- Department of Biology, University of Fribourg, Fribourg, Switzerland
Despite its central role in the control of plant architecture, strigolactone has been recognized as a phytohormone only 15 years ago. Together with auxin, it regulates shoot branching in response to genetically encoded programs, as well as environmental cues. A central determinant of shoot architecture is apical dominance, i.e., the tendency of the main shoot apex to inhibit the outgrowth of axillary buds. Hence, the execution of apical dominance requires long-distance communication between the shoot apex and all axillary meristems. While the role of strigolactone and auxin in apical dominance appears to be conserved among flowering plants, the mechanisms involved in bud activation may be more divergent, and include not only hormonal pathways but also sugar signaling. Here, we discuss how spatial aspects of SL biosynthesis, transport, and sensing may relate to apical dominance, and we consider the mechanisms acting locally in axillary buds during dormancy and bud activation.
Introduction
Its central role in the regulation of shoot architecture is arguably the most conspicuous function of the phytohormone strigolactone (SL; Domagalska and Leyser, 2011; Barbier et al., 2019). Indeed, most of the information about SL biosynthesis and SL sensing comes from bushy mutants identified in forward genetic screens in thale cress (Arabidopsis thaliana), rice (Oryza sativa), petunia (Petunia hybrida), and pea (Pisum sativum) (reviewed in Al-Babili and Bouwmeester, 2015; Yoneyama and Brewer, 2021). Plant architecture is to a large degree defined by branching patterns, that is the number, position, and size of lateral branches. The extent of branching is controlled by the activity of the main shoot apex, which inhibits the outgrowth of axillary buds along the stem. Axillary meristems are initiated in all leaf axils (Wang et al., 2016b); however, they usually only initiate a few leaf primordia and then become dormant, until they are activated to grow out either in response to endogenous/exogenous developmental signals, or as a consequence of removal (or inactivation) of the main shoot apex. This phenomenon is known as apical dominance (AD; Phillips, 1975).
The central feature of AD is systemic correlative inhibition of bud outgrowth, which is under the control of auxin and SL, involving a mechanism known as auxin canalization (Crawford et al., 2010; Shinohara et al., 2013; Zhang et al., 2020). On the other hand, inducing signals such as cytokinin and sugars are involved in the activation of axillary buds (Domagalska and Leyser, 2011; Rameau et al., 2015; Barbier et al., 2019). While several excellent reviews discuss the function of SL in AD (Domagalska and Leyser, 2011; Rameau et al., 2015; Barbier et al., 2019), we focus here more on spatial aspects of SL biosynthesis and sensing, and on local downstream events in the buds required to inhibit bud outgrowth, and to trigger bud activation, respectively. Furthermore, we discuss parallels in meristem dormancy in annual versus perennial plants.
Selective Advantage of Branching and Apical Dominance
Plants with just a single shoot meristem suffer extinction if the meristem is damaged. For example, a palm tree infested with the red palm weevil (Rhynchophorus ferrugineus) cannot recover after its meristem has been consumed by the larvae (Al-Dosary et al., 2016). This can result in serious damage in infested date palm plantations (El-Sabea et al., 2009). Hence, having extra axillary meristems and multiple branches, as in most dicots, is an important selective advantage. However, shoot branching has to be kept in check to avoid shoot overgrowth and a relative depletion of root biomass (low root:shoot ratio), which would interfere with overall plant fitness. Plants have characteristic root:shoot ratios that are species-specific and genetically determined (Wilson, 1988), but root:shoot ratio can also change in response to environmental factors, such as light, nutrient status, and altitude (Körner and Renhardt, 1987; Shipley and Meziane, 2002).
These considerations highlight the importance of regulation of axillary bud outgrowth. AD contributes to focus the resources of the plant to one (or few) growth points, and eventually, to a limited number of fruits and seeds. Hence, plant fitness and reproductive success are tightly linked with the degree of AD (Aarssen, 1995; Lennartsson et al., 2018). However, the relationship is not simple, since removal of apical buds (experimental or by animal grazing) can either reduce reproductive success, because less fruits can be produced, or it leads to increased reproductive success due to the release of multiple axillary branches with inflorescences, which over-compensate the loss of flowers at the original apex (Aarssen, 1995). These findings raise interesting questions concerning the adaptive mechanisms that may have shaped the evolution of AD and the control of bud outgrowth, in particular in the context of its plasticity towards environmental and developmental factors (e.g., light, mineral nutrients, damage, developmental stage, etc.; Aarssen, 1995). In this context, it is interesting to note that some taxa have integrated the loss-of-apical meristem activity in their developmental programs during the evolution of sympodial branching patterns (Danert, 1958; Schmitz and Theres, 1999; Reinhardt and Kuhlemeier, 2002). Sympodial branching involves the programmed arrest of the apical meristem (often with the production of a terminal flower) and the outgrowth of axillary (lateral) meristems which have a defined life-span before they terminate themselves in a reiterative “stop-and-go” fashion. This sympodial branching pattern is characteristic for the inflorescences of the Solanaceae (Danert, 1958; Schmitz and Theres, 1999; Reinhardt and Kuhlemeier, 2002).
As a general rule, high AD is advantageous in densely populated environments, in which plants compete for nutrients and/or light, whereas harsh conditions (e.g., cold, heat, UV radiation, and strong wind) with scarce vegetation favor bushy shoots with low AD, as for example in alpine environments (Körner, 2003). Considering agricultural crops, strong AD is a favored trait in panicoid cereal crops (e.g., maize and millet), since it tends to increase yield per surface area of cultivated soil, and because simpler shoot architecture facilitates harvest (Doust, 2007). Maize is a prominent example which has been bred from bushy ancestors (the Mexican wild maize teosinte) to plants with a single main shoot axis (Yang et al., 2019). In some high-value vegetable and ornamental crops, e.g., tomato, cucumber, and Chrysanthemum, breeding for desired strong AD has not been achieved yet. Hence, their axillary branches have to be manually pruned (Navarrete and Jeannequin, 2000; Xi et al., 2015; Shen et al., 2019), because they would represent sinks that consume resources and cause yield losses. In contrast, low apical dominance (i.e., high branching) is a favored trait in pooid cereal crops, such as wheat, barley, and oat, in which intense tillering increases yield (Doust, 2007). In addition, crops that were bred for simultaneous fruit ripening, e.g., soybean (Tian et al., 2010) and cotton (McGarry et al., 2016), show decreased indeterminacy of the main shoot, usually associated with increased branching.
In many plant species, AD is more pronounced during vegetative development, while the onset of flowering coincides with a stimulation of bud outgrowth and increased branching (Hempel and Feldman, 1994; Beveridge et al., 2003; McSteen and Leyser, 2005; Rameau et al., 2015). An example for such a strategy is Arabidopsis, which does not branch during vegetative development, and which initiates a single main inflorescence at the time of bolting (Figure 1A). During the generative phase, several axillary/caulinary branches grow out (Hempel and Feldman, 1994; McSteen and Leyser, 2005), but always much fewer than there are axillary buds. An example of a plant with low AD is the alpine species Silene acaulis, which is adapted to harsh climate with strong winds and abundant snow fall (Figure 1B). Mutants with defective AD are highly branched and dwarfed (Beveridge et al., 2003; Snowden and Napoli, 2003; Domagalska and Leyser, 2011; Rameau et al., 2015), in case of petunia to the extent that flowering is delayed (Napoli, 1996; Figures 1C,D), conceivably as a result of resource diversion from the apical inflorescence meristem to the actively growing lateral branches.
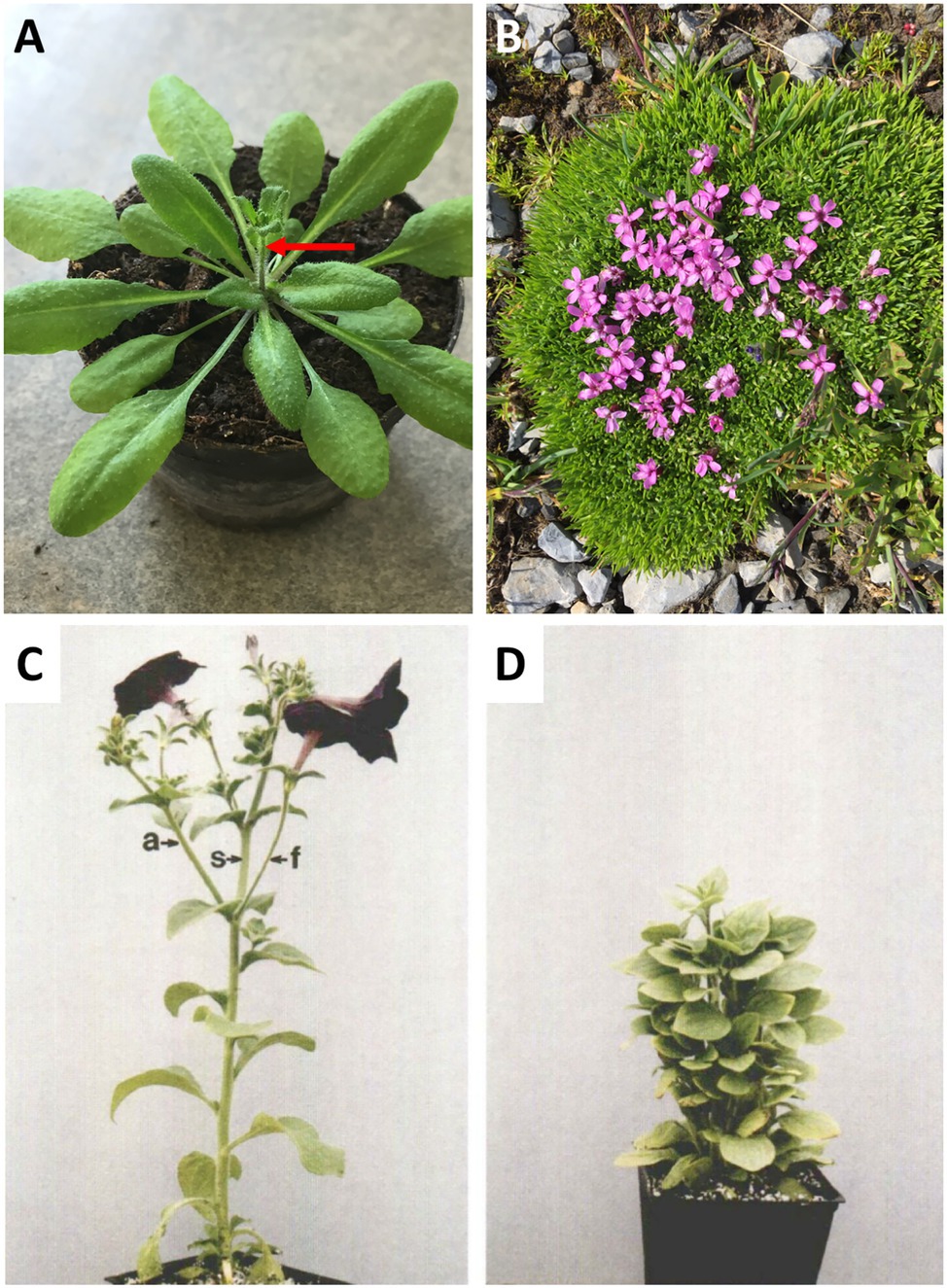
Figure 1. Apical dominance. (A) Apical dominance in the pioneer plant Arabidopsis thaliana at the onset of flowering. (B) The alpine plant Silene acaulis exhibits very low apical dominance. It exhibits a profusely branched shoot, in which no main shoot can be distinguished. (C,D) The model plant for apical dominance Petunia hybrida V26, and the isogenic mutant decreased apical dominance1 (dad1). The pleiotropic phenotype includes short stature, high branching, and late flowering (C,D, with permission from Napoli, 1996).
A Central Role for SL in Apical Dominance
A wealth of classical literature documents a central role for polar auxin transport (PAT) in AD and in the regulation of axillary bud outgrowth (Cline, 1991; Leyser, 2005; McSteen and Leyser, 2005). Auxin from apical tissues (in particular young leaves) is transported downward (basipetally) via PAT in xylem parenchyma cells, inhibiting bud outgrowth on the way through the stem, however, without entering the buds (Domagalska and Leyser, 2011). A well-founded theory of AD posits that PAT in the stem promotes AD by interfering with auxin canalization and export from the buds (Domagalska and Leyser, 2011). How the lack of auxin canalization is mechanistically related to growth arrest in the bud is not clear, but it may have to do with limited supply of signals and/or resources that would promote meristem activity in the bud. Alternatively, it may impinge on the cell cycle in order to attenuate meristem activity in the bud (Müller and Leyser, 2011). The identification of SL as a second inhibitory element in AD (Gomez-Roldan et al., 2008; Umehara et al., 2008) has raised the question how SL is linked with auxin action. The fact that mutants in either auxin or SL biology have strong defects in AD shows that the effects of the two phytohormones are not redundant. The currently available hypotheses for the action of SL are that it either inhibits auxin canalization from axillary buds to the main stem (by interfering with PAT), or that it directly inhibits bud outgrowth (Domagalska and Leyser, 2011). These hypotheses are not mutually exclusive, and several lines of evidence suggest that they are both valid (see below). Forward genetic screens in Arabidopsis, rice, pea, and petunia for mutants affected in shoot branching have led to the discovery of numerous genes encoding components of SL biosynthesis and signaling (reviewed in Al-Babili and Bouwmeester, 2015; Yoneyama and Brewer, 2021), and missing links in SL biosynthesis and sensing continue to be discovered (Wakabayashi et al., 2021). Taken together, these efforts document the prominent role of SL in apical dominance. The parallel work in these four model species showed how conserved SL biosynthesis and signaling is among flowering plants, and, on the other hand, revealed subtle species-specific differences. Importantly, the parallel approaches allowed to identify signaling elements that are genetically redundant in some of the species, and, therefore, evaded identification in forward mutant screens, as for example the duplicated MAX2 gene in petunia (Drummond et al., 2012), or the redundant SMAXL6, SMAXL7, and SMAXL8 in Arabidopsis (Soundappan et al., 2015). Additional evidence for the role of SL in branching came from crop species such as tomato and potato (Vogel et al., 2010; Pasare et al., 2013). Taken together, these findings substantiate the central and conserved role of SL in the regulation of shoot branching.
