- 1School of Life Sciences, Yunnan Normal University, Kunming, China
- 2Engineering Research Center of Sustainable Development and Utilization of Biomass Energy, Ministry of Education, Kunming, China
- 3Key Laboratory of Biomass Energy and Environmental Biotechnology, Yunnan Normal University, Kunming, China
Sulfur dioxide (SO2) has long been viewed as toxic gas and air pollutant, but now is being verified as a signaling molecule in mammalian cells. SO2 can be endogenously produced and rapidly transformed into sulfur-containing compounds (e.g., hydrogen sulfide, cysteine, methionine, glutathione, glucosinolate, and phytochelatin) to maintain its homeostasis in plant cells. Exogenous application of SO2 in the form of gas or solution can trigger the expression of thousands of genes. The physiological functions of these genes are involved in the antioxidant defense, osmotic adjustment, and synthesis of stress proteins, secondary metabolites, and plant hormones, thus modulating numerous plant physiological processes. The modulated physiological processes by SO2 are implicated in seed germination, stomatal action, postharvest physiology, and plant response to environmental stresses. However, the review on the signaling role of SO2 in plants is little. In this review, the anabolism and catabolism of SO2 in plants were summarized. In addition, the signaling role of SO2 in seed germination, stomatal movement, fruit fresh-keeping, and plant response to environmental stresses (including drought, cold, heavy metal, and pathogen stresses) was discussed. Finally, the research direction of SO2 in plants is also proposed.
Introduction
For so long, sulfur dioxide (SO2) has been viewed as a harmful gas and air pollutant. SO2 can dissolve in water and form sulfurous acid (H2SO3), which in turn dissociates into sulfite ( ), bisulfite ( ), and hydrogen ion (H+). In neutral solution, the ratio of / is 3/1 (M/M; Singh et al., 2012). Therefore, the toxicity of SO2 mainly roots in three derivants, that is, , , and H+. and are strong nucleophiles, which can deleteriously react with the sulfhydryl groups (–SH)-containing proteins (including enzymes) and then alter their functions and activities, thus disturbing physiological and biochemical metabolism (e.g., photosynthesis, respiration, and ion balance), and even leading to cell death. H+ can lower the pH value of the cells and their compartmentations, followed by affecting the activities of the enzymes and interfering with the cellular metabolism (Huang et al., 2021). In addition, SO2 can induce the accumulation of reactive oxygen species (ROS) by oxidizing sulfite into sulfate and/or activating NADPH oxidase (NOX), which in turn cause biomembrane damage, protein disintegration, DNA damage, chromosome aberration, gene mutation, Golgi body destruction, and programmed cell death (PCD) (Okpodu et al., 1996). Therefore, high concentration of SO2 can damage plant at morphological, physiological, biochemical, and molecular levels. For example, SO2 can reduce photosynthesis by disrupting thylakoid function, interfering with electron transport chain and membrane permeability, destroying pigments, and affecting carbon allocation, leads to leaf damage (e.g., yellow spots, discoloration, and necrosis), growth inhibition, and even plant death (Lee et al., 2017; Huang et al., 2021; Li and Yi, 2022).
SO2, as a signaling molecule, can endogenously produce by the catalysis of aspartate aminotransferase (AAT) and NOX using hydrogen sulfide (H2S) as substrate in animal system (Huang et al., 2021). Endogenous SO2 executes a positive role in reducing brain injury, lung injury, myocardial injury, and hypertension; regulating vascular remodeling, myocardial remodeling, collagen remodeling, ion channels, cell proliferation, endoplasmic reticulum stress, and protein posttranslational modification (mainly sulfenylation by H2O2). Therefore, the abnormal production of endogenous SO2 commonly leads to colitis, hypertension, atherosclerosis, neuronal damage, vascular calcification, myocardial hypertrophy, myocardial injury, pulmonary hypertension, and acute lung injury (Huang et al., 2021). These studies indicate that SO2, as signaling molecule, plays an essential role in many physiological and pathological processes.
In general, signaling molecules, such as calcium ion (Ca2+), ROS, nitric oxide (NO), and H2S, exhibit the common characteristics: small molecule, fast dispersal, dual effects, controllability of generation and elimination, biological activity, reprogramming gene expression, and so forth (Chen et al., 2011; Khan et al., 2019). Small molecules are easy to be quickly biosynthesized/released to initiate signaling, and then immediately eliminated to terminate signaling. In addition, signaling molecules can be rapidly spread from production sites to effect sites to exert their biological effects by their receptors/sensors. Dual effects of signaling molecules refer to their toxicity at high concentration and signaling role at low concentration. Therefore, they must be maintained homeostasis in cells (Khan et al., 2019). Finally, signaling molecules can specially bind to their receptors/sensors and then reprogram gene expression, further regulating cellular metabolism (Chen et al., 2011). It is quite clear that small molecule SO2 meets these criterions, indicating its signaling role in organisms.
In plants, SO2 can be generated in the form of during sulfate reduction (Li et al., 2020). Recently, exogenous application of SO2 in the form of gas (mg m−3) or solution (Na2SO3/NaHSO3, 3/1, M/M), at low concentrations, has been found to exert a positive role in seed germination (Wang et al., 2017; Sun et al., 2018; Guo et al., 2021), stomatal movement (Taylor et al., 1981; Wei et al., 2013; Hu et al., 2014; Yi et al., 2017), fruit fresh-keeping (Joradol et al., 2019; Zhang et al., 2022), and plant response to adverse environments (Hu et al., 2015; Zhu et al., 2015; Han et al., 2018, 2019; Xue and Yi, 2018; Li et al., 2021; Ma, 2021). These studies indicate that SO2 is emerging as a signaling molecule in plants. However, the review on SO2 signaling in plants is little summarized. In this review, SO2 homeostasis in plants and its signaling role in seed germination, stomatal action, fruit fresh-keeping, and plant response to environmental stress (including drought, cold, heavy metal, and pathogen infection) were concluded. The aim of this paper highlighted that SO2 is an emerging signaling molecule in plants.
Metabolism of SO2
Anabolism of SO2
Similar to other signaling molecules, SO2 homeostasis in plants is maintained by its production (i.e., anabolism) and elimination (i.e., catabolism; Figure 1). In plants, the endogenous production of SO2 mainly roots in the reduction of . absorbed by roots is activated by ATP sulfurylase (ATPS), producing adenosine 5′-phosphosulfate (APS), which is transformed into (main form of SO2 in solution) by APS reductase (APR) using glutathione (GSH) as reducing agent (Li et al., 2020). In addition, cysteine (Cys) can change into cysteine sulfinate (Cts) by the catalysis of cysteine dioxygenase (CDO), and then produce β-sulfinylpyruvate (SP), which automatically form SO2 without any enzyme catalysis and release pyruvate (Pyr; Yu et al., 2020; Huang et al., 2022). Also, SO2 can be released from hydrogen sulfide (H2S) and its derivant thiosulfate (TS) under the catalysis of NOX, sulfide oxidase (SO), and TS sulfurtransferase (TST), respectively (Li et al., 2009). Similarly, sulfur-containing amino acids (SAA) also can generate SO2 by the catalysis of amino acid oxidase (AAO; Rausch and Wachter, 2005). However, the detailed mechanisms of SO2-producing pathways in plant growth, development, and response to environmental stress need to be further dissected in the coming days.
