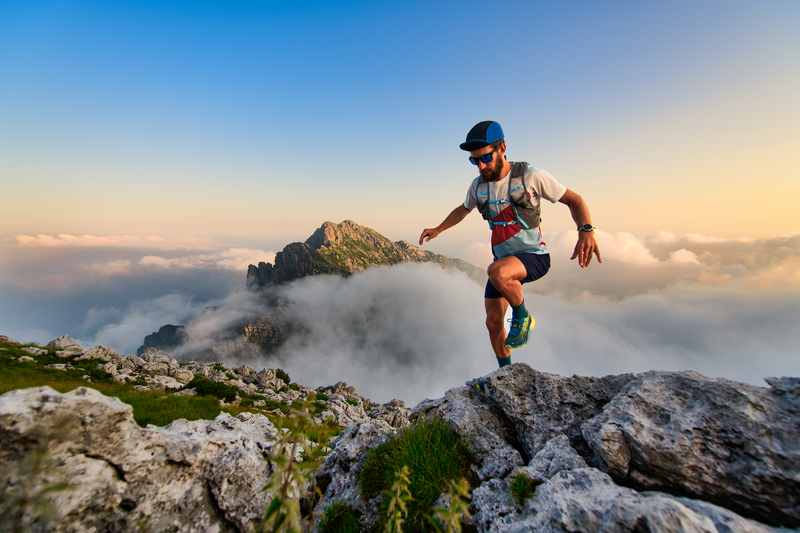
95% of researchers rate our articles as excellent or good
Learn more about the work of our research integrity team to safeguard the quality of each article we publish.
Find out more
ORIGINAL RESEARCH article
Front. Plant Sci. , 16 June 2022
Sec. Aquatic Photosynthetic Organisms
Volume 13 - 2022 | https://doi.org/10.3389/fpls.2022.889662
This article is part of the Research Topic Insights in Marine and Freshwater Plants: 2021 View all 6 articles
Peroxisomes participate in several important metabolic processes in eukaryotic cells, such as the detoxification of reactive oxygen species (ROS) or the degradation of fatty acids by β-oxidation. Recently, the presence of peroxisomes in the cryptophyte Guillardia theta and other “chromalveolates” was revealed by identifying proteins for peroxisomal biogenesis. Here, we investigated the subcellular localization of candidate proteins of G. theta in the diatom Phaeodactylum tricornutum, either possessing a putative peroxisomal targeting signal type 1 (PTS1) sequence or factors lacking a peroxisomal targeting signal but known to be involved in β-oxidation. Our results indicate important contributions of the peroxisomes of G. theta to the carbohydrate, ether phospholipid, nucleotide, vitamin K, ROS, amino acid, and amine metabolisms. Moreover, our results suggest that in contrast to many other organisms, the peroxisomes of G. theta are not involved in the β-oxidation of fatty acids, which exclusively seems to occur in the cryptophyte's mitochondria.
Peroxisomes are essential but enigmatic compartments found in the majority of eukaryotes (Platta and Erdmann, 2007; Bolte et al., 2015; Reumann and Bartel, 2016; Moog et al., 2017). These single membrane-bound organelles are highly dynamic, not only with regard to their metabolic capacities, but also their organization, shape, and abundance in different organisms, tissues, and even cell types (Gabaldón, 2010; Hu et al., 2012). The most commonly observed peroxisomal functions are detoxification of reactive oxygen species (ROS) and β-oxidation of fatty acids (Pieuchot and Jedd, 2012). Beyond these, there is huge plasticity in peroxisome biochemistry across eukaryotic diversity.
Although peroxisomes vary drastically in protein content, the machinery for biogenesis, division, and maintenance is strictly conserved (Michels et al., 2005). These processes are facilitated by the so-called peroxins (Pex), for which a conserved core set can be found in each peroxisome-containing organism described so far (Gabaldón et al., 2006; Schlüter et al., 2006; Moog et al., 2017). This fact clearly points to a single evolutionary origin of peroxisomes in eukaryotes (Pieuchot and Jedd, 2012; Bolte et al., 2015). The conserved set of peroxins constitutes the peroxisomal importomer – the translocation machinery on the cytosolic face of the organelle and in the peroxisomal membrane (Meinecke et al., 2010). Similar to the peroxisomal import machinery, targeting signals of proteins destined for import into peroxisomes are highly conserved. Such proteins, if soluble, contain either a peroxisomal targeting signal 1(PTS1) consisting of a C-terminal tripeptide or a PTS2 signal, which resembles a N-terminal nonapeptide (Petriv et al., 2004; Kim and Hettema, 2015; Reumann et al., 2016). Whereas PTS1 is recognized by the signal-specific receptor Pex5, the more rare PTS2 signal-containing proteins are imported via Pex7-mediated translocation (Smith and Aitchison, 2013; Platta et al., 2014; Reumann and Bartel, 2016).
Remarkably, and in contrast to most other translocation systems in eukaryotic cells, the peroxisomal importomer, which at least in parts shares common ancestry with the ER-associated protein degradation machinery (ERAD) (Gabaldón et al., 2006; Schlüter et al., 2006; Schliebs et al., 2010; Bolte et al., 2011; Kienle et al., 2016), is able to transport folded proteins and even oligomeric substrates (Erdmann and Schliebs, 2005; Schrader and Fahimi, 2008). This is the reason why some proteins without detectable PTS can be transported by the so-called piggyback mechanism into the peroxisomal lumen.
The conservation of cell biological traits on the one hand and the metabolic variation on the other shows the adaptability of peroxisomes in different taxa, which is beneficial to organisms responding to changing environmental conditions. Peroxisomes have clearly played an important role in the evolution of eukaryotic cells (Bolte et al., 2015). In multicellular eukaryotes such as mammals, peroxisomes are of critical importance: peroxisomal disorders are linked to several severe human diseases (Schrader and Fahimi, 2008; Smith and Aitchison, 2013). The extent to which peroxisomes are essential in other eukaryotes can only be guessed based on what has been determined for a handful of laboratory model organisms. These include animals, higher plants (Arabidopsis), and fungi such as yeast, whereas the majority of eukaryote diversity – predominantly single-celled organisms – has not been studied in detail (Gabaldón, 2010). Recently, a unicellular green alga became an important key player in peroxisomal research. It was shown that Chlamydomonas reinhardtii imports proteins containing typical and non-canonical PTS into peroxisomes (Hayashi and Shinozaki, 2012; Lauersen et al., 2016; Kato et al., 2021). Moreover, peroxisomes of C. reinhardtii are involved in several important metabolic processes such as the β-oxidation of fatty acids, the glyoxylate cycle, and H2O2 detoxification (Lauersen et al., 2016; Kong et al., 2017; Kato et al., 2021).
Microalgae include some of the most ecologically significant and abundant primary producers on earth. Some of them evolved by an endosymbiosis between two eukaryotic cells and have thus a highly complex subcellular compartmentalization. More specifically, they contain complex plastids surrounded by three or four membranes that stem from eukaryotic endosymbionts (red or green algae). One of them is the cryptophyte Guillardia theta, which contains a complex plastid of red algal ancestry. A unique feature of this organism is that its complex four membrane-bound plastid still maintains a remnant of the nucleus of the former red algal endosymbiont – the nucleomorph – between the two outer and two inner plastidial membrane pairs (Archibald, 2015). Although evolutionarily and ecologically important organisms, less is known about the presence and function of peroxisomes in microalgae.
Molecular biological studies on peroxisomes of exotic microalgae such as cryptophytes are rare and have only recently been initiated on a genome wide scale (Moog et al., 2017; Ludewig-Klingner et al., 2018; Mix et al., 2018). Recently, we have identified a complete set of peroxins in the cryptophyte G. theta, strongly indicating the presence of peroxisomes in these evolutionarily significant microalgae (Mix et al., 2018). Similar observation was made for other chromalveolates (organisms with complex plastids of red algal origin) including haptophytes, stramenopiles, and alveolates, the latter including apicomplexan parasites, which are supposed to lack peroxisomes, chromerids, and dinoflagellates (Moog et al., 2017; Mix et al., 2018; Ludewig-Klingner et al., 2018). In diatoms, not only the presence of peroxins was investigated, but also peroxisomal metabolic functions were studied via initial localization studies and bioinformatic predictions suggesting that diatom peroxisomes contain enzymes for fatty acid β-oxidation, ROS detoxification, and the glyoxylate cycle (Kroth et al., 2008; Gonzalez et al., 2011), but almost no enzymes for photorespiration (Davis et al., 2017), which are typical for peroxisomes of other phototrophs such as plants (Reumann and Bartel, 2016).
Our previous work in G. theta strongly implies that peroxisomes exist in these secondarily evolved algae, which – in contrast to, e.g., diatoms – appear to be capable of PTS1- and PTS2-dependent protein import. However, less is known about the metabolic, cell biological, and evolutionary significance of peroxisomes in cryptophytes. Here, we report on the putative metabolic functions and cell biology of peroxisomes in the cryptophyte G. theta.
Guillardia theta proteins (Guith1, gene catalog, and best model proteins, https://mycocosm.jgi.doe.gov/Guith1/Guith1.home.html) were used for the identification of PTS1-containing proteins via search for the C-terminal tripeptide with the consensus sequence [SAC]-[KRH]-[LM] using a local script. Identified candidates were analyzed via KOALA BLAST in the Kyoto Encyclopedia of Genes and Genomes (KEGG; https://www.kegg.jp/blastkoala/), and these sequences were additionally used as queries for BLASTP analyses in NCBI (https://blast.ncbi.nlm.nih.gov/Blast.cgi?PAGE=Proteins). Putative PTS1-containing candidates were further analyzed with localization prediction tools for signal peptides (SignalP 3.0 (https://services.healthtech.dtu.dk/service.php?SignalP-3.0) and SignalP 4.1 (https://services.healthtech.dtu.dk/service.php?SignalP-4.1)), mitochondrial targeting peptides (TargetP v1.1 (https://services.healthtech.dtu.dk/service.php?TargetP-1.1), Predotar (https://urgi.versailles.inra.fr/predotar/), PredSL (http://aias.biol.uoa.gr/PredSL/input.html)), and PTS1 (http://mendel.imp.ac.at/pts1/PTS1predictor.jsp) and were additionally screened for transmembrane domains (http://www.cbs.dtu.dk/services/TMHMM/).
For the identification of factors involved in β-oxidation known protein sequences of Phaeodactylum tricornutum, Arabidopsis thaliana or Saccharomyces cerevisiae were used as queries in BLAST analyses. Identified putative sequences were analyzed bioinformatically as described above. For further differentiation, candidates for Gt_ACOX/Gt_ACAD with the ID_1477553/ID_90066 were bioinformatically analyzed in PHYRE2 (Kelley et al., 2015; http://www.sbg.bio.ic.ac.uk/phyre2/html/page.cgi?id=index) and a protein data bank (https://www.rcsb.org/).
Putative PTS2-proteins in G. theta were identified using an in-house script searching for the nonapeptide consensus [RK]-[LVIQ]-X5-[HQ]-[LAF] within the first 50 amino acids. Furthermore, some identified protein sequences that might be located and connected to in vivo localized PTS1 proteins from the first approach were analyzed manually according to the presence of a PTS2-like signal.
The diatom P. tricornutum (Bohlin, UTEX646) was cultured in 500-ml Erlenmeyer flasks at 21°C under constant shaking (150–200 rpm) and constant light (24 h; 8,000–10,000 Lux) in 150 ml liquid f/2 medium (pH 8.0) containing 1.66% (w/v) Tropic Marin (Dr. Biener GmbH) salt, 2 mM Tris/HCl (pH 8.0), and 1.5 mM NH4Cl as a nitrogen source, or on solid f/2 plates containing agar (1,5% w/v). For the selection of positive transfected clones, Zeocin (InvivoGen) was added to a final concentration of 75 μg/ml. Protein overexpression was induced using f/2 medium with 0.89 mM NaNO3 as nitrogen source in 50 μl liquid f/2 medium for 24 h or on solid f/2 agar plates for 72 h as described before (Mix et al., 2018).
The cryptophyte G. theta (CCAM2327/CCMP2712) was cultured at 21°C in stationary Erlenmeyer flasks in a daily 14-h light and 10-h dark cycle (ca. 750 Lux) in 200 ml f/2 liquid medium (pH 7.2) containing 3.0% (w/v) Tropic Marin (Dr. Biener GmbH) salt, 5 mM Tris/HCl (pH 8.0), and 500 mM NH4Cl as a nitrogen source.
For DNA/RNA isolation, G. theta was cultured in 200 ml f/2 medium for 7–14 days, as described earlier (Mix et al., 2018). G. theta cells were centrifuged at 3,000 x g and 21°C for 10 min. Total DNAs were isolated from the pelletized G. theta cells via the CTAB method and were stored at −20°C. Total RNAs of G. theta were extracted using the RNeasy Mini kit (Qiagen) and were promptly treated with DNAseI (Thermo Fisher Scientific), following the manufacturer's instructions, to remove potential genomic DNA contamination. cDNA was synthesized with the FastGene Scriptase II Kit (Nippon Genetics) using random hexamer oligonucleotides provided by the manufacturer. Synthesized cDNA was used for amplification of gene sequences with specific oligonucleotides (refer to Supplementary Table S4).