SL Transport Within the Plant: Identifying Sources and Targets of SL by Grafting
The action of auxin in AD is non-cell autonomous, since it is transported throughout the plant and acts on the buds indirectly (Domagalska and Leyser, 2011). Similarly, SL acts in a systemic fashion and can be transported over long distances in the plant (Mashiguchi et al., 2021). Compelling evidence for spatially separated sites of SL biosynthesis and action comes from grafting experiments with mutants that are defective in SL biosynthesis or sensing (Figure 2). Shoot-to-root grafting in Arabidopsis, petunia, and pea revealed that a wild-type root stock can establish normal AD in an SL-deficient mutant scion, indicative of acropetal SL transport from the root to the shoot (Beveridge, 2000; Booker et al., 2005; Dun et al., 2009; Waldie et al., 2014). Even a relatively small inter-graft between a mutant stock and a mutant scion was sufficient to restore AD to the mutant scion (Napoli, 1996; Simons et al., 2007; Hepworth, 2012) but not to the mutant stock, showing that SL transport is strictly unidirectional (Figures 2A,B; Foo et al., 2001; Simons et al., 2007). Although SL can be transported over long distances (from the root to the shoot), it is not clear whether this transport is required for AD. Wild-type scions grafted on SL-defective mutant stocks grow normally, showing that for AD, SL production in the shoot can be sufficient, at least in such grafts, implying that SL transport from the root may not be necessary for normal AD.
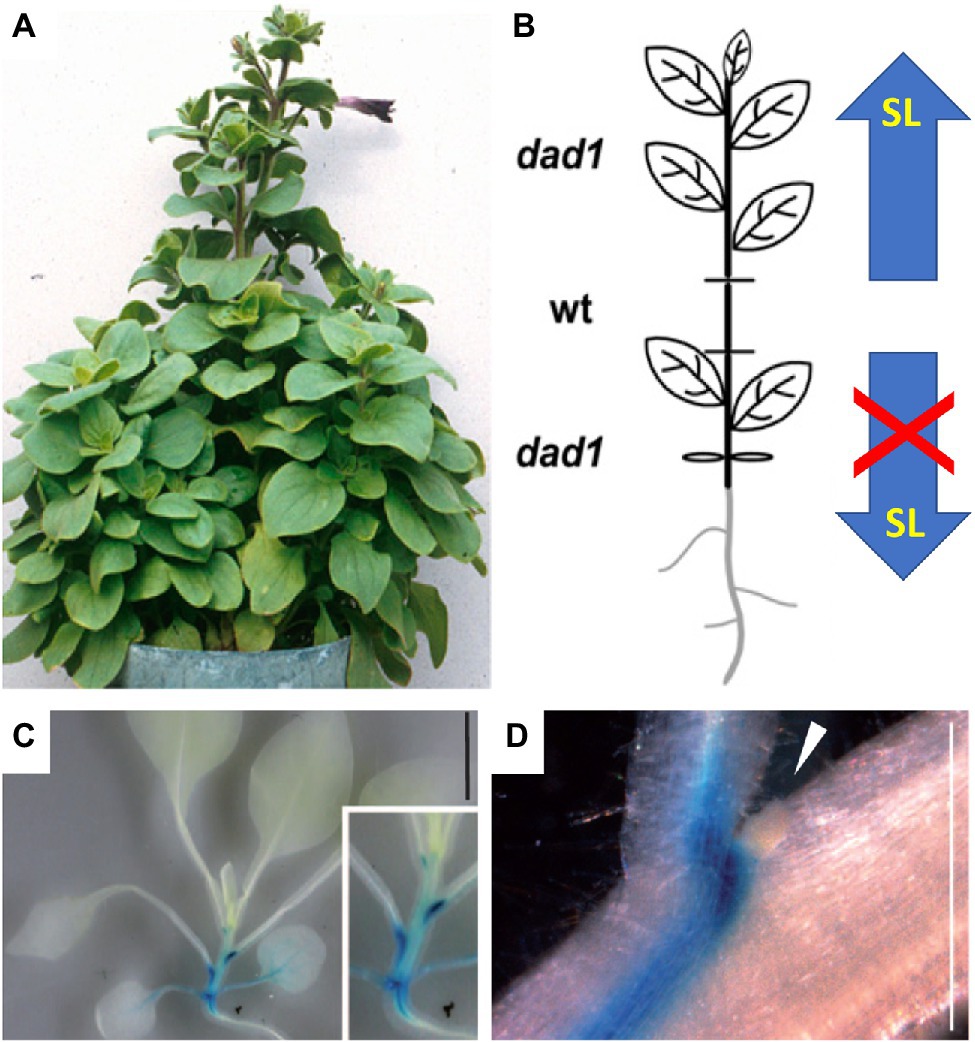
Figure 2. SL transport in the plant. (A,B) Grafting experiments have proven acropetal SL transport in the shoot. A small wild-type stem segment (wt) complements the dad1 phenotype in the scion (top), while the dad1 stock exhibits the non-complemented high branching phenotype (bottom); thus, only upward SL transport occurred. The scheme in (B) represents the organization of the grafted plant in (A). (C,D) Expression pattern of the cellular SL transporter pPhPDR1::GUS in the shoot of P. hybrida. Note highest expression just below the axillary buds, while the buds themselves [arrowhead in (D)] show no expression [modified with permission from Simons et al., 2007 (A,B) and Kretzschmar et al., 2012 (C,D)].
The fact that SL can be transported acropetally raises the question concerning the transport route. Root-to-shoot transport could proceed by mass flow with the transpiration stream in the xylem, or by cellular transport, as in the case of PAT (Petrasek and Friml, 2009; but in the reverse direction). Support for a xylem route of SL transport came from the detection of SL in xylem sap of Arabidopsis and tomato (Kohlen et al., 2011); however, these findings were not confirmed by subsequent work on various plant species, including Arabidopsis and tomato (Xie et al., 2015; see also below).
An alternative route of SL translocation is cell-to-cell transport in a way analogous to PAT (Borghi et al., 2016). Indeed, the first cellular SL transporter identified in P. hybrida (PDR1), an ABC transporter of the G-type subfamily, was shown to functionally contribute both to mycorrhizal symbiosis (by targeted secretion from the root), and to AD in the shoot (Kretzschmar et al., 2012). PDR1 is expressed in root and stem tissues (Kretzschmar et al., 2012), with highest levels at the nodes, next to the axillary buds (Figures 2C,D). PDR1 protein is localized to the plasma membrane, and, based on its expression pattern and loss-of-function mutant phenotype, is likely to function as an SL exporter (Kretzschmar et al., 2012). Pdr1 mutants exhibit premature bud outgrowth (Kretzschmar et al., 2012), indicating that SL transport to the buds contributes significantly to AD. However, long-distance transport of SL appears to be independent of PDR1 (Shiratake et al., 2019); thus, the mechanism of SL translocation from the root to the shoot remains unclear (Wheeldon and Bennett, 2021).
Spatial Regulation of SL-Biosynthetic Genes
Powerful tools to identify the sites of action of genes are promoter::reporter constructs (Jefferson et al., 1987; Chalfie et al., 1994) that show gene expression patterns with great spatial resolution. While fluorescent proteins are often the marker of choice because they allow identification in undisturbed live tissues with cellular resolution, they have the disadvantage that the optical permeability of live plant tissues is often limited, and, in addition, autofluorescence of many plant components (cell walls, secondary metabolites in vacuoles, etc.) considerably hampers their analysis. A widely used alternative is the use of enzymatic reporters such as the beta-glucuronidase gene (UidA), also known as the GUS gene, which generates (from the substrate X-gluc) a blue insoluble deposit (5,5′-dibrom-4,4′-dichlor-indigo), which is stable enough to allow for complete tissue clearing and embedding in paraffin or resin for sectioning. Importantly, cleared plant tissues have no blue background color, thus eliminating problems with endogenous background staining (Figures 2C,D).
Promoter::GUS analysis with the major SL-biosynthetic genes in Arabidopsis revealed that several of them are active in the vasculature (besides other sites of expression), and some of them (MAX3, MAX1; LBO) are expressed almost exclusively along vascular strands (Figure 3; Booker et al., 2005; Liang et al., 2011; Brewer et al., 2016). The expression of SL-biosynthetic genes along the vasculature was also found in rice for D27 (Lin et al., 2009) and CCD7 (Zou et al., 2006). Hence, it can be assumed that SL, or an SL precursor such as carlactone (Alder et al., 2012), is produced along the vascular system. Although all SL-biosynthetic genes are expressed mainly in the root, they also show expression in aerials tissues, in particular the stem, in Arabidopsis (Figure 3), as well as in other species (Zou et al., 2006; Drummond et al., 2009; Dun et al., 2009; Lin et al., 2009; Vogel et al., 2010; Pasare et al., 2013). This provides a plausible explanation for the fact that in Arabidopsis, petunia, and pea, wild-type scions grafted onto SL-defective mutant stocks are self-sufficient for SL production (Beveridge et al., 1994, 1996, 1997; Napoli, 1996; Morris et al., 2001; Turnbull et al., 2002; Sorefan et al., 2003; Booker et al., 2005; Simons et al., 2007; Drummond et al., 2009).
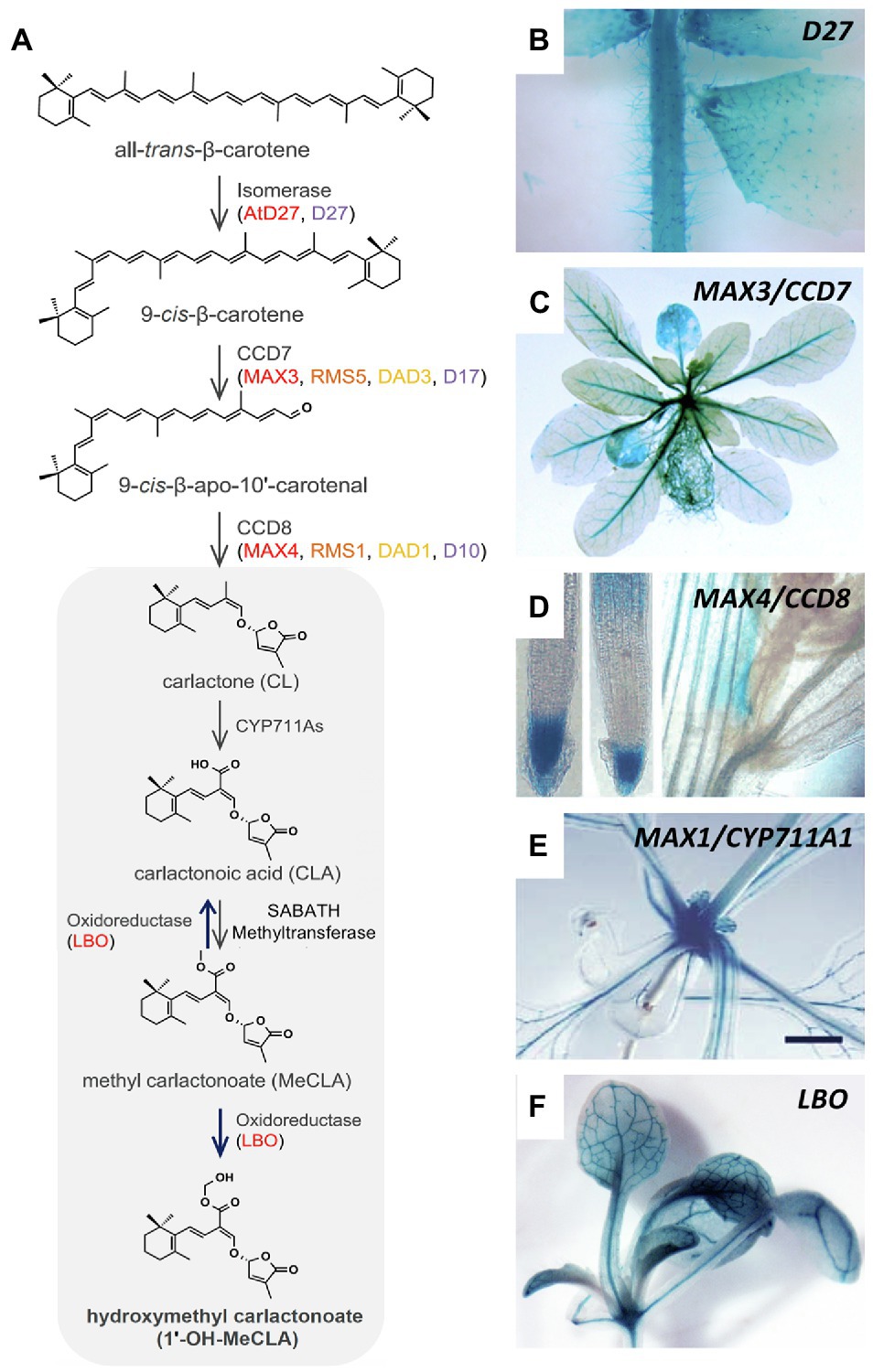
Figure 3. Expression pattern of SL-biosynthetic genes in Arabidopsis. (A) Biosynthetic pathway of strigolactone comprising the activity of the isomerase D27, the carotenoid cleavage dioxygenases CCD7 and CCD8, CYP711A, the oxidoreductase LBO, and the SABATH methyltransferase. (B–F) Expression pattern of Arabidopsis SL-biosynthetic genes such as D27 (B), CCD7/MAX3 (C), CCD8/MAX4 (D), CYP711A1/MAX1 (E), and LBO (F), as indicated, revealed by promoter::GUS analysis. Note prominent expression in the vasculature of MAX3, MAX1, and LBO [C,E,F; modified with permission from Yoneyama and Brewer, 2021 (A); Abuauf et al., 2018 (B); Liang et al., 2011 (C); Sorefan et al., 2003 (D); Booker et al., 2005 (E), and Brewer et al., 2016 (F)].