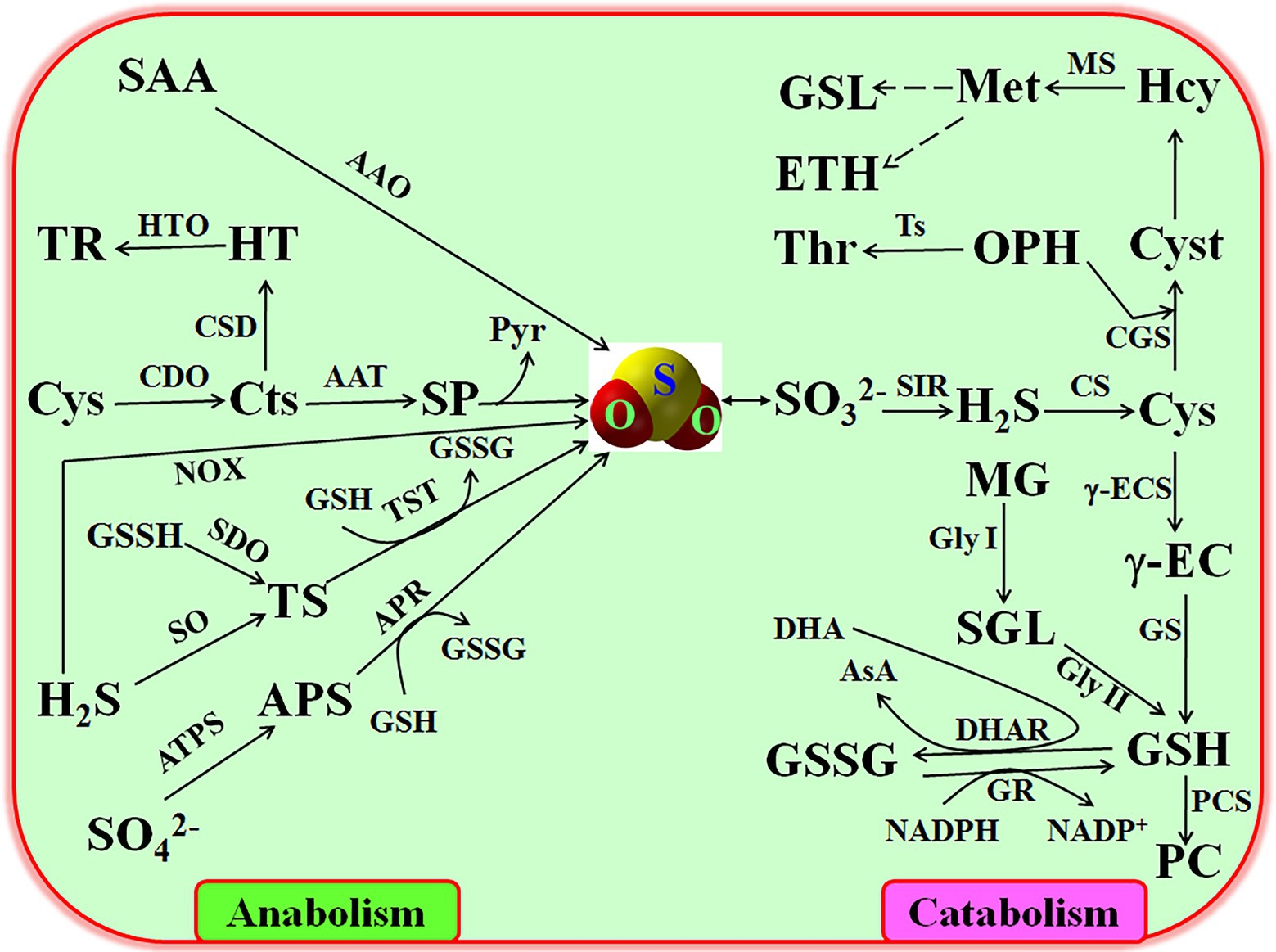
Figure 1. Anabolism and catabolism of sulfur dioxide (SO2) in plants. AAO, amino acid oxidase; APR, APS reductase; APS, adenosine 5′-phosphosulfate; ATPS, ATP sulfurylase; CDO, cysteine dioxygenase (CDO); CS, Cys synthetase; Cts, cysteine sulfinate; Cys, cysteine; Cyst, cystathionine; DHAR, dehydroascorbate reductase; γ-ECS, γ-glutamycysteine synthetase; Gly I, glyoxalase I; Gly II, glyoxalase II; GR, glutathione reductase; GS, GSH synthesis; GSH, glutathione; GSL, glucosinolate; GSSG, oxidized GSH; Hcy, homocystine; H2S, hydrogen sulfide; Met, methionine; MG, methylglyoxal; NOX, NADPH oxidase; OPH, O-phosphohomoserine; PC, phytochelatin; Pry, pyruvate; SAA, sulfur-containing amino acids; SGL, S-D-lactoylglutathione; SIR, sulfite reductase; SO, sulfide oxidase; SP, β-sulfinylpyruvate; Thr, threonine; Ts, threonine synthetase; TS, thiosulfate; TST, TS sulfurtransferase.
Catabolism of SO2
Analogue to its production, the scavenging of excessive SO2 is primarily achieved by the enzyme-catalysis pathways (Figure 1). Endogenous SO2 is easy to dissolve in water and produce , which is in turn reduced into H2S by reductase (SIR), followed by synthesizing Cys under the catalysis of Cys synthetase (CS; Hasanuzzaman et al., 2018; Li et al., 2020). Cys is a common precursor for biosynthesis of numerous biological molecules such as GSH, phytochelatin (PC), phytoalexins (PA), cystathionine (Cyst), homocystine (Hcy), methionine (Met), ethylene (ETH), and glucosinolate (GSL; Figure 1). GSH, as an important reducing agent in plant cells, which can be synthesized from Cys under the successive catalysis of γ-glutamycysteine synthetase (γ-ES) and GSH synthesis (GS; Yu et al., 2020; Huang et al., 2022). GSH and its oxidized form (GSSG) can mutually convert by dehydroascorbate reductase (DHAR) and glutathione reductase (GR) using dehydroascorbate and NADPH as electron acceptor and electron donor, respectively (Bartoli et al., 2017). Also, GSH can further synthesize PC to chelate heavy metal in plant cells, thus reducing the toxicity of heavy metal (Li et al., 2020). In addition to these, Cys can transform into other amino acids such as Cyst, Hcy, and Met (Li et al., 2009), further regulating the metabolism of amino acids in plants. These metabolic pathways are closely associated with the acquisition of stress tolerance in plants, also indicating the signaling role of SO2.
Signaling Role of SO2
Increasing evidences show that exogenous application of SO2 in a gas (mg m−3, fumigation) or solution (Na2SO3/NaHSO3, 3/1, irrigation) form could reprogramme the expression of thousands of genes. The physiological functions of these genes include antioxidant defense, osmotic adjustment, cell wall modification, and the synthesis of stress proteins (including heat shock proteins, HSP; and pathogen-related proteins, PR), secondary metabolites, and plant hormones (Li and Yi, 2012a,b, 2020; Zhao and Yi, 2014), which in turn modulated several physiological processes, such as seed germination, stomatal action, postharvest physiology, and plant response to environmental stresses, including drought, cold, heavy metal, and pathogen stresses (Table 1). In addition, the modulation of these physiological processes is involved in the interaction of SO2 and other signaling molecules, such as H2S, NO, ROS, cyclic guanosine monophosphate (cGMP), and plant hormones (Figure 2). In this section, the signaling role of SO2 in plants will be stated in detail.