For localization studies, selected candidate genes were amplified via standard polymerase chain reaction (PCR) on synthesized G. theta cDNA using the Q5® High-Fidelity DNA Polymerase (NEB) and gene-specific oligonucleotides synthesized by MERCK (Germany). Amplified PCR fragments were subcloned into pJet1.2/blunt vector using the CloneJET™ PCR Cloning Kit (Thermo Fisher Scientific), sequenced (Macrogen), and finally fused to the enhanced green fluorescence protein (GFP) into the pPhaNR vector (GenBank: JN180663) via specific restriction sites. Generated plasmids were purified from a 50 ml E. coli TOP10 culture using the NucleoBOND® MIDI Kit (Macherey-Nagel) and verified by sequencing (Macrogen). Tungsten M10 particles were coated with 5 μg of plasmid DNA and were used for biolistic transformation of P. tricornutum wildtype or mRuby3-SKL expressing strain with the Biolistic PDS-1000/He Particle Delivery System (Bio-Rad) using 1,350 psi rupture discs, as described previously (Apt et al., 1996).
A 2-week-old G. theta wildtype culture was used for protein extraction. The cells were harvested and washed with PBS buffer before lysis with an extraction buffer containing 125 mM Tris-HCl (pH 8), 3 % (v/v) SDS, 0.2 % Triton X-100, and 0.5 % (v/v) proteinase inhibitor cocktail (PIC). The proteins were precipitated 30 min on ice with trichloroacetic acid at final concentration of 10% (v/v). After precipitation, the proteins were washed at least three times with 80 % (v/v) acetone, dried, and resuspended in 2x urea buffer. Approximately 15 μg of total protein extract was loaded on a sodium dodecyl sulfate-polyacrylamide gel electrophoresis (SDS-PAGE), separated, and transferred on a nitrocellulose membrane using a Pierce Semi-Dry Blotter (Thermo Fisher Scientific). Immunodetection was performed with a specific anti-peptide antibody raised against the last 12 amino acids of the putative urate oxidase in G. theta (α-Gt_UO.12aaC, 1:3000, David's Biotechnology).
Gene expression in P. tricornutum was induced for 24 h in 50 μl f/2 medium containing 0.89 mM NaNO3 as nitrogen source (induction of nitrate reductase promoter in pPhaNR) or on solid f/2 plates for 72 h. To monitor the expressed proteins fused to GFP Leica TCS SP2 or TCS SP5 confocal laser scanning microscopes and an HCX PL APO 40_/1.25–0.75 Oil CS or HCX PL APO 40x/1.25-0.75 Oil Lbd. bl., objectives were used. The 65 or 100 mW Argon laser was used to excite the GFP fluorescence and chlorophyll autofluorescence at 488 nm with emission spectra of 500–520 nm for GFP and 625–720 nm for the plastid autofluorescence, respectively. The peroxisomal marker, mRuby3-SKL, was excited at 543 nm with the HeNe 1.2 mW laser at TCS SP2 or at 561 nm with DPSS 10 mW 561 nm at TCS SP5, and emission was detected in a range of 580–605 nm (Marter et al., 2020).
P. tricornutum clones expressing GFP-Gt_UO were further analyzed using the transmission electron microscopy (TEM). Therefore, 1-week-old liquid cultures (under non-inducing conditions) were induced for 24 h with 0.89 mM NaNO3. The cells were harvested (centrifugation at 1,500 × g, 21°C, 5 min) and were frozen by high pressure (Wohlwend HPF Compact 02). After subsequent freeze substitution (with acetone, containing 0.25% osmium tetroxide, 0.2% uranyl acetate, 0.05% ruthenium red, and 5% water) (Leica AFS2), the cells were embedded in Epon812 substitute resin (Fluka). Embedded cells were sectioned to 60-nm-thin sections with Leica EM UC7 RT, which were used for immunolabeling with α-GFP (Rockland; dilutions 1:500 and 1:1,000) or a specific anti-peptide antibody (α-Gt_UO.12aaC) against the last C-terminal 12 amino acids of Gt-UO provided by David's Biotechnology (dilutions 1:200, 1:500, and 1:1000). Additionally, ultrathin sections of G. theta wildtype cells were used for immunolabeling with α-Gt_UO.12aaC. As a secondary antibody, goat-anti-rabbit and rabbit-anti-goat antibodies coupled to ultrasmall gold particles were used, respectively (Aurion, dilution 1:100). Subsequently, a silver enhancement procedure was done (Danscher, 1981) and sections were post-stained with 2% uranyl acetyte and 0.5% lead citrate. Analysis of the samples was conducted with a JEOL JEM2100 TEM equipped with a fast-scan 2k CCD TVIPS (Gauting, Germany) F214 camera.
Less is known about the existence and function of peroxisomes in cryptophytes. Recently, the existence of a complete core set of peroxins (Pex) responsible for peroxisome biogenesis and maintenance was reported in G. theta, indicating the presence of peroxisomes in the cryptophyte (Mix et al., 2018). Here, we investigated the G. theta proteome regarding peroxisomal metabolism with the focus on PTS1-containing proteins. The most peroxisomal proteins reported so far contain the PTS1 signal (Gould et al., 1989; Brocard and Hartig, 2006), which is more abundant and conserved than the N-terminal nonapeptide PTS2. Besides, localization studies of PTS1-containing proteins can be conducted in P. tricornutum (Gonzalez et al., 2011; Mix et al., 2018).
A total of 24,840 nucleus-encoded G. theta proteins revealed by genome sequencing (Curtis et al., 2012) were analyzed for the presence of the C-terminal PTS1 signal consisting of [SAC]-[KRH]-[LM] within the last three amino acids of each sequence using an in-house script. The detected protein sequences were further analyzed via BLAST (refer to Materials and Methods). In total, 64 candidates were identified containing a putative PTS1 signal which were further analyzed with the PTS1 predictor (refer to Materials and Methods) to approve the character of the assumed PTS1 sequence. Thereby, for 58 of 64 putative PTS1 candidates (= 90.6%), the predicted PTS1 signal was confirmed (refer to Supplementary Table S1). Additionally, all PTS1-predicted proteins were screened for N-terminal targeting signals and transmembrane domains to identify potential false-positive candidates and sequences with a putative dual targeting. Using BlastKOALA, 1/3 of the 64 putative peroxisomal proteins were annotated and covered several essential metabolic functions, indicating the importance of the cryptomonad's peroxisomes within the cellular metabolic network in G. theta (refer to Supplementary Table S1). In summary, 31 out of 58 (53.44 %) predicted PTS1-containing proteins were identified to play a role in G. theta's peroxisomal metabolism, and 19 of the 31 (61.3 %) proteins were predicted without any additional targeting signals or transmembrane domains (refer to Supplementary Table S1).
Although the typical peroxisomal enzyme catalase is usually a significant factor in the cellular detoxification of H2O2, no homolog G. theta protein was detected. Furthermore, no factors involved in β-oxidation of fatty acids, photorespiration, or glyoxylate cycle were identified in the cryptomonad containing a classical PTS1 signal, which is, for example, contrary to diatoms P. tricornutum and Thalassiosira pseudonana, (Gonzalez et al., 2011; Davis et al., 2017), indicating a special role of peroxisomes in the cryptomonad. Nevertheless, in silico results proposed the existence of several classical peroxisomal enzymes: ascorbate peroxidase (APX), urate oxidase (UO), 1,4-dihydroxy-2-naphthoyl-CoA synthase (MenB), alkyldihydroxyacetonephosphate synthase (AGPS) that participate in ROS detoxification, purine and ether phospholipid metabolisms, and vitamin K synthesis (refer to Table 1). Additionally, enzymes involved in carbohydrate, amine, and amino acid metabolism were identified containing a putative PTS1 signal at the C-terminus (refer to Table 1). Although G. theta contains the PTS2 receptor Pex7 and the corresponding Pex5 as potential co-receptor with a Pex7-binding domain (Mix et al., 2018), bioinformatic analyses using an in-house script revealed only very few potential PTS2-containing candidates (refer to Supplementary Table S2) with the consensus [RK]-[LVIQ]-X5-[HQ]-[LAF] present within the first 50 amino acids (Osumi et al., 1991; Swinkels et al., 1991; Petriv et al., 2004; Rucktäschel et al., 2011). The most promising PTS2-candidate in G. theta is a homolog to the mevalonate kinase (MVK) with a putative PTS2 signal at the N-terminus consisting of “KLILFGEHF,” which participates in isoprenoid biosynthesis.
Table 1. Putative peroxisomal PTS1(like)-containing proteins of G. theta were heterologously localized in P. tricornutum.
In silico analyses predicted several proteins in G. theta that might be targeted to peroxisomes via PTS1-mediated import (refer to Table 1). For verification, heterologous in vivo localization studies were conducted in the diatom P. tricornutum, which in comparison with G. theta has a similar subcellular compartmentation. The diatom was used in several previous studies as a suitable model system for localization studies of cryptophyte proteins (e.g., Gould et al., 2006; Gile et al., 2015; Mix et al., 2018). However, it had to be verified whether the diatom is capable of importing cryptophyte peroxisomal matrix proteins via the PTS1 import pathway.
Gt_UO (urate oxidase) with the putative PTS1 “SKL” at its C-terminus has exemplarily been localized in full length and as a control with deleted PTS1 as a fusion protein with N-terminal GFP in P. tricornutum, respectively (refer to Figure 1A). Whereas expression of the GFP-Gt_UO (N-terminal GFP) resulted in a dot-like fluorescence structure typical for peroxisomes, GFP-Gt_UO-ΔSKL (N-terminal GFP, “SKL” deleted) localized to the cytoplasm of the diatom (refer to Figure 1A).
Figure 1. Heterologous in vivo localization and analysis of PTS1 function of G. theta urate oxidase (UO) expressed in P. tricornutum. (A) Expression of N-terminally fused GFP to the full-length Gt_UO-containing C-terminal “SKL” as PTS1 in P. tricornutum resulted in small dot-like structures next to the plastid. Without the C-terminal tripeptide “SKL,” GFP-Gt_UO-ΔSKL fluorescence was observed throughout the whole cell. (B) Immunolabeling of GFP-Gt_UO expressing P. tricornutum with α-GFP revealed electron-dense structures in round-shaped compartment near the plastid P, mitochondria M, and nucleus N; PM plasma membrane. (C) Co-expression of GFP-Gt_UO and the peroxisomal marker mRuby3-SKL (Marter et al., 2020) in P. tricornutum resulted in similar small dot-like structures next to the plastid. TL transmitted light, PAF plastidial autofluorescence, GFP enhanced green fluorescence protein, mR3-SKL mRuby3-SKL fluorescence protein as peroxisomal marker, scale bar in (A, C) 10 μm, scale bar in (B) overview 1 μm and 0.5 μm in section.
In addition to the in vivo localizations of Gt_UO, analyses of the GFP-Gt_UO expressing clone (Figure 1B) via transmission electron microscopy (TEM) using immunogold labeling on ultrathin sections were carried out. First, a P. tricornutum clone expressing GFP-Gt_UO was immunolabeled with α-GFP to verify the heterologous localization of GFP-Gt_UO. As shown in Figure 1B, a round-shaped electron-dense structure with 200–400 nm in diameter was marked by gold particles, indicating a compartment located next to the mitochondrion and the plastid. Similar structures were observed by McCarthy et al. (2017) and Mix et al. (2018). Further, co-localization studies with the peroxisomal marker mRuby3-SKL stably expressed by a P. tricornutum cell line (Marter et al., 2020) were established to fasten reliable heterologous localization. For GFP-Gt_UO, small dot-like GFP fluorescence was observed with the confocal microscopy clearly co-localizing with the mRuby3-SKL fluorescence (refer to Figure 1C).
Together with the results for the heterologously localized G. theta peroxins (Mix et al., 2018), these results illustrate that the peroxisomal targeting information of G. theta peroxins and other classical peroxisomal proteins containing a PTS1 signal is basically recognized in P. tricornutum, making the diatom a suitable model system for studying the localization of PTS1-containing candidates from G. theta.