The vicinity of SL-biosynthetic gene expression to the xylem strands could explain why SL can be detected in the transpiration stream (Kohlen et al., 2011). SL produced along the vasculature could be loaded to the xylem by cellular transporters or by diffusive release from biosynthetic cells. It would then be continuously translocated to the shoot with the transpiration stream, even at low concentrations. While acropetal SL transport may not be essential for AD (see grafting experiments discussed above), SL transport through the xylem could represent a significant contribution to SL function in other aspects of shoot development, e.g., for the regulation of leaf senescence (Ueda and Kusaba, 2015).
Nutritional Control of Shoot Branching Impinges on the SL Pathway
In addition to the above-mentioned factors that influence AD, nutrients influence shoot architecture, since well-fertilized plants tend to branch more than nutritionally starved plants (Cline, 1991; Czarnecki et al., 2013; Wang et al., 2019; Hou et al., 2021). This effect can be explained, at least partially, by the fact that nutrients, in particular nitrogen (N) and phosphorus (P), impinge on the auxin- and SL-related mechanisms involved in AD (Sakakibara et al., 2006; Yoneyama and Brewer, 2021; Marro et al., 2022). High N status not only promotes branching, an effect that requires auxin and SL signaling (De Jong et al., 2014), but also involves the activation of cytokinin biosynthesis (Takei et al., 2002; Sakakibara et al., 2006; Xu et al., 2015). High P status represses SL-biosynthetic genes, consistent with the observation that SL secretion from the root system is repressed by P fertilization (Yoneyama et al., 2007a,b; Kohlen et al., 2011). P-replenished plants exhibit increased branching (Czarnecki et al., 2013; Wang et al., 2019), conceivably as a result of reduced SL biosynthesis (Umehara et al., 2010; Abuauf et al., 2018; Yoneyama and Brewer, 2021). In P-starved plants, SL biosynthesis is induced (Yoneyama and Brewer, 2021), presumably resulting in acropetal SL transport into the buds. Under these conditions, xylem transport of SL could become relevant (Kohlen et al., 2011), in particular since SL-biosynthetic genes are expressed along the vasculature (Figure 3). However, the role of the xylem in acropetal SL transport is a matter of debate (Xie et al., 2015), and the broad expression pattern of SL-biosynthetic genes throughout the shoot suggests that acropetal SL transport may not be necessary for apical dominance (see above).
Sites of SL Sensing and Consequences for AD
Interestingly, several SL-sensing genes (D14, MAX2, SMAXL6, SMAXL7, and SMAXL8) share the expression pattern along the vascular strands with SL-biosynthetic genes (Figure 4; Gao et al., 2004; Shen et al., 2007; Stirnberg et al., 2007; Chevalier et al., 2014; Soundappan et al., 2015; Song et al., 2022). In general, the identity of the cells that express SL-sensing genes along the vasculature is uncertain; however, for the SL receptor D14, expression was attributed to the phloem in Arabidopsis roots (Chevalier et al., 2014), and in the axillary buds of rice (Kameoka et al., 2016), suggesting that SL perception may be possible in these tissues. An association of SL-sensing genes with the vasculature is striking given the function of SL as inhibitor of PAT, which is located to the xylem parenchyma cells (Petrasek and Friml, 2009). Hence, SL perception in these cells would allow for a direct regulation of PAT in these cells. It will be important to identify the sites of SL sensing in more detail, with refined promoter::reporter studies, and with complementation experiments, in which SL-sensing genes are expressed in the respective mutant background under the control of cell-specific promoters. Further insight into SL sensing will come from fluorescent SL reporters, which allow to identify sites of high SL levels in living plant tissues with cellular resolution (Song et al., 2022).
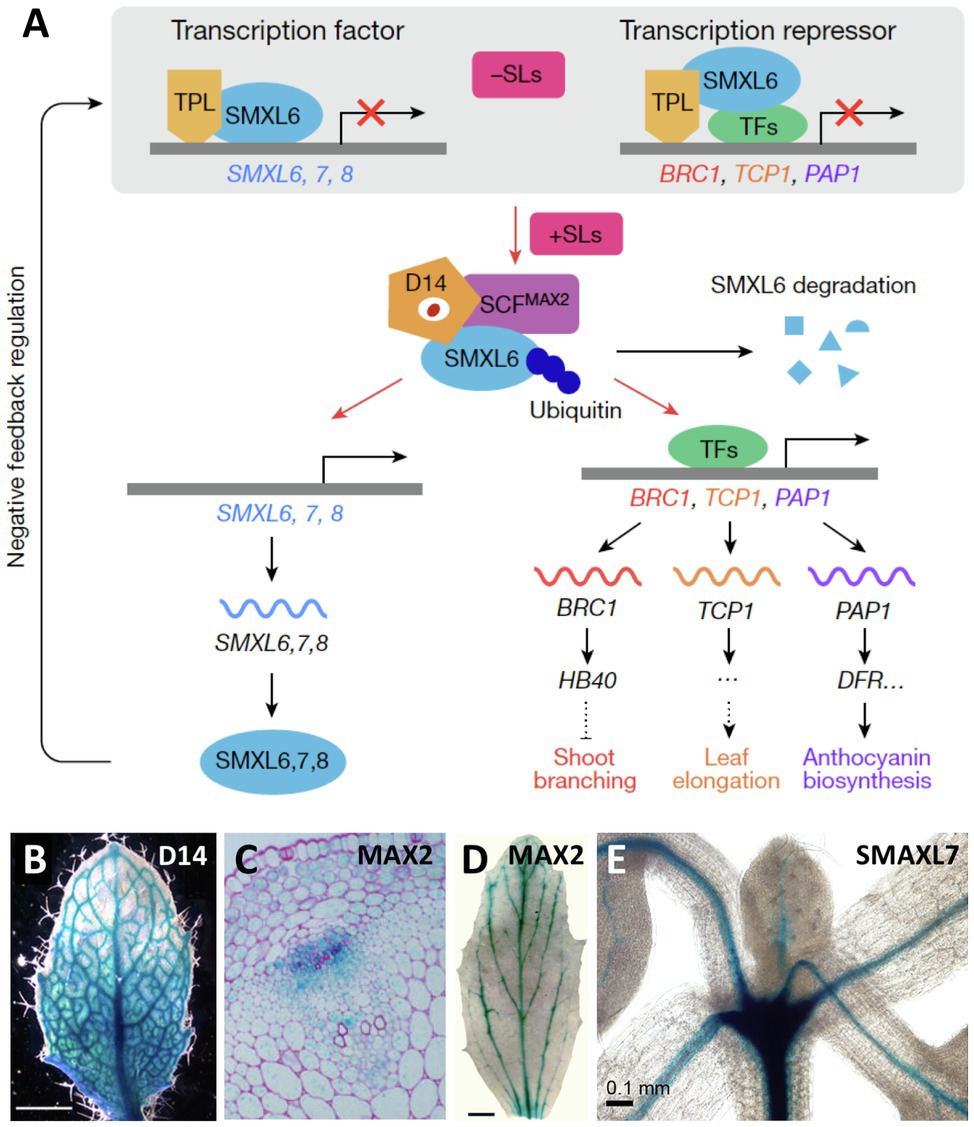
Figure 4. Expression pattern of SL-sensing genes in Arabidopsis. (A) Schematic representation of the elements involved in SL perception and signal transduction, including the SL receptor D14, the ubiquitin conjugating enzyme complex SCFMAX2 containing the F-box protein MAX2, and the redundantly acting transcriptional repressors SMAXL6, SMAXL7, and SMAXL8, which regulate the expression of target genes such as BRC1. (B–E) Expression pattern of the SL perception and signaling genes D14 (B), MAX2 (C,D), and D53/SMAXL7 (E), as indicated, revealed by promoter::GUS analysis. Note highest expression in vascular strands as revealed in whole-mount preparations (B,D,E), and in a transverse section of the stem [C; modified with permission from Wang et al., 2020 (A); Chevalier et al., 2014 (B); Stirnberg et al., 2007 (C); Shen et al., 2007 (D); and Soundappan et al., 2015 (E)].
A powerful tool to assign biological function to precisely defined cell populations is clonal analysis, in which the fate of genetically distinct cell lineages is followed in chimeras (Buckingham and Meilhac, 2011), a technique that has been pioneered in plants (Poethig, 1987). Clonal analysis has shown that SL perception in axillary buds acts locally (Stirnberg et al., 2007). Introduction of a mutation in the MAX2 gene encoding the F-BOX protein component of the SL-sensing machinery, comprising a sector with a single axillary bud, is sufficient to release its meristem from AD (Figures 5A,B). The fact that the surrounding wild-type tissues were not able to functionally complement the max2 defect in the axillary bud (Stirnberg et al., 2007) shows that SL perception acts locally to inhibit bud outgrowth. However, SL perception and sensing may not be entirely cell autonomous, since D14 was shown to be mobile over several cell diameters from the meristem base to the stem cells of axillary buds in rice (Kameoka et al., 2016), and was even graft-transmissible in pea (Beveridge et al., 1996). Furthermore, D14 protein was detected in phloem sap by proteomic analysis (Aki et al., 2008; Batailler et al., 2012), and by microscopic analysis of GFP-tagged D14 protein (Kameoka et al., 2016). Taken together, these results indicate that D14 protein is transported from cell to cell and by phloem transport (Chevalier et al., 2014; Kameoka et al., 2016; Barbier et al., 2019). This may allow D14 to function in the meristem proper of the axillary buds, in which the D14 promoter is not expressed (Kameoka et al., 2016).