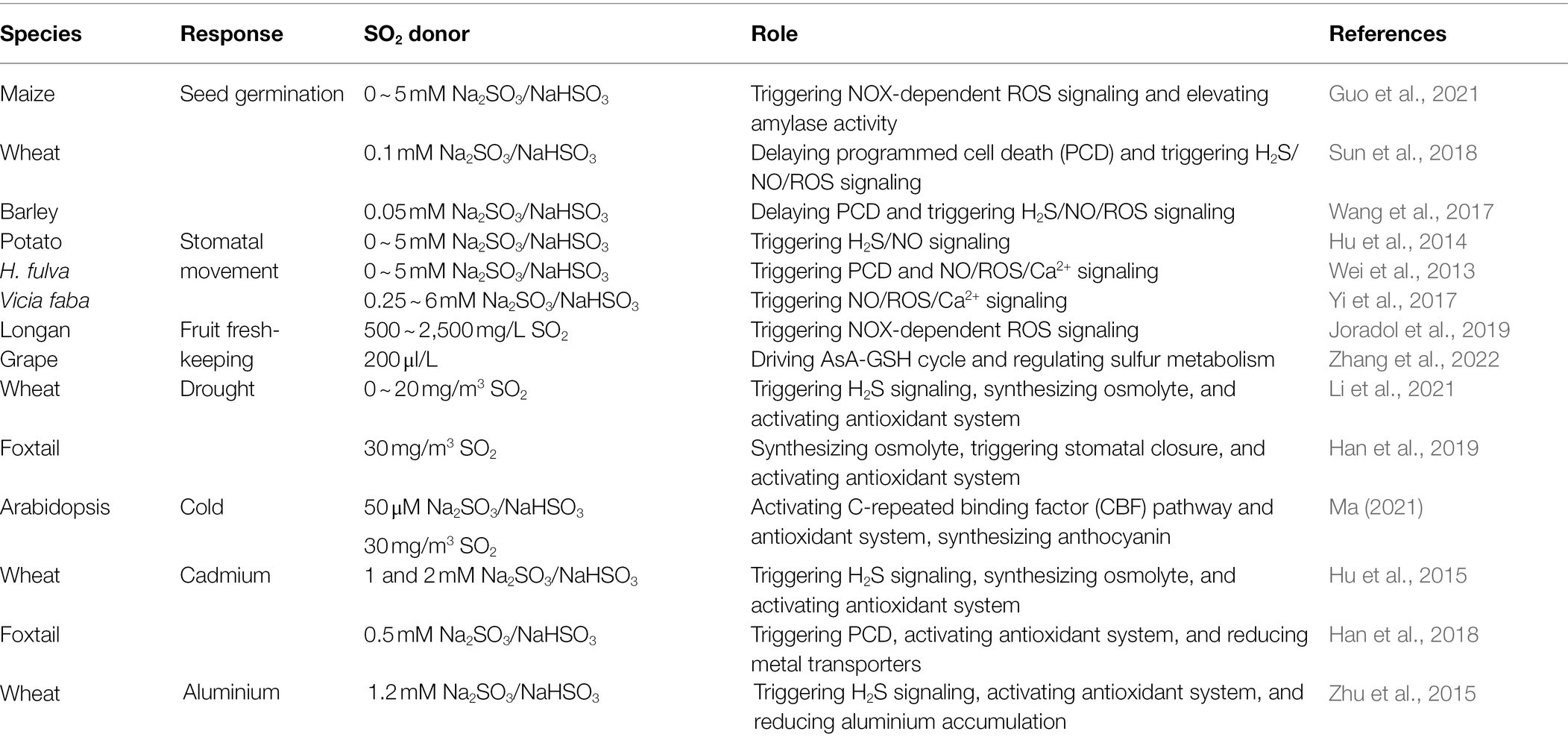
Table 1. Examples of the role of SO2 signaling in plant growth and response to environmental stress.
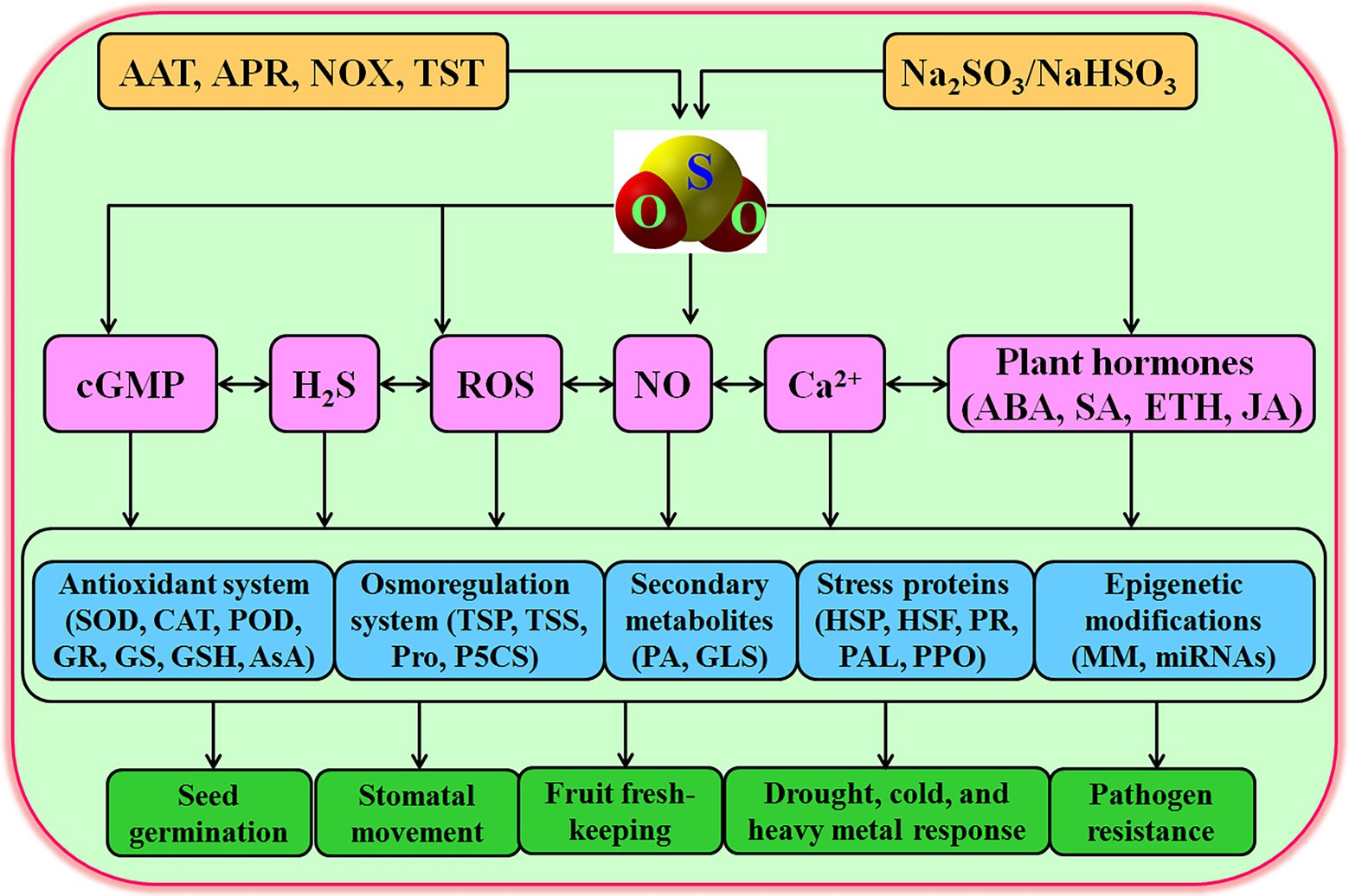
Figure 2. Signaling role of sulfur dioxide (SO2) in plants. AAT, aspartate aminotransferase; ABA, abscisic acid; APR, adenosine 5'-phosphosulfate reductase; AsA, ascorbic acid; CAT, catalase; cGMP, cyclic guanosine monophosphate; ETH, ethylene; GR, glutathione reductase; GS, glutathione synthetase; GSH, glutathione; GSL, glucosinolate; H2S, hydrogen sulfide; HSF, heat shock factor; HSP, heat shock proteins; JA, jasmonic acid; MM, methylation modification; NO, nitric oxide; NOX, NADPH oxidase; PA, phytoalexins; PAL, phenylalanine ammonia-lyase; P5CS, Δ1-pyrroline-5-carboxylate synthetase; POD, peroxidase; PPO, polyphenol oxidase; PR, pathogenesis-related proteins; Pro, proline; ROS, reactive oxygen species; SA, salicylic acid; SOD, superoxide dismutase; TSP, total soluble proteins; TSS, total soluble sugars; TST, thiosulfate sulfurtransferase.