To confirm peroxisomal localization of Gt_UO in G. theta, a specific anti-peptide antibody was derived from the last 12aa of the peptide sequence, including the C-terminal PTS1 signal “SKL” (PIDGPSGYITSSASLNRSKL). In western blot analyses, specific binding of the α-Gt_UO.12aaC could be determined (refer to Supplementary Figure 1). Further, the α-Gt_UO.12aaC antibody was used for immunolabeling on ultrathin sections of P. tricornutum clone expressing GFP-Gt_UO and on G. theta wildtype sections (refer to Figure 2). Similar to the labeling with α-GFP (refer to Figure 1B), a round-shaped immunogold-labeled electron-dense structure was observed in P. tricornutum (refer to Figure 2A), indicating a peroxisome or a small spherical compartment and confirming the specific binding of the anti-peptide antibody. Since the specific binding of the α-Gt_UO.12aaC was confirmed on ultrathin sections in the heterologously expressing diatom and in western blot analyses with whole protein extract of G. theta, endogenous localization studies of Gt_UO in G. theta were carried out via TEM. Immunolabeling with α-Gt_UO.12aaC on ultrathin G. theta sections revealed electron-dense structures in a tiny round-shaped compartment, with some additional labeling in the plastid (refer to Figure 2B). Through bioinformatic analyses, no N-terminal signal peptide for Gt_UO was determined; therefore, it has to be analyzed further if Gt_UO indeed localizes in both peroxisome and the plastid or if the electron-dense structures in the plastid are representing an unspecific binding of the antibody.
Figure 2. Immunolabeling with α-Gt_UO.12aaC in G. theta and P. tricornutum expressing GFP-Gt_UO. Electron-dense structures in small round-shaped compartments were immunolabeled with the specific antibody α-Gt_UO.12aaC on ultrathin sections of G. theta (B) wildtype and P. tricornutum (A) expressing GFP-Gt_UO indicating localization in peroxisomes. In G. theta additional labeling in the plastid could be observed. PM plasmid membrane, P plastid, N nucleus, M mitochondrion, scale bar 0.5 μm.
A stable transfected P. tricornutum cell line expressing mRuby3-SKL as a peroxisomal marker (Marter et al., 2020) was used to co-express GFP-tagged G. theta candidates containing putative PTS1. These include not only the peroxisome-specific UO that plays a role in purine metabolism but also APX for ROS detoxification and further enzymes participating in important metabolic pathways: vitamin K (MenB), ether phospholipid (FAR, AGPS), carbohydrate (MD, NME), amino acid (AAT), and amine metabolism (MAO). The corresponding genes were PCR amplified without introns from cDNA to ensure a correct open reading frame expression in the heterologous diatom model system. Most of the candidates were localized as full-length proteins with GFP fused N-terminally. This way, the C-terminal PTS1 signal necessary for recognition by the soluble Pex5 receptor responsible for peroxisomal import was not masked and a potential sterical hindrance was kept low. Localized proteins in different constructs are summarized in Table 1.
As mentioned above, Gt_UO was heterologously co-localized in the mRuby3-SKL expressing P. tricornutum strain (refer to Figure 1C). UO participates in the breakdown of purines producing H2O2 as a by-product. Although a typical peroxisomal H2O2-detoxifying catalase was not identified, bioinformatic analyses revealed putative PTS1-containing ascorbate peroxidase (APX). GFP-Gt_APX was co-localized in full-length heterologously in the P. tricornutum strain expressing mRuby3-SKL (refer to Figure 3) and might take over the detoxification of G. theta peroxisomes from ROS.
Figure 3. Heterologous in vivo co-localization of G. theta PTS1-containing proteins co-expressed in full length in P. tricornutum mR3-SKL. Heterologous localization studies of G. theta PTS1-containing enzymes in P. tricornutum expressing a peroxisomal marker (mR3-SKL). Peroxisomal localization was confirmed for APX (ascorbate peroxidase), MenB (1,4-dihydroxy-2-naphtoyl-CoA synthase), FAR (fatty acyl-CoA reductase), MD (malate dehydrogenase), and NME (NAD-dependent malic enzyme). TL transmitted light, PAF plastid autofluorescence, GFP enhanced green fluorescence protein, mR3-SKL mRuby3-SKL fluorescence protein as peroxisomal marker, scale bar 10 μm.
Identification of a naphthoate synthase (1,4-dihydroxy-2-naphthoyl-CoA synthase, MenB) containing a putative PTS1 in G. theta that is encoded by menB indicates the peroxisomal participation in vitamin K synthesis. GFP-Gt_MenB was co-localized with the peroxisomal marker in P. tricornutum, verifying peroxisomal localization of the fusion construct (refer to Figure 3). Note that GFP-Gt_MenB was also observed in bigger punctuate structures in P. tricornutum next to the plastid and peroxisomes not co-localizing these (refer to Supplementary Figure 6).
Peroxisomes of G. theta also seem to participate in ether phospholipid synthesis, as we could identify a PTS1-containing fatty-acyl-CoA reductase (FAR) and an alkyl-dihydroxyacetone-phosphate synthase (AGPS) with putative PTS1 signals. A co-localization with the peroxisomal marker was confirmed in P. tricornutum for GFP-Gt_FAR. The fatty alcohols produced by FAR are crucial during the synthesis of ether phospholipids since these provide the alkyl moiety for the AGPS reaction step, which generates alkyl-dihydroxyacetone phosphate (alkyl-DHAP) (Wanders and Brites, 2010; Dean and Lodhi, 2018). For the full-length Gt_AGPS protein with N-terminal GFP, a peroxisomal localization could not be confirmed. Instead, GFP-Gt_AGPS was observed throughout the cell, indicating cytosolic localization. In comparison, the GFP-AGPS.12aaC version showed a partial localization in peroxisomes and cytosol (refer to Supplementary Figure 2). Since the GFP fusion construct was highly overexpressed and due to heterologous localizations in P. tricornutum, these results should be interpreted with caution (refer to Discussion).
We identified two putative PTS1-containing proteins that might play a role in carbohydrate metabolism (refer to Table 1). A peroxisomal isoform of malate dehydrogenases was localized as GFP-Gt_MD in small dot-like structures, as shown by confocal microscopy. Visualized structures for GFP-Gt_MD were co-localized with the peroxisomal marker (mRuby3-SKL), indicating peroxisomal localization in P. tricornutum. A similar localization was observed for the G. theta NAD-dependent malic enzyme (NME) when localized as a GFP-Gt_NME fusion protein in P. tricornutum (refer to Figure 3).
An aspartate amino transferase (AAT) was detected in bioinformatic analyses containing a putative PTS1 signal. Similar to heterologous localization studies of Gt_AGPS in P. tricornutum, the full-length Gt_AAT with N-terminally fused GFP (GFP-Gt_AAT) was observed in the cytosol. Instead, GFP-Gt_AAT.12aaC co-localized with the peroxisomal marker expressed in P. tricornutum indicating that at least in the diatom, the last 12aa containing the C-terminal PTS1 tripeptide are recognized by the soluble Pex5 receptor for peroxisomal import (refer to Supplementary Figure 2).
A putative PTS1-containing monoamine oxidase (MAO) essential for amine metabolism was localized as a GFP-Gt_MAO.12aaC version in P. tricornutum to verify the capacity of the C-terminus for PTS1-mediated import into peroxisomes. Co-expressed with the peroxisomal marker mRuby3-SKL in P. tricornutum, fluorescence in small dot-like structures was observed (refer to Supplementary Figure 2). Due to overexpression in P. tricornutum via the nitrate reductase promoter, some GFP-labeled G. theta proteins showed not solely dot-like structures but seemed to localize partially in the cytosol (refer to Supplementary Figure 2).
It was remarkable that our bioinformatic research did not reveal any PTS1-containing candidates for peroxisomal β-oxidation. In mammals, peroxisomes and mitochondria share the degradation of fatty acids via β-oxidation, whereby in plants and yeast, only peroxisomal β-oxidation was reported (Poirier et al., 2006). In both organelles, acyl-CoA is produced by shortening acyl-CoA esters in similar reaction steps. We aimed to identify homologous proteins for all factors participating in β-oxidation and to analyze in which organelle or compartment β-oxidation takes place in the cryptomonad G. theta. However, in silico analyses suggest that the cryptophyte lacks peroxisome-targeted β-oxidation pathway. Heterologous in vivo localization studies and in-depth bioinformatic analyses of putative candidates were carried out to review these results.
In peroxisomes, the first step in β-oxidation of fatty acids is enforced by an acyl-CoA oxidase (ACOX) producing H2O2 as a by-product, whereas in mitochondria, an acyl-CoA dehydrogenase (ACAD) catalyzes the first reaction step transferring the electrons to the respiratory chain (Camões et al., 2015). Through bioinformatic analyses, we identified one candidate for ACOX (ID_147553) and five putative ACAD (ID_90066, ID_87673, ID_71272, ID_156886, and ID_113578) enzymes. All candidates were analyzed with several online prediction tools for putative targeting sequences, including mTP (mitochondrial targeting peptide) and PTS1 (refer to Materials and Methods). A mitochondrial localization was predicted for all Gt_ACOX and Gt_ACAD candidates. Interestingly, Gt_ACOX possesses “SRS” whereas Gt_ACAD with the protein ID_90066 contains “SAM” at the C-terminus. Both putative PTS1 signals distinguish in one amino acid from the known PTS1 consensus [SAC]-[KRH]-[LM]. Analyzing the last 12 C-terminal amino acids with the PTS1 prediction tool (Neuberger et al., 2003a,b), Gt_ACOX was predicted to be not targeted to peroxisomes whereby the putative PTS1 in Gt_ACAD could contribute to peroxisomal import, at least as predicted with general function (refer to Supplementary Table S3).
To determine in vivo location of putative peroxisomal Gt_ACOX (ID_147553) and Gt_ACAD (ID_90066), different variants of the corresponding genes were expressed as GFP fusion and localized heterologously in vivo in the P. tricornutum mRuby3-SKL cell line (Marter et al., 2020). To verify the mTP at the N-terminus, GFP was fused to the detected presequence and the full-length Gt_ACOX C-terminally. The Pre_Gt_ACOX-GFP (refer to Supplementary Figure 3) and Gt_ACOX-GFP (refer to Figure 4) were localized in elongated structures within P. tricornutum cells, not co-localizing with the peroxisomal marker mRuby3-SKL. Additionally, GFP was fused N-terminally to Gt_ACOX to check if the Gt_ACOX C-terminus was able to trigger peroxisomal import of the full-length Gt_ACOX via a potentially non-canonical PTS1-signal “SRS.” GFP-Gt_ACOX was localized cytosolically (refer to Figure 4 and Supplementary Figure 3), indicating that the putative PTS1 signal is not recognized in P. tricornutum. To verify the functionality of the C-terminal 12 amino acids (12aa), which were reported to have an impact on peroxisomal import (Neuberger et al., 2003a), GFP-Gt_ACOX.12aaC was constructed and localized in P. tricornutum. We observed similar localization throughout the cells compared to the GFP-Gt_ACOX version (refer to Figure 4 and Supplementary Figure 3).
Figure 4. Heterologous in vivo co-localization studies of factors involved in the first step of fatty acid β-oxidation in G. theta co-expressed in P. tricornutum mR3-SKL. GFP-Gt_ACOX (acyl-CoA oxidase) was localized in the cytosol, whereas Gt_ACOX-GFP is targeted to the mitochondrion when localized heterologously in P. tricornutum. The initial mitochondrial enzyme for β-oxidation ACAD (acyl-CoA dehydrogenase) was detected in G. theta containing both a mTP and a non-canonical putative PTS1 signal. When expressed heterologously in P. tricornutum, GFP-Gt_ACAD and GFP-Gt_ACAD.12aaC were localized in the cytosol, indicating no impact of the C-terminal tripeptide on PTS1 import into peroxisomes at least in P. tricornutum. Expression of C-terminal GFP fused to ACAD (Gt_ACAD-GFP) in P. tricornutum resulted in dot-like localization not correlating the peroxisomal marker. TL transmitted light, PAF plastid autofluorescence, GFP enhanced green fluorescence protein, mR3-SKL mRuby3-SKL fluorescence protein as peroxisomal marker, scale bar 10 μm.
Since the Gt_ACAD candidate with the protein ID_90066 contains the putative PTS1 signal “SAM” which was predicted in general to trigger peroxisomal import, we decided to verify the predicted localization in vivo. Gt_ACAD was localized in full length as GFP fusion N-/C-terminally. GFP-Gt_ACAD was localized throughout the cytosol, whereas Gt_ACAD-GFP showed in some cases dot-like structures (refer to Figure 4). However, these structures were clearly bigger than typical peroxisomes and did not co-localize with the peroxisomal marker mRuby3-SKL (refer to Figure 4).
Localized Gt_ACOX (ID_147553) and Gt_ACAD (ID_90066) candidates were analyzed bioinformatically in more detail. ACAD enzymes usually consist of an α-domain at the N-terminus, followed by a β-domain and a α-domain at the C-terminus (α-β-α), whereas ACOX has a similar structure with an additional C-terminal α-domain (α-β-α-α) (Kim and Miura, 2004). The additional α-domain results in a bigger reaction site of the protein and enables the binding of very long fatty acids, whereas ACADs usually bind long and short fatty acids. Since ACOX and ACAD enzymes are quite similar, the in silico differentiation can be difficult. Bioinformatic analyses of both protein sequences in PHYRE2 and a protein data bank (refer to Materials and methods) revealed similarities of Gt_ACOX to other known ACOX and ACAD sequences whereby for Gt_ACAD, only other ACAD sequences were identified. In any case, a doubtless, functional classification of the protein with ID 147553 is not possible at the moment.