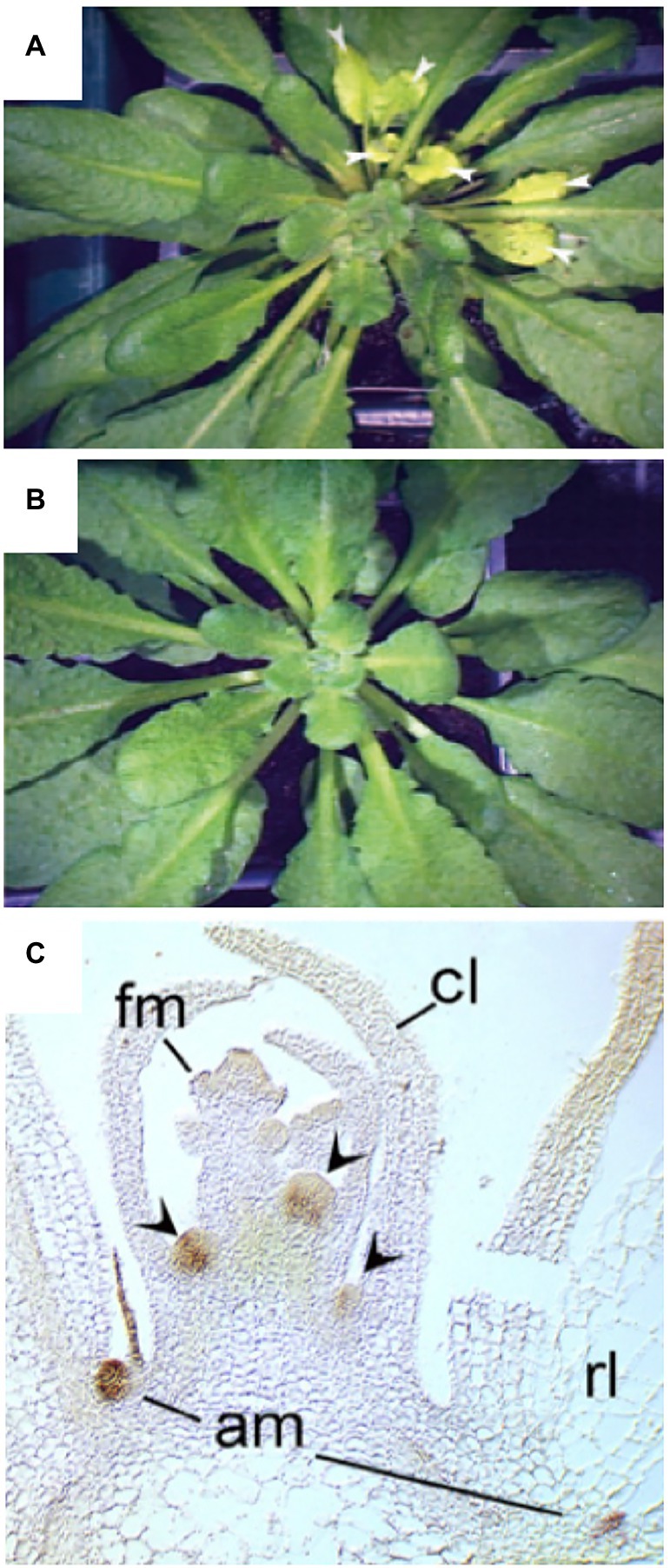
Figure 5. Local action of SL through MAX2 and BRC1 in leaf axillary meristems. (A,B) Clonal analysis with max2 mutant sectors reveals local function of MAX2 in axillary buds. A sector marked by chlorophyll deficiency (yellowish leaves) comprised a single axillary bud that grew out to produce numerous leaves (arrowheads), that are identified as a max2 mutant sector by the cosegregating genetic defect in chlorophyll biosynthesis (A). A wild-type control plant of the same age does not show bud outgrowth, nor yellowish leaves (B). (C) Expression analysis of BRC1 by in situ hybridization reveals expression in axillary meristems (am), but not in floral meristems (fm) at the shoot apex, cl, cauline leaf; rl, rosette leaf. Modified with permission from Stirnberg et al., 2007 (A,B), and Aguilar-Martinez et al., 2007 (C).
Which Pathways Act Downstream of SL to Prevent Bud Outgrowth?
While it is clear that auxin and SL impose dormancy on axillary buds, it is less clear how exactly growth and organogenesis is inhibited in the axillary meristems. Is the cell cycle attenuated? Are the meristems metabolically starved? Or is there an additional inhibitory principle involved? The auxin canalization model, including SL as a major player, can explain many aspects of correlative inhibition between the shoot tip and axillary buds, but how does it interfere––locally––with growth of the buds? On the other hand, the direct signaling model posits that SL prevents axillary growth through the inhibitory transcription factor BRANCHED1 (BRC1) in Arabidopsis (Aguilar-Martinez et al., 2007). BRC1 is functionally conserved in monocots and dicots (Aguilar-Martinez et al., 2007; Brewer et al., 2009; Finlayson et al., 2010). Interestingly, gain-of-function alleles of the BRC1 orthologue in maize, Teosinte branched1 (Tb1), have been selected for during domestication of modern maize for low branching (Dong et al., 2019a).
BRC1 is expressed at high levels in dormant buds (Figure 5C), and brc1 mutants exhibit excessive branching, consistent with a role of BRC1 in AD (Aguilar-Martinez et al., 2007). SL can activate BRC1 expression (Wang et al., 2019, 2020; Kerr et al., 2020, 2021), indicating that SL could act directly in the buds to inhibit bud outgrowth. Although BRC1 is probably not the only inhibitor of bud outgrowth (Seale et al., 2017), it is a conserved central player in Arabidopsis, pea, tomato, and maize (Martin-Trillo et al., 2011; Braun et al., 2012). Interestingly, TB1 homologues in cereals have functionally diversified to control ear architecture in crop-specific ways (Dong et al., 2019a). This is likely to reflect the particular development of reproductive structures in cereals (ears, tassels; Dong et al., 2019a), which represent highly branched generative shoot axes (Wang et al., 2021).
As a TCP-type transcription factor, BRC1 can be expected to act through activation (or repression) of downstream genes, which could provide a hint regarding the action mechanisms in AD. BRC1 itself is under transcriptional control by SL through the action of the transcription factors SMAXL6, SMAXL7, and SMAXL8, which promote bud outgrowth through inhibition of BRC1 expression (Figure 4A; Soundappan et al., 2015; Wang et al., 2020). BRC1 directly activates several homeobox proteins to mediate bud dormancy in Arabidopsis (Gonzalez-Grandio et al., 2017), and an orthologous transcriptional mechanism involving TB1 and GT1 controls branching in maize (Dong et al., 2019a). Hence, genetic evidence indicates that the molecular mechanism controlling bud dormancy may be conserved between monocots and dicots. How does the BRC1/TB1 nexus regulate branching? RNAseq and CHIPseq analysis in Arabidopsis showed that BRC1, in concert with several homeobox proteins, activates abscisic acid (ABA) biosynthesis by NCED3 in axillary buds (Gonzalez-Grandio et al., 2017). Similarly, TB1 acts through ABA to inhibit axillary buds in maize (Dong et al., 2019b). This includes activation of ABA biosynthetic genes in axillary buds during dormancy (Luo et al., 2019).
Interestingly, bud dormancy during the resting period (e.g., winter) in perennial plants such as poplar also involves ABA (Pan et al., 2021). The finding that BRC1 acts through ABA in axillary buds may explain the overlap between max2 phenotypes and ABA signaling in drought resistance (Bu et al., 2014) and in the resistance against bacterial pathogens (Piisilä et al., 2015). On the other hand, it is consistent with reports that have shown a role for ABA in the inhibition of axillary branching in Arabidopsis and maize (Cline and Oh, 2006; Yao and Finlayson, 2015; Cao et al., 2020). One might ask why then no ABA-related mutants were identified in screens for increased branching? ABA has numerous roles in plant development from seed dormancy to regulation of leaf transpiration and stress responses; therefore, ABA-deficient and ABA-insensitive mutants have rather pleiotropic phenotypes (Nambara and Marion-Poll, 2005; Cutler et al., 2010), which could potentially mask quantitative branching phenotypes. Nevertheless, ABA biosynthetic mutants such as nced3 and aba2 showed branching phenotypes in the context of phytochrome-dependent regulation of shoot branching (Reddy et al., 2013), a phenomenon that involves the canonical BRC1-dependent pathway (Gonzalez-Grandio et al., 2013).
How Are Buds Triggered to Grow Out When They Are Released From Dormancy?
Given the fact that bud dormancy is mediated by auxin and SL, it could be assumed that the activation of bud outgrowth (in response to environmental cues or after decapitation) may require simply the release from this inhibitory mechanism. Indeed, the highly branched mutant phenotypes of auxin-insensitive (Stirnberg et al., 1999) and SL-deficient (Beveridge et al., 2003; Domagalska and Leyser, 2011; Rameau et al., 2015) mutants show that the inactivation of auxin- and SL-mediated AD is sufficient to promote bud outgrowth. However, does this also apply to the rapid events triggered by decapitation? Several lines of evidence suggest that activation of dormant buds involves additional mechanisms independent of auxin and SL.
Cytokinin has long been known to promote growth of axillary branches in various plant species (Sachs and Thimann, 1967; Chatfield et al., 2000; Tanaka et al., 2006; Ferguson and Beveridge, 2009; Dun et al., 2012; Chen et al., 2013; Young et al., 2014), suggesting that it may contribute to bud activation following decapitation (Shimizu-Sato et al., 2009), or in response to favorable light conditions (Roman et al., 2016). Cytokinin biosynthesis is inhibited by auxin (Tanaka et al., 2006), while SL induces a CK-degrading oxidase (Duan et al., 2019), conversely, decapitation leads to the induction of CK biosynthetic genes and increased CK levels in the vicinity of the buds (Tanaka et al., 2006), consistent with a role of CK in bud activation (Shimizu-Sato et al., 2009; Müller et al., 2015).
However, CK may not be the first, and not the only element in bud activation. In pea, one of the first signs of bud activation can be observed after just a few hours from decapitation, long before changes in auxin transport and canalization can be expected to result in the release of buds, and before CK can have accumulated to induce bud outgrowth (Mason et al., 2014). This argues for the involvement of a rapid activating principle in bud activation. This signal has been assigned to sucrose and trehalose-6-phosphate (T6P), whose levels increase rapidly after decapitation (Fichtner et al., 2017, 2021; Barbier et al., 2019). In agreement with such a scenario, elegant recent work with cell-specific genetic manipulation of phloem transport (Paterlini et al., 2021) and sugar supply (Fichtner et al., 2021) indicated that sugars may indeed contribute to axillary bud activation in Arabidopsis. Since BRC1 expression is repressed by sugars (Mason et al., 2014; Barbier et al., 2015; Otori et al., 2019; Patil et al., 2022), a plausible model is that sugars contribute to bud outgrowth by attenuating BRC1-dependent dormancy (Wang et al., 2019). The role of sugars in bud activation is likely to represent a signaling function, since non-metabolizable sugars can mimic the effects of sucrose and T6P (Rabot et al., 2012; Barbier et al., 2015, 2021).
A connection between sugar activation and SL signaling has been revealed in rice, where sucrose interferes with SL signaling by repressing a component in SL perception (D3) and destabilizing the SL receptor D14, whereas their target D53, a promoter of bud outgrowth, is stabilized by sucrose, ultimately resulting in reduced expression of BRC1/TB1 (Patil et al., 2022). Similar effects were found in pea (Bertheloot et al., 2020; Patil et al., 2022), suggesting that the antagonistic action of sugars against SL signaling may be conserved in angiosperms. Ultimately, the release from BRC1/TB1, together with the induction of cytokinin levels (Müller and Leyser, 2011), results in the activation of the cell cycle and of basic cell metabolism (incl. protein synthesis and primary metabolism; Devitt and Stafstrom, 1995; Gonzalez-Grandio et al., 2013; Luo et al., 2019; Dong et al., 2019b), which are required to promote outgrowth and organogenesis in the axillary meristems (Müller and Leyser, 2011).
Is there a conflict between the models of bud inhibition (auxin canalization vs. direct SL-dependent inhibition), or between the mechanisms assumed to mediate bud activation (onset of local auxin canalization in the bud vs. sugar activation)? These alternative mechanisms are not necessarily mutually exclusive. The collective evidence shows that SL can promote AD both, locally in the buds (through BRC1), and systemically, by modulating auxin canalization (Figure 6). Similarly, bud activation could independently involve both sugar activation and the onset of auxin canalization and cytokinin accumulation in the bud (Figure 6). The relative importance, and the dynamics, of these processes may differ between plant species, to the extent that one or the other could become the dominating mechanism. It is plausible that in the rapidly responsive buds of decapitated pea, the first events are changes in sugar levels, while in Arabidopsis, this effect is less obvious. It is also possible that the sequence of events triggered by decapitation differs from the mechanisms involved in the slower bud activation conditions associated with developmental or nutritional changes (e.g., flowering or high P status).
