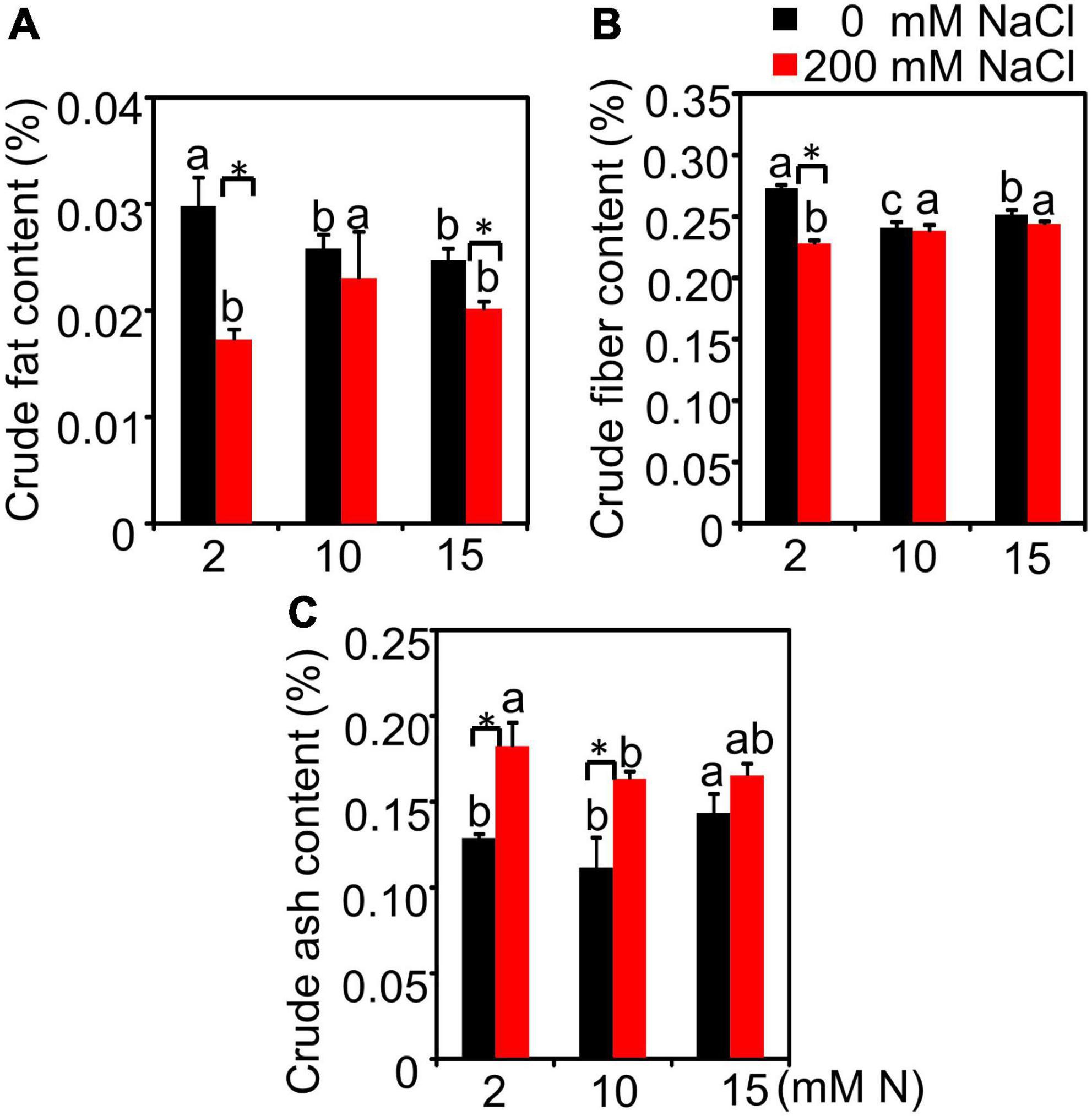
Figure 7. The quality-related traits of bermudagrass under different treatments. Crude fat content (A), crude fiber content (B), and crude ash content (C) in the leaves of plants grown with different nitrogen concentrations (2, 10, and 15 mM N) exposed to different NaCl levels (0 and 200 mM NaCl), respectively. Different letters above the columns indicate statistically significant differences at P < 0.05 under different N levels with the same NaCl level by Tukey’s test. * Shows significant differences (P < 0.05) under 200 mM NaCl treatment and 0 mM NaCl condition with the same N level by Tukey’s test.