The second and the third step in β-oxidation can either be catalyzed by bifunctional enzymes in peroxisomes or by individual enzymes in mitochondria that can be combined in a trifunctional enzyme including steps 2 to 4 (Camões et al., 2015). BLAST analyses to search for enoyl-CoA-hydratase (ECH) usually catalyzing the second step of β-oxidation resulted in the identification of three candidates in G. theta (ID_88345, ID_99846, ID_86481). For these candidates, no peroxisomal import was predicted; instead, each Gt_ECH candidate possesses a predicted mTP for mitochondrial targeting. Interestingly, one putative multifunctional enzyme was detected using the bifunctional enzyme from P. tricornutum (ID_ 55069) as query for BLAST analyses. Gt_ECH-3HCD (ID_121132) is probably catalyzing ECH and 3-hydroxyacyl-CoA dehydrogenase (3HCD) reactions, thereby combining the second and the third step of fatty acid β-oxidation. After adjusting the gene model with EST and gDNA data, no PTS but a putative mTP was predicted on gDNA, indicating solely mitochondrial targeting of Gt_ECH-3HCD. Although the mTP of Gt_ECH-3HCD is not confirmed by EST data and could not be amplified on cDNA, we decided to localize the full-length protein fused to GFP. When expressed heterologously in P. tricornutum, Gt_ECH-3HCD-GFP localized in the cytosol indicating the absence of a functional mTP (refer to Supplementary Figure 4).
Only one candidate, Gt_3HCD, for the individual third step of β-oxidation homologous to the bifunctional enzyme from P. tricornutum was identified via BLAST analyses with the protein ID_161810. Prediction analyses proposed mitochondrial targeting of Gt_3HCD with no putative PTS signals. Heterologous in vivo localization in P. tricornutum of Gt_3HCD-GFP resulted in elongated structures within P. tricornutum typical for mitochondria localized proteins (refer to Supplementary Figure 4).
For the fourth step in fatty acid β-oxidation, a 3-ketoacyl-CoA-thiolase (KCT) is required. Peroxisomal isoforms contain either a PTS1 or a PTS2 (Carrie et al., 2007; Lee et al., 2009; Gonzalez et al., 2011), and mitochondrial isoforms can also be integrated in the trifunctional enzyme combining steps 2 to 4 (Camões et al., 2015). Our bioinformatic analyses revealed a putative mitochondrial Gt_KCT (ID_159886) with no PTS that is homologous to a subunit of a trifunctional enzyme. Due to a transcript, encoding an open reading frame not supported by ESTs or transcript data, we decided to use the presequence of Gt_KCT including the predicted mTP for analyzing the targeting prediction of the N-terminus via GFP localization studies. In P. tricornutum, Pre-Gt_KCT-GFP localized in elongated structures around the plastid indicating a mitochondrial localization (refer to Supplementary Figure 4).
This study is based on the heterologous localization studies of G. theta proteins co-expressed with a peroxisomal marker in P. tricornutum. Since the diatom P. tricornutum is capable of PTS1 but not PTS2 import (Gonzalez et al., 2011), localization studies of peroxisomal matrix proteins are limited to putative PTS1-containing proteins. Mix et al. (2018) showed that the diatom P. tricornutum is capable of sorting the peroxisome-specific Gt_Pexs with putative transmembrane domains (TMDs) to the peroxisomal membrane (Gt_Pex2, Gt_Pex10, Gt_Pex11-1, Gt_Pex11-2, Gt_Pex14, Gt_Pex16, Gt_Pex19-1, and Gt_Pex22), proposing P. tricornutum as a suitable model organism for heterologous localization studies. Here, we aimed to give new insights into metabolic capacity of G. theta peroxisomes focusing on heterologous co-localization studies of PTS1-containing proteins.
PTS1-containig Gt_UO was exemplarily used to prove the PTS1-functionality as a targeting signal of heterologously expressed proteins in P. tricornutum. Heterologous expression of the full-length Gt_UO with N-terminal GFP (GFP-Gt_UO) was co-localizing with the peroxisomal marker in small-dot like structures in P. tricornutum (refer to Figure 1A). Additionally, electron-dense structures were observed in small round-shaped organelles with 200–400 nm in diameter indicating peroxisomes (refer to Figures1B, 2) and the deletion of the C-terminal PTS1 “SKL” relocated the Gt_UO-ΔSKL to the cytosol (refer to Figure 1C). These results demonstrated that a least the most abundant PTS1 signal “SKL” triggers the PTS1-mediated import of heterologously expressed proteins in the diatom.
However, some PTS1-containing proteins of G. theta did not co-localize with the peroxisomal marker when expressed heterologously in P. tricornutum. Gt_AGPS (“SHL”) and Gt_AAT (“SKL”) were located dominantly in the cytosol when expressed as a full-length protein, even though the last C-terminal 12aa were imported into peroxisomes in P. tricornutum when fused to the C-terminus of GFP (refer to Supplementary Figure 2). These results indicate that the last 12aa at the C-terminus of heterologously expressed fusion constructs were recognized by the endogenous Pt_Pex5 receptor responsible for PTS1 import. Probably, the PTS1 signal of some FL proteins is not available for the PTS1 receptor Pt_Pex5 due to steric arrangement of the tertiary structure of the eGFP fusion protein. If so, localizations should be interpreted carefully, since the intracellular protein location in G. theta could differ from the findings of the heterologous localization studies in P. tricornutum. Nevertheless, as the predicted localization of most G. theta proteins was proven to be correct in co-localization studies and was widely supported by detailed in silico analyses of the overall protein sequences, we strongly propose P. tricornutum as a suitable model organism for heterologous in vivo localization studies of G. theta proteins.
Bioinformatic analyses and in vivo localization studies provided a broad overview of the predicted metabolism of peroxisomes in the cryptomonad G. theta. Although some of the heterologous localization studies initially gave inconclusive results and thus have to be interpreted with caution (see above), the majority of our in silico targeting predictions were shown to be correct upon expression of G. theta candidate proteins as GFP fusions in P. tricornutum. Together with our previous study on the localization of Pex factors in G. theta (Mix et al., 2018), our presented work strongly suggests the presence of metabolically active peroxisomes in the cryptophyte. This conclusion is also supported by electron microscopic evidence marking the peroxisomal protein Gt_UO in a small spherical electron-dense structure most likely resembling a peroxisome in the cytoplasm of G. theta (refer to Figure 2B). In the following, we will discuss the possible metabolic processes that probably take place in the G. theta peroxisomes and how these might be connected to the metabolic network in the entire cell (refer to Figure 5).
Figure 5. Model of the predicted metabolic pathways in the cryptomonad G. theta. For details see main text. Abbreviations: ACAD acyl-CoA dehydrogenase, ACOX acyl-CoA oxidase, ECH enoyl-CoA hydratase, 3HCD 3-hydroxyacyl-CoA dehydrogenase, 3KCT 3-Ketoacyl-CoA thiolase, FAR fatty acid reductase, DHAP dihydroxyacetone phosphate, GPAT/DHAPAT glycerolphosphate acyltransferase/dihydroxyacetone phosphate acyltransferase, AGPS alkyl-dihydroxyacetone phosphate synthase, MAO monoamine oxidase, AsA ascorbate, APX ascorbate peroxidase, MDHA monodehydroascorbate, MDHAR MDHA reductase, DHA dehydroascorbate, DHAR DHA reductase, GR glutathione reductase, XDH/XO xanthine dehydrogenase/oxidase, UO urate oxidase, ALN allantoinase, ALC allantoicase, ASP asparaginase, AAT amino aspartate transferase, c/pMD cytosolic/peroxisomal malate dehydrogenase, NME NAD-dependent malic enzyme, PHYLLO consisting of MenF, MenD, MenH, MenC; MenB DNC synthetase, MenA phytyltransferase/polyprenyltransferase, MenG methyltransferase, MVK mevalonate kinase. Bold localized proteins, black bioinformatically predicted location (manually detected PTS1/PTS2-like containing proteins), gray not identified in G. theta; further, not localized PTS1-candidates are not included in this model.
In catabolic processes such as peroxisomal β-oxidation, purine degradation, and monoamine desamination, reactive H2O2 is produced as a by-product and has to be detoxified quickly before the cell is harmed. A typical peroxisomal enzyme for ROS detoxification is catalase which usually serves as a peroxisomal marker (Duve and Baudhuin, 1966; Ghosh and Hajra, 1986). In P. tricornutum and C. reinhardtii, a catalase for H2O2 detoxification was localized in vivo to peroxisomes whereby CAT1 of C. reinhardtii contains a non-canonical PTS1 “GCL” (Gonzalez et al., 2011; Kato et al., 2021). In G. theta, bioinformatic analyses did not reveal a homolog gene encoding a catalase. However, beside two isoforms predicted to be acting in the plastid, a further isoform of L-ascorbate peroxidase (Gt_APX) with the C-terminal tripeptide “SRM” was co-localized in peroxisomes of the diatom P. tricornutum (refer to Figure 3).
Ascorbate peroxidase catalyzes the H2O2-dependent oxidation of L-ascorbate to monodehydroascorbate (MDHA) releasing H2O and is an important key player in the ascorbate (AsA) – glutathione (GSH) cycle. MDHA is further reduced to AsA by a MDHA reductase (MDHAR). Alternately, MDHA can spontaneously disproportionate to AsA and dehydroascorbate (DHA). Using GSH as a cofactor, DHA is recycled to AsA by DHA reductase producing the oxidized glutathione-disulfide (GSSG). For regeneration of the oxidized GSH, GSSG is reduced by GSH-reductase (GR) (Shigeoka et al., 2002; Ishikawa and Shigeoka, 2008; Tamaki et al., 2021).
Enzymes participating in AsA-GSH cycle were identified by screening the genome database of G. theta. A scheme of the putative metabolic peroxisomal processes in G. theta including the AsA-GSH cycle is presented in Figure 5. Peroxisomal localization was confirmed in heterologous localization studies for Gt_APX (refer to Figure 3). A homolog encoding a MDHAR was not detected via bioinformatic analyses in G. theta. Most likely, MDHA disproportionates spontaneously to DHA which might be further reduced by Gt_DHAR (ID_153349) back to AsA. Interestingly, the predicted Gt_DHAR contains not only a nonapeptide “RLLLVLPLF” at the N-terminus, which has similarities to the PTS2, but also a signal peptide, proposing dual targeting to peroxisomes and the plastid. BLAST and KEGG analyses revealed a homolog to GR in G. theta with the protein ID_83774. This predicted protein is encoded with the C-terminal tripeptide “SRV” that could trigger peroxisomal import according to the PTS1 predictor (Neuberger et al., 2003a,b). However, “SRV” is not a classical PTS1 signal, and thus, its targeting function in the cryptophyte remains elusive. Nevertheless, “SRV” function as a PTS1 signal was validated in Arabidopsis thaliana (Reumann et al., 2012).
The typical peroxisomal enzyme urate oxidase (UO) produces H2O2 as a by-product by oxidizing urate to 5-hydroxyisorate that is further converted to allantoate. UO is an important enzyme in purine degradation and recycling of nitrogen as well as glyoxylate in higher plants (A. thaliana and legumes) and in some Apicomplexa (Nephromyces and Cardiosporidium) (Todd et al., 2006; Hauck et al., 2014; Paight et al., 2019).
Purine degradation starts with purine desamination to xanthine, which is further converted to urate by xanthine dehydrogenases/oxidases (XDH/XO). XDH/XO activities can be combined in xanthine oxidoreductase (XOR) (Nishino et al., 2008). UO oxides urate to 5-hydroxyisorate, which is further converted to (S)-allantoin (Kahn and Tipton, 1998; Lamberto et al., 2010). (S)-Allantoin is converted by an allantoinase (ALN) to allantoate which is then processed by allantoicase to urea and ureidoglycolate. In the following step, urea can be recycled to CO2 and ammonia by urease. Alternatively, either ureidoglycolate is processed to glyoxylate or urea excreted as waste.
Here, we report a partial peroxisomal purine degradation pathway in G. theta resulting most likely in recycling of ammonia. Bioinformatic analyses of homologs involved in purine degradation revealed cytosol-located purine desaminases (not included in Figure 5) producing xanthine that is further converted to urate by Gt_XDH/XO with the protein ID_164776 lacking both a predictable PTS1 and PTS2 (refer to Figure 5). In G. theta, peroxisomal localization was confirmed for Gt_UO (refer to Figures 1, 2) producing H2O2 as by-product. A putative Gt_ALN with the protein ID_67814 contains the classical PTS1 “AKL” as C-terminal tripeptide. Heterologous in vivo localization studies in P. tricornutum revealed cytosolic localization for GFP-Gt_ALN (full length), whereas the last 12aa at the C-terminus fused to GFP (GFP-Gt_ALN.12aaC) localized in small dot-like structures resembling peroxisomes (refer to Supplementary Figure 5). No peroxisomal localization could be confirmed for the putative peroxisomal isoform Gt_ALC (ID_71482) expressed as full length or 12aa.C version with N-terminal GFP fusion with the putative PTS1 “SKV” in P. tricornutum. The PTS1-like signal “SKV” is deviating from the typical PTS1 pattern in the last amino acid which might affect the PTS1 recognition in P. tricornutum by the endogenous PTS1 receptor Pex5. A second putative candidate for Gt_ALC (ID_71482) is annotated in KEGG and might be located in the cytosol of G. theta (refer to Supplementary Figure 5). The homolog of urease with the protein ID_116849 in G. theta contains no predicted PTS1 but “KLKRLARGL” as N-terminal nonapeptide reminding of a PTS2 signal. However, G. theta PTS2-containing proteins cannot be localized heterologously in P. tricornutum because the PTS2 import pathway is missing in the diatom (Gonzalez et al., 2011; Mix et al., 2018).