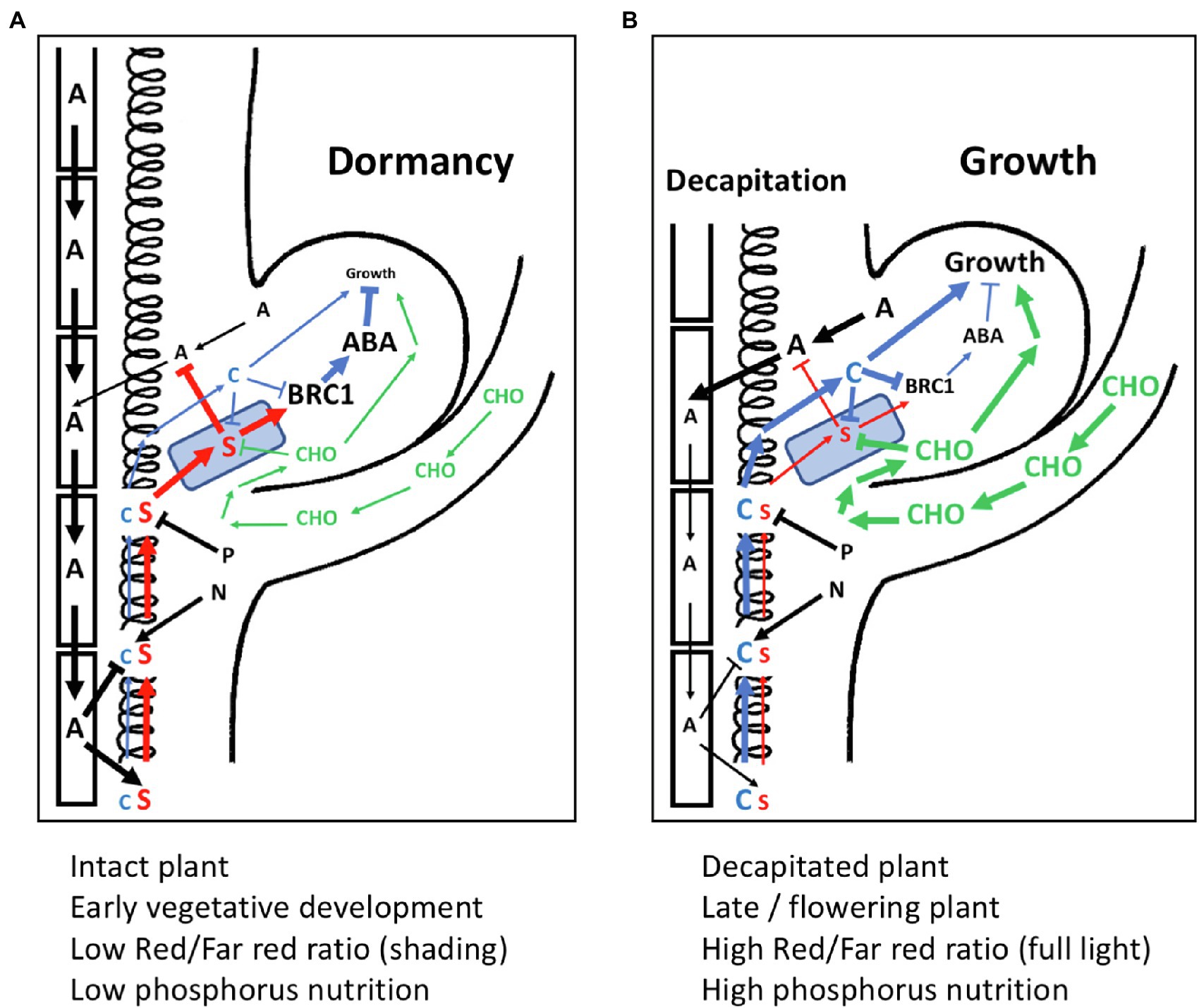
Figure 6. Model for the interactions of SL with other components in apical dominance. (A) An axillary bud under the influence of apical dominance with active polar auxin transport stream in the stem, which inhibits cytokinin (C) biosynthesis, and stimulates strigolactone (S) production. Strigolactone enters the buds via PDR1 transport (represented by a blue PDR1-expressing square). Strigolactone inhibits auxin canalization from the bud, and stimulates BRC1 gene expression. BRC1 activates the ABA dormancy program, resulting in growth arrest. The stimulatory effect of nitrogen (N) on cytokinin biosynthesis and the inhibition of strigolactone biosynthetic genes by phosphorus (P) are indicated, although they are at a steady-state intermediate level and do not influence bud activity. Relative signaling strength is represented by font size and thickness of the arrows. (B) Situation as in (A) depicting changes upon decapitation of the main shoot apex. Sugars (CHO) rapidly enter the bud, where they interfere with strigolactone sensing, and rapidly stimulate growth. Polar auxin transport in the stem is weak, reducing strigolactone biosynthesis and releasing the inhibition of cytokinin biosynthesis in the stem. Lower strigolactone levels allow canalization of auxin from the bud, while increased cytokinin levels further stimulate bud outgrowth by reducing BRC expression. Relative signaling strength is represented by font size and thickness of the arrows.
Competition Among Buds Kicks in via Auxin- and SL-Mediated Correlative Inhibition
Once a shoot is decapitated, numerous axillary meristems could potentially grow out. Even if not all of them are activated at the same time and with the same dynamics (depending, e.g., on their distance from the shoot tip), still several axillary buds may simultaneously be activated to grow. Hence, decapitation could potentially lead to bushy shoot phenotypes as in mutants with decreased AD (max, dad, rms, and dwarf). However, this is normally not the case, because the remaining buds are in mutual competition (Crawford et al., 2010; Shinohara et al., 2013; Balla et al., 2016; Paterlini et al., 2021), and often, one bud rapidly outcompetes all the others. Therefore, soon after decapitation, AD is reestablished resulting in a single new main shoot. It is plausible that this phenomenon is due to the rapid re-activation of correlative inhibition among the buds as a result of dominating auxin canalization in the new main shoot (Crawford et al., 2010; Shinohara et al., 2013; Balla et al., 2016; Paterlini et al., 2021). Hence, the SL-modulated auxin-based competition mechanism in AD is not only required to maintain axillary meristems in a silent state during normal development but also to quickly re-establish branching hierarchy after a disturbance (Domagalska and Leyser, 2011).
Activation of the Cell Cycle and Establishment of a Symplastic Conduit for Resource Supply: A Paradigm for the Evolutionary Origin of Bud Activation Mechanisms?
Bud dormancy is a common phenomenon in perennial plants that have to cope with periods of harsh environmental conditions (e.g., cold winters; Rohde and Bhalerao, 2007). Dormancy and bud induction have been studied particularly well in birch, hybrid aspen, and various fruit trees (Arora et al., 2003). The notion that, in annual plants, the activation of axillary buds upon decapitation involves inductive signals in addition to the release from AD, is paralleled by studies on bud activation in perennials (Rohde and Bhalerao, 2007). After a dormant phase during winter, such plants activate their meristems (including the most apical dormant buds) in spring (Arora et al., 2003). Although not directly comparable, the hypothesis that bud activation in annual plants, and the induction of the winter buds in perennials, may share common elements of regulation, has received substantial support (Rohde and Bhalerao, 2007).
The meristems of perennials in an inactive state during the winter period are comparable to silent axillary buds of annuals with strong AD. In both cases, the cell cycle is nearly arrested, and symplastic connectivity appears to be reduced, involving the accumulation of callose in the phloem and in plasmodesmata (Tylewicz et al., 2018). Notably, similar regulatory circuits are involved in bud dormancy of annuals and perennials, including ABA and BRC1 (Liu and Sherif, 2019; Maurya et al., 2020; Azeez et al., 2021; Pan et al., 2021). In addition, bud dormancy and sprouting of potato tubers appears to involve mechanisms related to AD in annuals (Sonnewald and Sonnewald, 2014). Hence, the regulation of bud dormancy and bud activation in annuals and perennials may involve a shared mechanism with a common evolutionary origin.
Interestingly, ABA is also a central element in seed dormancy (Finkelstein et al., 2008), indicating that seed dormancy and bud dormancy could be regulated by similar hormonal pathways (Ruttink et al., 2007; Wang et al., 2016a). This analogy extends to the notion that, as in seed dormancy (Skubacz and Daszkowska-Golec, 2017; Tuan et al., 2018), bud outgrowth in perennials involves antagonistic interactions of ABA and gibberellic acid (GA; Pan et al., 2021). However, the role of GA is complex and context-dependent (Katyayini et al., 2020; Pan et al., 2021). Indeed, GA can promote (Rinne et al., 2011, 2016; Ni et al., 2015; Katyayini et al., 2020) or inhibit (Scott et al., 1967; Zheng et al., 2018; Katyayini et al., 2020) bud outgrowth, depending on the plant species, and on the developmental and environmental conditions, but in most cases, GA contributes to bud activation (Liu and Sherif, 2019; Pan et al., 2021).
Conclusion
SL is produced in most parts of the plant, presumably along the vasculature, and it is mobile in an acropetal fashion by a mechanism that may involve the transpiration stream. The function of SL in AD acts at different levels of the plant. Attenuation of auxin transport capacity mediates bud dormancy by interfering with auxin canalization from the buds. In addition, SL can directly exert bud dormancy by inducing BRC1/TB1, and through the induction of ABA signaling. Bud release involves both inductive signals such as sucrose, T6P, and cytokinin, as well as the release from the inhibitory BRC1/TB1 and ABA. Ultimately, this results in the activation of the cell cycle and metabolism in the buds. Common patterns in the regulation of dormancy in axillary buds of annual plants, and in bud dormancy in perennials, suggest that the phenomena may be related.
Author Contributions
DR, KK, and FS discussed and wrote the manuscript. All authors contributed to the article and approved the submitted version.
Funding
The work has been funded by two grants from the Swiss National Science Foundation to DR (IZCSZ0 174608, 310030_200367), the COST action FA1206 (STREAM), and the “National Overseas Scholarship” to KK from the Indian Government.
Conflict of Interest
The authors declare that the research was conducted in the absence of any commercial or financial relationships that could be construed as a potential conflict of interest.
Publisher’s Note
All claims expressed in this article are solely those of the authors and do not necessarily represent those of their affiliated organizations, or those of the publisher, the editors and the reviewers. Any product that may be evaluated in this article, or claim that may be made by its manufacturer, is not guaranteed or endorsed by the publisher.
Abbreviations
SL, Strigolactone; AD, Apical dominance; ABA, Abscisic acid; BRC1, BRANCHED1; CK, Cytokinin; P, Phosphorus; N, Nitrogen; GA, Gibberellic acid.
References
Aarssen, L. W. (1995). Hypotheses for the evolution of apical dominance in plants—implications for the interpretation of overcompensation. Oikos 74, 149–156. doi: 10.2307/3545684
Abuauf, H., Haider, I., Jia, K. P., Ablazov, A., Mi, J. N., Blilou, I., et al. (2018). The Arabidopsis DWARF27 gene encodes an all-trans-/9-cis-beta-carotene isomerase and is induced by auxin, abscisic acid and phosphate deficiency. Plant Sci. 277, 33–42. doi: 10.1016/j.plantsci.2018.06.024
Aguilar-Martinez, J. A., Poza-Carrion, C., and Cubas, P. (2007). Arabidopsis BRANCHED1 acts as an integrator of branching signals within axillary buds. Plant Cell 19, 458–472. doi: 10.1105/tpc.106.048934
Aki, T., Shigyo, M., Nakano, R., Yoneyama, T., and Yanagisawa, S. (2008). Nano scale proteomics revealed the presence of regulatory proteins including three FT-like proteins in phloem and xylem saps from rice. Plant Cell Physiol. 49, 767–790. doi: 10.1093/pcp/pcn049
Al-Babili, S., and Bouwmeester, H. J. (2015). Strigolactones, a novel carotenoid-derived plant hormone. Annu. Rev. Plant Biol. 66, 161–186. doi: 10.1146/annurev-arplant-043014-114759
Alder, A., Jamil, M., Marzorati, M., Bruno, M., Vermathen, M., Bigler, P., et al. (2012). The path from beta-carotene to carlactone, a strigolactone-like plant hormone. Science 335, 1348–1351. doi: 10.1126/science.1218094
Al-Dosary, N. M. N., Al-Dobai, S., and Faleiro, J. R. (2016). Review on the management of red palm weevil Rhynchophorus ferrugineus olivier in date palm Phoenix dactylifera L. Emir. J. Food Agric. 28, 34–44. doi: 10.9755/ejfa.2015-10-897
Arora, R., Rowland, L. J., and Tanino, K. (2003). Induction and release of bud dormancy in woody perennials: a science comes of age. Hortscience 38, 911–921. doi: 10.21273/HORTSCI.38.5.911
Azeez, A., Zhao, Y. C., Singh, R. K., Yordanov, Y. S., Dash, M., Miskolczi, P., et al. (2021). EARLY BUD-BREAK 1 and EARLY BUD-BREAK 3 control resumption of poplar growth after winter dormancy. Nat. Commun. 12:1123. doi: 10.1038/s41467-021-21449-0
Balla, J., Medved’ova, Z., Kalousek, P., Matijescukova, N., Friml, J., Reinohl, V., et al. (2016). Auxin flow-mediated competition between axillary buds to restore apical dominance. Sci. Rep. 6:35955. doi: 10.1038/srep35955
Barbier, F. F., Cao, D., Fichtner, F., Weiste, C., Perez-Garcia, M. D., Caradeuc, M., et al. (2021). HEXOKINASE1 signalling promotes shoot branching and interacts with cytokinin and strigolactone pathways. New Phytol. 231, 1088–1104. doi: 10.1111/nph.17427
Barbier, F. F., Dun, E. A., Kerr, S. C., Chabikwa, T. G., and Beveridge, C. A. (2019). An update on the signals controlling shoot branching. Trends Plant Sci. 24, 220–236. doi: 10.1016/j.tplants.2018.12.001
Barbier, F., Peron, T., Lecerf, M., Perez-Garcia, M. D., Barriere, Q., Rolcik, J., et al. (2015). Sucrose is an early modulator of the key hormonal mechanisms controlling bud outgrowth in Rosa hybrida. J. Exp. Bot. 66, 2569–2582. doi: 10.1093/jxb/erv047
Batailler, B., Lemaitre, T., Vilaine, F., Sanchez, C., Renard, D., Cayla, T., et al. (2012). Soluble and filamentous proteins in Arabidopsis sieve elements. Plant Cell Environ. 35, 1258–1273. doi: 10.1111/j.1365-3040.2012.02487.x
Bertheloot, J., Barbier, F., Boudon, F., Perez-Garcia, M. D., Peron, T., Citerne, S., et al. (2020). Sugar availability suppresses the auxin-induced strigolactone pathway to promote bud outgrowth. New Phytol. 225, 866–879. doi: 10.1111/nph.16201