Discussion
Salt-induced inhibition, such as ion toxicity, osmotic effects, and nutrient imbalance, can be alleviated by the moderate use of N fertilizer (Chen et al., 2010; Fan et al., 2013; Duan and Chang, 2017). In forage, such as pasture grass, moderate N application has a positive effect on plant growth and yield production (Irshad et al., 2009; Shao et al., 2020). Due to possible reduction in the N requirement under higher salt stress in forage bermudagrass (10 mM N under high salt stress compared with 15 mM N under control condition) according to the plant growth rate, Na+ content, and antioxidant enzyme activity alteration, we mainly focused on exploring the underlying mitigation mechanism after moderate N application under high salt stress.
Salt stress can affect N uptake in plants (Murtaza et al., 2013). The ammonium N (NH4+) transported by AMT can be directly assimilated by plants (Giagnoni et al., 2016). In forage bermudagrass, after a high level of NaCl exposure, the expression of AMT had the highest value when plants were grown with 10 mM N compared with other N levels (Figure 5B). Previous studies reported that AMT gene expression was downregulated by salt in Brassica juncea (Goel and Singh, 2015), while most AMTs were upregulated in the moderate salt-treated roots of Populus simonii (Zhang et al., 2014). The different expression alteration of AMTs in different species might be due to the differential interaction of N and salt levels. In forage bermudagrass, N application could promote the expression of AMT within a certain range, suggesting that excessive NH4+ application had no positive effect on N absorption by upregulating the AMT expression under high salt conditions. Moreover, moderate N application without excess under high salt stress could help forage bermudagrass maintain or promote the expression level of N assimilation-related enzymes, such as NR, GS, and GOGAT, which can be affected by salt (Wang et al., 2012; Parul et al., 2015; Figure 5). Moreover, the green leaves N content also had the highest value when plants were supplied with 10 mM N, but not 15 mM N (Figures 4A,B) after NaCl exposure, which was consistent with the trend of N metabolism-related gene expression. Besides, the highest value of green leaves N content and lowest value of stems N content supplied with 10 mM N suggested that the moderate N application might promote the N accumulation in green leaves and reduce the residual N in stems or old leaves, which played a critical role in the rational distribution of N under salt stress.
N is a structural element of chlorophyll, affecting the formation of chloroplasts and the accumulation of chlorophyll in plants (Xu et al., 2012). In this study, N application significantly increased the chlorophyll a and total chlorophyll content of bermudagrass (Figures 6A,B and Supplementary Table 1). However, the chlorophyll content could not increase in proportion when N was applied over 10 mM, which was consistent with previous studies (Zhang et al., 2013; Yang et al., 2015; Shao et al., 2020). N deficiencies can also decrease leaf area and intensity of photosynthesis (Zhang et al., 2013). Previous studies reported that N application significantly improved physiological parameters and elevated the photosynthetic capacity of leaves in some plant species (Siddiqui et al., 2010; Zhang et al., 2013). However, other studies showed that the promotion of N application on photosynthetic characteristics might also have concentration effects. For instance, N fertilizer can improve the photosynthetic characteristics of soybeans and ryegrass, but their effects were gradually inhibited or decreased with the increase in N fertilization application level (Zhang et al., 2013; Shao et al., 2020). In our study, the chlorophyll fluorescence intensity of bermudagrass leaves grown under moderate N (10 mM) was highest compared with 15 or 2 mM N, especially under high salt conditions (Figure 6). Under salt stress, excessive application of N could not promote but weaken the fluorescent transients of bermudagrass, especially the J (2 ms) and P (30 ms) steps, suggesting that N over application under salt stress might lead to the photosynthetic electron transport traffic jam, especially beyond QA– (Figure 6). Moreover, the optimum amount of N might promote primary photochemical reactions of PSII under high salt stress. The PIABS and PICS value, which could accurately reflect the state of plant photosynthetic apparatus, peaked under 10 mM N (Figure 6E), indicating that N could promote the primary photochemical reactions of PSII in the waterside. Changes in various quantum efficiencies per reaction center reflected the elevated absorption and transformation of light energy and the reduction in heat dissipation of leaves after appropriate N application (Figures 6E,F; Yusuf et al., 2010).