Seed Germination
Seed germination is the first and key stage of plant life cycle, which is sensitive to environmental stress, especially soil environment stress. Therefore, seed germination is the basis for crop production and vegetation recovery. In general, seed priming, especially chemical priming, can improve seed vigor, seed germination, and seedling viability, as well as the resistance of seedlings to adverse environments (Paul et al., 2022). In maize seeds, pretreatment with SO2 (1 mM) facilitated seed germination and increased seed vigor. In addition, the SO2-pretreated germinating seeds had higher NOX activity and ROS level, while NOX inhibitor, diphenyleneiodinium, inhibited ROS accumulation and germination and vigor of maize seeds pretreated with SO2 (Guo et al., 2021). Also, SO2 pretreatment up-regulated the expression and activity of α-amylase in germinating maize seeds (Guo et al., 2021). These data imply that SO2 might function as signaling molecule in facilitating the germination of maize seeds by mobilizing reserves via activating NOX-dependent ROS production.
In wheat seeds, pretreatment with 100 μM SO2 donor (NaHSO3/Na2SO3, 1/3 M/M) postponed PCD, restrained the coalescence of small protein storage vacuoles, and reduced the accumulation of ROS (e.g., hydrogen peroxide, H2O2; and superoxide anion, O2–) in aleurone cells pretreated with gibberellin (Sun et al., 2018). In addition, SO2-pretreated germinating seeds, compared to treatment with gibberelin alone, sustained higher activities of catalase (CAT), guaiacol peroxidase (POD), ascorbate peroxidase (APX), and superoxide dismutase (SOD), while had lower activities of lipoxygenase (LOX) and polyphenol oxidase (PPO; Sun et al., 2018). Also, SO2-pretreated aleurone layers induced the production of endogenous H2S and NO, whereas the supplement of cPTIO (NO scavenger) accelerated PCD in both SO2– and H2S-pretreated aleurone cells (Sun et al., 2018). These results indicate that SO2 can trigger H2S/NO signaling to delay PCD in wheat aleurone layers pretreated with gibberellin via activating antioxidant enzyme system.
Similarly, in barley (Hordeum vulgare L.) seeds, pretreatment with SO2 donor (NaHSO3/Na2SO3, 50 μM) alleviated PCD induced by gibberelin in aleurone layers in a concentration-dependent fashion (Wang et al., 2017). Additionally, SO2 pretreatment increased the activities of SOD, CAT, APX, POD, and glutathione reductase (GR), while weakened that of LOX, reduced the levels of H2O2, O2–, and malondialdehyde (MDA) in aleurone layers (Wang et al., 2017). Furthermore, SO2 pretreatment triggered the accumulation of endogenous H2S and NO in aleurone layers (Wang et al., 2017), similar to the results reported by Sun et al. (2018) in wheat. These data suggest that SO2 might regulate the germination of barley seeds by attenuating PCD via the interaction among H2S, NO, and ROS signaling.
Stomatal Movement
Stomata is a major gate that plants exchange with carbon dioxide, oxygen, and water, its movement (i.e., opening and closure) influences photosynthesis, respiration, and transpiration, as well as plant resistance to environmental stress, especially drought stress (Jia and Zhang, 2008). Stomatal movement is strictly controlled by a signaling network composed of many second messengers, such as Ca2+, NO, ROS (mainly H2O2), H2S, and plant hormones (especially abscisic acid, ABA; ethylene, ETH; and cytokinin, CTK; Qi et al., 2018). Therefore, priming with signaling molecules can alter stomatal aperture and enhance the tolerance of plants to environmental stress. In sweet potato (Ipomoea batatas), treatment of epidermal strips with the different concentrations (0 ~ 5 mM) of Na2SO3/NaHSO3 solutions (SO2 donor) rapidly increased the levels of endogenous H2S and NO, and then induced stomatal closure in a dose-dependent manner (Hu et al., 2014). In addition, the stomatal closure induced by SO2 was reversed by hypotaurine (H2S scavenger) and cPTIO (NO scavenger; Hu et al., 2014), indicating that the SO2-triggered stomatal closure might be mediated by the H2S and NO signaling pathways. Also, in broad bean (Vicia faba L.), low concentrations (0.0001 ~ 0.1 μM) of sulfurous acid (H2SO3, as SO2 donor) facilitated stomatal opening by reducing the level of endogenous ABA to antagonize its action (Taylor et al., 1981). Adversely, a high concentration (10 μM) of H2SO3 inhibited stomatal opening by increasing the level of endogenous ABA (Taylor et al., 1981).
In Hemerocallis fulva, the epidermal strips treated with the different concentrations (1 ~ 5 mM) of SO2 donor (Na2SO3/NaHSO3) were found to reduce the guard cells vigor and induce cell death in a concentration-dependent manner (Wei et al., 2013). The SO2-induced death cells exhibited the features of apoptosis (i.e., nuclear condensation, nuclear elongation, and DNA fragmentation), indicating SO2 could trigger PCD in guard cells. Additionally, the levels of endogenous NO, ROS, and Ca2+ were increased by SO2, while reduced by NO scavenger (cPTIO), nitrate reductase (NO-producing enzyme) inhibitor (NaN3), ROS scavengers (ascorbic acid, AsA; and CAT), Ca2+ chelating agent (EGTA), and plasma membrane Ca2+ channel blocker (LaCl3), thus decreasing cell death (Wei et al., 2013). Also, AsA treatment decreased the levels of NO and Ca2+ compared with the SO2 treatment alone, whereas NaN3 treatment decreased ROS and Ca2+ levels, but LaCl3 treatment had no significant effect on NO and ROS levels (Wei et al., 2013). These data imply that SO2 could induce PCD in guard cells by intertwining NO, ROS, and Ca2+ signaling pathways in H. fulva, which might be a major cause for SO2-induced stomatal closure.
Similarly, in Vicia faba, SO2 hydrates (Na2SO3/NaHSO3) induced guard cell death in a dose-dependent fashion (ranging from 0.25 to 6 mM; Yi et al., 2017). Meanwhile, SO2 induced an increase in the level of endogenous NO, H2O2, and Ca2+ in guard cells of Vicia faba, similar to the data reported by Wei et al. (2013) in H. fulva. In addition, treatment with exogenous NO donor enhanced the toxicity of SO2, whereas NO scavenger (cPTIO) and synthesis inhibitors (L-NAME and tungstate) weakened SO2 toxicity (Yi et al., 2017). Likewise, the toxicity of SO2 was also blocked by ROS scavenger (AsA and CAT), Ca2+ chelating agent (EGTA), and Ca2+ channel inhibitor (LaCl3; Yi et al., 2017). Also, treatment with both cPTIO and AsA reversed SO2-induced increase in Ca2+ level in guard cells, while cPTIO and AsA treatment alone blocked SO2-induced H2O2 and NO accumulation (Yi et al., 2017). These data suggest that SO2 toxicity might be achieved by joint action of NO, ROS, and Ca2+ signaling in guard cells of Vicia faba, further supporting the hypothesis proposed by Wei et al. (2013). In addition to stomatal movement, Haworth et al. (2012) reported that SO2 could change stomatal density (SD), stomatal index (SI), and SD/SI ratio in Lepidozamia hopei, Lepidozamia peroffskyana, Ginkgo biloba, Nageia nagi, Podocarpus macrophyllus, Araucaria bidwillii, and Aagathis australis.