Beveridge, C. A. (2000). Long-distance signalling and a mutational analysis of branching in pea. Plant Growth Regul. 32, 193–203. doi: 10.1023/A:1010718020095
Beveridge, C. A., Ross, J. J., and Murfet, I. C. (1994). Branching mutant rms-2 in Pisum sativum—grafting studies and endogenous indole-3-acetic-acid levels. Plant Physiol. 104, 953–959. doi: 10.1104/pp.104.3.953
Beveridge, C. A., Ross, J. J., and Murfet, I. C. (1996). Branching in pea—action of genes rms3 and rms4. Plant Physiol. 110, 859–865. doi: 10.1104/pp.110.3.859
Beveridge, C. A., Symons, G. M., Murfet, I. C., Ross, J. J., and Rameau, C. (1997). The rms1 mutant of pea has elevated indole-3-acetic acid levels and reduced root-sap zeatin riboside content but increased branching controlled by graft-transmissible signal(s). Plant Physiol. 115, 1251–1258. doi: 10.1104/pp.115.3.1251
Beveridge, C. A., Weller, J. L., Singer, S. R., and Hofer, J. M. I. (2003). Axillary meristem development. Budding relationships between networks controlling flowering, branching, and photoperiod responsiveness. Plant Physiol. 131, 927–934. doi: 10.1104/pp.102.017525
Booker, J., Sieberer, T., Wright, W., Williamson, L., Willett, B., Stirnberg, P., et al. (2005). MAX1 encodes a cytochrome P450 family member that acts downstream of MAX3/4 to produce a carotenoid-derived branch-inhibiting hormone. Dev. Cell 8, 443–449. doi: 10.1016/j.devcel.2005.01.009
Borghi, L., Liu, G. W., Emonet, A., Kretzschmar, T., and Martinoia, E. (2016). The importance of strigolactone transport regulation for symbiotic signaling and shoot branching. Planta 243, 1351–1360. doi: 10.1007/s00425-016-2503-9
Braun, N., De Saint Germain, A., Pillot, J. P., Boutet-Mercey, S., Dalmais, M., Antoniadi, I., et al. (2012). The pea TCP transcription factor PsBRC1 acts downstream of strigolactones to control shoot branching. Plant Physiol. 158, 225–238. doi: 10.1104/pp.111.182725
Brewer, P. B., Dun, E. A., Ferguson, B. J., Rameau, C., and Beveridge, C. A. (2009). Strigolactone acts downstream of auxin to regulate bud outgrowth in pea and Arabidopsis. Plant Physiol. 150, 482–493. doi: 10.1104/pp.108.134783
Brewer, P. B., Yoneyama, K., Filardo, F., Meyers, E., Scaffidi, A., Frickey, T., et al. (2016). LATERAL BRANCHING OXIDOREDUCTASE acts in the final stages of strigolactone biosynthesis in Arabidopsis. Proc. Natl. Acad. Sci. U. S. A. 113, 6301–6306. doi: 10.1073/pnas.1601729113
Bu, Q. Y., Lv, T. X., Shen, H., Luong, P., Wang, J., Wang, Z. Y., et al. (2014). Regulation of drought tolerance by the F-Box protein MAX2 in Arabidopsis. Plant Physiol. 164, 424–439. doi: 10.1104/pp.113.226837
Buckingham, M. E., and Meilhac, S. M. (2011). Tracing cells for tracking cell lineage and clonal behavior. Dev. Cell 21, 394–409. doi: 10.1016/j.devcel.2011.07.019
Cao, D., Barbier, F., Yoneyama, K., and Beveridge, C. A. (2020). A rapid method for quantifying RNA and phytohormones from a small amount of plant tissue. Front. Plant Sci. 11:605069. doi: 10.3389/fpls.2020.605069
Chalfie, M., Tu, Y., Euskirchen, G., Ward, W. W., and Prasher, D. C. (1994). Green fluorescent protein as a marker for gene expression. Science 263, 802–805. doi: 10.1126/science.8303295
Chatfield, S. P., Stirnberg, P., Forde, B. G., and Leyser, O. (2000). The hormonal regulation of axillary bud growth in Arabidopsis. Plant J. 24, 159–169. doi: 10.1046/j.1365-313x.2000.00862.x
Chen, X. L., Zhou, X. Y., Xi, L., Li, J. X., Zhao, R. Y., Ma, N., et al. (2013). Roles of DgBRC1 in regulation of lateral branching in chrysanthemum (Dendranthema grandiflora cv. Jinba). Plos One 8:e61717. doi: 10.1371/journal.pone.0084522
Chevalier, F., Nieminen, K., Sanchez-Ferrero, J. C., Rodriguez, M. L., Chagoyen, M., Hardtke, C. S., et al. (2014). Strigolactone promotes degradation of DWARF14, an alpha/beta hydrolase essential for strigolactone signaling in Arabidopsis. Plant Cell 26, 1134–1150. doi: 10.1105/tpc.114.122903
Cline, M. G., and Oh, C. (2006). A reappraisal of the role of abscisic acid and its interaction with auxin in apical dominance. Ann. Bot. 98, 891–897. doi: 10.1093/aob/mcl173
Crawford, S., Shinohara, N., Sieberer, T., Williamson, L., George, G., Hepworth, J., et al. (2010). Strigolactones enhance competition between shoot branches by dampening auxin transport. Development 137, 2905–2913. doi: 10.1242/dev.051987
Cutler, S. R., Rodriguez, P. L., Finkelstein, R. R., and Abrams, S. R. (2010). “Abscisic acid: emergence of a core signaling network,” in Annual Review of Plant Biology. eds. S. Merchant, W. R. Briggs, and D. Ort, 651–679.
Czarnecki, O., Yang, J., Weston, D. J., Tuskan, G. A., and Chen, J. G. (2013). A dual role of strigolactones in phosphate acquisition and utilization in plants. Int. J. Mol. Sci. 14, 7681–7701. doi: 10.3390/ijms14047681
Danert, S. (1958). Die Verzweigung der Solanaceen im reproduktiven Bereich. Abh. Deutsch. Akad. Wiss. Berlin, Math.-Naturwiss. Kl. 1957 6, 1–184.
De Jong, M., George, G., Ongaro, V., Williamson, L., Willetts, B., Ljung, K., et al. (2014). Auxin and strigolactone signaling are required for modulation of Arabidopsis shoot branching by nitrogen supply. Plant Physiol. 166, 384–395. doi: 10.1104/pp.114.242388
Devitt, M. L., and Stafstrom, J. P. (1995). Cell-cycle regulation during growth-dormancy cycles in pea axillary buds. Plant Mol. Biol. 29, 255–265. doi: 10.1007/BF00043650
Domagalska, M. A., and Leyser, O. (2011). Signal integration in the control of shoot branching. Nat. Rev. Mol. Cell Biol. 12, 211–221. doi: 10.1038/nrm3088
Dong, Z. B., Alexander, M., and Chuck, G. (2019a). Understanding grass domestication through maize mutants. Trends Genet. 35, 118–128. doi: 10.1016/j.tig.2018.10.007
Dong, Z. B., Xiao, Y. G., Govindarajulu, R., Feil, R., Siddoway, M. L., Nielsen, T., et al. (2019b). The regulatory landscape of a core maize domestication module controlling bud dormancy and growth repression. Nat. Commun. 10:3810. doi: 10.1038/s41467-019-11774-w
Doust, A. N. (2007). Grass architecture: genetic and environmental control of branching. Curr. Opin. Plant Biol. 10, 21–25. doi: 10.1016/j.pbi.2006.11.015
Drummond, R. S. M., Martinez-Sanchez, N. M., Janssen, B. J., Templeton, K. R., Simons, J. L., Quinn, B. D., et al. (2009). Petunia hybrida CAROTENOID CLEAVAGE DIOXYGENASE7 is involved in the production of negative and positive branching signals in petunia. Plant Physiol. 151, 1867–1877. doi: 10.1104/pp.109.146720
Drummond, R. S. M., Sheehan, H., Simons, J. L., Martines-Sanchez, N. M., Turner, R. M., Putterill, J., et al. (2012). The expression of petunia strigolactone pathway genes is altered as part of the endogenous developmental program. Front. Plant Sci. 2:115. doi: 10.3389/fpls.2011.00115
Duan, J. B., Yu, H., Yuan, K., Liao, Z. G., Meng, X. B., Jing, Y. H., et al. (2019). Strigolactone promotes cytokinin degradation through transcriptional activation of CYTOKININ OXIDASE/DEHYDROGENASE9 in rice. Proc. Natl. Acad. Sci. U. S. A. 116, 14319–14324. doi: 10.1073/pnas.1810980116
Dun, E. A., De Saint Germain, A., Rameau, C., and Beveridge, C. A. (2012). Antagonistic action of strigolactone and cytokinin in bud outgrowth control. Plant Physiol. 158, 487–498. doi: 10.1104/pp.111.186783
Dun, E. A., Hanan, J., and Beveridge, C. A. (2009). Computational modeling and molecular physiology experiments reveal new insights into shoot branching in pea. Plant Cell 21, 3459–3472. doi: 10.1105/tpc.109.069013
El-Sabea, A. M. R., Faleiro, J. R., and Abo-El-Saad, M. M. (2009). The threat of red palm weevil Rhynchophorus ferrugineus to date plantations of the gulf region in the middle-east: an economic perspective. Outlooks Pest Manag. 20, 131–134. doi: 10.1564/20jun11
Ferguson, B. J., and Beveridge, C. A. (2009). Roles for auxin, cytokinin, and strigolactone in regulating shoot branching. Plant Physiol. 149, 1929–1944. doi: 10.1104/pp.109.135475
Fichtner, F., Barbier, F. F., Annunziata, M. G., Feil, R., Olas, J. J., Mueller-Roeber, B., et al. (2021). Regulation of shoot branching in Arabidopsis by trehalose 6-phosphate. New Phytol. 229, 2135–2151. doi: 10.1111/nph.17006
Fichtner, F., Barbier, F. F., Feil, R., Watanabe, M., Annunziata, M. G., Chabikwa, T. G., et al. (2017). Trehalose 6-phosphate is involved in triggering axillary bud outgrowth in garden pea (Pisum sativum L.). Plant J. 92, 611–623. doi: 10.1111/tpj.13705
Finkelstein, R., Reeves, W., Ariizumi, T., and Steber, C. (2008). Molecular aspects of seed dormancy. Annu. Rev. Plant Biol. 59, 387–415. doi: 10.1146/annurev.arplant.59.032607.092740
Finlayson, S. A., Krishnareddy, S. R., Kebrom, T. H., and Casal, J. J. (2010). Phytochrome regulation of branching in Arabidopsis. Plant Physiol. 152, 1914–1927. doi: 10.1104/pp.109.148833
Foo, E., Turnbull, C. G. N., and Beveridge, C. A. (2001). Long-distance signaling and the control of branching in the rms1 mutant of pea. Plant Physiol. 126, 203–209. doi: 10.1104/pp.126.1.203
Gao, L. L., Knogge, W., Delp, G., Smith, F. A., and Smith, S. E. (2004). Expression patterns of defense-related genes in different types of arbuscular mycorrhizal development in wild-type and mycorrhiza-defective mutant tomato. Mol. Plant Microbe Interact. 17, 1103–1113. doi: 10.1094/MPMI.2004.17.10.1103
Gomez-Roldan, V., Fermas, S., Brewer, P. B., Puech-Pagès, V., Dun, E. A., Pillot, J. P., et al. (2008). Strigolactone inhibition of shoot branching. Nature 455, 189–194. doi: 10.1038/nature07271
Gonzalez-Grandio, E., Pajoro, A., Franco-Zorrilla, J. M., Tarancon, C., Immink, R. G. H., and Cubas, P. (2017). Abscisic acid signaling is controlled by a BRANCHED1/HD-ZIP I cascade in Arabidopsis axillary buds. Proc. Natl. Acad. Sci. U. S. A. 114, E245–E254. doi: 10.1073/pnas.1613199114
Gonzalez-Grandio, E., Poza-Carrion, C., Sorzano, C. O. S., and Cubas, P. (2013). BRANCHED1 promotes axillary bud dormancy in response to shade in Arabidopsis. Plant Cell 25, 834–850. doi: 10.1105/tpc.112.108480
Hempel, F. D., and Feldman, L. J. (1994). Bidirectional inflorescence development in Arabidopsis thaliana—Acropetal initiation of flowers and basipetal initiation of paraclades. Planta 192, 276–286. doi: 10.1007/BF01089045
Hou, M. M., Wu, D. X., Li, Y., Tao, W. Q., Chao, L., and Zhang, Y. L. (2021). The role of auxin in nitrogen-modulated shoot branching. Plant Signal. Behav. 16:e1885888. doi: 10.1080/15592324.2021.1885888
Jefferson, R. A., Kavanagh, T. A., and Bevan, M. W. (1987). GUS fusions: ß-glucuronidase as a sensitive and versatile gene fusion marker in higher plants. EMBO J. 6, 3901–3907. doi: 10.1002/j.1460-2075.1987.tb02730.x
Kameoka, H., Dun, E. A., Lopez-Obando, M., Brewer, P. B., De Saint Germain, A., Rameau, C., et al. (2016). Phloem transport of the receptor DWARF14 protein is required for full function of strigolactones. Plant Physiol. 172, 1844–1852. doi: 10.1104/pp.16.01212
Katyayini, N. U., Rinne, P. L. H., Tarkowska, D., Strnad, M., and Van Der Schoot, C. (2020). Dual role of gibberellin in perennial shoot branching: inhibition and activation. Front. Plant Sci. 11:736 doi: 10.3389/fpls.2020.00736
Kerr, S. C., De Saint Germain, A., Dissanayanke, I. M., Mason, M. G., Dun, E. A., Beveridge, C. A., et al. (2020). Hormonal regulation of the BRC1-dependent strigolactone transcriptome involved in shoot branching responses. bioRxiv [Preprint].