It has to be noted that also, non-canonical PTS1 and PTS2 could contribute to peroxisomal import in G. theta peroxisomes which have not been identified via in silico predictions and might not be recognized in heterologous localization studies in P. tricornutum. For example, localization studies of non-canonical PTS in C. reinhardtii imply that PTS signals in certain microalgae might have a wider variation in amino acid composition than reported in higher plants (Lauersen et al., 2016; Kato et al., 2021).
Nevertheless, according to the heterologous localization studies, the purine degradation in G. theta seems to be partially located in peroxisomes. Moreover, bioinformatic analyses propose the degradation of urea resulting in reutilization of CO2 and ammonia. Green freshwater algae were shown to utilize allantoin as nitrogen source, supporting our results (Prasad, 1983). Upregulation of enzymes involved in purine degradation in Chlamydomonas reinhardtii proposed that the pathway is similar to higher plants with ammonia as end-product rather than urea (Park et al., 2015).
Another enzyme participating in H2O2 formation in G. theta peroxisomes was classified as monoamine oxidase (MAO) by signal search containing the C-terminal tripeptide “SRM.” Heterologous co-localization studies of the last 12aa of Gt_MAO in P. tricornutum confirmed peroxisomal localization (refer to Supplementary Figure 2). MAO is a flavoprotein with the cofactor FAD and uses oxygen for deamination of amines resulting in the corresponding ketone or aldehyde, H2O2, and ammonia (Gaweska and Fitzpatrick, 2011; Abad et al., 2013).
Taken together, in vivo localization studies and bioinformatic analyses propose at least partial compartmentalization of H2O2 formation during purine degradation or amine deamination and its rapid detoxification by AsA-GSH cycle in the peroxisomes of the cryptomonad G. theta (refer to Figure 5).
Besides the H2O2 formation and detoxification, several additional G. theta PTS1-containing proteins were identified and localized heterologously in peroxisomes of P. tricornutum, co-expressed with mRuby3-SKL as peroxisomal marker. The summarized putative metabolic map in G. theta is presented in Figure 5. G. theta peroxisomes seem to participate in carbohydrate metabolism since a PTS1-containing malate dehydrogenase and a NAD-dependent malic enzyme, which are involved in malate metabolism, co-localized with mRuby3-SKL in P. tricornutum peroxisomes.
Malate dehydrogenases (MD) convert malate to oxaloacetate and are therefore important key players in several metabolic processes (Kroth et al., 2008; Davis et al., 2017). In G. theta, three MD isoforms are annotated in KEGG and were analyzed via bioinformatics. We identified a cytosolic isoform (Gt_cMD) with the ID_163842, a predicted mitochondrial isoform (Gt_mMD) with the ID_154188, and a peroxisomal isoform (Gt_pMD) with the protein ID_64327 containing the typical “SKL” tripeptide at the C-terminus. Peroxisomal localization was confirmed for Gt_pMD in heterologous localizations studies in P. tricornutum (refer to Figure 3). Furthermore, Gt_pMD and Gt_cMD might participate in the glyoxylate cycle or the malate-aspartate shuttle that requires future in-depth analyses. Most likely, malate is synthetized in the cytosol or mitochondria and is transported into G. theta peroxisomes via the malate-aspartate shuttle (mitochondrial malate-aspartate shuttle is not included in Figure 5). One bioinformatically identified malate synthase (MS) with the protein ID_97605 was localized as GFP-Gt_MS.12aaC in the cytosol (data not shown), despite containing a non-canonical PTS1-like signal “SRK” which did not trigger PTS1-mediated import in P. tricornutum. Additionally, Gt_MS could be imported via a predicted N-terminal mTP into mitochondria. It has been proposed that N-terminal targeting signals might be more competitive in comparison with C-terminal PTS1 (Kunze and Berger, 2015; Stehlik et al., 2020). Nevertheless, dual targeting of Gt_MS cannot be excluded and should be analyzed in the future studies (not shown in Figure 5). Mitochondrial isoform Gt_mMD could participate in the TCA cycle (Sweetlove et al., 2010) (not shown in Figure 5) which has to be analyzed in further studies. Malate can be additionally converted by NAD(P)-dependent malic enzyme (ME) to pyruvate (Tronconi et al., 2008), contributing to the pyruvate metabolism. In G. theta, a NAD-dependent ME (Gt_NME) containing “CKL” as PTS1 was detected and peroxisomal localization was confirmed via heterologous localization studies in P. tricornutum (refer to Figure 3). The carbohydrate metabolism in the diatoms P. tricornutum and T. pseudonana was elucidated in detail (Kroth et al., 2008; Davis et al., 2017). Compared to the cryptomonad G. theta, the diatoms possess peroxisomal and mitochondrial isoforms for MD but only a mitochondrial NME (Kroth et al., 2008; Davis et al., 2017). In C. reinhardtii, two MD isoforms containing a PTS2 (MDH1 and MDH2) were in vivo localized to peroxisomes (Hayashi and Shinozaki, 2012; Hayashi et al., 2015; Lauersen et al., 2016). A knockout of MDH2 in C. reinhardtii affected peroxisomal β-oxidation of fatty acids, indicating that lipid metabolism is connected to a functional MDH2 in peroxisomes (Kong et al., 2018).
Previously known as glutamate oxaloacetate transaminase (GOT), aspartate amino transferase (AAT) converses aspartate with ketoglutarate to glutamate and oxaloacetate (Kirsch et al., 1984). For full functionality of AAT, pyridoxal 5'-phosphate is important, which is bound to AAT (Kirsch et al., 1984; Toney, 2014). AATs play usually an important role in the mitochondrial malate-aspartate shuttle and are also involved in breakdown of amino acids. For the identified Gt_AAT (ID_158574) with “SKL” as PTS1, a heterologous localization in P. tricornutum peroxisomes was confirmed (refer to Supplementary Figure 2). Further bioinformatic analyses of aspartate metabolism in G. theta revealed a putative asparaginase (Gt_ASP, ID_121809) with non-canonical “PRL” at the C-terminus indicating a putative PTS1 signal. In general, ASP hydrolyzes L-asparagine to L-aspartate releasing ammonia. Peroxisomal localization was confirmed for several proteins containing a “PRL” as the PTS1 signal in higher plants (A. thaliana), yeast (S. cerevisiae), and also an ascomycete pathogen (Colletotrichum higginsianum) (Reumann, 2004; Reumann et al., 2012; Nötzel et al., 2016; Robin et al., 2018). Whether the C-terminal tripeptide “PRL” also triggers peroxisomal PTS1-dependent import in P. tricornutum has to be validated in the future studies.
Heterologous in vivo localization studies of PTS1-containing proteins of G. theta in P. tricornutum partially confirmed peroxisomal localization of the in silico identified 1,4-dihydroxy-2-naphtoyl-CoA (DHNA-CoA) synthase (MenB) encoded by guithdraft_85732 in G. theta (refer to Figure 3). Next to peroxisomal GFP-Gt_MenB, deviating localization in bigger dot-structures was observed in P. tricornutum not co-localizing the peroxisomal marker which should be addressed in the future studies (refer to Supplementary Figure 6). MenB is one of the central enzymes involved in vitamin K synthesis. In photosynthetic organisms, vitamin K1 (phylloquinone) is an important electron carrier in photosystem I (Del Río, 2013; Cenci et al., 2018). Hence, the de novo biosynthesis of phylloquinone is an essential pathway in higher plants and photosynthetic organisms. Here, we investigated bioinformatically the enzymes involved in vitamin K synthesis and summarized the results in Figure 5.
In nine consecutive reaction steps, phylloquinone is synthesized from chorismate which is the product from shikimate pathway. In the first four steps, chorismate is converted to o-succinyl benzoate by plastid-targeted enzymes. The proteins involved in phylloquinone biosynthesis are functional similar to the enzymes involved in menaquinone (vitamin K2) biosynthesis and are therefore called Men enzymes. The first four steps are combined in a multifunctional protein referred as PHYLLO combining MenF, MenD, MenH, and MenC (Gross et al., 2006, 2008; Cenci et al., 2018). In higher plants, the isochorismate synthase (MenF) catalyzing the first reaction can also be encoded by individual genes enabling the independent regulation of other metabolic branches such as the synthesis of salicylic acids required for plant defense (Gross et al., 2006, 2008). Through bioinformatic analyses, we identified a putative Gt_PHYLLO protein with the protein ID_72208 in G. theta combining MenD, MenH, and MenC, which was predicted to localize to the plastid. Gt_MenF (ID_72109) is annotated in KEGG as menaquinone-specific isochorismate synthase. Additionally, a putative Gt_MenH (ID_72176) encoding gene was identified in the same gene cluster together with ID_72208 and ID_72109. If the identified Gt_MenF and Gt_MenH encoding individual genes or part of the PHYLLO cluster has to be addressed in future studies, the activation of o-succinyl-benzoate by an o-succinyl-benzoyl-CoA ligase (MenE) was determined in the peroxisomes in A. thaliana by peroxisomal acyl-CoA activating enzyme (AAE14, At1g30520) with non-canonical PTS1 “SSL” which might be also dually targeted to plastids (Kim et al., 2008; Babujee et al., 2010; Del Río, 2013 chapter 12 by S. Reumann). According to the localization prediction tools, the homologous Gt_MenE (ID_139711) contains no PTS-like signals and localizes to the plastid. It should be noted that only the very last C-terminal part of the putative Gt_MenE is predicted to function as o-succinyl benzoate-CoA ligase. After the activation of o-succinyl-benzoate, MenB catalyzes the naphtoate ring formation. MenB is encoded by a single gene and contains a conserved PTS2 in all putative orthologs in higher plants analyzed so far (Reumann et al., 2007; Babujee et al., 2010). Contrary to higher plants, Gt_MenB contains a more abundant C-terminal “SRL” and is imported into peroxisomes in a PTS1-dependent manner (refer to Supplementary Figure 6). Interestingly, in P. tricornutum, a “SKL”-containing homologous to MenB encoding gene (PHATRDRAFT_bd371) was identified with KEGG annotations proposing peroxisomal involvement in vitamin K biosynthesis in the diatom. DHNA-CoA thioesterase (DHNAT) produces DHNA by releasing CoA. In Arabidopsis thaliana, two genes encoding DHNAT were identified, both containing PTS1-signals, and were demonstrated to localize in plant peroxisomes (Reumann et al., 2009; Widhalm et al., 2012). No homologous gene encoding the specific DHNAT was identified in G. theta in our bioinformatic approach. Instead, we could identify several acyl-CoA thioesterases with putative hotdog-containing domains (refer to Supplementary Table S5), which were reported to be involved in the synthesis of phylloquinones (Dillon and Bateman, 2004; Widhalm et al., 2009). If one of these candidates is indeed a DHNAT participating in phylloquinone synthesis in G. theta, which has to be analyzed in future studies, the last two steps of vitamin K1 biosynthesis take place in the plastids of higher plants. MenA (DHNA phytyltransferase) attaches the phytyl chain to the naphthoate ring and MenG methylates the precursor to phylloquinone. No homologs encoding Gt_MenA or Gt_MenG were identified in G. theta. Usually, Men enzymes are located in one cluster on genomic level (Gross et al., 2008). The identified putative PHYLLO cluster (ID_72208 and ID_72109) should be examined in the future studies and investigated in respect to its catalyzed reactions. Finally, a vitamin-K-epoxide reductase (VKOR) was identified in G. theta (ID_106868, not included in Figure 5) and is predicted to localize in the plastidial membrane comparable with the thylakoid located VKOR in A. thaliana (Tie and Stafford, 2008; Feng et al., 2011).