Fruit Fresh-Keeping
Shelf-life of the fruits and vegetables determines their quality and economic value. To lengthen the shelf-life, numerous physical and chemical methods are used in the process of fruits and vegetables storage (Joradol et al., 2019). The physical methods are involved in water, fertilizer, gas (e.g., oxygen and carbon dioxide), heat (temperature), and light; while chemical methods are mainly fresh-keeping agent treatments (Zhang et al., 2022). In Longan (Dimocarpus longan Lour. cv. Daw) fruits, SO2 fumigation (500 ~ 2,500 mg L−1) reduced pericarp browning, lengthened shelf-life, and improved the quality of fruits by enhancing antioxidant capacity compared with the control without SO2 fumigation (Joradol et al., 2019). In addition, the fruits fumigated with SO2 had a higher content of endogenous H2O2, which reached a maximum within 6 ~ 12 h. Also, SO2 treatment up-regulated the gene expression of NOX and SOD (Joradol et al., 2019). These data indicate that SO2 fumigation can induce the NOX-dependent H2O2 signaling, which in turn enhance the antioxidant capacity, thereby lengthening the shelf-life of fruits.
Similarly, in table grapes, SO2 treatment (200 μl L−1) inhibited fruit decay and reduced the levels of H2O2 and MDA compared to the control without SO2 treatment (Zhang et al., 2022). Additionally, SO2 treatment up-regulated the expression of VvSiR, VvSAT1, VvSAT2, and VvOASTL, which in turn increased the activities of sulfite reductase, serine acetyltransferase, and O-acetylserine (thiol)-lyase, as well as the content of Cys (Zhang et al., 2022). Likewise, the gene expression level, enzyme activity, and antioxidant content related to AsA-GSH cycle was enhanced by SO2 treatment in grapes. Also, the expression of VvGS (GSH synthetase) was up-regulated by SO2 treatment in table grapes, while the transcription level of VvHPCA1-4 and VvHPCA3 (evaluating the degree of oxidative damage) was down-regulated (Zhang et al., 2022). These data indicate that SO2 can lengthen the shelf-life of table grapes by maintaining H2O2 homeostasis to reduce postharvest oxidative damage via driving the AsA-GSH cycle.
Drought Response
Drought stress commonly leads to osmotic and oxidative stress due to the shortage of water and the overaccumulation of ROS in plant cells. The approaches alleviating osmotic and oxidative stresses can boost the resistance of plants to drought stress (Salehi-Lisar and Bakhshayeshan-Agdam, 2016). In wheat seedlings, under drought stress, pretreatment with SO2 (0 ~ 20 mg m−3) improved the survival percentage and relative water content (Li et al., 2021), indicating that SO2 could increase drought tolerance. The further experiments showed that SO2 pretreatment improved the content of proline (Pro) and activities of SOD and POD, which in turn reduced the accumulation of H2O2 and MDA in drought-treated wheat seedlings. In addition, SO2 pretreatment down-regulated the expression of TaNAC69 (transcription factor gene), while the expression of other transcription factor genes (TaERF1 and TaMYB30) insignificantly changed but maintained a higher levels in wheat seedlings under drought stress conditions (Li et al., 2021). Also, SO2 pretreatment induced an increase in H2S in wheat seedlings under drought stress, while H2S scavenger hypotaurine decreased the activities of SOD and POD, as well as the expression of transcription factor genes, followed by increasing the accumulation of H2O2 and MDA, returning to the level of drought treatment alone (Li et al., 2021). These data suggest that SO2 can increase the drought tolerance of wheat seedlings by accumulating osmolytes and activating antioxidant enzmyes via H2S signaling pathway.
In like manner, in foxtail millet seedlings, SO2 fumigation (30 mg m−3) decreased stomatal apertures and leaf transpiration rate, which in turn improved the relative water content in the leaves of drought-stressed seedlings (Han et al., 2019), thus improving the drought tolerance of seedlings. Additionally, SO2 pretreatment increased the activity of Δ1-pyrroline-5-carboxylate synthetase (P5CS), reduced that of Pro dehydrogenase (ProDH), and corresponding gene expression, followed by accumulating Pro in the leaves of drought-stressed seedlings (Han et al., 2019). Moreover, application of SO2 up-regulated the gene expression of CAT and POD and increased the activities of corresponding enzymes in the leaves of drought-stressed plants, which in turn alleviated drought-induced oxidative damage (i.e., decreasing MDA content) by scavenging H2O2 (Han et al., 2019). These results imply that SO2 fumigation can increase drought tolerance in foxtail millet seedlings by combined effect of stomatal closure, Pro accumulation, and antioxidant defense. Similarly, under drought stress, SO2 pre-exposure (30 mg m−3) increased SOD, POD, and O-acetylserine(thio)lyase (OASTL) activities and GSH, Cys, and nonprotein thiol (NPT) contents in Arabidopsis plants, which in turn alleviated oxidative stress (i.e., reducing H2O2 and MDA accumulation; Li and Yi, 2022). Meanwhile, SO2 increased Pro level by up-regulating gene expression and activity of P5CS and down-regulating that of ProDH, followed by reducing water loss, stomatal conductance, and transpiration rate, thus increasing net photosynthetic rate, water use efficiency, and photosynthetic pigment contents (Li and Yi, 2022), indicating that SO2 can improve the tolerance of Arabidopsis plants to drought stress.
Cold Response
Cold stress includes chilling (above freezing point, resulting in chilling injury) and freezing stress (below freezing point, leading to freezing injury). Both chilling and freezing stress can trigger osmotic and oxidative stresses (Ritonga and Chen, 2020). Therefore, the enhanced osmoregulation and antioxidant capacity by chemical priming are closely related with low temperature stress tolerance in plants. Presoaking of seeds with the different concentrations of (0, 10, 25, 50, and 100 μM) SO2 alleviated the cold-induced growth inhibition. Among concentrations, 50 μM SO2 presoaking was the most efficient (Ma, 2021). Similarly, pretreatment of Arabidopsis thaliana seeds with 50 μM SO2 boosted the cold resistance of A. thaliana seedlings. Otherwise, SO2 presoaking up-regulated the expression of AtCAT3 and AtPOD, and increased the activities of corresponding CAT and POD, which in turn reduced the level of endogenous H2O2 (Ma, 2021). Meanwhile, 50 μM SO2 pretreatment up-regulated the expression of genes related to anthocyanin synthesis (i.e., AtPAL2, AtCHS, AtCHI, and AtF3H), followed by promoting the synthesis of anthocyanin. Interestingly, SO2 pretreatment also activated the expression of genes (i.e., AtICE1, AtICE2, AtCBF1, AtCBF2, AtCBF3, AtCOR15a, and AtCOR15b) involved in C-repeated binding factor (CBF) signaling pathways, mainly cold-response signaling pathways (Ma, 2021).
Parallelly, SO2 (30 mg m−3) exposure reduced stomatal aperture and ROS accumulation by enhancing POD activity under cold stress (Ma, 2021). Also, SO2 exposure promoted Pro accumulation by increasing P5CS activity and lowering PDH activity as well as activated the gene expression of CBF signaling pathways (Ma, 2021), thus improving the cold adaptability of Arabidopsis thaliana. Similarly, exposure of Arabidopsis thaliana (Col-0) to the different concentrations of SO2 increased O2– generation rate and H2O2 content in Arabidopsis shoots, and up-regulated the gene expression of POD, SOD, and glutathione peroxidase (GPX), as well as increased the activity of corresponding enzymes and the content of GSH. Additionally, SO2 exposure increased isoenzymatic isoforms of SOD (FeSOD and Cu/ZnSOD) and POD, while decreased CAT isoforms (CAT2 and CAT3; Li and Yi, 2012b). Similarly, in Arabidopsis, SO2 exposure (30 mg m−3) down-regulated the expression of miR398, which in turn increased the transcript level of its target genes Cu/Zn-SOD (CSD1 and CSD2) and increased the activity of SOD (Li et al., 2017). Similarly, SO2 up-regulated the expression of miR395 expression, followed by decline in the transcript level of its target genes, ATP sulfurylases (APS3 and APS4) and sulfate transporter (SULTR2;1), implying miR398 and miR395 participate in the resistance of Arabidopsis plants to oxidative stress induced by SO2 exposure. These data suggest that SO2-induced ROS act as a signal to trigger plant defense response.