For forage bermudagrass, the yield and forage quality maintenance should be taken into consideration when evaluating the optimal N application under salt stress. We noticed that different N application levels had no significantly different effects on biomass under NaCl treatment, which is consistent with the previous study in other plants (Jia et al., 2017). However, the plant height had a lower value when plants were grown with 10 mM N compared with 15 mM N. The reason might be because those effectors contributing to the biomass, such as the decrease in firing rate (Figure 1D), might make up for the possible biomass reduction. Nitrogen application has critical effects on forage quality traits (e.g., crude protein, crude fat, and crude fiber) (Collins, 1991). In this study, under salt stress, when 10 mM N was added, the crude protein of green leaves had the highest value compared with 15 mM N (Figure 4D). The similar tendency of N content and crude protein content suggested that N might be redistributed among different tissues under salt stress and more N might be transferred into the green leaves to synthesize crude protein after being supplied with moderate N in bermudagrass. The constituents of crude fiber and crude cash are both the most remarkable factors to evaluate forage quality, which can limit forage intake and digestibility (Bruun et al., 2010; Shaer, 2010; Wolf et al., 2012). In this study, 10 mM N application could maintain crude fiber content at a lower level with the lowest crude ash content under high salt conditions, suggesting better maintenance of forage quality when supplied with moderate N.
Together with the previous studies, N requirements for plants in a saline environment might be inconsistent with those in the normal environment and salt stress might lead to the reduction in nutrients absorbed from the surrounding environment (Shenker et al., 2003; Ramos et al., 2012; Zhang et al., 2012). For forage bermudagrass, the optimal N application under salt stress should be considered in terms of plant growth, salt-stress-responsive physiological parameters, and nutrient value maintenance. Moderate reduction in N application had the optimal effect on alleviating the damage of high salt stress to forage bermudagrass through regulating numerous interconnected physico-chemical activities. These results obtained in this study are important for the agricultural practice to guide rational N fertilization application rather than in excess on bermudagrass, especially under severe salinity environment to reduce N leaching and environmental burden.
Data Availability Statement
The original contributions presented in the study are included in the article/Supplementary Material, further inquiries can be directed to the corresponding author/s.
Author Contributions
AS and HW performed the experimental design and data analysis. AS wrote the first draft of the manuscript. XX and XL performed the experiments. EA modified the manuscript draft. JF supervised all the experiments. All authors contributed to seen and the study conception and design and approved the manuscript.
Funding
This work was supported by the National Key R&D Program of China (2019YFD0900702) and the Agricultural Variety Improvement Project of Shandong (2019LZGC010).
Conflict of Interest
The authors declare that the research was conducted in the absence of any commercial or financial relationships that could be construed as a potential conflict of interest.
Publisher’s Note
All claims expressed in this article are solely those of the authors and do not necessarily represent those of their affiliated organizations, or those of the publisher, the editors and the reviewers. Any product that may be evaluated in this article, or claim that may be made by its manufacturer, is not guaranteed or endorsed by the publisher.
Acknowledgments
We thank the National Key R&D Program of China and the Agricultural Variety Improvement Project of Shandong for the financial support.