Kerr, S. C., Patil, S. B., Germain, A. D., Pillot, J. P., Saffar, J., Ligerot, Y., et al. (2021). Integration of the SMXL/D53 strigolactone signalling repressors in the model of shoot branching regulation in Pisum sativum. Plant J. 107, 1756–1770. doi: 10.1111/tpj.15415
Kohlen, W., Charnikhova, T., Liu, Q., Bours, R., Domagalska, M. A., Beguerie, S., et al. (2011). Strigolactones are transported through the xylem and play a key role in shoot architectural response to phosphate deficiency in non-arbuscular mycorrhizal host arabidopsis. Plant Physiol. 155, 974–987. doi: 10.1104/pp.110.164640
Körner, C., and Renhardt, U. (1987). Dry-matter partitioning and root length leaf-area ratios in herbaceous perennial plants with diverse altitudinal distribution. Oecologia 74, 411–418. doi: 10.1007/BF00378938
Kretzschmar, T., Kohlen, W., Sasse, J., Borghi, L., Schlegel, M., Bachelier, J. B., et al. (2012). A petunia ABC protein controls strigolactone-dependent symbiotic signalling and branching. Nature 483, 341–346. doi: 10.1038/nature10873
Lennartsson, T., Ramula, S., and Tuomi, J. (2018). Growing competitive or tolerant? Significance of apical dominance in the overcompensating herb Gentianella campestris. Ecology 99, 259–269. doi: 10.1002/ecy.2101
Leyser, O. (2005). The fall and rise of apical dominance. Curr. Opin. Genet. Dev. 15, 468–471. doi: 10.1016/j.gde.2005.06.010
Liang, Y. S., Jeon, Y. A., Lim, S. H., Kim, J. K., Lee, J. Y., Kim, Y. M., et al. (2011). Vascular-specific activity of the Arabidopsis carotenoid cleavage dioxygenase 7 gene promoter. Plant Cell Rep. 30, 973–980. doi: 10.1007/s00299-010-0999-1
Lin, H., Wang, R. X., Qian, Q., Yan, M. X., Meng, X. B., Fu, Z. M., et al. (2009). DWARF27, an iron-containing protein required for the biosynthesis of strigolactones, regulates rice tiller bud outgrowth. Plant Cell 21, 1512–1525. doi: 10.1105/tpc.109.065987
Liu, J. Y., and Sherif, S. M. (2019). Hormonal orchestration of bud dormancy cycle in deciduous woody perennials. Front. Plant Sci. 10:1136. doi: 10.3389/fpls.2019.01136
Luo, L., Takahashi, M., Kameoka, H., Qin, R. Y., Shiga, T., Kanno, Y., et al. (2019). Developmental analysis of the early steps in strigolactone-mediated axillary bud dormancy in rice. Plant J. 97, 1006–1021. doi: 10.1111/tpj.14266
Marro, N., Lidoy, J., Chico, M. A., Rial, C., Garcia, J., Varela, R. M., et al. (2022). Strigolactones: new players in the nitrogen-phosphorus signalling interplay. Plant Cell Environ. 45, 512–527. doi: 10.1111/pce.14212
Martin-Trillo, M., Grandio, E. G., Serra, F., Marcel, F., Rodriguez-Buey, M. L., Schmitz, G., et al. (2011). Role of tomato BRANCHED1-like genes in the control of shoot branching. Plant J. 67, 701–714. doi: 10.1111/j.1365-313X.2011.04629.x
Mashiguchi, K., Seto, Y., and Yamaguchi, S. (2021). Strigolactone biosynthesis, transport and perception. Plant J. 105, 335–350. doi: 10.1111/tpj.15059
Mason, M. G., Ross, J. J., Babst, B. A., Wienclaw, B. N., and Beveridge, C. A. (2014). Sugar demand, not auxin, is the initial regulator of apical dominance. Proc. Natl. Acad. Sci. U. S. A. 111, 6092–6097. doi: 10.1073/pnas.1322045111
Maurya, J. P., Miskolczi, P. C., Mishra, S., Singh, R. K., and Bhalerao, R. P. (2020). A genetic framework for regulation and seasonal adaptation of shoot architecture in hybrid aspen. Proc. Natl. Acad. Sci. U. S. A. 117, 11523–11530. doi: 10.1073/pnas.2004705117
McGarry, R. C., Prewitt, S. F., Culpepper, S., Eshed, Y., Lifschitz, E., and Ayre, B. G. (2016). Monopodial and sympodial branching architecture in cotton is differentially regulated by the Gossypium hirsutum SINGLE FLOWER TRUSS and SELF-PRUNING orthologs. New Phytol. 212, 244–258. doi: 10.1111/nph.14037
McSteen, P., and Leyser, O. (2005). Shoot branching. Annu. Rev. Plant Biol. 56, 353–374. doi: 10.1146/annurev.arplant.56.032604.144122
Morris, S. E., Turnbull, C. G. N., Murfet, I. C., and Beveridge, C. A. (2001). Mutational analysis of branching in pea. Evidence that Rms1 and Rms5 regulate the same novel signal. Plant Physiol. 126, 1205–1213. doi: 10.1104/pp.126.3.1205
Müller, D., and Leyser, O. (2011). Auxin, cytokinin and the control of shoot branching. Ann. Bot. 107, 1203–1212. doi: 10.1093/aob/mcr069
Müller, D., Waldie, T., Miyawaki, K., To, J. P. C., Melnyk, C. W., Kieber, J. J., et al. (2015). Cytokinin is required for escape but not release from auxin mediated apical dominance. Plant J. 82, 874–886. doi: 10.1111/tpj.12862
Nambara, E., and Marion-Poll, A. (2005). Abscisic acid biosynthesis and catabolism. Annu. Rev. Plant Biol. 56, 165–185. doi: 10.1146/annurev.arplant.56.032604.144046
Napoli, C. (1996). Highly branched phenotype of the petunia dad1-1 mutant is reversed by grafting. Plant Physiol. 111, 27–37. doi: 10.1104/pp.111.1.27
Navarrete, M., and Jeannequin, B. (2000). Effect of frequency of axillary bud pruning on vegetative growth and fruit yield in greenhouse tomato crops. Sci. Hortic. 86, 197–210. doi: 10.1016/S0304-4238(00)00147-3
Ni, J., Gao, C. C., Chen, M. S., Pan, B. Z., Ye, K. Q., and Xu, Z. F. (2015). Gibberellin promotes shoot branching in the perennial woody plant Jatropha curcas. Plant Cell Physiol. 56, 1655–1666. doi: 10.1093/pcp/pcv089
Otori, K., Tanabe, N., Tamoi, M., and Shigeoka, S. (2019). Sugar Transporter Protein 1 (STP1) contributes to regulation of the genes involved in shoot branching via carbon partitioning in Arabidopsis. Biosci. Biotech. Bioch. 83, 472–481. doi: 10.1080/09168451.2018.1550355
Pan, W. Q., Liang, J. H., Sui, J. J., Li, J. R., Liu, C., Xin, Y., et al. (2021). ABA and bud dormancy in perennials: current knowledge and future perspective. Genes 12:1635. doi: 10.3390/genes12101635
Pasare, S. A., Ducreux, L. J. M., Morris, W. L., Campbell, R., Sharma, S. K., Roumeliotis, E., et al. (2013). The role of the potato (Solanum tuberosum) CCD8 gene in stolon and tuber development. New Phytol. 198, 1108–1120. doi: 10.1111/nph.12217
Paterlini, A., Dorussen, D., Fichtner, F., Van Rongen, M., Delacruz, R., Vojnovic, A., et al. (2021). Callose accumulation in specific phloem cell types reduces axillary bud growth in Arabidopsis thaliana. New Phytol. 231, 516–523. doi: 10.1111/nph.17398
Patil, S. B., Barbier, F. F., Zhao, J. F., Zafar, S. A., Uzair, M., Sun, Y. L., et al. (2022). Sucrose promotes D53 accumulation and tillering in rice. New Phytol. 234, 122–136. doi: 10.1111/nph.17834
Petrasek, J., and Friml, J. (2009). Auxin transport routes in plant development. Development 136, 2675–2688. doi: 10.1242/dev.030353
Phillips, I. D. J. (1975). Apical dominance. Annu. Rev. Plant. Physiol. Plant. Mol. Biol. 26, 341–367. doi: 10.1146/annurev.pp.26.060175.002013
Piisilä, M., Keceli, M. A., Brader, G., Jakobson, L., Joesaar, I., Sipari, N., et al. (2015). The F-box protein MAX2 contributes to resistance to bacterial phytopathogens in Arabidopsis thaliana. BMC Plant Biol. 15:53. doi: 10.1186/s12870-015-0434-4
Poethig, R. S. (1987). Clonal analysis of cell lineage patterns in plant development. Am. J. Bot. 74, 581–594. doi: 10.1002/j.1537-2197.1987.tb08679.x
Rabot, A., Henry, C., Ben Baaziz, K., Mortreau, E., Azri, W., Lothier, J., et al. (2012). Insight into the role of sugars in bud burst under light in the rose. Plant Cell Physiol. 53, 1068–1082. doi: 10.1093/pcp/pcs051
Rameau, C., Bertheloot, J., Leduc, N., Andrieu, B., Foucher, F., and Sakr, S. (2015). Multiple pathways regulate shoot branching. Front. Plant Sci. 5:741. doi: 10.3389/fpls.2014.00741
Reddy, S. K., Holalu, S. V., Casal, J. J., and Finlayson, S. A. (2013). Abscisic acid regulates axillary bud outgrowth responses to the ratio of red to far-red light. Plant Physiol. 163, 1047–1058. doi: 10.1104/pp.113.221895
Reinhardt, D., and Kuhlemeier, C. (2002). Plant architecture. EMBO Rep. 3, 846–851. doi: 10.1093/embo-reports/kvf177
Rinne, P. L. H., Paul, L. K., Vahala, J., Kangasjarvi, J., and Van Der Schoot, C. (2016). Axillary buds are dwarfed shoots that tightly regulate GA pathway and GA-inducible 1,3-beta-glucanase genes during branching in hybrid aspen. J. Exp. Bot. 67, 5975–5991. doi: 10.1093/jxb/erw352
Rinne, P. L. H., Welling, A., Vahala, J., Ripel, L., Ruonala, R., Kangasjarvi, J., et al. (2011). Chilling of dormant buds hyperinduces FLOWERING LOCUS T and recruits GA-inducible 1,3-beta-glucanases to reopen signal conduits and release dormancy in populus. Plant Cell 23, 130–146. doi: 10.1105/tpc.110.081307
Rohde, A., and Bhalerao, R. P. (2007). Plant dormancy in the perennial context. Trends Plant Sci. 12, 217–223. doi: 10.1016/j.tplants.2007.03.012
Roman, H., Girault, T., Barbier, F., Peron, T., Brouard, N., Pencik, A., et al. (2016). Cytokinins are initial targets of light in the control of bud outgrowth. Plant Physiol. 172, 489–509. doi: 10.1104/pp.16.00530
Ruttink, T., Arend, M., Morreel, K., Storme, V., Rombauts, S., Fromm, J., et al. (2007). A molecular timetable for apical bud formation and dormancy induction in poplar. Plant Cell 19, 2370–2390. doi: 10.1105/tpc.107.052811
Sachs, T., and Thimann, K. V. (1967). The role of auxins and cytokinins in the release of buds from dominance. Am. J. Bot. 54, 136–144. doi: 10.1002/j.1537-2197.1967.tb06901.x
Sakakibara, H., Takei, K., and Hirose, N. (2006). Interactions between nitrogen and cytokinin in the regulation of metabolism and development. Trends Plant Sci. 11, 440–448. doi: 10.1016/j.tplants.2006.07.004
Schmitz, G., and Theres, K. (1999). Genetic control of branching in Arabidopsis and tomato. Curr. Opin. Plant Biol. 2, 51–55. doi: 10.1016/S1369-5266(99)80010-7
Scott, T. K., Case, D. B., and Jacobs, W. P. (1967). Auxin-gibberellin interaction in apical dominance. Plant Physiol. 42, 1329–1333. doi: 10.1104/pp.42.10.1329
Seale, M., Bennett, T., and Leyser, O. (2017). BRC1 expression regulates bud activation potential but is not necessary or sufficient for bud growth inhibition in Arabidopsis. Development 144, 1661–1673. doi: 10.1242/dev.145649
Shen, H., Luong, P., and Huq, E. (2007). The F-Box protein MAX2 functions as a positive regulator of photomorphogenesis in Arabidopsis. Plant Physiol. 145, 1471–1483. doi: 10.1104/pp.107.107227
Shen, J. J., Zhang, Y. Q., Ge, D. F., Wang, Z. Y., Song, W. Y., Gu, R., et al. (2019). CsBRC1 inhibits axillary bud outgrowth by directly repressing the auxin efflux carrier CsPIN3 in cucumber. Proc. Natl. Acad. Sci. U. S. A. 116, 17105–17114. doi: 10.1073/pnas.1907968116
Shimizu-Sato, S., Tanaka, M., and Mori, H. (2009). Auxin-cytokinin interactions in the control of shoot branching. Plant Mol. Biol. 69, 429–435. doi: 10.1007/s11103-008-9416-3
Shinohara, N., Taylor, C., and Leyser, O. (2013). Strigolactone can promote or inhibit shoot branching by triggering rapid depletion of the auxin efflux protein PIN1 from the plasma membrane. PLoS Biol. 11:e1001474. doi: 10.1371/journal.pbio.1001474
Shipley, B., and Meziane, D. (2002). The balanced-growth hypothesis and the allometry of leaf and root biomass allocation. Funct. Ecol. 16, 326–331. doi: 10.1046/j.1365-2435.2002.00626.x
Shiratake, K., Notaguchi, M., Makino, H., Sawai, Y., and Borghi, L. (2019). Petunia PLEIOTROPIC DRUG RESISTANCE 1 is a strigolactone short-distance transporter with long-distance outcomes. Plant Cell Physiol. 60, 1722–1733. doi: 10.1093/pcp/pcz081
Simons, J. L., Napoli, C. A., Janssen, B. J., Plummer, K. M., and Snowden, K. C. (2007). Analysis of the DECREASED APICAL DOMINANCE genes of petunia in the control of axillary branching. Plant Physiol. 143, 697–706. doi: 10.1104/pp.106.087957
Skubacz, A., and Daszkowska-Golec, A. (2017). “Seed dormancy: the complex process regulated by abscisic acid, gibberellins, and other phytohormones that makes seed germination work,” in Phytohormones—Signaling Mechanisms and Crosstalk in Plant Development and Stress Responses. ed. M. A. El-Esawi (London: Intech Open), 77–100.