Heavy Metal Response
Heavy metals, such as cadmium (Cd), mercury, arsenic, and aluminum, have become the major pollutants with the development of modern agriculture and industry. Heavy metals can disturb cellular metabolism, inhibit seed germination and plant growth, and even influence on human health by entering into food chain (Ghori et al., 2019). Therefore, heavy metal pollutions have become a huge challenge for crop production, food safety, and human health. How to improve the resistance of crop plants to heavy metal stress and reduce its endogenous accumulation is an urgent issue. In wheat seeds, Cd-inhibited seed germination, while the inhibiting effects were alleviated by exogenous application of SO2 (Na2SO3/NaHSO3 (3/1) solution as SO2 donor) in a concentration-dependent manner and the optimal concentration was between 1 and 2 mM (Hu et al., 2015). Additionally, SO2 donor pretreatment enhanced the activities of amylase and esterase, which in turn resulted in the accumulation of total soluble sugars (TSS) and total soluble protein (TSP) in germinating seeds under Cd stress (Hu et al., 2015). Also, SO2 pretreatment reduced the overproduction of MDA, H2O2, and O2–, as well as the loss of plasma membrane integrity of the radicle tips of seedlings under Cd stress (Hu et al., 2015). Further experiment data showed that SO2 increased the activities of POD, APX, SOD, and CAT, lowered level of LOX in germinating wheat seeds (Hu et al., 2015). Interestingly, compared with the control, SO2 pretreatment increased the level of endogenous H2S in germinating wheat seeds (Hu et al., 2015). These data suggest that SO2 can promote the germination of wheat seeds under Cd stress by mobilizing reserves and activating antioxidant system via H2S signaling pathway.
In foxtail millet seedlings, application of SO2 derivatives (0.5 mM) reduced the Cd-inhibited seedling growth and Cd-induced oxidative damage (i.e., reducing MDA accumulation) in the leaves of seedlings (Han et al., 2018). Additionally, SO2 treatment enhanced the activities of CAT and SOD and drove AsA-GSH cycle, which in turn reduced the accumulation of Cd-elicited O2– and H2O2 in the leaves of seedlings (Han et al., 2018). Furthermore, SO2 application increased the contents of GSH and phytochelatin (PC) by promoting sulfur assimilation, followed by enhancing Cd-detoxification capacity in seedlings. Further experiments showed that SO2 derivatives down-regulated the expression of genes related to Cd uptake and translocation (i.e., NRAMP1, NRAMP6, IRT1, IRT2, HMA2, and HMA4; Han et al., 2018), thus reducing Cd accumulation in the shoots and roots of Cd-stressed seedlings.
Similarly, in wheat seeds, pretreatment with SO2 donor (NaHSO3/Na2SO3, 1/3) at 1.2 mM increased the activities of POD, CAT, and APX, and lowered that of LOX, and reduced the accumulation of O2–, H2O2, and MDA in germinating seeds under aluminum stress (Zhu et al., 2015). As expected, SO2 pretreatment increased the level of endogenous H2S in wheat seeds, while reduced the content of ROS, which in turn maintained the integrity of biomembrane and reduced aluminum accumulation in wheat seedling radicles (Zhu et al., 2015). Also, SO2 pretreatment down-regulated the expression of alumium-responsive genes (i.e., TaWali1, TaWali2, TaWali3, TaWali5, TaWali6, and TaALMT1) in seedling radicles under aluminum stress (Zhu et al., 2015). These data indicate that SO2 can improve seed germination of wheat under aluminum stress by enhancing antioxidant capacity and reducing the accumulation of aluminum via H2S signaling pathway.
Pathogen Response
Besides enhancing abiotic stress tolerance, exogenously applied SO2 also can improve the resistance of plants to biotic stress (Zhao and Yi, 2014). In Arabidopsis plants, SO2 pre-exposure (30 mg m−3) increased the resistance to Botrytis cinerea (Xue and Yi, 2018). Further experiments showed that SO2 pretreatment up-regulated the expression levels of the defense-related genes (PAL, PPO, PR2, and PR3), which encode phenylalanine ammonia-lyase (PAL), polyphenol oxidase (PPO), β-1,3-glucanase (BGL), and chitinase (CHI), and increased the activities of PAL, PPO, BGL, and CHI (Xue and Yi, 2018). Also, SO2 pre-exposure increased the transcript levels of microRNAs (MIR393, MIR160, and MIR167), correspondingly decreased the gene expression of their targets involved in the auxin signaling pathway. Adversely, the expression levels of the primary auxin-response genes (GH3-like, BDL/IAA12, and AXR3/IAA17) were down-regulated by SO2 pre-exposure in Arabidopsis plants (Xue and Yi, 2018). These data imply that SO2 increases the disease resistance of Arabidopsis plants to Botrytis cinerea by enhancing the defense-related gene expression and enzyme activity as well as suppressing the auxin signaling pathway mediated by miRNA.
In addition, Arabidopsis plants, the transcriptome analysis identified that SO2 fumigation (30 mg m−3) led to the change in the expression of 2,780 genes, the genes involved in biotic stress resistance, ROS production and scavenging, and sulfur assimilation were up-regulated (Zhao and Yi, 2014). Likewise, Li and Yi (2012b) reported that SO2 (30 mg m−3) treatment triggered the different expression of 2,780 genes, the functions of which were mainly involved in signal transduction, transcription regulation, molecular structure, transport, binding, and metabolism. Many different expression genes encoding antioxidant enzymes (POD, GPX, and SOD), heat shock proteins (HSP), pathogenesis-related (PR) proteins, and cytochrome P450 were up-regulated by SO2 in Arabidopsis shoots (Li and Yi, 2012b). In Arabidopsis, Li and Yi (2012a), using Affymetrix GeneChip technology, found that the expression of 494 genes was significantly changed by SO2 exposure (30 mg m−3), they encoded antioxidant enzymes (e.g., GST, POD, and thioredoxin), HSP, and PR, as well as were involved in the ETH signaling pathway, phenylpropanoid pathway, and cell wall modification. Similarly, SO2 exposure enhanced the production of ROS, as signaling molecule, increased the activities of SOD, POD, glutathione peroxidase (GPX), and GST in Arabidopsis plants (Li and Yi, 2012a). Also, in fresh table grapes (V. vinifera L. “Crimson Seedless”), transcriptomics approaches also found that SO2 treatment (140 μl L−1) up-regulated the expression of enzymes genes related to sulfur-metabolizing enzymes (especially directing towards chelation and conjugation), redox homeostasis, and plant hormones (e.g., auxin, AUX; ethylene, ETH; and jasmonic acid, JA) signaling pathways (Giraud et al., 2012). Generally, salicylic acid (SA) level is closely related to plant disease resistance. In Arabidopsis thaliana plants, Hao et al. (2011) found that the snc1 mutants (with high SA content) had higher tolerance to SO2 than nahG plants (with low SA content), implying that endogenous SA and signaling might play an essential role in plant responses to SO2 stress. These studies further support the fact that SO2 increases disease resistance in Arabidopsis plants.