Supplementary Material
The Supplementary Material for this article can be found online at: https://www.frontiersin.org/articles/10.3389/fpls.2022.896358/full#supplementary-material
References
Adavi, Z., Razmjoo, K., and Mobli, M. (2006). Salinity tolerance of bermudagrass (Cynodon spp. L. C. Rich) cultivars and shoot Na, K and Cl concentration under a high saline environment. J. Hortic. Sci. Biotech. 81, 1074–1078. doi: 10.1080/14620316.2006.11512174
Ahanger, M. A., Aziz, U., Alsahli, A. A., Alyemeni, M. N., and Ahmad, P. (2019). Influence of exogenous salicylic acid and nitric oxide on growth, photosynthesis, and Ascorbate-Glutathione Cycle in salt stressed Vigna angularis. Biomolecules 10:42. doi: 10.3390/biom10010042
Ahmad, P., Tripathi, D. K., Deshmukh, R., Singh, V. P., and Corpas, F. J. (2019). Revisiting the role of ROS and RNS in plants under changing environment. Environ. Exp. Bot. 161, 1–3. doi: 10.1016/j.envexpbot.2019.02.017
Akram, M., Ashraf, M. Y., Jamil, M., Iqbal, R. M., Nafees, M., and Khan, M. A. (2011). Nitrogen application improves gas exchange characteristics and chlorophyll fluorescence in maize hybrids under salinity conditions. Russ. J. Plant Physl. 58, 394–401. doi: 10.1134/s1021443711030022
Amin, I., Rasool, S., Mir, M. A., Wani, W., Masoodi, K. Z., and Ahmad, P. (2021). Ion homeostasis for salinity tolerance in plants: a molecular approach. Physiol. Plant. 171, 578–594. doi: 10.1111/ppl.13185
Baby, J., and Jini, D. (2010). Insight into the role of antioxidant enzymes for salt tolerance in plants. Int. J. Plant Sci. 6, 456–464. doi: 10.3923/ijb.2010.456.464
Bruun, S., Jensen, J. W., Magid, J., Lindedam, J., and Engelsen, S. R. B. (2010). Prediction of the degradability and ash content of wheat straw from different cultivars using near infrared spectroscopy. Ind. Crop. Prod. 31, 321–326. doi: 10.1016/j.indcrop.2009.11.011
Bustin, S. A., Benes, V., Garson, J. A., Hellemans, J., Huggett, J., Kubista, M., et al. (2009). The MIQE guidelines: minimum information for publication of quantitative realtime PCR experiments. Clin. Chem. 55, 611–622. doi: 10.1373/clinchem.2008.112797
Chen, J. B., and Liu, J. X. (2012). Salinity tolerance evaluation and mechanisms in bermudagrass (Cynodon spp.). Acta Pratacul. Turae Sin. 21, 302–310.
Chen, J. B., Yan, J., Zhang, T. T., and Liu, J. X. (2008). Growth responses of four warm season turfgrasses to long-term salt stress. Acta Pratacul. Turae Sin. 17, 30–36.
Chen, W., Hou, Z., Wu, L., Liang, Y., and Wei, C. (2010). Effects of salinity and nitrogen on cotton growth in arid environment. Plant Soil 326, 61–73. doi: 10.13227/j.hjkx.201910137
Collins, M. (1991). Nitrogen effects on yield and forage quality of perennial ryegrass and tall fescue. Agron. J. 83, 588–595. doi: 10.2134/agronj1991.00021962008300030017x
Dechorgnat, J., Francis, K. L., Dhugga, K. S., Rafalski, J. A., Tyerman, S. D., and Kaiser, B. N. (2019). Tissue and nitrogen-linked expression profiles of ammonium and nitrate transporters in maize. BMC Plant Biol. 19:206. doi: 10.1186/s12870-019-1768-0
Deinlein, U., Stephan, A. B., Horie, T., Luo, W., Xu, G., and Schroeder, J. I. (2014). Plant salt-tolerance mechanisms. Trends Plant Sci. 19, 371–379.