Bioinformatic analyses identified a putative peroxisomal Gt_AGPS, which catalyzes one of the peroxisomal reactions during ether phospholipid synthesis. Heterologous in vivo co-localization studies in P. tricornutum are inconclusive, since a cytosolic localization was observed for the full-length protein (GFP-Gt_AGPS) whereas GFP-AGPS.12aaC co-localized with the peroxisomal marker (refer to Supplementary Figure 2). Therefore, a peroxisomal localization of Gt_AGPS in G. theta can be assumed (refer to Figure 5). AGPS exchanges the acyl group with an alkyl group of acyl-dihydroxyacetone phosphate (acyl-DHAP), which is acetylated in advance by glycerolphosphate acyltransferase (GPAT) or dihydroxyacetone phosphate acyltransferase (DHAPAT) (Hajra and Bishop, 1982; Hajra and Das, 1996; Dean and Lodhi, 2018). Inspections with KEGG in G. theta propose two Gt_GPAT/DHAPAT isoforms (ID_139343 and ID_163230) with 5-6 transmembrane domains without a predicted PTS or mTP. Additionally, the second putative Gt_GPAT/DHAPAT (ID_163230) is predicted to be secreted (N-terminal signal peptide) and is most likely involved in triacylglycerol (TAG) synthesis. Therefore, Gt_GPAT/DHAPAT (ID_139343) is probably activating DHAP and is attached to peroxisomal membrane which requires more analyses in the future (refer to Figure 5). Besides DHAP as an intermediate, AGPS also requires fatty alcohols that provide the alkyl residue. Fatty alcohols are catabolized by FARs. Gt_FAR homolog was identified as PTS1-containing enzyme and co-localized heterologously in peroxisomes of P. tricornutum (refer to Figure 3). Together with Gt_AGPS, Gt_FAR is involved in first steps in ether phospholipids synthesis which is completed in the endoplasmic reticulum (ER) (refer to Figure 5).
In eukaryotic cells, β-oxidation of fatty acids can take place either in mitochondria or in peroxisomes. The enzymatic reactions are similar varying in single enzymes and/or complexes. Peroxisomal β-oxidation starts with ACOX, whereas ACAD is most likely to be present in mitochondrial pathway. Both enzymes, ACOX and ACAD, form a trans-double bond in the acyl-CoA thioester. Steps 2–3 are catalyzed by bifunctional enzymes in peroxisomes; in mitochondria, individual enzymes but also trifunctional enzymes were observed combining steps 2–4 (Camões et al., 2015). These reactions include the addition of a water molecule by ECH (step 2), dehydrogenation by 3HCD (step 3), and the final acylation by 3KCT (step 4) resulting in the formation of an acetyl-CoA and acyl-CoA shortened by two carbon atoms.
In plants and fungi, β-oxidation is exclusively peroxisomal, whereas in mammals, both enzymatic pathways (mitochondria and peroxisomes) are presented. In examined algae so far, several dispositions according to the location of enzymes participating in β-oxidation were observed. In the charophyte Mougeotia, an exclusively peroxisomal β-oxidation was reported (Stabenau et al., 1984). In the diatom P. tricornutum, PTS1-containing isoforms for every step in peroxisomal β-oxidation of fatty acids were determined (Gonzalez et al., 2011). Additionally, a mitochondrial isoform of KCT (ID_28068) is annotated in P. tricornutum, and a mitochondrial PtMACAD1 encoded by Phatrdraft3_J11014 was identified (Jallet et al., 2020) proposing that the degradation of fatty acids via β-oxidation is taking place in mitochondria and peroxisomes in the diatom. Dual localization of β-oxidation enzymes was observed in the chlorophyte Eremosphaera and in several Prasinophytes (Winkler et al., 1988; Stabenau et al., 1989), similar to the situation in mammals. Additionally, solely mitochondrial β-oxidation was observed in the xanthophyte Bumilleriopsis and in Cyanidium (Gross et al., 1985; Gross, 1989). In C. reinhardtii, an ACOX encoded by CrACX2 was co-localized with peroxisomal CrMDH2 in peroxisomes, indicating at least partial peroxisomal β-oxidation of fatty acids (Kong et al., 2017).
Remarkably, in silico analyses did not reveal any homologs to enzymes that participate in β-oxidation containing the typical PTS1 signal consisting of [SAC]-[KRH]-[LM] in G. theta. Candidates for the first step of β-oxidation (ACOX/ACAD) were identified by bioinformatics with non-canonical C-terminal tripeptide reminding of a PTS1 signal (“SRS”/“SAM”) and additionally containing a mTP at the N-terminus, proposing dual targeting to peroxisomes or mitochondria of G. theta, respectively. However, heterologous co-localization studies in P. tricornutum expressing the peroxisomal marker mRuby3-SKL proposed mitochondrial localization for both G. theta enzymes (refer to Figure 4 and Supplementary Figure 3). For further differentiation, phylogenetic analyses and/or in vitro assays of the G. theta ACOX and ACAD should be conducted.
The following steps in β-oxidation are either catalyzed by individual or multifunctional enzymes, depending on the organism and the intracellular location of this pathway. In G. theta, several individual homologs for ECH, the second step in β-oxidation, were identified with predicted mTPs. Gt_ECH (ID_161810) was localized to the mitochondria of P. tricornutum (refer to Supplementary Figure 4). One putative multifunctional Gt_ECH-3HCD (ID_121132) probably combining steps 2–3 was predicted with a mTP after gene model correction with gDNA sequence. However, the putative mTP of ID_121132 was not confirmed by EST data and could not be amplified on cDNA. Nevertheless, we localized Gt_ECH-3HCD without the predicted mTP to the cytosol of P. tricornutum (refer to Supplementary Figure 4). It has to be still analyzed in more detail, if Gt_ECH-3CH is indeed a cytosol located protein and if it is able to catalyze both reaction steps mentioned above. Additionally, the presequence of Gt_KCT (ID_159886) catalyzing the fourth and last step in β-oxidation was observed in mitochondria when expressed heterologously as a GFP fusion in P. tricornutum underpinning the solely mitochondrial localization of β-oxidation in G. theta (refer to Supplementary Figure 4). If Gt_KCT in G. theta is a part of the multifunctional enzyme or if it is catalyzing, an individual reaction should be examined in the future studies.
Based on the in silico and heterologous in vivo localization studies of G. theta isoforms, β-oxidation takes place in the mitochondria (refer to Figure 5). It cannot totally be excluded, that some reaction steps are peroxisomal or dually localized to peroxisomes and mitochondria, because Gt_ACOX/Gt_ACAD contain non-canonical PTS1-like sequences (“SRS”/”SAM”), which might trigger peroxisomal import in G. theta and are possibly not recognized in P. tricornutum. Interestingly, in the ACOX homolog of C. reinhardtii, no PTS signal was detected but mCherry-CrACX2 was co-localized with a peroxisomal marker indicating that CrACX2 is imported via a non-PTS1 pathway (Kong et al., 2017). Besides, both Gt_ACOX and Gt_ACAD contain a N-terminal mTP which could compete on in vivo targeting in G. theta (Kunze and Berger, 2015; Stehlik et al., 2020). Additionally, all G. theta homologs that might participate in β-oxidation were analyzed for PTS2 signals. None of the identified G. theta homologs to β-oxidation participating enzymes contain the PTS2 signal [RK]-[LVIQ]-X5-[HQ]-[LAF] (Petriv et al., 2004). Moreover, non-canonical PTS2 sequences might exist in those G. theta sequences that might not be able to achieve their potential signaling effect in the diatom system because the PTS2 import pathway is absent in P. tricornutum (Gonzalez et al., 2011). However, taken into consideration the confirmed peroxisomal localization of PTS1-containing G. theta proteins and studies by Mix et al., 2018 in the heterologous system, the localizations in P. tricornutum are in most cases reliable. For further analyses, Gt_ACOX and Gt_ACAD should be characterized in more detail directly in G. theta, e.g., by immunolabeling with specific antibodies on G. theta via TEM.
In this study, we present in silico and experimental proof for the presence of metabolically active peroxisomes in G. theta. Interestingly, in the cryptophyte, these organelles most likely lack enzymes for fatty acid β-oxidation, which is the classical metabolic pathway of peroxisomes. Our results suggest that β-oxidation in G. theta seems to be exclusively mitochondrial, which is in contrast to the most other organisms studied so far, although it cannot be excluded that at least some β-oxidation enzymes are targeted to peroxisomes by non-canonical signals or are dually localized. Moreover, G. theta peroxisomes are devoid of catalase, but have kept their ROS detoxification capability to shield the cell from harmful byproducts of metabolic reactions taking place in G. theta peroxisomes: e.g., purine degradation and deamination. Additionally, G. theta peroxisomes play an important role in amino acid metabolism, the ascorbate-glutathione cycle, vitamin K, and ether phospholipid synthesis, being connected to a multitude of other biochemical pathways in the cryptophyte cell. Thus, peroxisomes are essential compartments in the complex metabolic network of G. theta. In future studies, the PTS1 and PTS2 sequence motifs in G. theta should be analyzed in more detail since at least some algal PTSs seem to be more diverse compared to the known motifs of higher plants.
The datasets presented in this study can be found in online repositories. The names of the repository/repositories and accession number(s) can be found in the article/Supplementary Material.
DM conceived this study together with JV and UM. JV, A-KM, TH, and DM did the experimental work and in vivo localization studies in P. tricornutum. DM conducted the in silico analyses. TH performed the immunolabelling and electron microscopy analysis. The manuscript was written by JV, DM, and UM. All authors read and approved the manuscript.
This work was funded by the Deutsche Forschungsgemeinschaft (DFG, German Research Foundation) — 409750538 (MO 3129/3-1).
The authors declare that the research was conducted in the absence of any commercial or financial relationships that could be construed as a potential conflict of interest.
All claims expressed in this article are solely those of the authors and do not necessarily represent those of their affiliated organizations, or those of the publisher, the editors and the reviewers. Any product that may be evaluated in this article, or claim that may be made by its manufacturer, is not guaranteed or endorsed by the publisher.
We kindly thank to Marion Schön for technical assistance with sample preparation for electron microscopy as well as Luisa Raap for technical assistance with cloning and transformation of Gt_UO/ALN/ALC.12aaC in P. tricornutum wildtype.
The Supplementary Material for this article can be found online at: https://www.frontiersin.org/articles/10.3389/fpls.2022.889662/full#supplementary-material
Abad, E., Zenn, R. K., and Kästner, J. (2013). Reaction mechanism of monoamine oxidase from QM/MM calculations. J. Phys. Chem. B 117, 14238–14246. doi: 10.1021/jp4061522
Apt, K. E., Kroth-Pancic, P. G., and Grossmann, A. R. (1996). Stable nuclear transformation of the diatom phaedactylum tricornutum. Mol. Gen. Genet. 771–57. doi: 10.1007/s004380050264
Archibald, J. M. (2015). Endosymbiosis and eukaryotic cell evolution. Curr. Biol. 25, R911–R921. doi: 10.1016/j.cub.2015.07.055
Babujee, L., Wurtz, V., Ma, C., Lueder, F., Soni, P., van Dorsselaer, A., et al. (2010). The proteome map of spinach leaf peroxisomes indicates partial compartmentalization of phylloquinone (vitamin K1) biosynthesis in plant peroxisomes. J. Exp. Bot. 61, 1441–1453. doi: 10.1093/jxb/erq014
Bolte, K., Gruenheit, N., Felsner, G., Sommer, M. S., Maier, U. G., and Hempel, F. (2011). Making new out of old: recycling and modification of an ancient protein translocation system during eukaryotic evolution. Mechanistic comparison and phylogenetic analysis of ERAD, SELMA and the peroxisomal importomer. Bioessays 33, 368–376. doi: 10.1002/bies.201100007
Bolte, K., Rensing, S. A., and Maier, U. G. (2015). The evolution of eukaryotic cells from the perspective of peroxisomes: phylogenetic analyses of peroxisomal beta-oxidation enzymes support mitochondria-first models of eukaryotic cell evolution. Bioessays 37, 195–203. doi: 10.1002/bies.201400151
Brocard, C., and Hartig, A. (2006). Peroxisome targeting signal 1: is it really a simple tripeptide? Biochim. Biophys. Acta 1763, 1565–1573. doi: 10.1016/j.bbamcr.2006.08.022
Camões, F., Islinger, M., Guimarães, S. C., Kilaru, S., Schuster, M., Godinho, L. F., et al. (2015). New insights into the peroxisomal protein inventory: Acyl-CoA oxidases and -dehydrogenases are an ancient feature of peroxisomes. Biochim. Biophys. Acta 1853, 111–125. doi: 10.1016/j.bbamcr.2014.10.005
Carrie, C., Murcha, M. W., Millar, A. H., Smith, S. M., and Whelan, J. (2007). Nine 3-ketoacyl-CoA thiolases (KATs) and acetoacetyl-CoA thiolases (ACATs) encoded by five genes in Arabidopsis thaliana are targeted either to peroxisomes or cytosol but not to mitochondria. Plant Mol. Biol. 63, 97–108. doi: 10.1007/s11103-006-9075-1
Cenci, U., Qiu, H., Pillonel, T., Cardol, P., Remacle, C., Colleoni, C., et al. (2018). Host-pathogen biotic interactions shaped vitamin K metabolism in Archaeplastida. Sci. Rep. 8, 15243. doi: 10.1038/s41598-018-33663-w
Curtis, B. A., Tanifuji, G., Burki, F., Gruber, A., Irimia, M., Maruyama, S., et al. (2012). Algal genomes reveal evolutionary mosaicism and the fate of nucleomorphs. Nature 492, 59–65. doi: 10.1038/nature11681
Danscher, G. (1981). Localization of gold in biological tissue. Histochemistry 71, 81–88. doi: 10.1007/BF00592572
Davis, A., Abbriano, R., Smith, S. R., and Hildebrand, M. (2017). Clarification of photorespiratory processes and the role of malic enzyme in diatoms. Protist 168, 134–153. doi: 10.1016/j.protis.2016.10.005
Dean, J. M., and Lodhi, I. J. (2018). Structural and functional roles of ether lipids. Protein Cell 9, 196–206. doi: 10.1007/s13238-017-0423-5
Del Río, L. A. (2013). Peroxisomes and their Key Role in Cellular Signaling and Metabolism. Dordrecht: Springer Netherlands.