Conclusion and Perspectives
Mounting evidences show that SO2 can not only regulate seed germination, stomatal movement, and postharvest physiology, but also plants respond to environmental stresses, such as drought, cold, and heavy metal, and pathogen stresses. Numerous studies identified that SO2 meets the requirements of signaling molecules in plants, emerging as a novel signaling role in many plant physiological processes. SO2 as a novel signaling molecule can exert its physiological functions either alone or interaction with other signaling molecules, such as Ca2+, H2O2, NO, H2S, cGMP, and plant hormones (e.g., ABA, SA, ETH, and JA; Figure 2). SO2 can enhance antioxidant system (e.g., SOD, CAT, POD, APX, GR, GSH, and AsA) and osmoregulation system (e.g., Pro, TTP, TSS, and P5CS), drive secondary metabolism (e.g., GLS and PA accumulation), synthesize stress proteins (e.g., HSP, HSF, PR, PAL, and PPO), and modulate epigenetic modifications (e.g., DNA methylation modification (MM) and miRNAs), thus regulating several plant physiological functions (Figure 2). Though, the signaling role of SO2 in plants has been verified in a large amount of physiological processes, numerous open questions still need to be further answered in more detail in the coming days. In mammalian cells, the AAT/SO2 pathway is contributed to SO2 signaling; however, in plants, besides the APR/ pathway (Figure 1), the detailed metabolic pathways of SO2 are waiting for being further expounded. Correspondingly, the knowledge on the exact concentrations of SO2 in plant cells and subcellular structures as well as its receptors/sensors remains to be found. Though SO2 could improve multiple stress tolerance, whether SO2 can induce the tolerance of plants to heat, salt, and flooding stresses and the underlying mechanisms requires to be further explored. In addition, the signaling interaction of SO2 with Ca2+, ROS, NO, H2S, methylglyoxal, and cyclic nucleotide in plants under both physiological and stress conditions is necessary to be uncovered. With the development of omics, the effects of SO2 on transcriptome, metabolome, proteome, and phenome in plants have to be settled urgently.
Author Contributions
Z-GL conceived, designed, and wrote the manuscript, while X-EL and H-YC wrote the anabolism and catabolism of SO2, respectively. All authors contributed to the article and approved the submitted version.
Funding
This research was funded by the National Natural Science Foundation of China (32160065 and 31760069).
Conflict of Interest
The authors declare that the research was conducted in the absence of any commercial or financial relationships that could be construed as a potential conflict of interest.
Publisher’s Note
All claims expressed in this article are solely those of the authors and do not necessarily represent those of their affiliated organizations, or those of the publisher, the editors and the reviewers. Any product that may be evaluated in this article, or claim that may be made by its manufacturer, is not guaranteed or endorsed by the publisher.
References
Bartoli, C. G., Buet, A., Gergoff Grozeff, G., Galatro, A., and Simontacchi, M. (2017). “Ascorbate-glutathione cycle and abiotic stress tolerance in plants” in Ascorbic Acid in Plant Growth, Development and Stress Tolerance. eds. M. Hossain, S. Munné-Bosch, D. Burritt, P. Diaz-Vivancos, M. Fujita, and A. Lorence (Cham: Springer), 177–200.
Chen, S. S., Tang, C. S., Jin, H. F., and Du, J. B. (2011). Sulfur dioxide acts as a novel endogenous gaseous signaling molecule in the cardiovascular system. Chin. Med. J. 124, 1901–1905. doi: 10.3760/cma.j.issn.0366-6999.2011.12.024
Ghori, N. H., Ghori, T., Hayat, M. Q., Imadi, S. R., Gul, A., Altay, V., et al. (2019). Heavy metal stress and responses in plants. Int. J. Environ. Sci. Technol. 16, 1807–1828. doi: 10.1007/s13762-019-02215-8
Giraud, E., Ivanova, A., Gordon, C. S., Whelan, J., and Considine, M. J. (2012). Sulphur dioxide evokes a large scale reprogramming of the grape berry transcriptome associated with oxidative signalling and biotic defence responses. Plant Cell Environ. 35, 405–417. doi: 10.1111/j.1365-3040.2011.02379.x
Guo, Z., Zhao, J., Wang, M., Song, S., and Xia, Z. (2021). Sulfur dioxide promotes seed germination by modulating reactive oxygen species production in maize. Plant Sci. 312:111027. doi: 10.1016/j.plantsci.2021.111027
Han, Y., Wu, M., Hao, L., and Yi, H. (2018). Sulfur dioxide derivatives alleviate cadmium toxicity by enhancing antioxidant defence and reducing Cd2+ uptake and translocation in foxtail millet seedlings. Ecotox. Environ. Safe. 157, 207–215. doi: 10.1016/j.ecoenv.2018.03.084
Han, Y., Yang, H., Wu, M., and Yi, H. (2019). Enhanced drought tolerance of foxtail millet seedlings by sulfur dioxide fumigation. Ecotox. Environ. Safe. 178, 9–16. doi: 10.1016/j.ecoenv.2019.04.006
Hao, L., Wang, Y., Xu, J., Feng, S. D., Ma, C. Y., Liu, C., et al. (2011). Role of endogenous salicylic acid in Arabidopsis response to elevated sulfur dioxide concentration. Biol. Plant. 55, 297–304. doi: 10.1007/s10535-011-0042-6
Hasanuzzaman, M., Hossain, M. S., Bhuyan, M. H. M. B., Mahmud, J. A., Nahar, K., and Fujita, M. (2018). “The role of sulfur in plant abiotic stress tolerance: molecular interactions and defense mechanisms,” in Plant Nutrients and Abiotic Stress Tolerance. eds. M. Hasanuzzaman, M. Fujita, H. Oku, K. Nahar, and B. Hawrylak-Nowak (Singapore: Springer), 221–252.
Haworth, M., Elliott-Kingston, C., Gallagher, A., Fitzgerald, A., and McElwain, J. C. (2012). Sulphur dioxide fumigation effects on stomatal density and index of non-resistant plants: implications for the stomatal palaeo-[CO2] proxy method. Rev. Palaeobot. Palynol. 182, 44–54. doi: 10.1016/j.revpalbo.2012.06.006
Hu, K. D., Bai, G. S., Li, W. J., Yan, H., Hu, L. Y., Li, Y. H., et al. (2015). Sulfur dioxide promotes germination and plays an antioxidant role in cadmium-stressed wheat seeds. Plant Growth Regul. 75, 271–280. doi: 10.1007/s10725-014-9951-7
Hu, K. D., Tang, J., Zhao, D. L., Hu, L. Y., Li, Y. H., Liu, Y. S., et al. (2014). Stomatal closure in sweet potato leaves induced by sulfur dioxide involves H2S and NO signaling pathways. Biol. Plant. 58, 676–680. doi: 10.1007/s10535-014-0440-7
Huang, Y., Zhang, H., Lv, B., Tang, C., Du, J., and Jin, H. (2021). Endogenous sulfur dioxide is a new gasotransmitter with promising therapeutic potential in cardiovascular system. Sci. Bull. 66, 1604–1607. doi: 10.1016/j.scib.2021.04.003
Huang, Y., Zhang, H., Lv, B., Tang, C., Du, J., and Jin, H. (2022). Sulfur dioxide: endogenous generation, biological effects, detection, and therapeutic potential. Antioxidant Redox Signal. 36, 256–274. doi: 10.1089/ars.2021.0213
Jia, W., and Zhang, J. (2008). Stomatal movements and long-distance signaling in plants. Plant signal. behave. 3, 772–777. doi: 10.4161/psb.3.10.6294
Joradol, A., Uthaibutra, J., Lithanatudom, P., and Saengnil, K. (2019). Induced expression of NOX and SOD by gaseous sulfur dioxide and chlorine dioxide enhances antioxidant capacity and maintains fruit quality of ‘Daw’ longan fruit during storage through H2O2 signaling. Postharv. Biol. Technol. 156:110938. doi: 10.1016/j.postharvbio.2019.110938
Khan, M. R., Reddy, P. S., Ferrante, A., and Khan, N. A. (2019). Plant Signaling Molecules: Role and Regulation under Stressful Environments. Cambridge: Elsevier.