Dluzniewska, P., Geßler, A., Dietrich, H., Schnitzler, J. P., and Rennenberg, H. (2007). Nitrogen uptake and metabolism in Populus × canescens as affected by salinity. New Phytol. 173, 279–293. doi: 10.1111/j.1469-8137.2006.01908.x
Duan, M., and Chang, S. X. (2017). Nitrogen fertilization improves the growth of lodgepole pine and white spruce seedlings under low salt stress through enhancing photosynthesis and plant nutrition. For. Ecol. Manag. 404, 197–204. doi: 10.1016/j.foreco.2017.08.045
Dudeck, A. E., Singh, S., Giordano, C. E., and Nell, T. (1983). Effects of sodium chloride on Cynodon turfgrasses. Agron. J. 75, 927–930. doi: 10.2134/agronj1983.00021962007500060017x
Esmaili, E., Kapourchal, S., Malakouti, M., and Homaee, M. (2005). Interactive effect of salinity and two nitrogen fertilizers on growth and composition of sorghum. Plant Soil Environ. 54, 537–546. doi: 10.17221/425-pse
Fan, H., Meng, G. H., Cheng, R. R., Li, L., Yun, X., and Wang, B. S. (2013). Effect of different nitrogen levels on the photosynthesis and growth of sweet Sorghum seedlings under salt stress. Adv. Mat. Res. 72, 4352–4357. doi: 10.4028/www.scientific.net/amr.726-731.4352
Fu, J., and Huang, B. (2001). Involvement of antioxidant and lipid peroxidation in the adaptation of two cool-season grasses to localized drought stress. Environ. Exp. Bot. 45, 105–114. doi: 10.1016/s0098-8472(00)00084-8
Giagnoni, L., Pastorelli, R., Mocali, S., Arenella, M., and Renella, G. (2016). Availability of different nitrogen forms changes the microbial communities and enzyme activities in the rhizosphere of maize lines with different nitrogen use efficiency. Appl. Soil Ecol. 98, 30–38. doi: 10.1016/j.apsoil.2015.09.004
Goel, P., and Singh, A. K. (2015). Abiotic stresses downregulate key genes involved in nitrogen uptake and assimilation in Brassica juncea L. PLoS One 10:e0143645.
Hoai, N. T. T., Shim, I. S., Kobayashi, K., and Kenji, U. (2003). Accumulation of some nitrogen compounds in response to salt stress and their relationships with salt tolerance in rice (Oryza sativa L.) seedlings. Plant Growth Regul. 41, 159–164.
Homaee, M., Feddes, R. A., and Dirksen, C. (2002). A macroscopic water extraction model for nonuniform transient salinity and water stress. Soil Sci. Soc. Am. J. 66, 1764–1772. doi: 10.2136/sssaj2002.1764
Hu, T., Hu, L., Zhang, X., Zhang, P., Zhao, Z., and Fu, J. (2013). Differential responses of CO2 assimilation, carbohydrate allocation and gene expression to NaCl stress in perennial ryegrass with different salt tolerance. PLoS One 8:e66090. doi: 10.1371/journal.pone.0066090
International Standard Organization (2000). Animal Feeding Stuffs – Determination of Crude Fiber Content. Method With Intermediate Filtration. ISO 6865:2000. Geneva: ISA.
Irshad, M., Eneji, A. E., Khattak, R. A., and Khan, A. (2009). Influence of nitrogen and saline water on the growth and partitioning of mineral content in maize. J. Plant Nutr. 32, 458–469. doi: 10.1080/01904160802660768
Jia, J., Huang, C., Bai, J., Zhang, G., Zhao, Q., and Wen, X. (2017). Effects of drought and salt stresses on growth characteristics of euhalophyte Suaeda salsa in coastal wetlands. Phys. Chem. Earth. 103, 68–74. doi: 10.1016/j.pce.2017.01.002
Khan, M. G., and Srivastava, H. S. (1998). Changes in growth and nitrogen assimilation in maize plants induced by NaCl and growth regulators. Biol. Plant. 41, 93–99. doi: 10.1023/a:1001768601359
Kishorekumar, R., Bulle, M., Wany, A., and Gupta, K. J. (2020). “An overview of important enzymes involved in nitrogen assimilation of plants,” in Methods in Molecular Biology, Vol. 2057, ed. K. Gupta (New York, NY: Humana). doi: 10.1007/978-1-4939-9790-9_1
Lacerda, C. F., Ferreira, J. F. S., Liu, X., and Suarez, D. L. (2016). Evapotranspiration as a criterion to estimate nitrogen requirement of maize under salt stress. J. Agro. Crop Sci. 202, 192–202. doi: 10.1111/jac.12145
Maksup, S., Sengsai, S., Laosuntisuk, K., Asayot, J., and Pongprayoon, W. (2020). Physiological responses and the expression of cellulose and lignin associated genes in Napier grass hybrids exposed to salt stress. Acta Physiol. Plant. 42:109.