Dillon, S. C., and Bateman, A. (2004). The Hotdog fold: wrapping up a superfamily of thioesterases and dehydratases. BMC Bioinform. 5, 109. doi: 10.1186/1471-2105-5-109
Duve, C., and Baudhuin, P. (1966). Peroxisomes (microbodies and related particles). Physiol. Rev. 46, 323–357. doi: 10.1152/physrev.1966.46.2.323
Erdmann, R., and Schliebs, W. (2005). Peroxisomal matrix protein import: the transient pore model. Nat. Rev. Mol. Cell Biol. 6, 738–742. doi: 10.1038/nrm1710
Feng, W.-K., Wang, L., Lu, Y., and Wang, X.-Y. (2011). A protein oxidase catalysing disulfide bond formation is localized to the chloroplast thylakoids. FEBS J. 278, 3419–3430. doi: 10.1111/j.1742-4658.2011.08265.x
Gabaldón, T. (2010). Peroxisome diversity and evolution. Philos. Trans. R. Soc. Lond. B Biol. Sci. 365, 765–773. doi: 10.1098/rstb.2009.0240
Gabaldón, T., Snel, B., van Zimmeren, F., Hemrika, W., Tabak, H., and Huynen, M. A. (2006). Origin and evolution of the peroxisomal proteome. Biol. Direct. 1, 8. doi: 10.1186/1745-6150-1-8
Gaweska, H., and Fitzpatrick, P. F. (2011). Structures and mechanism of the monoamine oxidase family. Biomol. Concepts 2, 365–377. doi: 10.1515/BMC.2011.030
Ghosh, M. K., and Hajra, A. K. (1986). A rapid method for the isolation of peroxisomes from rat liver. Anal. Biochem. 159, 169–174. doi: 10.1016/0003-2697(86)90323-4
Gile, G. H., Moog, D., Slamovits, C. H., Maier, U. G., and Archibald, J. M. (2015). Dual Organellar Targeting of Aminoacyl-tRNA Synthetases in Diatoms and Cryptophytes. Genome Biol. Evol. 7, 1728–1742. doi: 10.1093/gbe/evv095
Gonzalez, N. H., Felsner, G., Schramm, F. D., Klingl, A., Maier, U.-G., and Bolte, K. (2011). A single peroxisomal targeting signal mediates matrix protein import in diatoms. PLoS ONE 6, e25316. doi: 10.1371/journal.pone.0025316
Gould, S. B., Sommer, M. S., Hadfi, K., Zauner, S., Kroth, P. G., and Maier, U.-G. (2006). Protein targeting into the complex plastid of cryptophytes. J. Mol. Evol. 62, 674–681. doi: 10.1007/s00239-005-0099-y
Gould, S. J., Keller, G.-A., Hosken, N., Wilkinsin, J., and Subramani, S. (1989). A conserved tripeptide sorts proteins to peroxisomes. J. Cell Biol. 108, 1657–1664. doi: 10.1083/jcb.108.5.1657
Gross, J., Cho, W. K., Lezhneva, L., Falk, J., Krupinska, K., Shinozaki, K., et al. (2006). A plant locus essential for phylloquinone (vitamin K1) biosynthesis originated from a fusion of four eubacterial genes. J. Biol. Chem. 281, 17189–17196. doi: 10.1074/jbc.M601754200
Gross, J., Meurer, J., and Bhattacharya, D. (2008). Evidence of a chimeric genome in the cyanobacterial ancestor of plastids. BMC Evol. Biol. 8, 117. doi: 10.1186/1471-2148-8-117
Gross, W. (1989). Intracellular localization of enzymes of fatty acid-beta-oxidation in the alga cyanidium caldarium. Plant Physiol. 91, 1476–1480. doi: 10.1104/pp.91.4.1476
Gross, W., Winkler, U., and Stabenau, H. (1985). Characterization of Peroxisomes from the Alga Bumilleriopsis filiformis. Plant Physiol. 77, 296–299. doi: 10.1104/pp.77.2.296
Hajra, A. K., and Bishop, J. E. (1982). Glycerolipid biosynthesis in peroxisomes via the acyl dihydroacetone phospahte pathway. Ann. N. Y. Acad. Sci. 386. doi: 10.1111/j.1749-6632.1982.tb21415.x
Hajra, A. K., and Das, A. K. (1996). Lipid Biosynthesis in Peroxisomes. Ann. N. Y. Acad. Sci. 804, 129–141. doi: 10.1111/j.1749-6632.1996.tb18613.x
Hauck, O. K., Scharnberg, J., Escobar, N. M., Wanner, G., Giavalisco, P., and Witte, C. P. (2014). Uric Acid Accumulation in an Arabidopsis Urate Oxidase Mutant Impairs Seedling Establishment by Blocking Peroxisome Maintenance. Plant Cell 26, 3090–3100. doi: 10.1105/tpc.114.124008
Hayashi, Y., Sato, N., Shinozaki, A., and Watanabe, M. (2015). Increase in peroxisome number and the gene expression of putative glyoxysomal enzymes in Chlamydomonas cells supplemented with acetate. J. Plant Res. 128, 177–185. doi: 10.1007/s10265-014-0681-8
Hayashi, Y., and Shinozaki, A. (2012). Visualization of microbodies in Chlamydomonas reinhardtii. J. Plant Res. 125, 579–586. doi: 10.1007/s10265-011-0469-z
Hu, J., Baker, A., Bartel, B., Linka, N., Mullen, R. T., Reumann, S., et al. (2012). Plant peroxisomes: biogenesis and function. Plant Cell 24, 2279–2303. doi: 10.1105/tpc.112.096586
Ishikawa, T., and Shigeoka, S. (2008). Recent advances in ascorbate biosynthesis and the physiological significance of ascorbate peroxidase in photosynthesizing organisms. Biosci. Biotechnol. Biochem. 72, 1143–1154. doi: 10.1271/bbb.80062
Jallet, D., Xing, D., Hughes, A., Moosburner, M., Simmons, M. P., Allen, A. E., et al. (2020). Mitochondrial fatty acid β-oxidation is required for storage-lipid catabolism in a marine diatom. New Phytol. 228, 946–958. doi: 10.1111/nph.16744
Kahn, K., and Tipton, P. A. (1998). Spectroscopic characterization of intermediates in the urate oxidase reaction. Biochemistry 37, 11651–11659. doi: 10.1021/bi980446g
Kato, N., Nelson, G., and Lauersen, K. J. (2021). Subcellular localizations of catalase and exogenously added fatty acid in chlamydomonas reinhardtii. Cells 10, 1940. doi: 10.3390/cells10081940
Kelley, L. A., Mezulis, S., Yates, C. M., Wass, M. N., and Sternberg, M. J. E. (2015). The Phyre2 web portal for protein modeling, prediction and analysis. Nat. Protoc. 10, 845–858. doi: 10.1038/nprot.2015.053
Kienle, N., Kloepper, T. H., and Fasshauer, D. (2016). Shedding light on the expansion and diversification of the Cdc48 protein family during the rise of the eukaryotic cell. BMC Evol. Biol. 16, 215. doi: 10.1186/s12862-016-0790-1
Kim, H. U., van Oostende, C., Basset, G. J. C., and Browse, J. (2008). The AAE14 gene encodes the Arabidopsis o-succinylbenzoyl-CoA ligase that is essential for phylloquinone synthesis and photosystem-I function. Plant J. 54, 272–283. doi: 10.1111/j.1365-313X.2008.03416.x
Kim, J.-J. P., and Miura, R. (2004). Acyl-CoA dehydrogenases and acyl-CoA oxidases: Structural basis fpr mechanistic similarities and differences. Eur. J. Biochem. 483–493. doi: 10.1046/j.1432-1033.2003.03948.x
Kim, P. K., and Hettema, E. H. (2015). Multiple pathways for protein transport to peroxisomes. J. Mol. Biol. 427, 1176–1190. doi: 10.1016/j.jmb.2015.02.005
Kirsch, J. F., Eichele, G., Ford, G. C., Vincent, M. G., Jansonius, J. N., Gehring, H., et al. (1984). Mechanism of action of aspartate aminotransferase proposed on the basis of its spatial structure. J. Mol. Biol. 174, 497–525. doi: 10.1016/0022-2836(84)90333-4
Kong, F., Burlacot, A., Liang, Y., Légeret, B., Alseekh, S., Brotman, Y., et al. (2018). Interorganelle communication: peroxisomal malate dehydrogenase2 connects lipid catabolism to photosynthesis through redox coupling in chlamydomonas. Plant Cell 30, 1824–1847. doi: 10.1105/tpc.18.00361
Kong, F., Liang, Y., Légeret, B., Beyly-Adriano, A., Blangy, S., Haslam, R. P., et al. (2017). Chlamydomonas carries out fatty acid β-oxidation in ancestral peroxisomes using a bona fide acyl-CoA oxidase. Plant J. 90, 358–371. doi: 10.1111/tpj.13498
Kroth, P. G., Chiovitti, A., Gruber, A., Martin-Jezequel, V., Mock, T., Parker, M. S., et al. (2008). A model for carbohydrate metabolism in the diatom Phaeodactylum tricornutum deduced from comparative whole genome analysis. PLoS ONE 3, e1426. doi: 10.1371/journal.pone.0001426
Kunze, M., and Berger, J. (2015). The similarity between N-terminal targeting signals for protein import into different organelles and its evolutionary relevance. Front. Physiol. 6, 259. doi: 10.3389/fphys.2015.00259
Lamberto, I., Percudani, R., Gatti, R., Folli, C., and Petrucco, S. (2010). Conserved alternative splicing of arabidopsis transthyretin-like determines protein localization and s-allantoin synthesis in peroxisomes. Plant Cell 1564–1574. doi: 10.1105/tpc.109.070102
Lauersen, K. J., Willamme, R., Coosemans, N., Joris, M., Kruse, O., and Remacle, C. (2016). Peroxisomal microbodies are at the crossroads of acetate assimilation in the green microalga Chlamydomonas reinhardtii. Algal Res. 16, 266–274. doi: 10.1016/j.algal.2016.03.026
Lee, J. R., Kim, S. Y., Chae, H. B., Jung, J. H., and Lee, S. Y. (2009). Antifungal activity of Saccharomyces cerevisiae peroxisomal 3-ketoacyl-CoA thiolase. BMB Rep. 42, 281–285. doi: 10.5483/BMBRep.2009.42.5.281
Ludewig-Klingner, A. K., Michael, V., Jarek, M., Brinkmann, H., and Petersen, J. (2018). Distribution and Evolution of Peroxisomes in Alveolates (Apicomplexa, Dinoflagellates, Ciliates). Genome Biol. Evol. 10, 1–13. doi: 10.1093/gbe/evx250
Marter, P., Schmidt, S., Kiontke, S., and Moog, D. (2020). Optimized mRuby3 is a Suitable Fluorescent Protein for in vivo Co-localization Studies with GFP in the Diatom Phaeodactylum tricornutum. Protist 171, 125715. doi: 10.1016/j.protis.2020.125715
McCarthy, J. K., Smith, S. R., McCrow, J. P., Tan, M., Zheng, H., Beeri, K., et al. (2017). Nitrate Reductase knockout uncouples nitrate transport from nitrate assimilation and drives repartitioning of carbon flux in a model pennate diatom. Plant Cell 29, 2047–2070. doi: 10.1105/tpc.16.00910
Meinecke, M., Cizmowski, C., Schliebs, W., Krüger, V., Beck, S., Wagner, R., et al. (2010). The peroxisomal importomer constitutes a large and highly dynamic pore. Nat. Cell Biol. 12, 273–277. doi: 10.1038/ncb2027
Michels, P. A. M., Moyersoen, J., Krazy, H., Galland, N., Herman, M., and Hannaert, V. (2005). Peroxisomes, glyoxysomes and glycosomes (review). Mol. Membr. Biol. 22, 133–145. doi: 10.1080/09687860400024186
Mix, A. K., Cenci, U., Heimerl, T., Marter, P., Wirkner, M. L., and Moog, D. (2018). Identification and localization of peroxisomal biogenesis proteins indicates the presence of peroxisomes in the cryptophyte guillardia theta and other “Chromalveolates”. Genome Biol. Evol. 10, 2834–2852. doi: 10.1093/gbe/evy214
Moog, D., Przyborski, J. M., and Maier, U. G. (2017). Genomic and proteomic evidence for the presence of a peroxisome in the apicomplexan parasite toxoplasma gondii and other coccidia. Genome Biol. Evol. 9, 3108–3121. doi: 10.1093/gbe/evx231
Neuberger, G., Maurer-Stroh, S., Eisenhaber, B., Hartig, A., and Eisenhaber, F. (2003a). Motif Refinement of the Peroxisomal Targeting Signal 1 and Evaluation of Taxon-specific Differences. J. Mol. Biol. 328, 567–579. doi: 10.1016/S0022-2836(03)00318-8