Lee, H. K., Khaine, I., Kwak, M. J., Jang, J. H., Lee, T. Y., Lee, J. K., et al. (2017). The relationship between SO2 exposure and plant physiology: a mini review. Hortic. Environ. Biotechnol. 58, 523–529. doi: 10.1007/s13580-017-0053-0
Li, X., Bazer, F. W., Gao, H., Jobgen, W., Johnson, G. A., Li, P., et al. (2009). Amino acids and gaseous signaling. Amino Acids 37, 65–78. doi: 10.1007/s00726-009-0264-5
Li, Q., Gao, Y., and Yang, A. (2020). Sulfur homeostasis in plants. Int. J. Mol. Sci. 21:8926. doi: 10.3390/ijms21238926
Li, L., and Yi, H. (2012a). Differential expression of Arabidopsis defense-related genes in response to sulfur dioxide. Chemosphere 87, 718–724. doi: 10.1016/j.chemosphere.2011.12.064
Li, L., and Yi, H. (2012b). Effect of sulfur dioxide on ROS production, gene expression and antioxidant enzyme activity in Arabidopsis plants. Plant Physiol. Biochem. 58, 46–53. doi: 10.1016/j.plaphy.2012.06.009
Li, L., and Yi, H. (2020). Photosynthetic responses of Arabidopsis to SO2 were related to photosynthetic pigments, photosynthesis gene expression and redox regulation. Ecotoxicol. Environ. Saf. 203:111019. doi: 10.1016/j.ecoenv.2020.111019
Li, L., and Yi, H. (2022). Enhancement of drought tolerance in Arabidopsis plants induced by sulfur dioxide. Ecotoxicology. doi: 10.1007/s10646-022-02530-w [Epub ahead of print].
Li, L. H., Yi, H. L., Liu, X. P., and Qi, H. X. (2021). Sulfur dioxide enhance drought tolerance of wheat seedlings through H2S signaling. Ecotox. Environ. Safe. 207:111248. doi: 10.1016/j.ecoenv.2020.111248
Li, L., Yi, H., Xue, M., and Yi, M. (2017). miR398 and miR395 are involved in response to SO2 stress in Arabidopsis thaliana. Ecotoxicology 26, 1181–1187. doi: 10.1007/s10646-017-1843-y
Ma, L. (2021). Sulfur Dioxide Enhanced Cold Stress Adaptability of Arabidopsis Thaliana. Taiyuan: Shanxi University.
Okpodu, C. M., Alscher, R. G., Grabau, E. A., and Cramer, C. L. (1996). Physiological, biochemical and molecular effects of sulfur dioxide. J. Plant Physiol. 148, 309–316. doi: 10.1016/S0176-1617(96)80258-6
Paul, S., Dey, S., and Kundu, R. (2022). Seed priming: an emerging tool towards sustainable agriculture. Plant Growth Regul. doi: 10.1007/s10725-021-00761-1 ( in press).
Qi, J., Song, C. P., Wang, B., Zhou, J., Kangasjärvi, J., Zhu, J. K., et al. (2018). Reactive oxygen species signaling and stomatal movement in plant responses to drought stress and pathogen attack. J. Integr. Plant Biol. 60, 805–826. doi: 10.1111/jipb.12654
Rausch, T., and Wachter, A. (2005). Sulfur metabolism: a versatile platform for launching defence operations. Trends Plant Sci. 10, 503–509. doi: 10.1016/j.tplants.2005.08.006
Ritonga, F. N., and Chen, S. (2020). Physiological and molecular mechanism involved in cold stress tolerance in plants. Plan. Theory 9:560. doi: 10.3390/plants9050560
Salehi-Lisar, S. Y., and Bakhshayeshan-Agdam, H. (2016). “Drought stress in plants: causes, consequences, and tolerance” in Drought Stress Tolerance in Plants. eds. M. Hossain, S. Wani, S. Bhattacharjee, D. Burritt, and L. S. Tran (Cham: Springer), 1–16.
Singh, L. P., Gill, S. S., Gill, R., and Tuteja, N. (2012). “Mechanism of sulfur dioxide toxicity and tolerance in crop plants” in Improving Crop Resistance to Abiotic Stress. eds. N. Tuteja, S. S. Gill, A. F. Tiburcio, and R. Tuteja (Weinheim: Wiley), 133–163.
Sun, K. K., Zhu, D. B., Yao, G. F., and Zhang, H. (2018). Sulfur dioxide acts as an antioxidant and delays programmed cell death in wheat aleurone layers upstream of H2S and NO signaling pathways. Biol. Plant. 62, 809–816. doi: 10.1007/s10535-018-0796-1
Taylor, J. S., Reid, D. M., and Pharis, R. P. (1981). Mutual antagonism of sulfur dioxide and abscisic acid in their effect on stomatal aperture in broad bean (Vicia faba L.) epidermal strips. Plant Physiol. 68, 1504–1507. doi: 10.1104/pp.68.6.1504
Wang, S. S., Zhang, Y. X., Yang, F., Huang, Z. Q., Tang, J., Hu, K. D., et al. (2017). Sulfur dioxide alleviates programmed cell death in barley aleurone by acting as an antioxidant. PLoS One 12:e0188289. doi: 10.1371/journal.pone.0188289
Wei, A., Xin, X., Wang, Y., Zhang, C., and Cao, D. (2013). Signal regulation involved in sulfur dioxide-induced guard cell apoptosis in Hemerocallis fulva. Ecotox. Environ. Safe. 98, 41–45. doi: 10.1016/j.ecoenv.2013.09.029
Xue, M., and Yi, H. (2018). Enhanced Arabidopsis disease resistance against Botrytis cinerea induced by sulfur dioxide. Ecotox. Environ. Safe. 147, 523–529. doi: 10.1016/j.ecoenv.2017.09.011
Yi, M., Bai, H., Xue, M., and Yi, H. (2017). NO and H2O2 contribute to SO2 toxicity via Ca2+ signaling in Vicia faba guard cells. Environ. Sci. Pollut. Res. 24, 9437–9446. doi: 10.1007/s11356-017-8612-6
Yu, B., Yuan, Z., Yang, X., and Wang, B. (2020). Prodrugs of persulfides, sulfur dioxide, and carbon disulfide: important tools for studying sulfur signaling at various oxidation states. Antioxidant Redox Signal. 33, 1046–1059. doi: 10.1089/ars.2019.7880
Zhang, Z., Wu, Z., Yuan, Y., Zhang, J., Wei, J., and Wu, B. (2022). Sulfur dioxide mitigates oxidative damage by modulating hydrogen peroxide homeostasis in postharvest table grapes. Postharv. Biol. Technol. 188:111877. doi: 10.1016/j.postharvbio.2022.111877
Zhao, J., and Yi, H. (2014). Genome-wide transcriptome analysis of Arabidopsis response to sulfur dioxide fumigation. Mol. Gen. Genomics. 289, 989–999. doi: 10.1007/s00438-014-0870-0
Keywords: sulfur dioxide, signaling molecule, seed germination, fruit fresh-keeping, stomatal movement, stress response
Citation: Li Z-G, Li X-E and Chen H-Y (2022) Sulfur Dioxide: An Emerging Signaling Molecule in Plants. Front. Plant Sci. 13:891626. doi: 10.3389/fpls.2022.891626
Edited by:
Girdhar Kumar Pandey, University of Delhi, IndiaReviewed by:
Gaurav Zinta, Institute of Himalayan Bioresource Technology (CSIR), IndiaMohammad Yusuf, United Arab Emirates University, United Arab Emirates
Copyright © 2022 Li, Li and Chen. This is an open-access article distributed under the terms of the Creative Commons Attribution License (CC BY). The use, distribution or reproduction in other forums is permitted, provided the original author(s) and the copyright owner(s) are credited and that the original publication in this journal is cited, in accordance with accepted academic practice. No use, distribution or reproduction is permitted which does not comply with these terms.
*Correspondence: Zhong-Guang Li, emhvbmdndWFuZ19saUAxNjMuY29t