Mansour, M. M. F. (2000). Nitrogen containing compounds and adaptation of plants to salinity stress. Biol. Plant. 43, 491–500. doi: 10.1023/a:1002873531707
Marcum, K. B., and Pessarakli, M. (2006). Salinity tolerance and salt gland excretion efficiency of bermudagrass turf cultivar. Crop Sci. 46, 2571–2574. doi: 10.2135/cropsci2006.01.0027
Maas, E. V., and Hoffman, G. J. (1977). Crop salt tolerance–current assessment. J. Irrig. Drain. Div. 103, 115–134. doi: 10.1061/JRCEA4.0001137
Munns, R., and Tester, M. (2008). Mechanisms of salinity tolerance. Annu. Rev. Plant Biol. 59, 651–681.
Murtaza, B., Usman, Y., and Saqib, M. (2013). “Nitrogen-Use-Efficiency (NUE) in plants under NaCl stress,” in Salt stress in plants, eds P. Ahmad, M. M. Azooz, and M. N. V. Prasad (New York, NY: Springer), 415–437. doi: 10.1080/15592324.2017.1297000
Parul, G., Kumar, S. A., and Kumar, T. P. (2015). Abiotic stresses downregulate key genes involved in nitrogen uptake and assimilation in Brassica juncea L. PLoS One 10:e0143645.
Ramos, T. B., Šimùnek, J., Gonçalves, M. C., Martins, J. C., Prazeres, A., and Pereira, L. S. (2012). Two-dimensional modeling of water and nitrogen fate from sweet sorghum irrigated with fresh and blended saline waters. Agr. Water Manage. 111, 87–104. doi: 10.1016/j.agwat.2012.05.007
Shaer, H. M. E. (2010). Halophytes and salt-tolerant plants as potential forage for ruminants in the Near East region. Small Ruminant Res. 91, 3–12. doi: 10.1016/j.smallrumres.2010.01.010
Shao, A., Sun, Z. C., Fan, S. G., Xu, X., Wang, W., Amombo, E., et al. (2020). Moderately low nitrogen application mitigates the negative effects of salt stress on annual ryegrass seedlings. PeerJ 8:e10427. doi: 10.7717/peerj.10427
Shenker, M., Ben-Gal, A., and Shani, U. (2003). Sweet corn response to combined nitrogen and salinity environmental stresses. Plant Soil 256, 139–147. doi: 10.1023/a:1026274015858
Siddiqui, M. H., Mohammad, F., Khan, M. N., Ha-Whaibi, M., and Bahkali, A. (2010). Nitrogen in relation to photosynthetic capacity and accumulation of osmoprotectant and nutrients in Brassica genotypes grown under salt stress. Agr. Sci. China 9, 671–680. doi: 10.1016/s1671-2927(09)60142-5
Singh, M., Singh, V. P., and Prasad, S. M. (2016). Responses of photosynthesis, nitrogen and proline metabolism to salinity stress in Solanum lycopersicum under different levels of nitrogen supplementation. Plant Physiol. Bioch. 109, 72–83. doi: 10.1016/j.plaphy.2016.08.021
Song, J., Ding, X., Feng, G., and Zhang, F. (2006). Nutritional and osmotic roles of nitrate in a euhalophyte and a xerophyte in saline conditions. New Phytol. 171, 357–366. doi: 10.1111/j.1469-8137.2006.01748.x
Soussi, M., Ocaña, A., and Lluch, C. (1998). Effects of salt stress on growth, photosynthesis and nitrogen fixation in chick-pea (L.). J. Exp. Bot. 49, 1329–1337. doi: 10.1093/jxb/49.325.1329
Sudmalis, D., Silva, P., Temmink, H., Bijmans, M. M., and Pereira, M. A. (2018). Biological treatment of produced water coupled with recovery of neutral lipids. Water Res. 147, 33–42. doi: 10.1016/j.watres.2018.09.050
Teh, C. Y., Shaharuddin, N. A., Ho, C. L., and Mahmood, M. (2016). Exogenous proline significantly affects the plant growth and nitrogen assimilation enzymes activities in rice (Oryza sativa) under salt stress. Acta. Physiol. Plant 38, 1–10.