Neuberger, G., Maurer-Stroh, S., Eisenhaber, B., Hartig, A., and Eisenhaber, F. (2003b). Prediction of peroxisomal targeting signal 1 containing proteins from amino acid sequence. J. Mol. Biol. 328, 581–592. doi: 10.1016/S0022-2836(03)00319-X
Nishino, T., Okamoto, K., Eger, B. T., Pai, E. F., and Nishino, T. (2008). Mammalian xanthine oxidoreductase - mechanism of transition from xanthine dehydrogenase to xanthine oxidase. FEBS J. 275, 3278–3289. doi: 10.1111/j.1742-4658.2008.06489.x
Nötzel, C., Lingner, T., Klingenberg, H., and Thoms, S. (2016). Identification of New Fungal Peroxisomal Matrix Proteins and Revision of the PTS1 Consensus. Traffic 17, 1110–1124. doi: 10.1111/tra.12426
Osumi, T., Tsukamoto, T., Hata, S., Yokota, S., Miura, S., Fujiki, Y., et al. (1991). Amino-terminal presequnce of the precursor of peroxisomal 3-ketoacyl-CoA thiolasa is a vleavable signal peptide for peroxisomal targeting. Biochem. Biophys. Res. Commun. 181, 947–954. doi: 10.1016/0006-291X(91)92028-I
Paight, C., Slamovits, C. H., Saffo, M. B., and Lane, C. E. (2019). Nephromyces encodes a urate metabolism pathway and predicted peroxisomes, demonstrating that these are not ancient losses of apicomplexans. Genome Biol. Evol. 11, 41–53. doi: 10.1093/gbe/evy251
Park, J.-J., Wang, H., Gargouri, M., Deshpande, R. R., Skepper, J. N., Holguin, F. O., et al. (2015). The response of Chlamydomonas reinhardtii to nitrogen deprivation: a systems biology analysis. Plant J. 81, 611–624. doi: 10.1111/tpj.12747
Petriv, O. I., Tang, L., Titorenko, V. I., and Rachubinski, R. A. (2004). A new definition for the consensus sequence of the peroxisome targeting signal type 2. J. Mol. Biol. 341, 119–134. doi: 10.1016/j.jmb.2004.05.064
Pieuchot, L., and Jedd, G. (2012). Peroxisome assembly and functional diversity in eukaryotic microorganisms. Annu. Rev. Microbiol., 237–263. doi: 10.1146/annurev-micro-092611-150126
Platta, H. W., and Erdmann, R. (2007). Peroxisomal dynamics. Trends Cell Biol. 17, 474–484. doi: 10.1016/j.tcb.2007.06.009
Platta, H. W., Hagen, S., Reidick, C., and Erdmann, R. (2014). The peroxisomal receptor dislocation pathway: to the exportomer and beyond. Biochimie 98, 16–28. doi: 10.1016/j.biochi.2013.12.009
Poirier, Y., Antonenkov, V. D., Glumoff, T., and Hiltunen, J. K. (2006). Peroxisomal beta-oxidation–a metabolic pathway with multiple functions. Biochim. Biophys. Acta 1763, 1413–1426. doi: 10.1016/j.bbamcr.2006.08.034
Prasad, D. P. V. (1983). Hypoxanthine and allantoin as nitrogen sources for the growth of some frechwater green algae. New Phytol. 83, 575–580. doi: 10.1111/j.1469-8137.1983.tb02708.x
Reumann, S. (2004). Specification of the peroxisome targeting signals type 1 and type 2 of plant peroxisomes by bioinformatics analyses. Plant Physiol. 135, 783–800. doi: 10.1104/pp.103.035584
Reumann, S., Babujee, L., Ma, C., Wienkoop, S., Siemsen, T., Antonicelli, G. E., et al. (2007). Proteome analysis of arabidopsis leaf peroxisomes reveals novel targeting peptides, metabolic pathways, and defense mechanisms. Plant Cell 19, 3170–3193. doi: 10.1105/tpc.107.050989
Reumann, S., and Bartel, B. (2016). Plant peroxisomes: recent discoveries in functional complexity, organelle homeostasis, and morphological dynamics. Curr. Opin. Plant Biol. 34, 17–26. doi: 10.1016/j.pbi.2016.07.008
Reumann, S., Buchwald, D., and Lingner, T. (2012). PredPlantPTS1: a web server for the prediction of plant peroxisomal proteins. Front. Plant Sci. 3, 194. doi: 10.3389/fpls.2012.00194
Reumann, S., Chowdhary, G., and Lingner, T. (2016). Characterization, prediction and evolution of plant peroxisomal targeting signals type 1 (PTS1s). Biochim. Biophys. Acta 1863, 790–803. doi: 10.1016/j.bbamcr.2016.01.001
Reumann, S., Quan, S., Aung, K., Yang, P., Manandhar-Shrestha, K., Holbrook, D., et al. (2009). In-depth proteome analysis of arabidopsis leaf peroxisomes combined with in vivo subcellular targeting verification indicates novel metabolic and regulatory functions of peroxisomes. Plant Physiol. 150, 125–143. doi: 10.1104/pp.109.137703
Robin, G. P., Kleemann, J., Neumann, U., Cabre, L., Dallery, J. F., Lapalu, N., et al. (2018). Subcellular localization screening of colletotrichum higginsianum effector candidates identifies fungal proteins targeted to plant peroxisomes, golgi bodies, and microtubules. Front. Plant Sci. 9, 562. doi: 10.3389/fpls.2018.00562
Rucktäschel, R., Girzalsky, W., and Erdmann, R. (2011). Protein import machineries of peroxisomes. Biochim. Biophys. Acta 1808, 892–900. doi: 10.1016/j.bbamem.2010.07.020
Schliebs, W., Girzalsky, W., and Erdmann, R. (2010). Peroxisomal protein import and ERAD: variations on a common theme. Nat. Rev. Mol. Cell Biol. 11, 885–890. doi: 10.1038/nrm3008
Schlüter, A., Fourcade, S., Ripp, R., Mandel, J. L., Poch, O., and Pujol, A. (2006). The evolutionary origin of peroxisomes: an ER-peroxisome connection. Mol. Biol. Evol. 23, 838–845. doi: 10.1093/molbev/msj103
Schrader, M., and Fahimi, H. D. (2008). The peroxisome: still a mysterious organelle. Histochem. Cell Biol. 129, 421–440. doi: 10.1007/s00418-008-0396-9
Shigeoka, S., Ishikawa, T., Tamoi, M., Miyagawa, Y., Takeda, T., Yabuta, Y., et al. (2002). Regulation and Function of Ascorbate Peroxidase Isoenzymes. J. Exp. Bot. 53, 1305–1319. doi: 10.1093/jexbot/53.372.1305
Smith, J. J., and Aitchison, J. D. (2013). Peroxisomes take shape. Nat. Rev. Mol. Cell. Biol. 14, 803–817. doi: 10.1038/nrm3700
Stabenau, H., Winkler, U., and Säftel, W. (1984). Enzymes of β-Oxidation in Different Types of Algal Microbodies. Plant Physiol. 531–533. doi: 10.1104/pp.75.3.531
Stabenau, H., Winkler, U., and Säftel, W. (1989). Compartmentalization of peroxisomal enzymes in algae of the group of prasinophyceae. Plant Physiol. 754–759. doi: 10.1104/pp.90.2.754
Stehlik, T., Kremp, M., Kahnt, J., Bölker, M., and Freitag, J. (2020). Peroxisomal targeting of a protein phosphatase type 2C via mitochondrial transit. Nat. Commun. 11, 2355. doi: 10.1038/s41467-020-16146-3
Sweetlove, L. J., Beard, K. F. M., Nunes-Nesi, A., Fernie, A. R., and Ratcliffe, R. G. (2010). Not just a circle: flux modes in the plant TCA cycle. Trends Plant Sci. 15, 462–470. doi: 10.1016/j.tplants.2010.05.006
Swinkels, B. W., Gould, S. J., Bodnar, A. G., Rachubinski, R. A., and Subramani, S. (1991). A novel, cleavable peroxisomal targeting signal at the amino-terminus of the rat 3-ketoacyl-CoA thiolase. EMBO J. 10, 3255–3262. doi: 10.1002/j.1460-2075.1991.tb04889.x
Tamaki, S., Mochida, K., and Suzuki, K. (2021). Diverse biosynthetic pathways and protective functions against environmental stress of antioxidants in microalgae. Plants (Basel) 10, 1250. doi: 10.3390/plants10061250
Tie, J.-K., and Stafford, D. W. (2008). Structure and Function of Vitamin K Epoxide reductase: Vitamins and Hormones. Academic Press, 103–130. doi: 10.1016/S0083-6729(07)00006-4
Todd, C. D., Tipton, P. A., Blevins, D. G., Piedras, P., Pineda, M., and Polacco, J. C. (2006). Update on ureide degradation in legumes. J. Exp. Bot. 57, 5–12. doi: 10.1093/jxb/erj013
Toney, M. D. (2014). Aspartate aminotransferase: an old dog teaches new tricks. Arch Biochem. Biophys. 544, 119–127. doi: 10.1016/j.abb.2013.10.002
Tronconi, M. A., Fahnenstich, H., Gerrard Weehler, M. C., Andreo Carlos, S., Flügge, U. I., Drincovich, M. F., et al. (2008). Arabidopsis NAD-Malic Enzyme Functions as a Homodimer and Heterodimer and Has a Major Impact on Nocturnal Metabolism. Plant Physiol. 146, 1540–1552. doi: 10.1104/pp.107.114975
Wanders, R. J. A., and Brites, P. (2010). Biosynthesis of ether-phospholipids including plasmalogens, peroxisomes and human disease: new insights into an old problem. Clin. Lipidol. 5, 379–386. doi: 10.2217/clp.10.16
Widhalm, J. R., Ducluzeau, A. L., Buller, N. E., Elowsky, C. G., Olsen, L. J., and Basset, G. J. C. (2012). Phylloquinone (vitamin K(1)) biosynthesis in plants: two peroxisomal thioesterases of Lactobacillales origin hydrolyze 1,4-dihydroxy-2-naphthoyl-CoA. Plant J. 71, 205–215. doi: 10.1111/j.1365-313X.2012.04972.x
Widhalm, J. R., van Oostende, C., Furt, F., and Basset, G. J. C. (2009). A dedicated thioesterase of the Hotdog-fold family is required for the biosynthesis of the naphthoquinone ring of vitamin K1. Proc. Natl. Acad. Sci. U. S. A. 106, 5599–5603. doi: 10.1073/pnas.0900738106
Keywords: peroxisomes, Guillardia theta, cryptophytes, Phaeodactylum tricornutum, ROS detoxification, β-oxidation, peroxisomal metabolism
Citation: Vasilev J, Mix A-K, Heimerl T, Maier UG and Moog D (2022) Inferred Subcellular Localization of Peroxisomal Matrix Proteins of Guillardia theta Suggests an Important Role of Peroxisomes in Cryptophytes. Front. Plant Sci. 13:889662. doi: 10.3389/fpls.2022.889662
Received: 04 March 2022; Accepted: 12 May 2022;
Published: 16 June 2022.
Edited by:
Michael Hippler, University of Münster, GermanyReviewed by:
Kyle J. Lauersen, King Abdullah University of Science and Technology, Saudi ArabiaCopyright © 2022 Vasilev, Mix, Heimerl, Maier and Moog. This is an open-access article distributed under the terms of the Creative Commons Attribution License (CC BY). The use, distribution or reproduction in other forums is permitted, provided the original author(s) and the copyright owner(s) are credited and that the original publication in this journal is cited, in accordance with accepted academic practice. No use, distribution or reproduction is permitted which does not comply with these terms.
*Correspondence: Daniel Moog, ZGFuaWVsLm1vb2dAbXBpLW1hcmJ1cmcubXBnLmRl
†Present address: Daniel Moog, Max Planck Institute for Terrestrial Microbiology, Marburg, Germany
Disclaimer: All claims expressed in this article are solely those of the authors and do not necessarily represent those of their affiliated organizations, or those of the publisher, the editors and the reviewers. Any product that may be evaluated in this article or claim that may be made by its manufacturer is not guaranteed or endorsed by the publisher.
Research integrity at Frontiers
Learn more about the work of our research integrity team to safeguard the quality of each article we publish.