Snowden, K. C., and Napoli, C. A. (2003). A quantitative study of lateral branching in petunia. Funct. Plant Biol. 30, 987–994. doi: 10.1071/FP03081
Song, C. Z., Zhao, J., Guichard, M., Shi, D. B., Grossmann, G., Schmitt, C., et al. (2022). Strigo-D2-a bio-sensor for monitoring spatio-temporal strigolactone signaling patterns in intact plants. Plant Physiol. 188, 97–110. doi: 10.1093/plphys/kiab504
Sonnewald, S., and Sonnewald, U. (2014). Regulation of potato tuber sprouting. Planta 239, 27–38. doi: 10.1007/s00425-013-1968-z
Sorefan, K., Booker, J., Haurogne, K., Goussot, M., Bainbridge, K., Foo, E., et al. (2003). MAX4 and RMS1 are orthologous dioxygenase-like genes that regulate shoot branching in Arabidopsis and pea. Genes Dev. 17, 1469–1474. doi: 10.1101/gad.256603
Soundappan, I., Bennett, T., Morffy, N., Liang, Y. Y., Stang, J. P., Abbas, A., et al. (2015). SMAX1-LIKE/D53 family members enable distinct MAX2-dependent responses to strigolactones and karrikins in Arabidopsis. Plant Cell 27, 3143–3159. doi: 10.1105/tpc.15.00562
Stirnberg, P., Chatfield, S. P., and Leyser, H. M. O. (1999). AXR1 acts after lateral bud formation to inhibit lateral bud growth in Arabidopsis. Plant Physiol. 121, 839–847. doi: 10.1104/pp.121.3.839
Stirnberg, P., Furner, I. J., and Leyser, H. M. O. (2007). MAX2 participates in an SCF complex which acts locally at the node to suppress shoot branching. Plant J. 50, 80–94. doi: 10.1111/j.1365-313X.2007.03032.x
Takei, K., Takahashi, T., Sugiyama, T., Yamaya, T., and Sakakibara, H. (2002). Multiple routes communicating nitrogen availability from roots to shoots: a signal transduction pathway mediated by cytokinin. J. Exp. Bot. 53, 971–977. doi: 10.1093/jexbot/53.370.971
Tanaka, M., Takei, K., Kojima, M., Sakakibara, H., and Mori, H. (2006). Auxin controls local cytokinin biosynthesis in the nodal stem in apical dominance. Plant J. 45, 1028–1036. doi: 10.1111/j.1365-313X.2006.02656.x
Tian, Z. X., Wang, X. B., Lee, R., Li, Y. H., Specht, J. E., Nelson, R. L., et al. (2010). Artificial selection for determinate growth habit in soybean. Proc. Natl. Acad. Sci. U. S. A. 107, 8563–8568. doi: 10.1073/pnas.1000088107
Tuan, P. A., Kumar, R., Rehal, P. K., Toora, P. K., and Ayele, B. T. (2018). Molecular mechanisms underlying abscisic acid/gibberellin balance in the control of seed dormancy and germination in cereals. Front. Plant Sci. 9:668. doi: 10.3389/fpls.2018.00668
Turnbull, C. G. N., Booker, J. P., and Leyser, H. M. O. (2002). Micrografting techniques for testing long-distance signalling in Arabidopsis. Plant J. 32, 255–262. doi: 10.1046/j.1365-313X.2002.01419.x
Tylewicz, S., Petterle, A., Marttila, S., Miskolczi, P., Azeez, A., Singh, R. K., et al. (2018). Photoperiodic control of seasonal growth is mediated by ABA acting on cell-cell communication. Science 360, 212–215. doi: 10.1126/science.aan8576
Ueda, H., and Kusaba, M. (2015). Strigolactone regulates leaf senescence in concert with ethylene in Arabidopsis. Plant Physiol. 169, 138–147. doi: 10.1104/pp.15.00325
Umehara, M., Hanada, A., Magome, H., Takeda-Kamiya, N., and Yamaguchi, S. (2010). Contribution of strigolactones to the inhibition of tiller bud outgrowth under phosphate deficiency in rice. Plant Cell Physiol. 51, 1118–1126. doi: 10.1093/pcp/pcq084
Umehara, M., Hanada, A., Yoshida, S., Akiyama, K., Arite, T., Takeda-Kamiya, N., et al. (2008). Inhibition of shoot branching by new terpenoid plant hormones. Nature 455, 195–200. doi: 10.1038/nature07272
Vogel, J. T., Walter, M. H., Giavalisco, P., Lytovchenko, A., Kohlen, W., Charnikhova, T., et al. (2010). SlCCD7 controls strigolactone biosynthesis, shoot branching and mycorrhiza-induced apocarotenoid formation in tomato. Plant J. 61, 300–311. doi: 10.1111/j.1365-313X.2009.04056.x
Wakabayashi, T., Yasuhara, R., Miura, K., Takikawa, H., Mizutani, M., and Sugimoto, Y. (2021). Specific methylation of (11R)-carlactonoic acid by an Arabidopsis SABATH methyltransferase. Planta 254:88. doi: 10.1007/s00425-021-03738-6
Waldie, T., Mcculloch, H., and Leyser, O. (2014). Strigolactones and the control of plant development: lessons from shoot branching. Plant J. 79, 607–622. doi: 10.1111/tpj.12488
Wang, D. L., Gao, Z. Z., Du, P. Y., Xiao, W., Tan, Q. P., Chen, X. D., et al. (2016a). Expression of ABA metabolism-related genes suggests similarities and differences between seed dormancy and bud dormancy of peach (Prunus persica). Front. Plant Sci. 6:1248. doi: 10.3389/fpls.2015.01248
Wang, Q., Hasson, A., Rossmann, S., and Theres, K. (2016b). Divide et impera: boundaries shape the plant body and initiate new meristems. New Phytol. 209, 485–498. doi: 10.1111/nph.13641
Wang, M., Le Moigne, M. A., Bertheloot, J., Crespel, L., Perez-Garcia, M. D., Oge, L., et al. (2019). BRANCHED1: A key hub of shoot branching. Front. Plant Sci. 10:1782. doi: 10.3389/fpls.2019.01782
Wang, L., Wang, B., Yu, H., Guo, H. Y., Lin, T., Kou, L. Q., et al. (2020). Transcriptional regulation of strigolactone signalling in Arabidopsis. Nature 583, 277–281. doi: 10.1038/s41586-020-2382-x
Wang, C. Y., Yang, X. J., and Li, G. (2021). Molecular insights into inflorescence meristem specification for yield potential in cereal crops. Int. J. Mol. Sci. 22:3508. doi: 10.3390/ijms22073508
Wheeldon, C. D., and Bennett, T. (2021). There and back again: An evolutionary perspective on long-distance coordination of plant growth and development. Semin. Cell Dev. Biol. 109, 55–67. doi: 10.1016/j.semcdb.2020.06.011
Wilson, J. B. (1988). A review of evidence on the control of shoot:root ratio, in relation to models. Ann. Bot. 61, 433–449. doi: 10.1093/oxfordjournals.aob.a087575
Xi, L., Wen, C., Fang, S., Chen, X. L., Nie, J., Chu, J. F., et al. (2015). Impacts of strigolactone on shoot branching under phosphate starvation in chrysanthemum (Dendranthema grandiflorum cv. Jinba). Front. Plant Sci. 6:694. doi: 10.3389/fpls.2015.00694
Xie, X., Yoneyama, K., Kisugi, T., Nomura, T., Akiyama, K., Asami, T., et al. (2015). Strigolactones are transported from roots to shoots, although not through the xylem. J. Pestic. Sci. 40, 214–216. doi: 10.1584/jpestics.D15-045
Xu, J. X., Zha, M. R., Li, Y., Ding, Y. F., Chen, L., Ding, C. Q., et al. (2015). The interaction between nitrogen availability and auxin, cytokinin, and strigolactone in the control of shoot branching in rice (Oryza sativa L.). Plant Cell Rep. 34, 1647–1662. doi: 10.1007/s00299-015-1815-8
Yang, C. J., Samayoa, L. F., Bradbury, P. J., Olukolu, B. A., Xue, W., York, A. M., et al. (2019). The genetic architecture of teosinte catalyzed and constrained maize domestication. Proc. Natl. Acad. Sci. U. S. A. 116, 5643–5652. doi: 10.1073/pnas.1820997116
Yao, C., and Finlayson, S. A. (2015). Abscisic acid is a general negative regulator of Arabidopsis axillary bud growth. Plant Physiol. 169, 611–626. doi: 10.1104/pp.15.00682
Yoneyama, K., and Brewer, P. (2021). Strigolactones, how are they synthesized to regulate plant growth and development? Curr. Opin. Plant Biol. 63:102072. doi: 10.1016/j.pbi.2021.102072
Yoneyama, K., Xie, X. N., Kusumoto, D., Sekimoto, H., Sugimoto, Y., and Takeuchi, Y. (2007a). Nitrogen deficiency as well as phosphorus deficiency in sorghum promotes the production and exudation of 5-deoxystrigol, the host recognition signal for arbuscular mycorrhizal fungi and root parasites. Planta 227, 125–132. doi: 10.1007/s00425-007-0600-5
Yoneyama, K., Yoneyama, K., Takeuchi, Y., and Sekimoto, H. (2007b). Phosphorus deficiency in red clover promotes exudation of orobanchol, the signal for mycorrhizal symbionts and germination stimulant for root parasites. Planta 225, 1031–1038. doi: 10.1007/s00425-006-0410-1
Young, N. F., Ferguson, B. J., Antoniadi, I., Bennett, M. H., Beveridge, C. A., and Turnbull, C. G. N. (2014). Conditional auxin response and differential cytokinin profiles in shoot branching mutants. Plant Physiol. 165, 1723–1736. doi: 10.1104/pp.114.239996
Zhang, J., Mazur, E., Balla, J., Gallei, M., Kalousek, P., Medvedova, Z., et al. (2020). Strigolactones inhibit auxin feedback on PIN-dependent auxin transport canalization. Nat. Commun. 11:3508. doi: 10.1038/s41467-020-17252-y
Zheng, C. L., Acheampong, A. K., Shi, Z. W., Halaly, T., Kamiya, Y., Ophir, R., et al. (2018). Distinct gibberellin functions during and after grapevine bud dormancy release. J. Exp. Bot. 69, 1635–1648. doi: 10.1093/jxb/ery022
Keywords: strigolactone, auxin, cytokinin, abscisic acid, branching, apical dominance, dormancy, BRANCHED1
Citation: Khuvung K, Silva Gutierrez FAO and Reinhardt D (2022) How Strigolactone Shapes Shoot Architecture. Front. Plant Sci. 13:889045. doi: 10.3389/fpls.2022.889045
Edited by:
Catherine Rameau, INRA UMR1318 Institut Jean Pierre Bourgin, FranceReviewed by:
Francois Fabien Barbier, The University of Queensland, AustraliaCatalina Iulia Pislariu, Texas Woman’s University, United States
Philip B. Brewer, University of Adelaide, Australia
Copyright © 2022 Khuvung, Silva Gutierrez and Reinhardt. This is an open-access article distributed under the terms of the Creative Commons Attribution License (CC BY). The use, distribution or reproduction in other forums is permitted, provided the original author(s) and the copyright owner(s) are credited and that the original publication in this journal is cited, in accordance with accepted academic practice. No use, distribution or reproduction is permitted which does not comply with these terms.
*Correspondence: Didier Reinhardt, didier.reinhardt@unifr.ch