Vandesompele, J., Preter, K. D., Pattyn, F., Poppe, B., Roy, N. V., Paepe, A. D., et al. (2002). Accurate normalization of real-time quantitative RT-PCR data by geometric averaging of multiple internal control genes. Genome Biol. 3:research0034.1. doi: 10.1186/gb-2002-3-7-research0034
Villa, M., Ulery, A., Catalan-Valencia, E., and Remmenga, M. (2003). Salinity and nitrogen rate effects on the growth and yield of Chile Pepper plants. Soil Sci. Soc. Amer. J. 6, 1781–1789. doi: 10.2136/sssaj2003.1781
Wang, H., Zhang, M., Guo, R., Shi, D., Liu, B., Lin, X., et al. (2012). Effects of salt stress on ion balance and nitrogen metabolism of old and young leaves in rice (Oryza sativa L.). BMC Plant Biol. 12:194. doi: 10.1186/1471-2229-12-194
Wolf, S., Hématy, K., and Höfte, H. (2012). Growth control and cell wall signaling in plants. Annu. Rev. Plant Biol. 63, 381–407. doi: 10.1146/annurev-arplant-042811-105449
Xu, G., Fan, X., and Miller, A. J. (2012). Plant nitrogen assimilation and use efficiency. Annu. Revi Plant Biol. 63, 153–182. doi: 10.1146/annurev-arplant-042811-105532
Yang, J., Shi, S., Gong, W., Du, L., Ma, Y. Y., Zhu, B., et al. (2015). Application of fluorescence spectrum to precisely inverse paddy rice nitrogen content. Plant Soil Environ. 61, 182–188. doi: 10.17221/7/2015-pse
Yusuf, M. A., Kumar, D., Rajwanshi, R., Strasser, R. J., Tsimilli-Michael, M., and Govindjee, et al. (2010). Overexpression of γ- tocopherol methyl transferase gene in transgenic Brassica juncea plants alleviates abiotic stress: physiological and chlorophyll a fluorescence measurements. Biochim. Biophys. Acta 1797, 1428–1438. doi: 10.1016/j.bbabio.2010.02.002
Zhang, C., Meng, S., Li, Y., and Zhao, Z. (2014). Net NH4+ and NO3– fluxes, and expression of NH4+ and NO3– transporter genes in roots of Populus simonii after acclimation to moderate salinity. Trees 28, 1813–1821. doi: 10.1007/s00468-014-1088-9
Zhang, D., Li, W., Xin, C., Tang, W., Eneji, A. E., and Dong, H. (2012). Lint yield and nitrogen use efficiency of field-grown cotton vary with soil salinity and nitrogen application rate. Field Crop. Res. 138, 63–70. doi: 10.1016/j.fcr.2012.09.013
Zhang, X., Huang, G., Bian, X., and Zhao, Q. (2013). Effects of root interaction and nitrogen fertilization on the chlorophyll content, root activity, photosynthetic characteristics of intercropped soybean and microbial quantity in the rhizosphere. Plant Soil Environ. 59, 80–88. doi: 10.17221/613/2012-pse
Zhao, C., Zhang, H., Song, C., Zhu, J. K., and Shabala, S. (2020). Mechanisms of plant responses and adaptation to soil salinity. Innovation 1:100017. doi: 10.1016/j.xinn.2020.100017
Keywords: forage bermudagrass, salt stress, nitrogen application, physiological response, forage quality
Citation: Shao A, Wang H, Xu X, Li X, Amombo E and Fu J (2022) Moderately Reducing Nitrogen Application Ameliorates Salt-Induced Growth and Physiological Damage on Forage Bermudagrass. Front. Plant Sci. 13:896358. doi: 10.3389/fpls.2022.896358
Received: 15 March 2022; Accepted: 04 April 2022;
Published: 29 April 2022.
Edited by:
Jin-Lin Zhang, Lanzhou University, ChinaReviewed by:
Jingjin Yu, Nanjing Agricultural University, ChinaJuanjuan Sun, Institute of Grassland Research (CAAS), China
Copyright © 2022 Shao, Wang, Xu, Li, Amombo and Fu. This is an open-access article distributed under the terms of the Creative Commons Attribution License (CC BY). The use, distribution or reproduction in other forums is permitted, provided the original author(s) and the copyright owner(s) are credited and that the original publication in this journal is cited, in accordance with accepted academic practice. No use, distribution or reproduction is permitted which does not comply with these terms.
*Correspondence: Jinmin Fu, dHVyZmNuQHFxLmNvbQ==
†These authors have contributed equally to this work